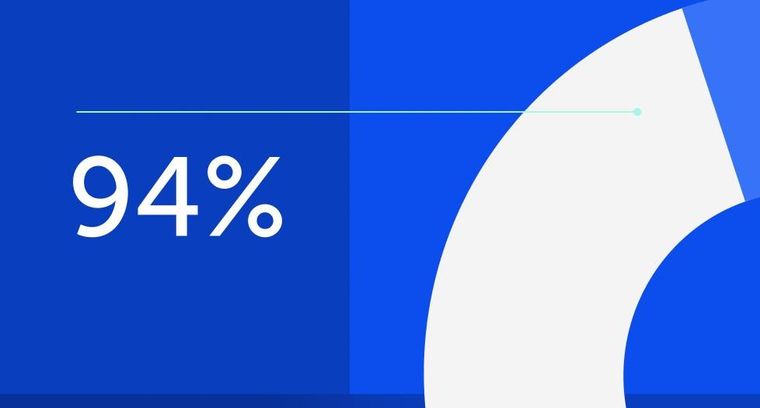
94% of researchers rate our articles as excellent or good
Learn more about the work of our research integrity team to safeguard the quality of each article we publish.
Find out more
MINI REVIEW article
Front. Neurosci., 21 May 2020
Sec. Neuropharmacology
Volume 14 - 2020 | https://doi.org/10.3389/fnins.2020.00513
The use of psychostimulants and alcohol disrupts blood-brain barrier (BBB) integrity, resulting in alterations to cellular function, and contributes to neurotoxicity. The BBB is the critical boundary of the central nervous system (CNS) where it maintains intracellular homeostasis and facilitates communication with the peripheral circulation. The BBB is regulated by tight junction (TJ) proteins that closely interact with endothelial cells (EC). The complex TJ protein network consists of transmembrane proteins, including claudins, occludins, and junction adhesion molecules (JAM), as well as cytoskeleton connected scaffolding proteins, zonula occludentes (ZO-1, 2, and 3). The use of psychostimulants and alcohol is known to affect the CNS and is implicated in various neurological disorders through neurotoxicity that partly results from increased BBB permeability. The present mini review primarily focuses on BBB structure and permeability. Moreover, we assess TJ protein and cytoskeletal changes induced by cocaine, methamphetamine, morphine, heroin, nicotine, and alcohol. These changes promote glial activation, enzyme potentiation, and BBB remodeling, which affect neuroinflammatory pathways. Although the effect of drugs of abuse on BBB integrity and the underlying mechanisms are well studied, the present review enhances the understanding of the underlying mechanisms through which substance abuse disorders cause BBB dysfunction.
The blood-brain barrier (BBB) is formed by an endothelial cell (EC) monolayer between the blood and central nervous system (CNS) that contributes to maintaining structural and functional homeostasis in the brain. The BBB structure interacts with perivascular pericytes, microglial cells, astrocytes, and neurons that, together, form the neurovascular units (Abbott et al., 2010; Obermeier et al., 2013; Chow and Gu, 2015; Banks, 2016). Notably, BBB permeability is –in part- a function of pericyte-regulated endothelial transcytosis. The BBB is formed by an EC network rigidly connected by complex junction systems comprised of smaller trans-membrane tight junction (TJ) proteins, including junction adhesion molecules (JAM), endothelial cell-selective adhesion molecules, occludins, and claudins (Ballabh et al., 2004; Van Itallie and Anderson, 2014). This creates a boundary between the CNS and peripheral circulation for regulating blood-CNS exchange (Kousik et al., 2012).
The BBB is critical to the maintenance of brain homeostasis as it regulates the entry of macromolecules, ions, and neurotransmitters from the blood to the brain (Abbott et al., 2010; Lippmann et al., 2013; Wilhelm and Krizbai, 2014; Erickson and Banks, 2018). Notably, the BBB limits the entry of neurotoxic substances from the periphery and contributes to maintenance of a stable microenvironment for optimal neuronal function to prevent critical CNS damage (Abbott et al., 2010). This highly selective permeable barrier allows passive diffusion of certain gases, water, and lipid-soluble molecules, which is necessary for efficient neural function (Bellettato and Scarpa, 2018). Recent research has found that drugs of abuse, including cocaine, methamphetamine (METH), morphine, heroin, nicotine, and alcohol, cause BBB dysfunction by altering TJ formation and protein expression (Hawkins and Davis, 2005; Abbott et al., 2006). The concentration and distribution of a drug regulate its passage (Pardridge, 2012).
Globally, studies have shown that approximately 240 million people are alcohol-dependent, more than one billion are smokers, and roughly 15 million are illicit drug users. Substance use disorder is either directly or indirectly responsible for 11.8 million annual deaths; moreover, the use of different drugs varies across geographical locations. In 2017, 70,237 people in the USA died from drug overdoses (Kariisa et al., 2019). The National Survey on Drug Use and Health estimated that approximately 20 million Americans have used illicit drugs within the past month, which is expected to reach 9.2% of the United States population. Moreover, there are significant gender-based differences in the initiation of drug usage, as well as neurotransmitter systems and neural circuitries, among individuals with substance use disorders. Individual differences in addiction behaviors depend on several factors, including the method of drug administration, sociocultural factors, genetics, personality traits, and several biological processes (Becker et al., 2017). Furthermore, preclinical studies have shown that females often show higher responsivity to drugs compared to males.
The menstrual cycle and estrogen are essential for treatment outcome in female drug users. Specifically, withdrawal symptom severity may differ between the luteal and follicular menstrual phase (Snively et al., 2000; Terner and de Wit, 2006; Allen et al., 2010). Males have a higher metabolic rate, which affects neural mechanisms (Fattore et al., 2014). Regardless, both males and females exhibit brain changes after using drugs of abuse (Leyton, 2007; Wegener and Koch, 2009; Willuhn et al., 2010; Andersen et al., 2012).
Drugs of abuse increase BBB permeability, which, in turn, increases influx of peripheral toxins into the brain. Consequently, BBB dysfunction activates neuro-inflammatory pathways by increasing astro-glial activation, which –in turn- increases BBB permeability and susceptibility of the CNS to foreign molecules (Kousik et al., 2012; Ronaldson and Davis, 2015). BBB integrity loss contributes to changes in transport pathways, disruption of EC-cell interactions, redistribution, and/or downregulation of TJ protein modifications (Kousik et al., 2012; Rochfort et al., 2014; Yang et al., 2019). The present review summarizes the signaling mechanisms that contribute to drug abuse-related BBB dysfunction (Figure 1).
Figure 1. Schematic representation showing drug-induced loss of blood brain barrier (BBB) permeability and the associated neurodegeneration. The neurovascular unit and the BBB are affected by various drugs of abuse, which alter vessel permeability via disruption of tight junction proteins complexes (junction adhesion molecules, endothelial cell-selective adhesion molecules, occludins, and claudins), transport systems, and intracellular signaling. BBB disruption, which affects immune cell transmigration and neuroinflammation and contributes to an imbalanced redox system, affects the brain’s microenvironment and homeostasis, leading to neurotoxicity (Created with Biorender.com).
