- Laboratory Molecular Physiology and Adaptation, CNRS UMR 7221, Muséum National d’Histoire Naturelle, Department Adaptations of Life, Paris, France
Neurodegenerative diseases are characterized by chronic neuronal and/or glial cell loss, while traumatic injury is often accompanied by the acute loss of both. Multipotent neural stem cells (NSCs) in the adult mammalian brain spontaneously proliferate, forming neuronal and glial progenitors that migrate toward lesion sites upon injury. However, they fail to replace neurons and glial cells due to molecular inhibition and the lack of pro-regenerative cues. A major challenge in regenerative biology therefore is to unveil signaling pathways that could override molecular brakes and boost endogenous repair. In physiological conditions, thyroid hormone (TH) acts on NSC commitment in the subventricular zone, and the subgranular zone, the two largest NSC niches in mammals, including humans. Here, we discuss whether TH could have beneficial actions in various pathological contexts too, by evaluating recent data obtained in mammalian models of multiple sclerosis (MS; loss of oligodendroglial cells), Alzheimer’s disease (loss of neuronal cells), stroke and spinal cord injury (neuroglial cell loss). So far, TH has shown promising effects as a stimulator of remyelination in MS models, while its role in NSC-mediated repair in other diseases remains elusive. Disentangling the spatiotemporal aspects of the injury-driven repair response as well as the molecular and cellular mechanisms by which TH acts, could unveil new ways to further exploit its pro-regenerative potential, while TH (ant)agonists with cell type-specific action could provide safer and more target-directed approaches that translate easier to clinical settings.
Introduction
The human brain and spinal cord contain approximately 86 billion neurons and a similar number of non-neuronal cells (Azevedo et al., 2009). They form the central nervous system (CNS), a giant network controlling most of our body and mind. Injury to the CNS causes a loss of neuronal and/or glial cells, compromising neural network integrity. CNS insults include for example chronic autoimmune diseases that elicit neurodegenerative processes, or acute traumatic events such as stroke or a stabbing injury. Damage to the human CNS is usually irreversible due to the extremely limited capacity for self-repair, causing life-long intellectual or motor disability. The increasing incidence of neurodegenerative diseases in the population is currently the most important cause of morbidity and mortality, with millions of people affected (Heemels, 2016). This is particularly true for the elderly population with worldwide more than 10% of the plus 80 years old suffering from dementia in 2016, and accounting for 8.6% of the deaths of individuals aged 70 or more (Feigin et al., 2019).
The therapeutic goal for all types of CNS injury is to preserve or restore neural network integrity, and maintain or re-establish cognitive and motor functions, aiming at the highest possible life quality. One possibility is to harness better the endogenous capacity of the adult brain’s neural stem cells (NSCs) to generate new neurons and glial cells that could facilitate repair. Adult NSCs are multipotent, radial glia-derived cells that were set aside during development and have an unlimited self-renewal capacity in vivo. In the telencephalon of adult mammals, the majority of NSCs reside in two restricted niches, the subventricular zone (SVZ) lining the lateral ventricles and the subgranular zone (SGZ) of the hippocampus.
The SVZ contains the largest NSC population in adult mammals. In rodents, quiescent NSCs, or type B cells, juxtapose ependymal cells and have cerebrospinal fluid (CSF)-sensing cilia as well as processes enveloping blood vessels (Mirzadeh et al., 2008). They co-exist with activated, proliferating NSCs that successively give rise to transient amplifying proliferators (TAPs, or type C cells) and neuroblasts (type A cells), or less commonly, to oligodendrocyte precursor cells (OPCs) (Doetsch et al., 1999; Menn et al., 2006). In normal conditions, neuroblasts migrate via the rostro-caudal stream toward the olfactory bulbs where they differentiate into GABAergic and dopaminergic interneurons involved in olfaction (Lim and Alvarez-Buylla, 2016). OPCs migrate to adjacent brain regions such as the corpus callosum and can differentiate into myelinating oligodendrocytes (Menn et al., 2006) to facilitate myelin remodeling (Young et al., 2013; McKenzie et al., 2014). During aging, the NSC and TAP pools decrease, NSCs become quiescent and the neuro/glia ratio decreases sharply (Ahlenius et al., 2009; Capilla-Gonzalez et al., 2013; Daynac et al., 2016). In primates, including humans, the adult SVZ differs in some ways from that of rodents. A cell-devoid space separates ependymal cells and NSCs, and the fraction of quiescent NSCs is larger (Sanai et al., 2004; Quiñones-Hinojosa et al., 2006; Gil-Perotin et al., 2009). SVZ-neurogenesis in primates including humans drops sharply during the first months of life, only to continue at a very low rate thereafter, producing neuroblasts that predominantly migrate to the striatum with a so far unknown role (Sanai et al., 2004; Ernst et al., 2014). In contrast, a fairly large population of SVZ-OPCs exists in humans that can rapidly respond to injury (Nait-Oumesmar et al., 2007). While the SVZ neurogenic capacity declines even further during aging, the rate of SVZ-oligodendrogenesis seems to be constant throughout life (Weissleder et al., 2016).
In the hippocampus, radial glial-like NSCs or type 1 cells reside in the SGZ of the dentate gyrus. They can generate type 2a and 2b TAPs that, with the exception of a few astrocyte progenitors, exclusively form type 3 neuroblasts (Winocur et al., 2006). Post-mitotic neuroblasts differentiate into calretinin immature neurons and then into calbindin granule neurons during tangential and radial migration pathways in the dentate gyrus (Kempermann et al., 2015). They rewire hippocampal circuits involved in learning and memory throughout life, although hippocampal neurogenesis decreases with aging across species (Kozareva et al., 2019). The human hippocampus also contains NSCs that generate granule neurons and function in learning-dependent plasticity (Eriksson et al., 1998; Kozareva et al., 2019). In a recent study, Sorrells et al. (2018) did not detect de novo DCX-positive neuroblasts in the hippocampus of aged individuals. However, brain samples were fixed relatively late after death, which could have compromised tissue integrity and impeded antigen detection by immunohistochemistry (IHC). Furthermore, a substantial amount of brain samples was collected from patients with chronic epilepsy in whom neurogenesis might differ from basal levels (Lima and Gomes-Leal, 2019; Steiner et al., 2019). Others have used brain samples from deceased individuals without any record of neurological conditions, and fixed them more rapidly after death had occurred. Several independent studies detected thousands of DCX-positive neuroblasts being generated, demonstrating hippocampal neurogenesis throughout life, although the decreasing daily output during aging indicates a certain degree of plasticity loss (Boldrini et al., 2018; Moreno-Jiménez et al., 2019; Tobin et al., 2019).
Several types of CNS injury elicit SVZ- and SGZ-NSCs to actively proliferate and generate post-mitotic cells that can differentiate into mature neurons or glial cells, but this response never leads to functional restoration in humans (Picard-Riera et al., 2004; Faiz et al., 2015). Similarly, physical exercise and omega 3-enriched diet can amplify hippocampal neurogenesis and diminish cognitive decline in Alzheimer’s and Parkinson’s disease (Tincer et al., 2016; Morris et al., 2017), but never halt disease progression. Animal studies show that molecular inhibition and the lack of pro-regenerative cues constrains CNS repair in adults (Silver et al., 2015). While the competence to regenerate is limited to development in amniotes (Weil et al., 2008), phylogenetically primitive vertebrates such as fish and urodeles maintain spectacular regenerative capacities throughout their entire life, replacing entire body extremities and rebuilding lost brain connections from scratch (Genovese et al., 2013; Slack, 2017; Zambusi and Ninkovic, 2020). Extensive damage to the adult zebrafish telencephalon elicited an NSC-mediated response that fully repaired the injury after only a few weeks (Kishimoto et al., 2012). However, a highly similar transcriptome of adult zebrafish and mammalian NSCs suggests the latter also have a hidden or blocked regenerative potential (Lange et al., 2020). The challenge is to relieve the brakes on molecular inhibition and modulate pathways that promote regeneration, eliciting the repair capacity that is found in many non-mammalian vertebrates. Identifying such intrinsic and extrinsic signals that are capable of doing so, can open new avenues for enhancing endogenous CNS repair. Many factors have been identified over the years, including Notch and Wnt pathways (Lie et al., 2005; Aguirre et al., 2010), as well as choroid plexus-derived factors (Silva-Vargas et al., 2016), and hormones (Ponti et al., 2018). Here, we discuss thyroid hormone (TH) as a key signal in NSC commitment in the mammalian stem cell niches.
The Potential of Thyroid Hormone as a Pro-Repair Cue
Thyroid hormone is a key endocrine signal conserved in all vertebrates, including humans, regulating many homeostatic processes such as growth, reproduction and energy metabolism. TH also regulates CNS development (Gothié et al., 2017) by influencing all neurodevelopmental processes, including cell cycle progression, fate choice, migration, differentiation, axo- and synaptogenesis, and myelination (Zoeller and Rovet, 2004; Moog et al., 2017; Krieger et al., 2019; Vancamp et al., 2020). Under pathophysiological conditions, TH acts on each of these processes, promoting regeneration in the adult fish brain that retained large numbers of NSCs (Grandel et al., 2006; Bhumika and Darras, 2014). On the contrary, in mammals, the regenerative potential is lost after a postnatal peak in THs, but THs continue to fine-tune exactly the same processes in the adult NSC niches as those occurring during neurodevelopment (Remaud et al., 2014; Kapoor et al., 2015; Gothié et al., 2020). Furthermore, our understanding of regulation of local TH action in NSCs has increased immensely over the past few years with TH, as well as novel TH analogs, emerging as interesting candidates for stem cell-based regenerative medicine.
Thyroid Hormone Regulation in Adult Stem Cell Zones of the Healthy Brain
Regulation of Thyroid Hormone Metabolism
The hypothalamus-pituitary-thyroid (HPT) axis maintains systemic TH homeostasis by controlling the synthesis and secretion of the largely inactive prohormone thyroxine (T4), and to a lesser extent the biologically active T3, by the thyroid gland. The hypothalamic thyrotropin-releasing hormone (TRH) releases thyroid-stimulating hormone (TSH, or thyrotropin) from the pituitary to induce TH secretion into the bloodstream, the latter providing negative feedback on the HPT axis (Fekete and Lechan, 2014). Around 50% of the circulating T4 in humans is converted into T3 by the enzyme deiodinase type 1 (DIO1) in the liver (Bianco et al., 2002), after which both THs reach target tissues.
At the brain level, transmembrane transporters take up the lipophilic hormones T4 and T3. These comprise the monocarboxylate transporter 8 (MCT8) with a high affinity for T4 and T3, and the T3-transporter MCT10. Other secondary TH transporters such as the T4-selective organic anion transporting polypeptide 1C1 (OATP1C1) and the large amino acid transporters (LATs) also contribute to TH influx and efflux (Kinne et al., 2011; Groeneweg et al., 2020). THs first cross the endothelial cells of the blood-brain barrier (BBB), or the epithelial cells of the blood-cerebrospinal fluid barrier (BCSFB). In contrast to the rodent BBB, that expresses both MCT8 and OATP1C1 (Roberts et al., 2008; Wilpert et al., 2020), the human BBB almost completely lacks OATP1C1 (Roberts et al., 2008; Chan et al., 2014). At the BCSFB, T4 binds the TH-distributor protein transthyretin (TTR) releasing T4 into the CSF. Then, T4 circulates in the brain ventricles bordering regions such as the SVZ and spinal cord (Richardson et al., 2015). Once taken up by a neural cell, the enzyme DIO2 converts T4 into T3, whereas DIO3 inactivates T4 to reverse T3 (rT3) and T3 to T2, tightly modulating T3 availability (Luongo et al., 2019). DIO2 is particularly enriched in astrocytes that convert a considerable amount of T4 to T3 to supply to neurons and oligodendrocytes. The latter two express DIO3 to control intracellular T3 levels (Morte and Bernal, 2014).
T3 is considered as the more biologically active form of TH, as it can act on gene transcriptional activity in TH-targeted cell types by binding to nuclear TH receptors (TRs). Its availability and action in each cell or cell-type ultimately depends on specific expression patterns of the TH transporters, DIOs and receptors, and as such determines gene activity and biological processes. THRA and THRB genes encode the TR isoforms TRα1, TRα2, TRβ1 and TRβ2 in mammals (Bernal, 2007). Co-localization with IHC markers in TRα1-GFP mice showed that the majority of neurons and oligodendroglial cells express TRα1 (Wallis et al., 2010), whereas TRβ1 is enriched in astrocytes and some neuronal subpopulations. TRβ2 is more confined to cells in the hypothalamus and pituitary (Bernal, 2007). TRα2 is strongly and widely expressed in the brain, but its role as a dominant-negative receptor lacking affinity for T3 is still unclear (Guissouma et al., 2014). Genome-wide analyses identified hundreds of directly and indirectly T3-regulated genes in the mammalian brain (Chatonnet et al., 2015; Gil-Ibañez et al., 2017).
Of note, some non-canonical effects on neural cells have been described for other TH forms as well, but are restricted in their actions. For instance, T4 binding to the membrane ανβ3 integrin receptor promotes embryonic neural progenitor proliferation (Stenzel et al., 2014), and both T4 and rT3 can stimulate cerebellar granule cell migration and actin polymerization (Farwell et al., 2006). T2 has the potency to act on TRs (Köhrle, 2019), but a role in the CNS remains to be shown.
Thyroid Hormone Action in the Healthy SVZ
Data on the regulation of TH metabolism in NSCs almost exclusively originates from rodent studies. MCT8 and OATP1C1 mediate TH uptake at the endothelial cells of the rodent BBB as they do in other brain regions (Mayerl et al., 2014; Vatine et al., 2017), while TTR provides at least a part of T4 to SVZ-NSCs via the CSF (Vancamp et al., 2019; Alshehri et al., 2020). It is currently unknown which transporters facilitate TH uptake into SVZ-NSCs themselves. T3 is a master regulator of the NSC cycle and fate choice in the murine SVZ. Liganded TRα1 represses a gate-keeper of NSC identity, Sox2, and downregulates the cell cycle genes c-Myc and Ccnd1, facilitating TAP exit from the cell cycle and neuroblast commitment (López-Juárez et al., 2012; Remaud et al., 2017; Figure 1). In contrast, reduced T3 action in TAPs due to expression of the T3-inactivating DIO3, as well as TRα1 absence, allows Egfr expression. EGFR signaling promotes the generation of proliferating OPCs within the adult SVZ (Aguirre et al., 2007; Gonzalez-Perez and Alvarez-Buylla, 2011; López-Juárez et al., 2012; Remaud et al., 2017). If a T3-free window is required for OL progenitor specification, T3 also accelerates OPC cycle exit and differentiation through TRα1 (Durand and Raff, 2000; Billon, 2002), a process that also requires the nuclear receptor RXRγ (Baldassarro et al., 2019). Cell culture experiments indicate that liganded TRβ-induced expression of oligodendrogenesis-promoting genes such as Klf9, Mbp, and Plp allows for stepwise differentiation into mature and ultimately myelinating oligodendrocytes (Barres et al., 1994; Baas et al., 1997; Billon et al., 2001; Dugas et al., 2012).
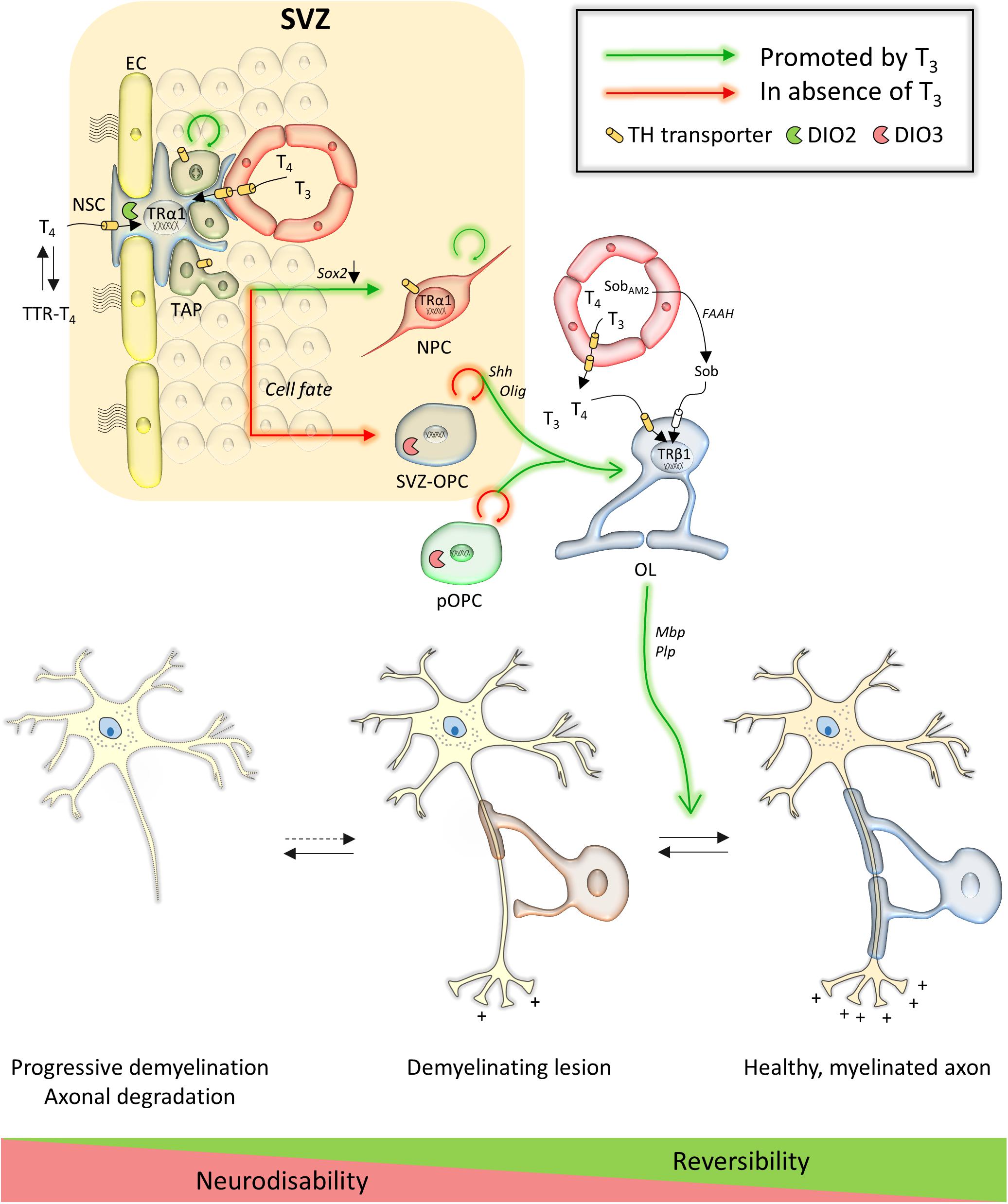
Figure 1. Overview of TH action in the subventricular zone and on oligodendroglial lineage cells and their contribution to repair in multiple sclerosis. Key genes are in italic next to arrows. Abbreviations: EC, endothelial cell; FAAH, fatty acid amide hydrolase; NPC, neural precursor cell; NSC, neural stem cell; OL, oligodendrocyte; Sob, sobetirome; SobAM2, methyl amide-derivative of sobetirome; SVZ, subventricular zone; SVZ-OPC, SVZ-derived oligodendrocyte precursor cell; pOPC, parenchymal oligodendrocyte precursor cell; TAP, transient amplifying progenitor; TTR, transthyretin.
