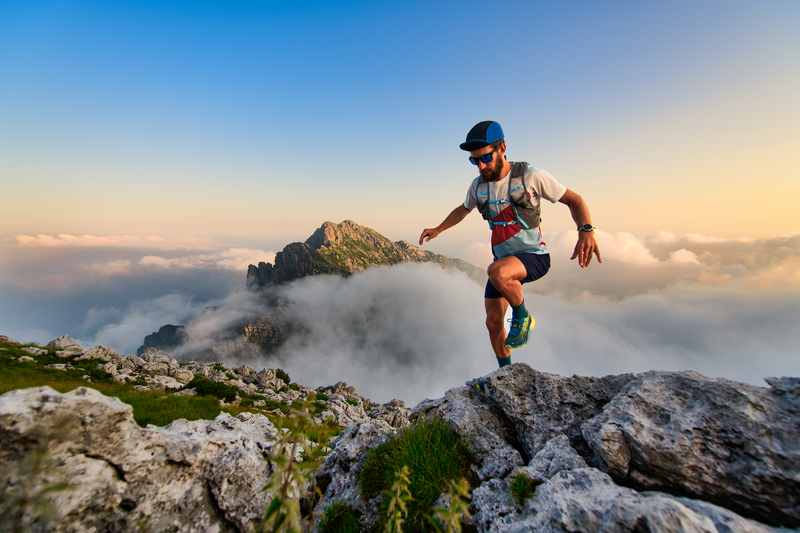
94% of researchers rate our articles as excellent or good
Learn more about the work of our research integrity team to safeguard the quality of each article we publish.
Find out more
REVIEW article
Front. Neurosci. , 04 September 2019
Sec. Neural Technology
Volume 13 - 2019 | https://doi.org/10.3389/fnins.2019.00911
This article is part of the Research Topic Neural Electroceuticals: Interfacing with the Nervous System with Electrical Stimulation View all 42 articles
Over the last several decades, vagus nerve stimulation (VNS) has evolved from a treatment for select neuropsychiatric disorders to one that holds promise in treating numerous inflammatory conditions. Growing interest has focused on the use of VNS for other indications, such as heart failure, rheumatoid arthritis, inflammatory bowel disease, ischemic stroke, and traumatic brain injury. As pre-clinical research often guides expansion into new clinical avenues, animal models of VNS have also increased in recent years. To advance this promising treatment, however, there are a number of experimental parameters that must be considered when planning a study, such as physiology of the vagus nerve, electrical stimulation parameters, electrode design, stimulation equipment, and microsurgical technique. In this review, we discuss these important considerations and how a combination of clinically relevant stimulation parameters can be used to achieve beneficial therapeutic results in pre-clinical studies of sub-acute to chronic VNS, and provide a practical guide for performing this work in rodent models. Finally, by integrating clinical and pre-clinical research, we present indeterminate issues as opportunities for future research.
Vagus nerve stimulation (VNS) is an FDA-approved treatment for select neurological and psychiatric conditions including epilepsy, treatment-resistant depression, and cluster headache (Heck et al., 2002; Ruffoli et al., 2011; Conway et al., 2016; Pisapia and Baltuch, 2016; Lainez and Guillamon, 2017; Kumar et al., 2019). There is also growing interest in using VNS to treat other conditions, such as heart failure, rheumatoid arthritis, inflammatory bowel disease, ischemic stroke, and traumatic brain injury (Zhang et al., 2009; Bonaz et al., 2013; Cai et al., 2014; Levine et al., 2014a,b, Levine et al., 2018a,b; Dawson et al., 2016; Guiraud et al., 2016; Koopman et al., 2016; Pruitt et al., 2016; Kanashiro et al., 2018), many of which are characterized by inflammation. Extensive pre-clinical evidence has demonstrated the utility of VNS in treating inflammatory conditions (Huston et al., 2006, 2007; Rosas-Ballina et al., 2008; Levine et al., 2014a,b; Olofsson et al., 2015), and a recent clinical study of rheumatoid arthritis (Koopman et al., 2016) further supports this treatment for widespread application. As VNS is applied to a broader range of conditions, it is important to recognize factors that influence study outcomes, such as stimulation settings, vagus nerve physiology, anatomical location of the target nerve branch, and electrode design. However, in many reports published thus far, these factors are often either not discussed, or are described as “customized” (i.e., as in the case of electrode design). A comprehensive discussion is therefore needed to inform the scientific design and reproducible execution of VNS studies.
The current review will provide a stepwise overview to inform the administration of VNS in rodent models, which often form the basis for higher-order study models. Key experimental conditions are discussed, including vagus nerve physiology, electrode design, stimulation equipment, microsurgical technique, and electrical stimulation parameters. Each step includes a detailed rationale to help inform modifications. Although a recent article outlined the surgical procedure for acute rodent VNS (Le Maitre et al., 2017), that method involved only a single stimulation and subsequent removal of the electrode. The current protocol will extend this work by outlining all of the steps necessary to conduct a full-scale sub-acute to chronic VNS study with an implanted cuff electrode. Here, we offer a practical guide to support pre-clinical VNS testing, anticipating the application of VNS for new clinical indications.
The vagus nerve is the tenth and longest cranial nerve and the primary mediator of the parasympathetic branch of the autonomic nervous system (Tracey, 2002; Bonaz et al., 2013). It also regulates immune system homeostasis through an intrinsic “cholinergic anti-inflammatory pathway” (Tracey, 2002; Bonaz et al., 2013). The vagus is a mixed nerve, largely composed of afferent sensory (∼80%) and efferent motor (∼20%) nerve fibers (George et al., 2003, 2004; Groves and Brown, 2005), the composition differing depending on the anatomical location of the nerve (Prechtl and Powley, 1990). The vagus nerve contains three main fiber types: A-, B-, and C-fibers, which are distinguished by fiber diameter, myelination, and activation thresholds (Heck et al., 2002; Groves and Brown, 2005; Ruffoli et al., 2011). A-fibers are large and myelinated (5–20 μm in diameter) and are activated by the lowest amount of current (0.01–0.2 mA) (Schnitzlein et al., 1958; Groves and Brown, 2005; Vuckovic et al., 2008; Ruffoli et al., 2011). B-fibers are mid-sized and myelinated (∼1–3 μm in diameter) and are also activated by low currents (0.04–0.6 mA) (Schnitzlein et al., 1958; Groves and Brown, 2005; Vuckovic et al., 2008; Ruffoli et al., 2011). C-fibers, which constitute the majority of vagus nerve fibers (∼65–80% of afferent fibers), are small and unmyelinated (0.4–2 μm in diameter) and require the highest activation currents (greater than 2.0 mA) (Schnitzlein et al., 1958; Heck et al., 2002; Groves and Brown, 2005; Vuckovic et al., 2008; Ruffoli et al., 2011; Yoo et al., 2013). Although the distribution of the vagus nerve has been shown to be comparable in some species (Mackay and Andrews, 1983; Asala and Bower, 1986), the morphology of the nerve changes depending on the anatomical location (Agostoni et al., 1957; Hoffman and Schnitzlein, 1961; McAllen and Spyer, 1978; Mei et al., 1980; Jammes et al., 1982; Powley et al., 1983; Prechtl and Powley, 1990; Henry, 2002; Ruffoli et al., 2011; Clancy et al., 2013; Hammer et al., 2015, 2018; Verlinden et al., 2015; Bonaz et al., 2016; Yuan and Silberstein, 2016; Planitzer et al., 2017).
Choosing an appropriate anatomical location to deliver stimulation is important when designing a VNS study. In most cases, VNS is delivered to the cervical vagus nerve (George et al., 2004; Howland, 2014) using an implanted electrode. Clinically, this anatomical location is the most common site for immune-modulation and treating epilepsy and depression (Lomarev et al., 2002; Nemeroff et al., 2006; Ben-Menachem et al., 2015; Koopman et al., 2016; Giordano et al., 2017). The left and right cervical branches differentially innervate the heart (O’Toole et al., 1986; Berthoud and Neuhuber, 2000; Ruffoli et al., 2011), where the right vagus nerve has more direct projections to the cardiac atria (Henry, 2002; Groves and Brown, 2005) and thus has a greater influence on heart rate. For this reason, left-sided VNS has been recommended to avoid adverse cardiovascular effects in humans (Henry, 2002; Groves and Brown, 2005; Van Leusden et al., 2015), even though equivalent anti-seizure effects are observed with either left, or right cervical VNS (Krahl et al., 2003; Navas et al., 2010). In rodent models, this lateral difference is not as clear and may differ depending on the stimulation parameters used (Stauss, 2017).
