- 1The George and Anne Ryan Institute for Neuroscience, The University of Rhode Island, Kingston, RI, United States
- 2Department of Biomedical and Pharmaceutical Sciences, College of Pharmacy, The University of Rhode Island, Kingston, RI, United States
The societal burden of Alzheimer’s disease (AD) is staggering, with current estimates suggesting that 50 million people world-wide have AD. Identification of new therapeutic targets is a critical barrier to the development of disease-modifying therapies. A large body of data implicates vascular pathology and cardiovascular risk factors in the development of AD, indicating that there are likely shared pathological mediators. Inflammation plays a role in both cardiovascular disease and AD, and recent evidence has implicated elements of the coagulation system in the regulation of inflammation. In particular, the multifunctional serine protease thrombin has been found to act as a mediator of vascular dysfunction and inflammation in both the periphery and the central nervous system. In the periphery, thrombin contributes to the development of cardiovascular disease, including atherosclerosis and diabetes, by inducing endothelial dysfunction and related inflammation. In the brain, thrombin has been found to act on endothelial cells of the blood brain barrier, microglia, astrocytes, and neurons in a manner that promotes vascular dysfunction, inflammation, and neurodegeneration. Thrombin is elevated in the AD brain, and thrombin signaling has been linked to both tau and amyloid beta, pathological hallmarks of the disease. In AD mouse models, inhibiting thrombin preserves cognition and endothelial function and reduces neuroinflammation. Evidence linking atrial fibrillation with AD and dementia indicates that anticoagulant therapy may reduce the risk of dementia, with targeting thrombin shown to be particularly effective. It is time for “outside-the-box” thinking about how vascular risk factors, such as atherosclerosis and diabetes, as well as the coagulation and inflammatory pathways interact to promote increased AD risk. In this review, we present evidence that thrombin is a convergence point for AD risk factors and as such that thrombin-based therapeutics could target multiple points of AD pathology, including neurodegeneration, vascular activation, and neuroinflammation. The urgent need for disease-modifying drugs in AD demands new thinking about disease pathogenesis and an exploration of novel drug targets, we propose that thrombin inhibition is an innovative tactic in the therapeutic battle against this devastating disease.
Introduction
The societal burden of Alzheimer’s disease (AD) is staggering, with current estimates suggesting that 5.8 million Americans and 50 million people world-wide have AD (Alzheimer’s Association, 2019). Identification of new therapeutic targets is a critical barrier to our ability to develop a disease-modifying therapy for this devastating disease. A large body of data implicates vascular pathology and cardiovascular risk factors, such as atherosclerosis and diabetes, in the development of AD. Though the mechanisms whereby these risk factors contribute to pathological processes in the AD brain have not been defined fully, it has been suggested that inflammation plays a key role in both cardiovascular disease and AD (Grammas, 2011). It is clear that a better understanding of the relationship(s) among cardiovascular risk factors, inflammation, and neurodegeneration has the potential to reveal novel therapeutic targets in the battle against AD.
Recently, a number of laboratories have provided evidence that certain elements of the coagulation cascade may initiate and/or support inflammation in the brain (Davalos and Akassoglou, 2012; De Luca et al., 2017; Göbel et al., 2018). The proinflammatory properties of the protein fibrin have attracted particular attention [reviewed in Cortes-Canteli et al. (2012), Petersen et al. (2018)]. Fibrin increases expression of a number of inflammatory and oxidative mediators, activates glial cells, and disrupts the blood-brain barrier (BBB). Another potentially important protein related to both coagulation and inflammation is thrombin. Thrombin is widely appreciated for its contribution to fibrin formation and platelet aggregation in response to vascular injury. Importantly, thrombin is also a pleiotropic enzyme that is capable of triggering a large and diverse number of cellular events through receptor-mediated activation of protease-activated receptors (PARs) (Coughlin, 2005). Levels of both thrombin and the thrombin receptor PAR-1 are elevated in AD (Sokolova and Reiser, 2008; Krenzlin et al., 2016) and thrombin expression is increased in brain microvessels collected from AD patients (Grammas et al., 2006). As we discuss below, thrombin can act in both a paracrine and autocrine manner to stimulate a noxious feed-forward cycle that likely contributes to neuroinflammation in the AD brain. The evidence summarized herein suggests that thrombin is a convergence point for AD risk factors and that thrombin-based therapeutics might target multiple points of AD pathology, including neurodegeneration, vascular activation, and neuroinflammation. We will present evidence that supports the hypothesis that thrombin could be a heretofore unexplored target for AD therapeutics.
Coagulation and Inflammation: Functionally Linked Processes
Close integration and extensive crosstalk between coagulation and inflammation pathways are critical to the body’s response to injury (Levi and van der Poll, 2005; Esmon, 2008). Both processes utilize numerous bioactive mediators and cellular effectors that interact in a coordinated manner. Inflammatory proteins, such as cytokines, play a central role in the activation of coagulation (Levi and van der Poll, 2005). Meanwhile major coagulation factors, such as tissue factor, fibrinogen, and thrombin, are drivers of inflammation (Jennewein et al., 2011; Davalos and Akassoglou, 2012; Kalz et al., 2014; Witkowski et al., 2016; De Luca et al., 2017). Under normal physiological conditions these intertwined systems work in homeostatic balance, but dysregulation of this crosstalk likely contributes to cellular injury and disease pathogenesis.
Effect of Cytokines on Coagulation
Cytokines, particularly interleukin (IL)-6, tumor necrosis factor-α (TNF-α), and IL-1β, stimulate procoagulant effects both directly and indirectly. These proteins initiate the extrinsic coagulation pathway through up-regulation and activation of tissue factor (Nawroth and Stern, 1986; Witkowski et al., 2016). Blocking tissue factor greatly inhibits inflammation-induced thrombosis, and inhibition of IL-6 specifically blocks tissue factor-dependent thrombin generation (Levi et al., 1997). Cytokines, especially TNF-α, can also initiate inflammation-mediated platelet activation and clumping in the blood (Page et al., 2018). Inflammatory cytokines can inhibit the anticoagulant feedback pathways, resulting in increased thrombin and fibrin production (Levi and van der Poll, 2005). Both TNF-α and IL-1β can reduce activated protein C (APC) via down-regulation of thrombomodulin, an important cofactor for APC’s anti-inflammatory and anti-coagulant activity (Nawroth and Stern, 1986).
Coagulation Factors Drive Inflammation
Tissue factor, the main driver of the extrinsic coagulation pathway, can induce proinflammatory effects. This includes increases in the production of inflammatory cytokines, adhesion molecules, chemokines, and growth factors (Witkowski et al., 2016). These proinflammatory effects are largely mediated through tissue factor-activation of the protease thrombin (see below). Fibrin is the primary end-product of the coagulation system, but it also has proinflammatory characteristics. Fibrinogen and fibrin both have been shown to induce leukocyte migration, and directly modulate the inflammatory response of both leukocytes and endothelial cells. Fibrin induces the expression of several inflammatory cytokines and chemokines and increases the production of reactive oxygen species (ROS) (Jennewein et al., 2011). Similarly, fibrin has been shown to have extensive proinflammatory effects within the central nervous system (CNS), including activation of glia and disruption of BBB function (Petersen et al., 2018). Fibrinogen activates microglia in a CD11b-dependent manner; this activation is related to perivascular clustering, axonal degeneration, spine elimination, and cognitive impairment in animal models (Davalos et al., 2012; Merlini et al., 2019). Finally, the coagulation and immune systems are directly linked through activation of IL-1α by thrombin (Burzynski et al., 2019).
Thrombin is a Key Mediator of Coagulation and Inflammation Via Proteolytic and Receptor-Mediated Mechanisms
The coagulation cascade consists of the intrinsic and extrinsic pathways, and thrombin is a key mediator in both (Palta et al., 2014). The extrinsic system is activated by tissue factor, which is found in the subendothelial surface and is only introduced to the blood following injury. Tissue factor in the blood complexes with factor VIIIa to initiate the cascade that will eventually lead to the formation of thrombin and the cleavage of fibrinogen to fibrin (Witkowski et al., 2016). The intrinsic system is activated by factor XII, which initiates a cascade that will lead to the production of thrombin via factors X and V (Palta et al., 2014). The activation of thrombin will also activate a positive feedback loop which will continue to drive the generation of thrombin (Palta et al., 2014). Together, these systems work to increase the amount of active thrombin in the blood, which will in turn increase the insoluble fibrin available to form a clot at the site of damage.
As noted above, thrombin is a multifunctional protease that can initiate many cellular events through action at and activation of PARs. PARs are a unique class of G-protein-coupled receptors due to their unusual tethered-ligand mechanism of activation (Coughlin, 2000). Thrombin is responsible for enzymatic cleavage of the PAR N-terminus to expose a tethered ligand that intramolecularly activates the receptor. Activation of PARs by thrombin affects a multitude of functions throughout the body, including the regulation of platelet activation, cell adhesion, cell migration, angiogenesis, and inflammation (Coughlin, 2005; Kalz et al., 2014).
The Vasculature Is a Critical Nexus for the Proinflammatory Actions of Thrombin
Thrombin moderates gene expression through a wide array signaling processes in vascular endothelial cells (Cheranova et al., 2013) and much of thrombin’s proinflammatory activity is likely due to its numerous effects on vascular endothelial cells. Thrombin acts on endothelial cells to stimulate synthesis and release of a large number of diverse bioactive proteins. Thrombin stimulation of human umbilical vein endothelial cells (HUVECs) produces changes in gene expression related to inflammation, apoptosis, and matrix integrity (Okada et al., 2006). Thrombin-treated HUVECs exhibit increased expression of intracellular adhesion molecule-1 (ICAM-1) and vascular cell adhesion molecule-1 (VCAM-1) and a related increase in monocyte adhesion (Kaplanski et al., 1998). In human aortic endothelial cells (HAECs), thrombin and high-glucose co-treatment produces increases in the expression of NADPH oxidase (NOX), inflammatory cytokines, and altered adhesion (Wang et al., 2014). Cultured endothelial cells exposed to thrombin release von Willebrand factor, express P-selectin at the plasma membrane, and produce chemokines thought to trigger the binding of platelets and leukocytes to endothelial surface (Collins et al., 1993). Thrombin can affect vessel diameter, alter endothelial cell shape, and increase permeability of the endothelial monolayer. Endothelial cells can both release thrombin and respond to this protein via functionally active thrombin receptors (PAR-1 and PAR-3). Thrombin causes endothelial activation and enhanced expression and/or release of many proinflammatory proteins including monocyte chemoattractant protein-1 (MCP-1), ICAM-1, IL-1, IL-6, and IL-8, which in turn can injure endothelial cells leading to increased release of thrombin (Colotta et al., 1994; Marin et al., 2001; Rahman et al., 2002; Miho et al., 2005; Okada et al., 2006; Yin et al., 2010; Ishibashi et al., 2014). Thus, thrombin can act in both a paracrine and autocrine manner stimulating a noxious feed-forward cycle.