Thyroid Hormone Action in the Healthy SGZ
Adult-onset hypothyroidism in rodents does not change the proliferative activity of type 1 SGZ-NSCs (Desouza et al., 2005; Kapoor et al., 2012; Sánchez-Huerta et al., 2016), nor does it change in TRα1–/– and TRα2–/– mice lacking and overexpressing TRα1, respectively (Kapoor et al., 2010), suggesting they are not responsive to TH. In contrast, thyroidectomized rats had less BrdU-positive and Ki67-positive hippocampal progenitor cells, an effect rescued by supplying T4 and T3 to the drinking water (Montero-Pedrazuela et al., 2006). Further studies have shown that SGZ-NSC self-renewal increases in TRβ KO mice (Kapoor et al., 2011). Hence, either unliganded TRβ isoforms, or T3 acting through TRβ, repress SGZ-NSC turnover (Kapoor et al., 2015). The former hypothesis seems more likely as type 1 SGZ-NSCs and type 2 TAPs only express the low affinity TH transporters Lat1 and Lat2 (Mayerl et al., 2020), although definite proof is missing.
T3-TRα1 action occurs from the post-mitotic stage, initiating type 2b TAPs to proceed to the differentiation phase and generate DCX-positive neuroblasts (Kapoor et al., 2010; Figure 2). The latter are in close proximity to the hippocampal blood vessel network, enabling BBB-mediated T3 uptake. qPCR on sorted SGZ cells and IHC showed that MCT8 is the only expressed TH transporter in type 3 neuroblasts. Deletion of Mct8 either globally or in the hippocampal neurogenic lineage alone decreased expression of the cell cycle regulator P27KIP1, impairing neuroblast differentiation and granule cell genesis. The results indicate that MCT8 is the primary TH uptake route for neuroblasts during hippocampal neurogenesis (Mayerl et al., 2020). Additionally, TH determines granule cell survival by altering the expression of pro- and anti-apoptotic factors under the influence of TRs, with a higher expression of TRβ compared to TRα1 and TRα2 (Guadaño-Ferraz et al., 2003; Desouza et al., 2005; Kapoor et al., 2010, 2011; Mayerl et al., 2020). Dio2 KO mice have a decreased hippocampal T3 content and display emotional alterations (Bárez-López and Guadaño-Ferraz, 2017), suggesting local DIO2 is another key component for generating sufficient T3 and stimulating cell differentiation and maturation, though its cellular expression pattern remains to be unraveled. Immature and mature granule cells also contained detectable levels of DIO3, LAT2 and MCT10 (Mayerl et al., 2020), but their functional roles remain unknown.
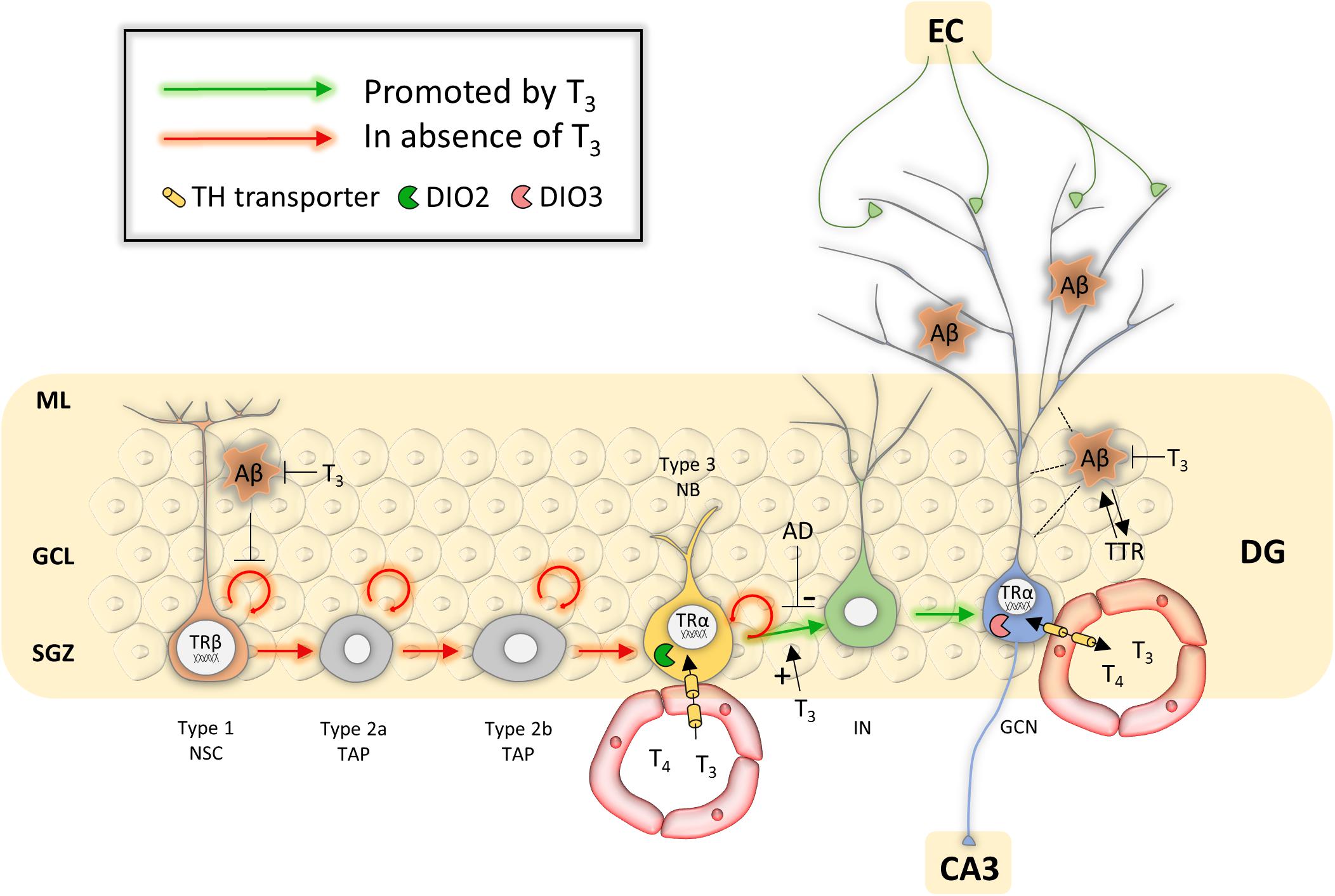
Figure 2. Overview of potential mechanisms of TH action during hippocampal neurogenesis in Alzheimer’s disease. Abbreviations: Aβ, amyloid β; AD, Alzheimer’s disease; DG, dentate gyrus; EC, entorhinal cortex; GCL, granule cell layer; GCN, granular cell neuron; IN, immature neuron; ML, molecular layer; NB, neuroblast; NSC, neural stem cell; SGZ, subgranular zone; TAP, transient amplifying progenitor; TTR, transthyretin.
Patient Studies Linking Thyroid Hormone Levels With CNS Injury Outcome
A last line of evidence showing that TH could aid in CNS repair comes from epidemiological studies. Such studies indicate that low TH levels can predispose to neurodegenerative diseases, and can also have unfavorable outcomes in terms of morbidity and mortality following CNS injury. Mild thyroid dysfunction, a common condition in the elderly (Biondi et al., 2019), or abnormally low TH levels, both increase the risk for developing neurodegenerative diseases, or correlate with worsened outcomes. For example, an inverse relation between free T3 levels and the risk for developing Alzheimer’s disease (AD) was demonstrated in a 302 patient cohort with mild cognitive impairment at the beginning of the study (Quinlan et al., 2019). Similarly, lower free T3 levels in pre-stroke individuals correlated with increased stroke severity, disability and mortality rates (Jiang et al., 2017; Lamba et al., 2018).
Unrelated to the patient’s endocrinological history, CNS disease or injury is often also followed by a physiological drop in T3 levels. Depressed serum T3 levels have been observed years ago in many patients with chronic spinal cord injury (SCI) (e.g., Chen et al., 2012), and between 32–62% of patients that suffered from a stroke had lower than normal T3 levels. Low circulating T3 levels were also found in multiple sclerosis (MS) patients (Zych-Twardowska and Wajgt, 2001). This is known as low T3 syndrome or euthyroid sick syndrome (ESS or Non-thyroidal Illness Syndrome), an adaptive body response that reduces metabolic and energetic needs during recovery (Mourouzis et al., 2013; Bunevicius et al., 2015). However, while being beneficial at first, sustained low T3 levels in the secondary phase of the disease provoke unwanted complications and poorer rehabilitation (Bunevicius et al., 2015; Fliers et al., 2015). Anticipating ESS by administering THs prior to major surgery has led to better recovery (Liu et al., 2014; Zhang et al., 2018). The correlation between TH status and outcome in unrelated CNS diseases implies that TH impacts repair processes in all of them, though these studies do not reveal the mechanisms implicated.
Altogether, TH seems a promising candidate for regenerative medicine because it (i) profoundly impacts neurodevelopmental plasticity, (ii) contributes to neural repair in primitive vertebrate species throughout life, (iii) controls NSC behavior in the healthy adult rodent SVZ and SGZ, (iv) finally, TH status plays a key role in CNS disease and injury outcome. Different research groups have therefore investigated how THs could promote endogenous repair in CNS injury or disease by acting on NSCs. We elaborate on results obtained in canonical CNS disorders that, despite different etiologies, could all benefit from cell replacement and neural network repair. These include the chronic neurodegenerative diseases MS (loss of oligodendrocytes) and AD (loss of neuronal cells), as well as acute traumas such as stroke and SCI, in which entire neural networks vanish in a matter of minutes. Each of them has unique features with regard to cell type loss, affected tissue/structure, location, and temporal disease patterns, posing different problems for stem cell-based approaches. Directing cell fate choice to generate the whole panel of cell types in correct ratios is paramount but injury-dependent. Newly generated precursor cells will also have to migrate enormous distances to the site of injury and incorporate existing but damaged neural networks, or re-join previously connected networks. An additional challenge is that many CNS insults predominantly affect the elderly in which TH homeostasis is altered (Bowers et al., 2013) and the endogenous repair potential in the stem cell zones is probably less effective in producing the various cell types as compared to young individuals (Capilla-Gonzalez et al., 2015). We discuss the scientific evidence that so far supports a role for TH in NSC-mediated repair in the above-mentioned CNS insults, and provide future perspectives on how to tackle current bottlenecks.
The Repair Potential of Thyroid Hormone in Multiple Sclerosis
Multiple sclerosis is a chronic demyelinating CNS disease with around 2.2 million cases worldwide in 2016, a 10% increase compared to 1990. Prevalence is highest in 55–64 year-olds, but the disease is often diagnosed in young adults around the age of 30. Recurring episodes of autoimmune-mediated oligodendrocyte apoptosis cause progressive, heterogeneous myelin degradation (Haider et al., 2016) and neurological disability, affecting life quality and decreasing average life expectancy by several years. While current treatments primarily focus on attenuating the relapsing immune reactions, reversing demyelination and restoring neurological functions also hold promise as definitive remedies. Repair comprises regenerating lost oligodendrocytes to remyelinate naked axons and protect them from a hostile microenvironment (Calzà et al., 2010). However, while a fairly large population of parenchymal OPCs (pOPCs), generated during development, persists in the adult brain, most of them fail to differentiate into new myelinating oligodendrocytes (Wolswijk, 1998; Chang et al., 2000). The few pOPCs that do, wrap axons in thinner than normal myelin sheaths, a hallmark visible as shadow plaques on brain magnetic resonance imaging (MRI) (Patrikios et al., 2006). Exhaustion of the resident pOPC pool and failed migration to distant sites of injury further contribute to progressive neurological deterioration in the majority of MS patients (Hartley et al., 2014; Dulamea, 2017).
The adult SVZ could reinforce endogenous repair in MS, providing a source of newly generated OPCs. Furthermore, and in contrast to pOPCs, SVZ-derived OPCs generate myelin sheaths with a normal thickness (Xing et al., 2014; Brousse et al., 2015; Remaud et al., 2017). Brain examinations of deceased patients showed activated generation of SVZ-OPCs that successfully migrated to lesion sites within the corpus callosum (Nait-Oumesmar et al., 2007). Boosting this inherent repair system could thus improve functional repair by (i) amplifying the generation of OPCs from cycling SVZ-NSCs and promoting glial cell fate, (ii) stimulating OPC migration to lesion sites and, (iii) promoting their differentiation into myelinating oligodendrocytes.
Thyroid Hormone Promotes Remyelination in Animal Models of Multiple Sclerosis
As a key signal that controls SVZ-NSC proliferation (Lemkine et al., 2005) and triggers OPC differentiation in the healthy adult brain (Dugas et al., 2012), it has been tested extensively if TH could improve remyelination in mammalian models of cuprizone-induced demyelination (Table 1). Cuprizone is a widely used gliotoxin inducing oligodendrocyte death and demyelination in brain regions, notably in the corpus callosum, provoking neurobehavioral deficits such as gait problems and sensorimotor impairments that mimic several symptoms observed in MS patients (Ludwin, 1978; Franco-Pons et al., 2007; Praet et al., 2014). Adult rats were fed with 0.6% cuprizone for 2 weeks to induce demyelination and received three subcutaneous T3 injections every 2 days during the first week of remyelination. Examination directly after the treatment showed a smaller pool of Nestin-positive progenitors and more OLIG-positive OPCs in the SVZ, suggesting that systemic T3 treatment stimulated SVZ-OPC cycle exit. The authors observed an upregulation of TRα by IHC in the treated and untreated cuprizone groups, although the TR was surprisingly absent in controls (Franco et al., 2008), conflicting with previous data (Lemkine et al., 2005). TRβ expression in the SVZ also increased following demyelination and T3 injection, whereas it was undetectable in healthy conditions or after cuprizone treatment alone (Lemkine et al., 2005; Franco et al., 2008). T3 is a potent inducer of THRB gene transcription (Baas et al., 1998), and increased TRβ1 protein levels in cultured rat OPCs as well as in vivo during postnatal development (Baxi et al., 2014). This suggests that T3-dependent upregulation of this TR isoform could precede OPC migration. Increased numbers of mature myelinating oligodendrocytes in the corpus callosum positive for O4, MBP, PLP and CC1 further indicated that T3 treatment applied after a cuprizone diet, improves remyelination (Franco et al., 2008). However, the 1-week treatment might not have been sufficient to reach control levels. While the origin of the de novo oligodendrocytes, derived from either SVZ-OPCs or pOPCs, was not determined, tracing experiments demonstrated that SVZ-OPCs successfully migrate toward the demyelinated corpus callosum adjacent to the SVZ (Xing et al., 2014; Brousse et al., 2015; Remaud et al., 2017) where they subsequently differentiate into immature and mature, myelin sheath-forming oligodendrocytes (Fernandez et al., 2004; Franco et al., 2008; Zhang et al., 2015; El-Tahry et al., 2016) (Figure 1).
In another study, mice were fed a 0.2% cuprizone diet for 12 weeks, reaching a profound demyelination (Harsan et al., 2008). Intraperitoneal T3 injections were given daily during the 3-week recovery period after withdrawal of the cuprizone diet, and mice were evaluated up until 9 weeks thereafter. At that time, remyelination in the corpus callosum and cerebellum had improved as seen on MRI and IHC for MBP, a key TR-target gene (Jeannin et al., 1998), and numbers of myelinated axons almost reached control levels (Harsan et al., 2008). Thus, a brief TH treatment after demyelination can have long-lasting beneficial effects on remyelination. Furthermore, T3 increased numbers of migrating PSA-NCAM-positive neuroblasts in the SVZ, suggesting a neurogenic response as well. Motor performance on rotarod tests gradually improved as compared to mice that did not receive exogenous T3 (Harsan et al., 2008). Similar behavioral improvements were observed in mice that received daily intraperitoneal T3 injections for 2 weeks after a 0.2% cuprizone diet for 6 weeks (Zhang et al., 2015). Numbers of CC1-positive mature oligodendrocytes, MBP immunoreactivity and the proportion of myelinated axons increased in the corpus callosum as shown by western blotting, IHC and electron microscopy. As expected, all these effects were abolished when T3 was replaced by the thyroid-blocking agent propylthiouracil during the remission period (Zhang et al., 2015).
The potential of TH to promote OPC differentiation and maturation was also investigated in rats and marmosets in which experimental autoimmune encephalomyelitis (EAE) elicited spontaneous neuroglial apoptosis and axonal degeneration in the CNS, causing long-term sensorimotor disability (Constantinescu et al., 2011) (Table 1). A pulse of T3 every other day increased proliferation in NSC cultures prepared from the SVZ of EAE Lewis rats, and stimulated OPC differentiation and maturation into myelinating oligodendrocytes. T3 had similar pro-oligodendrogenic effects in OPC cultures grown from healthy SVZ-NSCs that during in vitro differentiation were simultaneously exposed to a mix of cytokines that mimic inflammatory conditions (Fernández et al., 2009, 2016). These properties were also tested in vivo. Adult rats received three subcutaneous T4 injections every other day during the acute EAE phase, when animals suffer from a severe force deficit (Calza et al., 2002). The strongly increased proliferation of SVZ-NSCs and uncommitted progenitors (Calzà et al., 1998) returned to basal levels in EAE animals treated with T4. This contrasts with the increased proliferative activity following T3 treatment in vitro (Fernández et al., 2009), and could be a consequence of different treatment protocols as well as the influence of factors in the complex in vivo environment. IHC analysis in the spinal cord showed less BrdU-positive progenitors and more A2B5-, O4- and MBP-positive committed OPCs, pre-oligodendrocytes and differentiated oligodendrocytes, respectively (Calza et al., 2002). Using the same protocol but now on Dark Agouti rats that have a more pronounced demyelination phenotype than Lewis rats, pulsed T4 administration at 2 and 3 weeks after immunization also promoted spinal cord remyelination. PDGFRα and MBP protein levels increased, and myelin sheaths thickened in the chronic recovery phase (Fernandez et al., 2004). Similarly, a 3-week pulsed T3 treatment from 10 days post-immunization increased the fluoromyelin-positive area in the spinal cord of EAE rats and improved neurobehavioral functions. Twenty-four hours after the first T3 pulse, the increase in TRα1 and TRβ expression following EAE was prevented, suggesting rapid effects on gene transcription (Dell’Acqua et al., 2012). Similarly, a short 3-day treatment with T3, 2 weeks prior to and a second time 4 weeks after inducing EAE in marmosets (Callithrix Jacchus), ameliorated the clinical disability score and increased the expression of oligodendrocyte markers in the spinal cord (D’Intino et al., 2011).
Irrespective of the different treatments protocols and the fact that many observations were made in the spinal cord, these studies strongly suggest that TH treatment triggers NSC and progenitor cell-cycle exit and promotes OPC differentiation by stimulating several genes in post-mitotic OLs (Figure 1), and that in all animal models of demyelination (Table 1). The fact that T4 and T3 are both capable to mediate these effects on gene expression (Fernández et al., 2016) suggests an important role for DIO2-mediated conversion T4 to T3 either by OPCs, or by astrocytes that are known to provide T3 to neurons in other brain regions as well (Morte and Bernal, 2014). T4 and T3 additionally had anti-inflammatory and neuroprotective effects on axonal morphology by increasing Ngf expression (Calza et al., 2002; D’Intino et al., 2011; Castelo-Branco et al., 2014; Fernández et al., 2016).
