- 1Institute of Cardiological Research, University of Buenos Aires, National Research Council (ININCA-UBA-CONICET), Buenos Aires, Argentina
- 2Centro de Investigaciones en Psicología y Psicopedagogía (CIPP), Pontificia Universidad Católica Argentina, Buenos Aires, Argentina
- 3Departamento de Biología, Universidad Argentina John F. Kennedy (UAJK), Buenos Aires, Argentina
Perinatal asphyxia (PA) is a clinical condition brought by a birth temporary oxygen deprivation associated with long-term damage in the corpus striatum, one of the most compromised brain areas. Palmitoylethanolamide (PEA) is a neuromodulator well known for its protective effects in brain injury models, including PA, albeit not deeply studied regarding its particular effects in the corpus striatum following PA. Using Bjelke et al. (1991) PA model, full-term pregnant rats were decapitated, and uterus horns were placed in a water bath at 37°C for 19 min. One hour later, the pups were injected with PEA 10 mg/kg s.c., and placed with surrogate mothers. After 30 days, the animals were perfused, and coronal striatal sections were collected to analyze protein-level expression by Western blot and the reactive area by immunohistochemistry for neuron markers: phosphorylated neurofilament-heavy/medium-chain (pNF-H/M) and microtubule-associated protein-2 (MAP-2), and the astrocyte marker, glial fibrillary acidic protein (GFAP). Results indicated that PA produced neuronal damage and morphological changes. Asphyctic rats showed a decrease in pNF-H/M and MAP-2 reactive areas, GFAP+ cells number, and MAP-2 as well as pNF-H/M protein expression in the striatum. Treatment with PEA largely restored the number of GFAP+ cells. Most important, it ameliorated the decrease in pNF-H/M and MAP-2 reactive areas in asphyctic rats. Noticeably, PEA treatment reversed the decrease in MAP-2 protein expression and largely prevented PA-induced decrease in pNF-H/M protein expression. PA did not affect the GFAP protein level. Treatment with PEA attenuated striatal damage induced by PA, suggesting its therapeutic potential for the prevention of neurodevelopmental disorders.
Highlights:
- Palmitoylethanolamide ameliorated neuronal damage induced by PA in rat striatum.
- Perinatal asphyxia did not alter the protein expression of GFAP in rat striatum.
- Palmitoylethanolamide largely reversed the decrease in the number of GFAP+ cells induced by PA in rat striatum.
Introduction
Perinatal asphyxia (PA) is a clinical condition characterized by a transient disruption of oxygen availability, followed by metabolism deficits during birth that increases the risk of mortality and morbidity in neonates worldwide (Capani et al., 2001; Romero et al., 2015). Nearly 2–6/1,000 live births are estimated experiencing PA, rising to 10 times higher in developing countries (McGuire, 2007; Morales et al., 2011). Depending on the severity, the time elapsed from the insult, and the degree of fetal maturity, several biological processes are triggered after PA, like a decrease in the expression of synaptic proteins, cytoskeletal alterations, and protein aggregation (Herrera et al., 2017a). These events may eventually impair cellular function and lead to death and a proinflammatory response as secondary injury (Herrera et al., 2017a). Along with the hippocampus, the corpus striatum is one of the most vulnerable brain structures to PA, hypoxia (HI), and neurodegenerative injuries, partly due to its critical location in the mesolimbic and the corticostriatal circuits (Calabresi et al., 2000; Looi and Walterfang, 2013; Rocha-Ferreira and Hristova, 2016). Due to the neuronal loss and synaptic structure alterations induced by PA in these brain regions (Herrera et al., 2017a), 25% of the surviving newborns develop several neurodevelopmental abnormalities, including intellectual disability and autism spectrum disorders (Schieve et al., 2014; Modabbernia et al., 2016), attention-deficit/hyperactivity disorder (Perna and Cooper, 2012), schizophrenia (Pugliese et al., 2019), and neurodegenerative disorders (Gupta et al., 2018).
Previous studies from our group using an animal model of PA (Bjelke et al., 1991) showed that PA-induced alterations are characterized by increased astrogliosis in the hippocampus and dorsal striatum (Cebral et al., 2006; Saraceno et al., 2010; Holubiec et al., 2017), reduced microtubule-associated protein 2 (MAP-2) level, and alterations in the phosphorylation of high- (200 kDa) and medium- (160 kDa) molecular-weight neurofilaments (pNF-H/M) in the hippocampus in 120-day-old rats (P120, postnatal day 120) (Saraceno et al., 2010) or 30-day-old rats (P30, postnatal day 30) (Herrera et al., 2018). Importantly, MAP-2 is predominantly localized in neuronal dendrites of the caudate, hippocampus, cerebral cortex, and cerebellum of rodents, and its absence or diminished expression is considered as a marker of neurodegenerative disorders (Popp et al., 2009; Saraceno et al., 2012a; Romero et al., 2017). Neurofilaments (NFs) are highly phosphorylated proteins relevant to neuronal structure and function. Changes in phosphorylation alter their antigenicity and are considered a pathological hallmark of several neurodegenerative diseases (Mink and Johnston, 2000). Another biological landmark of neurodegeneration is the glial fibrillary acidic protein (GFAP), abundantly present in astrocytes in the entire central nervous system. Astroglial cells respond to brain injury and other neurological perturbations undergoing “reactive astrogliosis,” a process whereby they develop cellular hypertrophy with a concomitant increase in GFAP expression, and glial cell proliferation, indicative of neuroinflammatory processes involved in several neurodegenerative diseases (Villapol et al., 2008; Yang and Wang, 2015). There is an urgent need to improve our understanding of PA, which has currently no effective treatment and to advance in the development of effective pharmacological therapies based on specific biological targets.
One month after a severe, 20-min PA, the rats developed an alteration in the endocannabinoid system expression and related acylethanolamide pathways (Blanco et al., 2015). Palmitoylethanolamide (PEA) is an endogenous lipidic neuromodulator related to endocannabinoids, acting as a peroxisome proliferator-activated receptor alpha (PPARα) or G protein-coupled receptor 55 (GPR55) agonist that elicits anti-inflammatory, neuroprotective, and restorative properties (Mattace Raso et al., 2014; Petrosino and Di Marzo, 2017) in vitro (Avagliano et al., 2016), in animal models of both neurodegeneration (Esposito et al., 2011, 2012, 2014; Herrera et al., 2016) and hypoxia-ischemia (HI) (Fernandez-Lopez et al., 2013; England et al., 2015), and in human patients as well (Orefice et al., 2016). Interestingly, one study reported that natives from Puno at about 3,830 m above sea level in Peru, having high hemoglobinemia and low oxygen saturation, also exhibited a high plasma concentration of PEA (Alarcon-Yaquetto et al., 2017). This suggests that PEA might be involved in long-term adaptation to chronic high-altitude hypoxia, likely preventing the development of chronic mountain sickness (Alarcon-Yaquetto et al., 2017). Compared with other acylethanolamides, PEA showed stronger anti-inflammatory effects reducing PA-induced astrogliosis in P30 rats’ hippocampus (Holubiec et al., 2018) and preventing cortical neurons from HI-induced cell death (Portavella et al., 2018). Moreover, PEA treatment during the first hour of life in asphyctic P30 rats reduced hippocampal histological degeneration and prevented the anxiety-like behavior induced by PA (Herrera et al., 2018). However, the protective effects of PEA in the striatum of asphyctic rats have not been addressed. We hypothesized that PEA might counteract PA-induced neuronal and glial striatal damage in P30 rats. Therefore, this work aimed to investigate: (i) whether it is possible detecting neuronal cytoskeletal and glial alterations in the striatum of asphyctic P30 rats and (ii) if PEA administration within the first hour of life could ameliorate these alterations in P30 rats.
Materials and Methods
Animals
Sprague Dawley pregnant rats (mothers, n = 20) were obtained from the central vivarium at the School of Veterinary Sciences, University of Buenos Aires. Rats were maintained at 21 ± 2°C and 65% ± 5% air humidity with free access to food and water, under a 12:12-h light/dark cycle (lights on 7:00 a.m.). Fifty-three pups (and 20 mothers) were used in the study. Each animal was used only once, and all efforts were made to minimize the suffering of the animals and reduce the number of animals used. All procedures were performed by the National Institute of Health Guide for the Care and Use of Laboratory Animals (Animal Welfare Assurance, A-3033-01/protocol #S01084) and were approved by the Ethics Committee of our Institution (CICUAL #4091/04).