The World Drug Report estimates that worldwide, 18.8 million people used cocaine in 2014 (United Nations Office on Drugs and Crime, 2016). In 2016, the National Institute on Drug Abuse reported an age-adjusted cocaine-mediated death rate of 52.4% in the USA. Cocaine is a highly addictive stimulant that restricts dopamine and monoamine reuptake through dopamine transporter (DAT) antagonism (Kousik et al., 2012). Monoamine oxidase inhibition leads to imbalanced free-radical production, which generates oxidative stress and neuroinflammation. Continuous cocaine administration has been shown to contribute to a 50% increase in BBB permeability, with a concomitant decrease in trans endothelial electrical resistance (TEER) due to basement membrane and neurovascular capillary disruption, due to up-regulated matrix metalloproteinase (MMP) and tumor necrosis factor (TNF-α) expression (Sharma et al., 2009). Moreover, TJ protein loss and alteration, specifically decreased JAM-2 and zonula occludens-1 (ZO-1) levels, are characteristic of cocaine transit across the BBB (Dietrich, 2009). CCL2 (C-C motif chemokine ligand-2) and CCR2 (C-C motif chemokine receptor-2) expression upregulation has also been reported (Fiala et al., 2005). Cocaine use affects intercellular junctions and causes cell ruffling, which contributes to increased permeability and decreased TEER values across BBB monolayers (Fiala et al., 2005; Srinivasan et al., 2015).
An alternate pathway for cocaine-induced BBB permeability alteration involves platelet-derived growth factor (PDGF) intermediates (Yao et al., 2011). Cocaine binding to sigma receptors evokes a proteolytic signal cascade that initiates PDGF-B chain assembly, a fundamental intermediate for increased membrane permeability that inhibits store-operated calcium entry (Yao et al., 2011; Cristina Brailoiu et al., 2016; Rosado, 2016). Moreover, cocaine binding to sigma receptors has been associated with dopamine uptake inhibition and enhanced dopamine release that neutralizes the effects of antibody reversal on increased PDGF expression (Kumar, 2011). In rats, chronic cocaine exposure has been shown to increase BBB permeability in the hippocampus and striatum, suggesting that the hippocampus could be affected by glial and cytokine migration without significant changes in cortical or cerebellar permeability (Riley et al., 2017). Furthermore, it has been recently revealed that acute cocaine administration alters BBB permeability and may increase neurotoxicity in free-moving rats (Barr et al., 2019).
Astrocytes have complex morphologies involving extensive processes that communicate within the neurovascular unit and maintain the BBB. Cocaine exposure potentiates aberrant astroglial responses in cellular and animal models, which leads to loss of BBB integrity and function (Fattore et al., 2002; Yang et al., 2016). Other studies have reported cocaine-induced neuroinflammation and BBB disruption mediated by the activation of brain microglial cells to secrete several cytokines, chemokines, and other neurotoxic factors (Yao et al., 2010; Buch et al., 2012). Cocaine upregulates these inflammatory mediators and cell adhesion molecules, including intercellular adhesion molecule-1, vascular cell adhesion molecule, and activated leukocyte cell adhesion molecule in the BBB endothelium (Fiala et al., 1998; Yao et al., 2011).
Previous in vitro findings have shown that exposure of pericytes to cocaine upregulates pro-inflammatory cytokines [TNF-α, interleukin (IL)-1β, and IL-6] in both intracellular and extracellular compartments. In addition, cocaine activates the Src–PDGFR-β–NF-κB pathway, which enhances CXCL10 [chemokine (C-X-C motif) ligand-1] secretion. This causes increased neuroinflammation in human brain vascular pericytes (Table 1), which further leads to neurovascular unit disruption and immune cell transmigration across the BBB (Niu et al., 2019; Sil et al., 2019).
Table 1. Summary of cocaine- and methamphetamine-induced neurotoxicity based on their effect on the structural integrity of the blood brain barrier and their respective molecular pathways.
METH is a highly addictive and illicit psychostimulant and is the second most widely abused drug in the USA. It adversely affects brain homeostasis through BBB dysfunction and hyperthermia (O’Shea et al., 2014). Its high lipophilicity allows for rapid and comprehensive transmigration across the BBB. METH binding to the DAT induces reversal transport of norepinephrine, serotonin (5HT), and dopamine, which causes their excessive release into the synapse (Kousik et al., 2012). Moreover, it inhibits monoamine reuptake that leads to post-synaptic cleft stimulation (Kousik et al., 2012). Chronic METH administration causes irreversible impairment of serotonin and dopamine transport into synaptic terminals in various brain regions, especially in the hippocampus.
Various METH dosing paradigms significantly disturb endothelial TJ assembly by inducing downregulation, fragmentation, or redistribution of major TJ proteins, including claudin-5 and ZO-1, which are mediated by MMP-1 and MMP-9 peptidases. This leads to reduced endothelial barrier tightness and increased BBB paracellular permeability (Mahajan et al., 2008; Ramirez et al., 2009; Banerjee et al., 2010; Liu et al., 2012; Toborek et al., 2013; Sajja et al., 2016; Rubio-Araiz et al., 2017). Moreover, repeated intravenous METH administration downregulates TJ proteins, which causes glutathione depletion and increases endothelial reactive oxygen species (ROS) levels. This triggers actin polymerization that possibly involves activation of actin-related protein 2/3 complex or myosin light chain kinase and its downstream target RhoA (Mahajan et al., 2008; Ramirez et al., 2009; Banerjee et al., 2010; Park et al., 2013). In mice, research has shown that METH-induced glucose transporter and uptake downregulation is an important causative factor for BBB integrity loss (Abdul Muneer et al., 2011). Further, METH reduces TJ protein expression, rearranges the F-actin cytoskeleton, and increases BBB permeability through Rho-associated protein kinase-dependent pathway activation in the frontal lobes and isolated primary microvascular endothelial cells (Xue et al., 2019).
Other neurotoxicity mechanisms have also been suggested, including the METH-induced increase in reactive oxidative stress and ROS levels, which activate myosin light chain protein kinase, thereby reducing TJ protein expression (Gonçalves et al., 2010). Additionally, METH-induced TJ protein downregulation and resulting BBB integrity disruption may involve activation of NF-κB transcription and pro-inflammatory cytokines (TNF-α) in BBB endothelial cells (Coelho-Santos et al., 2015; Rom et al., 2015). METH transit across the BBB damages the nucleus accumbens shell region and prefrontal cortex and causes hyperthermia, neuroinflammation, and brain edema (Kousik et al., 2012). Recent studies have reported METH-induced pericyte migration via sigma-1 receptor activation, p53 upregulated modulator of apoptosis expression, and downstream mitogen-activated protein kinase and Akt/PI3K pathways in C3H/10T1/2 cells, leading to BBB dysfunction (Zhang et al., 2017). METH-activated microglia and astrocytes in the neurovascular unit may promote neurotoxicity and astroglial reactivity and induces BBB integrity loss (Asanuma et al., 2004; Dietrich, 2009). In addition, METH increases the expression of the glial fibrillary acidic protein, σ1 receptors, TNF-α, IL-6, and IL-8 in mouse and rat astrocytes. This leads to METH-induced inflammation in microglial cells where increased TNF-α release can activate BBB endothelium, which increases transmigration of circulating leukocytes through the leaky BBB (Malaplate-Armand et al., 2005; Shah et al., 2012; Zhang et al., 2015; Table 1).