A problem that would arise in clinical settings is that TH provokes adverse side effects on peripheral organs such as bone, muscle and heart. In order to circumvent that issue, Hartley and co-workers (Hartley et al., 2019) used the pro-drug Sob-AM2 that is converted into the TRβ agonist sobetirome by the CNS-specific enzyme fatty acid amide hydrolase (Meinig et al., 2019). Sobetirome stimulated differentiation of rodent and human cultured OPCs, and accelerated developmental myelination in mice (Baxi et al., 2014). Applying sobetirome to cultured rat OPCs demonstrated its capacity to induce expression of T3-responsive pro-oligodendrogenic genes (e.g., Mbp, Klf9, Hr). The potential of sobetirome and Sob-AM2 to promote remyelination was then tested in three different mouse models (Hartley et al., 2019): (i) mice that received lysolecithin injections to focally demyelinate the corpus callosum (Blakemore and Franklin, 2008) ± daily sobetirome injections for 5 days thereafter, (ii) mice fed a 12-week 0.2% cuprizone diet ± daily sobetirome injections for 3 weeks thereafter, and (iii) iCKO-Myrf mice in which mature oligodendrocytes death leads to gradual demyelination with preservation of the OPC pool (Koenning et al., 2012) ± daily sobetirome or Sob-AM2 chow for 22 weeks, starting 2 weeks after tamoxifen treatment was induced. All treatments were performed in parallel with T3. In all models, sobetirome increased the MBP signal, PDGFRα-positive mature oligodendrocytes, myelinated axons, myelin content in the corpus callosum, and rotarod motor performance (Table 1). Furthermore, Sob-AM2 successfully induced long-term remyelination in the iCKO-Myrf mouse model without any measurable side-effects (Hartley et al., 2019), highlighting the potential of a receptor isoform-specific TH analog as a powerful targeted approach (Figure 1).
Current Bottlenecks in Thyroid Hormone-Mediated Repair in Multiple Sclerosis
As discussed above, THs have beneficial and reproducible effects on stimulating spinal cord and SVZ-derived OPCs as well as pOPCs to differentiate and remyelinate in various models of MS. However, these exciting results also raised new questions that will need to be addressed in future studies. A first issue relates to the size of the SVZ-OPC pool. While SVZ-OPCs contributed strongly to remyelination in the mouse brain after a relatively short 5-week long cuprizone exposure, prolonged demyelination following a 12-week exposure, reflecting the human situation of MS more accurately, depleted the SVZ-OPC pool (Brousse et al., 2015). As TH stimulates OPC differentiation, protracted TH treatment may even accelerate exhaustion of the SVZ-OPC pool, stalling remyelination in the early, reversible phase of the disease. Prolonged T3 treatment in different mouse models of MS abrogated the increase in proliferating SVZ-NSCs and –OPCs that was seen in non-treated animals, and prematurely ceased OPC proliferation and oligodendrocyte formation (Franco et al., 2008; Hartley et al., 2019). Since glial lineage commitment occurs in the absence of TH signaling (Remaud et al., 2017), transient methimazole-induced hypothyroidism during the last 4 weeks of a 6-week cuprizone diet in adult mice, when new OPCs are proliferating to participate to myelin repair (Picard-Riera et al., 2002), enhanced SVZ-OPC generation. When followed by three T4 and T3 pulses after cuprizone withdrawal, remyelination in the corpus callosum accelerated compared to animals that were euthyroid during demyelination (Remaud et al., 2017). In addition, in normal conditions, downregulating Dio3 expression mainly in EGFR-positive and PDGFRα-positive OPCs by transfecting SVZ-cells with a short hairpin Dio3 construct, reduced global cell proliferation by 50% and OLIG2-positive cells by 30% (Remaud et al., 2017). This proves that reduced T3 signaling favors SVZ-OPC generation in physiological conditions, and also after a demyelinating lesion. It are these divergent results that relate to different treatments protocols (e.g., cuprizone diet, start of TH treatment, duration, and dose) in pre-clinical MS studies that will ultimately allow distilling an optimal treatment scenario. Ideally, TH treatment would be finely tuned to keep a steady balance between NSC-derived OPC generation, proliferation and OPC differentiation, promoting constant and long-term remyelination (Figure 1).
Another question is whether SVZ-OPCs can efficiently migrate to lesion sites. Demyelinating lesions are scattered throughout the gray and white matter of the brain, in regions distant from the SVZ, such as the cortex, cerebellum and brain stem (e.g., Kutzelnigg et al., 2005, 2007) as well as the spinal cord (Gilmore et al., 2006). Lesions in these CNS regions are most-likely responsible for motor disability in MS patients and hence are the prime targets for remyelination (Filippi et al., 2019). SVZ-OPCs migrate efficiently to the adjacent corpus callosum in demyelinating conditions in a matter of days (Xing et al., 2014; Brousse et al., 2015; Remaud et al., 2017). In contrast, colonization and remyelination by newly generated SVZ-OPCs after a 6-week cuprizone treatment in mice was slower and less complete in the cingulate cortex (± 60% vs. controls) and hippocampus (± 90% vs. controls) 6 weeks after demyelination, and mature oligodendrocyte numbers remained very low (Baxi et al., 2017). Accordingly, in MS patients, remyelination was successful in regions in close proximity to the SVZ, while shadow plaques remained in the cortex and spinal cord (Patrikios et al., 2006). Several reports showed that TH treatment improved white matter status in the murine cerebellum and cortex (Harsan et al., 2008; Silvestroff et al., 2012; Hartley et al., 2019). While the exact origin of the OPCs responsible for remyelination in these brain regions was not determined, selective Olig2 overexpression in SVZ-progenitors of postnatal mice showed that the OPC progeny was capable of migrating to the cortex (Maire et al., 2010).
It seems unlikely that SVZ-NSCs or –OPCs can abundantly migrate to the spinal cord. However, here, ependymal cells (ECs) surrounding the central canal in the spinal cord express the same NSC markers as those in the SVZ, such as Sox2, Sox9 and CD133. Multipotent ECs are largely quiescent, proliferate at low rates, and generate few astrocytes and oligodendrocytes in healthy conditions (Hachem et al., 2020), indicating that spinal cord tissue is by default pro-gliogenic. EAE activates ECs, that besides predominantly generating astrocytes, also form OPCs that subsequently differentiate into myelinating oligodendrocytes (Meletis et al., 2008). Systemic TH treatment counteracted a hypothyroid state in the spinal cord of EAE marmosets and facilitated remyelination (D’Intino et al., 2011), but whether or not that was by acting on ECs or by stimulating pOPCs to differentiate is unknown. Whether and how TH could act on ECs will be of interest for future research.
A final question in this context is that of the relative benefit of SVZ-OPCs over pOPCs. As already noted, SVZ-OPCs remyelinate axons with sheaths that have normal thickness (Xing et al., 2014; Brousse et al., 2015; Remaud et al., 2017), in contrast to otherwise thinner-than-normal sheaths produced by pOPCs. In addition, pOPCs isolated from adult rat brains had a reduced differentiation potential and remyelination capacity as compared to those of young brains (Neumann et al., 2019), making them a less attractive target in a disease that primarily affects older people. Remarkably, OPC generation in the SVZ and the rostral migratory stream remains stable throughout life, contrasting a sharp decrease in neurogenesis (Capilla-Gonzalez et al., 2013; Ernst et al., 2014; Weissleder et al., 2016). A mild hypofunction of the thyroid as observed in many elderly (Bowers et al., 2013; Biondi et al., 2019) might even favor SVZ-OPC genesis. The question remains though, if they are able to differentiate efficiently in aging conditions.
Further examination of TH-stimulated remyelination efficacy by SVZ- and EC-derived OPCs in MS models will be paramount to get an idea of the spatiotemporal white matter improvement, whether this process can be accelerated, and how long naked axons remain exposed to harming components of the extracellular matrix, which can potentially lead to irreversible neuronal and axonal degeneration (Franklin et al., 2012) as is the case in progressive MS (Michailidou et al., 2014). Intervening earlier might intensify recovery by endogenous repair mechanisms and increase chances of halting disease progression or even reverse damage. However, since plaque progression and demyelination occur heterogeneously, choosing an optimal therapeutic time window will be very complicated.
Translational Aspects of Thyroid Hormone-Induced Remyelination
Given that (i) TH has consistent effects on oligodendrocyte differentiation and remyelination in animal models of MS, (ii) SVZ-oligodendrogenesis is maintained throughout human life (Ernst et al., 2014; Weissleder et al., 2016) and (iii) SVZ-OPC generation increases upon MS-induced injury in humans (Nait-Oumesmar et al., 2007), we and others propose that there is strong potential for TH as a treatment in MS patients. A clinical phase 1 trial (NCT02760056) recently confirmed short-term safety and tolerance for low systemic doses of synthetic T3 in a cohort of 15 MS patients (Wooliscroft et al., 2020) paving the way for a phase 2 trial. Sobetirome is currently in a phase 1 trial in patients suffering from the demyelinating disease X-linked adrenoleukodystrophy (NCT01787578). Safety and efficacy of such compounds will require testing in combination with anti-inflammatory agents currently used to control relapsing immune reactions. Further exploring underlying signaling pathways and mapping interaction with other factors interacting with NSCs and progenitor commitment (Akkermann et al., 2017), should lead to better treatment scenarios, where definitive promotion of the endogenous repair potential by T3 or CNS-specific TH analogs could pave the way to a reparative cure in MS and more largely in demyelinating diseases.
The Repair Potential of Thyroid Hormone in Alzheimer’s Disease
Alzheimer’s disease is the most common cause of dementia, with a prevalence that tops of at 22.53% for individuals older than 85 years in Europe at 2016 (Niu et al., 2017). In AD, the accumulation of amyloid plaques and formation of neurofibrillary tangles trigger cerebral neuronal cell death and atrophy, causing progressive deterioration of cognitive functions and memory. An accelerated decrease in adult hippocampal neurogenesis (AHN) and hippocampal shrinkage are disease hallmarks in humans (Callen et al., 2001; Moreno-Jiménez et al., 2019) and compromise learning, memory and navigation (Tincer et al., 2016) as newly-generated granule cells normally rewire and strengthen connections in hippocampal circuits to promote learning- and memory-dependent plasticity. Most pharmacological approaches currently fail to reverse, halt or even slow down these symptoms. Counteracting both reduced AHN and neuronal cell loss could improve, or at least sustain memory processing (Tincer et al., 2016).
The Link Between Thyroid Hormone, Hippocampal Neurogenesis and Alzheimer’s Disease
For years now, there have been controversial links between systemic TH status and the pace of human hippocampal neurogenesis. Adult-onset hypothyroidism, the second-most common human endocrine disorder, associates with depression, and shares the hallmarks of reduced AHN and decreased hippocampal volume with AD, as well as memory impairments and emotional alterations (Cooke et al., 2014; Planchez et al., 2020). T4 treatment in (sub)clinical hypothyroid patients reversed underperformance on several cognitive tests specifically designed for assessing hippocampal function (Correia et al., 2009). Of the 159 patients with bipolar disorder, and of whom none responded to a wide range of antidepressants, 84% had improved cognitive symptoms after supplementing supraphysiological T3 doses (Kelly and Lieberman, 2009). However, in this and other small-scale clinical studies, T3 and also T4 were always given as an adjuvant therapy when antidepressants fail, leaving the effect of THs alone untested (Parmentier and Sienaert, 2018). Interestingly, low T3 levels as well as DIO2 polymorphisms predispose for developing AD (McAninch et al., 2018; Quinlan et al., 2019) and T3 levels were reduced in brains of deceased AD patients (Davis et al., 2008), suggesting that hypothyroid conditions may affect AHN similarly as in depression.
Animal studies showed that TH regulators limit TH action to specific stages of hippocampal neurogenesis that lead to the production of new granule neurons in normal conditions, cells that will eventually rewire dentate gyrus circuits to contribute to hippocampal neuroplasticity. Adult-onset hypothyroidism and KO of TRα1, Dio2 or Mct8 in rodents showed that NSC proliferation was not affected, but that hippocampal neuroblast generation, differentiation and survival of newly generated neurons were decreased (Desouza et al., 2005; Kapoor et al., 2012; Bárez-López et al., 2014; Sánchez-Huerta et al., 2016; Mayerl et al., 2020). Systemic T3 treatment effectively recovered these processes and rescued neurological functions (Desouza et al., 2005; Eitan et al., 2010; Kapoor et al., 2010). It is tempting to speculate that TH can thus stimulate AHN to counteract hippocampal neuronal loss in AD too (Figure 2), as long as it can be started soon enough. So far, only few studies have assessed this potential in appropriate AD models or patients.
Thyroid Hormone as a Repair Cue in Alzheimer’s Disease
The effect of TH treatment was tested in a mouse model whereby amyloid β, the main constituent of amyloid plaques in the AD brain, was injected in the hippocampus to mimic AD. Seven days later, a 4-day long daily intraperitoneal administration of low T4 doses rescued granule cell numbers, reduced hippocampal neuronal cell death, increased cholinergic function, and restored cognitive functions (Fu et al., 2010). Similarly, intraperitoneal administration of T4 to healthy 22–23-month-old rats stimulated Ngf and Nt-3 expression in the hippocampus, reflecting neuroprotective TH actions, even in these relatively aged rats (Giordano et al., 1992). These data coincide with 3-month-old thyroidectomized rats that displayed reduced AHN and performed less well in a forced-swimming test, a measure for depressive-like behavior. Both a single intraperitoneal injection of T4 + T3 and a daily supply via the drinking water rescued neurogenesis and restored behavioral performance, suggesting rapid effects of TH on AHN (Montero-Pedrazuela et al., 2006).
Apart from stimulating neurogenesis and providing neuroprotection, TH could act on NSCs via another, more indirect way. Amyloid β was shown to accumulate in SGZ-NSCs in mouse AD models, inhibiting proliferation and increasing apoptosis, thus blocking AHN (Haughey et al., 2002b). T3 negatively regulates the amyloid-β precursor protein (APP) gene (Belandia et al., 1998; Belakavadi et al., 2011), and rendering rats hyperthyroid by giving oral T3 for 5 days strongly suppressed overall brain APP expression (Belakavadi et al., 2011). However, the sole study that tested this trait in a rat model for AD by giving supraphysiological doses of T3 for 4 weeks showed no significant changes in brain amyloid β content by western blotting (Prieto-Almeida et al., 2018) (Figure 2). T3 did reduce Tau hyper-phosphorylation and glycogen synthase kinase 3 α/β (GSK-3β) expression (Prieto-Almeida et al., 2018). Hyperactivity of each of these factors underlies AD pathogenesis and associates with amyloid β deposition, plaque forming and memory impairment (Hooper et al., 2008).
Amyloid β also reduces SVZ-NSC renewal capacity (Haughey et al., 2002a), corresponding to decreased numbers of proliferating stem cells in the SVZ of AD patients (Ziabreva et al., 2006; Rodríguez et al., 2009). As AD patients often suffer from anosmia (McShane, 2001), this might suggest impaired SVZ-neurogenesis, although the role of SVZ-neuroblasts in human olfaction is still under debate (Lim and Alvarez-Buylla, 2016). T3-dependent restoration of SVZ-neurogenesis could be a potential source of neurons to counteract cerebral atrophy, as the SVZ is the largest NSC reservoir. In in vitro neurospheres generated from SVZs dissected from rats in which streptozotocin injection replicated AD hallmarks, T3 administration recovered NSC proliferation and Seladin-1 expression (Mokhtari et al., 2020), preventing caspase 3-mediated neuronal apoptosis (Peri and Serio, 2008).
TTR could represent another important factor in AD (Figure 2). TTR efficiently binds amyloid β in- and outside cells (Buxbaum et al., 2008; Li et al., 2011), preventing aggregation and toxicity in hippocampal neurons (Silva et al., 2017) and clearing amyloid β from the brain (Alemi et al., 2016). TTR levels in the brain and CSF have been reported to be decreased in several AD patients and could be involved in disease progression (Hansson et al., 2009). These reduced TTR levels, together with the possibility that TTR protein sequesters amyloid β, could reduce T4 availability (Li and Buxbaum, 2011) and hamper TH supply to SVZ-NSCs, affecting SVZ-neuro- and oligodendrogenesis. Ttr KO mice had decreased numbers of newly SVZ-generated neuroblasts and favored glial fate choice (Vancamp et al., 2019; Alshehri et al., 2020), reminiscent of the effects on SVZ-neurogliogenesis following hypothyroidism (López-Juárez et al., 2012; Remaud et al., 2017).
So far, only one study in human AD patients demonstrated that intravenous TRH injections improved memory and concentration (Mellow et al., 1989), probably through increased TH release by the thyroid gland, as patients experienced tachycardia, an indicator of hyperthyroidism. However, TRH could also have extra-hypothalamic effects, and directly regulate cellular processes associated with AHN. Reduced TRH levels were detected in the hippocampus of AD patients, and in vitro experiments with rat hippocampal neurons demonstrated that intracellular TRH depletion increased GSK-3β expression and Tau phosphorylation (Luo et al., 2002; Luo and Stopa, 2004). Hyperphosphorylated Tau can accumulate and have neurotoxic effects (Rajmohan and Reddy, 2017). In contrast, administrating TRH to neuronal cultures reduced GSK-3β expression and Tau phosphorylation, thereby increasing neuronal survival (Luo and Stopa, 2004). TRH can also directly modulate synaptic activity of hippocampal neurons (Zarif et al., 2016). Such non-classical functions indicate a cellular signaling role for TRH in neurodegenerative diseases (for a review, see Daimon et al., 2013), although the underlying mechanisms remain incompletely understood.
A potential pro-regenerative function of T3 or other TH signaling components in AD-accompanied (hippocampal) neurodegeneration still awaits more proof-of-concepts, but deserves further testing in appropriate models given their strong role in AHN in the brain, and the persistence of hippocampal neurogenesis in aged healthy and AD individuals (Moreno-Jiménez et al., 2019; Tobin et al., 2019). Ideally, the therapeutic potential is tested over long periods by administering TH in different phases of the disease to analyze long-term efficacy, reversibility of neurobehavioral hallmarks and potential side effects on other organs, determining the best treatment scenario and setting the base for clinical trials. Whether a TH treatment could ever replenish neurons in brain regions other than the hippocampus is less sure, notably given the strongly reduced neurogenesis elsewhere in the aged brain.
Thyroid Hormone as a Repair Cue in Acute Traumatic CNS Injury
Almost every chronic neurodegenerative disease is accompanied by unique symptoms that progress via well-documented, protracted stages. Identical disease-underlying mechanisms provide the possibility of developing a uniform treatment strategy that is applicable before symptoms worsen. Acute CNS trauma on the contrary, causes instantaneous neuroglial cell death eradicating entire neural networks, with either a fatal outcome, or a profound impact on a patient’s psychomotor well-being depending on injury size, location and duration. Repair is extremely challenging as neural networks need to be rebuilt from scratch, demanding a harmonized interplay between neuro- and oligodendrogenesis, axonal damage repair, regrowth and reconnection of preserved axons, and myelinogenesis to functionally restore and reconnect CNS regions (O’Shea et al., 2017). Furthermore, acute trauma elicits an inflammatory response that besides initial wound healing sets in place a repair-blocking environment, one that is hostile for remnants of damaged neural networks. Stroke and acute SCI are two traumatic CNS events that must face each of these challenges.