Thrombin Contributes to the Development of Alzheimer’s Risk Factors: Atherosclerosis and Diabetes
Given thrombin’s effect on endothelial cells, it is not surprising that thrombin has been implicated in the pathogenesis of atherosclerosis. Thrombin has been widely studied for its role in the pathology of atherosclerosis, a progressive and chronic inflammatory vascular disorder (Kalz et al., 2014; Jaberi et al., 2019). A role for thrombin in the development of atherosclerosis is suggested by the observation that elevated levels of thrombin and the thrombin receptor PAR are found around atherosclerotic plaques, and that thrombin formation is correlated with disease severity in coronary atherosclerosis (Borissoff et al., 2012). Thrombin facilitates recruitment of circulating monocytes to atherosclerosis plaques by increasing expression of MCP-1, ICAM-1, and VCAM-1 (Colotta et al., 1994; Minami et al., 2004; Kalz et al., 2014). The importance of thrombin is further highlighted by the finding that thrombomodulin, which inhibits thrombin by binding to it, diminishes thrombin-induced endothelial cell dysfunction in atherosclerosis (Wei et al., 2011). Thrombin produces damage to endothelial cell barriers causing leakiness and associated leukocyte migration (Kalz et al., 2014). Thrombin inhibition has been shown to decrease ROS production, improve endothelial cell and barrier function, and attenuate atherosclerotic plaque formation (Lee et al., 2012).
Thrombin activity can influence onset, progression, and qualitative properties of atherosclerotic plaques. In atherosclerotic models, increases in thrombin have been found to be associated with increases in inflammation, angiogenesis, and cell proliferation (ten Cate, 2012; Kalz et al., 2014). Genetic reduction of thrombin in APOE-/- mice diminished atherosclerosis severity (Borissoff et al., 2013). Studies have further defined thrombin’s role in atherosclerosis by finding reduced atherosclerosis-related pathology in animal models treated with thrombin inhibitors (Wei et al., 2011; Pingel et al., 2014; Preusch et al., 2015; Palekar et al., 2016). On the other hand, genetic manipulations resulting in elevated thrombin levels produce exacerbated atherosclerosis-related pathology (Borissoff et al., 2013). These findings illustrate a causative role for thrombin in the development of atherosclerosis-related pathology and inflammation, largely through thrombin’s endothelial cell-mediated effects.
Hyperglycemia, characteristic of Type 2 diabetes mellitus (T2DM), enhances thrombin generation and promotes a hypercoagulable state and high oxidative stress (Aoki et al., 1996; Undas et al., 2008; Chapman, 2013). Elevated thrombin activity has been linked with endothelial dysfunction in diabetes, including vascular inflammation and increased ROS production in Paneni et al. (2013). One mechanism whereby thrombin promotes diabetic oxidative stress is via calcium-mediated intracellular signaling pathways that regulate the transcription factor KLF14 and PLK1 kinase pathways, resulting in increased ROS production (Hao et al., 2018). Exogenous thrombin treatment has been shown to exacerbate pathology in experimental models of diabetes. In a mouse model of diabetes, thrombin treatment of pericytes results in increased barrier permeability, decreased expression of tight junction proteins, and increased expression of inflammatory cytokines (Machida et al., 2017). Induction of diabetes by streptozotocin (STZ) in mice increases the expression of PAR-1, PAR-3, and PAR-4 in the aorta. STZ-induced diabetic mice show impairment of endothelial function, while the administration of dabigatran etexilate, a direct thrombin inhibitor, significantly attenuates this endothelial dysfunction (Rahadian et al., 2020).
Patients with T2DM show increased blood thrombin levels correlated with the patient’s level of albuminuria, an indicator of cardiovascular morbidity and mortality in patients with T2DM. This suggests that thrombin may play a role in the development of macrovascular disease (Ay et al., 2012). Thrombin-related pathways have also been implicated in diabetic microvascular injury and retinopathy. Samples taken from diabetic retinopathy patients show elevated expression of thrombin and PAR-1; similar trends are found in samples from a rat model of diabetes (Abu El-Asrar et al., 2016). Obesity promotes a chronic inflammatory and hypercoagulable state that drives cardiovascular disease and T2DM. Recent studies have suggested a link between the thrombin/fibrin (ogen) axis and obesity (Kopec et al., 2017). In a high fat animal model, treatment with the direct thrombin inhibitor dabigatran etexilate ameliorates the development of obesity and severity of associated sequelae (Kopec et al., 2014).
Thrombin Causes Vascular Dysfunction and Promotes Inflammation in the Brain
Largely due to its proinflammatory effects on endothelial cells, thrombin has an important role in the pathology of various peripheral vascular diseases, including atherosclerosis and diabetes, as reviewed above. Thrombin may similarly act as a pathological mediator in the CNS, through effects on the endothelial cells of the BBB (Figure 1).
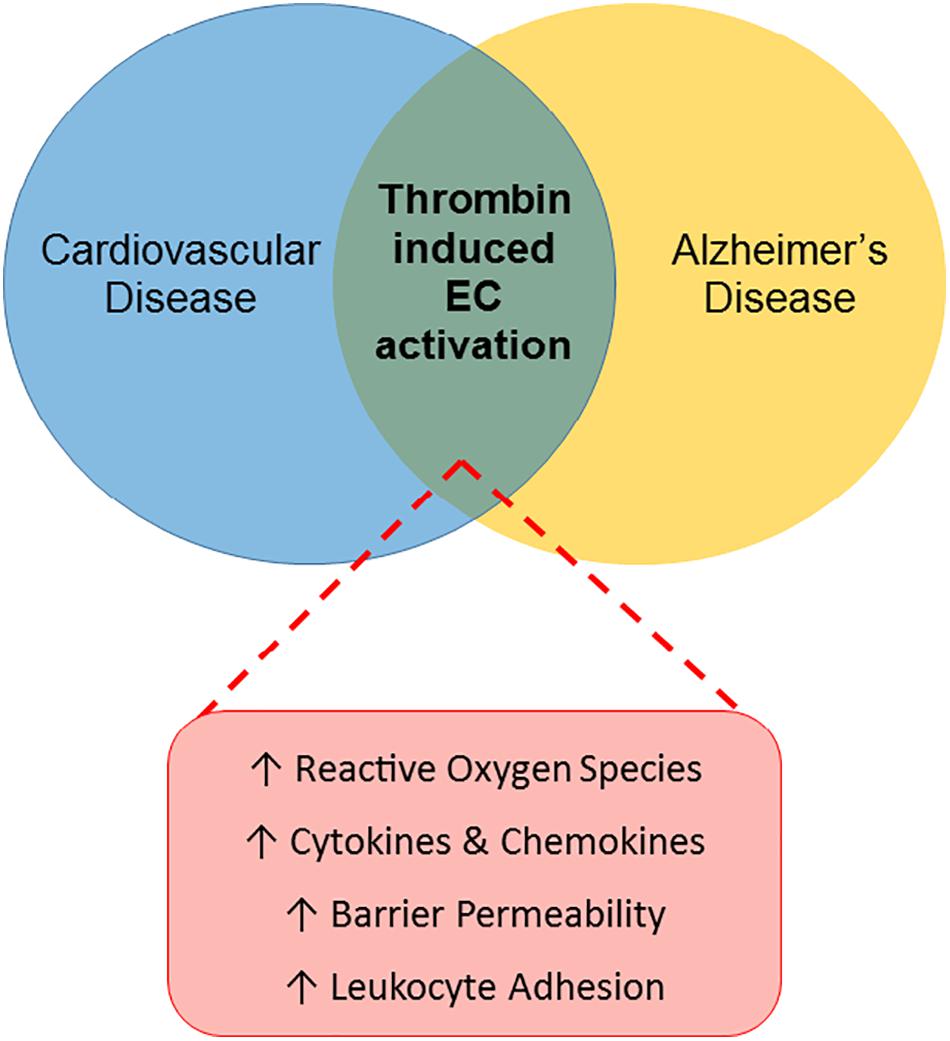
Figure 1. Thrombin-induced endothelial cell activation: a link between CVRFs and AD. The Venn diagram illustrates a proposed role for thrombin-mediated endothelial cell (EC) injury as a common link between cardiovascular disease and AD. Thrombin’s proinflammatory effects on ECs in the periphery, characterized by increased reactive oxygen species, cytokines and chemokines, barrier permeability and leukocyte adhesion, contribute to the pathogenesis of cardiovascular disease. Similarly, thrombin may contribute to the development of AD by causing EC activation in the cerebral microcirculation.
Effects of Thrombin on the BBB
Consistent with its ability to cause endothelial injury in the periphery, thrombin has been found to be involved in instances of damage and dysfunction to the brain endothelial cells of the BBB. Treatment of rat brain endothelial cells causes endothelial dysfunction characterized by increased production of ROS, nitric oxide (NO), inflammatory cytokines, and chemokines (Brailoiu et al., 2017). In human brain endothelial cells, thrombin treatment induces an inflammatory phenotype resulting in increased ICAM-1, VCAM-1, and increased mRNA expression for CXC chemokines (chemotactic for neutrophils) CXCL1 (GRO-alpha), CXCL2 (GRO-beta) CXCL8 (IL-8), and CXCL10 (IP-10) (Alabanza and Bynoe, 2012). Thrombin also increases F-actin stress fibers, disrupts tight junctions, and increases barrier permeability (Brailoiu et al., 2017). More specifically, thrombin has been found to alter barrier permeability by inducing microtubule disassembly (Birukova et al., 2004) and activating Src kinase (Liu et al., 2010). Treatment with a direct thrombin inhibitor reduces the ROS generation and expression of proinflammatory cytokines by cultured brain endothelial cells in response to hypoxia, indicating a mediating role for thrombin in the proinflammatory response of brain endothelial cells (Tripathy et al., 2013).
We have previously demonstrated that thrombin message is highly expressed in microvessels from AD brains but is not detectable in control vessels (Yin et al., 2010). Similarly, Western blot analysis of microvessels shows that the thrombin protein is highly expressed in AD- but not control-derived microvessels (Grammas et al., 2006). Furthermore, injuring brain endothelial cells in vitro with oxidant stress (sodium nitroprusside) or an inflammatory cocktail (IL-1β, IL-6, TNF-α, lipopolysaccharide (LPS), interferon (IFN)-γ) results in thrombin release (Grammas et al., 2004). Since brain endothelial cells can both synthesize and respond to thrombin, it could function as an autocrine factor at the BBB. The importance of findings regarding the effects of thrombin on the cells of the BBB is two-fold. Directly, thrombin damage to the BBB increases permeability and may allow damaging substances, including thrombin and other inflammatory mediators from the blood, to enter the brain. Indirectly, these injured brain endothelial cells can produce their own thrombin into the brain, where it may have untoward effects on microglia, astrocytes and neurons (Figure 2).