Induction of PA
Fifty-three male rat pups were subjected to PA using an experimental model (Bjelke et al., 1991), which produces moderate to severe PA (Van de Berg et al., 2003; Galeano et al., 2011) as we described previously (Capani et al., 2003, 2009; Saraceno et al., 2010, 2012a,b; Blanco et al., 2015; Holubiec et al., 2017; Herrera et al., 2018). The murine model of PA, originally developed by Bjelke et al. (1991), has been widely used (Barkhuizen et al., 2017; Herrera et al., 2017b) and accepted to mimic this condition (Capani et al., 1997, 2003, 2009; Boksa and El-Khodor, 2003; Saraceno et al., 2010, 2012a,b; Strackx et al., 2010; Muñiz et al., 2014; Romero et al., 2015; Wakuda et al., 2015; Herrera et al., 2018). The Bjelke model presents several advantages such as follows: (a) asphyxia is produced at birth (Capani et al., 2009); (b) acidosis, hypercapnia, and hypoxia are present in the whole body, replicating a global asphyxia, which is the most common type (Strackx et al., 2010); (c) it is non-invasive, avoiding the effects of surgical procedures; and (d) since hypoxia is produced in the whole body, it affects both brain hemispheres, which makes this model more suitable for behavioral studies (Arteni et al., 2010). The control group was represented by non-fostered vaginally delivered pups, mimicking the clinical situation (Herrera et al., 2018).
Neuroprotection Protocol
Fifty-three male rat pups received the respective treatment within the first hour of life. PEA (0879/10, Tocris Bioscience, Bristol, United Kingdom) was dissolved in vehicle (VHI), a solution containing 1:1:8 dimethyl sulfoxide, Tween 80, and NaCl and administered by subcutaneous injection in a volume of 10 mg/kg (Herrera et al., 2018). This dose has previously shown to be effective in animal models of experimental spinal cord injury (Genovese et al., 2008), 1-methyl-4-phenyl-1,2,3,6-tetrahydropyridine (MPTP)-inducedmodel of Parkinson’s disease (Esposito et al., 2012), lipopolysaccharide-induced neuroinflammation (Sayd et al., 2014), hippocampal damage and behavioral disturbances induced by PA (Herrera et al., 2018), and cognitive function impairment and astrogliosis induced by neonatal anoxia/ischemia in rats (Holubiec et al., 2018). A preparation similar to that used in this study inhibited the response of mesolimbic dopamine neurons to nicotine in the ventral tegmental area, suggesting the ability of PEA to cross the blood-brain barrier in rats (Melis et al., 2008). Control groups received the respective vehicle (VHI). The different groups were marked and mixed in litters with a surrogate mother. After weaning, rats were housed in cages of three to four animals from the same group. Cesarean controls were not used since previous studies revealed that this group did not present significant differences with vaginal controls (Galeano et al., 2011; Blanco et al., 2015). The selection of male animals is supported by the fact that estrogens exert neuroprotective properties (Saraceno et al., 2010). Four experimental groups were studied: rats subjected to PA and injected with VHI (PA–VHI group, n = 15), rats born vaginally (controls–CTL) and injected with VHI (CTL–VHI group, n = 13), rats subjected to PA and injected with PEA (PA+PEA group, n = 18), and rats born vaginally and injected with PEA (CTL+PEA group, n = 7). Four animals were used as controls each for immunolabeling and WB assays.
Tissue Fixation and Immunohistochemistry
We evaluated PA-induced dorsal striatal damage in rats (n = 16) at P30. This age fairly resembles 4–11 human years (Semple et al., 2013), when the first signs of neurodevelopmental disorders appear (Herrera-Marschitz et al., 2014). We studied the dorsal neostriatum for its high sensitivity to hypoxic injury focusing on the axon bundles found either in the matrix or the striosome (Capani et al., 1997; Gerfen, 1989). Four animals per group (four replicates) were used. Three sections were immunostained for each analyzed brain. Rats were anesthetized with ketamine 40 mg/kg i.p. and xylazine 5 mg/kg i.p. and intracardially perfused with 4% paraformaldehyde in a 0.1 M phosphate buffer (pH 7.4) (Saraceno et al., 2016). The brains were immediately removed, post-fixed in the 4% paraformaldehyde in 0.1 M phosphate buffer (pH 7.4) solution at room temperature for 2 h, and immersed in a 0.1 M phosphate buffer (pH 7.4) overnight at 4°C. Coronal dorsal striatum sections (sections at the coronal plane, 50 μm thick) were cut using a Vibratome (VT 1000 S, Leica Microsystems, Wetzlar, Germany). This well-set technique using a 50-micron-thick vibratome section avoids embedding the tissue in paraffin with the resulting decrease in the antigenicity, gaining label specificity.
Immunohistochemistry was performed on free-floating sections under moderate shaking. Before staining, the sections were incubated 10 min in 3% hydrogen peroxide to quench endogenous peroxidases. After three washing steps in 0.1 M phosphate buffer (pH 7.4), non-specific antibody binding sites were blocked with using 0.3% normal goat serum. Free-floating sections were incubated overnight at 4°C with anti-MAP-2 (1:250, polyclonal rabbit-IgG; ab32454, Abcam, Cambridge, United Kingdom); anti-pNF-H/M, which recognizes the KSP repeats of the phosphorylated form (1:500, monoclonal mouse-IgG; MAB1592, Millipore, Burlington, VT, United States); or anti-GFAP (1:200, monoclonal rabbit-IgG; Cell-Marque, Sigma-Aldrich Company, Code. EP672Y). After several washes, sections were incubated for 1 h at room temperature with secondary antibodies (biotinylated anti-mouse-IgG, 1:500, BA9200, or biotinylated anti-rabbit-IgG, 1:500, BA-1000; Vector, Burlingame, VT, United States). The streptavidin/horseradish peroxidase detection system (1:500, K0609; Dako, Santa Clara, CA, United States) was used for antigen staining according to the manufacturer’s recommendations. Sections were incubated with the substrate diaminobenzidine (D3939; Sigma-Aldrich Company, St. Louis, MO, United States) for 2 min at room temperature. Light microscopic images were obtained using a Leica microscope (Herrera et al., 2018).
Morphometric Analysis
The reactive area for pNF-H/M and MAP-2 was estimated using the Image J program (Image J 1.41o; NIH, United States) as described previously, and expressed as a percentage (Herrera et al., 2018). One hundred fifty by 150 μm was sampled in each photo to estimate the percentage of reactive area for pNF-H/M and MAP-2 using the Image J program (Image J 1.41o; NIH, United States). We analyzed the dorsal striatum because it is one of the most affected areas by PA insult (Holubiec et al., 2017). The number of GFAP immunoreactive astrocytes was manually estimated in the dorsal striatum. Eighty counting frames were assessed per animal. A blind observer selected five fields for each sector from three sections of the dorsal striatum. Triplicates were performed to estimate the percentage of the reactive area and the number of GFAP immunoreactive astrocytes. The NFs and microtubules were expressed as the percentage of the relative area since they cannot be individually counted. Conversely, GFAP was expressed as the absolute value since GFAP labeling allows for visualization per astrocyte and can be counted.