Thyroid Hormone Action on Neural Stem Cells in Stroke Injury
Stroke is a global leading cause of death and permanent disability, mostly affecting the elderly, and causing 5.5 million deaths 2016 alone (Gorelick, 2019). Stroke survivors often deal with permanent disabilities, including paralysis, sensory disturbances or impairments of higher cognitive functions such as language, functions that associate with regions in the cerebrum that are the most prone to stroke. Ischemic stroke occurs when a blood vessel in the brain is clogged, whereas a ruptured brain artery causes hemorrhagic stroke. A poor blood supply deprives brain tissue from oxygen and nutrients, and causes neuroglial apoptosis, forming an ischemic infarct region. NSCs that can potentially contribute to repair arise from regions next to the injury and from the stem cell zones, and rapidly respond to injury. Brain examinations of deceased stroke patients showed that capillary-associated pericytes convert into proliferative stem cells that express the NSC markers Sox2 and c-Myc, with neuron-generating properties in vitro (Tatebayashi et al., 2017). Numbers of proliferative and neuronal marker-expressing cells also increased in the human ipsilateral SVZ after stroke (Marti-Fabregas et al., 2010).
Transgenic ablation of SVZ-derived DCX-positive neuroblasts 14 days prior to stroke induction in adult mice increased infarct size and worsened neurobehavioral disabilities 24 h after stroke (Jin et al., 2010), suggesting that enhanced SVZ-neurogenesis is a first-line repair response. The gliogenic response is however predominant as the SVZ output 2 weeks after stroke comprised 59% GFAP-positive astrocytes, 15–20% OPCs and only 10% DCX-positive neuroblasts, a distribution that almost did not change in the following weeks (Li et al., 2010). Tracing experiments demonstrated that SVZ-derived uncommitted precursors migrate to the damaged murine cortex, the first arriving within 4 days post-stroke, where most of them give rise to reactive astrocytes that form a glial scar (Faiz et al., 2015). Some of them can convert into neurons under forced expression of Ascl1, but poor cell survival in a hostile inflammatory environment as well as a lack of pro-regenerative cues prohibit recovery of damaged cellular networks (Xing et al., 2012; Lindvall and Kokaia, 2015) leaving many stroke victims disabled for life (Mitsios et al., 2007).
In humans, lower free T3 levels correlate with increased stroke severity, complications and poorer rehabilitation (Bunevicius et al., 2015; Lamba et al., 2018) suggesting that therapeutically restoring TH levels could enhance endogenous repair responses. The potential of TH as a repair cue has been tested in adult mice and rats wherein middle cerebral artery occlusion (MCAO) simulates permanent or transient stroke, the latter allowing re-perfusion of tissue 45 – 120 min after the occlusion. T3 injection either once 24 h after cerebral ischemia, or daily for 6 days, increased cell proliferation and expression of the neurogenic markers Bdnf, Nestin and Sox2 in the SVZ 7 days later (Akhoundzadeh et al., 2017; Sabbaghziarani et al., 2017). Unfortunately, which cell types proliferated more actively was not investigated, nor was the impact on neuronal vs. glial specification. Sox2 is negatively regulated by T3 under physiological conditions (López-Juárez et al., 2012) but might here simply reflect higher NSC numbers, as T3 and TRα1 also maintain full proliferative activity of SVZ-NSCs (Lemkine et al., 2005), or alternatively a reactivation of quiescent NSCs. Apart from the SVZ, focal cerebral ischemia in adult rats also stimulated hippocampal neurogenesis (Jin et al., 2001). A single intra-cerebroventricular dose of T3 right after transient MCAO in adult rats increased neuronal survival within the SGZ 3 weeks later through enhancing expression of the neurotrophic factors Bdnf and Gdnf, thereby improving memory and learning capabilities (Mokhtari et al., 2017). A single T4 dose 1 h after traumatic brain injury in rats also induced Dcx in the hippocampus within 24 h (Li et al., 2017). This suggests that stroke occurring in distant brain regions such as the cortex can influence AHN and associated memory functions, and that TH can stimulate SGZ neurogenesis and hippocampal circuit formation during the recovery phase.
Interestingly, Ttr mRNA transcripts also increased in various hippocampal cell types (e.g., ependymal cells, microglia and mature neurons) 24 h after traumatic brain injury in adult mice. Although expression of membrane TH transporters did not change, the authors speculated that this increase reflected cellular hypothyroidism, and subsequently tested neurobehavioral recovery after giving a single intraperitoneal dose of T4 immediately after the injury. One week later, using a single-cell genomic approach, the authors observed that the dysregulated expression of 93 out of 121 genes linked to cellular metabolism and hormone response pathways in hippocampal cells, including Ttr, was restored, and that mice performed better on memory tests (Arneson et al., 2018). While TH promotion of SGZ-neurogenesis can thus improve hippocampal-related functions, new neuroblasts probably do not migrate away from their site of origin (Ibrahim et al., 2016), and hold less promise for repair at the actual site of injury in comparison to more efficiently migrating SVZ-OPCs and neuroblasts, at least in rodents (Flygt et al., 2013; Faiz et al., 2015).
New-born cells can be vulnerable to pro-apoptotic proteins that are found in injured gray matter in humans (Mitsios et al., 2007). Injection of a plasmid expressing the anti-apoptotic BCL2 protein in the lateral ventricles of rats following MCAO, protected neural progenitor cells and new-born mature neurons in the striatum from cell death (Zhang et al., 2006). Being a well-known TH-responsive gene (Morris et al., 2017), TH administration successfully reduced apoptosis 24 h after injury by inducing Bcl2 expression (Crupi et al., 2013; Genovese et al., 2013). Additionally, all these studies consistently found that post-stroke TH administration additionally preserves tissue integrity and has important anti-inflammatory effects, curtailing infarct volume and shaping a pro-survival environment in which improved synaptic plasticity enhances neural network recovery and mitigates post-injury behavioral functions (Crupi et al., 2013; Genovese et al., 2013; Akhoundzadeh et al., 2017; Li et al., 2017; Mokhtari et al., 2017; Rastogi et al., 2018; Talhada et al., 2019).
There are currently no data to which extent TH treatment repairs axonal, oligodendrocyte and myelin loss after stroke, and it has yet to be explored which local regulators of TH action could mediate effects in neurogenic zones. Certainly, governing NSC fate choice will be difficult requiring exact timing protocols. In addition, whether newly formed neurons are able to span the greater distances of the human brain is unknown (discussed in Nemirovich-Danchenko and Khodanovich, 2019). A diminished regenerative capacity during aging due to declining NSC pool sizes (Villeda et al., 2011) is another important issue as the elderly are especially prone to be stroke victims. Old mice recover less well than their younger counterparts due to exacerbated immune responses, data reflected by a higher neutrophil infiltration and infract size in aged post-mortem human stroke brains too (Ritzel et al., 2018). Most experimental studies investigating the effects of TH used young, 2–3 month-old animals, which may not represent the physiology of an older human being, notably with regard to the sharp drop in SVZ-neurogenesis and overall diminished TH levels during aging. One study demonstrated that the beneficial effects of T3 combined with bone marrow stem cell transplantation and mild exercise in 2-month-old mice on stroke outcome (Akhoundzadeh et al., 2017) were not replicated in 12-month-old mice (Akhoundzadeh et al., 2019). Thus, it is preferable that future experiments examine how NSCs in young and old animals respond to ischemic injury in parallel, hence acquiring a more reliable image of the potential therapeutic effects of TH. Lastly, determining the right timing, dose and administration route will be imperative, as subtle shifts in TH homeostasis, for instance due to ESS, can provoke adverse effects on stroke recovery. Infarct size and vascular integrity aggravated after MCAO-induced stroke in hyperthyroid rats (Keshavarz and Dehghani, 2017), and the T4 treatment that ameliorated dysregulated gene expression in hippocampal cells after injury in the mice from the study of Arneson et al., also changed non injury-related genes that are associated with pathways key for proper brain function (Arneson et al., 2018). Subclinical hyperthyroid stroke patients also had increased neurological disability and a higher risk of recurrences (Wollenweber et al., 2013). Each and every one of these issues needs to be thoroughly addressed to obtain a more comprehensive, systems biology picture of which TH treatment procedure could eventually comply with optimal injury repair.
Thyroid Hormone in Stem Cell Repair After Traumatic Spinal Cord Injury
Spinal cord trauma directly disrupts the bidirectional information flow between the brain and peripheral effectors, risking the loss of sensation and voluntary movement of tissues innervated by neurons below the site of injury. An estimated 27 million people worldwide suffer from a form of irreversible motor disability caused by SCI (James et al., 2019). As in stroke, neurons and glia die upon SCI injury and an exacerbated inflammatory and molecular inhibitory environment constrains repair (Rust and Kaiser, 2017). Endogenous NSCs could replace cells, but cannot be mobilized from distant brain stem cell zones except if they are transplanted into the lesion site (e.g., Vismara et al., 2017; Sankavaram et al., 2019). Endogenous repair could be however be stimulated by engaging the spinal cord ECs. Upon SCI, they proliferate, migrate and differentiate into astrocytes, forming a tissue scar in the first instance, and also less frequently OPCs (Meletis et al., 2008; Moreno-Manzano et al., 2009; Cawsey et al., 2015). Neurogenesis in the spinal cord is essentially non-existent in normal or injured conditions (Meletis et al., 2008), but ECs have neurogenic potential when transplanted in the SVZ (Shihabuddin et al., 2000) as well as in vitro after adding factors that stimulate neuronal differentiation (Moreno-Manzano et al., 2009). Modulating EC fate and boosting multi-lineage cell generation and differentiation could thus facilitate SCI repair.
The beneficial effects of THs on OPC differentiation as observed in EAE-induced spinal cord demyelination in rodent models of MS (Calza et al., 2002; Dell’Acqua et al., 2012), could also initiate myelin repair in SCI, although EAE does not reflect the loss of all cell types. A combination of T4 pulses with valproic acid right after disease onset improved clinical signs after EAE-induced demyelination in adult rat spinal cords, but did neither increase OPC numbers nor improve myelin status (Castelo-Branco et al., 2014) (Table 1). Continuous intraperitoneal T4 administration following transplantation of Nestin-positive NSCs, derived from embryonic stem cells, into the injury site 10 days after SCI in mice, did not improve coordinated motor behavior more than the stem cells grafts alone (Kimura et al., 2005). Whether or not manipulating local TH signaling could allow directing EC fate to stimulate neurogenesis remains unknown, and primary experiments should aim to determine how T3 affects cell fate choice in physiological conditions. Only one study demonstrated that local administration of a hydroxy gel containing T3 on the injury site caused by cervical contusion successfully increased oligodendrocytes and improved myelination (Shultz et al., 2017). ECs also express Sox2, which is a T3-responsive cell fate switch in SVZ-NSCs (López-Juárez et al., 2012). However, in contrast to repressing Sox2 in favor of SVZ neuroblast generation, stimulating Sox2 reprogrammed reactive astrocytes to form proliferative DCX-positive neuroblasts in mouse SCI, which further matured into synapsing interneurons when accompanied by valproic acid (Su et al., 2014). Also in adult zebrafish SCI, increased Sox2 expression stimulated EC proliferation and the formation of new motor neurons resulting in functional repair (Ogai et al., 2014). Another potential target might be Egfr, shown to trigger EC proliferation in SCI (Liu et al., 2019). Since Egfr is a gliogenic factor in SVZ-NSCs that is negatively regulated by T3 (Remaud et al., 2017), TH treatment could promote neuronal determination. Further research into cell-type specific expression patterns of TH regulators in both healthy- and disease-based contexts might uncover which cell types respond to TH in SCI (Boelen et al., 2009; D’Intino et al., 2011; Fernández et al., 2016).
Future Perspectives on Thyroid Hormone-Mediated CNS Repair
Endogenous NSCs are activated notably upon injury in the adult human CNS, but the NSC progeny fails to replace neurons and glial cells due to molecular inhibition and the lack of pro-regenerative cues, leaving most victims of CNS injury disabled for life. Pre-clinical studies emphasize that TH, as a key regulator of NSC fate and progenitor behavior in physiological conditions, has the potential to override the blocked endogenous repair response in various pathophysiological contexts, despite the different etiologies of disease. TH effectively regulates oligodendrogenesis and remyelination in models of MS, and while showing some beneficial actions in other CNS insults, future experiments have to elucidate how TH can stimulate endogenous repair pathways in the SVZ, SGZ and the spinal cord.
From Systemic to Local and Target-Directed TH Repair
A forthcoming challenge in TH-promoted CNS repair is to maximize and extend beneficial effects on NSCs and their progenitors while minimizing adverse effects on non-target tissues. The relative impact of parameters such as the dose, delivery route and treatment window will require scrupulous evaluation. For instance, the risk for developing (subclinical) hyperthyroidism increases with protracted TH treatment due to thyroid axis dyshomeostasis, or because disease progression or recovery enters a new phase that coincides with discrete alterations in the body’s hormone balance. Common side effects include cardiac arrhythmias and secondary osteoporosis (Flynn et al., 2010). Future pre-clinical studies applying systemic TH treatments to CNS injury models should therefore test therapeutic efficacy over longer periods while simultaneously monitoring TH serum levels and peripheral organ physiology.
A promising alternative are the TH analogs (e.g., Triac, Ditpa, sobetirome) that mimic TH action, but have CNS- or even cell type-selective actions because of TR isoform preference. Another, so far less explored way, is providing TH or TH analogs to stem cell zones by alternative routes, for instance via the cerebroventricular system (Bárez-López et al., 2019), using nanoparticles (Mdzinarishvili et al., 2013), or even via direct polyethylenimine-mediated NSC transfection (Lemkine et al., 2002). The benefit of such an approach is that TH signaling pathways relevant for a repair process could be directly manipulated in target brain regions or cell populations while leaving neighboring regions unscathed. The administration route can thereby be chosen in function of the disease or injury. Individuals suffering from the Allan-Herndon-Dudley Syndrome provide a clear example of the power such approach can have. Patients are permanently intellectually disabled due to severe cerebral hypothyroidism caused by a mutated MCT8, but have elevated serum TH levels, eliminating the option of systemic TH treatment. However, a brain implant safely delivered the TH analog Triac into the cerebral ventricles of Mct8 KO mice increasing its content in the brain, without worsening cerebral hypothyroidism nor peripheral thyrotoxicosis (Bárez-López et al., 2019). In the field of nanomedicine, NFL protein-coated lipid nanocapsules that specifically interact with NSC proteins were successfully taken up by rat NSCs after intra-cerebroventricular injection (Carradori et al., 2016). When loaded with retinoic acid, they successfully induced oligodendrogenesis after lysolecithin-induced focal demyelination of the corpus callosum (Carradori et al., 2020). These state-of-the-art methods remain to be thoroughly tested in TH-mediated CNS repair, but will definitely revolutionize neuroregenerative medicine.
Currently, we have only limited evidence of which cell types and subpopulations are more preferentially affected by systemic and local TH treatments in CNS injury models. While a beneficial effect was observed on a given cell type, for example, in the case of the OPCs in MS models, the impact on other cell types was often not evaluated. However, given the widespread expression of TRs (Bernal, 2007), one can assume that possibly all cells are affected to some extent by a TH treatment. Regulators of TH availability that confine TH action in a subset of neurogenic niche cells could represent novel ways to manipulate TH signaling tissue- or cell type-specifically, but only if we comprehend their temporal-spatial expression patterns and function in both health and disease. This goes hand in hand with dissecting how the (epi)genetic NSC landscape changes during disease to identify which TH target genes are key in repair. Such knowledge will have to be considered in light of emerging data showing that heterogeneous NSC populations in distinct SVZ microdomains contribute to specific neuronal and glial subpopulations, and thus may have varying repair potential (Chaker et al., 2016; Akkermann et al., 2017; Mizrak et al., 2019).
Other Future Aspects in TH-Mediated CNS Repair
Most studies that explored the repair potential of TH so far have been limited to rodent species in which NSC biology differs extensively from humans (Williamson and Lyons, 2018; Oppenheim, 2019). For instance, in contrast to mice, the generation of new neuroblasts is practically non-existent in the adult human SVZ and a higher percentage of NSCs is quiescent, whereas SVZ-oligodendrogenesis remains stable in both species (Sanai et al., 2004; Capilla-Gonzalez et al., 2013; Ernst et al., 2014). Such species-unique traits can be expected to partly explain why NSC-based therapies often lead to better functional outcomes in CNS injury in rodents as compared to humans. Use of non-human primate models can fill this evolutionary gap and help to estimate better to what extent beneficial effects of TH treatment on CNS repair will be replicated in humans (Tsintou et al., 2020). For example, the mouse lemur (Microcebus murinus) is a rapidly aging non-human primate, with 20% of the population developing aging-associated neurodegeneration at the age of 5 years, sharing many biological and behavioral characteristics with human AD, allowing to test disease progression and evaluate therapies better (Bons et al., 2006). On the other hand, comparative studies on the regenerative potential of TH in several non-mammalian vertebrates (Vanhunsel et al., 2020), deciphering which pathways enable successful repair, can give a better idea as to where, when and why they fail in mammals. Furthermore, most pre-clinical studies involving TH were performed on young animals, while many neurodegenerative diseases strike at older ages. It is not well understood how TH action on NSC processes such as fate choice changes during aging, and thus whether the potentially beneficial actions on CNS injury are fully preserved in the elderly. Such knowledge gaps emphasize the pertinence of fundamental research in these domains.
In addition, one cannot discuss TH signaling without considering other important, interconnected factors that regulate NSC behavior in normal and pathological conditions (see for instance Obernier and Alvarez-Buylla, 2019). In MS for example, Notch, Wnt and retinoid signaling, as well as the transmembrane signaling protein LINGO-1 also regulate oligodendrogenesis and thus represent additional therapeutic targets (Huang and Franklin, 2011). TH often interacts with such signaling pathways as well as with other hormones that influence neurogliogenesis (e.g., progesterone, testosterone, estrogen) (Hartley et al., 2014), demanding an integrative systems biology approach to understand the effects of TH-replacing therapy fully.
Lastly, in the case of chronic neurodegenerative diseases, many questions also remain with regard to the pathophysiological mechanisms or origins that lead to disease in the first place, that underlie disease progression, or that account for unexplained incidence trends and gender-specific effects. In MS for example, an unexplained global shift of 2/1 to 3/1 in the ratio of women versus men occurred over the past decades (Reich et al., 2018; Wallin et al., 2019). Recent evidence suggests that MS is at least partially provoked or exacerbated by environmental factors (Leray et al., 2016; Bove, 2018). Since MS incidence is considerably high in industrialized countries, one possibility is that endocrine disrupting chemicals (EDCs) to which we are daily exposed are implicated. Chemical production has increased more than 300-fold since 1970 (Demeneix, 2019), and many of them share the halogenated structure with THs, making them potentially able to provoke adverse, disrupting actions on TH signaling pathways (Boas et al., 2012). For several EDCs, it has already been shown they affect adult stem cell self-renewal, lineage decisions and differentiation programs (Kopras et al., 2014). As shown in post mortem brain samples, lipo-soluble EDCs also accumulate in myelin, in essence a stack of lipid sheaths (van der Meer et al., 2017) and could thus have toxic effects that trigger oligodendrocyte apoptosis. Developmental exposure could also compromise NSC repair potential in later life through epigenetic misprogramming (Jacobs et al., 2017). There is an urge to elucidate whether or not early-life EDC exposure renders the brain vulnerable to diseases or malfunctions later in life (Porta and Vandenberg, 2019), since developmentally disrupted TH signaling has already been linked to increased risk for autism spectrum disorder, attention deficit/hyperactivity disorder and schizophrenia (Modesto et al., 2015; Gyllenberg et al., 2016).
