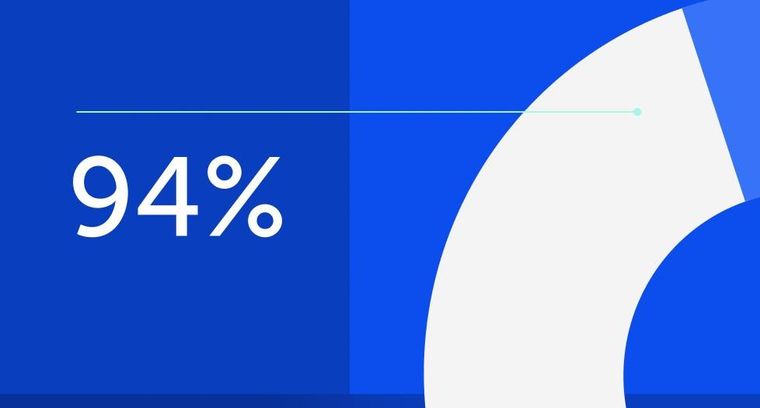
94% of researchers rate our articles as excellent or good
Learn more about the work of our research integrity team to safeguard the quality of each article we publish.
Find out more
REVIEW article
Front. Neurosci., 16 August 2019
Sec. Neurodegeneration
Volume 13 - 2019 | https://doi.org/10.3389/fnins.2019.00868
This article is part of the Research TopicBrain Insulin Resistance in Neurodevelopmental and Neurodegenerative Disorders: Mind the Gap!View all 15 articles
The advances in medicine, together with lifestyle modifications, led to a rising life expectancy. Unfortunately, however, aging is accompanied by an alarming boost of age-associated chronic pathologies, including neurodegenerative and metabolic diseases. Interestingly, a non-negligible interplay between alterations of glucose homeostasis and brain dysfunction has clearly emerged. In particular, epidemiological studies have pointed out a possible association between Type 2 Diabetes (T2D) and Parkinson’s Disease (PD). Insulin resistance, one of the major hallmark for etiology of T2D, has a detrimental influence on PD, negatively affecting PD phenotype, accelerating its progression and worsening cognitive impairment. This review aims to provide an exhaustive analysis of the most recent evidences supporting the key role of insulin resistance in PD pathogenesis. It will focus on the relevance of insulin in the brain, working as pro-survival neurotrophic factor and as a master regulator of neuronal mitochondrial function and oxidative stress. Insulin action as a modulator of dopamine signaling and of alpha-synuclein degradation will be described in details, too. The intriguing idea that shared deregulated pathogenic pathways represent a link between PD and insulin resistance has clinical and therapeutic implications. Thus, ongoing studies about the promising healing potential of common antidiabetic drugs such as metformin, exenatide, DPP IV inhibitors, thiazolidinediones and bromocriptine, will be summarized and the rationale for their use to decelerate neurodegeneration will be critically assessed.
The prevalence of aging-associated chronic pathologies, such as neurodegenerative and metabolic diseases, has dramatically increased along with life expectancy (Zochodne and Malik, 2014). Type 2 Diabetes (T2D) embraces almost 90% of all cases of diabetes and actually represents a major public health problem worldwide. Chronic hyperglycemia is the hallmark of T2D, resulting from insulin resistance and beta cell dysfunction, and is associated with long-term complications, including retinopathy, nephropathy, micro and macro vascular diseases. More recently, experimental, clinical and neuroimaging data provided evidence of a connection between T2D and brain injury (Hamed, 2017). T2D-associated brain injury is largely linked to hyperglycemia and involves several different pathological events, such as oxidative stress (Muriach et al., 2014), mitochondrial dysfunction (Shokrzadeh et al., 2018), neuroinflammation (Rom et al., 2018), decrease of neurotrophins (Franco-Robles et al., 2014), modification of neurotransmitters (Datusalia and Sharma, 2014), vascular derangements (Kooistra et al., 2013), amyloid β deposition (Wang X. et al., 2014), increased tau phosphorylation (Platt et al., 2016) and progressive cognitive dysfunction (Simo et al., 2017). Epidemiological studies also support the evidence of a crosstalk linking T2D and neurodegenerative disorders (Morsi et al., 2018). In particular, an interesting association between T2D and PD has recently emerged from clinical, experimental and genome-wide association studies (Biosa et al., 2018; De Pablo-Fernandez et al., 2018).
Deterioration of dopaminergic neurons in the extrapyramidal tract of the midbrain is the trigger for PD pathogenesis, resulting from an interplay of genetic and environmental factors (Olanow et al., 2009). Most of the time PD is a sporadic disease, but few cases have genetic origin and several genes associated to PD have been found (Hernandez et al., 2016). Impairment of the dopaminergic neurons leads to a reduction in dopamine signaling and may lead to a relative increase in acetylcholine release from cholinergic neurons in the striatum, thereby contributing to dyskinesia (Heumann et al., 2014). Other typical motor symptoms of PD are bradykinesia, resting tremor, muscular rigidity and abnormal posture and gait (Olanow et al., 2009). In many cases of PD, loss of dopaminergic neurons in the substantia nigra is accompanied by the formation of intracellular neuronal inclusions composed of alpha-synuclein, known as Lewy bodies, in the central, autonomic, and peripheral nervous system. The diagnosis of PD is essentially based on the neurological examination, aimed to identification of characteristic motor signs, deriving from the loss of nigral dopaminergic neurons. The presence of a sustained response to dopamine drugs (dopamine agonists or levodopa) is also commonly used in diagnosis. Several non-motor symptoms are associated to PD, too. They include hyposmia, sleep behavior disorder, loss of olfaction, constipation, depression and global cognitive decline and precede the clinical effects of dopamine deficiency, sometimes for several years (Schapira et al., 2017). Unlike motor symptoms, non-motor symptoms of PD are not improved by dopamine replacement therapy and seem to derive from the formation of Lewy bodies beyond midbrain dopaminergic neurons (Dickson et al., 2009). Cognitive impairment and dementia are the most disabling non-motor symptoms of PD, resulting from microvascular disease (Kim J.S. et al., 2014), deposition of Lewy bodies in neocortical and limbic areas, hyperphosphorylated tau-containing neurofibrillary tangles and formation of amyloid-beta-peptide plaques (Irwin et al., 2013).
The onset of diabetes appears to increase severity of symptoms in PD patients (Sandyk, 1993), and epidemiological studies suggest that diabetes is a risk factor for PD (Hu et al., 2007; Cereda et al., 2012). Several studies have tried to explain how T2D affects pathogenesis and progression of PD. In 1993, Sandyk (1993) found a relationship between PD and T2D, evidencing that up to 50–80% of patients with PD featured an altered glucose tolerance in response to a glucose load. Some years later, Schernhammer et al. (2011) evaluated a population of 1.931 cases and 9.651 controls, evidencing a 36% increased risk of developing PD among patients with T2D. Similarly, a major risk of developing PD among individuals with T2D was found in the study conducted by Sun et al. (2012). In this case-control study, by examining a Chinese population of 603.416 diabetics and comparing it with a diabetes-free control, they found that diabetic women had a higher incidence of PD compared to men. Moreover, young diabetic men aged 21–40 years or diabetic women aged 41–60 years were more susceptible to the risk of Parkinsonism. Additional studies have suggested a positive association between PD risk and T2D. In particular, Hu et al. (2007) have studied a Finnish population of 51.552 individuals, both men and women, aged between 25 and 74, without a history of PD at baseline, concluding that T2D is associated with an increased risk of PD. Very recently, De Pablo-Fernandez et al. (2018) have found an association between diabetes and PD in a retrospective study, where a cohort of 2,017,115 individuals admitted for hospital treatment with a codified diagnosis of type 2 diabetes was compared with a reference cohort of 6,173,208 people without diabetes.
Nevertheless, there is also opposite evidence, pointing out a lower risk of PD incidence in subjects with T2D (Powers et al., 2006) and an inverse association of hyperglycemia with the onset of PD in individuals without any neurodegenerative disease (Miyake et al., 2010). These conflicting results could be due to confounding sampling of the different populations. For instance, in the report performed by Miyake et al. (2010), T2D diagnosis is based on the filling up of self-reported questionnaires. An additional source of confusion may be that the considered populations are too small to obtain significant results. Differences in study design and methodology and the difficulty to rule out confounders (such as microvascular damage and diabetic treatment) as risk factors for PD negatively affect data reproducibility, too. However, notwithstanding the heterogeneity of the data, the existence of a positive association between T2D and PD has been recently supported by interventional studies showing a reduction in incidence of PD in T2D patients treated with antidiabetic drugs such as metformin, sulfonylureas and exenatide, which exert neuro-protection (Wahlqvist et al., 2012; Aviles-Olmos et al., 2013). Several lines of evidence suggest that impairment of insulin signaling increase the risk of PD (Morris et al., 2008; Bosco et al., 2012; Ashraghi et al., 2016; Pang et al., 2016). Indeed, it has been recently found that insulin resistance, the impaired responsiveness to insulin, typical of T2D, occurs in PD brains and plays a key role in the progressive development of PD pathological hallmarks. In this review, we examine the relevance of insulin signaling in brain, especially for dopaminergic function, the relationship between insulin resistance and PD and finally we give an overview of the rationale underlying the use of drugs currently used for T2D in PD patients.
Insulin is a peptide hormone secreted in response to postprandial hyperglycemia from pancreatic beta−cells in blood circulation. Historically, insulin was essentially known as the main regulator of peripheral glucose homeostasis, since it induces glucose uptake in adipose tissue and skeletal muscle and glycogen synthesis in the liver, inhibiting in parallel hepatic glycogenolysis and gluconeogenesis (Haeusler et al., 2018).
In addition to these peripheral targets, insulin also undertakes a neuroregulatory function, although the physiological significance of its role in the brain has only recently started to emerge in both murine models and humans (Schubert et al., 2004; Duarte et al., 2012; Grote and Wright, 2016). Detectable concentrations of insulin have been found in several brain regions, including hypothalamus, olfactory bulb and midbrain since many years (Baskin et al., 1983), but it is not yet clear whether insulin is locally produced in CNS. Experimental evidence supports the hypothesis of insulin biosynthesis in adult neuronal cells derived from the hippocampus and olfactory bulb (Kuwabara et al., 2011) and by pyramidal neurons in the cortex (Dorn et al., 1982). Immunoreactive insulin and C-peptide were found in the brain from human cadavers, and, in situ hybridization showed the presence of insulin mRNA in the periventricular nucleus of the rat hypothalamus (Blazquez et al., 2014). Furthermore, Havrankova et al. (1978) showed the presence of insulin in rat brain at concentrations between 10 and 100 times higher than that in plasma. On the contrary, other studies did not confirm these results, and conclusive evidence for significant amounts of insulin synthesized in brain is lacking (Gray et al., 2014). However, insulin may enter brain parenchyma and precapillary space via a receptor-mediated transport (Duffy and Pardridge, 1987; Banks et al., 1997). Studies performed in an experimental model of human blood brain barrier (BBB) formed by isolated capillaries deriving from fresh human brain autopsy have shown that BBB insulin receptor has physicochemical properties similar to the IRs present in peripheral tissues such as adipocytes and hepatocytes (Pardridge et al., 1985; Plata-Salaman, 1991). Insulin transport to the CNS is reduced in high-fat diet-induced obesity (Kaiyala et al., 2000) and suppressed by hyperglycemia (Banks et al., 1997). In addition, Alzheimer’s disease and aging are associated with a reduction in insulin transport across the BBB (Craft et al., 1998; Frolich et al., 1998). Several studies have been performed in order to assess the integrity of BBB in PD although the results are still unclear. The observation that peripheral decarboxylase inhibitors, such as carbidopa and benserazide, do not reduce levodopa efficacy in brain indicate that BBB integrity is not compromised in parkinsonian patients (Rinne and Molsa, 1979). In support of this hypothesis, current and future therapeutic strategies for PD treatment are based on lipophilic substances or on a direct injection of proteins, genes and cellular therapies into the brain (Christine et al., 2009). Nevertheless, recent studies have also indicated that BBB is damaged in PD patients. Indeed, compromised BBB integrity in the striatum has been observed in postmortem brain tissue from PD patients (Gray and Woulfe, 2015). Furthermore, Dohgu et al. have indicated that monomeric alpha-synuclein induces BBB dysfunction by activating pericytes which, in turn, release inflammatory mediators (Dohgu et al., 2019). In conclusion, it is not possible to establish if insulin resistance in the PD brain arise from altered insulin transport across BBB. Hopefully, in the next future, advances in imaging techniques will allow to more carefully identify the source of insulin in the brain.
Interestingly, Jimenez-Jimenez et al. have compared cerebrospinal fluid (CSF) insulin levels in PD patients and in healthy subjects without finding significant differences between them (Jimenez-Jimenez et al., 2000). In contrast, other experimental evidence has shown that non-diabetic PD patients have increased blood glucose after oral glucose tolerance test without the concomitant rise in insulin levels, probably due to an impaired adaptive insulin secretion (Marques et al., 2018). Thus, the relationship between CSF/brain and serum insulin levels in PD needs to be elucidated. However, the specific role of this hormone in the different brain areas remains undeniable. Indeed, insulin elicits its effects by binding a specific tyrosine kinase receptor, expressed in different brain regions (Plum et al., 2005), including dopaminergic neurons (Figlewicz et al., 2003; Konner et al., 2011). Glucose uptake into neurons is insulin independent, thus in the brain insulin signaling regulates olfaction, mood and memory (McNay et al., 2010; Ketterer et al., 2011; Aime et al., 2012; Kleinridders et al., 2014; Biessels and Reagan, 2015; Heni et al., 2015). In addition, acting on glucosensing neurons of the hypothalamus, insulin modulates peripheral metabolism, hepatic glucose output, food intake, body weight, lipolysis and white adipose tissue browning (Blazquez et al., 2014; Dodd et al., 2015).