Anatomical differences in vagus innervation may be useful to help researchers adjust stimulation parameters to achieve specific, clinically relevant outcomes. Although most clinical applications target the left cervical vagus nerve, certain conditions may benefit from targeting different anatomical branches. For example, VNS applied to the right cervical vagus nerve is currently being explored to treat heart failure, where direct cardiac effects are desired (Li et al., 2004; Howland, 2014). Morphological differences in the right and left cervical vagus nerve branches (Verlinden et al., 2015) may also explain different clinical effects. Specifically, it was recently reported that both cervical nerve branches contain tyrosine hydroxylase- and dopamine β-hydroxylase-positive nerve fibers, but that the right cervical branch has a larger surface area and double the number of tyrosine hydroxylase-positive nerve fibers (Verlinden et al., 2015). These findings may inform the use of VNS for select cholinergic or adrenergic effects (Onkka et al., 2013; Seki et al., 2014; Verlinden et al., 2015).
Anatomical differences between the cervical vagus nerves may be less important in the treatment of other conditions, likely due to the abundant crossover of fibers between branches of the vagus nerve (Berthoud and Neuhuber, 2000). For example, in a recent study, no significant differences in inflammatory cytokines were found in animals receiving unilateral VNS to the left cervical vagus nerve compared to those receiving bilateral stimulation (VNS applied to the right and left nerve branches) (Olofsson et al., 2015). Laterality concerns may also be less pertinent for the emergent interest in transcutaneous VNS at the auricular or cervical branch (Howland, 2014; Ben-Menachem et al., 2015) and subdiaphragmatic VNS (Greenway and Zheng, 2007; de Lartigue, 2016). It remains to be determined whether the same stimulation parameters can be used for different vagus nerve branches or implanted vs. non-implanted modalities. Further research is needed to elucidate stimulation parameters for each clinical indication and comparative efficacy for implanted vs. non-implanted approaches. Here, the current review will focus on the most common clinical and pre-clinical stimulation site, the left cervical vagus nerve (Howland, 2014), using an implanted electrode. As rodents are commonly used in physiological studies with clinical relevance and form the basis for higher-order models, this overview will discuss specifications pertinent to mouse and rat models in a clinically relevant context, starting with electrode design and implantation, and concluding with stimulation parameters.
The electrode design is an important factor to consider when planning a VNS study. In the most common clinical deployment using a can-and-lead system, the cervical vagus nerve is encircled with bipolar helical electrodes, and a pulse generator is implanted in the chest wall (Bonaz et al., 2013; Giordano et al., 2017). The electrode configuration consists of two spiral electrodes placed around the vagus nerve: the cathode is placed cranial and the anode caudal. A third helical tethering anchor is also placed around the nerve, directly caudal to the anode to provide strain relief (Pisapia and Baltuch, 2016; Giordano et al., 2017).
Electrodes used in rodents may differ, depending on the research objectives and study length (ranging from acute to chronic stimulation). The electrode configuration can include many designs (e.g., cuff or hook, and the inclusion of recording electrodes). A recent VNS methods paper described a needle electrode that was placed under the left cervical vagus nerve during a single stimulation (Le Maitre et al., 2017). For research involving multiple stimulation treatments, we implanted a bipolar cuff electrode with embedded sutures around the nerve (see Figure 1). Cuff electrodes are used in acute and chronic implantation to prevent current leakage into the surrounding tissue. We applied a strain-relieving suture close to the deployment site (detailed below), and the attached lead and pin connector was then tunneled under the skin and externalized at the base of the neck. Figure 2 depicts the externalized connector and an awake animal receiving stimulation. Stimulation was delivered with a commercially available external pulse generator and current-controlled stimulus isolator (see Figure 2), described below.
Figure 1. Different sized (0.3 and 0.5 mm) cuff electrodes with connectors. Embedded sutures on the electrode can facilitate the surgical implant (inset). Other important electrode elements described in the text are identified. Cuff image reproduced with permission from MicroProbes for Life Science (Gaithersburg, MD).
Figure 2. Left panel (top to bottom): Biphasic current-controlled stimulus isolator and biphasic pulse generator (BSI-1A, BPG-1P, respectively; Bak Electronics Incorporated, Umatilla, FL, United States). Right panel (top to bottom): Omnetics mating plug for the externalized connector and awake animal receiving stimulation. All procedures described and animal photography was performed with approval by the Institutional Animal Care and Use Committee (IACUC) at the University of Miami.
For control conditions (unstimulated), a sham electrode can be made by implanting a silicon tube that is the same size as the electrode. This relatively inexpensive inactive design is helpful for feasibility testing, as it compensates for the mechanical stimulation that occurs when the nerve is manipulated (Huston et al., 2007). However, other variables (e.g., the weight of the electrode and tension from the connecting wire-embedded lead) may influence study outcomes, and it is best to have a control condition that consists of an identical implanted electrode that does not receive electricity or a cuff and lead constructed without wires.
In animal experimentation, placement of the cathode and anode are typically not reported. Animal research often includes study factors not relevant to clinical practice, such as stimulation of the distal nerve trunk of a vagotomized animal (Borovikova et al., 2000; de Jonge et al., 2005). In a recent rodent study, no significant differences in inflammatory cytokines were found with either rostral or caudal placement of the cathodic lead (Olofsson et al., 2015), likely because action potentials are generated in both directions when an axon is depolarized. Additional research is needed to understand how cathodic placement and pulse parameters can be modified for specific treatment indications, a topic that is currently being explored (Ardell et al., 2017; Patel et al., 2017; Stauss, 2017).
Although many research groups construct their own electrodes, they can also be purchased and customized through commercial vendors. Nerve cuff electrodes can be made from several conductive materials, including platinum, platinum-iridium, and stainless steel. These electrodes are typically designed with monopolar, bipolar, or tripolar configurations, referring to the number of independent electrical contacts within the cuff. The tradeoff is generally increased control over current flow with a greater number of contacts, but also greater cost and cuff length. Monopolar electrodes are cheaper but require a return or ground, and current paths are less controllable. Bipolar electrodes are more expensive but allow better control over current flow than monopolar, as most of the current will flow directly between the two adjacent contacts. Bipolar cuff electrodes may be more practical and are widely used in pre-clinical research when the cost is a determining factor, especially in pilot studies. Tripolar electrodes may be connected in a pseudo-tripolar configuration (the two external electrodes linked together to form a common anode), which prevents current from leaking out of the cuff.
The size of the target nerve will determine the size of the electrode, as the inner diameter of the cuff should be approximately 1.4 times the outer diameter of the nerve. Use of a cuff that is too small could damage the nerve, and one that is too large could lead to insufficient surface contact or excessive current leakage (Agnew and McCreery, 1990; Yoshida and Riso, 2004). We used nerve cuffs with an inner diameter of 0.3 mm for both mice and small rats and 0.5 mm for larger rats. These sizes were informed by our experience and by published reports (Olofsson et al., 2015).
Besides inner diameter, other modifications include the number of contacts, the distance between contacts, and distance from the contacts to the end of the cuff. The number of contacts is determined by the stimulation paradigm desired. The distance between contacts (“inter-electrode spacing”) we used was 0.5 mm for mice and small rats and 1 mm for larger rats. The distance from the last contact to the end of the cuff is typically three times the distance between contacts to prevent significant current leakage outside the cuff. This distance can be much smaller in pseudo-tripolar electrode configuration. Other custom modifications can be made to suit the research experiment, for example, using an angled cuff design, a multichannel omnetics connector on the externalized cuff lead, embedded suture material on the cuff for convenient closure, suture rings exiting from the base of the connector, and protective silicone tubing around the wiring. A deep review of electrode design is beyond the scope of the current article, but has been reviewed extensively elsewhere (Loeb and Peck, 1996; Merrill et al., 2005; Foldes et al., 2011; Dweiri et al., 2016; Caravaca et al., 2017).
Finally, stimulation conditions in acute vs. chronic studies may differ, as it has been shown that connective tissue forms around the cuff within the first 2 months of implantation (Agnew and McCreery, 1990; Helmers et al., 2012). While this scar tissue will bind the nerve and cuff together and prevent movement abrasion, it can also increase the impedance, causing an increase in the amount of stimulation voltage required to excite the nerve (Agnew and McCreery, 1990; Helmers et al., 2012). Furthermore, it was recently shown that chronic cuffing of the vagus nerve changes the integrity of the nerve fibers, likely due to the inflammatory response to the foreign material (Somann et al., 2018). Although the inflammatory response should resolve upon the formation of fibrous tissue, in some instances, it can negatively affect the integrity of the nerve, including demyelination (Tyler and Durand, 2003; Thil et al., 2007; Somann et al., 2018). It is currently unknown whether this process affects afferent and efferent vagus nerve fibers equally, but it is a potential source of study variability that should be considered when planning a chronic VNS study (Somann et al., 2018). Recent advancements in cuff design show promise in addressing some of these concerns (Caravaca et al., 2017).
