- Department of Biomedical Sciences, Parkinson’s Disorder Research Laboratory, Iowa State University, Ames, IA, United States
Occupational or environmental exposure to manganese (Mn) can lead to the development of “Manganism,” a neurological condition showing certain motor symptoms similar to Parkinson’s disease (PD). Like PD, Mn toxicity is seen in the central nervous system mainly affecting nigrostriatal neuronal circuitry and subsequent behavioral and motor impairments. Since the first report of Mn-induced toxicity in 1837, various experimental and epidemiological studies have been conducted to understand this disorder. While early investigations focused on the impact of high concentrations of Mn on the mitochondria and subsequent oxidative stress, current studies have attempted to elucidate the cellular and molecular pathways involved in Mn toxicity. In fact, recent reports suggest the involvement of Mn in the misfolding of proteins such as α-synuclein and amyloid, thus providing credence to the theory that environmental exposure to toxicants can either initiate or propagate neurodegenerative processes by interfering with disease-specific proteins. Besides manganism and PD, Mn has also been implicated in other neurological diseases such as Huntington’s and prion diseases. While many reviews have focused on Mn homeostasis, the aim of this review is to concisely synthesize what we know about its effect primarily on the nervous system with respect to its role in protein misfolding, mitochondrial dysfunction, and consequently, neuroinflammation and neurodegeneration. Based on the current evidence, we propose a ‘Mn Mechanistic Neurotoxic Triad’ comprising (1) mitochondrial dysfunction and oxidative stress, (2) protein trafficking and misfolding, and (3) neuroinflammation.
Metals in Biology
At least 13 metals have been identified as essential for life, and four of these (sodium, potassium, magnesium, and calcium) occur in large amounts. The remaining nine trace metals (manganese, iron, cobalt, vanadium, chromium, molybdenum, nickel, copper, and zinc) assume vital roles in building organic biomolecules as well as in regulating biological functions. In the last couple of decades, the importance of metal ions in protein biology has been an increasingly attractive research subject given their association with many human diseases, for which metals have been identified as a causative or stimulatory agent. Metals are essential because of their integral role in enzymes that catalyze the basic metabolic or biochemical processes shared by all forms of life on earth. About one-third of all proteins depend on metal ions to carry out their biological functions (Holm et al., 1996). When considering all six classes of enzymes – oxidoreductases, transferases, hydrolases, lyases, isomerases, and ligases – over 40% of all enzymes contain metals (Andreini et al., 2008). Moreover, the chemistry of metals allows for a broader set of protein-metal reactions. For instance, redox-active metal ions are often interchangeable depending on the metal concentration and their affinities to protein. Protein affinities for trace metals are substantially determined by universal series, which for divalent metals is the Irving-Williams series (Mn2+ < Fe2+ < Co2+ < Ni2+ < Cu2+ > Zn2+), wherein Cu2+ is highly competitive and can replace lower order metals (Tottey et al., 2008).
These “metalloproteins” are involved in many key biological processes, such as gas transport, cell respiration, antioxidant defense, photosynthesis, and many other vital redox reactions driven by their interaction with metals. Well-characterized examples for redox-active metalloprotein systems are blue-copper proteins, heme-binding proteins and iron-sulfur-cluster proteins. Moreover, recent advances in synthetic chemistry have focused on the study of metal sites in metalloproteins and metalloenzymes to influence biological processes in the battle against many daunting human diseases. Advanced medicinal chemistry approaches have given us new, innovative medicinal applications of metal complexes and organometallic agents. Prime examples for such uses of metals include platinum-containing anticancer drugs (e.g., Cisplatin), lithium-containing depression drugs (e.g., Camcolit), and manganese (Mn)-containing anticancer drugs (e.g., SOD mimics) (Farrell, 2003).
Presumably, all metalloproteins would bind to their desired metal ligands, and this binding can regulate their folding. However, despite the wealth of structural information, the coupled protein-folding, metal-binding pathways for metalloproteins remain largely unknown (Wittung-Stafshede, 2002). Proper protein folding is critical to the conformational integrity and function of proteins. However, metal ligand binding can also induce undesirable structural transitions in proteins that eventually lead to the formation of pathological protein aggregates. Indeed, the pathologies of Alzheimer’s disease (AD), PD, and prion diseases are linked to abnormal misfolding of otherwise harmless neural proteins. For example, in AD, increased levels of metals, such as Cu2+ and Zn2+, are linked to the aggregation of Aβ protein in vitro (Kenche and Barnham, 2011). The theory of metal-induced aggregation is supported by numerous studies tying metal concentrations in the brain with AD, PD, and amyotrophic lateral sclerosis (ALS) in in vivo and in vitro studies employing recombinant proteins (Brown et al., 2005; Brown, 2011).
In this review, we will focus on α-synuclein, one of the major proteins implicated in PD, and its interactions with metals, specifically, its interaction with Mn in oxidative stress, protein aggregation and neurodegeneration.
Parkinson’s Disease
Parkinson’s disease is recognized as the second most prevalent neurodegenerative disorder after AD, affecting roughly 1% of the population over the age of 65. It is also the most common movement disorder in the elderly, resulting in bradykinesia, resting tremor, and rigidity (Lotharius and Brundin, 2002). Several non-motor symptoms involving the autonomic nervous system have also been gaining attention (Pfeiffer, 2009; Schapira et al., 2017). PD is characterized histopathologically by the degeneration of dopaminergic neurons in the substantia nigra pars compacta (SNpc), leading to the progressive loss of the neurotransmitter dopamine and hence the above-mentioned cardinal motor deficits. Even though PD is also often associated with the abnormal accumulation of misfolded proteins, primarily α-synuclein, in cytoplasmic inclusions called Lewy bodies (LB) and Lewy neurites, the pathophysiological association between Lewy pathology and disease pathogenesis is not well understood. Similar neuropathological lesions involving the deposition of abnormal proteins also characterize other neurological disorders (Ross and Poirier, 2004), including AD (Kotzbauer et al., 2001; Uchikado et al., 2006), Lewy body dementia (LBD) (McKeith et al., 2004), Huntington’s disease (HD) (Davis et al., 2014), multiple system atrophy (MSA) (Shoji et al., 2000), and some prion diseases (Aguzzi and Calella, 2009; Aguzzi and O’Connor, 2010).
Although aging remains the greatest risk factor for idiopathic PD, a small fraction of patients were identified with familial PD, which is caused by mutations in several genes associated with protein metabolism, ion transport and mitochondrial function. Genes associated with early-onset PD include α-synuclein (PARK1), parkin (PARK-2), PINK1 (PARK6), DJ-1 (PARK7) and ATP13A2 (PARK9), while those linked with late-onset PD include LRRK2 (PARK8) and VPS35 (PARK-17) (Dawson et al., 2010; Roth, 2014). A growing number of epidemiological and clinical studies have identified environmental risk factors for PD, including repeated head trauma, heavy metal toxicity, pesticide toxicity, obesity, and some surrogate measures such as rural living, contaminated well water, substance abuse, and farming (Priyadarshi et al., 2001; Dick et al., 2007). Interestingly, some of these environmental triggers and toxins induce pathophysiological features that mimic PD when they are administered in experimental animal settings. One such toxin is MPTP (methyl-4-phenyl-1,2,3,6-tetrahydropyridine), a compound produced as an impurity during synthesis of the illicit narcotic desmethylprodine. MPTP causes chronic and severe Parkinsonism by selectively damaging the SN, resulting in PD-related motor deficits (Langston et al., 1983; Ballard et al., 1985; Appendino et al., 2014). Other compounds widely used in experimental models to study the etiopathogenesis of PD include the narcotic methamphetamine, the dopamine derivative 6-hydroxydopamine, and pesticides such as rotenone, paraquat, and dieldrin. These neurotoxins cause nigrostriatal cell death by interfering with mitochondrial function, inducing oxidative stress, protein aggregation, and modifying proteasomal function (Kanthasamy et al., 2008; Latchoumycandane et al., 2011; Ghosh et al., 2013; Jin et al., 2015b). In addition, exposure to heavy metals (e.g., iron, lead, mercury, cadmium, arsenic, and Mn) and metal-based nanoparticles increases the risk of PD through the neurotoxic accumulation of metals in the SNpc and by increasing oxidative stress-induced apoptosis (Afeseh Ngwa et al., 2009; Milatovic et al., 2009; Afeseh Ngwa et al., 2011; Kanthasamy et al., 2012; Aboud et al., 2014; Harischandra et al., 2015a).