Well-characterized insulin functions in the central nervous system are the regulation of apoptosis during neuronal development and the enhancing of neuronal survival. This is not surprising since insulin binding to its receptor (IR) activates several intracellular effectors relevant to cell survival, such as PI3K/Akt pathway. Insulin, indeed, negatively modulates the expression of pro-apoptotic proteins protecting embryonic retinal cells during development from cell death (Diaz et al., 1999). Regarding the increase of neuronal survival, it is known that insulin signaling rescues rat hippocampal cells in culture injured by oxygen or glucose deprivation (Mielke and Wang, 2005) and has neuroprotective effects on H2O2-induced toxicity of retinoic acid (RA)-differentiated SH-SY5Y cells (Ramalingam and Kim, 2014). During the pathogenesis of PD, characterized by death of dopaminergic neurons in the substantia nigra pars compacta, insulin pro-survival ability is particularly relevant and clearly emerged in studies performed in SH-SY5Y cells pretreated with the neurotoxin MPP+ and in animal models (Moroo et al., 1994). In this cellular model of experimental PD, insulin prevented cell death in a dose dependent manner. It inhibits MPP + -induced iNOS and ERK activation, lowering in turn nitric oxide release, reactive oxygen species (ROS), calcium ion influx and finally decreasing the ratio of Bax to Bcl-2 through activating anti-apoptotic PI3K/Akt/GSK3 pathways (Ramalingam and Kim, 2016b).
Another characteristic neuropathologic feature in the PD brain is the accumulation of cytosolic inclusions of fibrillary forms of alpha-synuclein, called Lewy bodies. In C6 astrocytoma cells, a 24 h MPP + treatment induces a significant increase of a helically folded tetramer of alpha-synuclein accompanied by an augmentation of SNCA mRNA levels. Interestingly insulin affects alpha-synuclein expression and aggregation, too, by a mechanism involving the PI3K/Akt pathway (Ramalingam and Kim, 2017; Yang et al., 2018). Indeed, pretreatment with insulin induced a marked decrease in the tetrameric alpha-synuclein, preventing the cytotoxic effect of MPP + (Ramalingam and Kim, 2017). The molecular mechanisms underlying insulin protective action against MPP + neurotoxicity have been better clarified in SH-SY5Y cells, where insulin decreases alpha-synuclein and Cox-2 levels and blocks ROS-induced membrane damage. In parallel, it activates autophagy, integrins and syndecans signaling (Ramalingam and Kim, 2016a). Autophagy modulation by insulin is particularly relevant for PD pathogenesis, since it is crucial for elimination of abnormal and toxic protein aggregates. Insulin, indeed, blocking mTORC1 activity, stimulates autophagy of toxic proteins and activates Akt survival protein, through an mTORC2-mediated mechanism (Heras-Sandoval et al., 2014). The crucial importance of autophagy regulation by insulin is highlighted by the fact that the specific pharmacological inhibition of mTORC1 by rapamycin reduces alpha-synuclein aggregation (Sarkar et al., 2007) and prevents dopaminergic neuron loss (Tain et al., 2009). An additional plausible mechanism by which insulin promotes autophagy and negatively modulates alpha-synuclein toxicity is the inhibitory phosphorylation of GSK3beta by Akt. GSK3beta, indeed, co-localizes with alpha-synuclein in Lewy bodies and its expression is increased in postmortem brain from PD patients (Nagao and Hayashi, 2009) and in experimental models of PD associated with alpha-synuclein accumulation (Golpich et al., 2015). Recent evidences have revealed the presence of the microtubule associated protein tau in Lewy bodies, which is essentially known for its pathological role in Alzheimer disease, but it has recently been shown to participate in PD pathogenesis as well. GSK3beta inactivation by insulin is also involved in insulin-induced inhibition of tau phosphorylation which reduces neurotoxicity, increasing its binding to microtubules (Tokutake et al., 2012). Interestingly, insulin can directly affect alpha-synuclein turnover, reducing its aggregation and toxicity (Kao, 2009). Insulin action on alpha-synuclein aggregation is mediated by activation of IDE (insulin degrading enzyme), a highly conserved Zinc metallopeptidase which degrades amyloidogenic proteins. IDE, in turns, binds to alpha-synuclein oligomers, preventing them from further assembly into amyloid fibers that cause degeneration of dopaminergic neurons in PD patients (Sharma et al., 2015; Figure 1). Experiments performed in specific alpha-synuclein knockout mice have provided contrasting results. Indeed, while Rodriguez-Araujo et al. (2015) suggest that absence of alpha-synuclein in mice is associated with impairment in glucose metabolism during HFD-induced insulin-resistance, Geng et al. (2011) show an increased rate of insulin secretion in alpha-synuclein knockout mice, indicating alpha-synuclein as negative regulator of insulin secretion.
Figure 1. Insulin signaling regulates neuronal function. Insulin binding to its receptor, through the intracellular substrates IRSs, leads to activation of PI3-K pathway which, in turn, inhibits GSK3, mTORC1 and IDE, reducing alpha-synuclein aggregation, and enhances cell survival. In addition, insulin-induced PI3K activation stimulates dopamine synthesis and turnover and mitochondrial biogenesis. This figure includes experimental results obtained in cell cultures and partially confirmed in rodent and human brain.
In addition to modulate alpha-synuclein amount, insulin is able to regulate mitochondrial biogenesis and to directly affect mitochondrial electron transport chain activity through stimulation of the IR/PI3K/Akt pathway, which suppresses FoxO1/HMOX1 induction (Cheng et al., 2010). Importantly, in hippocampal neurons, compounds activating IR also activate the AMPK-SIRT1-PGC1alpha signaling axis, enhancing in parallel mitochondrial function (Barhwal et al., 2015). Insulin’s ability to modulate mitochondrial membrane potential has long been characterized (Huang et al., 2003, 2005), but Aghanoori et al. (2017) recently revealed that insulin also controls mitochondrial function up-regulating mitochondrial electron transport system protein expression and complex activity.
Conversely, experimental models of insulin resistance feature altered levels of mitochondrial proteins in the substantia nigra (Khang et al., 2015), reduced levels of mitochondrial complex I and dysregulated calcium homeostasis (Moreira et al., 2006; Duarte et al., 2012). These phenomena impair mitochondrial biogenesis, inducing membrane depolarization and generation of excessive ROS, oxidative stress and increased cell death (Huang et al., 2003; Kleinridders et al., 2015). The link between mitochondrial dysfunction, insulin resistance and dopaminergic neuronal degeneration probably relies in the disruption of the Parkin-PARIS-PGC1alpha pathway. In chronic insulin resistance condition, indeed, reduced levels of Parkin have been observed in parallel with accumulation of a zinc finger protein, named PARIS, able to repress PGC1alpha expression and highly expressed in the substantia nigra of sporadic PD patients (Khang et al., 2015).
Insulin represents also a master regulator of extracellular events involved in PD pathogenesis, such as microglial activation and increase of pro-inflammatory mediators that contributes to ROS generation. Interestingly, several different pathways downstream IR activation such as PI3K/Akt and p38/MAPK pathways, are involved in TGF-beta1 neuroprotective effect against MPP + -induced neurodegeneration (Liu et al., 2016). Moreover, PI3K/Akt pathway decreases neuroinflammation up-regulating IkBalpha, a selective endogenous blocker of NF-kB, the main transcription factor responsible for expression of inflammatory genes (Khasnavis et al., 2012).
Insulin itself represents also a physiological regulator of dopamine synthesis and clearance. A convincing demonstration for insulin relevance in modulation of dopamine signaling has been provided by the phenotype of NIRKO mice, featuring a neuron-specific knockout of IR. NIRKO mice, indeed, exhibit manifestations of anxiety and depressive-like behaviors. These hallmarks are accompanied by increased dopamine turnover, which in turn leads to decreased dopamine signaling in the striatum and nucleus accumbens. In vitro data indicate that in neuronal cells these alterations arise from a loss of insulin effect on expression of MAO A and MAO B, involved in inactivation of monoamine neurotransmitters (Kleinridders et al., 2015). Moreover, there is evidence in literature that insulin is able to regulate expression of tyrosine hydroxylase (TH), the rate-limiting step in the biosynthesis of dopamine. Insulin in rats was shown to induce a transient increase in TH mRNA in adrenal medulla (Rusnak et al., 1998; Xu et al., 2007). Conversely, pathological states characterized by impaired insulin signaling are associated with alterations of TH expression and/or activity. For instance, in experimental diabetes decreased TH activity in terminal fields for noradrenergic and dopaminergic neurons has been observed (Chu et al., 1986; Glanville and Anderson, 1986; Kono and Takada, 1994) and genetically diabetic Wistar rats show decreased immunoreactive TH (Nascimento et al., 2011). Moreover, in streptozotocin-treated rats, TH mRNA was increased in the locus coeruleus but decreased in the ventral tegmental area/substantia nigra pars compacta (Figlewicz et al., 1996). Part of the mechanism underlying TH modulation by insulin has been recently clarified in PC12 cells, where insulin regulates TH expression through the transcription factors HIF-1alpha and Nur77 (Fiory et al., 2018). These data have evidenced the critical role of insulin signaling in maintaining an appropriate dopaminergic tone by regulating TH expression in the central nervous system. In addition, studies in brain slices, in striatal synaptosomes, and in vivo have shown that insulin activation of IR increases dopamine uptake by the dopamine transporter (DAT). In particular, direct intracerebroventricular infusion of insulin results in increased DAT mRNA levels. Accordingly, when CNS insulin levels were reduced by 24- to 36-h food deprivation, DAT mRNA levels, assessed by in situ hybridization, were significantly decreased in the ventral tegmental area/substantia nigra pars compacta and the Vmax of dopamine uptake was significantly decreased in striatum from fasted rats. Interestingly, in vitro incubation with a physiological concentration of insulin augmented striatal dopamine uptake to control levels (Patterson et al., 1998). Similarly, insulin increases dopamine uptake and modulates DAT trafficking via PI3K in rat striatal synaptosomes (Carvelli et al., 2002). In particular, the key regulator downstream PI3K, responsible for DAT regulation by insulin, is Akt2 (Speed et al., 2010). These results suggest that synaptic dopamine signaling may be altered by reducing the available cell surface DATs in states of chronic hypoinsulinemia, such as diabetes (Carvelli et al., 2002). For instance, high fat feeding, impairs striatal insulin-induced activation of Akt, reducing in turns DAT cell surface expression and function and locomotor responses to amphetamine (Speed et al., 2011). Finally, it has been recently shown that insulin influences food choice amplifying action potential-dependent dopamine release in the nucleus accumbens and caudate-putamen through an indirect mechanism involving striatal cholinergic interneurons that express IR. Furthermore, the sensitivity of striatal dopamine release to insulin in rats is oppositely altered by chronic diet manipulations; indeed, food restriction enhances and obesogenic diet decreases responsiveness to insulin, respectively (Patel et al., 2018). On the other end, there is no known information about insulin-regulated food choice effect on PD onset and/or progression.
Insulin plays an acknowledged role in regulation of memory and cognitive function, too. This is particularly relevant for PD progression, since cognitive impairment represents a significant non-motor symptom of PD. PD patients, indeed, feature more rapid decline in cognitive domains and in memory (Aarsland et al., 2017), exhibiting a cognitive impairment which embraces a spectrum of severity from relatively mild symptoms to end-stage dementia (Davis and Racette, 2016). However, mild cognitive impairment can occur early in the course of PD, while dementia commonly characterizes advanced stages of PD (Hely et al., 2008). Interestingly, the prevalence of cognitive deficit is significantly higher in PD patients with diabetes mellitus than in patients with PD only, suggesting that diabetes may be one risk factor for cognitive dysfunction in PD patients (Yang et al., 2017). However, specific role of insulin in safeguarding cognitive function has been more clearly confirmed by studies showing that PD patients with dementia are prone to comorbid insulin resistance (Bosco et al., 2012; Ashraghi et al., 2016), even when they were unaffected by diabetes. Cognitive decline in PD and progression to dementia derive from alterations in hippocampal structure and function (Bouchard et al., 2008; Costa et al., 2012; Pan et al., 2013). This is plausible, since hippocampal neurons are particularly susceptible to alterations in insulin sensitivity (Fehm et al., 2006). Importantly, a high density of IRs has been found in the hippocampus, cortex and amygdala, where they participate in cognitive functions (Singh et al., 1997; Gerozissis, 2003). Furthermore, acute administration of insulin, through activation of hippocampal IRs, ameliorates performance on memory tasks in rats (Park et al., 2000) and enhances verbal memory and cognition in humans (Kern et al., 2001; Benedict et al., 2004). Insulin effects on cognition involves the PI3K/Akt pathway (McNay and Recknagel, 2011) and is probably mediated by its ability to affect synaptic plasticity. Activation of the PI3K/Akt pathway, indeed, maintains dendritic spine stabilization, necessary for memory consolidation (Goldin and Segal, 2003; Zhao and Townsend, 2009). The crucial insulin effector downstream PI3K/Akt pathway involved in preservation of cognitive function is GSK3beta. Insulin increases GSK3beta inhibitory phosphorylation through PI3K/Akt signaling. The phosphorylation of GSK3 beta, in turn, improves long-term memory in hippocampal-associated tasks, decreases tau and alpha-synuclein accumulation and neurotoxicity and reduces neuroinflammation and apoptosis. In conclusion, insulin alleviates cognitive impairment in PD via the inactivation of GSK3beta mediated by PI3K/Akt (Yang et al., 2018).