Commercially available equipment can be used to deliver a charge-balanced, biphasic square-wave pulse to a bipolar cuff electrode (Levine et al., 2014b; Olofsson et al., 2015). For investigators wanting readily available equipment, we have outlined the specifications related to one vendor, Bak Electronics Incorporated (Umatilla, FL, United States), but many of these specifications are also relevant to other vendors. For our studies, we delivered biphasic (cathodic-leading) stimulation (Levine et al., 2014b; Olofsson et al., 2015) using an external biphasic pulse generator and current-controlled biphasic stimulus isolator (BPG-1P and BSI-1A, respectively). Gently restrained rodents (without anesthesia) were connected to the equipment with a mating plug for stimulation delivery (De Herdt et al., 2009; Zhang et al., 2009). See Figure 2 for equipment configuration and stimulation. Stimulation can be delivered for a specific period (e.g., 60 s) using the manual trigger or it can be digitally triggered or gated to a particular stimulus with a laptop configuration. The waveform design of one charge-balanced, biphasic, cathodic-leading square pulse cycle is represented in Figure 3.
Figure 3. A depiction of the waveform of one charge-balanced, biphasic, cathodic-leading square pulse cycle, showing pulse width (PW), inter-phase interval (IPI), and pulse amplitude (PA). These components have been explained in detail elsewhere (Merrill et al., 2005). Image adapted with permission (Levine et al., 2014b; Olofsson et al., 2015) under the Creative Commons Attribution License.
The delivery specifications of each VNS “treatment” will depend on the physiological outcome measure to be studied. For example, in the context of anti-inflammatory effects, it has been shown that VNS delivered 24–48 h prior to endotoxin exposure resulted in a significant reduction in tumor necrosis factor (TNF) cytokine upon exposure (Huston et al., 2007). This period of therapeutic effects suggests that for certain indications (e.g., conditions characterized by an exaggerated inflammatory response to stimuli), VNS may be administered prophylactically or at specific intervals. As VNS treatment will likely be tailored to clinical symptoms, additional work is needed to determine the temporal response of VNS for other indications. Finally, it is important to include a measurable variable to determine stimulation effectiveness and rule out potential sources of study variability, such as incorrect placement or faulty electrodes, or mechanical damage to the nerve. One option is to record evoked potentials from the vagus nerve after VNS, for example, by stimulating the cervical branch and recording from the subdiaphragmatic branch (Olofsson et al., 2015). This approach can be taken just prior to euthanasia, or immediately after implantation; however, recording from the vagus nerve is technically challenging to perform in rodents (Silverman et al., 2018). To circumvent some of these challenges, investigators can record in a smaller cohort of animals, use electrodes that perform both stimulation and recording, or obtain electromyography (EMG) recordings of the laryngeal muscles that are innervated by the vagus. Another common option is to use heart rate to verify effective cuff placement, where stimulation is increased until a change in heart rate is observed (Levine et al., 2019). At a minimum, we recommend to visually inspect the nerve at euthanasia to ensure that it is still within the cuff and, when possible, to perform histology on the excised nerve to verify axon integrity.
The stepwise surgical procedure detailed below involves the placement of an electrode on the LEFT cervical vagus nerve. The lateral configuration will change if implanting on the RIGHT nerve branch. All survival surgeries should be performed with aseptic technique while the animal is anesthetized, as recommended by the local Institutional Animal Care and Use Committee. Standard post-operative care should also be administered, including hydration and analgesia. With the animal lying prone, make a small opening (∼2 mm) in the skin at the craniovertebral junction, then place the animal in supine position. Palpate the sternal notch. Using scissors, carefully make a vertical incision at the neck, 3 mm caudal to the sternal notch. Dissect the subcutaneous space and extend the midline opening toward the jaw. Identify the inferior border of the thyroid tissue (“V” shaped distinct white line at the cervico-sternal junction). Blunt dissect the thyroid tissue along this line and reflect rostrally. Using a curved, narrow hemostat, create a subcutaneous tunnel toward the opening made at the craniovertebral junction. Bring the electrode through the tunnel and secure to the side of the incision site with tape or weighted instruments. Using blunt-tipped surgical hooks or modified needle syringes as hooks, reflect the thyroid superiorly and the left sternocleidomastoid inferiorly to expose the carotid fossa. No cutting of subcutaneous tissue should be done at any time.
Isolate the left carotid sheath from the connective tissue using pickup forceps and gentle opening-closing movements parallel to the vessels. Identify the nerve (white in appearance) located directly behind or adjacent to the carotid artery. Note, gentle dissection can be accomplished with small, blunt-tipped forceps, minimizing the risk of vessel puncture; however, sharp forceps can also be carefully used to separate the nerve from the carotid artery. Gently dissect the nerve circumferentially taking care not to damage the vessels or tug on the nerve, and place background material under the nerve (a small piece of sterile glove will serve this purpose). Feed the suture strings from the same side of the electrode cuff under the nerve. Advance the electrode cuff under the nerve. Open the cuff by pulling on the opposing sutures, allow the nerve to slide into the open cuff, and secure the cuff by tying the suture strings (see Figure 4). If the visualized nerve is very thin, it is also possible to encircle the entire carotid sheath in mice without isolating the nerve (Olofsson et al., 2015). Attach the lead body to the subcutaneous tissue with a non-absorbable suture (e.g., 4–0 Webcryl) placed approximately 5 mm away from the cuff. This suture will secure the electrode and provide strain relief. With the animal lying prone, feed the excess encased wires evenly into the subcutaneous space (if necessary, create a pocket with forceps by gentle opening-closing movements). Secure the connector to the skin with non-absorbable suture (using embedded suture rings or suture carefully placed through the rubber encasement) and close the skin opening. Before closing the neck incision, place the animal back in a supine position to inspect the electrode and ensure the cuff is encircling the nerve without twisting or pulling. Close the incision on the neck. During surgery, care should be taken to avoid excessive manipulation of the nerve to prevent axonal damage, and, as has been reported, mechanical activation of neuroimmune reflexes (Huston et al., 2007).
Figure 4. Surgical preparation of the electrode implant. Close up (inset), where the white dotted line lies parallel to the nerve lying inside the cuff. All procedures described and animal photography was performed with approval by the Institutional Animal Care and Use Committee (IACUC) at the University of Miami.
Many of the lessons learned in the application of VNS to treat epilepsy are generalizable to other VNS applications, although stimulation should be optimized to the specific treatment condition. Several important parameters can be adjusted when delivering stimulation to the vagus nerve: output current, pulse width, pulse frequency, and duty cycle (i.e., “ON” and “OFF” time) (Heck et al., 2002; Groves and Brown, 2005; Wheless et al., 2018). Collectively, these parameters determine the total amount of electrical energy delivered to the vagus nerve during treatment (Heck et al., 2002). Current guidelines in epilepsy include output currents between 0.25 and 3.5 mA and pulse frequencies ranging between 20 and 30 Hz (Heck et al., 2002; Groves and Brown, 2005; Ruffoli et al., 2011). Continuously applied, high-frequency (50–100 Hz) current leads to irreversible axonal injury, which can be avoided by reducing the frequency to 20 Hz (Agnew and McCreery, 1990). These findings and others showing optimal pulse frequencies for seizure reduction ranging from 10 to 30 Hz (Woodbury and Woodbury, 1990; Zabara, 1992) led to current FDA-approved guidelines (Groves and Brown, 2005). These settings also correspond with stimulation parameters reported in clinical trials of depression (Rush et al., 2000, 2005a,b) and a recent clinical study of rheumatoid arthritis (Koopman et al., 2016). Once a target fiber type is identified, stimulation can be adjusted within approved limits.
The output current is the stimulation parameter typically adjusted first (Heck et al., 2002). A tolerable range of current can be used to target specific nerve fibers and achieve clinical efficacy. Initially, VNS treatment for clinical epilepsy was thought to activate C-fibers, an approach that coincided with observations of progressive anti-epileptic effects with increasing current (Heck et al., 2002). This approach was not without consequence, as elevated currents are less tolerable and increase adverse effects, such as bradycardia, dyspnea, and throat tightness (Heck et al., 2002). The need to increase output current to achieve clinical efficacy (DeGiorgio et al., 2000, 2001) has been questioned and may have been influenced by the high currents used for non-responders (Heck et al., 2002). It has since been shown that C-fiber activation is not required for anticonvulsant effects (Krahl et al., 2001; Henry, 2002; Ruffoli et al., 2011) and that currents above 2 mA may be unnecessary for most patients (Heck et al., 2002).