Western Blot
Western blot analysis was performed using four animals per treatment (n = 16, four replicates) by triplicate per brain. The rats were euthanized by decapitation, and the corpus striatum was dissected and homogenized in ice-cold lysis buffer, containing 10 mM Tris–HCl at pH 7.4, 10 mM NaCl, 3 mM MgCl2, 0.1% Triton X-100, and protease inhibitors. Tissues were thawed on ice and centrifuged at 14,000 rpm for 15 min at 4°C. The protein concentration was analyzed using the Bradford solution (500-0201; Bio-Rad, Richmond, CA, United States) and bovine serum albumin as the standard (Herrera et al., 2018). Total protein (80 μg) was diluted in buffer [0.3 M Tris–HCl (pH 7), 5% glycerol, 5% SDS, 1 mM EDTA, 0.1% bromophenol blue] and subjected to sodium dodecyl sulfate-polyacrylamide gel electrophoresis. Polyvinylidene difluoride membranes containing the transferred proteins were blocked with 5% non-fat milk powder and 1% bovine serum albumin in a Tris-buffered saline solution containing 0.05% Tween 20. The membranes were incubated overnight at 4°C with the following primary antibodies: anti-MAP-2 (1:1,000, polyclonal rabbit-IgG; ab32454, Abcam, Cambridge, United Kingdom), anti-PNF-H/M (1:500, monoclonal mouse-IgG; MAB1592, Millipore, Burlington, VT, United States), or anti-GFAP (1:1,000, monoclonal mouse-IgG; SC-33673, Santa Cruz Biotechnology), and anti-glyceraldehyde-3-phosphate dehydrogenase (1:3,000, rabbit-IgG, G9545; Sigma-Aldrich Company, St. Louis, MO, United States) as the loading control. Next, the membranes were incubated with rabbit and mouse, secondary horseradish peroxidase-conjugated antibodies (1:3,000, 170-6515, and 170-6516, respectively; Bio-Rad, Richmond, CA, United States) for 1 h at room temperature. The protein bands were detected using an enhanced chemiluminescence Western blotting analysis system (clarity Western enhanced chemiluminescence substrate, 1705061; Bio-Rad, Richmond, CA, United States). The films were scanned, and the optical density of protein bands was quantified using Gel-Pro Analyzer 3.1.00.00 (Media Cybernetics).
Statistical Analysis
Results are expressed as means ± SEM. Normal distribution was checked using the Shapiro–Wilk test. The data were submitted to a two-way ANOVA. Factors were the birth condition (PA or CTL) and treatment (PEA or VHI). When birth condition-treatment interaction was significant, multiple comparisons were performed using Tukey’s t-test. A p < 0.05 was conventionally set as the significance level. Statistical analysis was carried out using GraphPad PrismTM 7 software.
Results
Effect of PEA Treatment on PA-Induced Alterations in pNF-H/M Tissue Immunostaining and Protein Expression at P30
The phosphorylation of pNF-H/M, a measure of axonal dysfunction and degeneration, was analyzed by immunostaining at P30 in the rat dorsal striatum. Figure 1A shows PA-induced morphological changes in a representative striatal section immunostained for pNF-H/M. The percentage of reactive area for pNF-H/M was dependent on birth condition and treatment [F(1,8) = 74,552, p < 0.0001; F(1,8) = 15,608, p < 0.0001, respectively], showing between-interaction [F(1,8) = 15,639, p < 0.0001]. Post hoc analysis revealed that the reactive area for pNF-H/M decreased due to PA (PA–VHI) (p < 0.0001, Figure 1B), and that it was largely yet not completely attenuated by PEA treatment (PA+PEA) (p < 0.0001, Figure 1B). Consistently, at P30, striatal protein expression for pNF-H/M was dependent on birth condition and treatment [F(1,8) = 122.3, p < 0.0001; F(1,8) = 8.002, p = 0.0222, respectively], also showing between-interaction [F(1,8) = 10.18, p = 0.0128]. Post hoc analysis confirmed a decrease in pNF-H/M protein expression in the PA–VHI group (p < 0.0001), largely yet not completely ameliorated by PEA treatment (PA+PEA) (p = 0.0119, Figure 1C) (p = 0.0024, Figure 1C). Finally, pNF-H/M reactive area and protein level were not different between the control group treated with either vehicle or PEA [CTL–VHI vs. CTL+PEA, p = 0.9997 (reactive area); p > 0.9937 (protein level); Figures 1B,C]. For more detail, please see Supplementary Figures 1 and 2.
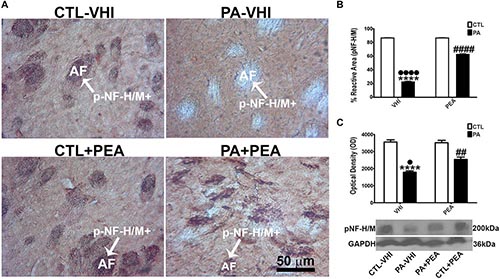
Figure 1. Phosphorylated neurofilament-heavy/medium-chain (pNF-H/M) immunostaining and protein level in the rat striatum in the experimental groups. (A) Representative images of dorsal striatum immunostained for pNF-H/M. The white arrow indicates the positive immunostaining area for pNF-H/M; AF indicates the axonal fascicles. Strictly dorsal striatum was used for immunohistochemistry due to its high vulnerability to hypoxia. Scale bar: 50 μm. (B) Percentage of pNF-H/M reactive area. (C) Optical density of bands showing pNF-H/M protein expression level. Bars and error bars represent the mean ± SEM. Two-way ANOVA followed by Tukey’s post hoc test. ∗∗∗∗p < 0.0001, PA–VHI vs. CTL–VHI, ••••p < 0.0001, and •p < 0.05, PA–VHI vs. PA+PEA; ####p < 0.0001 and ##p < 0.005, PA+PEA vs. CTL–VHI. PEA did not affect either pNF-H/M immunostaining or protein expression as there was no difference between CTL+PEA and CTL–VHI groups. CTL–VHI, control rats treated with vehicle; PA–VHI, rats subjected to PA and treated with vehicle; PA+PEA, rats subjected to PA and PEA treatment; CTL+PEA, control rats treated with PEA; PEA, palmitoylethanolamide.
Effects of PEA Treatment in Dendritic Cytoskeleton Alterations and MAP-2 Tissue Immunostaining and Protein Expression at P30 After PA
Since PA affected neuronal cytoskeleton, we also studied morphological changes through immunostaining of the dendrite-specific marker MAP-2 in the rat dorsal striatum at P30. Morphological changes were observed due to PA (PA–VHI) (Figure 2A). The MAP-2 reactive area was dependent on both birth condition and treatment [F(1,8) = 16,629, p < 0.0001; F(1,8) = 17,810, p < 0.0001, respectively]. Birth condition-treatment interaction was also significant [F(1,8) = 17,711, p < 0.0001]. Post hoc analysis revealed a decrease in the percentage of MAP-2 reactive area in the PA–VHI group compared with the control group (CTL–VHI) (p < 0.0001, Figure 2B), which was fully reversed by PEA treatment (PA+PEA) (p < 0.0001, Figure 2B) reaching control values (p = 0.0515 for reactive area; p = 0.9974 for protein level, Figure 2C). The analysis of MAP-2 protein expression in the rat striatum at P30 confirmed these findings. The protein level of MAP-2 was dependent on birth condition and treatment [F(1,8) = 40.14, p = 0.0002; F(1,8) = 36.83, p = 0.0003, respectively], with significant between-interaction [F(1,8) = 36.09, p = 0.0003]. The post hoc analysis confirmed that PA (PA–VHI group) decreased MAP-2 protein level (p < 0.001, Figure 2C), which was fully restored to control values by PEA treatment (PA+PEA) (p < 0.001, Figure 2C), reaching control values (p = 0.9974, Figure 2C). Finally, MAP-2 reactive area protein level was not different between the control group treated with either vehicle or PEA (CTL–VIH vs. CTL+PEA p = 0.9932 for the reactive area, p > 0.9999 for protein level) (Figures 2B,C, respectively).
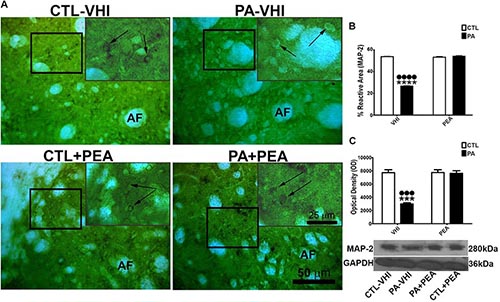
Figure 2. Microtubule-associated protein (MAP-2) immunostaining and protein level in the rat striatum in the experimental groups. (A) Representative images of the dorsal striatum immunostained for MAP-2. The figure shows MAP-2 immunostaining in the dorsal striatum; AF indicates the axonal fascicles. Scale bar: 50 μm. The main-image marked rectangular areas are shown magnified in the corresponding upper right margin. The black arrow indicates the positive immunostaining area for MAP-2. Scale bar: 25 μm. (B) Percentage of MAP-2 reactive area. (C) Optical density of bands showing for MAP-2 protein expression level. Bars and error bars represent the mean ± SEM. Two-way ANOVA followed by Tukey’s post hoc test. ∗∗∗∗p < 0.0001 and ∗∗∗p < 0.001, PA–VHI vs. CTL–VHI; ••••p < 0.0001 and •••p < 0.001, PA–VHI vs. PA+PEA. PEA had no effect on either MAP-2 immunostaining or protein expression level as there was no difference between CTL+PEA and CTL–VHI groups. No difference was found in MAP-2 immunostaining or protein expression level between PA+PEA and CTL–VHI groups. CTL–VHI, control rats treated with vehicle; PA–VHI, rats subjected to PA and treated with vehicle; PA+PEA, rats subjected to PA and PEA treatment; CTL+PEA, control rats treated with PEA.