Interestingly, patients with PD feature augmented autoimmune reactivity to insulin (Wilhelm et al., 2007). Moreover, in the substantia nigra pars compacta of patients with PD, death of dopaminergic neurons is often anticipated by marked loss of IR mRNA and enhanced levels of IRS phosphorylation at serine residues, with inhibitory action on insulin signaling and subsequent increased insulin resistance (Moroo et al., 1994; Takahashi et al., 1996; Duarte et al., 2012; Morris et al., 2014). In particular, increased levels of IRS-1 pSer312 in the putamen and of pSer616 in hippocampal tissue of PD patients were found (Athauda and Foltynie, 2016). Likewise, both 6-OHDA-induced PD models and alpha-synuclein overexpressing mice show increased IRS phosphorylation at serine residues in the dopamine-depleted striatum (Morris et al., 2008, 2011a,b; Gao et al., 2015). In addition, increased nuclear translocation of PTEN and GSK3beta, paralleled by an impaired insulin signaling cascade, was observed in postmortem substantia nigra from PD patients (Sekar and Taghibiglou, 2018). Similarly, other authors have found decreased Akt phosphorylation in sections of substantia nigra from parkinsonian and control subjects (Malagelada et al., 2008; Timmons et al., 2009). These alterations may contribute to the pathogenesis and/or progression of PD. However, all of these results have been obtained in absence of “ex vivo” stimulation with insulin and, at the best of our knowledge, there is no evidence about the ability of PD postmortem brains to respond to insulin. Thus, the physiological decline in insulin signaling, which represents a typical hallmark of aging (Zhao et al., 2004; Kushner, 2013), is clearly accelerated in PD. On the other hand, the alterations of insulin signaling exacerbate PD clinical-pathological symptoms, enhancing dopaminergic degeneration and worsening disease progression and, in parallel, both motor and cognitive decline (Papapetropoulos et al., 2004). Several studies performed in animal models confirmed the onset of this deleterious crosstalk between insulin resistance and PD. In 2014, Wang and collaborators highlighted the relevance of insulin resistance for PD etiology using ob/ob and db/db mice as T2D model. These mice show insulin signaling impairment, ER stress and inflammation not only in peripheral tissue, but also in midbrain. It is worth of notice that they feature accumulation of alpha-synuclein and microglia activation along with increased production of pro-inflammatory cytokines. All these events were shown to enhance the vulnerability of dopaminergic neurons to MPTP neurotoxicity in the substantia nigra of db/db mice (Wang L. et al., 2014; Wang S. et al., 2014). Similar results were obtained in mice become insulin resistant upon a high-fat diet (HFD), which are more susceptible to PD inducing toxins, such as 6-OHDA and MPTP, characterized by a significant increase in nigrostriatal neurodegeneration and by a reduced dopaminergic signaling. This leads to a more severe motor deficits compared to matched controls (Choi et al., 2005; Morris et al., 2010, 2011a,b).
Recently, Sharma and Taliyan (2018) standardized an animal model suitable to mimic the comorbidity between insulin resistance and PD. To this aim, male Wistar rats were administrated 6-OHDA in medial forebrain bundle after 8 weeks feeding with high fat diet. The phenotype of these rats confirmed the capacity of insulin resistance to exacerbate PD pathology. In HFD-fed rats, indeed, 6-OHDA induced more pronounced neuronal damage and loss of striatal dopamine, leading, in parallel, to worst performance in behavioral tasks such as rotarod, narrow beam walk test and locomotor activity, compared to rats fed with standard diet.
The relevance of insulin resistance for PD has been further confirmed by the phenotype of transgenic mice overexpressing PED/PEA-15, a scaffold protein highly expressed in the brain and overexpressed in T2D subjects. These insulin resistant mice, indeed, show loss of dopaminergic neurons in the striatum and hypokinetic movements resembling PD motor alterations (Perruolo et al., 2016). Not least, NIRKO mice with neuron-specific IR knockout are the proof that insulin resistance is involved also in the onset of PD non-motor symptoms, since these mice develop increased dopamine turnover responsible for anxiety and depressive behaviors (Kleinridders et al., 2015).
Surprisingly, several studies found that alpha-synuclein increases inhibitory phosphorylation of IRS at serine residues, negatively regulating insulin signaling (Gao et al., 2015). Different mechanisms have been proposed to explain the deleterious effect of alpha-synuclein on insulin signaling. First, alpha-synuclein increases degradation of IRS-1, inhibiting protein phosphatase 2A through mTORC1 activation (Gao et al., 2015). In addition, alpha-synuclein induces microglial production of pro-inflammatory cytokines (Beraud et al., 2013; Blandini, 2013; Gallegos et al., 2015).
Overproduction of pro-inflammatory cytokines such as TNF-alpha in the CSF and CNS of PD patients was, indeed, evidenced in postmortem studies (Reale et al., 2009a,b). Similarly, peripheral concentrations of IL-6, TNF-alpha, IL-1beta, IL-2, IL-10, and C-reactive protein in PD patients are significantly higher compared with age-matched controls (Qin et al., 2016). Moreover, among newly diagnosed PD patients, those with higher levels of pro-inflammatory markers feature lower cognitive assessment scores (MMSE) and more rapid motor decline (Williams-Gray et al., 2009). Pro-inflammatory cytokines are probably responsible for increased activity of IRS serine kinases such as JNK, involved in the onset of neuronal insulin resistance (Peng and Andersen, 2003; Klintworth et al., 2007; Morris et al., 2008).
Interestingly, in HFD fed mice, restoring of IR signaling by inhibition of protein tyrosine phosphatase 1B or by treatment with the small molecule IR sensitizing agent, TCS 401, re-establishes insulin positive action on dopamine release and reuptake at dopamine terminals in the nucleus accumbens (Fordahl and Jones, 2017). Similarly, treatment with insulin sensitizing drugs and normalizing HFD ameliorate depressive behaviors in rodents (Yamada et al., 2011; Sharma et al., 2012). This evidence further suggests that insulin resistance could represent a common risk factor involved in both T2D and PD pathogenesis.
Both insulin resistance and PD can be defined as multifactorial disorders due to the interaction of environmental factors with a genetic susceptibility. Thus, modification of lifestyle and health behaviors, such as diet, can improve and prevent the onset of these diseases. It is well established that the MD, a nutritional model widespread in some countries of the Mediterranean sea such as southern Italy, Spain and Greece, which is based on a relatively higher intake of cereals, fruit, vegetables, seeds, olive oil (unsaturated fat) compared to a more rare use of red meat and animal fats (saturated fats), has many beneficial effects on insulin-resistance and T2D (Giugliano and Esposito, 2008; Grosso et al., 2014; Garcia et al., 2016). More recently, some authors have shown that MD seems to play also a neuroprotective role, although not all of epidemiologic studies report a positive function of MD on neurodegenerative diseases and further studies are required to validate these evidences (Alcalay et al., 2012; Okubo et al., 2012; Martinez-Lapiscina et al., 2013a,b; Cassani et al., 2017; Table 1).
In parallel with the healthy lifestyle, insulin and several drugs currently used for the treatment of insulin resistance have been suggested to have therapeutic effects in patients with PD (Figure 2 and Table 1). These substances include metformin, exenatide, thiazolidinediones, and bromocriptine.
Figure 2. Role of the antidiabetic drugs on brain function. Several antihyperglycemic agents play a neuroprotective and anti-inflammatory role by directly acting on brain or by reducing insulin resistance.
The role of insulin in PD treatment has been firstly evidenced by several studies indicating the presence of frequent amnesic defects in T2D patients (Perlmuter et al., 1984; Helkala et al., 1995; Vanhanen et al., 1999). Subsequently, these observations have been confirmed by the fact that intranasal administration of insulin in the hippocampus can improve memory deficits in humans (Benedict et al., 2004). However, the importance of insulin is not limited to the learning and memory processes, but also extends to its ability to induce anti-inflammatory and neuro-protective responses. Indeed, intranasal insulin administration protects against substantia nigra dopaminergic neuronal loss and alleviates motor deficits induced by 6-OHDA in rats (Pang et al., 2016). The positive effects mediated by insulin can be probably related to the capacity of this hormone to improve brain oxidative stress, apoptosis, autophagy and neuroinflammation and to the presence of its receptor in the CNS, in particular in the hippocampus and medial temporal cortex and amygdala, as described before (Singh et al., 1997; Gerozissis, 2003).
Metformin belongs to the biguanide family and is the most frequently used oral antidiabetic drug (Viollet et al., 2012). Met, by activating AMPK or increasing the IR expression and tyrosine kinase activity (Musi et al., 2002; Viollet et al., 2012; Rena et al., 2017), reduces hepatic gluconeogenesis and increases insulin-stimulated glucose uptake in skeletal muscle and adipocytes. In addition, Met decreases free fatty acid oxidation, improving insulin sensitivity (Musi et al., 2002; Rena et al., 2017) and insulin secretion from pancreatic beta-cells (Sreenan et al., 1996). Further studies suggest that metformin can cross BBB and activate AMPK in the CNS (Nath et al., 2009). In addition, this drug has been shown to rescue dopaminergic dysfunction and mitochondrial abnormalities in Drosophila models of PD (Ng et al., 2012) and to reduce the phospho-Ser129 alpha-synuclein, the modified form of alpha-synuclein that occurs most frequently within PD, both in vitro and in vivo (Perez-Revuelta et al., 2014). Nevertheless, studies performed in humans revealed contrasting results. Indeed, while Hsu et al. have suggested that can reduce the risk of dementia (Hsu et al., 2011) in diabetic patients, other authors indicate that metformin exposure in patients with T2D may lead to the development of neuronal diseases, including dementia and PD (Imfeld et al., 2012; Moore et al., 2013; Kuan et al., 2017).
Glucagon-like peptide-1 (GLP-1) receptor agonists, by mimicking the effects of the incretin hormone GLP-1, increase glucose-mediated insulin secretion and reduce postprandial glucagon levels, gastric emptying rate, food intake and body weight. Differentially from GLP-1 hormone, having a short half-life, GLP-1 agonists have two important properties that include the longer duration of action after subcutaneous administration respect to GLP-1 and the resistance to degradation mediated by dipeptidyl-peptidase 4 (DPP-4) enzymes (Prasad-Reddy and Isaacs, 2015). Several GLP-1 receptor agonists, including lixisenatide, exenatide and liraglutide, induce neuroprotective effects, and, in particular, exenatide (Ex-4), a synthetic version of exendin 4, has been suggested to have an important role in PD. Indeed, both “in vitro” and “in vivo” studies have demonstrated the ability of exenatide to mediate neurotrophic and neuro-protective effects. In particular, Li et al. (2009) have shown that Ex-4 treatment protects dopaminergic neurons against degeneration, preserves dopamine levels and improves motor function in the MPTP mouse model of PD. Similar results have been obtained by Bertilsson et al. (2008) who suggest that that Ex-4 significantly increases the number of neurons positive for TH and vesicular MAO transporter 2 in the substantia nigra of animals lesioned with 6-OHDA. Several mechanisms by which exenatide protects form neurodegeneration have been hypothesized. Kim et al. (2009) have showed that this drug protects dopaminergic neurons by preventing MPTP-induced microglial activation and MMP-3 expression. Other authors have demonstrated that GLP-1 receptor stimulation reduces apoptosis by promoting Bcl-2 expression and inhibiting the activation of caspase 3 and preserves mitochondrial function in dopaminergic neurons (Chen et al., 2015). Toxin-based models of PD, despite their limited translational value, have allowed to clarify mechanisms of action of GLP-1 agonists. Nevertheless, it is still unclear which of the previously described pathways are crucial for the GLP-1 agonists therapeutic effects for PD (Foltynie and Athauda, 2018). Clinical trials have also validated the positive effects of GLP-1R agonists in PD, underlining the safety and tolerability of this drug (Aviles-Olmos et al., 2013, 2014; MacConell et al., 2015; Athauda et al., 2017). Nevertheless, the difficulty to compare each GLP-1R agonists under the same conditions limits, in part, the reproducibility of these studies.
DPP-4 is an enzyme which rapidly inactivates GLP-1 and GIP incretins, limiting their hypoglycemic action (Hansen et al., 1999). Furthermore, increased serological levels of DPP-4 have been observed in diabetic patients (Kim N.H. et al., 2014) and, thus, several DPP-4 inhibitors are used in the effective treatment of T2D. Treatment with DPP-4 inhibitors improves metabolism, insulin secretion and reduces glucagon secretion. Compared to GLP1 analogs, DPP-4 inhibitors are not able to induce weight loss, but in any case they do not lead to an increase in body weight, which instead occurs with sulfonylurea or insulin treatment.
Since the discovery of neurotrophic and immune regulating functions of DPP-4 inhibitors in the CNS, increasing studies supports the idea that DPP-4 might also be involved in the development of neurological disorders with a neuroinflammatory component.
Svenningsson et al. (2016) in a nationwide case-control study, found a significantly decreased incidence of PD among individuals with a record of DPP-4 inhibitor intake. The authors hypothesize that the this positive effect can be due not only to the increase of GLP-1/GLP-1R binding, but also by reducing the degradation of some neurotrophic neuropeptides, including pituitary adenylate cyclase-activating polypeptide (PACAP), substance P, neuropeptide Y, and gastrin-releasing peptide (Matteucci and Giampietro, 2015). Furthermore, DPP-4 inhibitors may have direct immunosuppressive effects, providing interesting insights for the future therapeutic development of treatments of neurological conditions with recognizable immune-related dysfunctions (Yazbeck et al., 2009; Svenningsson et al., 2016).