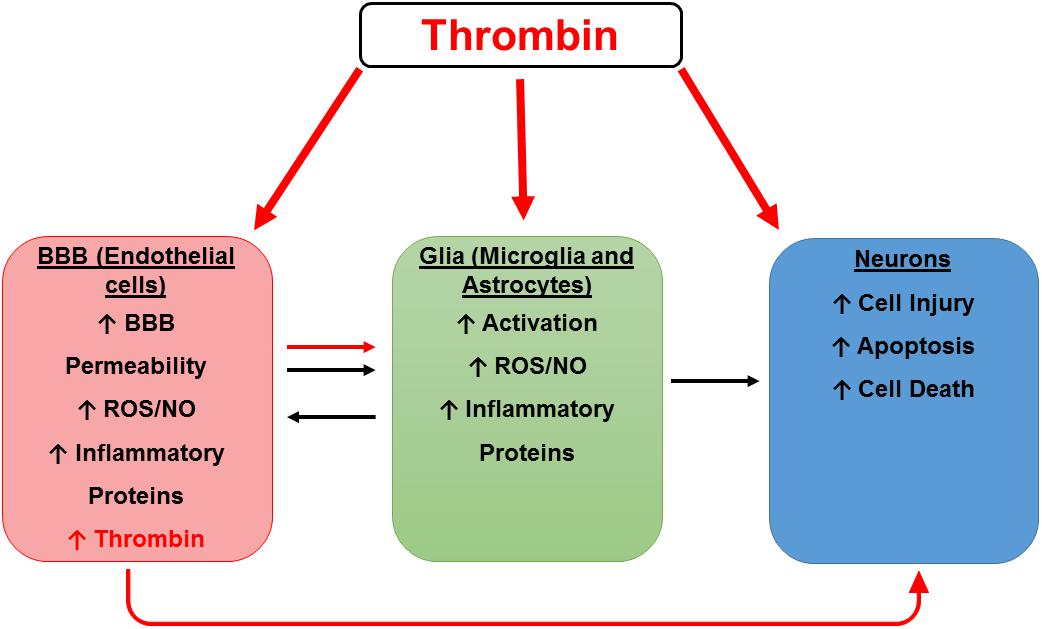
Figure 2. Hypothetical scheme for thrombin as a mediator of cerebrovascular activation, neuroinflammation, and neurodegeneration. A hypothetical scheme for thrombin as a mediator of cerebrovascular activation, neuroinflammation, and neurodegeneration. Thrombin can cause neuronal injury directly and indirectly by activation of endothelial cells (ECs) and glia. Thrombin can act on ECs of the blood-brain barrier (BBB) to cause endothelial activation characterized by increased permeability and an increase in reactive oxygen species (ROS), nitric oxide (NO), and inflammatory proteins including thrombin. These toxic vascular-derived products can injure neurons as well as activate neighboring glial cells to release noxious mediators which in turn cause neuronal injury.
Neuroinflammatory Effects of Thrombin on Microglia and Astrocytes
The response of microglia to thrombin encompasses a number of processes that contribute to microglia activation and/or apoptosis. In the microglial cell line BV2, thrombin induces IL-1β release (Han et al., 2019). Thrombin has also been shown to stimulate a proinflammatory phenotype in microglia, characterized by increases in ROS, NO, and cytokine production (Lee et al., 2006; Huang et al., 2008; Yang et al., 2015; Ye et al., 2017). PAR-1 activation was found to participate in activation of microglia, indicated by up-regulation of microglial CD40 and TNF-α production (Suo et al., 2002). Thrombin, via the TNF-α/TNFR-dependent pathway, downregulates expression of the mRNA species miR-181c which in turn promotes nuclear factor (NF)-κB activity, and upregulates NF-κB target gene expression as well as increasing mixed lineage leukemia-1 (MLL1), a putative gene target for miR-181c (Yin et al., 2017). BV2 cells treated with thrombin show an increase in ROS and the nucleotide-binding domain, leucine-rich-containing family, pyrin domain-containing-3 (NLRP3) inflammasome, a component of the innate immune system, which is associated with a wide range of human CNS disorders (Ye et al., 2017).
Astrocytes show a similar shift towards a proinflammatory phenotype in response to thrombin. In rat brain astrocytes, thrombin induces matrix metalloproteinase (MMP)-9 expression and promotes cell migration via activation of the c-Src/Jak2/PDGFR/PI3K/Akt/PKCδ pathway (Lin et al., 2013). Thrombin exposure can disrupt glutamate transport in astrocytes and modulate stellation, indicating an alteration in function (Cavanaugh et al., 1990; Piao et al., 2015). Thrombin treatment in vivo resulted in increased glial fibrillary acidic protein (GFAP) expression in the hippocampus, indicating a proinflammatory activation in astrocytes (Mhatre et al., 2004).
Thrombin: An Important Mediator of Neurotoxicity and Neurodegenerative Diseases
The multifunctional protease thrombin causes neuronal cell death both in vitro and in vivo, and the neurotoxic effects of thrombin are orchestrated by multiple pathways. In vitro, thrombin-induced neurotoxicity involves activation of PAR-1, followed by RhoA activation and cell cycle re-entry. In neurons treated with thrombin, cyclin D1 and E (early G1 cyclins) and the cyclin dependent kinase, cdk4, are activated and that these events lead to upregulation of the pro-apoptotic protein Bim and apoptosis (Rao et al., 2007). Additionally, thrombin has been demonstrated to cause a rapid influx of calcium in neurons leading to neuronal cell death (Smirnova et al., 1998). Delivery of thrombin directly into the brain by intracerebral injection causes significant neuropathology, such as enlargement of cerebral ventricles, an increased number of TUNEL-positive cells, astrogliosis, and an increase in the immunoreactivity for phosphorylated neurofilament and apolipoprotein-E (ApoE) fragments, as well as cognitive impairments including deficits in reference memory and an increase in task latency (Mhatre et al., 2004). The large body of data indicating thrombin is an important mediator of neuroinflammation and neurotoxicity support the idea that this protein is critically involved in pathological processes that contribute to the development of neurodegenerative diseases including multiple sclerosis (MS), amyolaterotrophic sclerosis (ALS), ischemia, traumatic brain injury (TBI), Parkinson’s disease (PD), and AD (Krenzlin et al., 2016).
Alterations in coagulation-related proteins have been indicated in motor-associated degenerative disorders. Both prothrombin and factor X are elevated in MS (Gobel et al., 2016). Proteomic analysis of chronic active MS lesions identified several dysregulated coagulation factors, highlighting a potential link between the coagulation cascade and MS pathology (Han et al., 2008). In experimental autoimmune encephalomyelitis (EAE), an experimental model of MS, thrombin activity precedes onset of neurological signs, increases at disease peak, and correlates with fibrin deposition, microglial activation, demyelination, axonal damage, and clinical severity (Davalos et al., 2014). The potential pathological significance of coagulation factors in neurological disease is underscored by the finding that the diminution of fibrin, the end product of thrombin’s proteolysis, either genetically or using anticoagulants, significantly reduces neurological signs, inflammation, and axonal damage in EAE (Davalos and Akassoglou, 2012). Additionally, thrombin has been linked to changes in interneuron calcium signaling and enhanced thrombospondin release in ALS (De Luca et al., 2017).
Thrombin has been extensively studied for its role in the response to ischemia (Matsuoka and Hamada, 2002). Thrombin was found to mediate neurovascular injury during ischemia (Chen et al., 2010), and increased thrombin activity was associated with subsequent neuronal damage in a model of acute focal ischemia (Chen et al., 2012). While high concentrations of thrombin seem to be neurotoxic, low concentrations of thrombin (0.01 U/mL) were found to protect against neuronal death in cellular and animal models of ischemia (Striggow et al., 2000). Increases in thrombin have also been associated with TBI. Alterations in BBB permeability have been found following TBI (Korn et al., 2005; Hay et al., 2015). In a model of TBI, BBB damage due to the injury was found to induce increases in thrombin (Piao et al., 2019).
A role for thrombin has also been identified in PD-related pathology. More specifically, thrombin-induced activation of microglia in the midbrain has been directly linked to dopaminergic neuron death (Choi et al., 2003). Thrombin receptor PAR-1 is upregulated in the brains of patients with PD (Ishida et al., 2006; Sokolova and Reiser, 2008). Recently, our lab has identified that treatment with the direct thrombin inhibitor dabigatran improved motor deficits and reduced markers of oxidative stress in a Drosophila melanogaster model of PD (Johnson et al., 2020). A role for thrombin in PD is further indicated by the finding that treatment with a direct thrombin inhibitor is neuroprotective in a rotenone-induced PD rodent model (Kandil et al., 2018). Finally, mice deficient in PAR-1 are protected against 1-methyl-4-phenyl-1,2,3,6-tetrahydropyridine (MPTP)-induced toxicity which causes PD-like syndrome (Hamill et al., 2007).
A Case for Thrombin as a Driver of Neurodegeneration and Vascular Activation in AD
Thrombin has previously been suggested as a possible pathological mediator in AD (Grammas and Martinez, 2014). Thrombin is associated with typical hallmarks of AD-related pathology. Thrombin has been detected in senile plaques and in neurofibrillary tangles characteristic of this disease (Akiyama et al., 1992; Arai et al., 2006) and levels of both thrombin and the thrombin receptor PAR-1 are elevated in AD (Sokolova and Reiser, 2008; Krenzlin et al., 2016). TBI, a condition in which neurons are exposed to high thrombin levels, is associated with an increased incidence of AD (Mortimer et al., 1991; Nemetz et al., 1999). Thrombin may also contribute to ApoE-associated pathology in AD. Intracerebral administration of thrombin to rodents increases ApoE levels in the hippocampus and results in neuronal injury and cognitive deficits (Mhatre et al., 2006). The 22 kDa N-terminal thrombin-cleavage fragment of ApoE is highly neurotoxic and could contribute to ApoE-associated AD pathology (Tolar et al., 1997). Persistent thrombin signaling induces tau aggregation and related hippocampal degeneration (Suo et al., 2003; Arai et al., 2005). Thrombin induces secretion of amyloid precursor protein (APP) in endothelial cells in vitro (Ciallella et al., 1999) and may be involved in altered processing of APP into fragments that are found in amyloid plaques of AD brains (Igarashi et al., 1992; Chong et al., 1994; Ciallella et al., 1999). Aβ promotes thrombin generation through factor XII-mediated factor XI activation (Zamolodchikov et al., 2016). In this regard, depletion of factor XII ameliorates brain pathology and cognitive impairment in AD mice. Additionally, the factor XII-initiated contact system, of which thrombin is a key driver, is activated in AD patients and mice (Zamolodchikov et al., 2015; Chen et al., 2017).
Vascular Activation: A Novel Pathway in AD: A Role for Thrombin
Microvessels isolated from the brains of AD patients overexpress a diverse array of neurotoxic and inflammatory proteins (Grammas and Ovase, 2001; Grammas et al., 2006). Expression of these diverse mediators is consistent with the process of vascular activation and reflects the transition of endothelial cells from a quiescent to a highly synthetic phenotype (Sumpio et al., 2002). A similar pattern of vascular activation has been identified in transgenic AD animal models (Grammas et al., 2014). Vascular activation in the AD brain has deleterious consequences for neuronal viability, as many vascular-derived factors are neurotoxic. The idea that vascular activation contributes to pathogenic events in the AD brain is strongly supported by pre-clinical studies in two AD mouse models where treatment with a vascular activation inhibitor reduced vascular-derived neuroinflammation and improved cognitive performance (Grammas et al., 2014).