Manganese
Manganese is considered to be a key inhaled environmental pollutant as well as a putative risk factor for environmentally linked PD and related neurodegenerative disorders. Being the 12th most abundant element and composing approximately 0.1% of the earth’s crust, Mn is ubiquitously present in the environment (Martinez-Finley et al., 2012). Besides the earth’s crust, other Mn sources include direct atmospheric deposition, wash-off from plant and other surfaces, leaching from plant tissues, ocean spray, and volcanic activity. Mn occurs in trace amounts in all body tissues as it is essential for many ubiquitous enzymatic reactions, including the synthesis of amino acids (AA), lipids, proteins, and carbohydrates. It also plays a key nutritional role in bone growth, fat and carbohydrate metabolism, blood sugar regulation, and calcium absorption (Bowman et al., 2011). Being present in whole grains, rice, nuts, tea, leafy green vegetables, and Mn-containing nutritional supplements, the primary route of Mn exposure in humans is through dietary intake. The abundance of Mn-enriched food in the typical daily diet makes it relatively easy to accrue the daily reference intake (DRI) of 2.3 mg/day for men and 1.8 mg/day for women (Aschner and Aschner, 2005), thereby minimizing the risk of Mn deficiency-related birth defects, impaired fertility, osteoporosis, and enhanced susceptibility to seizures (Dendle, 2001; Aschner and Aschner, 2005; Sarban et al., 2007).
Despite its nutritional benefits, prenatal and postnatal overexposure to Mn affects infant neurodevelopment, exemplifying its role as both an essential nutrient and a toxicant (Zota et al., 2009; Claus Henn et al., 2010). High Mn exposure in early life is associated with poor cognitive performance, especially in the verbal domain of children (Menezes-Filho et al., 2011). In older cohorts, chronic excessive exposure to occupational or environmental sources of Mn causes manganism, which is characterized by a severe neurological deficit that often resembles the involuntary extrapyramidal symptoms associated with PD (Kwakye et al., 2015). Couper (1837), at the University of Glasgow, reported the first case of Mn-induced neurotoxicity, which was discovered in employees of Charles Tennant and Co., a manufacturer of bleaching powder. Later, public awareness of Mn neurotoxicity arose as more clinical studies identified a PD-like syndrome in workers employed at a Mn ore-crushing plant and a ferromanganese factory (Cook et al., 1974; Huang et al., 1989). In addition, Rodier (1955) detailed clinical features of manganese neurotoxicity in Moroccan miners. Since then, the commercial applications for Mn have broadened considerably so that now Mn exposure also occurs through its use as an additive in gasoline (methylcyclopentadienyl manganese tricarbonyl, MMT) and fertilizers, and as manganese violet in paint and cosmetics (Martinez-Finley et al., 2012). Mn neurotoxicity occurs often in agricultural workers exposed to organic Mn-containing pesticides, such as manganese ethylene-bis-dithiocarbamate (Maneb) and in chronic abusers of the street drug ‘Bazooka’, a cocaine-based drug contaminated with manganese carbonate (Ensing, 1985). The other major anthropogenic sources of environmental Mn include municipal wastewater discharge, welding, mining and mineral processing, metal (alloy, steel, and iron) manufacturing emissions, fossil fuel combustion, and dry-cell manufacturing. Although the precise mechanisms through which Mn is absorbed into the body are not fully understood, it is known to accumulate predominantly in the brain’s basal ganglia region. Beyond the many commonalities shared between manganism and PD, it is also worth pointing out their differences. Behaviorally, manganism is mainly characterized by milder and less frequent resting tremor that tends to be postural or actional, a propensity to fall backward, excessive salivation, and frequent dystonia consisting of facial grimacing, hand dystonia, and/or plantar flexion (Calne et al., 1994). Manganism patients were also reported to have symptoms of irritability, emotional lability, and hallucinations and psychoses referred to as “manganese madness” (Huang, 2007). Pathologically, Mn neurotoxicity affects primarily neurons in both the globus pallidus and striatum, whereas PD predominantly affects dopaminergic neurons in the SNpc (Roth, 2014). Therefore, in fact, the PD-like behavior deficits in manganism result from Mn’s capability to suppress dopamine release from the striatum, thus generating behavioral dysfunctions common to both PD and manganism (Kim et al., 2002; Racette et al., 2005; Fitsanakis et al., 2006; Roth et al., 2013).
Manganese Homeostasis
The homeostasis of Mn and other metal ions is maintained through tightly regulated mechanisms of uptake, storage, and secretion that strictly limit their abundance in the cellular compartment. The distribution and neurotoxicity of Mn is governed largely by the routes of exposure, which are primarily ingestion and inhalation. In humans, the primary route of exposure is through Mn-enriched food and well water. However, the molecular mechanisms of oral Mn absorption are not well understood. Roughly 3–5% of the Mn ingested gets absorbed into the body from the gastrointestinal tract (GIT) (Finley et al., 1994). Under homeostatic conditions, Mn enters the portal circulation through either passive diffusion (Bell et al., 1989) or active transport via divalent metal transporter 1 (DMT1) (Erikson and Aschner, 2006; Fitsanakis et al., 2007), which was the first mammalian transmembrane iron transporter to be identified. Formerly known as Nramp2 or DCT1, DMT1 is a 12-transmembrane domain protein responsible for the uptake of various divalent metals including Fe2+, Mn2+, Zn2+, Co2+, and Ni2+, and it transfers iron across the apical surface of intestinal cells and out via transferrin (Tf)-cycle endosomes (Andrews, 1999). Besides using a mechanism similar to that for iron, there are no known metal transporters specific for transporting Mn into cells. In plasma, approximately 80% of Mn2+ is bound to α-macroglobulin or albumin, while only a small fraction (<1%) of Mn3+ is bound to Tf. It has been proposed that, like iron, Mn in plasma is oxidized from Mn2+ to the Mn3+ valence state by the ferroxidase enzyme ceruloplasmin and loaded onto plasma Tf for circulating into tissues (Davidsson et al., 1989). Circulating Mn diffuses throughout the body, including bone, kidney, pancreas, liver, and brain (Martinez-Finley et al., 2012).
Once in the brain, Mn3+ entry into neurons occurs by the Tf-Mn3+ complex binding to the transferring receptor (TfR) and it becomes localized in endosomes. Subsequent recruitment of v-ATPases acidifies endosomes and dissociates Mn3+ from the Tf/TfR complex, reducing it to Mn2+, which is quite stable at physiological pH, and thereafter, neuronal transport occurs via DMT1 independent of the Tf pathway. In the brain, DMT1 is highly expressed in the DA-rich basal ganglia, putamen, cortex, and SN (Huang et al., 2004; Salazar et al., 2008), which may account for Mn’s pattern of accumulation and neurotoxicity. Other primary transport mechanisms for Mn are through capillary endothelial cells of the blood-brain-barrier (BBB) (Crossgrove et al., 2003) or through the CSF via the choroid plexus (Murphy et al., 1991). Since Mn neurotoxicity primarily occurs through occupational exposure, such as inhalation of Mn fumes or dust in welding, dry-cell battery manufacturing, and the smelting industry, the nasal passage through the olfactory epithelium to the olfactory nerve is another major Mn transport mechanism into the brain (Tjalve et al., 1996). In fact, DMT1 is highly expressed in the olfactory epithelium and is required for Mn transport across the olfactory epithelium, as has been shown in the rat (Thompson et al., 2007). Evidence also exists for Mn transport into the central nervous system (CNS) through store-operated calcium channels (Crossgrove and Yokel, 2005), ionotropic glutamate receptor calcium channels (Kannurpatti et al., 2000), and Mn citrate transporters (Crossgrove et al., 2003).
Another mechanism regulating Mn homeostasis in the brain involves Mn being transferred with high affinity into cells by the Zinc transporters ZIP-8 and ZIP-14, which are Zrt-/Irt-related protein (ZIP) family metal transporters encoded by SLC39A8 and SLC39A14, respectively. These transporters are highly expressed in the liver, duodenum, kidney, and testis, and are localized on apical surfaces of brain capillaries (Girijashanker et al., 2008; Wang et al., 2012). Taking advantage of its particular magnetic properties, Aoki et al. (2004) employed magnetic resonance imaging (MRI) to show that Mn uptake also occurs through the choroid plexus. One day after they systemically administered Mn2 + to rats, the distribution of Mn in the brain extended to the olfactory bulb, cortex, basal forebrain, and basal ganglia, overlapping specific brain structures vulnerable to Mn-induced neurotoxicity (Aoki et al., 2004). In cells such as neurons and astrocytes, toxic accumulations of Mn are found primarily in the mitochondria, heterochromatin, and nucleoli (Lai et al., 1999; Morello et al., 2008).