The second parameter to consider is the pulse width, the duration of a single stimulation pulse (Labiner and Ahern, 2007), which can be adjusted to avoid the neural damage associated with continuous stimulation (Agnew and McCreery, 1990). Rodent seizure models report optimal pulse widths as high as 1,000 μs; however, the pulse width is inversely related to tolerance in people (Heck et al., 2002). Fortunately, this parameter can be adjusted to improve tolerance without loss of efficacy, for example, by shortening the pulse width from 500 μs to 250 μs (Heck et al., 2002). Pulse width is inversely related to the current required to stimulate a nerve (Heck et al., 2002; Labiner and Ahern, 2007; Levine et al., 2019), and together these two parameters determine the total charge per pulse (Labiner and Ahern, 2007; Levine et al., 2019). Although shorter and longer pulse durations may be preferred in different clinical applications (Agnew and McCreery, 1990), this is an area of active investigation (Mu et al., 2004; Kong et al., 2012; Aaronson et al., 2013; Loerwald et al., 2018). Thus, adjusting pulse width in concert with output current can meet stimulation requirements and reduce the risks of excessive stimulation current, while achieving a balance of clinical efficacy and tolerability.
A third parameter, pulse frequency (number of pulses per second), is less often varied in studies compared to other stimulation settings. Pulse frequencies that are generally used in rodent seizure models are similar to those used clinically, ranging from 10 to 30 Hz (Heck et al., 2002). For treatment indications besides epilepsy, the utility of specific pulse frequencies remains under investigation. In clinical practice, it may be helpful to use the natural firing rates of specific fiber types to guide pulse frequency (Bonaz et al., 2013). For example, it has been reported that the physiological firing frequency of A- and C-fibers is above and below 10 Hz, respectively (Binks et al., 2001). It is currently unclear whether pulse frequency can be used to preferentially stimulate afferent vs. efferent fibers without the use of chemical or electrical nerve block (Osharina et al., 2006; Bonaz et al., 2013; Olofsson et al., 2015; Patel et al., 2017; Patel and Butera, 2018), as generally, when an axon is depolarized beneath a cathodic electrode, action potentials travel in both directions.
To date, pulse frequencies that are responsible for specific clinical effects remain to be determined; however, as more studies are performed, we will better understand whether a combination of stimulation settings will be useful in targeting specific nerve fibers within efferent and afferent pathways [e.g., motor A-type or sensory group I, II, and III fibers (Yoo et al., 2013)]. The use of different stimulation paradigms to selectively target different fiber types remains an area of active investigation (Vuckovic et al., 2004, 2008; Howland, 2014; Peclin and Rozman, 2014; Guiraud et al., 2016; Patel et al., 2017; Nuntaphum et al., 2018; Patel and Butera, 2018).
A final parameter that affects stimulation is the duty cycle (ON/OFF cycle) (Heck et al., 2002; Labiner and Ahern, 2007). The standard duty cycle to treat persons with epilepsy is 30 s ON/5 min OFF (Heck et al., 2002). Although shorter OFF times can improve clinical efficacy, rapid cycling (i.e., very short duty cycles) is much more energy intensive and may not be necessary for effective treatment (Heck et al., 2002), at least for anti-seizure use. A more in-depth discussion on considerations regarding electrical stimulation can be found in previous reviews (Merrill et al., 2005; Mortimer and Bhadra, 2009; Cogan et al., 2018; Grill, 2018). As an example using our equipment setup (Figure 2), a stimulation “treatment” consisting of symmetrical biphasic square pulses with current intensity of 500 μA, 250 μs pulse width, and 50 μs inter-phase interval at 10 Hz for 60 s (Olofsson et al., 2015) can be delivered with the following settings. Stimulus isolator: 100 μA of constant current, AC coupling, continuous stimulation, and an input gain of 1. Pulse generator: 5.0 amplitude (5 × 100 μA × gain of 1), 250 μs pulse width, 100 ms period. It should be noted that the pulse period is the inverse of the desired frequency (i.e., 100 ms = 1/10 Hz × 1000 ms/s). To summarize, the stimulation “treatment” using the parameters from our previous example will consist of a 550 μs pulse (250 μs for each phase of the pulse, plus 50 μs inter-phase interval), and a 99,450 μs inter-pulse interval (total of 100,000 μs period).
Commonly reported VNS adverse effects include cough, throat tightening or discomfort, shortness of breath, voice alterations, and cardiovascular symptoms, such as bradycardia (Ben-Menachem, 2001; Heck et al., 2002; Ben-Menachem et al., 2015; Jacobs et al., 2015). These transient effects are limited to when the device is actively stimulating and are proportional to increases in output current, pulse width, frequency, and duty cycle (Heck et al., 2002; Jacobs et al., 2015; Olofsson et al., 2015). Pulmonary effects are mainly associated with C-fiber activation (Banzett et al., 1999; Heck et al., 2002; Henry, 2002), which were previously associated with cardiovascular effects. However, it has been shown that cardioinhibitory effects can be attributed to activation of B-fibers (Jones et al., 1995; Banzett et al., 1999; Yoo et al., 2016; McAllen et al., 2018; Qing et al., 2018). Importantly, no evidence of “clinically relevant” bradycardia has been reported for stimulation within FDA-approved guidelines (Heck et al., 2002). It is also notable that anti-inflammatory and cardioinhibitory effects are separable (Huston et al., 2007), further indicating that stimulation parameters can be tailored for precise, clinically relevant outcomes.
Early anti-epileptic work targeted C-fibers under the assumption that these abundant afferent fibers mediate the clinical effects of VNS (George et al., 2003, 2004; Groves and Brown, 2005; Yoo et al., 2013). As such, early VNS treatment utilized the high output currents needed to stimulate C-fibers (Heck et al., 2002). As A-, B-, and C-fibers are successively recruited with increased electrical current (Woodbury and Woodbury, 1990; Yoo et al., 2013), subsequent research demonstrated anticonvulsant effects without specifically targeting C-fibers, thus allowing for smaller amounts of current (Krahl et al., 2001; Henry, 2002; Ruffoli et al., 2011). The activation thresholds of A- and B-fibers overlap, but both require substantially less current than C-fibers (Groves and Brown, 2005; Castoro et al., 2011), which, when activated, are associated with most of the reported adverse effects (Heck et al., 2002; Henry, 2002).
As the field has progressed, this iterative process of associating specific fiber types with therapeutic effects has occurred in the use of VNS for other indications, where anti-inflammatory effects have been attributed to A-fibers (Huston et al., 2007) and B-fibers (Olofsson et al., 2015). Importantly, recent research shows that minimal stimulation can achieve beneficial therapeutic outcomes. A seminal pre-clinical study demonstrated that a minimal amount of current (0.5 mA) activated the inflammatory reflex in both mice and rats (Olofsson et al., 2015), effects of which were observed up to 2.5 mA. These output currents remain below FDA-approved levels (Heck et al., 2002) and are similar to currents used clinically for rheumatoid arthritis (Koopman et al., 2016). Thus, minimized stimulation may provide therapeutic benefits while avoiding the adverse effects associated with higher output currents (Heck et al., 2002).
Decades of research has brought extensive progress to the field of neuromodulation, and specifically to the clinical use of techniques such as VNS. Although VNS is a promising neuromodulation tool, it has been challenging to incorporate study findings from clinical and pre-clinical research. Pre-clinical VNS work often involves mechanistic study aspects not employed in clinical settings, such as the use of lidocaine to block efferent or afferent signaling or electrical stimulation of nerve stumps after vagotomy (Borovikova et al., 2000; de Jonge et al., 2005; Niederbichler et al., 2009; Olofsson et al., 2015). In clinical VNS application, decisions regarding stimulation parameters may not be explicitly defined, such as the number of treatment sessions; similarly, a clear rationale for pulse-design modifications are often not addressed. In addition to pulse frequencies, several other key stimulation parameters can influence study outcomes and reproducibility; however, selection of specific treatment parameters are often not detailed or are simply reported as “customized.” Furthermore, as activation thresholds may differ depending on conditions of stimulation, it is critical that study conditions are outlined in ongoing research efforts.