Other studies have shown the antiparkinsonian effect of vildagliptin, a dipeptidyl peptidase (DPP)-4 inhibitor, in rotenone−induced PD model in rats. Indeed, in these animals, vildagliptin by blocking the RAGE/NFκB cascade, suppresses inflammatory, oxidative stress, and apoptotic mediators reducing death of dopaminergic neurons and motor impairment (Abdelsalam and Safar, 2015). Similar results have been obtained by Nassar et al. (2015) using saxagliptin, another DPP-4 inhibitor.
Thiazoledinediones, that include rosiglitazone and pioglitazone, are oral hypoglycemic agents which bind and activate the nuclear receptor PPARγ. This protein is expressed not only in many insulin target tissues, but also in the substantia nigra and in the putamen nucleus (Swanson and Emborg, 2014). TZDs improve insulin-resistance in several ways, including the reduction of circulating fatty acids, the activation of the Glut4-mediated glucose transport and the decrease of the levels of inflammatory cytokines (Davidson et al., 2018).
Moreover, pioglitazone mediates its neuro protective effect, by binding a protein residing in the mitochondrial outer membrane, called MitoNEEt and regulating the activity of complex I in neuronal cells (Rabchevsky et al., 2017). In addition, this drug blocks the nitric oxide-mediated toxicity in MPTP-treated mice (Dehmer et al., 2004). As for the other anti-diabetic medications, studies performed in humans about the efficacy of TZDs in PD are still disappointing (NINDS Exploratory Trials in Parkinson Disease (NET-PD) FS-ZONE Investigators, 2015). A possible explanation for difficulty to obtain significant data in neurological disorder such as parkinsonism can be probably related to the poor capacity of TZDs to cross the BBB. Indeed, pioglitazone and rosiglitazone are substrates of the P-glycoprotein. This protein increases during the inflammatory state that occurs in PD and acts as a stereoselective barrier preventing the entry of TZDs into the brain (Chang et al., 2015).
A class of drugs capable of activating D2R dopaminergic receptors is represented by the dopaminergic agonists. In particular, an ergoline derivative, bromocriptine, indicated for the treatment of patients with parkinsonism who no longer respond to treatment with levodopa, improves glycemic homeostasis and is used in the treatment of T2D since 2009. The mechanism of action of bromocriptine is still not very clear. This drug, by activating D2 and blocking D1 receptors, is able to reduce blood glucose and serum triglycerides levels and to decrease body weight (Kalra et al., 2011; Lopez Vicchi et al., 2016). Furthermore, bromocriptine directly activates the alpha 2-adrenergic receptors, inhibiting glucose-stimulated insulin secretion in pancreatic beta cells (Kalra et al., 2011; Lopez Vicchi et al., 2016).
Studies performed on animal models, in particular on ob/ob mice and Syrian hamsters suggest that bromocriptine treatment improves obesity and associated metabolic dysfunctions and inhibits the seasonally occurring obesity, hyperinsulinemia, insulin resistance and impaired glucose tolerance (Cincotta and Meier, 1995; Liang et al., 1998; Luo et al., 1998). In addition, several clinical trials have demonstrated the beneficial effect of bromocriptine on glycemia and weight in obese non-diabetic and diabetic subjects (Meier et al., 1992; Pijl et al., 2000; Aminorroaya et al., 2004; Gaziano et al., 2010). Thus, despite bromocriptine has been used since the 1960s for treatment of PD, acromegaly and prolactinomas, only recently its relevance has been demonstrated in T2D, encouraging its future application.
From these data, it is clear that the correction of metabolic disorders is of fundamental importance in the care of PD. However, actually, there is no resolutive antihyperglycemic treatment able to improve PD, slow down its progression and alleviate its symptoms. Thus, the future challenge of the PD research aims to identify new molecules that are more effective and tolerable both in PD and in insulin-resistance than the traditional ones.
Several clinical and experimental studies indicate a higher prevalence of PD in patients diagnosed with diabetes. Indeed, it is now clear that the loss of insulin signaling may cause neuronal mitochondrial dysfunction and oxidative stress followed by loss of dopaminergic neurons and impaired memory functioning. These results have been corroborated by studies performed in animal models and by the positive action that some antidiabetic drugs induce with significant benefits in patients diagnosed with PD. However, although the scientific research has reached several promising results, further and more detailed investigations are necessary to validate these studies in order to discover new therapeutic avenues.
FF and GP prepared the first draft of the manuscript. IC, SC, FP, and CM were involved in the literature search. FB and PF critically revised the manuscript. PF and FO supervised the work and wrote the final version of the manuscript.
This work was supported by grants from the University of Naples “Federico II” (Progetto di Ricerca di Ateneo) to FO and Associazione Italiana per la Ricerca sul Cancro - AIRC (IG19001) to PF.
The authors declare that the research was conducted in the absence of any commercial or financial relationships that could be construed as a potential conflict of interest.
6-OHDA, 6-hydroxydopmin; AMPK, Adenosine Monophosphate-Activated Protein Kinase; BAX, (B Cell Lymphoma)-Associated X; BCL, B Cell Lymphoma; COX, Cyclooxygenase; DPP-4, dipeptidyl-peptidase IV; ERK, Extracellular Receptor Kinase; Ex-4, Exenatide; GLP, Glucagon-like peptide; GSK, Glycogen Synthase Kinase; HFD, high fat diet-treated; IL, Interleukin; iNOS, Inducible nitric oxide synthase; IR, Insulin receptor; IRS, Insulin receptor substrate; JNK, Jun N-terminal Kinase; MD, Mediterranean-style diet; Met, Metformin; MMP-3, matrix metalloprotease 3; MPP+, 1-methyl-4-phenyl pyridinium; MPTP, 1-methyl-4-phenyl-1,2,3,6-tetrahydropyridine; NIRKO, neuron-specific insulin receptor knockout; PD, Parkinson’s disease; PED/PEA, Phosphoprotein enriched in diabetes/phosphoprotein enriched in astrocytes; PGC-1 α, peroxisome proliferator-activated receptor gamma coactivator 1-alpha; PI3K, Phosphoinositide 3-kinases; PPAR γ, Peroxisome proliferator-activated receptor gamma; RA, Retinoic acid; ROS, Reactive oxygen species; T2DM, Type 2 Diabetes Mellitus; TORC, cytochrome c-type protein; TNF, Tumor Necrosis Factor; TZD, Thiazoledinediones.
Aarsland, D., Creese, B., Politis, M., Chaudhuri, K. R., Ffytche, D. H., Weintraub, D., et al. (2017). Cognitive decline in Parkinson disease. Nat. Rev. Neurol. 13, 217–231. doi: 10.1038/nrneurol.2017.27
Abdelsalam, R. M., and Safar, M. M. (2015). Neuroprotective effects of vildagliptin in rat rotenone Parkinson’s disease model: role of RAGE-NFkappaB and Nrf2-antioxidant signaling pathways. J. Neurochem. 133, 700–707. doi: 10.1111/jnc.13087
Aghanoori, M. R., Smith, D. R., Roy Chowdhury, S., Sabbir, M. G., Calcutt, N. A., and Fernyhough, P. (2017). Insulin prevents aberrant mitochondrial phenotype in sensory neurons of type 1 diabetic rats. Exp. Neurol. 297, 148–157. doi: 10.1016/j.expneurol.2017.08.005
Aime, P., Hegoburu, C., Jaillard, T., Degletagne, C., Garcia, S., Messaoudi, B., et al. (2012). A physiological increase of insulin in the olfactory bulb decreases detection of a learned aversive odor and abolishes food odor-induced sniffing behavior in rats. PLoS One 7:e51227. doi: 10.1371/journal.pone.0051227
Alcalay, R. N., Gu, Y., Mejia-Santana, H., Cote, L., Marder, K. S., and Scarmeas, N. (2012). The association between mediterranean diet adherence and Parkinson’s disease. Mov. Disord. 27, 771–774. doi: 10.1002/mds.24918
Aminorroaya, A., Janghorbani, M., Ramezani, M., Haghighi, S., and Amini, M. (2004). Does bromocriptine improve glycemic control of obese type-2 diabetics? Horm. Res. 62, 55–59. doi: 10.1159/000078932
Ashraghi, M. R., Pagano, G., Polychronis, S., Niccolini, F., and Politis, M. (2016). Parkinson’s disease, diabetes and cognitive impairment. Recent Pat. Endocr. Metab. Immune Drug Discov. 10, 11–21. doi: 10.2174/1872214810999160628105549
Athauda, D., and Foltynie, T. (2016). Insulin resistance and Parkinson’s disease: a new target for disease modification? Prog. Neurobiol. 145-146, 98–120. doi: 10.1016/j.pneurobio.2016.10.001
Athauda, D., Maclagan, K., Skene, S. S., Bajwa-Joseph, M., Letchford, D., Chowdhury, K., et al. (2017). Exenatide once weekly versus placebo in Parkinson’s disease: a randomised, double-blind, placebo-controlled trial. Lancet 390, 1664–1675. doi: 10.1016/S0140-6736(17)31585-31584
Aviles-Olmos, I., Dickson, J., Kefalopoulou, Z., Djamshidian, A., Ell, P., Soderlund, T., et al. (2013). Exenatide and the treatment of patients with Parkinson’s disease. J. Clin. Invest. 123, 2730–2736. doi: 10.1172/JCI68295
Aviles-Olmos, I., Dickson, J., Kefalopoulou, Z., Djamshidian, A., Kahan, J., Ell, P., et al. (2014). Motor and cognitive advantages persist 12 months after exenatide exposure in Parkinson’s disease. J. Parkinsons Dis. 4, 337–344. doi: 10.3233/JPD-140364
Banks, W. A., Jaspan, J. B., and Kastin, A. J. (1997). Effect of diabetes mellitus on the permeability of the blood-brain barrier to insulin. Peptides 18, 1577–1584. doi: 10.1016/s0196-9781(97)00238-6
Barhwal, K., Das, S. K., Kumar, A., Hota, S. K., and Srivastava, R. B. (2015). Insulin receptor A and Sirtuin 1 synergistically improve learning and spatial memory following chronic salidroside treatment during hypoxia. J. Neurochem. 135, 332–346. doi: 10.1111/jnc.13225
Baskin, D. G., Porte, D. Jr., Guest, K., and Dorsa, D. M. (1983). Regional concentrations of insulin in the rat brain. Endocrinology 112, 898–903. doi: 10.1210/endo-112-3-898
Benedict, C., Hallschmid, M., Hatke, A., Schultes, B., Fehm, H. L., Born, J., et al. (2004). Intranasal insulin improves memory in humans. Psychoneuroendocrinology 29, 1326–1334. doi: 10.1016/j.psyneuen.2004.04.003
Beraud, D., Hathaway, H. A., Trecki, J., Chasovskikh, S., Johnson, D. A., Johnson, J. A., et al. (2013). Microglial activation and antioxidant responses induced by the Parkinson’s disease protein alpha-synuclein. J. Neuroimmune Pharmacol. 8, 94–117. doi: 10.1007/s11481-012-9401-9400