Mn also shares the Ca2+ uniporter mechanism and the rapid mode (RaM) of Ca2+ uptake of mitochondrial calcium influx, resulting in Mn sequestration in mitochondria, which gets removed only very slowly from the brain (Gavin et al., 1990). This Mn accumulation inhibits the efflux of calcium, decreases MAO activity, and inhibits the respiratory chain and ATP production (Zhang et al., 2003), which may partly explain the role of mitochondrial dysfunction in Mn neurotoxicity. Previously, Mn detoxification and efflux from cells was thought to be primarily regulated by ferroportin (Fpn), also known as HFE4, MTP1, and IREG1, which are proteins encoded by the SLC40A1 gene. Although Fpn was initially identified as the iron exporter, more recent findings suggest that Fpn also interacts with Mn, zinc, and cobalt to export them from the cell (Troadec et al., 2010; Yin et al., 2010; Madejczyk and Ballatori, 2012). Furthermore, Mn exposure increases Fpn mRNA levels in mouse bone marrow macrophages (Troadec et al., 2010) and it significantly increases Fpn protein levels in HEK293T cells (Yin et al., 2010). Increasing Fpn levels were linked to reduced Mn accumulation in both the cerebellum and cortex of mice treated with Mn (Yin et al., 2010), further confirming that Fpn removes Mn and reduces Mn-induced neurotoxicity.
Recently, the secretory pathway of the Ca2+/Mn2+ ATPases SPCA1 and SPCA2, which are localized at the Golgi, was suggested as an alternative way of cytosolic Mn detoxification by sequestering into the Golgi lumen (Sepulveda et al., 2009). Overexpressing SPCA1 in HEK293T cells conferred tolerance of manganese (Mn2+) toxicity by facilitating Mn2+ accumulation in the Golgi, thereby increasing cell viability (Leitch et al., 2011). However, the degree to which SPCA1 and SPCA2 regulate Mn homeostasis has yet to be determined. Another mode for Mn egress through Golgi has been attributed to SLC30A10 in humans (Tuschl et al., 2012). Recently, SLC30A10 was shown to be localized on the cell surface where it acted as a Mn efflux transporter to reduce cellular Mn levels and protect against Mn-induced toxicity (Leyva-Illades et al., 2014). Mutations in the SLC30A10 gene have been associated with hepatic cirrhosis, dystonia, polycythemia, Parkinsonian-like gait disturbances, and hypermanganesemia in cases unrelated to environmental Mn exposure (Tuschl et al., 2012). A genome-wide association study mapping genes involved in regulating Mn homeostasis mapped serum Mn levels to SLC30A10 (Ng et al., 2015). Along with its expression in the liver and CNS, SLC30A10 is also expressed in the GIT. Interestingly, this transporter is present mainly on the apical surface of enterocytes that line the GIT and presumably help transport Mn to the lumen. In fact, it is the liver and GIT that are primarily responsible for maintaining Mn homeostasis in the body as indicated by whole body as well as endoderm-specific SLC30A10 knockouts (KOs) resulting in hypermanganesemia, while pan neuronal/glial SLC30A10 KOs produce normal levels of Mn in the CNS (Taylor et al., 2019). The authors also found that a lack of SLC30A10 in the CNS led to an increased accumulation of Mn in the basal ganglia and thalamus when these mice were exposed to elevated Mn levels. Importantly, these recent discoveries involving SLC30A10 and its mutations reinforce its crucial role in humans as a Mn transporter, broadening our understanding of familial Parkinsonism as a result of SLC30A10 mutations.
The p-type transmembrane ATPase protein ATP13A2 (or PARK9) located at the lysosome also protects cells from Mn-induced toxicity (Tan et al., 2011). Although ATP13A2’s function in mammalian cells remains elusive, loss-of-function mutations in ATP13A2 cause Kufor-Rakeb Syndrome (KRS), an autosomal recessive form of early-onset Parkinsonism with pyramidal degeneration and dementia (Ramirez et al., 2006). Overexpression of wild-type (WT) ATP13A2, but not KRS pathogenic ATP13A2 mutants, protects mammalian cell lines and primary rat neuronal cultures from Mn2+-induced cell death by reducing intracellular Mn concentrations and cytochrome c release, suggesting a role of ATP13A2 in Mn detoxification and homeostasis (Tan et al., 2011). A summary of the above-mentioned receptors and channels involved in cellular Mn homeostasis appears in Figure 1.
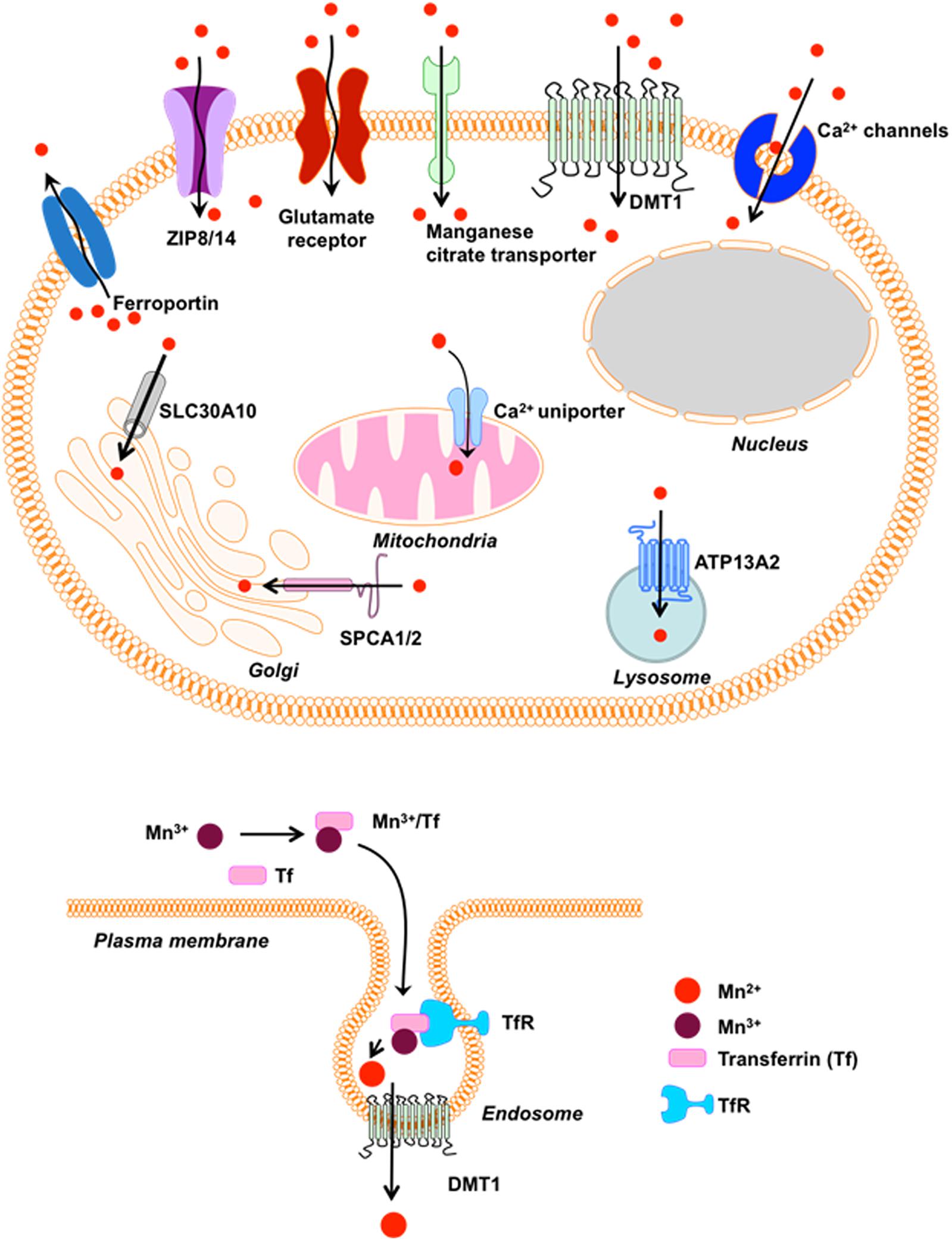
Figure 1. Receptors/Channels involved in Mn homeostasis. Various cellular receptors such as divalent metal transporter 1 (DMT1) and transferrin receptor (TfR), as well as ion channels, store-operated Ca+2 channels (SOCC) or voltage-gated Ca+2 channels (VGCC) that facilitate the entry of divalent Mn into cells, whereas SLC40A (ferroportin) and Ca+2 facilitate its expulsion from cells and mitochondria, respectively. Mn+2 is passively transported via VGCC and glutamate-activated ion channels while Mn+3 entry is facilitated via transferrin.