Several lines of evidence support the idea that the cerebral vasculature is a convergence point for both the expression of thrombin as well as thrombin-mediated effects that contribute to neuroinflammation and neuronal injury in AD. While the majority of thrombin is produced in the liver, extrahepatic sources of locally generated thrombin, including in the brain, have been documented (Deschepper et al., 1991). Although not widely appreciated, evidence suggests the cerebrovasculature is an important source of thrombin in AD. Immunoreactivity for the major brain thrombin inhibitor, protease nexin-1, is found to be significantly decreased in AD brains, particularly around blood vessels, suggesting vascular release of thrombin (Vaughan et al., 1994). As previously stated, thrombin mRNA and protein are expressed in brain microvessels from AD patients but not detectable in brain microvessels isolated from age-matched control brains (Grammas et al., 2006; Yin et al., 2010). It is not surprising that thrombin is expressed in AD-derived brain microvessels, as in vitro experiments with brain endothelial cells have shown that injuring these cells causes release of thrombin (Grammas et al., 2004). Because brain endothelial cells not only produce thrombin in response to injury but respond to thrombin with a robust inflammatory response, thrombin is likely a key mediator of cerebrovascular activation in AD.
The release of vascular-derived inflammatory proteins could stimulate/activate neighboring glial cells, both microglia and astrocytes, to release inflammatory proteins as well as noxious ROS and proteases (Grammas, 2011). This noxious, neurotoxic cycle could be augmented by vascular-derived thrombin. In addition to thrombin’s inflammatory effects on brain endothelia, thrombin could contribute to deleterious and self-perpetuating neuroinflammation via induction of proinflammatory cytokines including IL-1β, IL-6, TNF-α in microglia and astrocytes (Choi et al., 2003, 2008; Lee et al., 2006; Huang et al., 2008). A recent study in the TgCRND8 AD mouse showed that long-term treatment with the thrombin inhibitor dabigatran preserved cognition, cerebral perfusion, and BBB function, and ameliorated neuroinflammation and amyloid deposition (Cortes-Canteli et al., 2019). Administration of dabigatran to transgenic AD mice diminishes ROS levels in brain and reduces cerebrovascular expression of inflammatory proteins, further supporting an important role for thrombin as a mediator of neuroinflammation in the AD brain (Tripathy et al., 2013).
Inhibiting Thrombin: Implications for Therapeutic Intervention in AD
Increasing evidence supports a chronic procoagulant state in AD, highlighting a possible pathogenic role for thrombin in this disease (Cortes-Canteli et al., 2012). A number of studies in both human populations and animal models utilizing anticoagulant therapies support the notion that abnormalities of coagulation may promote AD pathology. Treatment of transgenic AD mice with enoxaparin, a low molecular weight heparin, reduces plaques, and Aβ accumulation (Bergamaschini et al., 2004) and improves spatial memory (Timmer et al., 2010). As previously stated, TgCRND8 AD mice on long-term dabigatran administration also showed significant improvement in AD pathology (Cortes-Canteli et al., 2019).
Earlier human studies showed improved cognition in dementia patients receiving the anticoagulant warfarin compared to untreated patients (Puccio et al., 2009). Results from these older studies in human patients hinted at a connection between dementia and coagulation abnormalities, but these data were not rigorously pursued. A more recent community-based study found that use of the thrombin inhibitor dabigatran was associated with a lower risk of new-onset dementia (Jacobs et al., 2016). An epidemiological study on atrial fibrillation (AF) patients shows increased thrombin generation and fibrin turnover in subjects with AF and dementia compared to those without dementia, and that long-term warfarin treatment appears to be protective against dementia (Barber et al., 2004; Madhavan et al., 2018).
The evidence supporting a direct connection between AF and dementia suggests the possibility that anticoagulant therapy for AF may reduce the incidence of dementia in this population, and results obtained in a number of recent population-based studies suggest that this is indeed the case (Ding et al., 2018; Field et al., 2019; Mongkhon et al., 2019; Silva et al., 2019). Interestingly, while some groups have found that all anticoagulants provide a level of protection (Friberg and Rosenqvist, 2018) most studies report that the direct oral anticoagulants (DOACs) that target thrombin are particularly efficacious (Jacobs et al., 2016; Chen et al., 2018; Cheng et al., 2018). It is tempting to conclude that DOACs reduce the risk of AF-related dementia by decreasing the incidence of thrombotic and/or embolic events, but this scenario may be overly simplistic. While there is clear evidence that AF is associated with an increased risk of ischemic stroke (Glotzer and Zieglerm, 2015) an ambitious meta-analysis of 21 studies found that the association between AF and dementia was not always dependent on the presence of clinical stroke (Kalantarian et al., 2013), raising questions regarding the mechanisms underlying this association (Chopard et al., 2018). Dietzel et al. (2018) have postulated that AF initiates and perpetuates an increase in systemic inflammation that may lead to dementia, noting recent evidence that AF is associated with increased levels of C-reactive protein (CRP), IL-2, IL-6, IL-8, TNF-α, and other inflammatory cytokines. It is probable that thrombin is a key mediator of this inflammatory state, as it is for the inflammation that accompanies AD dementia. Furthermore, the utility of DOACs in the reduction of AF-related dementia is at least partially due to the ability of these agents to inhibit thrombin’s PAR-mediated inflammatory actions.
It is therefore possible to interrupt the inflammatory cascade that contributes to AD by utilizing DOACs to inhibit thrombin. There is as yet no direct evidence that this approach will be useful in humans, but an open-label study of a hirudin (natural antithrombin anticoagulant) compound in 84 patients with mild-to-moderate AD found that hirudin plus donepezil reduced the rate of cognitive decline compared to donepezil alone, suggesting that direct thrombin inhibition may indeed be an effective strategy for treating this neurodegenerative disease (Li et al., 2012).
Although the use of any anticoagulant raises the possibility of unwanted bleeding, several studies have shown the safety and efficacy of dabigatran. Dabigatran does not interact with food, has minimal drug-drug interactions, and has a low risk of intracranial bleeding. A study documented chronic use (over 30 months) of dabigatran in patients with an average age of 71.4 ± 8.6 had minimal side effects, supporting the efficacy and safety of this drug in an elderly population (Jacobs et al., 2016). Although not without caveats, a body of data implicating thrombin in AD pathogenesis and the relative safety of dabigatran suggest a pilot clinical trial in AD patients is warranted.
Conclusion
The urgent need for disease-modifying drugs in AD demands new thinking about disease pathogenesis and an exploration of novel drug targets. A large, and growing, literature implicates vascular pathology and vascular risk factors in the development of AD, but the specific mechanisms whereby these factors contribute to injury in the AD brain have not yet been clearly defined. It is time for “outside-the-box” thinking about how vascular risk factors, such as atherosclerosis and diabetes, as well as the coagulation and inflammatory pathways interact to promote increased AD risk. The evidence summarized here suggests that thrombin is a convergence point for AD risk factors and as such that thrombin-based therapeutics may therefore target multiple points of AD pathology, including neurodegeneration, vascular activation, and neuroinflammation. Next generation AD therapeutics should not focus on single-target drugs but rather employ a broader, combinatorial approach. We propose that thrombin inhibitors be considered as potential contributors to the dementia therapy pharmacopeia.
Future Directions
The use of dabigatran and other DOACs as a novel strategy for the treatment of AD and possibly other neurodegenerative diseases where thrombin plays a role is warranted. In addition, with the increasing interest/use of newer tools, such as microRNAs, other innovative therapeutic approaches that target thrombin could be employed. MicroRNAs are short regulatory RNA molecules that control gene expression at the posttranscriptional level by affecting the translation and stability of mRNA targets (Simonson and Das, 2015). Evidence suggests that microRNAs are important regulators of neuronal survival, endothelial injury, and inflammatory signaling (Suárez et al., 2007; Fish et al., 2008; Harris et al., 2008; Wang et al., 2008; Wojciechowska et al., 2017; Slota and Booth, 2019). Thrombin, which is at the nexus of all three of these processes, could mediate its effects, in part, via microRNAs. The literature in this area is evolving but studies are beginning to identify thrombin-targeted microRNAs in the development of vascular injury and neuroinflammation (Table 1; Shen et al., 2011; Cowan et al., 2014; Kim et al., 2014; Mehi et al., 2014; Pan et al., 2014; Wang et al., 2014; Lin et al., 2016; Li et al., 2017; Yin et al., 2017; Barwari et al., 2018; Miao et al., 2018; Yao et al., 2019; Cui et al., 2020).
There is increased recognition that effective therapies for AD must shift from the classical “one-size-fits-all” approach to a personalized precision medicine strategy (Hampel et al., 2017). Inter-individual variability in response to pharmacotherapy as well as molecular/physiologic “subtypes” of patients within the broad category of AD support the rationale for a personalized therapeutic approach based on unique genetic characteristics and individual lifestyle attributes (Hahn and Lee, 2019). There is growing interest in the use of pharmacogenomics to optimize the safety and efficacy of DOACs in anticoagulation therapy (Tseng et al., 2018). As more data using this approach become available, incorporating DOACs into a personalized precision medicine approach for the treatment of AD could be accomplished in the near future.
Author Contributions
JI and PG contributed to the initial conception of the review. JI performed the literature search and wrote the first draft of the review. PG contributed to the additional literature search and assisted in writing of subsequent drafts. WR wrote an individual section of the review, prepared the figure, and assisted with editing. All authors read and approved the final version before submission.
Funding
This research was funded by National Institutes of Health grant number 1R21NS110628-01. This work was also generously supported by funding from Cure Alzheimer’s Fund.
Conflict of Interest
The authors declare that the research was conducted in the absence of any commercial or financial relationships that could be construed as a potential conflict of interest.