Bertilsson, G., Patrone, C., Zachrisson, O., Andersson, A., Dannaeus, K., Heidrich, J., et al. (2008). Peptide hormone exendin-4 stimulates subventricular zone neurogenesis in the adult rodent brain and induces recovery in an animal model of Parkinson’s disease. J. Neurosci. Res. 86, 326–338. doi: 10.1002/jnr.21483
Biessels, G. J., and Reagan, L. P. (2015). Hippocampal insulin resistance and cognitive dysfunction. Nat. Rev. Neurosci. 16, 660–671. doi: 10.1038/nrn4019
Biosa, A., Outeiro, T. F., Bubacco, L., and Bisaglia, M. (2018). Diabetes mellitus as a risk factor for Parkinson’s disease: a molecular point of view. Mol. Neurobiol. 55, 8754–8763. doi: 10.1007/s12035-018-1025-1029
Blandini, F. (2013). Neural and immune mechanisms in the pathogenesis of Parkinson’s disease. J. Neuroimmune Pharmacol. 8, 189–201. doi: 10.1007/s11481-013-9435-y
Blazquez, E., Velazquez, E., Hurtado-Carneiro, V., and Ruiz-Albusac, J. M. (2014). Insulin in the brain: its pathophysiological implications for states related with central insulin resistance, type 2 diabetes and Alzheimer’s disease. Front. Endocrinol. 5:161. doi: 10.3389/fendo.2014.00161
Bosco, D., Plastino, M., Cristiano, D., Colica, C., Ermio, C., De Bartolo, M., et al. (2012). Dementia is associated with insulin resistance in patients with Parkinson’s disease. J. Neurol. Sci. 315, 39–43. doi: 10.1016/j.jns.2011.12.008
Bouchard, T. P., Malykhin, N., Martin, W. R., Hanstock, C. C., Emery, D. J., Fisher, N. J., et al. (2008). Age and dementia-associated atrophy predominates in the hippocampal head and amygdala in Parkinson’s disease. Neurobiol. Aging 29, 1027–1039. doi: 10.1016/j.neurobiolaging.2007.02.002
Carvelli, L., Moron, J. A., Kahlig, K. M., Ferrer, J. V., Sen, N., Lechleiter, J. D., et al. (2002). PI 3-kinase regulation of dopamine uptake. J. Neurochem. 81, 859–869. doi: 10.1046/j.1471-4159.2002.00892.x
Cassani, E., Barichella, M., Ferri, V., Pinelli, G., Iorio, L., Bolliri, C., et al. (2017). Dietary habits in Parkinson’s disease: adherence to mediterranean diet. Parkinsonism Relat. Disord. 42, 40–46. doi: 10.1016/j.parkreldis.2017.06.007
Cereda, E., Barichella, M., Cassani, E., Caccialanza, R., and Pezzoli, G. (2012). Clinical features of Parkinson disease when onset of diabetes came first: a case-control study. Neurology 78, 1507–1511. doi: 10.1212/WNL.0b013e3182553cc9
Chang, K. L., Pee, H. N., Yang, S., and Ho, P. C. (2015). Influence of drug transporters and stereoselectivity on the brain penetration of pioglitazone as a potential medicine against Alzheimer’s disease. Sci. Rep. 5:9000. doi: 10.1038/srep09000
Chen, Y., Zhang, Y., Li, L., and Holscher, C. (2015). Neuroprotective effects of geniposide in the MPTP mouse model of Parkinson’s disease. Eur. J. Pharmacol. 768, 21–27. doi: 10.1016/j.ejphar.2015.09.029
Cheng, Z., Tseng, Y., and White, M. F. (2010). Insulin signaling meets mitochondria in metabolism. Trends Endocrinol. Metab. 21, 589–598. doi: 10.1016/j.tem.2010.06.005
Choi, J. Y., Jang, E. H., Park, C. S., and Kang, J. H. (2005). Enhanced susceptibility to 1-methyl-4-phenyl-1,2,3,6-tetrahydropyridine neurotoxicity in high-fat diet-induced obesity. Free Radic. Biol. Med. 38, 806–816. doi: 10.1016/j.freeradbiomed.2004.12.008
Christine, C. W., Starr, P. A., Larson, P. S., Eberling, J. L., Jagust, W. J., Hawkins, R. A., et al. (2009). Safety and tolerability of putaminal AADC gene therapy for Parkinson disease. Neurology 73, 1662–1669. doi: 10.1212/WNL.0b013e3181c29356
Chu, P. C., Lin, M. T., Shian, L. R., and Leu, S. Y. (1986). Alterations in physiologic functions and in brain monoamine content in streptozocin-diabetic rats. Diabetes 35, 481–485. doi: 10.2337/diabetes.35.4.481
Cincotta, A. H., and Meier, A. H. (1995). Bromocriptine inhibits in vivo free fatty acid oxidation and hepatic glucose output in seasonally obese hamsters (Mesocricetus auratus). Metabolism 44, 1349–1355. doi: 10.1016/0026-0495(95)90041-1
Costa, C., Sgobio, C., Siliquini, S., Tozzi, A., Tantucci, M., Ghiglieri, V., et al. (2012). Mechanisms underlying the impairment of hippocampal long-term potentiation and memory in experimental Parkinson’s disease. Brain 135(Pt 6), 1884–1899. doi: 10.1093/brain/aws101
Craft, S., Peskind, E., Schwartz, M. W., Schellenberg, G. D., Raskind, M., Porte, D. Jr., et al. (1998). Cerebrospinal fluid and plasma insulin levels in Alzheimer’s disease: relationship to severity of dementia and apolipoprotein E genotype. Neurology 50, 164–168. doi: 10.1212/wnl.50.1.164
Datusalia, A. K., and Sharma, S. S. (2014). Amelioration of diabetes-induced cognitive deficits by GSK-3beta inhibition is attributed to modulation of neurotransmitters and neuroinflammation. Mol. Neurobiol. 50, 390–405. doi: 10.1007/s12035-014-8632-x
Davidson, M. A., Mattison, D. R., Azoulay, L., and Krewski, D. (2018). Thiazolidinedione drugs in the treatment of type 2 diabetes mellitus: past, present and future. Crit. Rev. Toxicol. 48, 52–108. doi: 10.1080/10408444.2017.1351420
Davis, A. A., and Racette, B. (2016). Parkinson disease and cognitive impairment: five new things. Neurol. Clin. Pract. 6, 452–458. doi: 10.1212/CPJ.0000000000000285
De Pablo-Fernandez, E., Goldacre, R., Pakpoor, J., Noyce, A. J., and Warner, T. T. (2018). Association between diabetes and subsequent Parkinson disease: a record-linkage cohort study. Neurology 91, e139–e142. doi: 10.1212/WNL.0000000000005771
Dehmer, T., Heneka, M. T., Sastre, M., Dichgans, J., and Schulz, J. B. (2004). Protection by pioglitazone in the MPTP model of Parkinson’s disease correlates with I kappa B alpha induction and block of NF kappa B and iNOS activation. J. Neurochem. 88, 494–501. doi: 10.1046/j.1471-4159.2003.02210.x
Diaz, B., Pimentel, B., de Pablo, F., and de La Rosa, E. J. (1999). Apoptotic cell death of proliferating neuroepithelial cells in the embryonic retina is prevented by insulin. Eur. J. Neurosci. 11, 1624–1632. doi: 10.1046/j.1460-9568.1999.00577.x
Dickson, D. W., Fujishiro, H., Orr, C., DelleDonne, A., Josephs, K. A., Frigerio, R., et al. (2009). Neuropathology of non-motor features of Parkinson disease. Parkinsonism Relat. Disord. 15(Suppl. 3), S1–S5. doi: 10.1016/S1353-8020(09)70769-70762
Dodd, G. T., Decherf, S., Loh, K., Simonds, S. E., Wiede, F., Balland, E., et al. (2015). Leptin and insulin act on POMC neurons to promote the browning of white fat. Cell 160, 88–104. doi: 10.1016/j.cell.2014.12.022
Dohgu, S., Takata, F., Matsumoto, J., Kimura, I., Yamauchi, A., and Kataoka, Y. (2019). Monomeric alpha-synuclein induces blood-brain barrier dysfunction through activated brain pericytes releasing inflammatory mediators in vitro. Microvasc. Res. 124, 61–66. doi: 10.1016/j.mvr.2019.03.005
Dorn, A., Rinne, A., Hahn, H. J., Bernstein, H. G., and Ziegler, M. (1982). C-peptide immunoreactive neurons in human brain. Acta Histochem. 70, 326–330. doi: 10.1016/S0065-1281(82)80080-80089
Duarte, A. I., Moreira, P. I., and Oliveira, C. R. (2012). Insulin in central nervous system: more than just a peripheral hormone. J. Aging Res. 2012:384017. doi: 10.1155/2012/384017
Duffy, K. R., and Pardridge, W. M. (1987). Blood-brain barrier transcytosis of insulin in developing rabbits. Brain Res. 420, 32–38. doi: 10.1016/0006-8993(87)90236-90238
Fehm, H. L., Kern, W., and Peters, A. (2006). The selfish brain: competition for energy resources. Prog. Brain Res. 153, 129–140. doi: 10.1016/S0079-6123(06)53007-53009
Figlewicz, D. P., Brot, M. D., McCall, A. L., and Szot, P. (1996). Diabetes causes differential changes in CNS noradrenergic and dopaminergic neurons in the rat: a molecular study. Brain Res. 736, 54–60. doi: 10.1016/s0006-8993(96)00727-5
Figlewicz, D. P., Evans, S. B., Murphy, J., Hoen, M., and Baskin, D. G. (2003). Expression of receptors for insulin and leptin in the ventral tegmental area/substantia nigra (VTA/SN) of the rat. Brain Res. 964, 107–115. doi: 10.1016/s0006-8993(02)04087-8
Fiory, F., Mirra, P., Nigro, C., Pignalosa, F. C., Zatterale, F., Ulianich, L., et al. (2018). Role of the HIF-1alpha/Nur77 axis in the regulation of the tyrosine hydroxylase expression by insulin in PC12 cells. J. Cell Physiol. 234, 11861–11870. doi: 10.1002/jcp.27898
Foltynie, T., and Athauda, D. (2018). Glucagon-like peptides (GLP-1) perspectives in synucleinopathies treatment. Mov. Disord. Clin. Pract. 5, 255–258. doi: 10.1002/mdc3.12611
Fordahl, S. C., and Jones, S. R. (2017). High-Fat-Diet-Induced deficits in dopamine terminal function are reversed by restoring insulin signaling. ACS Chem. Neurosci. 8, 290–299. doi: 10.1021/acschemneuro.6b00308
Franco-Robles, E., Campos-Cervantes, A., Murillo-Ortiz, B. O., Segovia, J., Lopez-Briones, S., Vergara, P., et al. (2014). Effects of curcumin on brain-derived neurotrophic factor levels and oxidative damage in obesity and diabetes. Appl. Physiol. Nutr. Metab. 39, 211–218. doi: 10.1139/apnm-2013-2133
Frolich, L., Blum-Degen, D., Bernstein, H. G., Engelsberger, S., Humrich, J., Laufer, S., et al. (1998). Brain insulin and insulin receptors in aging and sporadic Alzheimer’s disease. J. Neural Transm. 105, 423–438. doi: 10.1007/s007020050068
Gallegos, S., Pacheco, C., Peters, C., Opazo, C. M., and Aguayo, L. G. (2015). Features of alpha-synuclein that could explain the progression and irreversibility of Parkinson’s disease. Front. Neurosci. 9:59. doi: 10.3389/fnins.2015.00059
Gao, S., Duan, C., Gao, G., Wang, X., and Yang, H. (2015). Alpha-synuclein overexpression negatively regulates insulin receptor substrate 1 by activating mTORC1/S6K1 signaling. Int. J. Biochem. Cell Biol. 64, 25–33. doi: 10.1016/j.biocel.2015.03.006
Garcia, M., Bihuniak, J. D., Shook, J., Kenny, A., Kerstetter, J., and Huedo-Medina, T. B. (2016). The effect of the traditional mediterranean-style diet on metabolic risk factors: a meta-analysis. Nutrients 8:168. doi: 10.3390/nu8030168
Gaziano, J. M., Cincotta, A. H., O’Connor, C. M., Ezrokhi, M., Rutty, D., Ma, Z. J., et al. (2010). Randomized clinical trial of quick-release bromocriptine among patients with type 2 diabetes on overall safety and cardiovascular outcomes. Diabetes Care 33, 1503–1508. doi: 10.2337/dc09-2009
Geng, X., Lou, H., Wang, J., Li, L., Swanson, A. L., Sun, M., et al. (2011). alpha-Synuclein binds the K(ATP) channel at insulin-secretory granules and inhibits insulin secretion. Am. J. Physiol. Endocrinol. Metab. 300, E276–E286. doi: 10.1152/ajpendo.00262.2010
Gerozissis, K. (2003). Brain insulin: regulation, mechanisms of action and functions. Cell Mol. Neurobiol. 23, 1–25.