These issues highlight the challenge of translating rodent work to clinical application. The inflammatory reflex appears to be conserved across species; where anti-inflammatory effects are observed with similar stimulation parameters in rodents with endotoxemia (Olofsson et al., 2015), rodents with collagen-induced arthritis (Levine et al., 2014b), and persons with rheumatoid arthritis (Koopman et al., 2016). However, it is unknown whether specific fiber types that mediate the reflex are similarly conserved. Additionally, as disease-related factors may influence VNS pathways, stimulation may be most effective if delivered at specific time points of disease progression. These and other questions remain to be determined and highlight the importance of translating pre-clinical findings to heterogeneous clinical populations.
The current review aims to advance VNS research by providing a comprehensive discussion on performing pre-clinical VNS studies in rodent models. We have provided a microsurgical technique, discussed stimulation equipment, and provided a rationale for choosing electrode design and electrical stimulation settings. We outlined how a combination of clinically relevant stimulation parameters can be adjusted to achieve selected therapeutic effects. Indeterminate issues are discussed and presented as avenues for future research.
CN, YL, TU, JA, and MN conceived, structured, and participated in writing and revising the review.
This work was supported in part by a project grant from the State of Florida to The Miami Project to Cure Paralysis (W. Dalton Dietrich, PI), a pilot grant from the Morton Cure Paralysis Fund awarded to CN, and an individual NIDILRR Mary Switzer Fellowship awarded to CN (90SFGE0006).
YL is an employee of SetPoint Medical Corporation, a company that is developing bioelectronic devices to target the vagus nerve in humans.
The remaining authors declare that the research was conducted in the absence of any commercial or financial relationships that could be construed as a potential conflict of interest.
We acknowledge Dr. Alexander Marcillo for his surgical assistance and April Mann for her assistance in the technical review of the manuscript.
Aaronson, S. T., Carpenter, L. L., Conway, C. R., Reimherr, F. W., Lisanby, S. H., Schwartz, T. L., et al. (2013). Vagus nerve stimulation therapy randomized to different amounts of electrical charge for treatment-resistant depression: acute and chronic effects. Brain Stimul. 6, 631–640. doi: 10.1016/j.brs.2012.09.013
Agnew, W. F., and McCreery, D. B. (1990). Considerations for safety with chronically implanted nerve electrodes. Epilepsia 31(Suppl. 2), S27–S32.
Agostoni, E., Chinnock, J. E., De Daly, M. B., and Murray, J. G. (1957). Functional and histological studies of the vagus nerve and its branches to the heart, lungs and abdominal viscera in the cat. J. Physiol. 135, 182–205. doi: 10.1113/jphysiol.1957.sp005703
Ardell, J. L., Nier, H., Hammer, M., Southerland, E. M., Ardell, C. L., Beaumont, E., et al. (2017). Defining the neural fulcrum for chronic vagus nerve stimulation: implications for integrated cardiac control. J. Physiol. 595, 6887–6903. doi: 10.1113/JP274678
Asala, S. A., and Bower, A. J. (1986). An electron microscope study of vagus nerve composition in the ferret. Anat. Embryol. 175, 247–253. doi: 10.1007/bf00389602
Banzett, R. B., Guz, A., Paydarfar, D., Shea, S. A., Schachter, S. C., and Lansing, R. W. (1999). Cardiorespiratory variables and sensation during stimulation of the left vagus in patients with epilepsy. Epilepsy Res. 35, 1–11. doi: 10.1016/s0920-1211(98)00126-0
Ben-Menachem, E. (2001). Vagus nerve stimulation, side effects, and long-term safety. J. Clin. Neurophysiol. 18, 415–418. doi: 10.1097/00004691-200109000-00005
Ben-Menachem, E., Revesz, D., Simon, B. J., and Silberstein, S. (2015). Surgically implanted and non-invasive vagus nerve stimulation: a review of efficacy, safety and tolerability. Eur. J. Neurol. 22, 1260–1268. doi: 10.1111/ene.12629
Berthoud, H. R., and Neuhuber, W. L. (2000). Functional and chemical anatomy of the afferent vagal system. Auton. Neurosci. 85, 1–17. doi: 10.1016/s1566-0702(00)00215-0
Binks, A. P., Paydarfar, D., Schachter, S. C., Guz, A., and Banzett, R. B. (2001). High strength stimulation of the vagus nerve in awake humans: a lack of cardiorespiratory effects. Respir. Physiol. 127, 125–133. doi: 10.1016/s0034-5687(01)00252-3
Bonaz, B., Picq, C., Sinniger, V., Mayol, J. F., and Clarencon, D. (2013). Vagus nerve stimulation: from epilepsy to the cholinergic anti-inflammatory pathway. Neurogastroenterol. Motil. 25, 208–221. doi: 10.1111/nmo.12076
Bonaz, B., Sinniger, V., and Pellissier, S. (2016). Anti-inflammatory properties of the vagus nerve: potential therapeutic implications of vagus nerve stimulation. J. Physiol. 594, 5781–5790. doi: 10.1113/JP271539
Borovikova, L. V., Ivanova, S., Zhang, M., Yang, H., Botchkina, G. I., Watkins, L. R., et al. (2000). Vagus nerve stimulation attenuates the systemic inflammatory response to endotoxin. Nature 405, 458–462. doi: 10.1038/35013070
Cai, P. Y., Bodhit, A., Derequito, R., Ansari, S., Abukhalil, F., Thenkabail, S., et al. (2014). Vagus nerve stimulation in ischemic stroke: old wine in a new bottle. Front. Neurol. 5:107. doi: 10.3389/fneur.2014.00107
Caravaca, A. S., Tsaava, T., Goldman, L., Silverman, H., Riggott, G., Chavan, S. S., et al. (2017). A novel flexible cuff-like microelectrode for dual purpose, acute and chronic electrical interfacing with the mouse cervical vagus nerve. J. Neural. Eng. 14:066005. doi: 10.1088/1741-2552/aa7a42
Castoro, M. A., Yoo, P. B., Hincapie, J. G., Hamann, J. J., Ruble, S. B., Wolf, P. D., et al. (2011). Excitation properties of the right cervical vagus nerve in adult dogs. Exp. Neurol. 227, 62–68. doi: 10.1016/j.expneurol.2010.09.011
Clancy, J. A., Deuchars, S. A., and Deuchars, J. (2013). The wonders of the wanderer. Exp. Physiol. 98, 38–45. doi: 10.1113/expphysiol.2012.064543
Cogan, S. F., Hara, S., and Ludwig, K. A. (2018). “Chapter 7 - the safe delivery of electrical currents and neuromodulation,” in Neuromodulation, 2 Edn, eds E. S. Krames, P. H. Peckham, and A. R. Rezai, (Cambridge, MA: Academic Press), 83–94. doi: 10.1016/b978-0-12-805353-9.00007-3
Conway, C. R., Gott, B. M., and Azhar, N. H. (2016). “Vagus nerve stimulation for treatment-refractory depression,” in Neuromodulation in Psychiatry, eds C. Hamani, P. Holtzheimer, A. M. Lozano, and H. Mayberg, (Hoboken: John Wiley & Sons), 335–352. doi: 10.1002/9781118801086.ch18
Dawson, J., Pierce, D., Dixit, A., Kimberley, T. J., Robertson, M., Tarver, B., et al. (2016). Safety, feasibility, and efficacy of vagus nerve stimulation paired with upper-limb rehabilitation after ischemic stroke. Stroke 47, 143–150. doi: 10.1161/STROKEAHA.115.010477
De Herdt, V., Puimege, L., De Waele, J., Raedt, R., Wyckhuys, T., El Tahry, R., et al. (2009). Increased rat serum corticosterone suggests immunomodulation by stimulation of the vagal nerve. J. Neuroimmunol. 212, 102–105. doi: 10.1016/j.jneuroim.2009.04.013
de Jonge, W. J., van der Zanden, E. P., The, F. O., Bijlsma, M. F., van Westerloo, D. J., Bennink, R. J., et al. (2005). Stimulation of the vagus nerve attenuates macrophage activation by activating the Jak2-STAT3 signaling pathway. Nat. Immunol. 6, 844–851. doi: 10.1038/ni1229
de Lartigue, G. (2016). Role of the vagus nerve in the development and treatment of diet-induced obesity. J. Physiol. 594, 5791–5815. doi: 10.1113/JP271538
DeGiorgio, C. M., Schachter, S. C., Handforth, A., Salinsky, M., Thompson, J., Uthman, B., et al. (2000). Prospective long-term study of vagus nerve stimulation for the treatment of refractory seizures. Epilepsia 41, 1195–1200.