In conclusion, improved understanding and new established concepts in TH physiology at the cellular and molecular level, are opening up new avenues for boosting NSC-dependent repair with TH as a pro-repair cue. Clinical trials could lead to therapies that might one day facilitate reversing currently untreatable disabilities that accompany CNS injury.
Author Contributions
PV and SR worked out the concept of the manuscript. PV made the figures and table. PV, LB, BD, and SR wrote the manuscript and all authors verified the final version of the manuscript.
Funding
PV was supported by the European Thyroid Association (basic science grant) and the Fondation pour la Recherche Médicale (FRM) (Grant: SPF201909009111). This work was additionally supported by the Centre National de la Recherche Scientifique (CNRS) and the EU H2020 contract Thyrage (Grant no. 666869).
Conflict of Interest
The authors declare that the research was conducted in the absence of any commercial or financial relationships that could be construed as a potential conflict of interest.
Abbreviations
AD, Alzheimer’s disease; AHN, adult hippocampal neurogenesis; APP, amyloid precursor protein; BBB, blood-brain barrier; BCSFB, blood-cerebrospinal fluid barrier; CNS, central nervous system; CSF, cerebrospinal fluid; DIO, deiodinase; EAE, experimental autoimmune encephalomyelitis; ECs, ependymal cells; EDCs, endocrine disrupting chemicals; ESS, euthyroid sick syndrome; GSK-3 α / β, glycogen synthase kinase 3 α / β; HPT, hypothalamus-pituitary-thyroid; IHC, immunohistochemistry; LAT, large neutral amino acid transporter; MBP, myelin basic protein; MCAO, middle cerebral artery occlusion; MCT, monocarboyxlate transporter; MRI, magnetic resonance imaging; MS, multiple sclerosis; NSC, neural stem cell; OATP1C1, organic anion transporting polypeptide 1C1; OPC, oligodendrocyte precursor cell; pOPC, parenchymal oligodendrocyte precursor cell; rT3, reverse T3 or 3,3 ′,5 ′ -triiodothyronine; SCI, spinal cord injury; SGZ, subgranular zone; SVZ, subventricular zone; T3, 3,5,3 ′ -triiodothyronine; T4, 3,5,3 ′,5 ′ -tetraiodothyronine or thyroxine; TAP, transient amplifying progenitor; TH, thyroid hormone; TR, thyroid hormone receptor; TRH, thyrotropin-releasing hormone; TSH, thyroid-stimulating hormone or thyrotropin; TTR, transthyretin.
References
Aguirre, A., Dupree, J. L., Mangin, J. M., and Gallo, V. (2007). A functional role for EGFR signaling in myelination and remyelination. Nat. Neurosci. 10, 990–1002. doi: 10.1038/nn1938
Aguirre, A., Rubio, M. E., and Gallo, V. (2010). Notch and EGFR pathway interaction regulates neural stem cell number and self-renewal. Nature 467, 323–327. doi: 10.1038/nature09347
Ahlenius, H., Visan, V., Kokaia, M., Lindvall, O., and Kokaia, Z. (2009). Neural stem and progenitor cells retain their potential for proliferation and differentiation into functional neurons despite lower number in aged brain. J. Neurosci. 29, 4408–4419. doi: 10.1523/JNEUROSCI.6003-08.2009
Akhoundzadeh, K., Vakili, A., and Sameni, H. R. (2019). Bone marrow stromal cells with exercise and thyroid hormone effect on post-stroke injuries in middle-aged mice. Basic Clin. Neurosci. 10, 73–84. doi: 10.32598/bcn.9.10.355
Akhoundzadeh, K., Vakili, A., Sameni, H. R., Vafaei, A. A., Rashidy-Pour, A., Safari, M., et al. (2017). Effects of the combined treatment of bone marrow stromal cells with mild exercise and thyroid hormone on brain damage and apoptosis in a mouse focal cerebral ischemia model. Metab. Brain Dis. 32, 1267–1277. doi: 10.1007/s11011-017-0034-0
Akkermann, R., Beyer, F., and Küry, P. (2017). Heterogeneous populations of neural stem cells contribute to myelin repair. Neural Regen. Res. 12, 509–517. doi: 10.4103/1673-5374.204999
Alemi, M., Gaiteiro, C., Ribeiro, C. A., Santos, L. M., Gomes, J. R., Oliveira, S. M., et al. (2016). Transthyretin participates in beta-amyloid transport from the brain to the liver- involvement of the low-density lipoprotein receptor-related protein 1? Sci. Rep. 6:20164. doi: 10.1038/srep20164
Alshehri, B., Pagnin, M., Lee, J. Y., Petratos, S., and Richardson, S. J. (2020). The role of transthyretin in oligodendrocyte development. Sci. Rep. 10:4189. doi: 10.1038/s41598-020-60699-8
Arneson, D., Zhang, G., Ying, Z., Zhuang, Y., Byun, H. R., Ahn, I. S., et al. (2018). Single cell molecular alterations reveal target cells and pathways of concussive brain injury. Nat. Commun. 9:3894. doi: 10.1038/s41467-018-06222-0
Azevedo, F. A. C., Carvalho, L. R. B., Grinberg, L. T., Farfel, J. M., Ferretti, R. E. L., Leite, R. E. P., et al. (2009). Equal numbers of neuronal and nonneuronal cells make the human brain an isometrically scaled-up primate brain. J. Comp. Neurol. 513, 532–541. doi: 10.1002/cne.21974
Baas, D., Bourbeau, D., Sarliève, L. L., Ittel, M. E., Dussault, J. H., and Puymirat, J. (1997). Oligodendrocyte maturation and progenitor cell proliferation are independently regulated by thyroid hormone. Glia 19, 324–332. doi: 10.1002/(sici)1098-1136(199704)19:4<324::aid-glia5>3.0.co;2-x
Baas, D., Fressinaud, C., Vitkovic, L., and Sarlieve, L. L. (1998). Glutamine synthetase expression and activity are regulated by 3,5,3’ -triiodo-L-thyronine and hydrocortisone in rat oligodendrocyte cultures. Int. J. Dev. Neurosci. 16, 333–340. doi: 10.1016/S0736-5748(98)00040-9
Baldassarro, V. A., Krêżel, W., Fernández, M., Schuhbaur, B., Giardino, L., and Calzà, L. (2019). The role of nuclear receptors in the differentiation of oligodendrocyte precursor cells derived from fetal and adult neural stem cells. Stem Cell Res. 37:101443. doi: 10.1016/j.scr.2019.101443
Bárez-López, S., Bosch-García, D., Gómez-Andrés, D., Pulido-Valdeolivas, I., Montero-Pedrazuela, A., Obregon, M. J., et al. (2014). Abnormal motor phenotype at adult stages in mice lacking type 2 deiodinase. PLoS One 9:e103857. doi: 10.1371/journal.pone.0103857
Bárez-López, S., Grijota-Martínez, C., Liao, X.-H. H., Refetoff, S., and Guadaño-Ferraz, A. (2019). Intracerebroventricular administration of the thyroid hormone analog TRIAC increases its brain content in the absence of MCT8. PLoS One 14:e0226017. doi: 10.1371/journal.pone.0226017
Bárez-López, S., and Guadaño-Ferraz, A. (2017). Thyroid hormone availability and action during brain development in rodents. Front. Cell. Neurosci. 11:240. doi: 10.3389/fncel.2017.00240
Barres, B. A., Lazar, M. A., and Raff, M. C. (1994). A novel role for thyroid hormone, glucocorticoids and retinoic acid in timing oligodendrocyte development. Development 120, 1097–1108.
Baxi, E. G., DeBruin, J., Jin, J., Strasburger, H. J., Smith, M. D., Orthmann-Murphy, J. L., et al. (2017). Lineage tracing reveals dynamic changes in oligodendrocyte precursor cells following cuprizone-induced demyelination. Glia 65, 2087–2098. doi: 10.1002/glia.23229
Baxi, E. G., Schott, J. T., Fairchild, A. N., Kirby, L. A., Karani, R., Uapinyoying, P., et al. (2014). A selective thyroid hormone β receptor agonist enhances human and rodent oligodendrocyte differentiation. Glia 62, 1513–1529. doi: 10.1002/glia.22697
Belakavadi, M., Dell, J., Grover, G. J., and Fondell, J. D. (2011). Thyroid hormone suppression of β-amyloid precursor protein gene expression in the brain involves multiple epigenetic regulatory events. Mol. Cell. Endocrinol. 339, 72–80. doi: 10.1016/j.mce.2011.03.016
Belandia, B., Latasa, M. J., Villa, A., and Pascual, A. (1998). Thyroid hormone negatively regulates the transcriptional activity of the β-amyloid precursor protein gene. J. Biol. Chem. 273, 30366–30371. doi: 10.1074/jbc.273.46.30366
Bernal, J. (2007). Thyroid hormone receptors in brain development and function. Nat. Clin. Pract. Endocrinol. Metab. 3, 249–259. doi: 10.1038/ncpendmet0424
Bhumika, S., and Darras, V. M. (2014). Role of thyroid hormones in different aspects of nervous system regeneration in vertebrates. Gen. Comp. Endocrinol. 203, 86–94. doi: 10.1016/j.ygcen.2014.03.017
Bianco, A. C., Salvatore, D., Gereben, B., Berry, M. J., and Larsen, P. R. (2002). Biochemistry, cellular and molecular biology, and physiological roles of the iodothyronine selenodeiodinases. Endocr. Rev. 23, 38–89. doi: 10.1210/edrv.23.1.0455
Billon, N. (2002). Normal timing of oligodendrocyte development depends on thyroid hormone receptor alpha 1 (TRalpha1). EMBO J. 21, 6452–6460. doi: 10.1093/emboj/cdf662
Billon, N., Tokumoto, Y., Forrest, D., and Raff, M. (2001). Role of thyroid hormone receptors in timing oligodendrocyte differentiation. Dev. Biol. 235, 110–120. doi: 10.1006/dbio.2001.0293
Biondi, B., Cappola, A. R., and Cooper, D. S. (2019). Subclinical hypothyroidism. JAMA 322, 153–160. doi: 10.1001/jama.2019.9052
Blakemore, W. F., and Franklin, R. J. M. (2008). “Remyelination in experimental models of toxin-induced demyelination,” in Advances in multiple Sclerosis and Experimental Demyelinating Diseases, Vol. 318, ed. M. Rodriguez (Berlin: Springer), 193–212. doi: 10.1007/978-3-540-73677-6_8
Boas, M., Feldt-Rasmussen, U., and Main, K. M. (2012). Thyroid effects of endocrine disrupting chemicals. Mol. Cell. Endocrinol. 355, 240–248. doi: 10.1016/j.mce.2011.09.005
Boelen, A., Mikita, J., Boiziau, C., Chassande, O., Fliers, E., and Petry, K. G. (2009). Type 3 deiodinase expression in inflammatory spinal cord lesions in rat experimental autoimmune encephalomyelitis. Thyroid 19, 1401–1406. doi: 10.1089/thy.2009.0228
Boldrini, M., Fulmore, C. A., Tartt, A. N., Simeon, L. R., Pavlova, I., Poposka, V., et al. (2018). Human hippocampal neurogenesis persists throughout aging. Cell Stem Cell 22, 589. doi: 10.1016/j.stem.2018.03.015
Bons, N., Rieger, F., Prudhomme, D., Fisher, A., and Krause, K.-H. (2006). Microcebus murinus: a useful primate model for human cerebral aging and Alzheimer’s disease? Genes Brain Behav. 5, 120–130. doi: 10.1111/j.1601-183X.2005.00149.x
Bove, R. M. (2018). Why monkeys do not get multiple sclerosis (spontaneously). Evol. Med. Public Heal. 2018, 43–59. doi: 10.1093/emph/eoy002
Bowers, J., Terrien, J., Clerget-Froidevaux, M. S., Gothié, J. D., Rozing, M. P., Westendorp, R. G. J., et al. (2013). Thyroid hormone signaling and homeostasis during aging. Endocr. Rev. 34, 556–589. doi: 10.1210/er.2012-1056
Brousse, B., Magalon, K., Durbec, P., and Cayre, M. (2015). Region and dynamic specificities of adult neural stem cells and oligodendrocyte precursors in myelin regeneration in the mouse brain. Biol. Open 4, 980–992. doi: 10.1242/bio.012773
Bunevicius, A., Iervasi, G., and Bunevicius, R. (2015). Neuroprotective actions of thyroid hormones and low-T3 syndrome as a biomarker in acute cerebrovascular disorders. Expert Rev. Neurother. 15, 315–326. doi: 10.1586/14737175.2015.1013465
Buxbaum, J. N., Ye, Z., Reixach, N., Friske, L., Levy, C., Das, P., et al. (2008). Transthyretin protects Alzheimer’s mice from the behavioral and biochemical effects of A toxicity. Proc. Natl. Acad. Sci. U.S.A. 105, 2681–2686. doi: 10.1073/pnas.0712197105
Callen, D. J. A., Black, S. E., Gao, F., Caldwell, C. B., and Szalai, J. P. (2001). Beyond the hippocampus: MRI volumetry confirms widespread limbic atrophy in AD. Neurology 57, 1669–1674. doi: 10.1212/WNL.57.9.1669
Calzà, L., Fernandez, M., and Giardino, L. (2010). Cellular approaches to central nervous system remyelination stimulation: thyroid hormone to promote myelin repair via endogenous stem and precursor cells. J. Mol. Endocrinol. 44, 13–23. doi: 10.1677/JME-09-0067
Calza, L., Fernandez, M., Giuliani, A., Aloe, L., and Giardino, L. (2002). Thyroid hormone activates oligodendrocyte precursors and increases a myelin-forming protein and NGF content in the spinal cord during experimental allergic encephalomyelitis. Proc. Natl. Acad. Sci. U.S.A. 99, 3258–3263. doi: 10.1073/pnas.052704499
Calzà, L., Giardino, L., and Hökfelt, T. (1998). Thyroid hormone-dependent regulation of galanin synthesis in neurons and glial cells after colchicine administration. Neuroendocrinology 68, 428–436. doi: 10.1159/000054393
Capilla-Gonzalez, V., Cebrian-Silla, A., Guerrero-Cazares, H., Garcia-Verdugo, J. M., and Quiñones-Hinojosa, A. (2013). The generation of oligodendroglial cells is preserved in the rostral migratory stream during aging. Front. Cell. Neurosci. 7:147. doi: 10.3389/fncel.2013.00147
Capilla-Gonzalez, V., Herranz-Pérez, V., and García-Verdugo, J. M. (2015). The aged brain: genesis and fate of residual progenitor cells in the subventricular zone. Front. Cell. Neurosci. 9:365. doi: 10.3389/fncel.2015.00365
Carradori, D., Labrak, Y., Miron, V. E., Saulnier, P., Eyer, J., Préat, V., et al. (2020). Retinoic acid-loaded NFL-lipid nanocapsules promote oligodendrogenesis in focal white matter lesion. Biomaterials 230, 119653. doi: 10.1016/j.biomaterials.2019.119653
Carradori, D., Saulnier, P., Préat, V., des Rieux, A., and Eyer, J. (2016). NFL-lipid nanocapsules for brain neural stem cell targeting in vitro and in vivo. J. Control. Release 238, 253–262. doi: 10.1016/j.jconrel.2016.08.006
Castelo-Branco, G., Stridh, P., Guerreiro-Cacais, A. O., Adzemovic, M. Z., Falcão, A. M., Marta, M., et al. (2014). Acute treatment with valproic acid and l-thyroxine ameliorates clinical signs of experimental autoimmune encephalomyelitis and prevents brain pathology in DA rats. Neurobiol. Dis. 71, 220–233. doi: 10.1016/j.nbd.2014.08.019
Cawsey, T., Duflou, J., Weickert, C. S., and Gorrie, C. A. (2015). Nestin-positive ependymal cells are increased in the human spinal cord after traumatic central nervous system injury. J. Neurotrauma 32, 1393–1402. doi: 10.1089/neu.2014.3575
Chaker, Z., Codega, P., and Doetsch, F. (2016). A mosaic world: puzzles revealed by adult neural stem cell heterogeneity. Wiley Interdiscip. Rev. Dev. Biol. 5, 640–658. doi: 10.1002/wdev.248
Chan, S. Y., Hancox, L. A., Martín-Santos, A., Loubière, L. S., Walter, M. N. M., González, A.-M., et al. (2014). MCT8 expression in human fetal cerebral cortex is reduced in severe intrauterine growth restriction. J. Endocrinol. 220, 85–95. doi: 10.1530/JOE-13-0400
Chang, A., Nishiyama, A., Peterson, J., Prineas, J., and Trapp, B. D. (2000). NG2-positive oligodendrocyte progenitor cells in adult human brain and multiple sclerosis lesions. J. Neurosci. 20, 6404–6412. doi: 10.1523/JNEUROSCI.20-17-06404.2000
Chatonnet, F., Flamant, F., and Morte, B. (2015). A temporary compendium of thyroid hormone target genes in brain. Biochim. Biophys. Acta 1849, 122–129. doi: 10.1016/j.bbagrm.2014.05.023
Chen, Y., Sjölinder, M., Wang, X., Altenbacher, G., Hagner, M., Berglund, P., et al. (2012). Thyroid hormone enhances nitric oxide-mediated bacterial clearance and promotes survival after meningococcal infection. PLoS One 7:e41445. doi: 10.1371/journal.pone.0041445
Constantinescu, C. S., Farooqi, N., O’Brien, K., and Gran, B. (2011). Experimental autoimmune encephalomyelitis (EAE) as a model for multiple sclerosis (MS). Br. J. Pharmacol. 164, 1079–1106. doi: 10.1111/j.1476-5381.2011.01302.x
Cooke, G. E., Mullally, S., Correia, N., O’Mara, S. M., and Gibney, J. (2014). Hippocampal volume is decreased in adults with hypothyroidism. Thyroid 24, 433–440. doi: 10.1089/thy.2013.0058
Correia, N., Mullally, S., Cooke, G., Tun, T. K., Phelan, N., Feeney, J., et al. (2009). Evidence for a specific defect in hippocampal memory in overt and subclinical hypothyroidism. J. Clin. Endocrinol. Metab. 94, 3789–3797. doi: 10.1210/jc.2008-2702
Crupi, R., Paterniti, I., Campolo, M., Di Paola, R., Cuzzocrea, S., and Esposito, E. (2013). Exogenous T3 administration provides neuroprotection in a murine model of traumatic brain injury. Pharmacol. Res. 70, 80–89. doi: 10.1016/j.phrs.2012.12.009
Daimon, C., Chirdon, P., Maudsley, S., and Martin, B. (2013). The role of thyrotropin releasing hormone in aging and neurodegenerative diseases. Am. J. Alzheimers Dis. 1:10.7726/ajad.2013.1003. doi: 10.7726/ajad.2013.1003
Davis, J., Podolanczuk, A., Donahue, J., Stopa, E., Hennessey, J., Luo, L.-G., et al. (2008). Thyroid hormone levels in the prefrontal cortex of post-mortem brains of Alzheimers disease patients. Curr. Aging Sci. 1, 175–181. doi: 10.2174/1874609810801030175