Giugliano, D., and Esposito, K. (2008). Mediterranean diet and metabolic diseases. Curr. Opin. Lipidol. 19, 63–68. doi: 10.1097/MOL.0b013e3282f2fa4d
Glanville, N. T., and Anderson, G. H. (1986). Hypothalamic catecholamine metabolism in diabetic rats: the effect of insulin deficiency and meal ingestion. J. Neurochem. 46, 753–759. doi: 10.1111/j.1471-4159.1986.tb13036.x
Goldin, M., and Segal, M. (2003). Protein kinase C and ERK involvement in dendritic spine plasticity in cultured rodent hippocampal neurons. Eur. J. Neurosci. 17, 2529–2539. doi: 10.1046/j.1460-9568.2003.02694.x
Golpich, M., Amini, E., Hemmati, F., Ibrahim, N. M., Rahmani, B., Mohamed, Z., et al. (2015). Glycogen synthase kinase-3 beta (GSK-3beta) signaling: implications for Parkinson’s disease. Pharmacol. Res. 97, 16–26. doi: 10.1016/j.phrs.2015.03.010
Gray, M. T., and Woulfe, J. M. (2015). Striatal blood-brain barrier permeability in Parkinson’s disease. J. Cereb. Blood Flow Metab. 35, 747–750. doi: 10.1038/jcbfm.2015.32
Gray, S. M., Meijer, R. I., and Barrett, E. J. (2014). Insulin regulates brain function, but how does it get there? Diabetes 63, 3992–3997. doi: 10.2337/db14-0340
Grosso, G., Mistretta, A., Marventano, S., Purrello, A., Vitaglione, P., Calabrese, G., et al. (2014). Beneficial effects of the mediterranean diet on metabolic syndrome. Curr. Pharm. Des. 20, 5039–5044. doi: 10.2174/1381612819666131206112144
Grote, C. W., and Wright, D. E. (2016). A role for insulin in diabetic neuropathy. Front. Neurosci. 10:581. doi: 10.3389/fnins.2016.00581
Haeusler, R. A., McGraw, T. E., and Accili, D. (2018). Biochemical and cellular properties of insulin receptor signalling. Nat. Rev. Mol. Cell Biol. 19, 31–44. doi: 10.1038/nrm.2017.89
Hamed, S. A. (2017). Brain injury with diabetes mellitus: evidence, mechanisms and treatment implications. Expert Rev. Clin. Pharmacol. 10, 409–428. doi: 10.1080/17512433.2017.1293521
Hansen, L., Deacon, C. F., Orskov, C., and Holst, J. J. (1999). Glucagon-like peptide-1-(7-36)amide is transformed to glucagon-like peptide-1-(9-36)amide by dipeptidyl peptidase IV in the capillaries supplying the L cells of the porcine intestine. Endocrinology 140, 5356–5363. doi: 10.1210/endo.140.11.7143
Havrankova, J., Schmechel, D., Roth, J., and Brownstein, M. (1978). Identification of insulin in rat brain. Proc. Natl. Acad. Sci. U.S.A. 75, 5737–5741. doi: 10.1073/pnas.75.11.5737
Helkala, E. L., Niskanen, L., Viinamaki, H., Partanen, J., and Uusitupa, M. (1995). Short-term and long-term memory in elderly patients with NIDDM. Diabetes Care 18, 681–685. doi: 10.2337/diacare.18.5.681
Hely, M. A., Reid, W. G., Adena, M. A., Halliday, G. M., and Morris, J. G. (2008). The Sydney multicenter study of Parkinson’s disease: the inevitability of dementia at 20 years. Mov. Disord. 23, 837–844. doi: 10.1002/mds.21956
Heni, M., Kullmann, S., Preissl, H., Fritsche, A., and Haring, H. U. (2015). Impaired insulin action in the human brain: causes and metabolic consequences. Nat. Rev. Endocrinol. 11, 701–711. doi: 10.1038/nrendo.2015.173
Heras-Sandoval, D., Perez-Rojas, J. M., Hernandez-Damian, J., and Pedraza-Chaverri, J. (2014). The role of PI3K/AKT/mTOR pathway in the modulation of autophagy and the clearance of protein aggregates in neurodegeneration. Cell Signal. 26, 2694–2701. doi: 10.1016/j.cellsig.2014.08.019
Hernandez, D. G., Reed, X., and Singleton, A. B. (2016). Genetics in Parkinson disease: mendelian versus non-Mendelian inheritance. J. Neurochem. 139(Suppl. 1), 59–74. doi: 10.1111/jnc.13593
Heumann, R., Moratalla, R., Herrero, M. T., Chakrabarty, K., Drucker-Colin, R., Garcia-Montes, J. R., et al. (2014). Dyskinesia in Parkinson’s disease: mechanisms and current non-pharmacological interventions. J. Neurochem. 130, 472–489. doi: 10.1111/jnc.12751
Hsu, C. C., Wahlqvist, M. L., Lee, M. S., and Tsai, H. N. (2011). Incidence of dementia is increased in type 2 diabetes and reduced by the use of sulfonylureas and metformin. J. Alzheimers Dis. 24, 485–493. doi: 10.3233/JAD-2011-101524
Hu, G., Jousilahti, P., Bidel, S., Antikainen, R., and Tuomilehto, J. (2007). Type 2 diabetes and the risk of Parkinson’s disease. Diabetes Care 30, 842–847. doi: 10.2337/dc06-2011
Huang, T. J., Price, S. A., Chilton, L., Calcutt, N. A., Tomlinson, D. R., Verkhratsky, A., et al. (2003). Insulin prevents depolarization of the mitochondrial inner membrane in sensory neurons of type 1 diabetic rats in the presence of sustained hyperglycemia. Diabetes 52, 2129–2136. doi: 10.2337/diabetes.52.8.2129
Huang, T. J., Verkhratsky, A., and Fernyhough, P. (2005). Insulin enhances mitochondrial inner membrane potential and increases ATP levels through phosphoinositide 3-kinase in adult sensory neurons. Mol. Cell Neurosci. 28, 42–54. doi: 10.1016/j.mcn.2004.08.009
Imfeld, P., Bodmer, M., Jick, S. S., and Meier, C. R. (2012). Metformin, other antidiabetic drugs, and risk of Alzheimer’s disease: a population-based case-control study. J. Am. Geriatr. Soc. 60, 916–921. doi: 10.1111/j.1532-5415.2012.03916.x
Irwin, D. J., Lee, V. M., and Trojanowski, J. Q. (2013). Parkinson’s disease dementia: convergence of alpha-synuclein, tau and amyloid-beta pathologies. Nat. Rev. Neurosci. 14, 626–636. doi: 10.1038/nrn3549
Jimenez-Jimenez, F. J., Molina, J. A., Vargas, C., Gomez, P., De Bustos, F., Zurdo, M., et al. (2000). Normal cerebrospinal fluid levels of insulin in patients with Parkinson’s disease. J. Neural Transm. 107, 445–449. doi: 10.1007/s007020070086
Kaiyala, K. J., Prigeon, R. L., Kahn, S. E., Woods, S. C., and Schwartz, M. W. (2000). Obesity induced by a high-fat diet is associated with reduced brain insulin transport in dogs. Diabetes 49, 1525–1533. doi: 10.2337/diabetes.49.9.1525
Kalra, S., Kalra, B., Agrawal, N., and Kumar, S. (2011). Dopamine: the forgotten felon in type 2 diabetes. Recent Pat. Endocr. Metab. Immune Drug Discov. 5, 61–65. doi: 10.2174/187221411794351842
Kao, S. Y. (2009). Rescue of alpha-synuclein cytotoxicity by insulin-like growth factors. Biochem. Biophys. Res. Commun. 385, 434–438. doi: 10.1016/j.bbrc.2009.05.089
Kern, W., Peters, A., Fruehwald-Schultes, B., Deininger, E., Born, J., and Fehm, H. L. (2001). Improving influence of insulin on cognitive functions in humans. Neuroendocrinology 74, 270–280. doi: 10.1159/000054694
Ketterer, C., Heni, M., Thamer, C., Herzberg-Schafer, S. A., Haring, H. U., and Fritsche, A. (2011). Acute, short-term hyperinsulinemia increases olfactory threshold in healthy subjects. Int. J. Obes. 35, 1135–1138. doi: 10.1038/ijo.2010.251
Khang, R., Park, C., and Shin, J. H. (2015). Dysregulation of parkin in the substantia nigra of db/db and high-fat diet mice. Neuroscience 294, 182–192. doi: 10.1016/j.neuroscience.2015.03.017
Khasnavis, S., Jana, A., Roy, A., Mazumder, M., Bhushan, B., Wood, T., et al. (2012). Suppression of nuclear factor-kappaB activation and inflammation in microglia by physically modified saline. J. Biol. Chem. 287, 29529–29542. doi: 10.1074/jbc.M111.338012
Kim, J.S., Oh, Y. S., Lee, K. S., Song, I. U., Park, I. S., Yang, D. W., et al. (2014). Carotid artery thickening and neurocirculatory abnormalities in de novo Parkinson disease. J. Neural Transm. 121, 1259–1268. doi: 10.1007/s00702-014-1203-1205
Kim, N.H., Yu, T., and Lee, D. H. (2014). The nonglycemic actions of dipeptidyl peptidase-4 inhibitors. Biomed. Res. Int. 2014:368703. doi: 10.1155/2014/368703
Kim, S., Moon, M., and Park, S. (2009). Exendin-4 protects dopaminergic neurons by inhibition of microglial activation and matrix metalloproteinase-3 expression in an animal model of Parkinson’s disease. J. Endocrinol. 202, 431–439. doi: 10.1677/JOE-09-0132
Kleinridders, A., Cai, W., Cappellucci, L., Ghazarian, A., Collins, W. R., Vienberg, S. G., et al. (2015). Insulin resistance in brain alters dopamine turnover and causes behavioral disorders. Proc. Natl. Acad. Sci. U. S. A. 112, 3463–3468. doi: 10.1073/pnas.1500877112
Kleinridders, A., Ferris, H. A., Cai, W., and Kahn, C. R. (2014). Insulin action in brain regulates systemic metabolism and brain function. Diabetes 63, 2232–2243. doi: 10.2337/db14-0568
Klintworth, H., Newhouse, K., Li, T., Choi, W. S., Faigle, R., and Xia, Z. (2007). Activation of c-Jun N-terminal protein kinase is a common mechanism underlying paraquat- and rotenone-induced dopaminergic cell apoptosis. Toxicol. Sci. 97, 149–162. doi: 10.1093/toxsci/kfm029
Konner, A. C., Hess, S., Tovar, S., Mesaros, A., Sanchez-Lasheras, C., Evers, N., et al. (2011). Role for insulin signaling in catecholaminergic neurons in control of energy homeostasis. Cell Metab. 13, 720–728. doi: 10.1016/j.cmet.2011.03.021
Kono, T., and Takada, M. (1994). Dopamine depletion in nigrostriatal neurons in the genetically diabetic rat. Brain Res. 634, 155–158. doi: 10.1016/0006-8993(94)90269-0
Kooistra, M., Geerlings, M. I., Mali, W. P., Vincken, K. L., van der Graaf, Y., Biessels, G. J., et al. (2013). Diabetes mellitus and progression of vascular brain lesions and brain atrophy in patients with symptomatic atherosclerotic disease. The SMART-MR study. J. Neurol. Sci. 332, 69–74. doi: 10.1016/j.jns.2013.06.019
Kuan, Y. C., Huang, K. W., Lin, C. L., Hu, C. J., and Kao, C. H. (2017). Effects of metformin exposure on neurodegenerative diseases in elderly patients with type 2 diabetes mellitus. Prog. Neuropsychopharmacol. Biol. Psychiatry 79(Pt B), 77–83. doi: 10.1016/j.pnpbp.2017.06.002
Kushner, J. A. (2013). The role of aging upon beta cell turnover. J. Clin. Invest. 123, 990–995. doi: 10.1172/JCI64095
Kuwabara, T., Kagalwala, M. N., Onuma, Y., Ito, Y., Warashina, M., Terashima, K., et al. (2011). Insulin biosynthesis in neuronal progenitors derived from adult hippocampus and the olfactory bulb. EMBO Mol. Med. 3, 742–754. doi: 10.1002/emmm.201100177
Li, Y., Perry, T., Kindy, M. S., Harvey, B. K., Tweedie, D., Holloway, H. W., et al. (2009). GLP-1 receptor stimulation preserves primary cortical and dopaminergic neurons in cellular and rodent models of stroke and Parkinsonism. Proc. Natl. Acad. Sci. U.S.A. 106, 1285–1290. doi: 10.1073/pnas.0806720106
Liang, Y., Jetton, T. L., Lubkin, M., Meier, A. H., and Cincotta, A. H. (1998). Bromocriptine/SKF38393 ameliorates islet dysfunction in the diabetic (db/db) mouse. Cell Mol. Life Sci. 54, 703–711. doi: 10.1007/s000180050197
Liu, Z., Chen, H. Q., Huang, Y., Qiu, Y. H., and Peng, Y. P. (2016). Transforming growth factor-beta1 acts via TbetaR-I on microglia to protect against MPP(+)-induced dopaminergic neuronal loss. Brain Behav. Immun. 51, 131–143. doi: 10.1016/j.bbi.2015.08.006
Lopez Vicchi, F., Luque, G. M., Brie, B., Nogueira, J. P., Garcia Tornadu, I., and Becu-Villalobos, D. (2016). Dopaminergic drugs in type 2 diabetes and glucose homeostasis. Pharmacol. Res. 109, 74–80. doi: 10.1016/j.phrs.2015.12.029
Luo, S., Meier, A. H., and Cincotta, A. H. (1998). Bromocriptine reduces obesity, glucose intolerance and extracellular monoamine metabolite levels in the ventromedial hypothalamus of Syrian hamsters. Neuroendocrinology 68, 1–10. doi: 10.1159/000054344
MacConell, L., Gurney, K., Malloy, J., Zhou, M., and Kolterman, O. (2015). Safety and tolerability of exenatide once weekly in patients with type 2 diabetes: an integrated analysis of 4,328 patients. Diabetes Metab. Syndr. Obes. 8, 241–253. doi: 10.2147/DMSO.S77290
Malagelada, C., Jin, Z. H., and Greene, L. A. (2008). RTP801 is induced in Parkinson’s disease and mediates neuron death by inhibiting Akt phosphorylation/activation. J. Neurosci. 28, 14363–14371. doi: 10.1523/JNEUROSCI.3928-08.2008
Marques, A., Dutheil, F., Durand, E., Rieu, I., Mulliez, A., Fantini, M. L., et al. (2018). Glucose dysregulation in Parkinson’s disease: too much glucose or not enough insulin? Parkinsonism Relat. Disord. 55, 122–127. doi: 10.1016/j.parkreldis.2018.05.026
Martinez-Lapiscina, E. H., Clavero, P., Toledo, E., Estruch, R., Salas-Salvado, J., San Julian, B., et al. (2013a). Mediterranean diet improves cognition: the PREDIMED-NAVARRA randomised trial. J. Neurol. Neurosurg. Psychiatry 84, 1318–1325. doi: 10.1136/jnnp-2012-304792
Martinez-Lapiscina, E. H., Clavero, P., Toledo, E., San Julian, B., Sanchez-Tainta, A., Corella, D., et al. (2013b). Virgin olive oil supplementation and long-term cognition: the PREDIMED-NAVARRA randomized, trial. J. Nutr. Health Aging 17, 544–552. doi: 10.1007/s12603-013-0027-26
Matteucci, E., and Giampietro, O. (2015). Mechanisms of neurodegeration in type 2 diabetes and the neuroprotective potential of dipeptidyl peptidase 4 inhibitors. Curr. Med. Chem. 22, 1573–1581. doi: 10.2174/0929867322666150227153308
McNay, E. C., Ong, C. T., McCrimmon, R. J., Cresswell, J., Bogan, J. S., and Sherwin, R. S. (2010). Hippocampal memory processes are modulated by insulin and high-fat-induced insulin resistance. Neurobiol. Learn. Mem. 93, 546–553. doi: 10.1016/j.nlm.2010.02.002
McNay, E. C., and Recknagel, A. K. (2011). Brain insulin signaling: a key component of cognitive processes and a potential basis for cognitive impairment in type 2 diabetes. Neurobiol. Learn. Mem. 96, 432–442. doi: 10.1016/j.nlm.2011.08.005
Meier, A. H., Cincotta, A. H., and Lovell, W. C. (1992). Timed bromocriptine administration reduces body fat stores in obese subjects and hyperglycemia in type II diabetics. Experientia 48, 248–253. doi: 10.1007/bf01930467
Mielke, J. G., and Wang, Y. T. (2005). Insulin exerts neuroprotection by counteracting the decrease in cell-surface GABA receptors following oxygen-glucose deprivation in cultured cortical neurons. J. Neurochem. 92, 103–113. doi: 10.1111/j.1471-4159.2004.02841.x
Miyake, Y., Tanaka, K., Fukushima, W., Sasaki, S., Kiyohara, C., Tsuboi, Y., et al. (2010). Case-control study of risk of Parkinson’s disease in relation to hypertension, hypercholesterolemia, and diabetes in Japan. J. Neurol. Sci. 293, 82–86. doi: 10.1016/j.jns.2010.03.002
Moore, E. M., Mander, A. G., Ames, D., Kotowicz, M. A., Carne, R. P., Brodaty, H., et al. (2013). Increased risk of cognitive impairment in patients with diabetes is associated with metformin. Diabetes Care 36, 2981–2987. doi: 10.2337/dc13-0229
Moreira, P. I., Cardoso, S. M., Santos, M. S., and Oliveira, C. R. (2006). The key role of mitochondria in Alzheimer’s disease. J. Alzheimers Dis. 9, 101–110.