Opioids are widely-used analgesics that bind with opioid and/or toll-like receptors (TLR) in the CNS (Chaves et al., 2017; Yang et al., 2018). Transcellular solute and xenobiotic transport across the BBB is selectively controlled by the local influx and efflux transporters, including ATP-binding cassette (ABC), P-glycoprotein (P-gp, ABCB1), breast cancer resistance protein (ABCG2), multidrug resistance-associated proteins (ABCC) transporters, and solute carrier transporters (Abbott et al., 2010; Chaves et al., 2017). Among the four central opioid receptor families [mu (μ), delta (δ), kappa (κ), and opioid receptor like-1 (ORL1) receptor], μ-opioid receptors are primarily responsible for the analgesic effects. Microvascular endothelial cells have high affinity and specific opiate binding sites that mediate morphine’s effects on the CNS (Stefano et al., 1995).
Morphine exerts its effects by directly acting on the CNS with its illicit use leading to tolerance and drug dependence (Gach et al., 2011). Drug transmigration is essential to psychological dependence. Morphine alters BBB homeostasis and permeability through pro-inflammatory cytokine activity, intracellular calcium release dysregulation, and myosin light chain protein kinase activation, which results in ROS-mediated neurotoxicity (Kousik et al., 2012).
P-gp limits the net transport of several foreign substrates into the brain through active unidirectional efflux. This transporter regulates foreign-agent pharmacokinetics in the brain by inhibiting or augmenting their movement across the BBB, which restrains morphine entry into the brain (Tournier et al., 2011). Moreover, P-gp attenuates morphine-induced migratory properties and transcriptional effects (Miller, 2010). Acute morphine treatment inhibits P-gp expression, which increases morphine uptake in the brain, which modifies the acute analgesic and locomotive morphine effects and selectively alters critical transcriptional responses in the nucleus accumbens (Seleman et al., 2014). This indicates that the transporter system significantly contributes to mediating BBB integrity and permeability of carrier mediated transport (Table 2).
Table 2. Summary of morphine-, heroin-, nicotine-, and alcohol-induced neurotoxicity according to their effect on the structural integrity of the BBB and their respective molecular pathways.
There has been a rapid increase in opioid abuse in the USA with approximately 580 new heroin users every day. Deaths resulting from opiate overdose, including pain relievers and heroin, increased by 200% between 2000 and 2014 (Rudd et al., 2016). Heroin can be reversibly metabolized into morphine; upon selective transmigration across the BBB, heroin is transformed into morphine and metabolized into 6-monoacetylmorphine (6-MAM). The superior heroin lipophilicity allows faster transit across the BBB than morphine (Boix et al., 2013). The acetylation of both hydroxyl groups while synthesizing heroin increases its BBB penetration rate by 100-fold, which could contribute to its high addictive potential (Boix et al., 2013). These addictive properties are regulated by the μ-opioid receptor (MOR), which mediates the rewarding effects of heroin. A recent study reported that 6-MAM has a higher affinity for μ-opioid receptor G-protein activation than morphine (Seleman et al., 2014).
Heroin’s effects indirectly involve its metabolites (morphine and 6-MAM) that act as substrates in P-gp membrane regulation. Upon heroin transition into the brain, it has a higher synthesized concentration than morphine. This suggests that the metabolite is the primary effector of the detrimental effects of heroin on the BBB. In the extracellular brain fluid, these metabolites bind and activate MORs, which regulates crucial neurological automatic processes (Boix et al., 2013). P-gp inhibition at the BBB acutely disrupts the BBB permeability and selectivity in the nucleus accumbens (Seleman et al., 2014). Moreover, increased levels of these metabolites in the brain downregulate TJ protein expression, especially ZO-1, which increases BBB permeability. Contrastingly, there have been reports of increased JAM-2 TJ protein expression (Seleman et al., 2014; Table 2).
Nicotine is a stimulant that acts as a nicotinic acetylcholine receptor agonist. Its high lipophilicity allows for rapid (10–20 s after inhalation) transit across the BBB. Chronic exposure to nicotine disrupts TJ proteins and results in an ionic imbalance within the BBB microenvironment. Consequently, this causes ischemic hypoxia and exacerbates stroke-associated brain edema and neuronal injury (Paulson et al., 2006; Bradford et al., 2011). Nicotine exposure alters BBB permeability through TJ protein modulation. It does not affect ZO-1, 2; claudin-1, -3; or -5 TJ protein expression, however, it disrupts the distribution of claudin-3 and ZO-1 TJ proteins (Kousik et al., 2012). Moreover, nicotine-induced BBB impairment has been shown to involve decreased ZO-1 expression, which affects brain homeostasis (Hutamekalin et al., 2008). Similarly, static- or flow-based in vitro BBB model studies have reported tobacco-induced alterations in TJ protein expression and re-distribution, which increases intracellular ROS/RNS and the secretory profile of various pro-inflammatory markers (Hossain et al., 2011; Naik et al., 2014). This oxidative stress promotes atherosclerotic lesions and injures biliary epithelial cells (BECs) and TJ proteins via low-density lipoprotein oxidation enhanced by ROS activity (Kousik et al., 2012). Moreover, this results in increased transcytotic activity across the BBB through induced pinocytosis (Kousik et al., 2012).
Direct nicotine binding to nicotinic acetylcholine receptors on BECs induces acetylcholine-dependent nitric oxide (NO) release via activation of neurovascular endothelial NO synthase (Mazzone et al., 2010). Here, increased NO2 enhances vascular membrane permeability at the BBB. Furthermore, chronic nicotine administration compromises BBB integrity through TJ protein loss and alteration (ZO-1, claudin-3, and JAM-1). It affects regulated BBB transport and receptor systems that are essential for normal BBB function, as well as decrease the functional activity of ion transporters (Mazzone et al., 2010). Nicotine has been shown to decrease TEER and disturb the BBB transport system, which leads to increased xenobiotic uptake (Hutamekalin et al., 2008; Manda et al., 2010; Rodriguez-Gaztelumendi et al., 2011). Nicotine affects the functional activity of ion transporters, including Na+, K+, 2Cl– cotransporter and Na+, K+-ATPase on BECs and inhibits P-gp activity in the CNS (Abbruscato et al., 2004; Paulson et al., 2006; Manda et al., 2010). Recent studies indicate that the H+/organic cation antiporter system is involved in blood-to-brain nicotine transport across BBB endothelial cells TR-BBB13 (Tega et al., 2018; Table 2). The precipitated ion gradient change induces brain edema, which further disrupts BBB integrity (Kousik et al., 2012).