GFAP Immunostaining and Protein Expression at P30 After PA: Protective Effect of PEA Treatment
We used immunohistochemistry and Western blot to analyze glial response to PA injury and PEA treatment in the rat dorsal striatum at P30 (n = 16). Figure 3A shows a representative striatal section immunostained for GFAP+ immunoreactive astrocytes. The number of GFAP+ immunoreactive astrocytes was dependent on birth condition and treatment [F(1,56) = 58.73, p < 0.0001; F(1,56) = 16.44, p = 0.0002, respectively] showing between-interaction [F(1,56) = 19.4, p < 0.0001]. The post hoc analysis revealed that PA (PA–VHI) reduced the number of GFAP+ immunoreactive astrocytes compared with the control group (CTL–VHI) (p < 0.0001, Figure 3B). This decrease was, to some extent, reversed by PEA treatment (PA+PEA) (p < 0.0001, Figure 3B) without reaching control values (p = 0.0048, Figure 3B). Also, the number of GFAP+ immunoreactive astrocytes was not different between vehicle-treated (CTL–VHI) or PEA-treated control rats (CTL+PEA) (p = 0.9946, Figure 3B). However, neither birth condition or treatment affected GFAP expression [F(1,8) = 0.04421, p = 0.8387; F(1,8) = 0.01157, p = 0.917, respectively] nor between-interaction was observed [F(1,8) = 0.002636, p = 0.9603, Figure 3C].
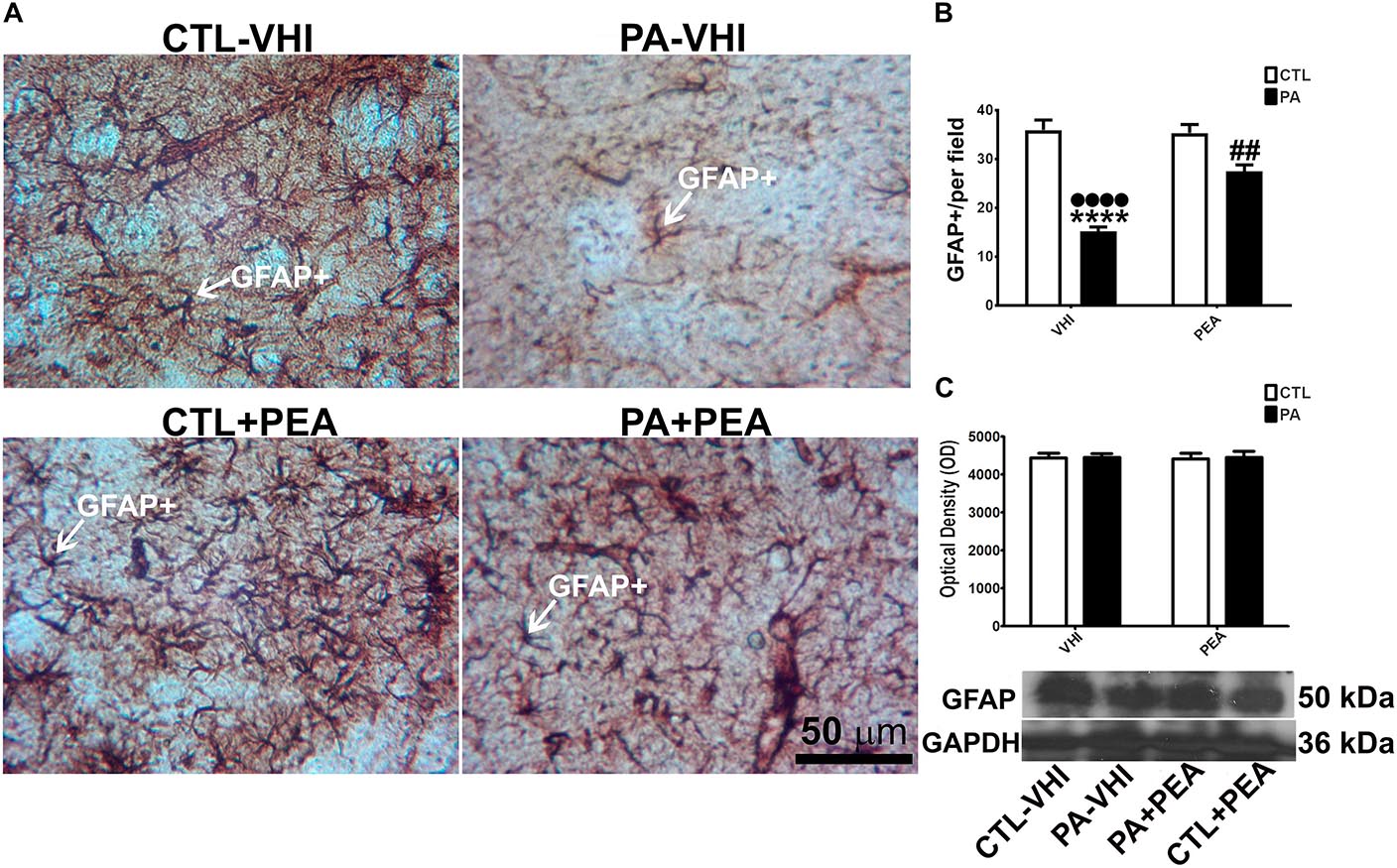
Figure 3. GFAP+ cells and protein expression level in the rat striatum. (A) Representative images of the dorsal striatum immunostained for glial fibrillary acidic protein (GFAP). The white arrow shows an astrocyte immunostained for GFAP. Scale bar: 50 μm. (B) Number of the GFAP+ cells measured per experimental group. (C) Optical density of the bands for GFAP protein expression level. Bars and error bars represent mean ± SEM. Two-way ANOVA followed by Tukey’s post hoc test. ∗∗∗∗p < 0.0001, PA–VHI vs. CTL–VHI, ••••p < 0.0001, PA–VHI vs. PA+PEA and ##p < 0.005, PA+PEA vs. CTL–VHI. PEA had no effect on GFAP+ immunostaining as there was no difference between CTL+PEA and CTL–VHI groups. Neither PA nor PEA affected GFAP protein level. CTL, control rats treated with vehicle; PA–VHI, rats subjected to PA and treated with vehicle; PA+PEA, rats subjected to PA and PEA treatment; CTL+PEA, control rats treated with PEA.
Discussion
Present findings show PA-induced morphological alterations like dendritic damage (MAP-2 reduction), axonal injury (pNF-H/M reduction), and a decrease in the number of astrocytes (GFAP+ cells) in the dorsal corpus striatum at P30. The effects on both MAP-2 and pNF-H/M were confirmed by Western blot. The MAP-2 is a commonly used biomarker to assess the extension and distribution of dendritic cytoskeletal degeneration induced by hypoxia-ischemia (HI) (Mink and Johnston, 2000; Kühn et al., 2005; Graham et al., 2018). Immunoreactivity for MAP-2 decreased in the human post-mortem hippocampus and cerebral cortex after carbon monoxide (CO) exposure (Kühn et al., 2005). Transient or permanent middle cerebral artery occlusion followed by reperfusion reduced MAP-2 expression mainly in rat striatum and neocortex (Popp et al., 2009). Also, MAP-2 immunostaining decreased in the cerebral cortex, caudate, and thalamus in a perinatal stroke rat model (Malinak and Silverstein, 1996), and in the cerebral cortex of neonatal rats after transient cerebral HI (Blomgren et al., 1995). In our study, PA induced a marked decrease both in protein level and immunoreactivity of MAP-2 in rat striatum at P30. In agreement, previous studies from our laboratory reported a significant reduction of MAP-2 at P30 in the striatum (Saraceno et al., 2012a). Similar results were found in the hippocampus at the same time point (Herrera et al., 2018), and later at P120 (Saraceno et al., 2010). This reduction of MAP-2 could be related to a decrease of adenosine triphosphate synthesis triggered by the low availability of oxygen after the hypoxic event (Islam and Burns, 1980).