Moroo, I., Yamada, T., Makino, H., Tooyama, I., McGeer, P. L., McGeer, E. G., et al. (1994). Loss of insulin receptor immunoreactivity from the substantia nigra pars compacta neurons in Parkinson’s disease. Acta Neuropathol. 87, 343–348. doi: 10.1007/bf00313602
Morris, J. K., Bomhoff, G. L., Gorres, B. K., Davis, V. A., Kim, J., Lee, P. P., et al. (2011a). Insulin resistance impairs nigrostriatal dopamine function. Exp. Neurol. 231, 171–180. doi: 10.1016/j.expneurol.2011.06.005
Morris, J. K., Seim, N. B., Bomhoff, G. L., Geiger, P. C., and Stanford, J. A. (2011b). Effects of unilateral nigrostriatal dopamine depletion on peripheral glucose tolerance and insulin signaling in middle aged rats. Neurosci. Lett. 504, 219–222. doi: 10.1016/j.neulet.2011.09.027
Morris, J. K., Bomhoff, G. L., Stanford, J. A., and Geiger, P. C. (2010). Neurodegeneration in an animal model of Parkinson’s disease is exacerbated by a high-fat diet. Am. J. Physiol. Regul. Integr. Comp. Physiol. 299, R1082–R1090. doi: 10.1152/ajpregu.00449.2010
Morris, J. K., Vidoni, E. D., Perea, R. D., Rada, R., Johnson, D. K., Lyons, K., et al. (2014). Insulin resistance and gray matter volume in neurodegenerative disease. Neuroscience 270, 139–147. doi: 10.1016/j.neuroscience.2014.04.006
Morris, J. K., Zhang, H., Gupte, A. A., Bomhoff, G. L., Stanford, J. A., and Geiger, P. C. (2008). Measures of striatal insulin resistance in a 6-hydroxydopamine model of Parkinson’s disease. Brain Res. 1240, 185–195. doi: 10.1016/j.brainres.2008.08.089
Morsi, M., Maher, A., Aboelmagd, O., Johar, D., and Bernstein, L. (2018). A shared comparison of diabetes mellitus and neurodegenerative disorders. J. Cell Biochem. 119, 1249–1256. doi: 10.1002/jcb.26261
Muriach, M., Flores-Bellver, M., Romero, F. J., and Barcia, J. M. (2014). Diabetes and the brain: oxidative stress, inflammation, and autophagy. Oxid. Med. Cell Longev. 2014:102158. doi: 10.1155/2014/102158
Musi, N., Hirshman, M. F., Nygren, J., Svanfeldt, M., Bavenholm, P., Rooyackers, O., et al. (2002). Metformin increases AMP-activated protein kinase activity in skeletal muscle of subjects with type 2 diabetes. Diabetes 51, 2074–2081. doi: 10.2337/diabetes.51.7.2074
Nagao, M., and Hayashi, H. (2009). Glycogen synthase kinase-3beta is associated with Parkinson’s disease. Neurosci. Lett. 449, 103–107. doi: 10.1016/j.neulet.2008.10.104
Nascimento, P. S., Lovatel, G. A., Barbosa, S., Ilha, J., Centenaro, L. A., Malysz, T., et al. (2011). Treadmill training improves motor skills and increases tyrosine hydroxylase immunoreactivity in the substantia nigra pars compacta in diabetic rats. Brain Res. 1382, 173–180. doi: 10.1016/j.brainres.2011.01.063
Nassar, N. N., Al-Shorbagy, M. Y., Arab, H. H., and Abdallah, D. M. (2015). Saxagliptin: a novel antiparkinsonian approach. Neuropharmacology 89, 308–317. doi: 10.1016/j.neuropharm.2014.10.007
Nath, N., Khan, M., Paintlia, M. K., Singh, I., Hoda, M. N., and Giri, S. (2009). Metformin attenuated the autoimmune disease of the central nervous system in animal models of multiple sclerosis. J. Immunol. 182, 8005–8014. doi: 10.4049/jimmunol.0803563
Ng, C. H., Guan, M. S., Koh, C., Ouyang, X., Yu, F., Tan, E. K., et al. (2012). AMP kinase activation mitigates dopaminergic dysfunction and mitochondrial abnormalities in Drosophila models of Parkinson’s disease. J. Neurosci. 32, 14311–14317. doi: 10.1523/JNEUROSCI.0499-12.2012
NINDS Exploratory Trials in Parkinson Disease (NET-PD) FS-ZONE Investigators (2015). Pioglitazone in. (early)Parkinson’s disease: a phase 2, multicentre, double-blind, randomised trial. Lancet Neurol. 14, 795–803. doi: 10.1016/S1474-4422(15)00144-141
Okubo, H., Miyake, Y., Sasaki, S., Murakami, K., Tanaka, K., Fukushima, W., et al. (2012). Dietary patterns and risk of Parkinson’s disease: a case-control study in Japan. Eur. J. Neurol. 19, 681–688. doi: 10.1111/j.1468-1331.2011.03600.x
Olanow, C. W., Stern, M. B., and Sethi, K. (2009). The scientific and clinical basis for the treatment of Parkinson disease (2009). Neurology 72(21 Suppl. 4), S1–S136. doi: 10.1212/WNL.0b013e3181a1d44c
Pan, P. L., Shi, H. C., Zhong, J. G., Xiao, P. R., Shen, Y., Wu, L. J., et al. (2013). Gray matter atrophy in Parkinson’s disease with dementia: evidence from meta-analysis of voxel-based morphometry studies. Neurol. Sci. 34, 613–619. doi: 10.1007/s10072-012-1250-1253
Pang, Y., Lin, S., Wright, C., Shen, J., Carter, K., Bhatt, A., et al. (2016). Intranasal insulin protects against substantia nigra dopaminergic neuronal loss and alleviates motor deficits induced by 6-OHDA in rats. Neuroscience 318, 157–165. doi: 10.1016/j.neuroscience.2016.01.020
Papapetropoulos, S., Ellul, J., Argyriou, A. A., Talelli, P., Chroni, E., and Papapetropoulos, T. (2004). The effect of vascular disease on late onset Parkinson’s disease. Eur. J. Neurol. 11, 231–235. doi: 10.1046/j.1468-1331.2003.00748.x
Pardridge, W. M., Eisenberg, J., and Yang, J. (1985). Human blood-brain barrier insulin receptor. J. Neurochem. 44, 1771–1778. doi: 10.1111/j.1471-4159.1985.tb07167.x
Park, C. R., Seeley, R. J., Craft, S., and Woods, S. C. (2000). Intracerebroventricular insulin enhances memory in a passive-avoidance task. Physiol. Behav. 68, 509–514. doi: 10.1016/S0031-9384(99)00220-6
Patel, J. C., Stouffer, M. A., Mancini, M., Nicholson, C., Carr, K. D., and Rice, M. E. (2018). Interactions between insulin and diet on striatal dopamine uptake kinetics in rodent brain slices. Eur. J. Neurosci. 49, 794–804. doi: 10.1111/ejn.13958
Patterson, T. A., Brot, M. D., Zavosh, A., Schenk, J. O., Szot, P., and Figlewicz, D. P. (1998). Food deprivation decreases mRNA and activity of the rat dopamine transporter. Neuroendocrinology 68, 11–20. doi: 10.1159/000054345
Peng, J., and Andersen, J. K. (2003). The role of c-Jun N-terminal kinase (JNK) in Parkinson’s disease. IUBMB Life 55, 267–271. doi: 10.1080/1521654031000121666
Perez-Revuelta, B. I., Hettich, M. M., Ciociaro, A., Rotermund, C., Kahle, P. J., Krauss, S., et al. (2014). Metformin lowers Ser-129 phosphorylated alpha-synuclein levels via mTOR-dependent protein phosphatase 2A activation. Cell Death Dis. 5:e1209. doi: 10.1038/cddis.2014.175
Perlmuter, L. C., Hakami, M. K., Hodgson-Harrington, C., Ginsberg, J., Katz, J., Singer, D. E., et al. (1984). Decreased cognitive function in aging non-insulin-dependent diabetic patients. Am. J. Med. 77, 1043–1048. doi: 10.1016/0002-9343(84)90186-4
Perruolo, G., Viggiano, D., Fiory, F., Cassese, A., Nigro, C., Liotti, A., et al. (2016). Parkinson-like phenotype in insulin-resistant PED/PEA-15 transgenic mice. Sci. Rep. 6:29967. doi: 10.1038/srep29967
Pijl, H., Ohashi, S., Matsuda, M., Miyazaki, Y., Mahankali, A., Kumar, V., et al. (2000). Bromocriptine: a novel approach to the treatment of type 2 diabetes. Diabetes Care 23, 1154–1161. doi: 10.2337/diacare.23.8.1154
Plata-Salaman, C. R. (1991). Insulin in the cerebrospinal fluid. Neurosci. Biobehav. Rev. 15, 243–258.
Platt, T. L., Beckett, T. L., Kohler, K., Niedowicz, D. M., and Murphy, M. P. (2016). Obesity, diabetes, and leptin resistance promote tau pathology in a mouse model of disease. Neuroscience 315, 162–174. doi: 10.1016/j.neuroscience.2015.12.011
Plum, L., Schubert, M., and Bruning, J. C. (2005). The role of insulin receptor signaling in the brain. Trends Endocrinol. Metab. 16, 59–65. doi: 10.1016/j.tem.2005.01.008
Powers, K. M., Smith-Weller, T., Franklin, G. M., Longstreth, W. T. Jr., Swanson, P. D., and Checkoway, H. (2006). Diabetes, smoking, and other medical conditions in relation to Parkinson’s disease risk. Parkinsonism Relat. Disord. 12, 185–189. doi: 10.1016/j.parkreldis.2005.09.004
Prasad-Reddy, L., and Isaacs, D. (2015). A clinical review of GLP-1 receptor agonists: efficacy and safety in diabetes and beyond. Drugs Context 4:212283. doi: 10.7573/dic.212283
Qin, X. Y., Zhang, S. P., Cao, C., Loh, Y. P., and Cheng, Y. (2016). Aberrations in peripheral inflammatory cytokine levels in parkinson disease: a systematic review and meta-analysis. JAMA Neurol. 73, 1316–1324. doi: 10.1001/jamaneurol.2016.2742
Rabchevsky, A. G., Patel, S. P., and Sullivan, P. G. (2017). Targeting mitoNEET with pioglitazone for therapeutic neuroprotection after spinal cord injury. Neural Regen. Res. 12, 1807–1808. doi: 10.4103/1673-5374.219040
Ramalingam, M., and Kim, S. J. (2014). The role of insulin against hydrogen peroxide-induced oxidative damages in differentiated SH-SY5Y cells. J. Recept. Signal. Transduct. Res. 34, 212–220. doi: 10.3109/10799893.2013.876043
Ramalingam, M., and Kim, S. J. (2016a). Insulin on activation of autophagy with integrins and syndecans against MPP(+)-induced alpha-synuclein neurotoxicity. Neurosci. Lett. 633, 94–100. doi: 10.1016/j.neulet.2016.09.023
Ramalingam, M., and Kim, S. J. (2016b). The neuroprotective role of insulin against MPP(+) -induced parkinson’s disease in differentiated SH-SY5Y Cells. J. Cell Biochem. 117, 917–926. doi: 10.1002/jcb.25376
Ramalingam, M., and Kim, S. J. (2017). Protective effects of activated signaling pathways by insulin on C6 glial cell model of MPP(+)-induced Parkinson’s disease. J. Recept. Signal. Transduct. Res. 37, 100–107. doi: 10.3109/10799893.2016.1171342
Reale, M., Greig, N. H., and Kamal, M. A. (2009a). Peripheral chemo-cytokine profiles in Alzheimer’s and Parkinson’s diseases. Mini Rev. Med. Chem. 9, 1229–1241. doi: 10.2174/138955709789055199
Reale, M., Iarlori, C., Thomas, A., Gambi, D., Perfetti, B., Di Nicola, M., et al. (2009b). Peripheral cytokines profile in Parkinson’s disease. Brain Behav. Immun. 23, 55–63. doi: 10.1016/j.bbi.2008.07.003
Rena, G., Hardie, D. G., and Pearson, E. R. (2017). The mechanisms of action of metformin. Diabetologia 60, 1577–1585. doi: 10.1007/s00125-017-4342-z
Rinne, U. K., and Molsa, P. (1979). Levodopa with benserazide or carbidopa in Parkinson disease. Neurology 29, 1584–1589. doi: 10.1212/wnl.29.12.1584
Rodriguez-Araujo, G., Nakagami, H., Takami, Y., Katsuya, T., Akasaka, H., Saitoh, S., et al. (2015). Low alpha-synuclein levels in the blood are associated with insulin resistance. Sci. Rep. 5:12081. doi: 10.1038/srep12081
Rom, S., Zuluaga-Ramirez, V., Gajghate, S., Seliga, A., Winfield, M., Heldt, N. A., et al. (2018). Hyperglycemia-Driven neuroinflammation compromises BBB leading to memory loss in both diabetes mellitus (DM) Type 1 and Type 2 mouse models. Mol. Neurobiol. 56, 1883–1896. doi: 10.1007/s12035-018-1195-5
Rusnak, M., Jelokova, J., Vietor, I., Sabban, E. L., and Kvetnansky, R. (1998). Different effects of insulin and 2-deoxy-D-glucose administration on tyrosine hydroxylase gene expression in the locus coeruleus and the adrenal medulla in rats. Brain Res. Bull. 46, 447–452. doi: 10.1016/s0361-9230(98)00033-1
Sandyk, R. (1993). The relationship between diabetes mellitus and Parkinson’s disease. Int. J. Neurosci. 69, 125–130.