Alcohol is a widely used recreational drug responsible for 5.3% of deaths worldwide. In the USA, there are 23 million alcohol addicts with 88,000 people dying from alcohol use disorder. Alcohol acts on neurotransmitter receptors, including GABA, glutamate, and dopamine, with each receptor contributing to various physiologic effects, with chronic alcohol administration increasing tolerance and addiction (Burnett et al., 2016). Further, occasional alcohol consumption could lead to alcohol use disorder due to addiction and tolerance (Costin and Miles, 2014). Regular and excessive alcohol consumption causes brain injury, white matter loss, reduced brain volume, and neuronal loss associated with the BBB (Mann et al., 2001; Muneer et al., 2012; Bjork and Gilman, 2014). Moreover, gray matter loss is positively correlated with years of alcohol abuse (Fein et al., 2002). Chronic alcohol abuse induces neuroplastic changes and loss of neural circuit structure and strength (Mende, 2019).
The brains of individuals with alcohol dependence have increased proinflammatory cytokines, chemokines, microglial markers, and inflammasome proteins (He and Crews, 2008; Crews et al., 2013). Inflammatory cytokine and ROS activation contributes to BBB integrity disruption in TLR4-knockout mice (Rubio-Araiz et al., 2017). Further, postmortem alcoholic brains have shown increased TLR2, TLR3, and TLR4 expression in the orbitofrontal cortex, which correlates with BBB integrity loss (Crews et al., 2013). Moreover, they indicate that chronic alcohol intake increases TJ and neuroinflammatory protein (ERK1/2 and p-38) degradation, which may promote leukocyte brain infiltration (Rubio-Araiz et al., 2017).
Brain microvascular endothelial cells (BMVEC) are interconnected with TJ to form a tight monolayer in the BBB. Exposure of BMVEC to alcohol increases oxidative stress through myosin light chain and TJ protein phosphorylation. This leads to decreased TEER and increased leukocyte migration across the BBB (Haorah et al., 2007). Further, alcohol induces BBB dysfunction and neuroinflammation through MMP-3/9 activation and angiogenesis (VEGF)-A/VEGFR-2) impairment in primary endothelial cells in the brain (Muneer et al., 2012). Ethanol (EtOH) disrupts BBB integrity via endothelial transient receptor potential (TRP) channels, which affects the intracellular Ca2+ and Mg2+ dynamics. This increases endothelial permeability and alters inflammatory responses (Chang et al., 2018). EtOH-mediated TRPM7 expression downregulation causes BBB dysfunction and endothelium integrity loss (Macianskiene et al., 2008; Oh et al., 2012). Overall, TRP channels are involved in alcohol-mediated BBB dysfunction (Table 2).
The BBB is crucial in drug abuse-mediated neurotoxicity. The BBB network characteristics are involved in functional restriction and transport control, as well as maintaining a constant CNS environment. TJ protein disruption, neuroinflammation, oxidative stress, and ROS production are fundamental mechanisms through which drugs alter the BBB structure and integrity. In adults, the mature CNS lacks substantial regenerative capacity while terminally differentiated neurons cannot divide and supplant themselves. Increased cell death due to neurotoxin entry could lead to a premature disabling condition. Although there has been previous research on the effects of drugs of abuse on the BBB, there is a need for further studies to identify novel therapeutic targets. Awareness regarding the effect of drugs of abuse on BBB integrity is paramount due to their toxic effects, which could induce immune reactions and neurodegeneration. There are current studies on potential therapeutic targets for preventing this neurotoxicity and propagation. Detailed knowledge regarding the physiology of drug abuse-associated BBB dysfunction, with respect to TJ protein complexes, transport systems, and intracellular signaling pathways, could allow the determination of effective therapeutic interventions. Moreover, a deep understanding of brain mechanisms could improve future prevention and treatment interventions. Comprehensive research on the mechanistic aspects of drug abuse-mediated BBB dysfunction could identify better therapeutic targets. Polysubstance abuse is among the significant challenges faced by drug abusers. Since each drug of abuse has a different mechanism of BBB disruption, understanding the effect of polysubstance abuse on BBB could allow the evaluation of novel therapeutic agents and systemic prediction of clinical efficacy. Future studies should explore means of restoring BBB integrity, which could extend scientific knowledge and contribute to novel therapeutic targets.
TS, EP, and KS designed and wrote the main manuscript. KS and MD contributed to the figure, reference sited, and proofread. All authors reviewed this manuscript.
The present study was supported by grants from the National Institute of Health (NIH): DA044872.
The authors declare that the research was conducted in the absence of any commercial or financial relationships that could be construed as a potential conflict of interest.
Abbott, N. J., Patabendige, A. A. K., Dolman, D. E. M., Yusof, S. R., and Begley, D. J. (2010). Structure and function of the blood-brain barrier. Neurobiol. Dis. 37, 13–25. doi: 10.1016/j.nbd.2009.07.030
Abbott, N. J., Rönnbäck, L., and Hansson, E. (2006). Astrocyte-endothelial interactions at the blood-brain barrier. Nat. Rev. Neurosci. 7, 41–53. doi: 10.1038/nrn1824