Daynac, M., Morizur, L., Chicheportiche, A., Mouthon, M.-A., and Boussin, F. D. (2016). Age-related neurogenesis decline in the subventricular zone is associated with specific cell cycle regulation changes in activated neural stem cells. Sci. Rep. 6:21505. doi: 10.1038/srep21505
Dell’Acqua, M. L., Lorenzini, L., D’Intino, G., Sivilia, S., Pasqualetti, P., Panetta, V., et al. (2012). Functional and molecular evidence of myelin- and neuroprotection by thyroid hormone administration in experimental allergic encephalomyelitis. Neuropathol. Appl. Neurobiol. 38, 454–470. doi: 10.1111/j.1365-2990.2011.01228.x
Demeneix, B. A. (2019). Evidence for prenatal exposure to thyroid disruptors and adverse effects on brain development. Eur. Thyroid J. 8, 283–292. doi: 10.1159/000504668
Desouza, L. A., Ladiwala, U., Daniel, S. M., Agashe, S., Vaidya, R. A., and Vaidya, V. A. (2005). Thyroid hormone regulates hippocampal neurogenesis in the adult rat brain. Mol. Cell. Neurosci. 29, 414–426. doi: 10.1016/j.mcn.2005.03.010
D’Intino, G., Lorenzini, L., Fernandez, M., Taglioni, A., Perretta, G., Del Vecchio, G., et al. (2011). Triiodothyronine administration ameliorates the demyelination/remyelination ratio in a non-human primate model of multiple sclerosis by correcting tissue hypothyroidism. J. Neuroendocrinol. 23, 778–790. doi: 10.1111/j.1365-2826.2011.02181.x
Doetsch, F., Caillé, I., Lim, D. A., García-Verdugo, J. M., and Alvarez-Buylla, A. (1999). Subventricular zone astrocytes are neural stem cells in the adult mammalian brain. Cell 97, 703–716. doi: 10.1016/S0092-8674(00)80783-7
Dugas, J. C., Ibrahim, A., and Barres, B. A. (2012). The T3-induced gene KLF9 regulates oligodendrocyte differentiation and myelin regeneration. Mol. Cell. Neurosci. 50, 45–57. doi: 10.1016/j.mcn.2012.03.007
Dulamea, A. O. (2017). Role of oligodendrocyte dysfunction in demyelination, remyelination and neurodegeneration in multiple sclerosis. Adv. Exp. Med. Biol. 958, 91–127. doi: 10.1007/978-3-319-47861-6_7
Durand, B., and Raff, M. (2000). A cell-intrinsic timer that operates during oligodendrocyte development. Bioessays 22, 64–71. doi: 10.1002/(sici)1521-1878(200001)22:1<64::aid-bies11>3.0.co;2-q
Eitan, R., Landshut, G., Lifschytz, T., Einstein, O., Ben-Hur, T., and Lerer, B. (2010). The thyroid hormone, triiodothyronine, enhances fluoxetine-induced neurogenesis in rats: possible role in antidepressant-augmenting properties. Int. J. Neuropsychopharmacol. 13, 553–561. doi: 10.1017/S1461145709990769
El-Tahry, H., Marei, H. E., Shams, A., El-Shahat, M., Abdelaziz, H., and Abd El-kader, M. (2016). The effect of triiodothyronine on maturation and differentiation of oligodendrocyte progenitor cells during remyelination following induced demyelination in male albino rat. Tissue Cell 48, 242–251. doi: 10.1016/j.tice.2016.03.001
Eriksson, P. S., Perfilieva, E., Björk-Eriksson, T., Alborn, A.-M., Nordborg, C., Peterson, D. A., et al. (1998). Neurogenesis in the adult human hippocampus. Nat. Med. 4, 1313–1317. doi: 10.1038/3305
Ernst, A., Alkass, K., Bernard, S., Salehpour, M., Perl, S., Tisdale, J., et al. (2014). Neurogenesis in the Striatum of the Adult Human Brain. Cell 156, 1072–1083. doi: 10.1016/j.cell.2014.01.044
Faiz, M., Sachewsky, N., Gascón, S., Bang, K. W. W. A. A., Morshead, C. M., and Nagy, A. (2015). Adult neural stem cells from the subventricular zone give rise to reactive astrocytes in the cortex after stroke. Cell Stem Cell 17, 624–634. doi: 10.1016/j.stem.2015.08.002
Farwell, A. P., Dubord-Tomasetti, S. A., Pietrzykowski, A. Z., and Leonard, J. L. (2006). Dynamic nongenomic actions of thyroid hormone in the developing rat brain. Endocrinology 147, 2567–2574. doi: 10.1210/en.2005-1272
Feigin, V. L., Nichols, E., Alam, T., Bannick, M. S., Beghi, E., Blake, N., et al. (2019). Global, regional, and national burden of neurological disorders, 1990–2016: a systematic analysis for the Global Burden of Disease Study 2016. Lancet Neurol. 18, 459–480. doi: 10.1016/S1474-4422(18)30499-X
Fekete, C., and Lechan, R. M. (2014). central regulation of hypothalamic-pituitary-thyroid axis under physiological and pathophysiological conditions. Endocr. Rev. 35, 159–194. doi: 10.1210/er.2013-1087
Fernández, M., Baldassarro, V. A., Sivilia, S., Giardino, L., and Calzà, L. (2016). Inflammation severely alters thyroid hormone signaling in the central nervous system during experimental allergic encephalomyelitis in rat: direct impact on OPCs differentiation failure. Glia 64, 1573–1589. doi: 10.1002/glia.23025
Fernandez, M., Giuliani, A., Pirondi, S., D’Intino, G., Giardino, L., Aloe, L., et al. (2004). Thyroid hormone administration enhances remyelination in chronic demyelinating inflammatory disease. Proc. Natl. Acad. Sci. U.S.A. 101, 16363–16368. doi: 10.1073/pnas.0407262101
Fernández, M., Paradisi, M., Del Vecchio, G., Giardino, L., and Calzà, L. (2009). Thyroid hormone induces glial lineage of primary neurospheres derived from non-pathological and pathological rat brain: implications for remyelination-enhancing therapies. Int. J. Dev. Neurosci. 27, 769–778. doi: 10.1016/j.ijdevneu.2009.08.011
Filippi, M., Brück, W., Chard, D., Fazekas, F., Geurts, J. J. G., Enzinger, C., et al. (2019). Association between pathological and MRI findings in multiple sclerosis. Lancet Neurol. 18, 198–210. doi: 10.1016/S1474-4422(18)30451-4
Fliers, E., Bianco, A. C., Langouche, L., and Boelen, A. (2015). Thyroid function in critically ill patients. Lancet Diabetes Endocrinol. 3, 816–825. doi: 10.1016/S2213-8587(15)00225-9
Flygt, J., Djupsjö, A., Lenne, F., and Marklund, N. (2013). Myelin loss and oligodendrocyte pathology in white matter tracts following traumatic brain injury in the rat. Eur. J. Neurosci. 38, 2153–2165. doi: 10.1111/ejn.12179
Flynn, R. W., Bonellie, S. R., Jung, R. T., MacDonald, T. M., Morris, A. D., and Leese, G. P. (2010). Serum thyroid-stimulating hormone concentration and morbidity from cardiovascular disease and fractures in patients on long-term thyroxine therapy. J. Clin. Endocrinol. Metab. 95, 186–193. doi: 10.1210/jc.2009-1625
Franco, P. G., Silvestroff, L., Soto, E. F., and Pasquini, J. M. (2008). Thyroid hormones promote differentiation of oligodendrocyte progenitor cells and improve remyelination after cuprizone-induced demyelination. Exp. Neurol. 212, 458–467. doi: 10.1016/j.expneurol.2008.04.039
Franco-Pons, N., Torrente, M., Colomina, M. T., and Vilella, E. (2007). Behavioral deficits in the cuprizone-induced murine model of demyelination/remyelination. Toxicol. Lett. 169, 205–213. doi: 10.1016/j.toxlet.2007.01.010
Franklin, R. J. M., Ffrench-Constant, C., Edgar, J. M., and Smith, K. J. (2012). Neuroprotection and repair in multiple sclerosis. Nat. Rev. Neurol. 8, 624–634. doi: 10.1038/nrneurol.2012.200
Fu, A. L., Zhou, C. Y., and Chen, X. (2010). Thyroid hormone prevents cognitive deficit in a mouse model of Alzheimer’s disease. Neuropharmacology 58, 722–729. doi: 10.1016/j.neuropharm.2009.12.020
Genovese, T., Impellizzeri, D., Ahmad, A., Cornelius, C., Campolo, M., Cuzzocrea, S., et al. (2013). Post-ischaemic thyroid hormone treatment in a rat model of acute stroke. Brain Res. 1513, 92–102. doi: 10.1016/j.brainres.2013.03.001
Gil-Ibañez, P., García-García, F., Dopazo, J., Bernal, J., and Morte, B. (2017). Global transcriptome analysis of primary cerebrocortical cells: identification of genes regulated by triiodothyronine in specific cell types. Cereb. Cortex 27, 706–717. doi: 10.1093/cercor/bhv273
Gilmore, C. P., Bo, L., Owens, T., Lowe, J., Esiri, M. M., and Evangelou, N. (2006). Spinal cord gray matter demyelination in multiple sclerosis—a novel pattern of residual plaque morphology. Brain Pathol. 16, 202–208. doi: 10.1111/j.1750-3639.2006.00018.x
Gil-Perotin, S., Duran-Moreno, M., Belzunegui, S., Luquin, M. R., and Garcia-Verdugo, J. M. (2009). Ultrastructure of the subventricular zone in Macaca fascicularis and evidence of a mouse-like migratory stream. J. Comp. Neurol. 514, 533–554. doi: 10.1002/cne.22026
Giordano, T., Pan, J. B., Casuto, D., Watanabe, S., and Arneric, S. P. (1992). Thyroid hormone regulation of NGF, NT-3 and BDNF RNA in the adult rat brain. Mol. Brain Res. 16, 239–245. doi: 10.1016/0169-328X(92)90231-Y
Gonzalez-Perez, O., and Alvarez-Buylla, A. (2011). Oligodendrogenesis in the subventricular zone and the role of epidermal growth factor. Brain Res. Rev. 67, 147–156. doi: 10.1016/j.brainresrev.2011.01.001
Gorelick, P. B. (2019). The global burden of stroke: persistent and disabling. Lancet Neurol. 18, 417–418. doi: 10.1016/S1474-4422(19)30030-4
Gothié, J.-D., Demeneix, B., and Remaud, S. (2017). Comparative approaches to understanding thyroid hormone regulation of neurogenesis. Mol. Cell. Endocrinol. 459, 104–115. doi: 10.1016/j.mce.2017.05.020
Gothié, J. D., Vancamp, P., Demeneix, B., and Remaud, S. (2020). Thyroid hormone regulation of neural stem cell fate: from development to ageing. Acta Physiol. 228:e13316. doi: 10.1111/apha.13316
Grandel, H., Kaslin, J., Ganz, J., Wenzel, I., and Brand, M. (2006). Neural stem cells and neurogenesis in the adult zebrafish brain: origin, proliferation dynamics, migration and cell fate. Dev. Biol. 295, 263–277. doi: 10.1016/j.ydbio.2006.03.040
Groeneweg, S., van Geest, F. S., Peeters, R. P., Heuer, H., and Visser, W. E. (2020). Thyroid hormone transporters. Endocr. Rev. 41, 146–201. doi: 10.1210/endrev/bnz008
Guadaño-Ferraz, A., Benavides-Piccione, R., Venero, C., Lancha, C., Vennström, B., Sandi, C., et al. (2003). Lack of thyroid hormone receptor α1 is associated with selective alterations in behavior and hippocampal circuits. Mol. Psychiatry 8, 30–38. doi: 10.1038/sj.mp.4001196
Guissouma, H., Ghaddab-Zroud, R., Seugnet, I., Decherf, S., Demeneix, B., and Clerget-Froidevaux, M.-S. (2014). TR Alpha 2 exerts dominant negative effects on hypothalamic Trh transcription in vivo. PLoS One 9:e95064. doi: 10.1371/journal.pone.0095064
Gyllenberg, D., Sourander, A., Surcel, H.-M., Hinkka-Yli-Salomäki, S., McKeague, I. W., and Brown, A. S. (2016). Hypothyroxinemia during gestation and offspring schizophrenia in a national birth cohort. Biol. Psychiatry 79, 962–970. doi: 10.1016/j.biopsych.2015.06.014
Hachem, L. D., Mothe, A. J., and Tator, C. H. (2020). Unlocking the paradoxical endogenous stem cell response after spinal cord injury. Stem Cells 38, 187–194. doi: 10.1002/stem.3107
Haider, L., Zrzavy, T., Hametner, S., Höftberger, R., Bagnato, F., Grabner, G., et al. (2016). The topograpy of demyelination and neurodegeneration in the multiple sclerosis brain. Brain 139, 807–815. doi: 10.1093/brain/awv398
Hansson, S. F., Andréasson, U., Wall, M., Skoog, I., Andreasen, N., Wallin, A., et al. (2009). Reduced levels of amyloid-β-binding proteins in cerebrospinal fluid from Alzheimer’s disease patients. J. Alzheimers Dis. 16, 389–397. doi: 10.3233/JAD-2009-0966
Harsan, L.-A., Steibel, J., Zaremba, A., Agin, A., Sapin, R., Poulet, P., et al. (2008). Recovery from chronic demyelination by thyroid hormone therapy: myelinogenesis induction and assessment by diffusion tensor magnetic resonance imaging. J. Neurosci. 28, 14189–14201. doi: 10.1523/JNEUROSCI.4453-08.2008
Hartley, M. D., Altowaijri, G., and Bourdette, D. (2014). Remyelination and multiple sclerosis: therapeutic approaches and challenges. Curr. Neurol. Neurosci. Rep. 14:485. doi: 10.1007/s11910-014-0485-1
Hartley, M. D., Banerji, T., Tagge, I. J., Kirkemo, L. L., Chaudhary, P., Calkins, E., et al. (2019). Myelin repair stimulated by CNS-selective thyroid hormone action. JCI Insight 4:e126329. doi: 10.1172/jci.insight.126329
Haughey, N. J., Liu, D., Nath, A., Borchard, A. C., and Mattson, M. P. (2002a). Disruption of neurogenesis in the subventricular zone of adult mice, and in human cortical neuronal precursor cells in culture, by amyloid β-Peptideby amyloid β-Peptide. Neuromol. Med. 1, 125–136. doi: 10.1385/nmm:1:2:125
Haughey, N. J., Nath, A., Chan, S. L., Borchard, A. C., Rao, M. S., and Mattson, M. P. (2002b). Disruption of neurogenesis by amyloid β-peptide, and perturbed neural progenitor cell homeostasis, in models of Alzheimer’s disease. J. Neurochem. 83, 1509–1524. doi: 10.1046/j.1471-4159.2002.01267.x
Hooper, C., Killick, R., and Lovestone, S. (2008). The GSK3 hypothesis of Alzheimer’s disease. J. Neurochem. 104, 1433–1439. doi: 10.1111/j.1471-4159.2007.05194.x
Huang, J. K., and Franklin, R. J. M. (2011). Regenerative medicine in multiple sclerosis: identifying pharmacological targets of adult neural stem cell differentiation. Neurochem. Int. 59, 329–332. doi: 10.1016/j.neuint.2011.01.017
Ibrahim, S., Hu, W., Wang, X., Gao, X., He, C., and Chen, J. (2016). Traumatic brain injury causes aberrant migration of adult-born neurons in the hippocampus. Sci. Rep. 6:21793. doi: 10.1038/srep21793
Jacobs, M. N., Marczylo, E. L., Guerrero-Bosagna, C., and Rüegg, J. (2017). Marked for life: epigenetic effects of endocrine disrupting chemicals. Annu. Rev. Environ. Res. 42, 105–160. doi: 10.1146/annurev-environ-102016-061111
James, S. L., Theadom, A., Ellenbogen, R. G., Bannick, M. S., Montjoy-Venning, W., Lucchesi, L. R., et al. (2019). Global, regional, and national burden of traumatic brain injury and spinal cord injury, 1990–2016: a systematic analysis for the Global Burden of Disease Study 2016. Lancet Neurol. 18, 56–87. doi: 10.1016/S1474-4422(18)30415-0
Jeannin, E., Robyr, D., and Desvergne, B. (1998). Transcriptional regulatory patterns of the myelin basic protein and malic enzyme genes by the thyroid hormone receptors α1 and β1. J. Biol. Chem. 273, 24239–24248. doi: 10.1074/jbc.273.37.24239
Jiang, X., Xing, H., Wu, J., Du, R., Liu, H., Chen, J., et al. (2017). Prognostic value of thyroid hormones in acute ischemic stroke – a meta analysis. Sci. Rep. 7:16256. doi: 10.1038/s41598-017-16564-2
Jin, K., Minami, M., Lan, J. Q., Mao, X. O., Batteur, S., Simon, R. P., et al. (2001). Neurogenesis in dentate subgranular zone and rostral subventricular zone after focal cerebral ischemia in the rat. Proc. Natl. Acad. Sci. U.S.A. 98, 4710–4715. doi: 10.1073/pnas.081011098
Jin, K., Wang, X., Xie, L., Mao, X. O., and Greenberg, D. A. (2010). Transgenic ablation of doublecortin-expressing cells suppresses adult neurogenesis and worsens stroke outcome in mice. Proc. Natl. Acad. Sci. U.S.A. 107, 7993–7998. doi: 10.1073/pnas.1000154107
Kapoor, R., Desouza, L. A., Nanavaty, I. N., Kernie, S. G., and Vaidya, V. A. (2012). Thyroid hormone accelerates the differentiation of adult hippocampal progenitors. J. Neuroendocrinol. 24, 1259–1271. doi: 10.1111/j.1365-2826.2012.02329.x
Kapoor, R., Fanibunda, S. E., Desouza, L. A., Guha, S. K., and Vaidya, V. A. (2015). Perspectives on thyroid hormone action in adult neurogenesis. J. Neurochem. 133, 599–616. doi: 10.1111/jnc.13093
Kapoor, R., Ghosh, H., Nordstrom, K., Vennstrom, B., and Vaidya, V. A. (2011). Loss of thyroid hormone receptor beta is associated with increased progenitor proliferation and NeuroD positive cell number in the adult hippocampus. Neurosci. Lett. 487, 199–203. doi: 10.1016/j.neulet.2010.10.022
Kapoor, R., van Hogerlinden, M., Wallis, K., Ghosh, H., Nordstrom, K., Vennstrom, B., et al. (2010). Unliganded thyroid hormone receptor α1 impairs adult hippocampal neurogenesis. FASEB J. 24, 4793–4805. doi: 10.1096/fj.10-161802
Kelly, T., and Lieberman, D. Z. (2009). The use of triiodothyronine as an augmentation agent in treatment-resistant bipolar II and bipolar disorder NOS. J. Affect. Disord. 116, 222–226. doi: 10.1016/j.jad.2008.12.010
Kempermann, G., Song, H., and Gage, F. H. (2015). Neurogenesis in the adult hippocampus. Cold Spring Harb. Perspect. Biol. 7:a018812. doi: 10.1101/cshperspect.a018812
Keshavarz, S., and Dehghani, G. A. (2017). Cerebral Ischemia/reperfusion injury in the hyperthyroid rat. Iran. J. Med. Sci. 42, 48–56.