Sarkar, S., Davies, J. E., Huang, Z., Tunnacliffe, A., and Rubinsztein, D. C. (2007). Trehalose, a novel mTOR-independent autophagy enhancer, accelerates the clearance of mutant huntingtin and alpha-synuclein. J. Biol. Chem. 282, 5641–5652. doi: 10.1074/jbc.M609532200
Schapira, A. H. V., Chaudhuri, K. R., and Jenner, P. (2017). Non-motor features of Parkinson disease. Nat. Rev. Neurosci. 18, 435–450. doi: 10.1038/nrn.2017.62
Schernhammer, E., Hansen, J., Rugbjerg, K., Wermuth, L., and Ritz, B. (2011). Diabetes and the risk of developing Parkinson’s disease in Denmark. Diabetes Care 34, 1102–1108. doi: 10.2337/dc10-1333
Schubert, M., Gautam, D., Surjo, D., Ueki, K., Baudler, S., Schubert, D., et al. (2004). Role for neuronal insulin resistance in neurodegenerative diseases. Proc. Natl. Acad. Sci. U.S.A. 101, 3100–3105. doi: 10.1073/pnas.0308724101
Sekar, S., and Taghibiglou, C. (2018). Elevated nuclear phosphatase and tensin homolog (PTEN) and altered insulin signaling in substantia nigral region of patients with Parkinson’s disease. Neurosci. Lett. 666, 139–143. doi: 10.1016/j.neulet.2017.12.049
Sharma, A. N., Elased, K. M., and Lucot, J. B. (2012). Rosiglitazone treatment reversed depression- but not psychosis-like behavior of db/db diabetic mice. J. Psychopharmacol. 26, 724–732. doi: 10.1177/0269881111434620
Sharma, S., and Taliyan, R. (2018). High fat diet feeding induced insulin resistance exacerbates 6-OHDA mediated neurotoxicity and behavioral abnormalities in rats. Behav. Brain Res. 351, 17–23. doi: 10.1016/j.bbr.2018.05.025
Sharma, S. K., Chorell, E., Steneberg, P., Vernersson-Lindahl, E., Edlund, H., and Wittung-Stafshede, P. (2015). Insulin-degrading enzyme prevents alpha-synuclein fibril formation in a nonproteolytical manner. Sci. Rep. 5:12531. doi: 10.1038/srep12531
Shokrzadeh, M., Mirshafa, A., Yekta Moghaddam, N., Birjandian, B., and Shaki, F. (2018). Mitochondrial dysfunction contribute to diabetic neurotoxicity induced by streptozocin in mice: protective effect of Urtica dioica and pioglitazone. Toxicol. Mech. Methods 28, 499–506. doi: 10.1080/15376516.2018.1459993
Simo, R., Ciudin, A., Simo-Servat, O., and Hernandez, C. (2017). Cognitive impairment and dementia: a new emerging complication of type 2 diabetes-The diabetologist’s perspective. Acta Diabetol. 54, 417–424. doi: 10.1007/s00592-017-0970-975
Singh, B. S., Rajakumar, P. A., Eves, E. M., Rosner, M. R., Wainer, B. H., and Devaskar, S. U. (1997). Insulin gene expression in immortalized rat hippocampal and pheochromocytoma-12 cell lines. Regul. Pept. 69, 7–14. doi: 10.1016/s0167-0115(96)02120-9
Speed, N., Saunders, C., Davis, A. R., Owens, W. A., Matthies, H. J., Saadat, S., et al. (2011). Impaired striatal Akt signaling disrupts dopamine homeostasis and increases feeding. PLoS One 6:e25169. doi: 10.1371/journal.pone.0025169
Speed, N. K., Matthies, H. J., Kennedy, J. P., Vaughan, R. A., Javitch, J. A., Russo, S. J., et al. (2010). Akt-dependent and isoform-specific regulation of dopamine transporter cell surface expression. ACS Chem. Neurosci. 1, 476–481. doi: 10.1021/cn100031t
Sreenan, S., Sturis, J., Pugh, W., Burant, C. F., and Polonsky, K. S. (1996). Prevention of hyperglycemia in the Zucker diabetic fatty rat by treatment with metformin or troglitazone. Am. J. Physiol. 271(4 Pt 1), E742–E747. doi: 10.1152/ajpendo.1996.271.4.E742
Sun, Y., Chang, Y. H., Chen, H. F., Su, Y. H., Su, H. F., and Li, C. Y. (2012). Risk of Parkinson disease onset in patients with diabetes: a 9-year population-based cohort study with age and sex stratifications. Diabetes Care 35, 1047–1049. doi: 10.2337/dc11-1511
Svenningsson, P., Wirdefeldt, K., Yin, L., Fang, F., Markaki, I., Efendic, S., et al. (2016). Reduced incidence of Parkinson’s disease after dipeptidyl peptidase-4 inhibitors-A nationwide case-control study. Mov. Disord. 31, 1422–1423. doi: 10.1002/mds.26734
Swanson, C., and Emborg, M. (2014). Expression of peroxisome proliferator-activated receptor-gamma in the substantia nigra of hemiparkinsonian nonhuman primates. Neurol. Res. 36, 634–646. doi: 10.1179/1743132813Y.0000000305
Tain, L. S., Mortiboys, H., Tao, R. N., Ziviani, E., Bandmann, O., and Whitworth, A. J. (2009). Rapamycin activation of 4E-BP prevents parkinsonian dopaminergic neuron loss. Nat. Neurosci. 12, 1129–1135. doi: 10.1038/nn.2372
Takahashi, M., Yamada, T., Tooyama, I., Moroo, I., Kimura, H., Yamamoto, T., et al. (1996). Insulin receptor mRNA in the substantia nigra in Parkinson’s disease. Neurosci. Lett. 204, 201–204. doi: 10.1016/0304-3940(96)12357-0
Timmons, S., Coakley, M. F., Moloney, A. M., and O’ Neill, C. (2009). Akt signal transduction dysfunction in Parkinson’s disease. Neurosci. Lett. 467, 30–35. doi: 10.1016/j.neulet.2009.09.055
Tokutake, T., Kasuga, K., Yajima, R., Sekine, Y., Tezuka, T., Nishizawa, M., et al. (2012). Hyperphosphorylation of Tau induced by naturally secreted amyloid-beta at nanomolar concentrations is modulated by insulin-dependent Akt-GSK3beta signaling pathway. J. Biol. Chem. 287, 35222–35233. doi: 10.1074/jbc.M112.348300
Vanhanen, M., Kuusisto, J., Koivisto, K., Mykkanen, L., Helkala, E. L., Hanninen, T., et al. (1999). Type-2 diabetes and cognitive function in a non-demented population. Acta Neurol Scand. 100, 97–101. doi: 10.1111/j.1600-0404.1999.tb01045.x
Viollet, B., Guigas, B., Sanz Garcia, N., Leclerc, J., Foretz, M., and Andreelli, F. (2012). Cellular and molecular mechanisms of metformin: an overview. Clin. Sci. 122, 253–270. doi: 10.1042/CS20110386
Wahlqvist, M. L., Lee, M. S., Hsu, C. C., Chuang, S. Y., Lee, J. T., and Tsai, H. N. (2012). Metformin-inclusive sulfonylurea therapy reduces the risk of Parkinson’s disease occurring with Type 2 diabetes in a Taiwanese population cohort. Parkinsonism Relat. Disord. 18, 753–758. doi: 10.1016/j.parkreldis.2012.03.010
Wang, L., Zhai, Y. Q., Xu, L. L., Qiao, C., Sun, X. L., Ding, J. H., et al. (2014). Metabolic inflammation exacerbates dopaminergic neuronal degeneration in response to acute MPTP challenge in type 2 diabetes mice. Exp. Neurol. 251, 22–29. doi: 10.1016/j.expneurol.2013.11.001
Wang, S., Zhang, C., Sheng, X., Zhang, X., Wang, B., and Zhang, G. (2014). Peripheral expression of MAPK pathways in Alzheimer’s and Parkinson’s diseases. J. Clin. Neurosci. 21, 810–814. doi: 10.1016/j.jocn.2013.08.017
Wang, X., Yu, S., Hu, J. P., Wang, C. Y., Wang, Y., Liu, H. X., et al. (2014). Streptozotocin-induced diabetes increases amyloid plaque deposition in AD transgenic mice through modulating AGEs/RAGE/NF-kappaB pathway. Int. J. Neurosci. 124, 601–608. doi: 10.3109/00207454.2013.866110
Wilhelm, K. R., Yanamandra, K., Gruden, M. A., Zamotin, V., Malisauskas, M., Casaite, V., et al. (2007). Immune reactivity towards insulin, its amyloid and protein S100B in blood sera of Parkinson’s disease patients. Eur. J. Neurol. 14, 327–334. doi: 10.1111/j.1468-1331.2006.01667.x
Williams-Gray, C. H., Evans, J. R., Goris, A., Foltynie, T., Ban, M., Robbins, T. W., et al. (2009). The distinct cognitive syndromes of Parkinson’s disease: 5 year follow-up of the CamPaIGN cohort. Brain 132(Pt 11), 2958–2969. doi: 10.1093/brain/awp245
Xu, L., Chen, X., Sun, B., Sterling, C., and Tank, A. W. (2007). Evidence for regulation of tyrosine hydroxylase mRNA translation by stress in rat adrenal medulla. Brain Res. 1158, 1–10. doi: 10.1016/j.brainres.2007.04.080
Yamada, N., Katsuura, G., Ochi, Y., Ebihara, K., Kusakabe, T., Hosoda, K., et al. (2011). Impaired CNS leptin action is implicated in depression associated with obesity. Endocrinology 152, 2634–2643. doi: 10.1210/en.2011-2014
Yang, L., Chen, Z., Li, B., Wang, M., Yu, L., Wan, Y., et al. (2017). Multiple evidences for association between cognitive impairment and dysglycemia in Parkinson’s disease: implications for clinical practice. Front. Aging Neurosci. 9:355. doi: 10.3389/fnagi.2017.00355
Yang, L., Wang, H., Liu, L., and Xie, A. (2018). The role of insulin/IGF-1/PI3K/Akt/GSK3beta signaling in parkinson’s disease dementia. Front. Neurosci. 12:73. doi: 10.3389/fnins.2018.00073
Yazbeck, R., Howarth, G. S., and Abbott, C. A. (2009). Dipeptidyl peptidase inhibitors, an emerging drug class for inflammatory disease? Trends Pharmacol. Sci. 30, 600–607. doi: 10.1016/j.tips.2009.08.003
Zhao, W. Q., Chen, H., Quon, M. J., and Alkon, D. L. (2004). Insulin and the insulin receptor in experimental models of learning and memory. Eur. J. Pharmacol. 490, 71–81. doi: 10.1016/j.ejphar.2004.02.045
Zhao, W. Q., and Townsend, M. (2009). Insulin resistance and amyloidogenesis as common molecular foundation for type 2 diabetes and Alzheimer’s disease. Biochim. Biophys. Acta 1792, 482–496. doi: 10.1016/j.bbadis.2008.10.014
Keywords: type 2 diabetes mellitus, insulin resistance, Parkinson’s disease, dopamine, neurodegeneration
Citation: Fiory F, Perruolo G, Cimmino I, Cabaro S, Pignalosa FC, Miele C, Beguinot F, Formisano P and Oriente F (2019) The Relevance of Insulin Action in the Dopaminergic System. Front. Neurosci. 13:868. doi: 10.3389/fnins.2019.00868
Received: 27 February 2019; Accepted: 02 August 2019;
Published: 16 August 2019.
Edited by:
Eugenio Barone, Sapienza University of Rome, ItalyReviewed by:
Elizabeth M. Rhea, VA Puget Sound Health Care System, United StatesCopyright © 2019 Fiory, Perruolo, Cimmino, Cabaro, Pignalosa, Miele, Beguinot, Formisano and Oriente. This is an open-access article distributed under the terms of the Creative Commons Attribution License (CC BY). The use, distribution or reproduction in other forums is permitted, provided the original author(s) and the copyright owner(s) are credited and that the original publication in this journal is cited, in accordance with accepted academic practice. No use, distribution or reproduction is permitted which does not comply with these terms.
*Correspondence: Pietro Formisano, ZnBpZXRyb0B1bmluYS5pdA==
†These authors have contributed equally to this work
Disclaimer: All claims expressed in this article are solely those of the authors and do not necessarily represent those of their affiliated organizations, or those of the publisher, the editors and the reviewers. Any product that may be evaluated in this article or claim that may be made by its manufacturer is not guaranteed or endorsed by the publisher.
Research integrity at Frontiers
Learn more about the work of our research integrity team to safeguard the quality of each article we publish.