References
Abu El-Asrar, A. M., Alam, M. I., Nawaz, G., Mohammad, K., Van den Eynde, M. M., Siddiquei, A., et al. (2016). Upregulation of Thrombin/Matrix metalloproteinase-1/protease-activated receptor-1 chain in proliferative diabetic retinopathy. Curr. Eye Res. 41, 1590–1600. doi: 10.3109/02713683.2016.1141964
Akiyama, H., Ikeda, H., Kondo, R., and McGeer, M. (1992). Thrombin accumulation in brains of patients with Alzheimer’s disease. Neurosci. Lett. 146, 152–154. doi: 10.1016/0304-3940(92)90065-f
Alabanza, L. M., and Bynoe, M. (2012). Thrombin induces an inflammatory phenotype in a human brain endothelial cell line. J. Neuroimmunol. 245, 48–55. doi: 10.1016/j.jneuroim.2012.02.004
Alzheimer’s Association (2019). 2019 Alzheimer’s disease facts and figures. Alzheimers Dement. 15, 321–387. doi: 10.1016/j.jalz.2019.01.010
Aoki, I., Shimoyama, N., Aoki, M., Homori, A., Yanagisawa, K., Nakahara, Y., et al. (1996). Platelet-dependent thrombin generation in patients with diabetes mellitus: effects of glycemic control on coagulability in diabetes. J. Am. Coll. Cardiol. 27, 560–566. doi: 10.1016/0735-1097(95)00518-8
Arai, T., Guo, J., and McGeer, P. L. (2005). Proteolysis of non-phosphorylated and phosphorylated tau by thrombin. J. Biol. Chem. 280, 5145–5153. doi: 10.1074/jbc.m409234200
Arai, T., Miklossy, A., Klegeris, J. P., Guo, P., and McGeer, P. L. (2006). Thrombin and prothrombin are expressed by neurons and glial cells and accumulate in neurofibrillary tangles in Alzheimer disease brain. J. Neuropathol. Exp. Neurol. 65, 19–25. doi: 10.1097/01.jnen.0000196133.74087.cb
Ay, L., Hoellerl, C., Ay, J. M., Brix, S., Koder, G. H., Schernthaner, I., et al. (2012). Thrombin generation in type 2 diabetes with albuminuria and macrovascular disease. Eur. J. Clin. Invest. 42, 470–477. doi: 10.1111/j.1365-2362.2011.02602.x
Barber, M., Tait, J., Scott, A., Rumley, G. D., Lowe, G., and Stott, D. (2004). Dementia in subjects with atrial fibrillation: hemostatic function and the role of anticoagulation. J. Thromb. Haemost. 2, 1873–1878. doi: 10.1111/j.1538-7836.2004.00993.x
Barwari, T., Eminaga, U., Mayr, R., Lu, P. C., Armstrong, M. V., Chan, M., et al. (2018). Inhibition of profibrotic microRNA-21 affects platelets and their releasate. JCI Insight 3:e123335.
Bergamaschini, L., Rossi, C., Storini, S., Pizzimenti, M., Distaso, C., Perego, A., et al. (2004). Peripheral treatment with enoxaparin, a low molecular weight heparin, reduces plaques and beta-amyloid accumulation in a mouse model of Alzheimer’s disease. J. Neurosci. 24, 4181–4186. doi: 10.1523/jneurosci.0550-04.2004
Birukova, A. A., Birukov, K., Smurova, D., Adyshev, K., Kaibuchi, I., Alieva, J. G., et al. (2004). Novel role of microtubules in thrombin-induced endothelial barrier dysfunction. FASEB J. 18, 1879–1890. doi: 10.1096/fj.04-2328com
Borissoff, J. I., Joosen, M. O., Versteylen, H. M., Spronk, H., ten Cate, R., and Hofstra, L. (2012). Accelerated in vivo thrombin formation independently predicts the presence and severity of CT angiographic coronary atherosclerosis. JACC Cardiovasc. Imaging 5, 1201–1210. doi: 10.1016/j.jcmg.2012.01.023
Borissoff, J. I., Otten, S., Heeneman, P., Leenders, R., van Oerle, O., Soehnlein, S. T., et al. (2013). Genetic and pharmacological modifications of thrombin formation in apolipoprotein e-deficient mice determine atherosclerosis severity and atherothrombosis onset in a neutrophil-dependent manner. PLoS One 8:e55784. doi: 10.1371/journal.pone.0055784
Brailoiu, E., Shipsky, G., Yan, M. E., Abood, T., and Brailoiu, G. (2017). Mechanisms of modulation of brain microvascular endothelial cells function by thrombin. Brain Res. 1657, 167–175. doi: 10.1016/j.brainres.2016.12.011
Burzynski, L. C., Humphry, K., Pyrillou, K. A., Wiggins, J. N. E., Chan, N., Figg, L. L., et al. (2019). The coagulation and immune systems are directly linked through the activation of interleukin-1alpha by thrombin. Immunity 50, 1033–1042.e6. doi: 10.1016/j.immuni.2019.03.003
Cavanaugh, K. P., Gurwitz, D. D., Cunningham, S., and Bradshaw, R. (1990). Reciprocal modulation of astrocyte stellation by thrombin and protease nexin-1. J. Neurochem. 54, 1735–1743. doi: 10.1111/j.1471-4159.1990.tb01228.x
Chapman, J. (2013). Coagulation in inflammatory diseases of the central nervous system. Semin. Thromb. Hemost. 39, 876–880. doi: 10.1055/s-0033-1357482
Chen, B., Cheng, K., Yang, K., and Lyden, P. (2010). Thrombin mediates severe neurovascular injury during ischemia. Stroke 41, 2348–2352. doi: 10.1161/strokeaha.110.584920
Chen, B., Friedman, M. A., Whitney, J. A., Winkle, I. F., Lei, E. S., Olson, Q., et al. (2012). Thrombin activity associated with neuronal damage during acute focal ischemia. J. Neurosci. 32, 7622–7631. doi: 10.1523/jneurosci.0369-12.2012
Chen, N., Lutsey, R. F., MacLehose, J. S., Claxton, F. L., Norby, A. M., Chamberlain, L. G. S., et al. (2018). Association of oral anticoagulant type with risk of dementia among patients with nonvalvular atrial fibrillation. J. Am. Heart Assoc. 7:e009561.
Chen, Z. L., Revenko, P., Singh, A. R., MacLeod, E. H., Norris, H., and Strickland, S. (2017). Depletion of coagulation factor XII ameliorates brain pathology and cognitive impairment in Alzheimer disease mice. Blood 129, 2547–2556. doi: 10.1182/blood-2016-11-753202
Cheng, W., Liu, B., Li, P., and Li, D. (2018). Relationship of anticoagulant therapy with cognitive impairment among patients with atrial fibrillation: a meta-analysis and systematic review. J. Cardiovasc. Pharmacol. 71, 380–387. doi: 10.1097/fjc.0000000000000575
Cheranova, D., Gibson, S., Chaudhary, L. Q., Zhang, D. P., Heruth, D. N., Grigoryev, M., et al. (2013). RNA-seq analysis of transcriptomes in thrombin-treated and control human pulmonary microvascular endothelial cells. J. Vis. Exp. 72:4393.
Choi, M. S., Kim, W. J., Lee, J. W., Choi, G. H., Park, S. D., Kim, S. J., et al. (2008). Activation of protease-activated receptor1 mediates induction of matrix metalloproteinase-9 by thrombin in rat primary astrocytes. Brain Res. Bull. 76, 368–375. doi: 10.1016/j.brainresbull.2008.02.031
Choi, S. H., Joe, S. U., Kim, S., and Jin, B. K. (2003). Thrombin-induced microglial activation produces degeneration of nigral dopaminergic neurons in vivo. J. Neurosci. 23, 5877–5886. doi: 10.1523/jneurosci.23-13-05877.2003
Chong, Y. H., Jung, W., Choi, C. W., Park, K. S., Choi, S., and Suh, Y. H. (1994). Bacterial expression, purification of full length and carboxyl terminal fragment of Alzheimer amyloid precursor protein and their proteolytic processing by thrombin. Life Sci. 54, 1259–1268. doi: 10.1016/0024-3205(94)00853-1
Chopard, R., Piazza, S. A., Gale, U., Campia, I. E., Albertsen, J., Kim, L., et al. (2018). Dementia and atrial fibrillation: pathophysiological mechanisms and therapeutic implications. Am. J. Med. 131, 1408–1417. doi: 10.1016/j.amjmed.2018.06.035
Ciallella, J. R., Figueiredo, V., Smith-Swintosky, H., and McGillis, J. (1999). Thrombin induces surface and intracellular secretion of amyloid precursor protein from human endothelial cells. Thromb. Haemost. 81, 630–637. doi: 10.1055/s-0037-1614537
Collins, P. W., Macey, M. R., Cahill, N., and Newland, A. (1993). von Willebrand factor release and P-selectin expression is stimulated by thrombin and trypsin but not IL-1 in cultured human endothelial cells. Thromb. Haemost. 70, 346–350. doi: 10.1055/s-0038-1649578
Colotta, F., Sciacca, M., Sironi, W., Luini, M. J., Rabiet, M., and Mantovani, A. (1994). Expression of monocyte chemotactic protein-1 by monocytes and endothelial cells exposed to thrombin. Am. J. Pathol. 144, 975–985.
Cortes-Canteli, M., Kruyer, I., Fernandez-Nueda, A., Marcos-Diaz, C., Ceron, A. T., Richards, O. C., et al. (2019). Long-term dabigatran treatment delays Alzheimer’s disease pathogenesis in the TgCRND8 mouse model. J. Am. Coll. Cardiol. 74, 1910–1923. doi: 10.1016/j.jacc.2019.07.081
Cortes-Canteli, M., Zamolodchikov, H., Ahn, S., Strickland, L., and Norris, E. R. (2012). Fibrinogen and altered hemostasis in Alzheimer’s disease. J. Alzheimers Dis. 32, 599–608. doi: 10.3233/jad-2012-120820
Coughlin, S. R. (2000). Thrombin signalling and protease-activated receptors. Nature 407, 258–264. doi: 10.1038/35025229
Coughlin, S. R. (2005). Protease-activated receptors in hemostasis, thrombosis and vascular biology. J. Thromb. Haemost. 3, 1800–1814. doi: 10.1111/j.1538-7836.2005.01377.x
Cowan, C., Muraleedharan, J. J., O’Donnell, P. K., Singh, H., Lum, A., Kumar, T., et al. (2014). MicroRNA-146 inhibits thrombin-induced NF-κB activation and subsequent inflammatory responses in human retinal endothelial cells. Invest. Ophthalmol. Vis. Sci. 55, 4944–4951.
Cui, H., Yang, H., Zhou, Y., Wang, J., Luo, J., Zhou, T., et al. (2020). Thrombin-induced miRNA-24-1-5p upregulation promotes angiogenesis by targeting prolyl hydroxylase domain 1 in intracerebral hemorrhagic rats. J. Neurosurg. 1–12. doi: 10.3171/2020.2.JNS193069 [Epub ahead of print]
Davalos, D., and Akassoglou, K. (2012). Fibrinogen as a key regulator of inflammation in disease. Semin. Immunopathol. 34, 43–62. doi: 10.1007/s00281-011-0290-8
Davalos, D., Baeten, M. A., Whitney, E. S., Mullins, B., Friedman, E. S., Olson, J. K., et al. (2014). Early detection of thrombin activity in neuroinflammatory disease. Ann. Neurol. 75, 303–308. doi: 10.1002/ana.24078
Davalos, D., Ryu, M., Merlini, K. M., Baeten, N., Le Moan, M. A., Petersen, T. J., et al. (2012). Fibrinogen-induced perivascular microglial clustering is required for the development of axonal damage in neuroinflammation. Nat. Commun. 3:1227.