This study also shows a substantial decrease in protein level and immunoreactivity of pNF-H/M in the corpus striatum in response to PA at P30 that might result from calpain, a calcium-dependent protease largely distributed in the brain, activation (Schlaepfer, 1987) by hypoxia (Stys and Jiang, 2002). Likewise, a decrease in volume and a loss of phosphorylated NFs were found in the corpus callosum and the internal capsule in experimental cerebral palsy (Drobyshevsky et al., 2007). Although we have recently demonstrated that PA was associated with an increase in protein level and pNF-H/M immunoreactivity in the CA1 hippocampus at P30 (Herrera et al., 2018), the level of pNF-H varies depending on the brain region, being low in the cerebral cortex and intermediate to high in the large-diameter brain stem axons, cerebellum, thalamus, subcortical white matter, and basal ganglia, as well as in varied pathological conditions (Shaw et al., 2005; Drobyshevsky et al., 2007; Anderson et al., 2008; Guy et al., 2008; Lewis et al., 2008; Boylan et al., 2009; Herrera et al., 2018). Bearing this in mind, the choice of different tissue sources may account for the discrepancies between studies, making further research necessary.
Unlike the consistency found for either pNF-H/M or MAP-2, discrepant findings were observed between a change in GFAP measured as the percentage of the reactive area (immunohistochemistry and the lack of change in optical density in the amount of protein (Western blot). The difference in the anatomical region used in each case might well account for the discrepancy. The dorsal striatum, the most vulnerable area to hypoxia, was used for immunohistochemical determinations. Conversely, due to methodological issues, the entire corpus striatum, both ventral and dorsal, was used for Western blot analysis, likely what might dilute any changes that might have eventually been observed otherwise. On top of this, negligible differences might have gone undetected due to insufficient sensitivity in the Western blot determinations.
Notwithstanding that GFAP did not appear as an early marker of hippocampal damage after PA, we assessed the number of GFAP+ astrocytes in the dorsal striatum based on our previously reported PA-induced changes in GFAP immunostaining in this region at P30 (Holubiec et al., 2017). Presently, PA induced a remarkable decrease in the number of astroglial cells. This finding likely attributable to astrocytic death might precede neuronal loss and inflammatory reaction according to previous evidence (Villapol et al., 2008; Hostenbach et al., 2014; Romero et al., 2014). In perinatal ischemia followed by reperfusion, the proapoptotic protein Bax was up-regulated in GFAP+ astrocytes, particularly in the peri-infarct region in the cortex at P30 post-ischemia (Benjelloun et al., 1999; Villapol et al., 2008), and apoptotic and necrotic morphological features are seen in the same astrocytes 12–24 h after oxygen-glucose deprivation, accompanied by autophagosomes that appear in the astrocytes’ cytoplasm in both ischemic cortices (Xu and Zhang, 2011). After neonatal ischemia, these dying reactive astrocytes might participate in the ongoing deleterious process and promote the formation of cystic lesions as clinically observed in the newborn brain (Villapol et al., 2008). However, our results in GFAP immunostaining were not confirmed by Western blot. Therefore, further studies are necessary to clarify this point.
Treatment with PEA (10 mg/kg) within the first hour of life attenuated PA-induced dendritic and axonal cytoskeletal damage in the dorsal striatum at P30 according to the change in MAP-2 and pNF-H/M both immunostaining and protein levels, respectively. In addition, PEA treatment prevented the decrease in the number of GFAP+ cells in the PA dorsal striatum at P30. Recently, we reported that PEA neuroprotected from dendritic and axonal cytoskeletal alterations induced by PA in the hippocampus (Herrera et al., 2018). Interestingly, Blanco et al. (2015) showed that PA induced a substantial decrease in the protein level of the PEA receptor, PPARα, and in N-acyl phosphatidylethanolamine-specific phospholipase D (Blanco et al., 2015), an enzyme that synthesizes acylethanolamides like PEA (Harrison et al., 2014) also at P30. This reduction could lead to dysregulation and a decrease in endocannabinoids level, inhibiting PPARα activity (Blanco et al., 2015). Then, reasonably enough, the exogenous administration of PEA may be responsible for the protective effects reported in this study.
Furthermore, our findings agree with previous reports on the protective effects of PEA in experimental neurodegeneration (Skaper et al., 1996; Esposito et al., 2012; Scuderi et al., 2014; Herrera et al., 2016). PEA protected from the decrease in MAP-2 expression induced by amyloid peptides (Scuderi et al., 2014; Tomasini et al., 2015) and by MPTP in an animal model of Parkinson’s disease (Esposito et al., 2012). Similarly, PEA could prevent MAP-2 reduction after hypoxia (Kühn et al., 2005; Fernandez-Lopez et al., 2013; England et al., 2015).
All in all, the experimental evidence supports the protective role of PEA against neuronal damage in several brain injuries, including PA-induced brain damage. Consistently with a vast number of studies, the present work reinforces the evidence of the neuroprotective effects of PEA against HI in the corpus striatum, a vulnerable brain structure to HI injuries, fostering our further investigation of the mechanisms involved.
A next study should examine the ultrastructural effects of PEA treatment regarding its presently reported effects on the expression of pNF-H/M and MAP-2, ruling out any possible bias in the interpretation of optical microscopy images.
Data Availability Statement
All datasets generated for this study are included in the article/Supplementary Material.
Ethics Statement
The animal study was reviewed and approved by the National Institute of Health Guide for the Care and Use of Laboratory Animals, School of Medicine, University of Buenos Aires.
Author Contributions
LU and AR-H: acquisition, analysis, and interpretation of the data and drafting the work. TK and MH: interpretation of the data, bibliographic research, and drafting the work. NT-U, CK, RK-F, and JL: acquisition, analysis, and interpretation of the data. MO-L: critical revision for intellectual content and substantive editing, grammar and language style. FC: conception and design of the work and supervision.
Funding
This work was supported by grants to FC from CONICET (PIP 2016–2019 no. 0779), the University of Buenos Aires (UBACyT 2014-2017 no. 20720130100014BA), IBRO-PROLAB 2017, and FONCyT (PICD 0031 2016-2020).
Conflict of Interest
The authors declare that the research was conducted in the absence of any commercial or financial relationships that could be construed as a potential conflict of interest.
Supplementary Material
The Supplementary Material for this article can be found online at: https://www.frontiersin.org/articles/10.3389/fnins.2019.01345/full#supplementary-material
Abbreviations
ANOVA, analysis of variance; CNS, central nervous system; CO, carbon monoxide; GFAP, glial fibrillary acidic protein; HI, hypoxia-ischemia; HIE, hypoxia-ischemia encephalopathy; MAP-2, microtubule-associated protein-2; MPTP, 1-methyl-4-phenyl-1,2,3,6-tetrahydropyridine; PEA, palmitoylethanolamide; PA, perinatal asphyxia; PPAR α, peroxisome proliferator-activated receptor alpha; pNF-H/M, phosphorylated high/medium-molecular-weight NF.