Manganese and α-Synuclein Protein Misfolding
Belonging to a family that includes β- and γ-synuclein, α-synuclein (αSyn) is a small 140-AA, highly conserved vertebrate protein encoded by a single 7-exon gene located on chromosome 4. It is predominantly a neuronal protein expressed in presynaptic terminals throughout the mammalian brain and CSF where it is estimated to account for as much as 1% of total protein in soluble cytosolic brain fractions. Functionally, αSyn remains poorly understood, but emerging evidence points to roles in membrane trafficking, dopamine regulation, and synaptic plasticity. The link between αSyn and PD pathogenesis is based on case studies of familial and sporadic PD patients presenting with misfolded αSyn-rich Lewy pathology during autopsy (Poulopoulos et al., 2012). Also, compelling evidence demonstrates that mutations in the gene encoding αSyn are directly linked to the onset of PD (Liu et al., 2012). Furthermore, rare familial forms of PD also have been linked to the overexpression of αSyn due to SNCA gene duplication and triplication.
The aggregation and fibrillation of αSyn, forming intracellular proteinaceous aggregates, have been implicated in several other neurodegenerative disorders besides PD, including LBD, Lewy body variant of AD, MSA, and Hallervorden–Spatz disease. The idea that extracellular αSyn species can accelerate the spread of PD pathology throughout the brain gained much consideration with the findings of host-to-graft propagation of αSyn-positive Lewy pathology in fetal ventral mesencephalic and embryonic nigral neurons transplanted in human PD patients (Kordower et al., 2008; Li et al., 2008) and misfolded αSyn species in human CSF and plasma (El-Agnaf et al., 2003; Kordower et al., 2008). Although multiple studies have hypothesized the intercellular transmission of pathological αSyn species in PD (Lee et al., 2008; Desplats et al., 2009; Dunning et al., 2013), its exact mechanistic role in disease pathogenesis and related synucleinopathies largely remains unknown. Available in vitro evidence thus far postulates that extracellular αSyn induces pathogenic actions by multiple mechanisms including, but not limited to, the triggering of neuroinflammatory responses and mitochondrial dysfunction leading to neurodegenerative processes (Su et al., 2008; Emmanouilidou et al., 2010).
As a member of the family of intrinsically unstructured proteins, αSyn is natively unfolded and lacks a defined secondary protein structure. However, upon interaction with lipid membranes, it adopts an α-helical conformational change, and under conditions that trigger aggregation, αSyn undertakes the characteristic crossed β-conformation and self-aggregates into soluble oligomers, which gradually form insoluble amyloid-like fibrils. The αSyn protein comprises three main structural domains (Figure 2): (1) an N-terminal amphipathic region, (2) an amyloid-binding central domain (NAC), and (3) a C-terminal acidic tail. The N-terminus (residues 1-60) contains four series of 11-AA repeats containing the highly conserved consensus sequence KTKEGV, which also is important for α-helix conformation upon binding to phospholipid membranes. The core NAC region (residues 61–91) is important in protein aggregation and it also contains two additional KTKEGV repeats. Within the NAC, a hydrophobic GAV peptide motif (residues 66–74), consisting of Ala, Val, and Gly AA residues, has been identified as the required core for human αSyn protein fibrillization and cytotoxicity (Du et al., 2006). Finally, the proline-rich C-terminus (residues 91–140) is highly acidic and accounts for the intrinsically disordered properties of αSyn (Harischandra et al., 2015a). The N-terminal and NAC regions form αSyn’s membrane binding domain, whereas the C-terminal region is believed to contain protein–protein and protein–small molecule interaction sites.
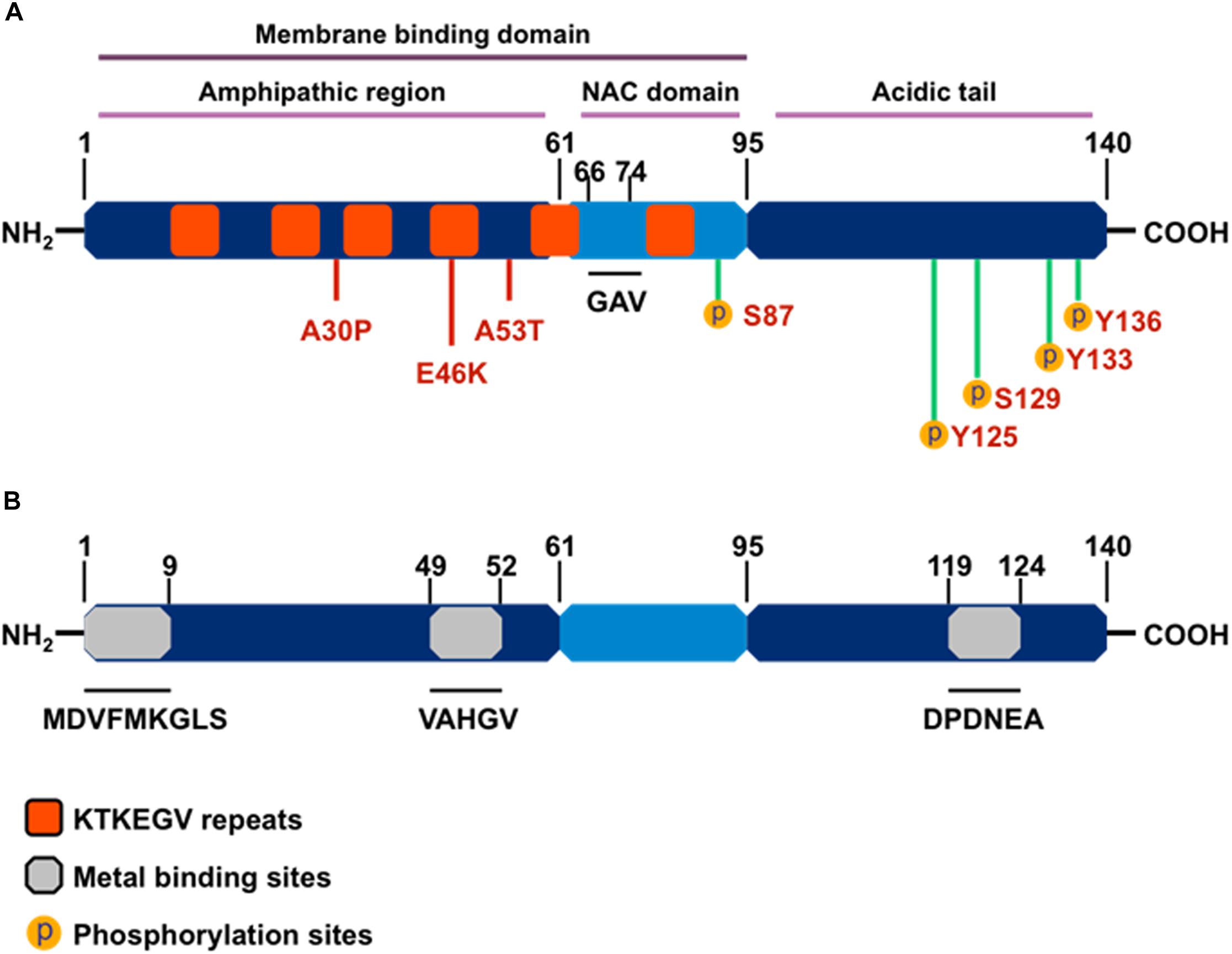
Figure 2. The structure of α-synuclein. (A) Orange boxes denote the characteristic KTKEGV repeat and red lines denote amino acid mutations seen in familial PD patients. The central hydrophobic core (middle light blue), or NAC domain, promotes α-synuclein aggregation. The C-terminal domain (right dark blue) is the acidic tail and contains most known phosphorylation sites. (B) Gray boxes denote known metal-binding sites in the α-synuclein structure.