DeGiorgio, C. M., Thompson, J., Lewis, P., Arrambide, S., Naritoku, D., Handforth, A., et al. (2001). Vagus nerve stimulation: analysis of device parameters in 154 patients during the long-term XE5 study. Epilepsia 42, 1017–1020. doi: 10.1046/j.1528-1157.2001.0420081017.x
Dweiri, Y. M., Stone, M. A., Tyler, D. J., McCallum, G. A., and Durand, D. M. (2016). Fabrication of high contact-density, flat-interface nerve electrodes for recording and stimulation applications. J. Vis. Exp. 116:54388. doi: 10.3791/54388
Foldes, E. L., Ackermann, D. M., Bhadra, N., Kilgore, K. L., and Bhadra, N. (2011). Design, fabrication and evaluation of a conforming circumpolar peripheral nerve cuff electrode for acute experimental use. J. Neurosci. Methods 196, 31–37. doi: 10.1016/j.jneumeth.2010.12.020
George, M. S., Nahas, Z., Bohning, D. E., Mu, Q., Andrew Kozel, F., Borckhardt, J., et al. (2004). Mechanisms of action of vagus nerve stimulation (VNS). Clin. Neurosci. Res. 4, 71–79. doi: 10.1016/j.cnr.2004.06.006
George, M. S., Rush, A. J., Sackeim, H. A., and Marangell, L. B. (2003). Vagus nerve stimulation (VNS): utility in neuropsychiatric disorders. Int. J. Neuropsychopharmacol. 6, 73–83. doi: 10.1017/s1461145703003250
Giordano, F., Zicca, A., Barba, C., Guerrini, R., and Genitori, L. (2017). Vagus nerve stimulation: surgical technique of implantation and revision and related morbidity. Epilepsia 58(Suppl. 1), 85–90. doi: 10.1111/epi.13678
Greenway, F., and Zheng, J. (2007). Electrical stimulation as treatment for obesity and diabetes. J. Diabetes Sci. Technol. 1, 251–259. doi: 10.1177/193229680700100216
Grill, W. M. (2018). “Chapter 8 - waveforms for neural stimulation,” in Neuromodulation, 2Edn Edn, eds E. S. Krames, P. H. Peckham, and A. R. Rezai, (Cambridge, MA: Academic Press), 95–102. doi: 10.1016/b978-0-12-805353-9.00008-5
Groves, D. A., and Brown, V. J. (2005). Vagal nerve stimulation: a review of its applications and potential mechanisms that mediate its clinical effects. Neurosci. Biobehav. Rev. 29, 493–500. doi: 10.1016/j.neubiorev.2005.01.004
Guiraud, D., Andreu, D., Bonnet, S., Carrault, G., Couderc, P., Hagege, A., et al. (2016). Vagus nerve stimulation: state of the art of stimulation and recording strategies to address autonomic function neuromodulation. J. Neural. Eng. 13:041002. doi: 10.1088/1741-2560/13/4/041002
Hammer, N., Glatzner, J., Feja, C., Kuhne, C., Meixensberger, J., Planitzer, U., et al. (2015). Human vagus nerve branching in the cervical region. PLoS One 10:e0118006. doi: 10.1371/journal.pone.0118006
Hammer, N., Loffler, S., Cakmak, Y. O., Ondruschka, B., Planitzer, U., Schultz, M., et al. (2018). Cervical vagus nerve morphometry and vascularity in the context of nerve stimulation - A cadaveric study. Sci. Rep. 8:7997. doi: 10.1038/s41598-018-26135-8
Heck, C., Helmers, S. L., and DeGiorgio, C. M. (2002). Vagus nerve stimulation therapy, epilepsy, and device parameters: scientific basis and recommendations for use. Neurology 59(6 Suppl. 4), S31–S37.
Helmers, S. L., Begnaud, J., Cowley, A., Corwin, H. M., Edwards, J. C., Holder, D. L., et al. (2012). Application of a computational model of vagus nerve stimulation. Acta Neurol. Scand. 126, 336–343. doi: 10.1111/j.1600-0404.2012.01656.x
Henry, T. R. (2002). Therapeutic mechanisms of vagus nerve stimulation. Neurology 59(6 Suppl. 4), S3–S14.
Hoffman, H. H., and Schnitzlein, H. N. (1961). The numbers of nerve fibers in the vagus nerve of man. Anat. Rec. 139, 429–435. doi: 10.1002/ar.1091390312
Huston, J. M., Gallowitsch-Puerta, M., Ochani, M., Ochani, K., Yuan, R., Rosas-Ballina, M., et al. (2007). Transcutaneous vagus nerve stimulation reduces serum high mobility group box 1 levels and improves survival in murine sepsis. Crit. Care Med. 35, 2762–2768. doi: 10.1097/00003246-200712000-00014
Huston, J. M., Ochani, M., Rosas-Ballina, M., Liao, H., Ochani, K., Pavlov, V. A., et al. (2006). Splenectomy inactivates the cholinergic antiinflammatory pathway during lethal endotoxemia and polymicrobial sepsis. J. Exp. Med. 203, 1623–1628. doi: 10.1084/jem.20052362
Jacobs, H. I., Riphagen, J. M., Razat, C. M., Wiese, S., and Sack, A. T. (2015). Transcutaneous vagus nerve stimulation boosts associative memory in older individuals. Neurobiol. Aging 36, 1860–1867. doi: 10.1016/j.neurobiolaging.2015.02.023
Jammes, Y., Fornaris, E., Mei, N., and Barrat, E. (1982). Afferent and efferent components of the bronchial vagal branches in cats. J. Auton. Nerv. Syst. 5, 165–176. doi: 10.1016/0165-1838(82)90037-6
Jones, J. F., Wang, Y., and Jordan, D. (1995). Heart rate responses to selective stimulation of cardiac vagal C fibres in anaesthetized cats, rats and rabbits. J. Physiol. 489(Pt 1), 203–214. doi: 10.1113/jphysiol.1995.sp021042
Kanashiro, A., Shimizu Bassi, G., de Queiroz Cunha, F., and Ulloa, L. (2018). From neuroimunomodulation to bioelectronic treatment of rheumatoid arthritis. Bioelectron. Med. 1, 151–165. doi: 10.2217/bem-2018-0001
Kong, S. S., Liu, J. J., Hwang, T. C., Yu, X. J., Zhao, M., Zhao, M., et al. (2012). Optimizing the parameters of vagus nerve stimulation by uniform design in rats with acute myocardial infarction. PLoS One 7:e42799. doi: 10.1371/journal.pone.0042799
Koopman, F. A., Chavan, S. S., Miljko, S., Grazio, S., Sokolovic, S., Schuurman, P. R., et al. (2016). Vagus nerve stimulation inhibits cytokine production and attenuates disease severity in rheumatoid arthritis. Proc. Natl. Acad. Sci. U.S.A. 113, 8284–8289. doi: 10.1073/pnas.1605635113
Krahl, S. E., Senanayake, S. S., and Handforth, A. (2001). Destruction of peripheral C-fibers does not alter subsequent vagus nerve stimulation-induced seizure suppression in rats. Epilepsia 42, 586–589. doi: 10.1046/j.1528-1157.2001.09700.x
Krahl, S. E., Senanayake, S. S., and Handforth, A. (2003). Right-sided vagus nerve stimulation reduces generalized seizure severity in rats as effectively as left-sided. Epilepsy Res. 56, 1–4. doi: 10.1016/s0920-1211(03)00122-0
Kumar, A., Bunker, M. T., Aaronson, S. T., Conway, C. R., Rothschild, A. J., Mordenti, G., et al. (2019). Durability of symptomatic responses obtained with adjunctive vagus nerve stimulation in treatment-resistant depression. Neuropsychiatr. Dis. Treat. 15, 457–468. doi: 10.2147/NDT.S196665
Labiner, D. M., and Ahern, G. L. (2007). Vagus nerve stimulation therapy in depression and epilepsy: therapeutic parameter settings. Acta Neurol. Scand. 115, 23–33. doi: 10.1111/j.1600-0404.2006.00732.x
Lainez, M. J., and Guillamon, E. (2017). Cluster headache and other TACs: Pathophysiology and neurostimulation options. Headache 57, 327–335. doi: 10.1111/head.12874
Le Maitre, E., Revathikumar, P., Estelius, J., and Lampa, J. (2017). Increased Recovery time and decreased LPS administration to study the vagus nerve stimulation mechanisms in limited inflammatory responses. J. Vis. Exp. 121, 54890. doi: 10.3791/54890
Levine, Y. A., Faltys, M., and Chernoff, D. (2019). Harnessing the inflammatory reflex for the treatment of inflammation-mediated diseases. Cold Spring Harb. Perspect. Med. doi: 10.1101/cshperspect.a034330 [Epub ahead of print].