References
Alarcon-Yaquetto, D. E., Caballero, L., and Gonzales, G. F. (2017). Association between plasma N-acylethanolamides and high hemoglobin concentration in Southern Peruvian highlanders. High Alt. Med. Biol. 18, 322–329. doi: 10.1089/ham.2016.0148
Anderson, K. J., Scheff, S. W., Miller, K. M., Roberts, K. N., Gilmer, L. K., Yang, C., et al. (2008). The phosphorylated axonal form of the neurofilament subunit NF-H (pNF-H) as a blood biomarker of traumatic brain injury. J. Neurotrauma 25, 1079–1085. doi: 10.1089/neu.2007.0488
Arteni, N. S., Pereira, L. O., Rodrigues, A. L., Lavinsky, D., Achaval, M. E., and Netto, C. A. (2010). Lateralized and sex-dependent behavioral and morphological effects of unilateral neonatal cerebral hypoxia-ischemia in the rat. Behav. Brain Res. 210, 92–98. doi: 10.1016/j.bbr.2010.02.015
Avagliano, C., Russo, R., De Caro, C., Cristiano, C., La Rana, G., Piegari, G., et al. (2016). Palmitoylethanolamide protects mice against 6-OHDA-induced neurotoxicity and endoplasmic reticulum stress: in vivo and in vitro evidence. Pharmacol. Res. 113, 276–289. doi: 10.1016/j.phrs.2016.09.004
Barkhuizen, M., van den Hove, D. L., Vles, J. S., Steinbusch, H. W., Kramer, B. W., and Gavilanes, A. W. (2017). 25 years of research on global asphyxia in the immature rat brain. Neurosci. Biobehav. Rev. 75, 166–182. doi: 10.1016/j.neubiorev.2017.01.042
Benjelloun, N., Renolleau, S., Represa, A., Ben-Ari, Y., and Charriaut-Marlangue, C. (1999). Inflammatory responses in the cerebral cortex after ischemia in the P7 neonatal Rat. Stroke 30, 1916–1923. doi: 10.1161/01.STR.30.9.1916
Bjelke, B., Andersson, K., Ogren, S. O., and Bolme, P. (1991). Asphyctic lesion: proliferation of tyrosine hydroxylase-immunoreactive nerve cell bodies in the rat Substantia nigra and functional changes in dopamine neurotransmission. Brain Res. 543, 1–9. doi: 10.1016/0006-8993(91)91041-x
Blanco, E., Galeano, P., Holubiec, M. I., Romero, J. I., Logica, T., Rivera, P., et al. (2015). Perinatal asphyxia results in altered expression of the hippocampal acylethanolamide/endocannabinoid signaling system associated to memory impairments in postweaned rats. Front. Neuroanat. 9:141. doi: 10.3389/fnana.2015.00141
Blomgren, K., McRae, A., Bona, E., Saido, T. C., Karlsson, J. O., and Hagberg, H. (1995). Degradation of fodrin and MAP 2 after neonatal cerebral hypoxic-ischemia. Brain Res. 684, 136–142. doi: 10.1016/0006-8993(95)00398-a
Boksa, P., and El-Khodor, B. F. (2003). Birth insult interacts with stress at adulthood to alter dopaminergic function in animal models: possible implications for schizophrenia and other disorders. Neurosci. Biobehav. Rev. 27, 91–101. doi: 10.1016/s0149-7634(03)00012-5
Boylan, K., Yang, C., Crook, J., Overstreet, K., Heckman, M., Wang, Y., et al. (2009). Immunoreactivity of the phosphorylated axonal neurofilament H subunit (pNF-H) in blood of ALS model rodents and ALS patients: evaluation of blood pNF-H as a potential ALS biomarker. J. Neurochem. 111, 1182–1191. doi: 10.1111/j.1471-4159.2009.06386.x
Calabresi, P., Centonze, D., Gubellini, P., Marfia, G. A., Pisani, A., Sancesario, G., et al. (2000). Synaptic transmission in the striatum: from plasticity to neurodegeneration. Prog. Neurobiol. 61, 231–265. doi: 10.1016/s0301-0082(99)00030-1
Capani, F., Loidl, C. F., Aguirre, F., Piehl, L., Facorro, G., Hager, A., et al. (2001). Changes in reactive oxygen species (ROS) production in rat brain during global perinatal asphyxia: an ESR study. Brain Res. 914, 204–207. doi: 10.1016/s0006-8993(01)02781-0
Capani, F., Loidl, C. F., Piehl, L. L., Facorro, G., De Paoli, T., and Hager, A. (2003). Long term production of reactive oxygen species during perinatal asphyxia in the rat central nervous system: effects of hypothermia. Int. J. Neurosci. 113, 641–654. doi: 10.1080/00207450390200099
Capani, F., Loidl, F., Lopez-Costa, J. J., Selvin-Testa, A., and Saavedra, J. P. (1997). Ultrastructural changes in nitric oxide synthase immunoreactivity in the brain of rats subjected to perinatal asphyxia: neuroprotective effects of cold treatment. Brain Res. 775, 11–23. doi: 10.1016/s0006-8993(97)00714-2
Capani, F., Saraceno, G. E., Botti, V., Aon-Bertolino, L., de Oliveira, D. M., Barreto, G., et al. (2009). Protein ubiquitination in postsynaptic densities after hypoxia in rat neostriatum is blocked by hypothermia. Exp. Neurol. 219, 404–413. doi: 10.1016/j.expneurol.2009.06.007
Cebral, E., Capani, F., Selvín-Testa, A., Funes, M. R., Coirini, H., and Loidl, C. F. (2006). Neostriatal cytoskeleton changes following perinatal asphyxia: effect of hypothermia treatment. Int. J. Neurosci. 116, 697–714. doi: 10.1080/00207450600674970
Drobyshevsky, A., Derrick, M., Wyrwicz, A. M., Ji, X., Englof, I., Ullman, L. M., et al. (2007). White matter injury correlates with hypertonia in an animal model of cerebral palsy. J. Cereb. Blood Flow Metab. 27, 270–281. doi: 10.1038/sj.jcbfm.9600333
England, T. J., Hind, W. H., Rasid, N. A., and O’Sullivan, S. E. (2015). Cannabinoids in experimental stroke: a systematic review and meta-analysis. J. Cereb. Blood Flow Metab. 35, 348–358. doi: 10.1038/jcbfm.2014.218
Esposito, E., Cordaro, M., and Cuzzocrea, S. (2014). Roles of fatty acid ethanolamides (FAE) in traumatic and ischemic brain injury. Pharmacol. Res. 86, 26–31. doi: 10.1016/j.phrs.2014.05.009
Esposito, E., Impellizzeri, D., Mazzon, E., Paterniti, I., and Cuzzocrea, S. (2012). Neuroprotective activities of palmitoylethanolamide in an animal model of Parkinson’s disease. PLoS One 7:e41880. doi: 10.1371/journal.pone.0041880
Esposito, E., Paterniti, I., Mazzon, E., Genovese, T., Di Paola, R., Galuppo, M., et al. (2011). Effects of palmitoylethanolamide on release of mast cell peptidases and neurotrophic factors after spinal cord injury. Brain Behav. Immun. 25, 1099–1112. doi: 10.1016/j.bbi.2011.02.006
Fernandez-Lopez, D., Lizasoain, I., Moro, M. A., and Martinez-Orgado, J. (2013). Cannabinoids: well-suited candidates for the treatment of perinatal brain injury. Brain Sci. 3, 1043–1059. doi: 10.3390/brainsci3031043
Galeano, P., Blanco Calvo, E., Madureira de Oliveira, D., Cuenya, L., Kamenetzky, G. V., Mustaca, A. E., et al. (2011). Long-lasting effects of perinatal asphyxia on exploration, memory and incentive downshift. Int. J. Dev. Neurosci. 29, 609–619. doi: 10.1016/j.ijdevneu.2011.05.002
Genovese, T., Esposito, E., Mazzon, E., Di Paola, R., Meli, R., Bramanti, P., et al. (2008). Effects of palmitoylethanolamide on signaling pathways implicated in the development of spinal cord injury. J. Pharmacol. Exp. Ther. 326, 12–23. doi: 10.1124/jpet.108.136903
Gerfen, C. R. (1989). The neostriatal mosaic: striatal patch-matrix organization is related to cortical lamination. Science 246, 385–388. doi: 10.1126/science.2799392
Graham, E. M., Everett, A. D., Delpech, J. C., and Northington, F. J. (2018). Blood biomarkers for evaluation of perinatal encephalopathy: state of the art. Curr. Opin. Pediatr. 30, 199–203. doi: 10.1097/MOP.0000000000000591
Gupta, K., Jadhav, J. P., and Shrikhande, D. Y. (2018). Study of clinical manifestations and complications of perinatal asphyxia: observational study. Indian J. Basic Appl. Med. Res. 7, 342–345.
Guy, J., Shaw, G., Ross-Cisneros, F. N., Quiros, P., Salomao, S. R., Berezovsky, A., et al. (2008). Phosphorylated neurofilament heavy chain is a marker of neurodegeneration in Leber hereditary optic neuropathy (LHON). Mol. Vis. 14, 2443–2450.