Importantly, αSyn wields its metalloprotein properties through its three metal-binding sites: two each at the N-terminus and one at the C-terminus. A systematic analysis of mono-, di-, and trivalent metal ligands (Li+, K+, Na+, Cs+, Ca2+, Co2+, Cd2+, Cu2+, Fe2+, Mg2+, Mn2+, Zn2+, Co3+, Al3+, and Fe3+) revealed that metal binding induces conformational changes that cause normally benign αSyn protein to aggregate (Uversky et al., 2001). Of the 15 metal cations studied, Uversky et al. (2001) determined Al3+ to be the most effective stimulator of protein fibril formation followed by Cu2+, Fe2+, Co3+, and Mn2+, with each causing conformational changes detectable by intrinsic protein fluorescence and far UV-circular dichroism. Furthermore, Uversky’s team also showed that Mn3+ induced immediate di-tyrosine formation, suggesting that Mn is responsible for the metal-induced oxidation of αSyn. Among the three metal-binding sites, those located at the N-terminus, specifically the 1MDVFMKGLS9 and 48VAHGV52 regions, demonstrated high-affinity binding for Cu2+ (Kd ∼ 0.1 μM) (Rasia et al., 2005), whereas metal-interaction sites near residues 49–52 and residues 110–140 are known to bind with divalent metals like Mn (Uversky et al., 2001; Binolfi et al., 2006, 2008). In a detailed study, the metal ions Mn2+, Fe2+, Co2+, and Ni2+ bound preferentially to the 119DPDNEA124 motif, in which Asp121 acted as the main anchoring site with low affinity (mM) to metal ligands (Binolfi et al., 2006). These discoveries on the structural components of αSyn’s interaction with metals strengthen the link between metal neurotoxicity and PD, further suggesting that metal dyshomeostasis plays an even more important role in the development of neurodegenerative disorders than previously acknowledged (Binolfi et al., 2006).
Our in vitro studies show that physiological levels of human WT αSyn attenuate acute Mn-induced dopaminergic neuronal degeneration. However, this neuroprotective effect is diminished by chronic exposure to Mn toxicity, which accelerates αSyn misfolding (Harischandra et al., 2015a). Furthermore, using a genetically modified Caenorhabditis elegans model system, Bornhorst et al. (2014) reported enhanced Mn accumulation and oxidative stress in pdr1 and djr1.1 mutants, which were reduced by αSyn expression. This protective role of αSyn in Mn-induced neurotoxicity was further validated using αSyn transgenic animals (Yan et al., 2019). By treating αSyn KO (αSyn–/–) and WT (αSyn+/+) mice with different Mn concentrations, this study demonstrated that the presence of αSyn ameliorates high-dose Mn-induced neurotoxicity. Taken together, these findings point to a novel, neuroprotective role of WT αSyn in attenuating acute Mn toxicity, an effect which may stem directly from its metal-binding capability (Figure 3).
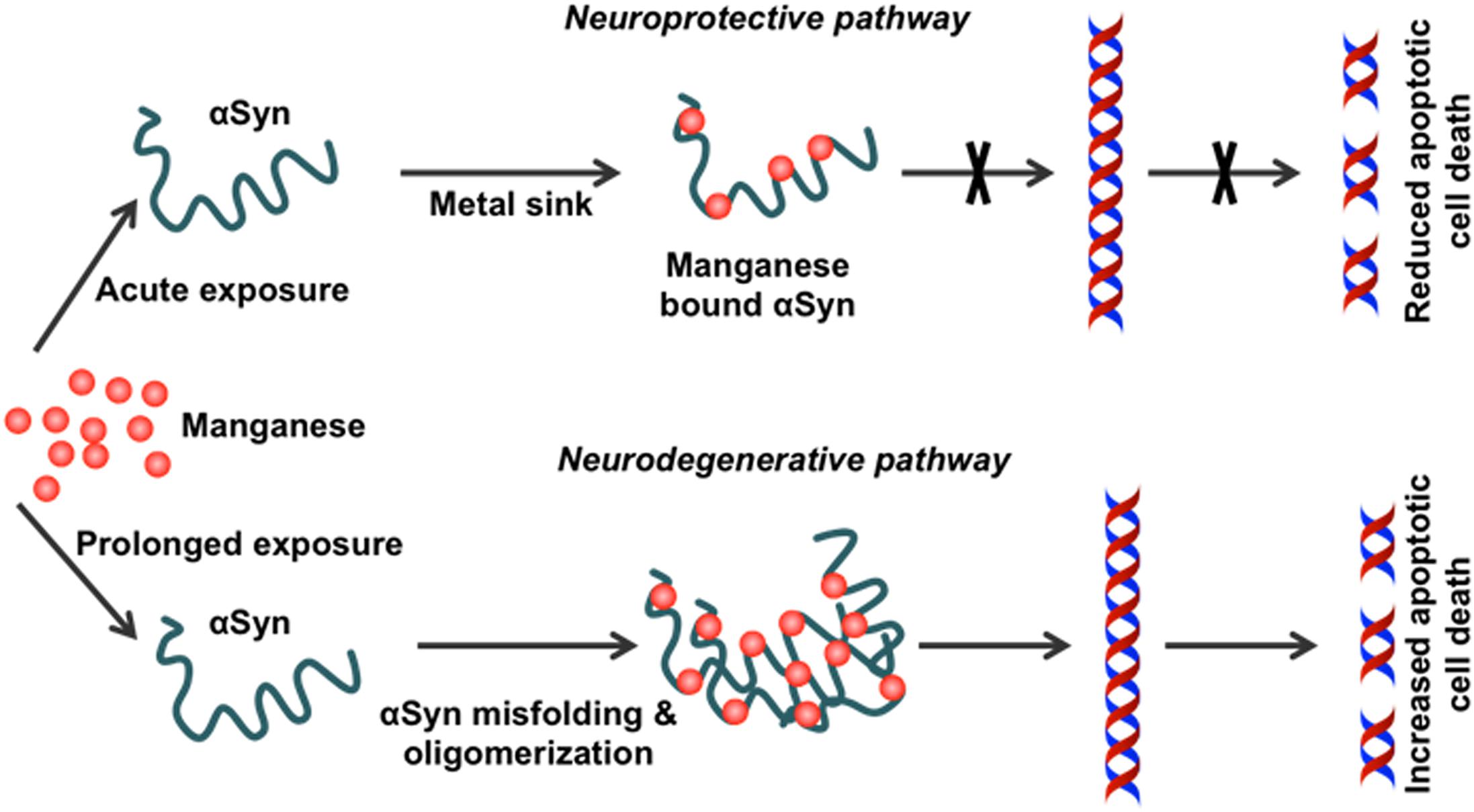
Figure 3. Effect of chronic Mn overexposure on α-synuclein misfolding. Metal-binding sites on α-synuclein (αSyn) allow it to become a metal sink for free-roaming metals in cells. During an acute exposure to Mn, free-roaming Mn binds to the metal-binding sites on the C-terminus of this protein. In this way, αSyn effectively works as a chelator for different metal ions, including Mn. However, continued exposure to Mn can saturate this chelating property. Once saturation occurs following additional binding of Mn to the C-terminus, the natively conformed protein misfolds. Misfolded αSyn leads to the production of pro-inflammatory factors. Thus, Mn overexposure leads to progressive protein misfolding in the neurons and induces inflammation and finally neurodegeneration.
Although the physiological role of αSyn with respect to Mn toxicity still needs to be fully validated, the effects of Mn on αSyn expression, aggregation, and subsequent cytotoxicity have been studied in in vitro, in vivo, and ex vivo models of PD (Gitler et al., 2009; Verina et al., 2013; Xu et al., 2013). Studies conducted with neuronal cell culture models show that Mn treatment upregulates cellular αSyn levels and leads to αSyn aggregation (Cai et al., 2010). In contrast, knocking down αSyn using antisense αSyn treatment (Li et al., 2010) or siRNA (Cai et al., 2010) can reverse Mn-induced cytotoxicity. In parallel studies, overexpressing αSyn in rat mesencephalic cells (MES 23.5) not only enhanced their susceptibility to Mn exposure (Prabhakaran et al., 2011), but also attenuated Mn release from Mn-treated cells without significantly attenuating the major Mn transporter proteins DMT1, VGCC, and Fpn1 (Ducic et al., 2015). Thus, these studies further suggest that αSyn’s metal-binding capacity serves as an intracellular Mn store that helps to regulate free-roaming Mn cations.
Manganese and Endosomal Trafficking
Accumulating evidence indicates that secretion and cell-to-cell trafficking of pathological forms of αSyn may explain the typical progression of PD. In particular, vesicular trafficking has attracted considerable attention as an initiator or enhancer of the neurodegenerative process underlying PD. Dysfunction of the cellular trafficking pathway can compromise synaptic function and lead to the accumulation of misfolded αSyn. Similarly, changes in endosomal sorting and degradation greatly influence the intracellular trafficking of misfolded proteins, thereby enabling the cell-to-cell transmission of toxic αSyn species in a prion-like manner. Recent genetic studies also suggest that defects of endolysosomal function could disrupt αSyn homeostasis and mitochondrial function, causing neurotoxicity through unknown mechanisms (Kett and Dauer, 2016). Indeed, several PD-linked gene mutations or polymorphisms (DNAJC13/RME-8, VPS35, ATP13A2, ATP6AP2, RAB7L1, GBA, GAK, LRRK2) interrupt protein trafficking and degradation via the endosomal pathway (Perrett et al., 2015), highlighting the importance of the endosomal pathway in the progression of neurodegenerative disease.