De Luca, C., Virtuoso, N., Maggio, L., and Papa, M. (2017). Neuro-Coagulopathy: blood coagulation factors in central nervous system diseases. Int. J. Mol. Sci. 18:2128. doi: 10.3390/ijms18102128
Deschepper, C. F., Bigornia, M. E., Berens, V., and Lapointe, M. C. (1991). Production of thrombin and antithrombin III by brain and astroglial cell cultures. Brain Res. Mol. Brain Res. 11, 355–358. doi: 10.1016/0169-328x(91)90045-y
Dietzel, J., Haeusler, K., and Endres, M. (2018). Does atrial fibrillation cause cognitive decline and dementia? Europace 20, 408–419. doi: 10.1093/europace/eux031
Ding, M., Fratiglioni, K., Johnell, G., Santoni, J., Fastbom, P., Ljungman, A., et al. (2018). Atrial fibrillation, antithrombotic treatment, and cognitive aging: a population-based study. Neurology 91, e1732–e1740. doi: 10.1212/wnl.0000000000006456
Esmon, C. T. (2008). Crosstalk between inflammation and thrombosis. Maturitas 61, 122–131. doi: 10.1016/j.maturitas.2008.11.008
Field, T. S., Weijs, A., Curcio, M., Giustozzi, S., Sudikas, A., Katholing, C., et al. (2019). Incident atrial fibrillation, dementia and the role of anticoagulation: a population-based cohort study. Thromb. Haemost. 119, 981–991. doi: 10.1055/s-0039-1683429
Fish, J. E., Santoro, S. U., Morton, S., Yu, R. F., Yeh, J. D., Wythe, K. N., et al. (2008). miR-126 regulates angiogenic signaling and vascular integrity. Dev. Cell 15, 272–284. doi: 10.1016/j.devcel.2008.07.008
Friberg, L., and Rosenqvist, M. (2018). Less dementia with oral anticoagulation in atrial fibrillation. Eur. Heart J. 39, 453–460. doi: 10.1093/eurheartj/ehx579
Glotzer, T. V., and Zieglerm, P. D. (2015). Cryptogenic stroke: Is silent atrial fibrillation the culprit? Heart Rhythm. 12, 234–241. doi: 10.1016/j.hrthm.2014.09.058
Göbel, K., Eichler, H., Wiendl, T., Chavakis, C., Kleinschnitz, P., and Meuth, S. G. (2018). The coagulation factors fibrinogen, thrombin, and factor XII in inflammatory disorders—a systematic review. Front. Immunol. 9:1731. doi: 10.3389/fimmu.2018.01731
Gobel, K., Kraft, S., Pankratz, C. C., Gross, C., Korsukewitz, R., Kwiecien, R., et al. (2016). Prothrombin and factor X are elevated in multiple sclerosis patients. Ann. Neurol. 80, 946–951. doi: 10.1002/ana.24807
Grammas, P. (2011). Neurovascular dysfunction, inflammation and endothelial activation: implications for the pathogenesis of Alzheimer’s disease. J. Neuroinflammation 8:26. doi: 10.1186/1742-2094-8-26
Grammas, P., Martinez, A., Sanchez, X., Yin, J., Riley, D., Gay, K., et al. (2014). A new paradigm for the treatment of Alzheimer’s disease: targeting vascular activation. J. Alzheimers Dis. 40, 619–630. doi: 10.3233/jad-2014-132057
Grammas, P., and Martinez, M. (2014). Targeting thrombin: an inflammatory neurotoxin in Alzheimer’s disease. J. Alzheimers Dis. 42(Suppl. 4), S537–S544.
Grammas, P., Ottman, U., Reimann-Philipp, J., Larabee, H., and Weigel, P. H. (2004). Injured brain endothelial cells release neurotoxic thrombin. J. Alzheimers Dis. 6, 275–281. doi: 10.3233/jad-2004-6308
Grammas, P., and Ovase, R. (2001). Inflammatory factors are elevated in brain microvessels in Alzheimer’s disease. Neurobiol. Aging 22, 837–842. doi: 10.1016/s0197-4580(01)00276-7
Grammas, P., Samany, P. G., and Thirumangalakudi, L. (2006). Thrombin and inflammatory proteins are elevated in Alzheimer’s disease microvessels: implications for disease pathogenesis. J. Alzheimers Dis. 9, 51–58. doi: 10.3233/jad-2006-9105
Hahn, C., and Lee, C. U. (2019). A brief review of paradigm shifts in prevention of Alzheimer’s disease: from cognitive reserve to precision medicine. Front. Psychiatry 10:786. doi: 10.3389/fpsyt.2019.00786
Hamill, C. E., Caudle, J. R., Richardson, H., Yuan, K. D., Pennell, J. G., Greene, G. W., et al. (2007). Exacerbation of dopaminergic terminal damage in a mouse model of Parkinson’s disease by the G-protein-coupled receptor protease-activated receptor 1. Mol. Pharmacol. 72, 653–664. doi: 10.1124/mol.107.038158
Hampel, H., O’Bryant, S., Durrleman, E., Younesi, K., Rojkova, V., Escott-Price, J. C., et al. (2017). A Precision Medicine Initiative for Alzheimer’s disease: the road ahead to biomarker-guided integrative disease modeling. Climacteric 20, 107–118. doi: 10.1080/13697137.2017.1287866
Han, C., Xia, S., Jiao, G., Li, Q., Ran, S., and Yao, S. (2019). Tripartite motif containing protein 37 involves in thrombin stimulated BV-2 microglial cell apoptosis and interleukin 1beta release. Biochem. Biophys. Res. Commun. 516, 1252–1257. doi: 10.1016/j.bbrc.2019.06.158
Han, M. H., Hwang, D. B., Roy, D. H., Lundgren, J. V., Price, S. S., Ousman, G. H., et al. (2008). Proteomic analysis of active multiple sclerosis lesions reveals therapeutic targets. Nature 451, 1076–1081. doi: 10.1038/nature06559
Hao, J. S., Zhu, B. Y., Yan, C. Y., Yan, T., and Ling, R. (2018). Stimulation of KLF14/PLK1 pathway by thrombin signaling potentiates endothelial dysfunction in Type 2 diabetes mellitus. Biomed. Pharmacother. 99, 859–866. doi: 10.1016/j.biopha.2018.01.151
Harris, T. A., Yamakuchi, M., Ferlito, J. T., Mendell, L., and Lowenstein, C. (2008). MicroRNA-126 regulates endothelial expression of vascular cell adhesion molecule 1. Proc. Natl. Acad. Sci. U.S.A. 105, 1516–1521. doi: 10.1073/pnas.0707493105
Hay, J. R., Johnson, A. M., Young, D. H., Smith, D., and Stewart, W. (2015). Blood-brain barrier disruption is an early event that may persist for many years after traumatic brain injury in humans. J. Neuropathol. Exp. Neurol. 74, 1147–1157. doi: 10.1093/jnen/74.12.1147
Huang, C., Ma, S., Sun, G., Wei, Y., Fang, R., Liu, T., et al. (2008). JAK2-STAT3 signaling pathway mediates thrombin-induced proinflammatory actions of microglia in vitro. J. Neuroimmunol. 204, 118–125. doi: 10.1016/j.jneuroim.2008.07.004
Igarashi, K., Murai, H., and Asaka, J. (1992). Proteolytic processing of amyloid beta protein precursor (APP) by thrombin. Biochem. Biophys. Res. Commun. 185, 1000–1004. doi: 10.1016/0006-291x(92)91726-7
Ishibashi, Y., Matsui, S., Ueda, K., Fukami, L., and Yamagishi, S. (2014). Advanced glycation end products potentiate citrated plasma-evoked oxidative and inflammatory reactions in endothelial cells by up-regulating protease-activated receptor-1 expression. Cardiovasc. Diabetol. 13:60. doi: 10.1186/1475-2840-13-60
Ishida, Y., Nagai, S., Kobayashi, S., and Kim, S. U. (2006). Upregulation of protease-activated receptor-1 in astrocytes in Parkinson disease: astrocyte-mediated neuroprotection through increased levels of glutathione peroxidase. J. Neuropathol. Exp. Neurol. 65, 66–77. doi: 10.1097/01.jnen.0000195941.48033.eb
Jaberi, N., Soleimani, M., Pashirzad, H., Abdeahad, F., Mohammadi, M., Khoshakhlagh, M., et al. (2019). Role of thrombin in the pathogenesis of atherosclerosis. J. Cell. Biochem. 120, 4757–4765.
Jacobs, V., May, T.L., Bair, B.G., Crandall, M.J., Cutler, J.D., Day, C., et al. (2016). Long-term population-based cerebral ischemic event and cognitive outcomes of direct oral anticoagulants compared with warfarin among long-term anticoagulated patients for atrial fibrillation.. Am. J. Cardiol. 118, 210–214.
Jennewein, C., Tran, P., Paulus, P., Ellinghaus, J. A., Eble, S., and Zacharowski, K. (2011). Novel aspects of fibrin(ogen) fragments during inflammation. Mol. Med. 17, 568–573. doi: 10.2119/molmed.2010.00146
Johnson, S. L., Iannucci, N. P., Seeram, J., and Grammas, P. (2020). Inhibiting thrombin improves motor function and decreases oxidative stress in the LRRK2 transgenic Drosophila melanogaster model of Parkinson’s disease. Biochem. Biophys. Res. Commun. 527, 532–538. doi: 10.1016/j.bbrc.2020.04.068
Kalantarian, S., Stern, M., Mansour, T., and Ruskin, J. N. (2013). Cognitive impairment associated with atrial fibrillation: a meta-analysis. Ann. Intern. Med. 158(5 Pt 1), 338–346.
Kalz, J., ten Cate, H., and Spronk, M. (2014). Thrombin generation and atherosclerosis. J. Thromb. Thrombolysis 37, 45–55.
Kandil, E. A., Sayed, L. A., Ahmed, M. A., Abd El Fattah, R., and El-Sayeh, M. (2018). Modulatory role of Nurr1 activation and thrombin inhibition in the neuroprotective effects of dabigatran etexilate in rotenone-induced Parkinson’s disease in rats. Mol. Neurobiol. 55, 4078–4089.