Harrison, N., Lone, M. A., Kaul, T. K., Reis Rodrigues, P., Ogungbe, I. V., and Gill, M. S. (2014). Characterization of N-acyl phosphatidylethanolamine-specific phospholipase-D isoforms in the nematode Caenorhabditis elegans. PLoS One 9:e113007. doi: 10.1371/journal.pone.0113007
Herrera, M. I., Kölliker-Frers, R., Barreto, G., Blanco, E., and Capani, F. (2016). Glial modulation by N-acylethanolamides in brain injury and neurodegeneration. Front. Aging Neurosci. 8:81. doi: 10.3389/fnagi.2016.00081
Herrera, M. I., Mucci, S., Barreto, G. E., Kolliker-Frers, R., and Capani, F. (2017a). Neuroprotection in hypoxic-ischemic brain injury targeting glial cells. Curr. Pharm. Des. 23, 3899–3906. doi: 10.2174/1381612823666170727145422
Herrera, M. I., Otero-Losada, M., Udovin, L. D., Kusnier, C., Kölliker-Frers, R., de Souza, W., et al. (2017b). Could perinatal asphyxia induce a synaptopathy? New highlights from an experimental model. Neural Plast. 2017:3436943. doi: 10.1155/2017/3436943
Herrera, M. I., Udovin, L. D., Toro-Urrego, N., Kusnier, C. F., Luaces, J. P., and Capani, F. (2018). Palmitoylethanolamide ameliorates hippocampal damage and behavioral dysfunction after perinatal asphyxia in the immature rat brain. Front. Neurosci. 12:145. doi: 10.3389/fnins.2018.00145
Herrera-Marschitz, M., Neira-Pena, T., Rojas-Mancilla, E., Espina-Marchant, P., Esmar, D., Perez, R., et al. (2014). Perinatal asphyxia: CNS development and deficits with delayed onset. Front. Neurosci. 8:47. doi: 10.3389/fnins.2014.00047
Holubiec, M. I., Romero, J. I., Blanco, E., Tornatore, T. L., Suarez, J., Rodriguez de Fonseca, F., et al. (2017). Acylethanolamides and endocannabinoid signaling system in dorsal striatum of rats exposed to perinatal asphyxia. Neurosci. Lett. 653, 269–275. doi: 10.1016/j.neulet.2017.05.068
Holubiec, M. I., Romero, J. I., Suárez, J., Portavella, M., Fernández-Espejo, E., Blanco, E., et al. (2018). Palmitoylethanolamide prevents neuroinflammation, reduces astrogliosis and preserves recognition and spatial memory following induction of neonatal anoxia-ischemia. Psychopharmacology 235, 2929–2945. doi: 10.1007/s00213-018-4982-9
Hostenbach, S., Cambron, M., D’haeseleer, M., Kooijman, R., and De Keyser, J. (2014). Astrocyte loss and astrogliosis in neuroinflammatory disorders. Neurosci. Lett. 565, 39–41. doi: 10.1016/j.neulet.2013.10.012
Islam, K., and Burns, R. (1980). Multiple phosphorylation sites of microtubule-associated protein (MAP2) observed at high ATP concentrations. FEBS Lett. 123, 181–185. doi: 10.1016/0014-5793(81)80282-7
Kühn, J., Meissner, C., and Oehmichen, M. (2005). Microtubule-associated protein 2 (MAP2)–a promising approach to diagnosis of forensic types of hypoxia-ischemia. Acta Neuropathol. 110, 579–586. doi: 10.1007/s00401-005-1090-9
Lewis, S. B., Wolper, R. A., Miralia, L., Yang, C., and Shaw, G. (2008). Detection of phosphorylated NF-H in the cerebrospinal fluid and blood of aneurysmal subarachnoid hemorrhage patients. J. Cereb. Blood Flow Metab. 28, 1261–1271. doi: 10.1038/jcbfm.2008.12
Looi, J. C., and Walterfang, M. (2013). Striatal morphology as a biomarker in neurodegenerative disease. Mol. Psychiatry 18, 417–424. doi: 10.1038/mp.2012.54
Malinak, C., and Silverstein, F. S. (1996). Hypoxic-ischemic injury acutely disrupts microtubule-associated protein 2 immunostaining in neonatal rat brain. Biol. Neonate 69, 257–267. doi: 10.1159/000244319
Mattace Raso, G., Russo, R., Calignano, A., and Meli, R. (2014). Palmitoylethanolamide in CNS health and disease. Pharmacol. Res. 86, 32–41. doi: 10.1016/j.phrs.2014.05.006
Melis, M., Pillolla, G., Luchicchi, A., Muntoni, A. L., Yasar, S., Goldberg, S. R., et al. (2008). Endogenous fatty acid ethanolamides suppress nicotine-induced activation of mesolimbic dopamine neurons through nuclear receptors. J. Neurosci. 28, 13985–13994. doi: 10.1523/JNEUROSCI.3221-08.2008
Mink, R. B., and Johnston, J. A. (2000). Changes in brain neurofilament and beta-tubulin proteins after cerebral hypoxia-ischemia in rabbits. Pathobiology 68, 43–52. doi: 10.1159/000028114
Modabbernia, A., Mollon, J., Boffetta, P., and Reichenberg, A. (2016). Impaired gas exchange at birth and risk of intellectual disability and autism: a meta-analysis. J. Autism Dev. Disord. 46, 1847–1859. doi: 10.1007/s10803-016-2717-5
Morales, P., Bustamante, D., Espina-Marchant, P., Neira-Pena, T., Gutierrez-Hernandez, M. A., Allende-Castro, C., et al. (2011). Pathophysiology of perinatal asphyxia: can we predict and improve individual outcomes? EPMA J. 2, 211–230. doi: 10.1007/s13167-011-0100-3
Muñiz, J., Romero, J., Holubiec, M., Barreto, G., Gonzalez, J., Saint-Martin, M., et al. (2014). Neuroprotective effects of hypothermia on synaptic actin cytoskeletal changes induced by perinatal asphyxia. Brain Res. 1563, 81–90. doi: 10.1016/j.brainres.2014.03.023
Orefice, N. S., Alhouayek, M., Carotenuto, A., Montella, S., Barbato, F., Comelli, A., et al. (2016). Oral palmitoylethanolamide treatment is associated with reduced cutaneous adverse effects of interferon-β1a and circulating proinflammatory cytokines in relapsing-remitting multiple sclerosis. Neurotherapeutics 13, 428–438. doi: 10.1007/s13311-016-0420-z
Perna, R., and Cooper, D. (2012). Perinatal cyanosis: long-term cognitive sequelae and behavioral consequences. Appl. Neuropsychol. Child 1, 48–52. doi: 10.1080/09084282.2011.643946
Petrosino, S., and Di Marzo, V. (2017). The pharmacology of palmitoylethanolamide and first data on the therapeutic efficacy of some of its new formulations. Br. J. Pharmacol. 174, 1349–1365. doi: 10.1111/bph.13580
Popp, A., Jaenisch, N., Witte, O. W., and Frahm, C. (2009). Identification of ischemic regions in a rat model of stroke. PLoS One 4:e4764. doi: 10.1371/journal.pone.0004764
Portavella, M., Rodriguez-Espinosa, N., Galeano, P., Blanco, E., Romero, J. I., Holubiec, M. I., et al. (2018). Oleoylethanolamide and palmitoylethanolamide protect cultured cortical neurons against hypoxia. Cannabis Cannabinoid Res. 3, 171–178. doi: 10.1089/can.2018.0013
Pugliese, V., Bruni, A., Carbone, E. A., Calabrò, G., Cerminara, G., Sampogna, G., et al. (2019). Maternal stress, prenatal medical illnesses and obstetric complications: risk factors for schizophrenia spectrum disorder, bipolar disorder and major depressive disorder. Psychiatry Res. 271, 23–30. doi: 10.1016/j.psychres.2018.11.023
Rocha-Ferreira, E., and Hristova, M. (2016). Plasticity in the neonatal brain following hypoxic-ischaemic injury. Neural Plast. 2016:4901014. doi: 10.1155/2016/4901014
Romero, J., Muñiz, J., Logica Tornatore, T., Holubiec, M., González, J., Barreto, G. E., et al. (2014). Dual role of astrocytes in perinatal asphyxia injury and neuroprotection. Neurosci. Lett. 565, 42–46. doi: 10.1016/j.neulet.2013.10.046