It has been shown that αSyn overexpression blocks endoplasmic reticulum (ER)-to-Golgi vesicular trafficking (Cooper et al., 2006) and that αSyn is functionally associated with endocytic vesicular trafficking, retromer complex proteins, phosphatases, and Rab GTPases (Breda et al., 2015; Chung et al., 2017). In this regard, recent attempts to identify molecular regulators of αSyn oligomerization have identified several Rab proteins, including Rab8b, Rab11a, Rab13, Slp5 (Goncalves et al., 2016), and Rab1, which (Cooper et al., 2006) promote the clearance of αSyn inclusions and attenuate αSyn-induced toxicity. Furthermore, Rab11a and Rab13 expression enhanced the endocytic recycling and secretion in cells accumulating αSyn inclusions (Goncalves et al., 2016). In contrast, Rab11 regulates the recycling of extracellular αSyn (Liu et al., 2009) and modulates αSyn-mediated defects in synaptic transmission and locomotor behavior in experimental PD models (Breda et al., 2015). This is particularly interesting as Rab11 has been identified as a major regulator of endosomal recycling (Grant and Donaldson, 2009) and controls the secretion of smaller αSyn oligomers by exosomes (Poehler et al., 2014).
Exosomes are nano-sized vesicles (50–150 nm) that are released from cells into the extracellular space (Thery et al., 2002). Exosomes circulate throughout the body and readily cross the blood–brain and other barriers. Great interest in exosomes is emerging because of their potential role in disease progression as well as their possible use in early biomarker discovery (Sarko and McKinney, 2017) and drug delivery (Luan et al., 2017). Toxicology researchers are building upon the discovery that environmental toxicants change the exosome signature of human health conditions such as cancer and neurodegenerative diseases (Harischandra et al., 2017; Munson et al., 2018; Ngalame et al., 2018). In this regard, the impact that Mn exposure has on the neuronal exosome signature and its subsequent effect on neuroinflammation and neurodegeneration have been studied in great detail in our laboratory (Harischandra et al., 2015a, b, 2017, 2018, 2019). We have shown that Mn exposure significantly upregulates the small GTPase Rab27a, which mediates the membrane fusion of multivesicular bodies (Pfeffer, 2010) that subsequently release exosomes into the extracellular environment (Harischandra et al., 2018). Furthermore, our miRNA profiling analysis of Mn-induced neuronal exosomes indicates increased expression of certain miRNAs (e.g., miR-210, miR-325, miR-125b, miR-450b) known to control key biological mechanisms, including inflammation, autophagy, protein aggregation, and hypoxia (Harischandra et al., 2018). In subsequent studies, we show how Mn exposure promotes the exosomal secretion of aggregated αSyn into the extracellular medium. These exosomes were endocytosed through caveolae-mediated endocytosis, thereby inducing neuroinflammation that subsequently evoked neurodegenerative processes in both cell culture and animal models (Harischandra et al., 2019). Interestingly, serum exosome samples collected from welders chronically exposed to Mn-containing welding fumes show increased misfolded αSyn in their exosomes, further implicating environmental Mn exposure in developing Parkinsonism (Harischandra et al., 2019). In parallel studies, we revealed Mn’s role in inflammasome activation in microglial cells. We found that Mn acts as signal 2 for NLRP3 inflammasome activation in LPS-primed microglial cells, triggering the exosomal release of ACS “prionoids,” resulting in inflammasome propagation (Sarkar et al., 2019). Together, our results highlight Mn’s role in modulating endosomal trafficking through the exosomal release of cargo capable of triggering neuroinflammation and progressive neurodegeneration.
Manganese and Neuroinflammation
In addition to the importance of oxidative stress in the Mn-induced dysfunction of dopaminergic neurons, glial cell activation also plays an important role in potentiating Mn neurotoxicity by inducing the release of non-neuronal-derived ROS and inflammatory mediators such as proinflammatory cytokines. The state of glial activation is defined by its morphology and by the proliferation, migration and expression of immune modulatory molecules. The two major types of glial cells in the CNS are astrocytes and microglia, with the latter constituting about 10% of all glial cells in the CNS.
It is now well documented that glial activation is prominent in the brains of humans exposed to Mn, as well as in non-human primate and rodent models of Mn neurotoxicity (Erikson and Aschner, 2006; Huang, 2007; Perl and Olanow, 2007; Cordova et al., 2013). Neuroinflammation is regarded as a key mediator in mechanisms underlying the loss of dopaminergic neurons in PD. The activation of microglia plays a major role in the response to environmental stress and immunological challenges by scavenging excess neurotoxins, removing dying cells and cellular debris, and releasing proinflammatory cytokines (Carson et al., 2007; Tansey et al., 2008). Inducible nitric oxide synthase (iNOS), which produces large amounts of nitric oxide (NO), is released by microglia in response to inflammatory mediators such as LPS and cytokines. The levels of NO are reported to be elevated in the CNS of human PD cases and in animal models of PD (Mogi et al., 1994). Consistent with this finding, iNOS KO animals are resistant to MPTP-induced dopaminergic neuronal loss in the SN (Przedborski and Vila, 2003). The transcription factor NF-κB, required for transcribing proinflammatory molecules, is also activated in the SN of PD patients and MPTP-treated mice (Ghosh et al., 2007). In contrast to microglia, astrocytes do not attack any pathological targets, but instead produce factors that mediate inflammatory reactions seen in the SN of PD brains (Miklossy et al., 2006). Activated astroglial cells were recently found in human PD brains and in the MPTP mouse model of PD (Ghosh et al., 2007; Ghosh et al., 2009).
Astrocytes play a major role in Mn-induced neuroinflammation as they represent a “hub” for brain Mn homeostasis (Wedler and Denman, 1984). The transferrin receptors found on astrocytes readily bind to Tf-Mn3+, so it is not surprising to find more Mn in astrocytes than in any other neural cell types. Indeed, astrocytes can exhibit Mn concentrations 10- to 50-fold greater than those measured in neurons, making them more susceptible to Mn toxicity than other cell types. During glutamate-induced excitotoxicity, excess glutamate abruptly increases intracellular Ca2+ to levels that block Mn2+ uptake, prompting a release of mitochondrial Mn2+ into the cytosol. High levels of cytosolic Mn2+ in astrocytes activate glutamine synthetase, which removes excess glutamate (Wedler et al., 1994). However, excessive extracellular Mn2+ can disrupt intracellular Ca2+ signaling in astrocytes by competitively occupying Ca2+-binding sites, thus interfering with mitochondrial Ca2+ homeostasis (Farina et al., 2013), which triggers astrogliosis. In addition, Mn3+ causes astrocyte swelling via oxidative/nitrosative pathways (Rama Rao et al., 2007). Increased Mn levels in astrocytes elevate the expression of proinflammatory signals such as iNOS and IL-6 (Moreno et al., 2008). In vitro studies show that Mn-treated astrocytes use larger amounts of L-arginine, which is a substrate for NO (Hazell and Norenberg, 1998). While timely expression of these signals is necessary in response to neuronal stress or cellular damage, excessive production is counter-productive, often exacerbating the toxic insult. Microarray gene expression profiling of primary human astrocytes exposed to Mn reveals an upregulation of genes encoding proinflammatory cytokines with a concurrent downregulation of genes involved in cell cycle regulation and DNA replication and repair (Sengupta et al., 2007).
The glutamate-GABA cycle (GGC) is important especially in the context of astrocyte-neuron metabolism. The AA glutamine is a precursor for the production of both glutamate and GABA (Bak et al., 2006). Deamidation of neuronal glutamine to glutamate produces ammonia, which is then transferred to astrocytes and utilized in the amidation of glutamate. Glutamine released by astrocytes is taken up by glutamatergic and GABAergic neurons that incidentally show projections in the basal ganglia and help regulate voluntary movements (Sidoryk-Wegrzynowicz and Aschner, 2013). However, in response to excessive Mn in the brain, Mn rapidly enters astrocytic mitochondria. As mentioned in the previous section, high levels of mitochondrial Mn impair cellular respiration and prevent the production and activation of glutathione peroxidase (GPx). Taken together, astrocytes appear to be particularly affected by a disruption of Mn homeostasis in the brain. This in turn could negatively affect GABAergic and glutamatergic projections in the basal ganglia, leading to the motor deficits characterizing Mn neurotoxicity.
Manganese in Oxidative Stress and Neurodegeneration
Although the mechanisms of Mn-induced nigrostriatal cell death are not well characterized, Mn neurotoxicity appears to be regulated by multiple factors, including oxidative injury, mitochondrial dysfunction, protein misfolding, and neuroinflammation.