Levine, Y. A., Koopman, F., Faltys, M., Zitnik, R., and Tak, P.-P. (2014a). Neurostimulation of the cholinergic antiinflammatory pathway in rheumatoid arthritis and inflammatory bowel disease. Bioelectron. Med. 1, 34–43. doi: 10.15424/bioelectronmed.2014.00008
Levine, Y. A., Koopman, F. A., Faltys, M., Caravaca, A., Bendele, A., Zitnik, R., et al. (2014b). Neurostimulation of the cholinergic anti-inflammatory pathway ameliorates disease in rat collagen-induced arthritis. PLoS One 9:e104530. doi: 10.1371/journal.pone.0104530
Levine, Y. A., Faltys, M., and Zitnik, R. (2018a). “Chapter 126 - Activation of the inflammatory reflex in rheumatoid arthritis and inflammatory bowel disease; preclinical evidence, in Neuromodulation, 2nd Edn. eds. E. S. Krames, P. H. Peckham and A. R., Rezai, (Cambridge, MA: Academic Press), 1493–1502.
Levine, Y. A., Simon, J., Faltys, M., and Zitnik, R. (2018b). “Chapter 127 - Bioelectronic therapy for the treatment of rheumatoid arthritis and inflammatory bowel disease,” in Neuromodulation, 2nd Edn. eds. E. S. Krames, P. H. Peckham and A. R. Rezai, (Cambridge, MA: Academic Press) 1503–1511.
Li, M., Zheng, C., Sato, T., Kawada, T., Sugimachi, M., and Sunagawa, K. (2004). Vagal nerve stimulation markedly improves long-term survival after chronic heart failure in rats. Circulation 109, 120–124. doi: 10.1161/01.cir.0000105721.71640.da
Loeb, G. E., and Peck, R. A. (1996). Cuff electrodes for chronic stimulation and recording of peripheral nerve activity. J. Neurosci. Methods 64, 95–103. doi: 10.1016/0165-0270(95)00123-9
Loerwald, K. W., Borland, M. S., Rennaker, R. L. II, Hays, S. A., and Kilgard, M. P. (2018). The interaction of pulse width and current intensity on the extent of cortical plasticity evoked by vagus nerve stimulation. Brain Stimul. 11, 271–277. doi: 10.1016/j.brs.2017.11.007
Lomarev, M., Denslow, S., Nahas, Z., Chae, J. H., George, M. S., and Bohning, D. E. (2002). Vagus nerve stimulation (VNS) synchronized BOLD fMRI suggests that VNS in depressed adults has frequency/dose dependent effects. J. Psychiatr. Res. 36, 219–227. doi: 10.1016/s0022-3956(02)00013-4
Mackay, T. W., and Andrews, P. L. (1983). A comparative study of the vagal innervation of the stomach in man and the ferret. J. Anat. 136(Pt 3), 449–481.
McAllen, R. M., Shafton, A. D., Bratton, B. O., Trevaks, D., and Furness, J. B. (2018). Calibration of thresholds for functional engagement of vagal A, B and C fiber groups in vivo. Bioelectron. Med. 1, 21–27. doi: 10.2217/bem-2017-0001
McAllen, R. M., and Spyer, K. M. (1978). Two types of vagal preganglionic motoneurones projecting to the heart and lungs. J. Physiol. 282, 353–364. doi: 10.1113/jphysiol.1978.sp012468
Mei, N., Condamin, M., and Boyer, A. (1980). The composition of the vagus nerve of the cat. Cell Tissue Res. 209, 423–431.
Merrill, D. R., Bikson, M., and Jefferys, J. G. (2005). Electrical stimulation of excitable tissue: design of efficacious and safe protocols. J. Neurosci. Methods 141, 171–198. doi: 10.1016/j.jneumeth.2004.10.020
Mortimer, J. T., and Bhadra, N. (2009). “Chapter 11 - fundamentals of electrical stimulation,” in Neuromodulation, eds E. S. Krames, P. H. Peckham, and A. R. Rezai, (San Diego: Academic Press), 109–121. doi: 10.1016/b978-0-12-374248-3.00012-4
Mu, Q., Bohning, D. E., Nahas, Z., Walker, J., Anderson, B., Johnson, K. A., et al. (2004). Acute vagus nerve stimulation using different pulse widths produces varying brain effects. Biol. Psychiatry 55, 816–825. doi: 10.1016/j.biopsych.2003.12.004
Navas, M., Navarrete, E. G., Pascual, J. M., Carrasco, R., Nunez, J. A., Shakur, S. F., et al. (2010). Treatment of refractory epilepsy in adult patients with right-sided vagus nerve stimulation. Epilepsy Res. 90, 1–7. doi: 10.1016/j.eplepsyres.2010.04.007
Nemeroff, C. B., Mayberg, H. S., Krahl, S. E., McNamara, J., Frazer, A., Henry, T. R., et al. (2006). VNS therapy in treatment-resistant depression: clinical evidence and putative neurobiological mechanisms. Neuropsychopharmacology 31, 1345–1355. doi: 10.1038/sj.npp.1301082
Niederbichler, A. D., Papst, S., Claassen, L., Jokuszies, A., Steinstraesser, L., Hirsch, T., et al. (2009). Burn-induced organ dysfunction: vagus nerve stimulation attenuates organ and serum cytokine levels. Burns 35, 783–789. doi: 10.1016/j.burns.2008.08.023
Nuntaphum, W., Pongkan, W., Wongjaikam, S., Thummasorn, S., Tanajak, P., Khamseekaew, J., et al. (2018). Vagus nerve stimulation exerts cardioprotection against myocardial ischemia/reperfusion injury predominantly through its efferent vagal fibers. Basic Res. Cardiol. 113:22. doi: 10.1007/s00395-018-0683-0
Olofsson, P. S., Levine, Y. A., Caravaca, A., Chavan, S. S., Pavlov, V. A., Faltys, M., et al. (2015). Single-pulse and unidirectional electrical activation of the cervical vagus nerve reduces tumor necrosis factor in endotoxemia. Bioelectron. Med. 2, 37–42. doi: 10.15424/bioelectronmed.2015.00006
Onkka, P., Maskoun, W., Rhee, K. S., Hellyer, J., Patel, J., Tan, J., et al. (2013). Sympathetic nerve fibers and ganglia in canine cervical vagus nerves: localization and quantitation. Heart Rhythm 10, 585–591. doi: 10.1016/j.hrthm.2012.12.015
Osharina, V., Bagaev, V., Wallois, F., and Larnicol, N. (2006). Autonomic response and Fos expression in the NTS following intermittent vagal stimulation: importance of pulse frequency. Auton. Neurosci. 12, 72–80. doi: 10.1016/j.autneu.2006.03.011
O’Toole, M. F., Ardell, J. L., and Randall, W. C. (1986). Functional interdependence of discrete vagal projections to SA and AV nodes. Am. J. Physiol. 251(2 Pt 2), H398–H404.
Patel, Y. A., and Butera, R. J. (2018). Challenges associated with nerve conduction block using kilohertz electrical stimulation. J. Neural. Eng. 15:031002. doi: 10.1088/1741-2552/aaadc0
Patel, Y. A., Saxena, T., Bellamkonda, R. V., and Butera, R. J. (2017). Kilohertz frequency nerve block enhances anti-inflammatory effects of vagus nerve stimulation. Sci. Rep. 7:39810. doi: 10.1038/srep39810
Peclin, P., and Rozman, J. (2014). Alternative paradigm of selective vagus nerve stimulation tested on an isolated porcine vagus nerve. Sci. World J. 2014:310283. doi: 10.1155/2014/310283
Pisapia, J., and Baltuch, G. (2016). “Vagus nerve stimulation,” in Neuromodulation in Psychiatry, eds C. Hamani, P. Holtzheimer, A. M. Lozano, and H. Mayberg, (Hoboken: John Wiley & Sons), 325–334.
Planitzer, U., Hammer, N., Bechmann, I., Glatzner, J., Loffler, S., Mobius, R., et al. (2017). Positional relations of the cervical vagus nerve revisited. Neuromodulation 20, 361–368. doi: 10.1111/ner.12557
Powley, T. L., Prechtl, J. C., Fox, E. A., and Berthoud, H. R. (1983). Anatomical considerations for surgery of the rat abdominal vagus: distribution, paraganglia and regeneration. J. Auton. Nerv. Syst. 9, 79–97. doi: 10.1016/0165-1838(83)90133-9
Prechtl, J. C., and Powley, T. L. (1990). The fiber composition of the abdominal vagus of the rat. Anat. Embryol. 181, 101–115.