Kaplanski, G., Marin, M., Fabrigoule, V., Boulay, A. M., Benoliel, P., Bongrand, S., et al. (1998). Thrombin-activated human endothelial cells support monocyte adhesion in vitro following expression of intercellular adhesion molecule-1 (ICAM-1; CD54) and vascular cell adhesion molecule-1 (VCAM-1; CD106). Blood 92, 1259–1267. doi: 10.1182/blood.v92.4.1259.416k11_1259_1267
Kim, J. M., Lee, K., Chu, K. H., Jung, J. H., Kim, J. S., Yu, S., et al. (2014). Inhibition of Let7c microRNA is neuroprotective in a rat intracerebral hemorrhage model. PLoS One 9:e97946. doi: 10.1371/journal.pone.0097946
Kopec, A. K., Abrahams, S., Thornton, J. S., Palumbo, E. S., Mullins, S., Divanovic, H., et al. (2017). Thrombin promotes diet-induced obesity through fibrin-driven inflammation. J. Clin. Invest. 127, 3152–3166. doi: 10.1172/jci92744
Kopec, A. K., Joshi, K. L., Towery, K. M., Kassel, B. P., Sullivan, M. J., and Flick, M. (2014). Thrombin inhibition with dabigatran protects against high-fat diet-induced fatty liver disease in mice. J. Pharmacol. Exp. Ther. 351, 288–297. doi: 10.1124/jpet.114.218545
Korn, A., Golan, I., Melamed, R., Pascual-Marqui, S., and Friedman, A. (2005). Focal cortical dysfunction and blood-brain barrier disruption in patients with Postconcussion syndrome. J. Clin. Neurophysiol. 22, 1–9. doi: 10.1097/01.wnp.0000150973.24324.a7
Krenzlin, H., Lorenz, S., Danckwardt, O., Kempskim, P., and Alessandri, B. (2016). The importance of thrombin in cerebral injury and disease. Int. J. Mol. Sci. 17:84. doi: 10.3390/ijms17010084
Lee, D. Y., Park, K. W., and Jin, B. K. (2006). Thrombin induces neurodegeneration and microglial activation in the cortex in vivo and in vitro: proteolytic and non-proteolytic actions. Biochem. Biophys. Res. Commun. 346, 727–738. doi: 10.1016/j.bbrc.2006.05.174
Lee, I. O., Kratz, S. H., Schirmer, M., Baumhakel, P., and Bohm, M. (2012). The effects of direct thrombin inhibition with dabigatran on plaque formation and endothelial function in apolipoprotein E-deficient mice. J. Pharmacol. Exp. Ther. 343, 253–257. doi: 10.1124/jpet.112.194837
Levi, M., van der Poll, H., ten Cate, L., and van Deventer, S. (1997). The cytokine-mediated imbalance between coagulant and anticoagulant mechanisms in sepsis and endotoxaemia. Eur. J. Clin. Invest. 27, 3–9. doi: 10.1046/j.1365-2362.1997.570614.x
Levi, M., and van der Poll, T. (2005). Two-way interactions between inflammation and coagulation. Trends Cardiovasc. Med. 15, 254–259. doi: 10.1016/j.tcm.2005.07.004
Li, D. Q., Zhou, P., and Yang, H. (2012). Donepezil combined with natural hirudin improves the clinical symptoms of patients with mild-to-moderate Alzheimer’s disease: a 20-week open-label pilot study. Int. J. Med. Sci. 9, 248–255. doi: 10.7150/ijms.4363
Li, J., Tan, Q., Xiang, Z., Zhou, C., and Yan, H. (2017). Thrombin-activated platelet-derived exosomes regulate endothelial cell expression of ICAM-1 via microRNA-223 during the thrombosis-inflammation response. Thromb. Res. 154, 96–105. doi: 10.1016/j.thromres.2017.04.016
Lin, C. C., Lee, W. B., Wu, C. J., Liu, H. L., Hsieh, L. D., Hsiao, C. C., et al. (2013). Thrombin mediates migration of rat brain astrocytes via PLC, Ca(2)(+), CaMKII, PKCalpha, and AP-1-dependent matrix metalloproteinase-9 expression. Mol. Neurobiol. 48, 616–630. doi: 10.1007/s12035-013-8450-6
Lin, J., He, X., Sun, G., Franck, Y., Deng, D., Yang, S., et al. (2016). MicroRNA-181b inhibits thrombin-mediated endothelial activation and arterial thrombosis by targeting caspase recruitment domain family member 10. FASEB J. 30, 3216–3226. doi: 10.1096/fj.201500163r
Liu, D. Z., Ander, H., Xu, Y., Shen, P., Kaur, W., Deng, P., et al. (2010). Blood-brain barrier breakdown and repair by Src after thrombin-induced injury. Ann. Neurol. 67, 526–533. doi: 10.1002/ana.21924
Machida, T., Takata, J., Matsumoto, T., Miyamura, R., Hirata, I., Kimura, Y., et al. (2017). Contribution of thrombin-reactive brain pericytes to blood-brain barrier dysfunction in an in vivo mouse model of obesity-associated diabetes and an in vitro rat model. PLoS One 12:e0177447. doi: 10.1371/journal.pone.0177447
Madhavan, M., Graff-Radford, J. P., Piccini, J., and Gersh, B. J. (2018). Cognitive dysfunction in atrial fibrillation. Nat. Rev. Cardiol. 15, 744–756.
Marin, V., Montero-Julian, S., Gres, V., Boulay, P., Bongrand, C., and Farnarier, L. (2001). The IL-6-soluble IL-6Ralpha autocrine loop of endothelial activation as an intermediate between acute and chronic inflammation: an experimental model involving thrombin. J. Immunol. 167, 3435–3442. doi: 10.4049/jimmunol.167.6.3435
Matsuoka, H., and Hamada, R. (2002). Role of thrombin in CNS damage associated with intracerebral haemorrhage: opportunity for pharmacological intervention? CNS Drugs 16, 509–516. doi: 10.2165/00023210-200216080-00001
Mehi, S. J., Maltare, C. R., Abraham, K., and King, G. D. (2014). MicroRNA-339 and microRNA-556 regulate Klotho expression in vitro. Age 36, 141–149. doi: 10.1007/s11357-013-9555-6
Merlini, M., Rafalski, P. E., Rios Coronado, T. M., Gill, M., Ellisman, G., Muthukumar, K. S., et al. (2019). Fibrinogen induces microglia-mediated spine elimination and cognitive impairment in an Alzheimer’s disease model. Neuron 101, 1099–1108.e6. doi: 10.1016/j.neuron.2019.01.014
Mhatre, M., Hensley, A., Nguyen, S., and Grammas, P. (2006). Chronic thrombin exposure results in an increase in apolipoprotein-E levels. J. Neurosci. Res. 84, 444–449. doi: 10.1002/jnr.20887
Mhatre, M., Nguyen, S., Kashani, T., Pham, A., Adesina, M., and Grammas, P. (2004). Thrombin, a mediator of neurotoxicity and memory impairment. Neurobiol. Aging 25, 783–793. doi: 10.1016/j.neurobiolaging.2003.07.007
Miao, X., Rahman, L., Jiang, Y., Min, S., Tan, H., Xie, L., et al. (2018). Thrombin-reduced miR-27b attenuates platelet angiogenic activities in vitro via enhancing platelet synthesis of anti-angiogenic thrombospondin-1. J. Thromb. Haemost. 16, 791–801. doi: 10.1111/jth.13978
Miho, N., Ishida, N., Kuwaba, M., Ishida, K., Shimote-Abe, K., Tabuchi, T., et al. (2005). Role of the JNK pathway in thrombin-induced ICAM-1 expression in endothelial cells. Cardiovasc. Res. 68, 289–298. doi: 10.1016/j.cardiores.2005.05.029
Minami, T., Sugiyama, S. Q., Wu, R., Abid, T., Kodama, D., and Aird, W. (2004). Thrombin and phenotypic modulation of the endothelium. Arterioscler. Thromb. Vasc. Biol. 24, 41–53. doi: 10.1161/01.atv.0000099880.09014.7d
Mongkhon, P., Naser, L., Fanning, G., Tse, W. C. Y., Lau, I. C. K., Wong, K., et al. (2019). Oral anticoagulants and risk of dementia: a systematic review and meta-analysis of observational studies and randomized controlled trials. Neurosci. Biobehav. Rev. 96, 1–9. doi: 10.1016/j.neubiorev.2018.10.025
Mortimer, J. A., van Duijn, V., Chandra, L., Fratiglioni, A. B., Graves, A., Heyman, A. F., et al. (1991). Head trauma as a risk factor for Alzheimer’s disease: a collaborative re-analysis of case-control studies. EURODEM Risk Factors Research Group. Int. J. Epidemiol. 20(Suppl. 2), S28–S35.
Nawroth, P. P., and Stern, D. M. (1986). Modulation of endothelial cell hemostatic properties by tumor necrosis factor. J. Exp. Med. 163, 740–745. doi: 10.1084/jem.163.3.740
Nemetz, P. N., Leibson, J. M., Naessens, M., Beard, E., Kokmen, J. F., Annegers, L., et al. (1999). Traumatic brain injury and time to onset of Alzheimer’s disease: a population-based study. Am. J. Epidemiol. 149, 32–40. doi: 10.1093/oxfordjournals.aje.a009724
Okada, M., Suzuki, K., Takada, M., Nakashima, T., Nakanishi, P., and Shinohara, T. (2006). Detection of up-regulated genes in thrombin-stimulated human umbilical vein endothelial cells. Thromb. Res. 118, 715–721. doi: 10.1016/j.thromres.2005.11.008
Page, M. J., Bester, J., and Pretorius, E. (2018). The inflammatory effects of TNF-alpha and complement component 3 on coagulation. Sci. Rep. 8:1812.
Palekar, R. U., Jallouk, J. W., Myerson, H., Pan, H., and Wickline, S. A. (2016). Inhibition of thrombin with PPACK-nanoparticles restores disrupted endothelial barriers and attenuates thrombotic risk in experimental atherosclerosis. Arterioscler. Thromb. Vasc. Biol. 36, 446–455. doi: 10.1161/atvbaha.115.306697
Palta, S., Saroa, R., and Palta, A. (2014). Overview of the coagulation system. Indian J. Anaesth. 58, 515–523.
Pan, Y., Liang, H., Liu, D., Li, X., Chen, L., Li, C. Y., et al. (2014). Platelet-secreted microRNA-223 promotes endothelial cell apoptosis induced by advanced glycation end products via targeting the insulin-like growth factor 1 receptor. J. Immunol. 192, 437–446. doi: 10.4049/jimmunol.1301790
Paneni, F., Beckman, M. A., Creager, M., and Cosentino, F. (2013). Diabetes and vascular disease: pathophysiology, clinical consequences, and medical therapy: part I. Eur. Heart J. 34, 2436–2443. doi: 10.1093/eurheartj/eht149
Petersen, M. A., Ryu, J. K., and Akassoglou, K. (2018). Fibrinogen in neurological diseases: mechanisms, imaging and therapeutics. Nat. Rev. Neurosci. 19, 283–301. doi: 10.1038/nrn.2018.13
Piao, C., Ranaivo, A., Rusie, N., Wadhwani, S., Koh, S., and Wainwright, M. (2015). Thrombin decreases expression of the glutamate transporter GLAST and inhibits glutamate uptake in primary cortical astrocytes via the Rho kinase pathway. Exp. Neurol. 273, 288–300. doi: 10.1016/j.expneurol.2015.09.009
Piao, C. S., Holloway, S., Hong-Routson, L., and Wainwright, M. (2019). Depression following traumatic brain injury in mice is associated with down-regulation of hippocampal astrocyte glutamate transporters by thrombin. J. Cereb. Blood Flow Metab. 39, 58–73. doi: 10.1177/0271678x17742792
Pingel, S., Tiyerili, J., Mueller, N., Werner, G., Nickenig, P., and Mueller, C. (2014). Thrombin inhibition by dabigatran attenuates atherosclerosis in ApoE deficient mice. Arch. Med. Sci. 10, 154–160. doi: 10.5114/aoms.2014.40742
Preusch, M. R., Ieronimakis, E. S., Wijelath, S., Cabbage, J., Ricks, F., Bea, M., et al. (2015). Dabigatran etexilate retards the initiation and progression of atherosclerotic lesions and inhibits the expression of oncostatin M in apolipoprotein E-deficient mice. Drug Des. Devel. Ther. 9, 5203–5211.