Abbruscato, T. J., Lopez, S. P., Roder, K., and Paulson, J. R. (2004). Regulation of blood-brain barrier Na,K,2Cl-cotransporter through phosphorylation during in vitro stroke conditions and nicotine exposure. J. Pharmacol. Exp. Ther. 310, 459–468. doi: 10.1124/jpet.104.066274
Abdul Muneer, P. M., Alikunju, S., Szlachetka, A. M., Murrin, L. C., and Haorah, J. (2011). Impairment of brain endothelial glucose transporter by methamphetamine causes blood-brain barrier dysfunction. Mol. Neurodegener. 6:23. doi: 10.1186/1750-1326-6-23
Allen, A. M., Allen, S. S., Lunos, S., and Pomerleau, C. S. (2010). Severity of withdrawal symptomatology in follicular versus luteal quitters: the combined effects of menstrual phase and withdrawal on smoking cessation outcome. Addict. Behav. 35, 549–552. doi: 10.1016/j.addbeh.2010.01.003
Andersen, M. L., Sawyer, E. K., and Howell, L. L. (2012). Contributions of neuroimaging to understanding sex differences in cocaine abuse. Exp. Clin. Psychopharmacol. 20, 2–15. doi: 10.1037/a0025219
Asanuma, M., Miyazaki, I., Higashi, Y., Tsuji, T., and Ogawa, N. (2004). Specific gene expression and possible involvement of inflammation in methamphetamine-induced neurotoxicity. Ann. N. Y. Acad. Sci. (N. Y. Acad. Sci.) 1025, 69–75. doi: 10.1196/annals.1316.009
Ballabh, P., Braun, A., and Nedergaard, M. (2004). The blood-brain barrier: an overview: structure, regulation, and clinical implications. Neurobiol. Dis. 16, 1–13. doi: 10.1016/j.nbd.2003.12.016
Banerjee, A., Zhang, X., Manda, K. R., Banks, W. A., and Ercal, N. (2010). HIV proteins (gp120 and Tat) and methamphetamine in oxidative stress-induced damage in the brain: potential role of the thiol antioxidant N-acetylcysteine amide. Free Radic. Biol. Med. 48, 1388–1398. doi: 10.1016/j.freeradbiomed.2010.02.023
Banks, W. A. (2016). From blood-brain barrier to blood-brain interface: new opportunities for CNS drug delivery. Nat. Rev. Drug Discov. 15, 275–292. doi: 10.1038/nrd.2015.21
Barr, J. L., Brailoiu, G. C., Abood, M. E., Rawls, S. M., Unterwald, E. M., and Brailoiu, E. (2019). Acute cocaine administration alters permeability of blood-brain barrier in freely-moving rats— Evidence using miniaturized fluorescence microscopy. Drug Alcohol. Depend. 206:107637. doi: 10.1016/j.drugalcdep.2019.107637
Becker, J. B., McClellan, M. L., and Reed, B. G. (2017). Sex differences, gender and addiction. J. Neurosci. Res. 95, 136–147. doi: 10.1002/jnr.23963
Bellettato, C. M., and Scarpa, M. (2018). Possible strategies to cross the blood-brain barrier. Ital. J. Pediatr. 44:131. doi: 10.1186/s13052-018-0563-0
Bjork, J. M., and Gilman, J. M. (2014). The effects of acute alcohol administration on the human brain: insights from neuroimaging. Neuropharmacology 84, 101–110. doi: 10.1016/j.neuropharm.2013.07.039
Boix, F., Andersen, J. M., and Mørland, J. (2013). Pharmacokinetic modeling of subcutaneous heroin and its metabolites in blood and brain of mice. Addict. Biol. 18, 1–7. doi: 10.1111/j.1369-1600.2010.00298.x
Bradford, S. T., Stamatovic, S. M., Dondeti, R. S., Keep, R. F., and Andjelkovic, A. V. (2011). Nicotine aggravates the brain postischemic inflammatory response. Am. J. Physiol. – Hear. Circ. Physiol. 300, H1518–H1529. doi: 10.1152/ajpheart.00928.2010
Buch, S., Yao, H., Guo, M., Mori, T., Mathias-Costa, B., Singh, V., et al. (2012). Cocaine and HIV-1 interplay in CNS: cellular and molecular mechanisms. Curr. HIV Res. 10, 425–428. doi: 10.2174/157016212802138823
Burnett, E. J., Chandler, L. J., and Trantham-Davidson, H. (2016). Glutamatergic plasticity and alcohol dependence-induced alterations in reward, affect and cognition. Prog. Neuro-Psychopharmacol. Biol. Psychiatry 65, 309–320. doi: 10.1016/j.pnpbp.2015.08.012
Chang, S. L., Huang, W., Mao, X., and Mack, M. L. (2018). Ethanol’s effects on transient receptor potential channel expression in brain microvascular endothelial cells. J. Neuroimmune Pharmacol. 13, 498–508. doi: 10.1007/s11481-018-9796-3
Chaves, C., Remiao, F., Cisternino, S., and Decleves, X. (2017). Opioids and the blood-brain barrier: a dynamic interaction with consequences on drug disposition in brain. Curr. Neuropharmacol. 15, 1156–1173. doi: 10.2174/1570159x15666170504095823
Coelho-Santos, V., Leitão, R. A., Cardoso, F. L., Palmela, I., Rito, M., Barbosa, M., et al. (2015). The TNF-α/NF-κB signaling pathway has a key role in methamphetamine-induced blood-brain barrier dysfunction. J. Cereb. Blood Flow Metab. 35, 1260–1271. doi: 10.1038/jcbfm.2015.59
Costin, B. N., and Miles, M. F. (2014). “Molecular and neurologic responses to chronic alcohol use,” in Handbook of Clinical Neurology, eds P. J. Vinken and G. W. Bruyn (Amsterdam: Elsevier B.V.), 157–171. doi: 10.1016/B978-0-444-62619-6.00010-0
Chow, B. W., and Gu, C. (2015). The molecular constituents of the blood–brain barrier. Trends Neurosci. 38, 598–608. doi: 10.1016/j.tins.2015.08.003
Crews, F. T., Qin, L., Sheedy, D., Vetreno, R. P., and Zou, J. (2013). High mobility group box 1/toll-like receptor danger signaling increases brain neuroimmune activation in alcohol dependence. Biol. Psychiatry 73, 602–612. doi: 10.1016/j.biopsych.2012.09.030
Cristina Brailoiu, G., Deliu, E., Console-Bram, L. M., Soboloff, J., Abood, M. E., Unterwald, E. M., et al. (2016). Cocaine inhibits store-operated Ca2+ entry in brain microvascular endothelial cells: critical role for sigma-1 receptors. Biochem. J. 473, 1–5. doi: 10.1042/BJ20150934
Dietrich, J. B. (2009). Alteration of blood-brain barrier function by methamphetamine and cocaine. Cell Tissue Res. 336, 385–392. doi: 10.1007/s00441-009-0777-y