Mn is a redox-active metal whose high reduction potential aids the removal of harmful byproducts of oxygen metabolism, such as superoxide (O2.–) and hydrogen peroxide (H2O2), when as a cofactor it forms manganese superoxide dismutase (MnSOD). However, when allowed to accumulate, Mn exacerbates oxidative damage. At just 2% of body weight while consuming 20% of the total oxygen and calories, the brain is highly metabolically active and hence highly susceptible to oxidative damage. Since Mn is known to accumulate in the globus pallidus and striatum, these regions are especially vulnerable to oxidative injury because of their intense oxygen consumption, significant dopamine content, and their high content of non-heme iron. A recent study evaluating the effect of Mn on dopamine transporter (DAT)-transfected and non-transfected HEK cells shows that Mn prevents dopamine reuptake in transfected cells and also mobilizes DAT receptors from the cell surface to intracellular compartments. Consequently, dopamine-induced cell toxicity is observed (Roth et al., 2013). Our laboratory systematically characterized the cell signaling mechanisms underlying Mn-induced oxidative stress. We showed that Mn treatment in rat-derived mesencephalic dopaminergic neuronal (N27) cells increases reactive oxygen species (ROS) production (Harischandra et al., 2015a) that can sequentially activate proapoptotic processes like mitochondrial cytochrome c release, caspase-3 activation, and DNA fragmentation. This mitochondria-dependent apoptotic cascade did not involve caspase-8 activation, but was triggered by the Mn treatment (Figure 4) (Latchoumycandane et al., 2005). Moreover, the redox-sensitive protein kinase C delta (PKCδ), involved in neurodegenerative disorders such as AD, prion disease, and PD (Kanthasamy et al., 2006; Ciccocioppo et al., 2008; Jin et al., 2011; Harischandra et al., 2014), is reported to be a key mediator in Mn-induced apoptosis (Anantharam et al., 2002; Latchoumycandane et al., 2005). Later studies in differentiated N27 cells also demonstrate that chronic low-dose Mn exposure impairs tyrosine hydroxylase (TH), the rate-limiting enzyme in dopamine synthesis, through activation of PKCδ and protein phosphatase-2A (PP2A) activity (Zhang et al., 2011). Notably, in vitro and in vivo administration of the hydrophilic antioxidant vitamin E analog trolox (6-hydroxy-2,5,7,8-tetramethylchroman-2-carboxylic acid) reverses Mn-induced neurotoxicity and rescues dysfunctional dopaminergic transmission and Mn-induced motor coordination deficits (Milatovic et al., 2011; Cordova et al., 2013), further emphasizing the relationship between oxidative stress and Mn-related neurodegeneration.
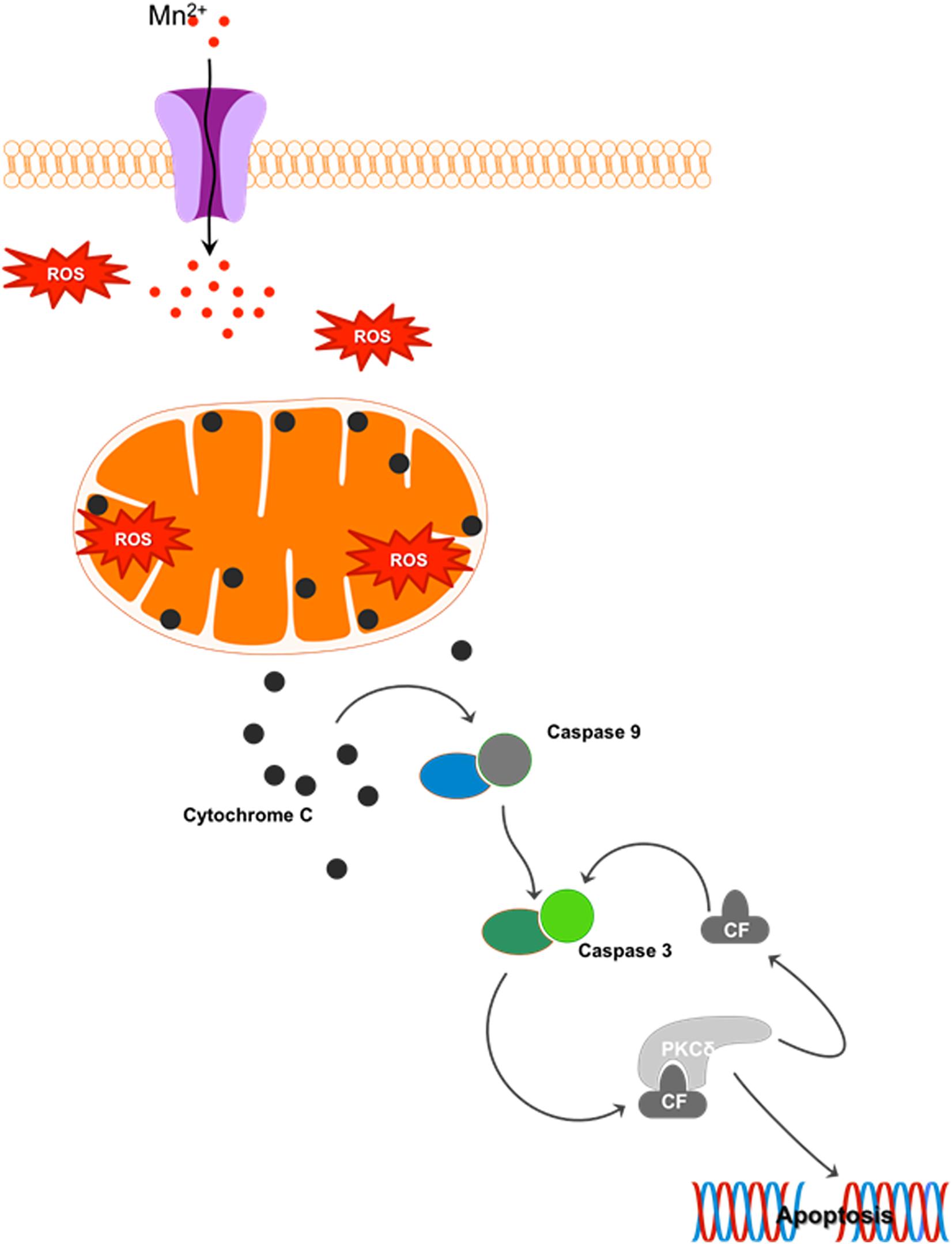
Figure 4. Mn-induced cell death: Cellular Mn homeostasis is dependent upon efficient uptake, retention and excretion by various cell receptors and/or ion channels. In the presence of excess Mn, homeostatic mechanisms will down-regulate the receptors involved in the uptake of this metal, while up-regulating those involved in its release from the cell. However, during chronic exposure to high concentrations of Mn, these system checks are not maintained. Continued uptake of Mn under these conditions increases the production of reactive oxygen species (ROS), leading to mitochondrial dysfunction. Impaired mitochondrial function leads to the release of cytochrome c, activating the apoptosis initiator caspase-9, which in turn cleaves caspase-3. The cleaved fragment of caspase-3 interacts with protein kinase C delta (PKCδ), a pro-apoptotic protein. Caspase-3-mediated proteolytic cleavage of PKCδ leads to DNA fragmentation and apoptosis.
The neurotransmitter dopamine belongs to the catecholamine and phenethylamine families. The chemical structure of catecholamines predisposes them to oxidation, and their well-characterized metabolic routes can yield quinones and free radicals, suggesting that dopamine may also serve as a neurotoxin contributing to the neurodegenerative process through oxidative metabolism. By promoting dopamine auto-oxidation, Mn potentiates dopamine toxicity in high Mn-accumulating areas of the brain (e.g., globus pallidus and striatum). Under homeostatic conditions, monoamine oxidases (MAO) enzymatically oxidize dopamine to produce dihydroxyphenylacetic acid (DOPAC), which catechol-O-methyltransferase (COMT) methylates to homovanillic acid (HVA). Alternatively, COMT can convert dopamine to 3-methoxytyramine (3-MT), which MAO then oxidizes to HVA. H2O2 is another byproduct of this dopamine turnover or deamination, generating inherent oxidative stress conditions in the nigrostriatal system. Dopamine can also be non-enzymatically oxidized by molecular oxygen, yielding H2O2 and quinones. These quinones also undergo intramolecular cyclization and oxidative reactions to produce neuromelanin (Graham, 1978; Hermida-Ameijeiras et al., 2004). In dopaminergic SN neurons, neuromelanin augments dopamine’s vulnerability to auto-oxidation through quinone modification (Graham, 1978). Therefore, the degradation of dopamine, either enzymatically or non-enzymatically, produces H2O2. Two prominent Mn valence states, Mn2+ and Mn3+, are found in biological systems. In the presence of high levels of divalent Mn2+, H2O2 can convert to highly toxic hydroxyl radicals (OH) via the Fenton reaction. But because of its higher oxidative state, Ali et al. (1995) found Mn3+ to be an order of magnitude more cytotoxic than Mn2+ in Mn-dosed rats. In fact, Mn3+-induced dopamine oxidation, generating quinones and H2O2, appears to be independent of oxygen and far more rapid than that mediated by Mn2+ (Archibald and Tyree, 1987). Since Mn2+ can readily oxidize to Mn3+ in the human brain via superoxides, the auto-oxidation of catecholamines can only further potentiate oxidative stress.