Kimura, H., Yoshikawa, M., Matsuda, R., Toriumi, H., Nishimura, F., Hirabayashi, H., et al. (2005). Transplantation of embryonic stem cell-derived neural stem cells for spinal cord injury in adult mice. Neurol. Res. 27, 812–819. doi: 10.1179/016164105X63629
Kinne, A., Schülein, R., and Krause, G. (2011). Primary and secondary thyroid hormone transporters. Thyroid Res. 4:S7. doi: 10.1186/1756-6614-4-S1-S7
Kishimoto, N., Shimizu, K., and Sawamoto, K. (2012). Neuronal regeneration in a zebrafish model of adult brain injury. Dis. Model. Mech. 5, 200–209. doi: 10.1242/dmm.007336
Koenning, M., Jackson, S., Hay, C. M., Faux, C., Kilpatrick, T. J., Willingham, M., et al. (2012). Myelin gene regulatory factor is required for maintenance of myelin and mature oligodendrocyte identity in the adult CNS. J. Neurosci. 32, 12528–12542. doi: 10.1523/JNEUROSCI.1069-12.2012
Köhrle, J. (2019). The colorful diversity of thyroid hormone metabolites. Eur. Thyroid J. 8, 115–129. doi: 10.1159/000497141
Kopras, E., Potluri, V., Bermudez, M.-L., Williams, K., Belcher, S., and Kasper, S. (2014). Actions of endocrine-disrupting chemicals on stem/progenitor cells during development and disease. Endocr. Relat. Cancer 21, T1–T12. doi: 10.1530/ERC-13-0360
Kozareva, D. A., Cryan, J. F., and Nolan, Y. M. (2019). Born this way: hippocampal neurogenesis across the lifespan. Aging Cell 18:e13007. doi: 10.1111/acel.13007
Krieger, T. G., Moran, C. M., Frangini, A., Visser, W. E., Schoenmakers, E., Muntoni, F., et al. (2019). Mutations in thyroid hormone receptor α1 cause premature neurogenesis and progenitor cell depletion in human cortical development. Proc. Natl. Acad. Sci. U.S.A. 116, 22754–22763. doi: 10.1073/pnas.1908762116
Kutzelnigg, A., Faber-Rod, J. C., Bauer, J., Lucchinetti, C. F., Sorensen, P. S., Laursen, H., et al. (2007). Widespread demyelination in the cerebellar cortex in multiple sclerosis. Brain Pathol. 17, 38–44. doi: 10.1111/j.1750-3639.2006.00041.x
Kutzelnigg, A., Lucchinetti, C. F., Stadelmann, C., Brück, W., Rauschka, H., Bergmann, M., et al. (2005). Cortical demyelination and diffuse white matter injury in multiple sclerosis. Brain 128, 2705–2712. doi: 10.1093/brain/awh641
Lamba, N., Liu, C., Zaidi, H., Broekman, M. L. D. L. D., Simjian, T., Shi, C., et al. (2018). A prognostic role for Low tri-iodothyronine syndrome in acute stroke patients: a systematic review and meta-analysis. Clin. Neurol. Neurosurg. 169, 55–63. doi: 10.1016/j.clineuro.2018.03.025
Lange, C., Rost, F., Machate, A., Reinhardt, S., Lesche, M., Weber, A., et al. (2020). Single cell sequencing of radial glia progeny reveals the diversity of newborn neurons in the adult zebrafish brain. Development 147:dev185595. doi: 10.1242/dev.185595
Lemkine, G. F., Mantero, S., Migné, C., Raji, A., Goula, D., Normandie, P., et al. (2002). Preferential transfection of adult mouse neural stem cells and their immediate progeny in vivo with polyethylenimine. Mol. Cell. Neurosci. 19, 165–174. doi: 10.1006/mcne.2001.1084
Lemkine, G. F., Raji, A., Alfama, G., Turque, N., Hassani, Z., Alegria-Prévot, O., et al. (2005). Adult neural stem cell cycling in vivo requires thyroid hormone and its alpha receptor. FASEB J. 19, 863–865. doi: 10.1096/fj.04-2916fje
Leray, E., Moreau, T., Fromont, A., and Edan, G. (2016). Epidemiology of multiple sclerosis. Rev. Neurol. 172, 3–13. doi: 10.1016/j.neurol.2015.10.006
Li, J., Donangelo, I., Abe, K., Scremin, O., Ke, S., Li, F., et al. (2017). Thyroid hormone treatment activates protective pathways in both in vivo and in vitro models of neuronal injury. Mol. Cell. Endocrinol. 452, 120–130. doi: 10.1016/j.mce.2017.05.023
Li, L., Harms, K. M., Ventura, P. B., Lagace, D. C., Eisch, A. J., and Cunningham, L. A. (2010). Focal cerebral ischemia induces a multilineage cytogenic response from adult subventricular zone that is predominantly gliogenic. Glia 58, 1610–1619. doi: 10.1002/glia.21033
Li, X., and Buxbaum, J. N. (2011). Transthyretin and the brain re-visited: Is neuronal synthesis of transthyretin protective in Alzheimer’s disease? Mol. Neurodegener. 6:79. doi: 10.1186/1750-1326-6-79
Li, X., Masliah, E., Reixach, N., and Buxbaum, J. N. (2011). Neuronal production of transthyretin in human and murine Alzheimer’s disease: Is it protective? J. Neurosci. 31, 12483–12490. doi: 10.1523/JNEUROSCI.2417-11.2011
Lie, D.-C., Colamarino, S. A., Song, H.-J., Désiré, L., Mira, H., Consiglio, A., et al. (2005). Wnt signalling regulates adult hippocampal neurogenesis. Nature 437, 1370–1375. doi: 10.1038/nature04108
Lim, D. A., and Alvarez-Buylla, A. (2016). The Adult Ventricular–Subventricular Zone (V-SVZ) and Olfactory Bulb (OB) Neurogenesis. Cold Spring Harb. Perspect. Biol. 8:a018820. doi: 10.1101/cshperspect.a018820
Lima, S. A., and Gomes-Leal, W. (2019). Neurogenesis in the hippocampus of adult humans: controversy “fixed” at last. Neural Regen. Res. 14, 1917–1918. doi: 10.4103/1673-5374.259616
Lindvall, O., and Kokaia, Z. (2015). Neurogenesis following stroke affecting the adult brain. Cold Spring Harb. Perspect. Biol. 7:a019034. doi: 10.1101/cshperspect.a019034
Liu, H., Yan, W., and Xu, G. (2014). Thyroid hormone replacement for nephrotic syndrome patients with euthyroid sick syndrome: a meta-analysis. Ren. Fail. 36, 1360–1365. doi: 10.3109/0886022X.2014.949559
Liu, S., Xiao, Z., Li, X., Zhao, Y., Wu, X., Han, J., et al. (2019). Vascular endothelial growth factor activates neural stem cells through epidermal growth factor receptor signal after spinal cord injury. CNS Neurosci. Ther. 25, 375–385. doi: 10.1111/cns.13056
López-Juárez, A., Remaud, S., Hassani, Z., Jolivet, P., Pierre Simons, J., Sontag, T., et al. (2012). Thyroid hormone signaling acts as a neurogenic switch by repressing Sox2 in the adult neural stem cell niche. Cell Stem Cell 10, 531–543. doi: 10.1016/j.stem.2012.04.008
Ludwin, S. K. (1978). Central nervous system demyelination and remyelination in the mouse: an ultrastructural study of cuprizone toxicity. Lab. Invest. 39, 597–612.
Luo, L., and Stopa, E. G. (2004). Thyrotropin releasing hormone inhibits tau phosphorylation by dual signaling pathways in hippocampal neurons. J. Alzheimers Dis. 6, 527–536. doi: 10.3233/JAD-2004-6510
Luo, L., Yano, N., Mao, Q., Jackson, I. M. D., and Stopa, E. G. (2002). Thyrotropin releasing hormone (TRH) in the hippocampus of Alzheimer patients. J. Alzheimers Dis. 4, 97–103. doi: 10.3233/JAD-2002-4204
Luongo, C., Dentice, M., and Salvatore, D. (2019). Deiodinases and their intricate role in thyroid hormone homeostasis. Nat. Rev. Endocrinol. 15, 479–488. doi: 10.1038/s41574-019-0218-2
Maire, C. L., Wegener, A., Kerninon, C., and Nait Oumesmar, B. (2010). Gain-of-Function of Olig transcription factors enhances oligodendrogenesis and Myelination. Stem Cells 28, 1611–1622. doi: 10.1002/stem.480
Marti-Fabregas, J., Romaguera-Ros, M., Gomez-Pinedo, U., Martinez-Ramirez, S., Jimenez-Xarrie, E., Marin, R., et al. (2010). Proliferation in the human ipsilateral subventricular zone after ischemic stroke. Neurology 74, 357–365. doi: 10.1212/WNL.0b013e3181cbccec
Mayerl, S., Heuer, H., and Ffrench-Constant, C. (2020). Hippocampal neurogenesis requires cell-autonomous thyroid hormone signaling. Stem Cell Rep. 14, 845–860. doi: 10.1016/j.stemcr.2020.03.014
Mayerl, S., Müller, J., Bauer, R., Richert, S., Kassmann, C. M., Darras, V. M., et al. (2014). Transporters MCT8 and OATP1C1 maintain murine brain thyroid hormone homeostasis. J. Clin. Invest. 124, 1987–1999. doi: 10.1172/JCI70324
McAninch, E. A., Rajan, K. B., Evans, D. A., Jo, S., Chaker, L., Peeters, R. P., et al. (2018). A Common DIO2 polymorphism and Alzheimer disease dementia in African and European Americans. J. Clin. Endocrinol. Metab. 103, 1818–1826. doi: 10.1210/jc.2017-01196
McKenzie, I. A., Ohayon, D., Li, H., de Faria, J. P., Emery, B., Tohyama, K., et al. (2014). Motor skill learning requires active central myelination. Science 346, 318–322. doi: 10.1126/science.1254960
McShane, R. H. (2001). Anosmia in dementia is associated with Lewy bodies rather than Alzheimer’s pathology. J. Neurol. Neurosurg. Psychiatry 70, 739–743. doi: 10.1136/jnnp.70.6.739
Mdzinarishvili, A., Sutariya, V., Talasila, P. K., Geldenhuys, W. J., and Sadana, P. (2013). Engineering triiodothyronine (T3) nanoparticle for use in ischemic brain stroke. Drug Deliv. Transl. Res. 3, 309–317. doi: 10.1007/s13346-012-0117-8
Meinig, J. M., Ferrara, S. J., Banerji, T., Banerji, T., Sanford-Crane, H. S., Bourdette, D., et al. (2019). Structure–activity relationships of central nervous system penetration by fatty acid amide hydrolase (FAAH)-targeted thyromimetic prodrugs. ACS Med. Chem. Lett. 10, 111–116. doi: 10.1021/acsmedchemlett.8b00501
Meletis, K., Barnabé-Heider, F., Carlén, M., Evergren, E., Tomilin, N., Shupliakov, O., et al. (2008). Spinal cord injury reveals multilineage differentiation of ependymal cells. PLoS Biol. 6:e182. doi: 10.1371/journal.pbio.0060182
Mellow, A. M., Sunderland, T., Cohen, R. M., Lawlor, B. A., Hill, J. L., Newhouse, P. A., et al. (1989). Acute effects of high-dose thyrotropin releasing hormone infusions in Alzheimer’s disease. Psychopharmacology 98, 403–407. doi: 10.1007/BF00451695
Menn, B., Garcia-Verdugo, J. M., Yaschine, C., Gonzalez-Perez, O., Rowitch, D., and Alvarez-Buylla, A. (2006). Origin of oligodendrocytes in the subventricular zone of the adult brain. J. Neurosci. 26, 7907–7918. doi: 10.1523/JNEUROSCI.1299-06.2006
Michailidou, I., de Vries, H. E., Hol, E. M., and van Strien, M. E. (2014). Activation of endogenous neural stem cells for multiple sclerosis therapy. Front. Neurosci. 8:454. doi: 10.3389/fnins.2014.00454
Mirzadeh, Z., Merkle, F. T., Soriano-Navarro, M., Garcia-Verdugo, J. M., and Alvarez-Buylla, A. (2008). neural stem cells confer unique pinwheel architecture to the ventricular surface in neurogenic regions of the adult brain. Cell Stem Cell 3, 265–278. doi: 10.1016/j.stem.2008.07.004
Mitsios, N., Gaffney, J., Krupinski, J., Mathias, R., Wang, Q., Hayward, S., et al. (2007). Expression of signaling molecules associated with apoptosis in human ischemic stroke tissue. Cell Biochem. Biophys. 47, 73–85. doi: 10.1385/cbb:47:1:73
Mizrak, D., Levitin, H. M., Delgado, A. C., Crotet, V., Yuan, J., Chaker, Z., et al. (2019). Single-cell analysis of regional differences in adult V-SVZ neural stem cell lineages. Cell Rep. 26, 394–406.e5. doi: 10.1016/j.celrep.2018.12.044
Modesto, T., Tiemeier, H., Peeters, R. P., Jaddoe, V. W. V., Hofman, A., Verhulst, F. C., et al. (2015). Maternal mild thyroid hormone insufficiency in early pregnancy and attention-deficit/hyperactivity disorder symptoms in children. JAMA Pediatr. 169, 838–845. doi: 10.1001/jamapediatrics.2015.0498
Mokhtari, T., Akbari, M., Malek, F., Kashani, I. R., Rastegar, T., Noorbakhsh, F., et al. (2017). Improvement of memory and learning by intracerebroventricular microinjection of T3 in rat model of ischemic brain stroke mediated by upregulation of BDNF and GDNF in CA1 hippocampal region. DARU J. Pharm. Sci. 25:4. doi: 10.1186/s40199-017-0169-x
Mokhtari, T., Mahahakizadeh, S., Aligholi, H., Ijaz, S., Noori, L., and Hassanzadeh, G. (2020). Triiodothyronine improves morphology and upregulates seladin-1 of neurospheres extracted from subventricular zone in streptozotocin-induced rat model of Alzheimer’s disease. J. Contemp. Med. Sci. 6, 32–38. doi: 10.22317/jcms.02202007
Montero-Pedrazuela, A., Venero, C., Lavado-Autric, R., Fernández-Lamo, I., García-Verdugo, J. M., Bernal, J., et al. (2006). Modulation of adult hippocampal neurogenesis by thyroid hormones: implications in depressive-like behavior. Mol. Psychiatry 11, 361–371. doi: 10.1038/sj.mp.4001802
Moog, N. K., Entringer, S., Heim, C., Wadhwa, P. D., Kathmann, N., and Buss, C. (2017). Influence of maternal thyroid hormones during gestation on fetal brain development. Neuroscience 342, 68–100. doi: 10.1016/j.neuroscience.2015.09.070
Moreno-Jiménez, E. P., Flor-García, M., Terreros-Roncal, J., Rábano, A., Cafini, F., Pallas-Bazarra, N., et al. (2019). Adult hippocampal neurogenesis is abundant in neurologically healthy subjects and drops sharply in patients with Alzheimer’s disease. Nat. Med. 25, 554–560. doi: 10.1038/s41591-019-0375-9
Moreno-Manzano, V., Rodríguez-Jiménez, F. J., García-Roselló, M., Laínez, S., Erceg, S., Calvo, M. T., et al. (2009). Activated spinal cord ependymal stem cells rescue neurological function. Stem Cells 27, 733–743. doi: 10.1002/stem.24
Morris, J. K., Vidoni, E. D., Johnson, D. K., Van Sciver, A., Mahnken, J. D., Honea, R. A., et al. (2017). Aerobic exercise for Alzheimer’s disease: a randomized controlled pilot trial. PLoS One 12:e0170547. doi: 10.1371/journal.pone.0170547
Morte, B., and Bernal, J. (2014). Thyroid hormone action: astrocyte-neuron communication. Front. Endocrinol. 5:82. doi: 10.3389/fendo.2014.00082
Mourouzis, I., Politi, E., and Pantos, C. (2013). Thyroid hormone and tissue repair: New tricks for an old hormone? J. Thyroid Res. 2013, 1–5. doi: 10.1155/2013/312104
Nait-Oumesmar, B., Picard-Riera, N., Kerninon, C., Decker, L., Seilhean, D., Hoglinger, G. U., et al. (2007). Activation of the subventricular zone in multiple sclerosis: evidence for early glial progenitors. Proc. Natl. Acad. Sci. U.S.A. 104, 4694–4699. doi: 10.1073/pnas.0606835104
Nemirovich-Danchenko, N. M., and Khodanovich, M. Y. (2019). New neurons in the post-ischemic and injured brain: Migrating or resident? Front. Neurosci. 13:588. doi: 10.3389/fnins.2019.00588
Neumann, B., Baror, R., Zhao, C., Segel, M., Dietmann, S., Rawji, K. S., et al. (2019). Metformin restores CNS remyelination capacity by rejuvenating aged stem cells. Cell Stem Cell 25, 473–485.e8. doi: 10.1016/j.stem.2019.08.015
Niu, H., Álvarez-Álvarez, I., Guillén-Grima, F., and Aguinaga-Ontoso, I. (2017). Prevalence and incidence of Alzheimer’s disease in Europe: a meta-analysis. Neurología 32, 523–532. doi: 10.1016/j.nrl.2016.02.016
Obernier, K., and Alvarez-Buylla, A. (2019). Neural stem cells: origin, heterogeneity and regulation in the adult mammalian brain. Development 146:dev156059. doi: 10.1242/dev.156059
Ogai, K., Nakatani, K., Hisano, S., Sugitani, K., Koriyama, Y., and Kato, S. (2014). Function of Sox2 in ependymal cells of lesioned spinal cords in adult zebrafish. Neurosci. Res. 88, 84–87. doi: 10.1016/j.neures.2014.07.010
Oppenheim, R. W. (2019). Adult hippocampal neurogenesis in mammals (and humans): the death of a central dogma in neuroscience and its replacement by a new dogma. Dev. Neurobiol. 79, 268–280. doi: 10.1002/dneu.22674
O’Shea, T. M., Burda, J. E., and Sofroniew, M. V. (2017). Cell biology of spinal cord injury and repair. J. Clin. Invest. 127, 3259–3270. doi: 10.1172/JCI90608
Parmentier, T., and Sienaert, P. (2018). The use of triiodothyronine (T3) in the treatment of bipolar depression: a review of the literature. J. Affect. Disord. 229, 410–414. doi: 10.1016/j.jad.2017.12.071
Patrikios, P., Stadelmann, C., Kutzelnigg, A., Rauschka, H., Schmidbauer, M., Laursen, H., et al. (2006). Remyelination is extensive in a subset of multiple sclerosis patients. Brain 129, 3165–3172. doi: 10.1093/brain/awl217
Peri, A., and Serio, M. (2008). Neuroprotective effects of the Alzheimer’s disease-related gene seladin-1. J. Mol. Endocrinol. 41, 251–261. doi: 10.1677/JME-08-0071
Picard-Riera, N., Decker, L., Delarasse, C., Goude, K., Nait-Oumesmar, B., Liblau, R., et al. (2002). Experimental autoimmune encephalomyelitis mobilizes neural progenitors from the subventricular zone to undergo oligodendrogenesis in adult mice. Proc. Natl. Acad. Sci. U.S.A. 99, 13211–13216. doi: 10.1073/pnas.192314199
Picard-Riera, N., Nait-Oumesmar, B., and Baron-Van Evercooren, A. (2004). Endogenous adult neural stem cells: limits and potential to repair the injured central nervous system. J. Neurosci. Res. 76, 223–231. doi: 10.1002/jnr.20040
Planchez, B., Surget, A., and Belzung, C. (2020). Adult hippocampal neurogenesis and antidepressants effects. Curr. Opin. Pharmacol. 50, 88–95. doi: 10.1016/j.coph.2019.11.009
Ponti, G., Farinetti, A., Marraudino, M., Panzica, G., and Gotti, S. (2018). Sex steroids and adult neurogenesis in the ventricular-subventricular zone. Front. Endocrinol. 9:156. doi: 10.3389/fendo.2018.00156