Erickson, M. A., and Banks, W. A. (2018). Neuroimmune axes of the blood-brain barriers and blood-brain interfaces: bases for physiological regulation, disease states, and pharmacological interventions. Pharmacol. Rev. 70, 278–314. doi: 10.1124/pr.117.014647
Fattore, L., Melis, M., Fadda, P., and Fratta, W. (2014). Sex differences in addictive disorders. Front. Neuroendocrinol. 35:272–284. doi: 10.1016/j.yfrne.2014.04.003
Fattore, L., Puddu, M. C., Picciau, S., Cappai, A., Fratta, W., Serra, G. P., et al. (2002). Astroglial in vivo response to cocaine in mouse dentate gyrus: a quantitative and qualitative analysis by confocal microscopy. Neuroscience 110, 1–6. doi: 10.1016/s0306-4522(01)00598-x
Fein, G., Di Sclafani, V., Cardenas, V. A., Goldmann, H., Tolou-Shams, M., and Meyerhoff, D. J. (2002). Cortical gray matter loss in treatment-naïve alcohol dependent individuals. Alcohol. Clin. Exp. Res. 26, 558–564. doi: 10.1097/00000374-200204000-00017
Fiala, M., Eshleman, A. J., Cashman, J., Lin, J., Lossinsky, A. S., Suarez, V., et al. (2005). Cocaine increases human immunodeficiency virus type 1 neuroinvasion through remodeling brain microvascular endothelial cells. J. Neurovirol. 11, 281–291. doi: 10.1080/13550280590952835
Fiala, M., Gan, X. H., Zhang, L., House, S. D., Newton, T., Graves, M. C., et al. (1998). “Cocaine enhances monocyte migration across the blood-brain barrier. Cocaine’s connection to aids dementia and vasculitis?,” in Advances in Experimental Medicine and Biology, ed. I. S. Grewal (New York, NY: Springer), 199–205. doi: 10.1007/978-1-4615-5347-2_22
Gach, K., Wyrêbska, A., Fichna, J., and Janecka, A. (2011). The role of morphine in regulation of cancer cell growth. Naunyn. Schmiedebergs Arch. Pharmacol. 384, 221–230. doi: 10.1007/s00210-011-0672-4
Gonçalves, J., Baptista, S., Martins, T., Milhazes, N., Borges, F., Ribeiro, C. F., et al. (2010). Methamphetamine-induced neuroinflammation and neuronal dysfunction in the mice hippocampus: preventive effect of indomethacin. Eur. J. Neurosci. 31, 315–326. doi: 10.1111/j.1460-9568.2009.07059.x
Haorah, J., Knipe, B., Gorantla, S., Zheng, J., and Persidsky, Y. (2007). Alcohol-induced blood-brain barrier dysfunction is mediated via inositol 1,4,5-triphosphate receptor (IP3R)-gated intracellular calcium release. J. Neurochem. 100, 324–336. doi: 10.1111/j.1471-4159.2006.04245.x
Hawkins, B. T., and Davis, T. P. (2005). The blood-brain barrier/neurovascular unit in health and disease. Pharmacol. Rev. 57, 173–185. doi: 10.1124/pr.57.2.4
He, J., and Crews, F. T. (2008). Increased MCP-1 and microglia in various regions of the human alcoholic brain. Exp. Neurol. 210, 349–358. doi: 10.1016/j.expneurol.2007.11.017
Hossain, M., Mazzone, P., Tierney, W., and Cucullo, L. (2011). In vitro assessment of tobacco smoke toxicity at the BBB: do antioxidant supplements have a protective role? BMC Neurosci. 12:92. doi: 10.1186/1471-2202-12-92
Hutamekalin, P., Farkas, A. E., Orbók, A., Wilhelm, I., Nagyoszi, P., Veszelka, S., et al. (2008). Effect of nicotine and polyaromtic hydrocarbons on cerebral endothelial cells. Cell Biol. Int. 32, 198–209. doi: 10.1016/j.cellbi.2007.08.026
Kariisa, M., Scholl, L., Wilson, N., Seth, P., and Hoots, B. (2019). Drug overdose deaths involving cocaine and psychostimulants with abuse potential — United States, 2003–2017. Morb. Mortal. Wkly. Rep. 68, 388–395. doi: 10.15585/mmwr.mm6817a3
Kousik, S. M., Napier, T. C., and Carvey, P. M. (2012). The effects of psychostimulant drugs on blood brain barrier function and neuroinflammation. Front. Pharmacol. 3:121. doi: 10.3389/fphar.2012.00121
Kumar, M. (2011). Cocaine and the blood-brain-barrier. Blood 117:2303. doi: 10.1182/blood-2010-12-324939
Leyton, M. (2007). Conditioned and sensitized responses to stimulant drugs in humans. Prog. Neuro-Psychopharmacol. Biol. Psychiatry 31, 1601–1613. doi: 10.1016/j.pnpbp.2007.08.027
Lippmann, E. S., Al-Ahmad, A., Palecek, S. P., and Shusta, E. V. (2013). Modeling the blood-brain barrier using stem cell sources. Fluids Barriers CNS 10:2. doi: 10.1186/2045-8118-10-2
Liu, W. Y., Wang, Z., Bin, Zhang, L. C., Wei, X., and Li, L. (2012). Tight junction in blood-brain barrier: an overview of structure, regulation, and regulator substances. CNS Neurosci. Ther. 18, 609–615. doi: 10.1111/j.1755-5949.2012.00340.x
Macianskiene, R., Gwanyanya, A., Vereecke, J., and Mubagwa, K. (2008). Inhibition of the magnesium-sensitive TRPM7-like channel in cardiac myocytes by nonhydrolysable GTP analogs: involvement of phosphoinositide metabolism. Cell. Physiol. Biochem. 22, 109–118. doi: 10.1159/000149788
Mahajan, S. D., Aalinkeel, R., Sykes, D. E., Reynolds, J. L., Bindukumar, B., Adal, A., et al. (2008). Methamphetamine alters blood brain barrier permeability via the modulation of tight junction expression: implication for HIV-1 neuropathogenesis in the context of drug abuse. Brain Res. 1203, 133–148. doi: 10.1016/j.brainres.2008.01.093
Malaplate-Armand, C., Becuwe, P., Ferrari, L., Masson, C., Dauça, M., Visvikis, S., et al. (2005). Effect of acute and chronic psychostimulant drugs on redox status, AP-1 activation and pro-enkephalin mRNA in the human astrocyte-like U373 MG cells. Neuropharmacology 48, 673–684. doi: 10.1016/j.neuropharm.2004.12.010
Manda, V. K., Mittapalli, R. K., Bohn, K. A., Adkins, C. E., and Lockman, P. R. (2010). Nicotine and cotinine increases the brain penetration of saquinavir in rat. J. Neurochem. 115, 1495–1507. doi: 10.1111/j.1471-4159.2010.07054.x