Puccio, D., Novo, V., Baiamonte, A., Nuccio, G., Fazio, E., Corrado, G., et al. (2009). Atrial fibrillation and mild cognitive impairment: what correlation? Minerva Cardioangiol. 57, 143–150.
Rahadian, A., Fukuda, H. M., Salim, S., Yagi, K., Kusunose, H., Yamada, T., et al. (2020). Thrombin inhibition by dabigatran attenuates endothelial dysfunction in diabetic mice. Vascul. Pharmacol. 124:106632. doi: 10.1016/j.vph.2019.106632
Rahman, A., True, K. N., Anwar, R. D., Ye, T. A., Voyno-Yasenetskaya, R., and Malik, A. (2002). Galpha(q) and Gbetagamma regulate PAR-1 signaling of thrombin-induced NF-kappaB activation and ICAM-1 transcription in endothelial cells. Circ. Res. 91, 398–405. doi: 10.1161/01.res.0000033520.95242.a2
Rao, H. V., Thirumangalakudi, P., Desmond, T., and Grammas, P. (2007). Cyclin D1, cdk4, and Bim are involved in thrombin-induced apoptosis in cultured cortical neurons. J. Neurochem. 101, 498–505. doi: 10.1111/j.1471-4159.2006.04389.x
Shen, X., Fang, X., Lv, Z., Pei, Y., Wang, S., Jiang, T., et al. (2011). Heparin impairs angiogenesis through inhibition of microRNA-10b. J. Biol. Chem. 286, 26616–26627. doi: 10.1074/jbc.m111.224212
Silva, R., Miranda, T., Liu, G., Tse, M., and Roever, L. (2019). Atrial fibrillation and risk of dementia: epidemiology, mechanisms, and effect of anticoagulation. Front. Neurosci. 13:18. doi: 10.3389/fnins.2019.00018
Simonson, B., and Das, S. (2015). MicroRNA therapeutics: The next magic bullet? Mini Rev. Med. Chem. 15, 467–474. doi: 10.2174/1389557515666150324123208
Slota, J. A., and Booth, B. (2019). MicroRNAs in neuroinflammation: implications in disease pathogenesis, biomarker discovery and therapeutic applications. Noncoding RNA 5:35. doi: 10.3390/ncrna5020035
Smirnova, I. V., Vamos, T., Wiegmann, B. A., Citron, P. M., Arnold, P., and Festoff, B. (1998). Calcium mobilization and protease-activated receptor cleavage after thrombin stimulation in motor neurons. J. Mol. Neurosci. 10, 31–44. doi: 10.1007/bf02737083
Sokolova, E., and Reiser, G. (2008). Prothrombin/thrombin and the thrombin receptors PAR-1 and PAR-4 in the brain: localization, expression and participation in neurodegenerative diseases. Thromb. Haemost. 100, 576–581. doi: 10.1160/th08-03-0131
Striggow, F., Riek, J., Breder, P., Henrich-Noack, K., Reymann, L., and Reiser, G. (2000). The protease thrombin is an endogenous mediator of hippocampal neuroprotection against ischemia at low concentrations but causes degeneration at high concentrations. Proc. Natl. Acad. Sci. U.S.A. 97, 2264–2269. doi: 10.1073/pnas.040552897
Suárez, Y., Fernández-Hernando, J. S., Pober, F., and Sessa, W. (2007). Dicer dependent microRNAs regulate gene expression and functions in human endothelial cells. Circ. Res. 100, 1164–1173. doi: 10.1161/01.res.0000265065.26744.17
Sumpio, B. E., Riley, T., and Dardik, A. (2002). Cells in focus: endothelial cell. Int. J. Biochem. Cell Biol. 34, 1508–1512. doi: 10.1016/s1357-2725(02)00075-4
Suo, Z., Wu, S., Ameenuddin, H. E., Anderson, J. E., Zoloty, B. A., Citron, P., et al. (2002). Participation of protease-activated receptor-1 in thrombin-induced microglial activation. J. Neurochem. 80, 655–666. doi: 10.1046/j.0022-3042.2001.00745.x
Suo, Z., Wu, M., Citron, B. A., Palazzo, R. E., and Festoff, B. W. (2003). Rapid tau aggregation and delayed hippocampal neuronal death induced by persistent thrombin signaling. J. Biol. Chem. 278, 37681–37689. doi: 10.1074/jbc.m301406200
ten Cate, H. (2012). Tissue factor-driven thrombin generation and inflammation in atherosclerosis. Thromb. Res. 129(Suppl. 2), S38–S40.
Timmer, N. M., van Dijk, C. E., van der Zee, A., Kiliaan, R. M., de Waal, T., and Verbeek, M. (2010). Enoxaparin treatment administered at both early and late stages of amyloid beta deposition improves cognition of APPswe/PS1dE9 mice with differential effects on brain Abeta levels. Neurobiol. Dis. 40, 340–347. doi: 10.1016/j.nbd.2010.06.008
Tolar, M., Marques, J. A., Harmony, S., and Crutcher, K. A. (1997). Neurotoxicity of the 22 kDa thrombin-cleavage fragment of apolipoprotein E and related synthetic peptides is receptor-mediated. J. Neurosci. 17, 5678–5686. doi: 10.1523/jneurosci.17-15-05678.1997
Tripathy, D., Sanchez, X., Yin, J., Luo, J., Martinez, R., and Grammas, P. (2013). Thrombin, a mediator of cerebrovascular inflammation in AD and hypoxia. Front. Aging Neurosci. 5:19. doi: 10.3389/fnagi.2013.00019
Tseng, A. S., Patel, H. E., Quist, A., Kekic, J. T., Maddux, C. B., and Grilli, B. (2018). Clinical review of the pharmacogenomics of direct oral anticoagulants. Cardiovasc. Drugs Ther. 32, 121–126.
Undas, A., Wiek, E., Stepien, K., Zmudka, R., and Tracz, W. (2008). Hyperglycemia is associated with enhanced thrombin formation, platelet activation, and fibrin clot resistance to lysis in patients with acute coronary syndrome. Diabetes Care 31, 1590–1595. doi: 10.2337/dc08-0282
Vaughan, P. J., Su, C. W., Cotman, J., and Cunningham, D. (1994). Protease nexin-1, a potent thrombin inhibitor, is reduced around cerebral blood vessels in Alzheimer’s disease. Brain Res. 668, 160–170. doi: 10.1016/0006-8993(94)90521-5
Wang, H. J., Huang, Y. Y., Shih, H. Y., Wu, C. T., Peng, T., and Lo, W. (2014). MicroRNA-146a decreases high glucose/thrombin-induced endothelial inflammation by inhibiting NAPDH oxidase 4 expression. Mediators Inflamm. 2014:379537.
Wang, S., Aurora, B. A., Johnson, X., Qi, J., McAnally, J. A., Hill, J. A., et al. (2008). The endothelial-specific microRNA miR-126 governs vascular integrity and angiogenesis. Dev. Cell 15, 261–271. doi: 10.1016/j.devcel.2008.07.002
Wei, H. J., Li, G. Y., Shi, S. L., Liu, P. C., Chang, C. H., and Kuo, R. (2011). Thrombomodulin domains attenuate atherosclerosis by inhibiting thrombin-induced endothelial cell activation. Cardiovasc. Res. 92, 317–327. doi: 10.1093/cvr/cvr220
Witkowski, M., Landmesser, U., and Rauch, U. (2016). Tissue factor as a link between inflammation and coagulation. Trends Cardiovasc. Med. 26, 297–303. doi: 10.1016/j.tcm.2015.12.001
Wojciechowska, A., Braniewska, A., and Kozar-Kamińska, K. (2017). MicroRNA in cardiovascular biology and disease. Adv. Clin. Exp. Med. 26, 865–874.
Yang, Y., Zhang, X., Kang, C., Jiang, H., Zhang, P., and Wang, R. (2015). Thrombin-induced microglial activation impairs hippocampal neurogenesis and spatial memory ability in mice. Behav. Brain Funct. 11:30.
Yao, Y., Sun, Q., Sun, B., Jing, S., Liu, X., Liu, G., et al. (2019). Platelet-derived exosomal microRNA-25-3p inhibits coronary vascular endothelial cell inflammation through Adam10 via the NF-κB Signaling Pathway in ApoE–/– Mice. Front. Immunol. 10:2205. doi: 10.3389/fimmu.2019.02205
Ye, X., Zuo, L., Yu, L., Zhang, J., Tang, C., Cui, L., et al. (2017). ROS/TXNIP pathway contributes to thrombin induced NLRP3 inflammasome activation and cell apoptosis in microglia. Biochem. Biophys. Res. Commun. 485, 499–505. doi: 10.1016/j.bbrc.2017.02.019
Yin, M., Chen, Y., Ouyang, H., Zhang, Z., Wan, H., Wang, W., et al. (2017). Thrombin-induced, TNFR-dependent miR-181c downregulation promotes MLL1 and NF-κB target gene expression in human microglia. J. Neuroinflammation 14:132.
Yin, X., Wright, J., Wall, T., and Grammas, P. (2010). Brain endothelial cells synthesize neurotoxic thrombin in Alzheimer’s disease. Am. J. Pathol. 176, 1600–1606. doi: 10.2353/ajpath.2010.090406
Zamolodchikov, D., Chen, B. A., Conti, T., Renne, P., and Strickland, S. (2015). Activation of the factor XII-driven contact system in Alzheimer’s disease patient and mouse model plasma. Proc. Natl. Acad. Sci. U.S.A. 112, 4068–4073. doi: 10.1073/pnas.1423764112
Keywords: vascular, inflammation, Alzheimer’s, endothelial, coagulation, therapeutics
Citation: Iannucci J, Renehan W and Grammas P (2020) Thrombin, a Mediator of Coagulation, Inflammation, and Neurotoxicity at the Neurovascular Interface: Implications for Alzheimer’s Disease. Front. Neurosci. 14:762. doi: 10.3389/fnins.2020.00762
Received: 15 April 2020; Accepted: 29 June 2020;
Published: 24 July 2020.
Edited by:
Musthafa Mohamed Essa, Sultan Qaboos University, OmanReviewed by:
Sudhanshu P. Raikwar, University of Missouri, United StatesJudie Arulappan, Sultan Qaboos University, Oman
Copyright © 2020 Iannucci, Renehan and Grammas. This is an open-access article distributed under the terms of the Creative Commons Attribution License (CC BY). The use, distribution or reproduction in other forums is permitted, provided the original author(s) and the copyright owner(s) are credited and that the original publication in this journal is cited, in accordance with accepted academic practice. No use, distribution or reproduction is permitted which does not comply with these terms.
*Correspondence: Jaclyn Iannucci, amFjbHluX2lhbm51Y2NpQHVyaS5lZHU=