Porta, M., and Vandenberg, L. N. (2019). There are good clinical, scientific, and social reasons to strengthen links between biomedical and environmental research. J. Clin. Epidemiol. 111, 124–126. doi: 10.1016/j.jclinepi.2019.03.009
Praet, J., Guglielmetti, C., Berneman, Z., Van der Linden, A., and Ponsaerts, P. (2014). Cellular and molecular neuropathology of the cuprizone mouse model: clinical relevance for multiple sclerosis. Neurosci. Biobehav. Rev. 47, 485–505. doi: 10.1016/j.neubiorev.2014.10.004
Prieto-Almeida, F., Panveloski-Costa, A. C., Crunfli, F., da Silva Teixeira, S., Nunes, M. T., Torrão, A., et al. (2018). Thyroid hormone improves insulin signaling and reduces the activation of neurodegenerative pathway in the hippocampus of diabetic adult male rats. Life Sci. 192, 253–258. doi: 10.1016/j.lfs.2017.11.013
Quinlan, P., Horvath, A., Wallin, A., and Svensson, J. (2019). Low serum concentration of free triiodothyronine (FT3) is associated with increased risk of Alzheimer’s disease. Psychoneuroendocrinology 99, 112–119. doi: 10.1016/j.psyneuen.2018.09.002
Quiñones-Hinojosa, A., Sanai, N., Soriano-Navarro, M., Gonzalez-Perez, O., Mirzadeh, Z., Gil-Perotin, S., et al. (2006). Cellular composition and cytoarchitecture of the adult human subventricular zone: a niche of neural stem cells. J. Comp. Neurol. 494, 415–434. doi: 10.1002/cne.20798
Rajmohan, R., and Reddy, P. H. (2017). Amyloid-beta and phosphorylated Tau accumulations cause abnormalities at synapses of Alzheimer’s disease neurons. J. Alzheimers Dis. 57, 975–999. doi: 10.3233/JAD-160612
Rastogi, L., Godbole, M. M., Sinha, R. A., and Pradhan, S. (2018). Reverse triiodothyronine (rT3) attenuates ischemia-reperfusion injury. Biochem. Biophys. Res. Commun. 506, 597–603. doi: 10.1016/j.bbrc.2018.10.031
Reich, D. S., Lucchinetti, C. F., and Calabresi, P. A. (2018). Multiple sclerosis. N. Engl. J. Med. 378, 169–180. doi: 10.1056/NEJMra1401483
Remaud, S., Gothié, J.-D., Morvan-Dubois, G., and Demeneix, B. A. (2014). Thyroid hormone signaling and adult neurogenesis in mammals. Front. Endocrinol 5:62. doi: 10.3389/fendo.2014.00062
Remaud, S., Ortiz, F. C., Perret-Jeanneret, M., Aigrot, M.-S. S., Gothié, J.-D. D., Fekete, C., et al. (2017). Transient hypothyroidism favors oligodendrocyte generation providing functional remyelination in the adult mouse brain. eLife 6:e29996. doi: 10.7554/eLife.29996
Richardson, S. J., Wijayagunaratne, R. C., D’Souza, D. G., Darras, V. M., and Van Herck, S. L. J. (2015). Transport of thyroid hormones via the choroid plexus into the brain: the roles of transthyretin and thyroid hormone transmembrane transporters. Front. Neurosci. 9:66. doi: 10.3389/fnins.2015.00066
Ritzel, R. M., Lai, Y.-J. J., Crapser, J. D., Patel, A. R., Schrecengost, A., Grenier, J. M., et al. (2018). Aging alters the immunological response to ischemic stroke. Acta Neuropathol. 136, 89–110. doi: 10.1007/s00401-018-1859-2
Roberts, L. M., Woodford, K., Zhou, M., Black, D. S., Haggerty, J. E., Tate, E. H., et al. (2008). Expression of the thyroid hormone transporters monocarboxylate transporter-8 (SLC16A2) and organic ion transporter-14 (SLCO1C1) at the blood-brain barrier. Endocrinology 149, 6251–6261. doi: 10.1210/en.2008-0378
Rodríguez, J. J., Jones, V. C., and Verkhratsky, A. (2009). Impaired cell proliferation in the subventricular zone in an Alzheimer’s disease model. Neuroreport 20, 907–912. doi: 10.1097/WNR.0b013e32832be77d
Rust, R., and Kaiser, J. (2017). Insights into the dual role of inflammation after spinal cord injury. J. Neurosci. 37, 4658–4660. doi: 10.1523/JNEUROSCI.0498-17.2017
Sabbaghziarani, F., Mortezaee, K., Akbari, M., Kashani, I. R., Soleimani, M., Hassanzadeh, G., et al. (2017). Stimulation of neurotrophic factors and inhibition of proinflammatory cytokines by exogenous application of triiodothyronine in the rat model of ischemic stroke. Cell Biochem. Funct. 35, 50–55. doi: 10.1002/cbf.3244
Sanai, N., Tramontin, A. D., Quiñones-Hinojosa, A., Barbaro, N. M., Gupta, N., Kunwar, S., et al. (2004). Unique astrocyte ribbon in adult human brain contains neural stem cells but lacks chain migration. Nature 427, 740–744. doi: 10.1038/nature02301
Sánchez-Huerta, K., García-Martínez, Y., Vergara, P., Segovia, J., and Pacheco-Rosado, J. (2016). Thyroid hormones are essential to preserve non-proliferative cells of adult neurogenesis of the dentate gyrus. Mol. Cell. Neurosci. 76, 1–10. doi: 10.1016/j.mcn.2016.08.001
Sankavaram, S. R., Hakim, R., Covacu, R., Frostell, A., Neumann, S., Svensson, M., et al. (2019). Adult neural progenitor cells transplanted into spinal cord injury differentiate into oligodendrocytes, enhance myelination, and contribute to recovery. Stem Cell Reports 12, 950–966. doi: 10.1016/j.stemcr.2019.03.013
Shihabuddin, L. S., Horner, P. J., Ray, J., and Gage, F. H. (2000). Adult spinal cord stem cells generate neurons after transplantation in the adult dentate gyrus. J. Neurosci. 20, 8727–8735. doi: 10.1523/JNEUROSCI.20-23-08727.2000
Shultz, R. B., Wang, Z., Nong, J., Zhang, Z., and Zhong, Y. (2017). Local delivery of thyroid hormone enhances oligodendrogenesis and myelination after spinal cord injury. J. Neural Eng. 14:036014. doi: 10.1088/1741-2552/aa6450
Silva, C. S., Eira, J., Ribeiro, C. A., Oliveira, Â., Sousa, M. M., Cardoso, I., et al. (2017). Transthyretin neuroprotection in Alzheimer’s disease is dependent on proteolysis. Neurobiol. Aging 59, 10–14. doi: 10.1016/j.neurobiolaging.2017.07.002
Silva-Vargas, V., Maldonado-Soto, A. R., Mizrak, D., Codega, P., and Doetsch, F. (2016). Age-dependent niche signals from the choroid plexus regulate adult neural stem cells. Cell Stem Cell 19, 643–652. doi: 10.1016/j.stem.2016.06.013
Silver, J., Schwab, M. E., and Popovich, P. G. (2015). Central nervous system regenerative failure: role of oligodendrocytes, astrocytes, and microglia. Cold Spring Harb. Perspect. Biol. 7:a020602. doi: 10.1101/cshperspect.a020602
Silvestroff, L., Bartucci, S., Pasquini, J., and Franco, P. (2012). Cuprizone-induced demyelination in the rat cerebral cortex and thyroid hormone effects on cortical remyelination. Exp. Neurol. 235, 357–367. doi: 10.1016/j.expneurol.2012.02.018
Slack, J. M. (2017). Animal regeneration: Ancestral character or evolutionary novelty? EMBO Rep. 18, 1497–1508. doi: 10.15252/embr.201643795
Sorrells, S. F., Paredes, M. F., Cebrian-Silla, A., Sandoval, K., Qi, D., Kelley, K. W., et al. (2018). Human hippocampal neurogenesis drops sharply in children to undetectable levels in adults. Nature 555, 377–381. doi: 10.1038/nature25975
Steiner, E., Tata, M., and Frisén, J. (2019). A fresh look at adult neurogenesis. Nat. Med. 25, 542–543. doi: 10.1038/s41591-019-0408-4
Stenzel, D., Wilsch-Brauninger, M., Wong, F. K., Heuer, H., and Huttner, W. B. (2014). Integrin αvβ3 and thyroid hormones promote expansion of progenitors in embryonic neocortex. Development 141, 795–806. doi: 10.1242/dev.101907
Su, Z., Niu, W., Liu, M.-L., Zou, Y., and Zhang, C.-L. (2014). In vivo conversion of astrocytes to neurons in the injured adult spinal cord. Nat. Commun. 5:3338. doi: 10.1038/ncomms4338
Talhada, D., Feiteiro, J., Costa, A. R., Talhada, T., Cairrão, E., Wieloch, T., et al. (2019). Triiodothyronine modulates neuronal plasticity mechanisms to enhance functional outcome after stroke. Acta Neuropathol. Commun. 7:216. doi: 10.1186/s40478-019-0866-4
Tatebayashi, K., Tanaka, Y., Nakano-Doi, A., Sakuma, R., Kamachi, S., Shirakawa, M., et al. (2017). Identification of multipotent stem cells in human brain tissue following stroke. Stem Cells Dev. 26, 787–797. doi: 10.1089/scd.2016.0334
Tincer, G., Mashkaryan, V., Bhattarai, P., and Kizil, C. (2016). Neural stem/progenitor cells in Alzheimer’s disease. Yale J. Biol. Med. 89, 23–35. doi: 10.1038/s41380-018-0036-2
Tobin, M. K., Musaraca, K., Disouky, A., Shetti, A., Bheri, A., Honer, W. G., et al. (2019). Human hippocampal neurogenesis persists in aged adults and Alzheimer’s disease patients. Cell Stem Cell 24, 974–982.e3. doi: 10.1016/j.stem.2019.05.003
Tsintou, M., Dalamagkas, K., and Makris, N. (2020). Taking central nervous system regenerative therapies to the clinic: curing rodents versus nonhuman primates versus humans. Neural Regen. Res. 15, 425–437. doi: 10.4103/1673-5374.266048
van der Meer, T. P., Artacho-Cordón, F., Swaab, D. F., Struik, D., Makris, K. C., Wolffenbuttel, B. H. R., et al. (2017). Distribution of non-persistent endocrine disruptors in two different regions of the human brain. Int. J. Environ. Res. Public Health 14:1059. doi: 10.3390/ijerph14091059
Vancamp, P., Demeneix, B. A., and Remaud, S. (2020). Monocarboxylate transporter 8 deficiency: Delayed or permanent hypomyelination? Front. Endocrinol. 11:283. doi: 10.3389/fendo.2020.00283
Vancamp, P., Gothié, J.-D. D., Luongo, C., Sébillot, A., Le Blay, K., Butruille, L., et al. (2019). Gender-specific effects of transthyretin on neural stem cell fate in the subventricular zone of the adult mouse. Sci. Rep. 9:19689. doi: 10.1038/s41598-019-56156-w
Vanhunsel, S., Beckers, A., and Moons, L. (2020). Designing neuroreparative strategies using aged regenerating animal models. Ageing Res. Rev. 62:101086. doi: 10.1016/j.arr.2020.101086
Vatine, G. D., Al-Ahmad, A., Barriga, B. K., Svendsen, S., Salim, A., Garcia, L., et al. (2017). Modeling psychomotor retardation using iPSCs from MCT8-deficient patients indicates a prominent role for the blood-brain barrier. Cell Stem Cell 20, 831–843.e5. doi: 10.1016/j.stem.2017.04.002
Villeda, S. A., Luo, J., Mosher, K. I., Zou, B., Britschgi, M., Bieri, G., et al. (2011). The ageing systemic milieu negatively regulates neurogenesis and cognitive function. Nature 477, 90–94. doi: 10.1038/nature10357
Vismara, I., Papa, S., Rossi, F., Forloni, G., and Veglianese, P. (2017). Current options for cell therapy in spinal cord injury. Trends Mol. Med. 23, 831–849. doi: 10.1016/j.molmed.2017.07.005
Wallin, M. T., Culpepper, W. J., Campbell, J. D., Nelson, L. M., Langer-Gould, A., Marrie, R. A., et al. (2019). The prevalence of MS in the United States. Neurology 92, e1029–e1040. doi: 10.1212/WNL.0000000000007035
Wallis, K., Dudazy, S., van Hogerlinden, M., Nordström, K., Mittag, J., and Vennstroöm, B. (2010). The thyroid hormone receptor α1 protein is expressed in embryonic postmitotic neurons and persists in most adult neurons. Mol. Endocrinol. 24, 1904–1916. doi: 10.1210/me.2010-0175
Weil, Z. M., Norman, G. J., DeVries, A. C., and Nelson, R. J. (2008). The injured nervous system: a darwinian perspective. Prog. Neurobiol. 86, 48–59. doi: 10.1016/j.pneurobio.2008.06.001
Weissleder, C., Fung, S. J., Wong, M. W., Barry, G., Double, K. L., Halliday, G. M., et al. (2016). Decline in proliferation and immature neuron markers in the human subependymal zone during aging: relationship to EGF- and FGF-related transcripts. Front. Aging Neurosci. 8:274. doi: 10.3389/fnagi.2016.00274
Williamson, J. M., and Lyons, D. A. (2018). Myelin dynamics throughout life: An ever-changing landscape? Front. Cell. Neurosci. 12:424. doi: 10.3389/fncel.2018.00424
Wilpert, N.-M., Krueger, M., Opitz, R., Sebinger, D., Paisdzior, S., Mages, B., et al. (2020). Spatiotemporal changes of cerebral monocarboxylate transporter 8 expression. Thyroid. doi: 10.1089/thy.2019.0544 [Epub ahead of print].
Winocur, G., Wojtowicz, J. M., Sekeres, M., Snyder, J. S., and Wang, S. (2006). Inhibition of neurogenesis interferes with hippocampus-dependent memory function. Hippocampus 16, 296–304. doi: 10.1002/hipo.20163
Wollenweber, F. A., Zietemann, V., Gschwendtner, A., Opherk, C., and Dichgans, M. (2013). Subclinical hyperthyroidism is a risk factor for poor functional outcome after ischemic stroke. Stroke 44, 1446–1448. doi: 10.1161/STROKEAHA.113.000833
Wolswijk, G. (1998). Chronic stage multiple sclerosis lesions contain a relatively quiescent population of oligodendrocyte precursor cells. J. Neurosci. 18, 601–609. doi: 10.1523/JNEUROSCI.18-02-00601.1998
Wooliscroft, L., Altowaijri, G., Hildebrand, A., Samuels, M., Oken, B., Bourdette, D., et al. (2020). Phase I randomized trial of liothyronine for remyelination in multiple sclerosis: a dose-ranging study with assessment of reliability of visual outcomes. Mult. Scler. Relat. Disord. 41:102015. doi: 10.1016/j.msard.2020.102015
Xing, C., Arai, K., Lo, E. H., and Hommel, M. (2012). Pathophysiologic cascades in ischemic stroke. Int. J. Stroke 7, 378–385. doi: 10.1111/j.1747-4949.2012.00839.x
Xing, Y. L., Roth, P. T., Stratton, J. A. S., Chuang, B. H. A., Danne, J., Ellis, S. L., et al. (2014). Adult neural precursor cells from the subventricular zone contribute significantly to oligodendrocyte regeneration and remyelination. J. Neurosci. 34, 14128–14146. doi: 10.1523/JNEUROSCI.3491-13.2014
Young, K. M., Psachoulia, K., Tripathi, R. B., Dunn, S.-J., Cossell, L., Attwell, D., et al. (2013). Oligodendrocyte dynamics in the healthy adult CNS: evidence for myelin remodeling. Neuron 77, 873–885. doi: 10.1016/j.neuron.2013.01.006
Zambusi, A., and Ninkovic, J. (2020). Regeneration of the central nervous system-principles from brain regeneration in adult zebrafish. World J. Stem Cells 12, 8–24. doi: 10.4252/wjsc.v12.i1.8
Zarif, H., Petit-Paitel, A., Heurteaux, C., Chabry, J., and Guyon, A. (2016). TRH modulates glutamatergic synaptic inputs on CA1 neurons of the mouse hippocampus in a biphasic manner. Neuropharmacology 110, 69–81. doi: 10.1016/j.neuropharm.2016.04.004
Zhang, J.-Q. Q., Yang, Q.-Y. Y., Xue, F.-S. S., Zhang, W., Yang, G.-Z. Z., Liao, X., et al. (2018). Preoperative oral thyroid hormones to prevent euthyroid sick syndrome and attenuate myocardial ischemia-reperfusion injury after cardiac surgery with cardiopulmonary bypass in children: a randomized, double-blind, placebo-controlled trial. Medicine 97:e12100. doi: 10.1097/MD.0000000000012100
Zhang, M., Zhan, X. L., Ma, Z. Y., Chen, X. S., Cai, Q. Y., and Yao, Z. X. (2015). Thyroid hormone alleviates demyelination induced by cuprizone through its role in remyelination during the remission period. Exp. Biol. Med. 240, 1183–1196. doi: 10.1177/1535370214565975
Zhang, R., Xue, Y.-Y., Lu, S.-D., Wang, Y., Zhang, L.-M., Huang, Y.-L., et al. (2006). Bcl-2 enhances neurogenesis and inhibits apoptosis of newborn neurons in adult rat brain following a transient middle cerebral artery occlusion. Neurobiol. Dis. 24, 345–356. doi: 10.1016/j.nbd.2006.07.012
Ziabreva, I., Perry, E., Perry, R., Minger, S. L., Ekonomou, A., Przyborski, S., et al. (2006). Altered neurogenesis in Alzheimer’s disease. J. Psychosom. Res. 61, 311–316. doi: 10.1016/j.jpsychores.2006.07.017
Zoeller, R. T., and Rovet, J. (2004). Timing of thyroid hormone action in the developing brain: clinical observations and experimental findings. J. Neuroendocrinol. 16, 809–818. doi: 10.1111/j.1365-2826.2004.01243.x
Keywords: brain and spinal cord injury, neurodegenerative disease, endogenous repair, neural stem cells, thyroid hormone, multiple sclerosis, stroke, Alzheimer’s disease
Citation: Vancamp P, Butruille L, Demeneix BA and Remaud S (2020) Thyroid Hormone and Neural Stem Cells: Repair Potential Following Brain and Spinal Cord Injury. Front. Neurosci. 14:875. doi: 10.3389/fnins.2020.00875
Received: 08 June 2020; Accepted: 28 July 2020;
Published: 26 August 2020.
Edited by:
Jose Angel Morales-Garcia, Center for Biomedical Research on Neurodegenerative Diseases (CIBERNED), SpainReviewed by:
Steven W. Levison, The State University of New Jersey, United StatesVivian Capilla-González, Andalusian Center of Molecular Biology and Regenerative Medicine (CABIMER), Spain
Copyright © 2020 Vancamp, Butruille, Demeneix and Remaud. This is an open-access article distributed under the terms of the Creative Commons Attribution License (CC BY). The use, distribution or reproduction in other forums is permitted, provided the original author(s) and the copyright owner(s) are credited and that the original publication in this journal is cited, in accordance with accepted academic practice. No use, distribution or reproduction is permitted which does not comply with these terms.
*Correspondence: Sylvie Remaud, c3JlbWF1ZEBtbmhuLmZy