Mann, K., Agartz, I., Harper, C., Shoaf, S., Rawlings, R. R., Momenan, R., et al. (2001). Neuroimaging in alcoholism: ethanol and brain damage. Alcohol. Clin. Exp. Res. 25, 104S–109S. doi: 10.1111/j.1530-0277.2001.tb02383.x
Mazzone, P., Tierney, W., Hossain, M., Puvenna, V., Janigro, D., and Cucullo, L. (2010). Pathophysiological impact of cigarette smoke exposure on the cerebrovascular system with a focus on the blood-brain barrier: expanding the awareness of smoking toxicity in an underappreciated area. Int. J. Environ. Res. Public Health 7, 4111–4126. doi: 10.3390/ijerph7124111
Mende, M. A. (2019). Alcohol in the aging brain-the interplay between alcohol consumption, cognitive decline and the cardiovascular system. Front. Neurosci. 13:713. doi: 10.3389/fnins.2019.00713
Miller, D. S. (2010). Regulation of P-glycoprotein and other ABC drug transporters at the blood-brain barrier. Trends Pharmacol. Sci. 31, 246–254. doi: 10.1016/j.tips.2010.03.003
Muneer, P. M. A., Alikunju, S., Szlachetka, A. M., and Haorah, J. (2012). The mechanisms of cerebral vascular dysfunction and neuroinflammation by MMP-mediated degradation of VEGFR-2 in alcohol ingestion. Arterioscler. Thromb. Vasc. Biol. 32, 1167–1177. doi: 10.1161/ATVBAHA.112.247668
Naik, P., Fofaria, N., Prasad, S., Sajja, R. K., Weksler, B., Couraud, P. O., et al. (2014). Oxidative and pro-inflammatory impact of regular and denicotinized cigarettes on blood brain barrier endothelial cells: is smoking reduced or nicotine-free products really safe? BMC Neurosci. 15:51. doi: 10.1186/1471-2202-15-51
Niu, F., Liao, K., Hu, G., Sil, S., Callen, S., Guo, M. L., et al. (2019). Cocaine-induced release of CXCL10 from pericytes regulates monocyte transmigration into the CNS. J. Cell Biol. 218, 700–721. doi: 10.1083/jcb.201712011
Obermeier, B., Daneman, R., and Ransohoff, R. M. (2013). Development, maintenance and disruption of the blood-brain barrier. Nat. Med. 19, 1584–1596. doi: 10.1038/nm.3407
Oh, H. G., Chun, Y. S., Kim, Y., Youn, S. H., Shin, S., Park, M. K., et al. (2012). Modulation of transient receptor potential melastatin related 7 channel by presenilins. Dev. Neurobiol. 72, 865–877. doi: 10.1002/dneu.22001
O’Shea, E., Urrutia, A., Green, A. R., and Colado, M. I. (2014). Current preclinical studies on neuroinflammation and changes in blood-brain barrier integrity by MDMA and methamphetamine. Neuropharmacology 87, 125–134. doi: 10.1016/j.neuropharm.2014.02.015
Pardridge, W. M. (2012). Drug transport across the blood-brain barrier. J. Cereb. Blood Flow Metab. 32, 1959–1972. doi: 10.1038/jcbfm.2012.126
Park, M., Kim, H. J., Lim, B., Wylegala, A., and Toborek, M. (2013). Methamphetamine-induced occludin endocytosis is mediated by the Arp2/3 complex-regulated actin rearrangement. J. Biol. Chem. 288, 33324–33334. doi: 10.1074/jbc.M113.483487
Paulson, J. R., Roder, K. E., McAfee, G., Allen, D. D., Van Der Schyf, C. J., and Abbruscato, T. J. (2006). Tobacco smoke chemicals attenuate brain-to-blood potassium transport mediated by the Na,K,2Cl-cotransporter during hypoxia-reoxygenation. J. Pharmacol. Exp. Ther. 316, 248–254. doi: 10.1124/jpet.105.090738
Ramirez, S. H., Potula, R., Fan, S., Eidem, T., Papugani, A., Reichenbach, N., et al. (2009). Methamphetamine disrupts blood-brain barrier function by induction of oxidative stress in brain endothelial cells. J. Cereb. Blood Flow Metab. 29, 1933–1945. doi: 10.1038/jcbfm.2009.112
Riley, A. L., Kearns, D., Hargrave, S., and Davidson, T. (2017). Cocaine increases permeability of blood-brain-barrier in the hippocampus and striatum: implications for drug use and abuse. Drug Alcohol Depend. 171:e177. doi: 10.1016/j.drugalcdep.2016.08.485
Rochfort, K. D., Collins, L. E., Murphy, R. P., and Cummins, P. M. (2014). Downregulation of blood-brain barrier phenotype by proinflammatory cytokines involves NADPH oxidase-dependent ROS generation: consequences for interendothelial adherens and tight junctions. PLoS ONE 9:e101815. doi: 10.1371/journal.pone.0101815
Rodriguez-Gaztelumendi, A., Alvehus, M., Andersson, T., and Jacobsson, S. O. P. (2011). Comparison of the effects of nicotine upon the transcellular electrical resistance and sucrose permeability of human ECV304/rat C6 co-cultures and human CaCo 2 cells. Toxicol. Lett. 207, 1–6. doi: 10.1016/j.toxlet.2011.08.014
Rom, S., Zuluaga-Ramirez, V., Dykstra, H., Reichenbach, N. L., Ramirez, S. H., and Persidsky, Y. (2015). Poly(ADP-ribose) polymerase-1 inhibition in brain endothelium protects the blood-brain barrier under physiologic and neuroinflammatory conditions. J. Cereb. Blood Flow Metab. 35, 28–36. doi: 10.1038/jcbfm.2014.167
Ronaldson, P. T., and Davis, T. P. (2015). Targeting transporters: promoting blood-brain barrier repair in response to oxidative stress injury. Brain Res. 1623, 39–52. doi: 10.1016/j.brainres.2015.03.018
Rosado, J. A. (2016). Sigma-1 receptors: a new pathway for the modulation of store-operated calcium entry. Biochem. J. 473, e9–e10. doi: 10.1042/BJ20151144
Rubio-Araiz, A., Porcu, F., Pérez-Hernández, M., García-Gutiérrez, M. S., Aracil-Fernández, M. A., Gutierrez-López, M. D., et al. (2017). Disruption of blood–brain barrier integrity in postmortem alcoholic brain: preclinical evidence of TLR4 involvement from a binge-like drinking model. Addict. Biol. 22, 1103–1116. doi: 10.1111/adb.12376
Rudd, R. A., Aleshire, A., Zibbell, J. E., and Matthew Gladden, R. (2016). Increases in Drug and Opioid Overdose Deaths — United States, 2000–2014. 1378–82. Available online at: https://www.cdc.gov/mmwr/preview/mmwrhtml/mm6450a3.htm (accessed January 2, 2020).