Romero, J. I., Hanschmann, E. M., Gellert, M., Eitner, S., Holubiec, M. I., Blanco-Calvo, E., et al. (2015). Thioredoxin 1 and glutaredoxin 2 contribute to maintain the phenotype and integrity of neurons following perinatal asphyxia. Biochim. Biophys. Acta 1850, 1274–1285. doi: 10.1016/j.bbagen.2015.02.015
Romero, J. I., Holubiec, M. I., Tornatore, T. L., Rivière, S., Hanschmann, E. M., Kölliker-Frers, R. A., et al. (2017). Neuronal damage induced by perinatal asphyxia is attenuated by postinjury glutaredoxin-2 administration. Oxid. Med. Cell. Longev. 2017:4162465. doi: 10.1155/2017/4162465
Saraceno, G. E., Ayala, M. V., Badorrey, M. S., Holubiec, M., Romero, J. I., Galeano, P., et al. (2012a). Effects of perinatal asphyxia on rat striatal cytoskeleton. Synapse 66, 9–19. doi: 10.1002/syn.20978
Saraceno, G. E., Castilla, R., Barreto, G. E., Gonzalez, J., Kolliker-Frers, R. A., and Capani, F. (2012b). Hippocampal dendritic spines modifications induced by perinatal asphyxia. Neural Plast. 2012:873532. doi: 10.1155/2012/873532
Saraceno, G. E., Bertolino, M. L., Galeano, P., Romero, J. I., Garcia-Segura, L. M., and Capani, F. (2010). Estradiol therapy in adulthood reverses glial and neuronal alterations caused by perinatal asphyxia. Exp. Neurol. 223, 615–622. doi: 10.1016/j.expneurol.2010.02.010
Saraceno, G. E., Caceres, L. G., Guelman, L. R., Castilla, R., Udovin, L. D., Ellisman, M. H., et al. (2016). Consequences of excessive plasticity in the hippocampus induced by perinatal asphyxia. Exp. Neurol. 286, 116–123. doi: 10.1016/j.expneurol.2016.08.017
Sayd, A., Anton, M., Alen, F., Caso, J. R., Pavon, J., Leza, J. C., et al. (2014). Systemic administration of oleoylethanolamide protects from neuroinflammation and anhedonia induced by LPS in rats. Int. J. Neuropsychopharmacol. 18:yu111. doi: 10.1093/ijnp/pyu111
Schieve, L. A., Tian, L. H., Baio, J., Rankin, K., Rosenberg, D., Wiggins, L., et al. (2014). Population attributable fractions for three perinatal risk factors for autism spectrum disorders, 2002 and 2008 autism and developmental disabilities monitoring network. Ann. Epidemiol. 24, 260–266. doi: 10.1016/j.annepidem.2013.12.014
Schlaepfer, W. W. (1987). Neurofilaments: structure, metabolism and implications in disease. J. Neuropathol. Exp. Neurol. 46, 117–129. doi: 10.1097/00005072-198703000-00001
Scuderi, C., Stecca, C., Valenza, M., Ratano, P., Bronzuoli, M. R., Bartoli, S., et al. (2014). Palmitoylethanolamide controls reactive gliosis and exerts neuroprotective functions in a rat model of Alzheimer’s disease. Cell Death Dis. 5:e1419. doi: 10.1038/cddis.2014.376
Semple, B. D., Blomgren, K., Gimlin, K., Ferriero, D. M., and Noble-Haeusslein, L. J. (2013). Brain development in rodents and humans: identifying benchmarks of maturation and vulnerability to injury across species. Prog. Neurobiol. 106–107, 1–16. doi: 10.1016/j.pneurobio.2013.04.001
Shaw, G., Yang, C., Ellis, R., Anderson, K., Parker Mickle, J., Scheff, S., et al. (2005). Hyperphosphorylated neurofilament NF-H is a serum biomarker of axonal injury. Biochem. Biophys. Res. Commun. 336, 1268–1277. doi: 10.1016/j.bbrc.2005.08.252
Skaper, S. D., Buriani, A., Dal Toso, R., Petrelli, L., Romanello, S., Facci, L., et al. (1996). The ALIAmide palmitoylethanolamide and cannabinoids, but not anandamide, are protective in a delayed postglutamate paradigm of excitotoxic death in cerebellar granule neurons. Proc. Natl. Acad. Sci. U.S.A. 93, 3984–3989. doi: 10.1073/pnas.93.9.3984
Strackx, E., Zoer, B., Van den Hove, D., Steinbusch, H., Blanco, C., Vles, J. S., et al. (2010). Brain apoptosis and carotid artery reactivity in fetal asphyctic preconditioning. Front. Biosci. 2, 781–790. doi: 10.2741/s101
Stys, P. K., and Jiang, Q. (2002). Calpain-dependent neurofilament breakdown in anoxic and ischemic rat central axons. Neurosci. Lett. 328, 150–154. doi: 10.1016/S0304-3940(02)00469-X
Tomasini, M. C., Borelli, A. C., Beggiato, S., Ferraro, L., Cassano, T., Tanganelli, S., et al. (2015). Differential effects of palmitoylethanolamide against amyloid-β induced toxicity in cortical neuronal and astrocytic primary cultures from wild-type and 3xTg-AD mice. J. Alzheimers Dis. 46, 407–421. doi: 10.3233/JAD-143039
Van de Berg, W. D., Kwaijtaal, M., de Louw, A. J., Lissone, N. P., Schmitz, C., Faull, R. L., et al. (2003). Impact of perinatal asphyxia on the GABAergic and locomotor system. Neuroscience 117, 83–96. doi: 10.1016/s0306-4522(02)00787-x
Villapol, S., Gelot, A., Renolleau, S., and Charriaut-Marlangue, C. (2008). Astrocyte responses after neonatal ischemia: the yin and the yang. Neuroscientist 14, 339–344. doi: 10.1177/1073858408316003
Wakuda, T., Iwata, K., Iwata, Y., Anitha, A., Takahashi, T., Yamada, K., et al. (2015). Perinatal asphyxia alters neuregulin-1 and COMT gene expression in the medial prefrontal cortex in rats. Prog. Neuropsychopharmacol. Biol. Psychiatry 56, 149–154. doi: 10.1016/j.pnpbp.2014.08.002
Xu, M., and Zhang, H. (2011). Death and survival of neuronal and astrocytic cells in ischemic brain injury: a role of autophagy. Acta Pharmacol. Sin. 32, 1089–1099. doi: 10.1038/aps.2011.50
Keywords: neuroprotection, striatum, palmitoylethanolamide, perinatal asphyxia, neurofilaments
Citation: Udovin LD, Kobiec T, Herrera MI, Toro-Urrego N, Kusnier CF, Kölliker-Frers RA, Ramos-Hryb AB, Luaces JP, Otero-Losada M and Capani F (2020) Partial Reversal of Striatal Damage by Palmitoylethanolamide Administration Following Perinatal Asphyxia. Front. Neurosci. 13:1345. doi: 10.3389/fnins.2019.01345
Received: 14 July 2019; Accepted: 29 November 2019;
Published: 08 January 2020.
Edited by:
Stefano Comai, Vita-Salute San Raffaele University, ItalyReviewed by:
Carina Rodrigues Boeck, UFN – Universidade Franciscana, BrazilIria Gonzalez Dopeso-Reyes, UMR 5535 Institut de Génétique Moléculaire de Montpellier (IGMM), France
Copyright © 2020 Udovin, Kobiec, Herrera, Toro-Urrego, Kusnier, Kölliker-Frers, Ramos-Hryb, Luaces, Otero-Losada and Capani. This is an open-access article distributed under the terms of the Creative Commons Attribution License (CC BY). The use, distribution or reproduction in other forums is permitted, provided the original author(s) and the copyright owner(s) are credited and that the original publication in this journal is cited, in accordance with accepted academic practice. No use, distribution or reproduction is permitted which does not comply with these terms.
*Correspondence: Matilde Otero-Losada, bW9sbHkxMDYzQGdtYWlsLmNvbQ==
†These authors have contributed equally to this work