Pruitt, D. T., Schmid, A. N., Kim, L. J., Abe, C. M., Trieu, J. L., Choua, C., et al. (2016). Vagus nerve stimulation delivered with motor training enhances recovery of function after traumatic brain injury. J. Neurotrauma. 33, 871–879. doi: 10.1089/neu.2015.3972
Qing, K. Y., Wasilczuk, K. M., Ward, M. P., Phillips, E. H., Vlachos, P. P., Goergen, C. J., et al. (2018). B fibers are the best predictors of cardiac activity during Vagus nerve stimulation. Bioelectron. Med. 4, 5.
Rosas-Ballina, M., Ochani, M., Parrish, W. R., Ochani, K., Harris, Y. T., Huston, J. M., et al. (2008). Splenic nerve is required for cholinergic antiinflammatory pathway control of TNF in endotoxemia. Proc. Natl. Acad. Sci. U.S.A. 105, 11008–11013. doi: 10.1073/pnas.0803237105
Ruffoli, R., Giorgi, F. S., Pizzanelli, C., Murri, L., Paparelli, A., and Fornai, F. (2011). The chemical neuroanatomy of vagus nerve stimulation. J. Chem. Neuroanat. 42, 288–296. doi: 10.1016/j.jchemneu.2010.12.002
Rush, A. J., George, M. S., Sackeim, H. A., Marangell, L. B., Husain, M. M., Giller, C., et al. (2000). Vagus nerve stimulation (VNS) for treatment-resistant depressions: a multicenter study∗∗see accompanying editorial, in this issue. Biol. Psychiatry 47, 276–286. doi: 10.1016/s0006-3223(99)00304-2
Rush, A. J., Marangell, L. B., Sackeim, H. A., George, M. S., Brannan, S. K., Davis, S. M., et al. (2005a). Vagus nerve stimulation for treatment-resistant depression: a randomized, controlled acute phase trial. Biol. Psychiatry 58, 347–354. doi: 10.1016/j.biopsych.2005.05.025
Rush, A. J., Sackeim, H. A., Marangell, L. B., George, M. S., Brannan, S. K., Davis, S. M., et al. (2005b). Effects of 12 months of vagus nerve stimulation in treatment-resistant depression: a naturalistic study. Biol. Psychiatry 58, 355–363. doi: 10.1016/j.biopsych.2005.05.024
Schnitzlein, H. N., Rowe, L. C., and Hoffman, H. H. (1958). The myelinated component of the vagus nerves in man. Anat. Rec. 131, 649–667. doi: 10.1002/ar.1091310404
Seki, A., Green, H. R., Lee, T. D., Hong, L., Tan, J., Vinters, H. V., et al. (2014). Sympathetic nerve fibers in human cervical and thoracic vagus nerves. Heart Rhythm 11, 1411–1417. doi: 10.1016/j.hrthm.2014.04.032
Silverman, H. A., Stiegler, A., Tsaava, T., Newman, J., Steinberg, B. E., Masi, E. B., et al. (2018). Standardization of methods to record Vagus nerve activity in mice. Bioelectron. Med. 4:3.
Somann, J. P., Albors, G. O., Neihouser, K. V., Lu, K. H., Liu, Z., Ward, M. P., et al. (2018). Chronic cuffing of cervical vagus nerve inhibits efferent fiber integrity in rat model. J. Neural. Eng. 15:036018. doi: 10.1088/1741-2552/aaa039
Stauss, H. M. (2017). Differential hemodynamic and respiratory responses to right and left cervical vagal nerve stimulation in rats. Physiol. Rep. 5, e13244. doi: 10.14814/phy2.13244
Thil, M.-A., Duy, D. T., Colin, I. M., and Delbeke, J. (2007). Time course of tissue remodelling and electrophysiology in the rat sciatic nerve after spiral cuff electrode implantation. J. Neuroimmunol. 185, 103–114. doi: 10.1016/j.jneuroim.2007.01.021
Tyler, D. J., and Durand, D. M. (2003). Chronic response of the rat sciatic nerve to the flat interface nerve electrode. Ann. Biomed. Eng. 31, 633–642. doi: 10.1114/1.1569263
Van Leusden, J. W., Sellaro, R., and Colzato, L. S. (2015). Transcutaneous vagal nerve stimulation (tVNS): a new neuromodulation tool in healthy humans? Front. Psychol. 6:102.
Verlinden, T. J., Rijkers, K., Hoogland, G., and Herrler, A. (2015). Morphology of the human cervical vagus nerve: implications for vagus nerve stimulation treatment. Acta Neurol. Scand. 133, 173–182. doi: 10.1111/ane.12462
Vuckovic, A., Rijkhoff, N. J., and Struijk, J. J. (2004). Different pulse shapes to obtain small fiber selective activation by anodal blocking–a simulation study. IEEE Trans. Biomed. Eng. 51, 698–706. doi: 10.1109/tbme.2004.826663
Vuckovic, A., Tosato, M., and Struijk, J. J. (2008). A comparative study of three techniques for diameter selective fiber activation in the vagal nerve: anodal block, depolarizing prepulses and slowly rising pulses. J. Neural. Eng. 5, 275–286. doi: 10.1088/1741-2560/5/3/002
Wheless, J. W., Gienapp, A. J., and Ryvlin, P. (2018). Vagus nerve stimulation (VNS) therapy update. Epilepsy Behav. 88s, 2–10. doi: 10.1016/j.yebeh.2018.06.032
Woodbury, D. M., and Woodbury, J. W. (1990). Effects of Vagal stimulation on experimentally induced seizures in rats. Epilepsia 31, S7–S19.
Yoo, P. B., Liu, H., Hincapie, J. G., Ruble, S. B., Hamann, J. J., and Grill, W. M. (2016). Modulation of heart rate by temporally patterned vagus nerve stimulation in the anesthetized dog. Physiol. Rep. 4:e12689. doi: 10.14814/phy2.12689
Yoo, P. B., Lubock, N. B., Hincapie, J. G., Ruble, S. B., Hamann, J. J., and Grill, W. M. (2013). High-resolution measurement of electrically-evoked vagus nerve activity in the anesthetized dog. J. Neural. Eng. 10:026003. doi: 10.1088/1741-2560/10/2/026003
Yoshida, K., and Riso, R. (2004). “Peripheral nerve recording electrodes and techniques,” in Neuroprosthetics - Theory And Practice, eds K. W. Horch, and G. S. Dhillon, (Singapore: World Scientific), 683–744. doi: 10.1142/9789812561763_0021
Yuan, H., and Silberstein, S. D. (2016). Vagus nerve and vagus nerve stimulation, a comprehensive review: part I. Headache 56, 71–78. doi: 10.1111/head.12647
Zabara, J. (1992). Inhibition of experimental seizures in canines by repetitive vagal stimulation. Epilepsia 33, 1005–1012. doi: 10.1111/j.1528-1157.1992.tb01751.x
Zhang, Y., Popovic, Z. B., Bibevski, S., Fakhry, I., Sica, D. A., Van Wagoner, D. R., et al. (2009). Chronic vagus nerve stimulation improves autonomic control and attenuates systemic inflammation and heart failure progression in a canine high-rate pacing model. Circ. Heart Fail. 2, 692–699. doi: 10.1161/CIRCHEARTFAILURE.109.873968
Keywords: vagus nerve stimulation, vagus nerve, neuromodulation, nerve cuff electrode, electrical stimulation
Citation: Noller CM, Levine YA, Urakov TM, Aronson JP and Nash MS (2019) Vagus Nerve Stimulation in Rodent Models: An Overview of Technical Considerations. Front. Neurosci. 13:911. doi: 10.3389/fnins.2019.00911
Received: 20 March 2019; Accepted: 16 August 2019;
Published: 04 September 2019.
Edited by:
Mikhail Lebedev, Duke University, United StatesReviewed by:
Stefan Kampusch, Vienna University of Technology, AustriaCopyright © 2019 Noller, Levine, Urakov, Aronson and Nash. This is an open-access article distributed under the terms of the Creative Commons Attribution License (CC BY). The use, distribution or reproduction in other forums is permitted, provided the original author(s) and the copyright owner(s) are credited and that the original publication in this journal is cited, in accordance with accepted academic practice. No use, distribution or reproduction is permitted which does not comply with these terms.
*Correspondence: Crystal M. Noller, Q3J5c3RhbC5NLk5vbGxlckBkYXJ0bW91dGguZWR1
Disclaimer: All claims expressed in this article are solely those of the authors and do not necessarily represent those of their affiliated organizations, or those of the publisher, the editors and the reviewers. Any product that may be evaluated in this article or claim that may be made by its manufacturer is not guaranteed or endorsed by the publisher.
Research integrity at Frontiers
Learn more about the work of our research integrity team to safeguard the quality of each article we publish.