Sajja, R. K., Rahman, S., and Cucullo, L. (2016). Drugs of abuse and blood-brain barrier endothelial dysfunction: a focus on the role of oxidative stress. J. Cereb. Blood Flow Metab. 36, 539–554. doi: 10.1177/0271678X15616978
Seleman, M., Chapy, H., Cisternino, S., Courtin, C., Smirnova, M., Schlatter, J., et al. (2014). Impact of P-glycoprotein at the blood-brain barrier on the uptake of heroin and its main metabolites: behavioral effects and consequences on the transcriptional responses and reinforcing properties. Psychopharmacology (Berl) 231, 3139–3149. doi: 10.1007/s00213-014-3490-9
Shah, A., Silverstein, P. S., Singh, D. P., and Kumar, A. (2012). Involvement of metabotropic glutamate receptor 5, AKT/PI3K Signaling and NF-κB pathway in methamphetamine-mediated increase in IL-6 and IL-8 expression in astrocytes. J. Neuroinflamm. 9:52. doi: 10.1186/1742-2094-9-52
Sharma, H. S., Muresanu, D., Sharma, A., and Patnaik, R. (2009). Cocaine-induced breakdown of the blood-brain barrier and neurotoxicity. Int. Rev. Neurobiol. 88, 297–334. doi: 10.1016/S0074-7742(09)88011-2
Sil, S., Niu, F., Tom, E., Liao, K., Periyasamy, P., and Buch, S. (2019). Cocaine mediated neuroinflammation: role of dysregulated autophagy in pericytes. Mol. Neurobiol. 56, 3576–3590. doi: 10.1007/s12035-018-1325-0
Snively, T. A., Ahijevych, K. L., Bernhard, L. A., and Wewers, M. E. (2000). Smoking behavior, dysphoric states and the menstrual cycle: results from single smoking sessions and the natural environment. Psychoneuroendocrinology 25, 677–691. doi: 10.1016/S0306-4530(00)00018-4
Srinivasan, B., Kolli, A. R., Esch, M. B., Abaci, H. E., Shuler, M. L., and Hickman, J. J. (2015). TEER measurement techniques for in vitro barrier model systems. J. Lab. Autom. 20, 107–126. doi: 10.1177/2211068214561025
Stefano, G. B., Hartman, A., Bilfinger, T. V., Magazine, H. I., Liu, Y., Casares, F., et al. (1995). Presence of the μ3 opiate receptor in endothelial cells: coupling to nitric oxide production and vasodilation. J. Biol. Chem. 270, 30290–30293. doi: 10.1074/jbc.270.51.30290
Tega, Y., Yamazaki, Y., Akanuma, S., Kubo, Y., and Hosoya, K. (2018). Impact of nicotine transport across the blood–brain barrier: carrier-mediated transport of nicotine and interaction with central nervous system drugs. Biol. Pharm. Bull. 41, 1330–1336. doi: 10.1248/bpb.b18-00134
Terner, J. M., and de Wit, H. (2006). Menstrual cycle phase and responses to drugs of abuse in humans. Drug Alcohol Depend. 84, 1–13. doi: 10.1016/j.drugalcdep.2005.12.007
Toborek, M., Seelbach, M. J., Rashid, C. S., András, I. E., Chen, L., Park, M., et al. (2013). Voluntary exercise protects against methamphetamine-induced oxidative stress in brain microvasculature and disruption of the blood-brain barrier. Mol. Neurodegener. 8:22. doi: 10.1186/1750-1326-8-22
Tournier, N., Decleves, X., Saubamea, B., Scherrmann, J.-M., and Cisternino, S. (2011). Opioid Transport by ATP-Binding Cassette Transporters at the Blood-Brain Barrier: implications for Neuropsychopharmacology. Curr. Pharm. Des. 17, 2829–2842. doi: 10.2174/138161211797440203
United Nations Office on Drugs and Crime (2016). Strengthening the State Service on Drug Control of the Kyrgyz Republic. Vienna: United Nations Office on Drugs and Crime. Available online at: www.unodc.org (accessed February 24, 2020).
Van Itallie, C. M., and Anderson, J. M. (2014). Architecture of tight junctions and principles of molecular composition. Semin. Cell Dev. Biol. 36, 157–165. doi: 10.1016/j.semcdb.2014.08.011
Wegener, N., and Koch, M. (2009). Behavioural disturbances and altered Fos protein expression in adult rats after chronic pubertal cannabinoid treatment. Brain Res. 1253, 81–91. doi: 10.1016/j.brainres.2008.11.081
Wilhelm, I., and Krizbai, I. A. (2014). In vitro models of the blood-brain barrier for the study of drug delivery to the brain. Mol. Pharm. 11, 1949–1963. doi: 10.1021/mp500046f
Willuhn, I., Wanat, M. J., Clark, J. J., and Phillips, P. E. M. (2010). Dopamine signaling in the nucleus accumbens of animals self-administering drugs of abuse. Curr. Top. Behav. Neurosci. 3, 29–71. doi: 10.1007/7854_2009_27
Xue, Y., He, J. T., Zhang, K. K., Chen, L. J., Wang, Q., and Xie, X. L. (2019). Methamphetamine reduces expressions of tight junction proteins, rearranges F-actin cytoskeleton and increases the blood brain barrier permeability via the RhoA/ROCK-dependent pathway. Biochem. Biophys. Res. Commun. 509, 395–401. doi: 10.1016/j.bbrc.2018.12.144
Yang, C., Hawkins, K. E., Doré, S., and Candelario-Jalil, E. (2019). Neuroinflammatory mechanisms of blood-brain barrier damage in ischemic stroke. Am. J. Physiol. – Cell Physiol. 316, C135–C153. doi: 10.1152/ajpcell.00136.2018
Yang, J., Reilly, B. G., Davis, T. P., and Ronaldson, P. T. (2018). Modulation of opioid transport at the blood-brain barrier by altered atp-binding cassette (ABC) transporter expression and activity. Pharmaceutics 10:E192. doi: 10.3390/pharmaceutics10040192
Yang, L., Yao, H., Chen, X., Cai, Y., Callen, S., and Buch, S. (2016). Role of sigma receptor in cocaine-mediated induction of glial fibrillary acidic protein: implications for HAND. Mol. Neurobiol. 53, 1329–1342. doi: 10.1007/s12035-015-9094-5
Yao, H., Duan, M., and Buch, S. (2011). Cocaine-mediated induction of platelet-derived growth factor: implication for increased vascular permeability. Blood 117, 2538–2547. doi: 10.1182/blood-2010-10-313593
Yao, H., Yang, Y., Kim, K. J., Bethel-Brown, C., Gong, N., Funa, K., et al. (2010). Molecular mechanisms involving sigma receptor-mediated induction of MCP-1: implication for increased monocyte transmigration. Blood 115, 4951–4962. doi: 10.1182/blood-2010-01-266221
Zhang, Y., Lv, X., Bai, Y., Zhu, X., Wu, X., Chao, J., et al. (2015). Involvement of sigma-1 receptor in astrocyte activation induced by methamphetamine via up-regulation of its own expression. J. Neuroinflammation 12:29. doi: 10.1186/s12974-015-0250-7
Keywords: blood-brain barrier, cocaine, methamphetamine, morphine, heroin, nicotine
Citation: Pimentel E, Sivalingam K, Doke M and Samikkannu T (2020) Effects of Drugs of Abuse on the Blood-Brain Barrier: A Brief Overview. Front. Neurosci. 14:513. doi: 10.3389/fnins.2020.00513
Received: 25 February 2020; Accepted: 24 April 2020;
Published: 21 May 2020.
Edited by:
Monique Stins, Johns Hopkins University, United StatesReviewed by:
Shilpa Jagdeep Buch, University of Nebraska Medical Center, United StatesCopyright © 2020 Pimentel, Sivalingam, Doke and Samikkannu. This is an open-access article distributed under the terms of the Creative Commons Attribution License (CC BY). The use, distribution or reproduction in other forums is permitted, provided the original author(s) and the copyright owner(s) are credited and that the original publication in this journal is cited, in accordance with accepted academic practice. No use, distribution or reproduction is permitted which does not comply with these terms.
*Correspondence: Kalaiselvi Sivalingam, c2l2YWxpbmdhbUBwaGFybWFjeS50YW1oc2MuZWR1; Thangavel Samikkannu, dGhhbmdhdmVsQHRhbXUuZWR1; dGhhbmdhdmVsQHRhbWhzYy5lZHU=
Disclaimer: All claims expressed in this article are solely those of the authors and do not necessarily represent those of their affiliated organizations, or those of the publisher, the editors and the reviewers. Any product that may be evaluated in this article or claim that may be made by its manufacturer is not guaranteed or endorsed by the publisher.
Research integrity at Frontiers
Learn more about the work of our research integrity team to safeguard the quality of each article we publish.