Impairment of the cellular antioxidant machinery, causing an imbalance between ROS generation and its elimination, plays a major role in the development of certain neurodegenerative processes. The antioxidant glutathione (GSH), present in both neurons and astrocytes, provides the first line of cellular defense against ROS. GSH actively disposes of exogenous peroxides by acting as a co-substrate in reactions catalyzed by GPx, thus playing important functional roles in the CNS. Altered striatal concentrations of GSH, glutathione disulfide (GSSG), ascorbic acid, malondialdehyde (MDA), and the activities of glutathione reductase (GR) and GPx have been previously reported with Mn neurotoxicity, suggesting that an impaired neuronal antioxidant system renders the brain susceptible to Mn-induced neurotoxicity (Chen and Liao, 2002; Dukhande et al., 2006; Maddirala et al., 2015). Moreover, inhibiting GSH synthesis potentiates the Mn-induced increase in inosine, hypoxanthine, xanthine, and uric acid levels in the striatum and brainstem of aged rats (Desole et al., 2000), indicating that Mn-induced cytotoxicity is mediated through mitochondrial dysfunction. Therefore, the specific vulnerability of dopamine neurons to Mn plays a pivotal role in impairing cellular antioxidant defenses, wherein breakdown of the mitochondrial oxidative energy metabolism cascade leads to dopaminergic cell death. Excess ROS fuels the oxidation of membrane polyunsaturated fatty acids (PUFA), yielding numerous arachidonic acid (ARA) peroxidation products, including reactive aldehydes such as 4-hydroxy-trans-2-nonenal (4-HNE), 4-oxo-trans-2-nonenal (4-ONE), MDA, acrolein, F2-isoprostanes (F2-IsoPs), and isofurans (Esterbauer et al., 1991; Aluru et al., 2015). The lipid ARA had been released from neural membrane glycerophospholipids through the activation of cytosolic phospholipases A2 (cPLA2), which are enzymes coupled to NMDA receptors (Farooqui and Horrocks, 2007; Farooqui and Farooqui, 2011). Since most biological membranes of cells and organelles are composed of PUFA, lipid peroxidation is the main molecular mechanism involved in the oxidative damage to cell structures and in toxicity-mediated cell death. Consistent with these observations, primary rat cortical neurons exposed to a very high Mn dose (500 μM) for 6 h show structural damage to neurons and a roughly 50% increase in F2-IsoPs levels compared to controls (Milatovic and Aschner, 2009). Likewise, in primary astrocyte cultures exposed to the same experimental conditions, F2-IsoPs levels increased 51% compared to control cultures (Milatovic et al., 2007). However, the direct role of Mn in CNS toxicity associated with lipid peroxidation remains debatable as some investigators argue that in vivo administration of Mn alters cellular Ferrous (Fe2+), which plays a permissive role in increasing lipid peroxidation and augmenting neuronal vulnerability (Shukla and Chandra, 1981; Chen et al., 2000, 2006).
Moreover, dopamine-derived quinones are known to bind and modify several PD-related proteins such as αSyn, DJ-1, and parkin (Conway et al., 2001; LaVoie et al., 2005; Girotto et al., 2012). However, of all the cellular macromolecules prone to oxidative damage, damaged nucleic acids are particularly hazardous due to the elevated risk of potentially irreparable genetic base mutations. Among the five nucleobases, guanine is the most susceptible to hydroxyl radical-mediated oxidation (Cooke et al., 2003; Cerchiaro et al., 2009), which produces the well-studied oxidized DNA product 8-hydroxyguanosine (8-OHG). Interestingly, elevated 8-OHG as well as reduced 8-hydroxyl-2-deoxyguanosine (8-OHdG) have been observed in the SN and cerebrospinal fluid (CSF) of PD patients (Zhang et al., 1999; Isobe et al., 2010). In contrast, in vitro studies of Mn toxicity reported increased 8-oxo-7,8-dihydro-2′-deoxyguanosine (8-oxodG) content in the DNA of dopamine-treated PC12 cells (Oikawa et al., 2006). Stephenson et al. (2013) have also shown that Mn catalyzes the auto-oxidation of catecholamines in SH-SY5Y cells with the ensuing oxidative damage to thymine and guanine DNA bases, further indicating the damaging effect of Mn-induced semi-quinone radical ions and ROS production on DNA.
Mn preferentially accumulates in mitochondria, through the mitochondrial Ca2+ uniporter, where it is mainly bound to mitochondrial membrane or matrix proteins (Gavin et al., 1999). Succinate, malate, and glutamate are important substrates for mitochondrial respiration, but at high concentrations, Mn2+ binds to these substrates effectively inhibiting mitochondrial respiration (Gavin et al., 1999). Interference in oxidative phosphorylation triggers the downstream release of inflammatory signals, leading ultimately to apoptosis. Recent evidence sheds light on Mn-induced ER stress and ER-mediated cellular apoptosis. Rats given three different doses of Mn for 4 weeks showed a dose-dependent increase in apoptotic cells in the striatum, as evidenced by chromatin condensation, as well as up-regulation of markers of mitochondrial and ER stress-mediated apoptosis (Wang et al., 2015). Furthermore, Mn induces the transcriptional and translational up-regulation of αSyn (Li et al., 2010), promoting susceptibility to Mn-induced neurotoxicity through ERK1/2 MAPK activation, NF-κB nuclear translocation, and activation of apoptotic signaling cascades leading to dopaminergic cell death (Li et al., 2010; Prabhakaran et al., 2011).
Mn affects not only cellular viability, but also various factors involved in neurotransmitter regulation. Acetylcholine esterase (AChE) is an enzyme that hydrolyses acetylcholine (ACh), thus regulating its availability in the synaptic cleft (Whittaker, 1990; Pohanka, 2012). Chronic exposure to high levels of Mn can inhibit the activity of AChE, thereby allowing ACh to accumulate in the synaptic cleft and subsequently overstimulating muscarinic and nicotinic ACh receptors. While the precise mechanism has not been determined, inhibiting AChE increases levels of ROS and RNS (Milatovic et al., 2006; Santos et al., 2012), which further leads to lipid peroxidation as well as production of citrulline, a marker of RNS activity. Ali et al. (1983) reported that Mn overexposure in rats on a low-protein diet reduces the level of GABA in the brain while increasing the animals’ susceptibility to seizures. However, the effect depended on the treatment regime and age of rats. For instance, low-dose Mn given thrice weekly for 5 weeks increased GABA levels (Takagi et al., 1990). Additional mechanistic studies are needed to better understand Mn’s role in GABA dysregulation. In the case of glutamate, high levels of Mn in the brain may trigger constitutive NMDA activation leading to excitotoxic-related neuronal death. Once released into the synaptic cleft, most glutamate is removed by astrocytes via the glutamate-aspartate transporter (GLAST). However, high levels of extracellular Mn2+ decrease the expression of GLAST and induce astrocyte apoptosis (Erikson et al., 2002). Chronic exposure to Mn can also increase the amplitude of excitatory postsynaptic potentials (EPSPs) in striatal neurons. With respect to the neurotransmitter dopamine, Ingersoll et al. (1999) demonstrated Mn transport to dopaminergic neurons via DAT. Another study done on DAT–/– mice receiving high doses of Mn reported a lower amount of striatal Mn compared to WT mice given the same dose. Interestingly, only the normally DAT-rich region of the striatum showed this contrasting pattern, which was not seen in areas not expressing DAT (Erikson et al., 2005). Young non-human primates exposed to a low dose of Mn twice weekly for about 9 weeks show retracted microglial processes even while dopaminergic neurons remained unchanged (Verina et al., 2011). More information is needed on the effect of this microglial disturbance on nigrostriatal neurons.
To conclude, Mn influx and efflux are tightly controlled in the body by various receptors and ion channels. However, overexposure to Mn can lead to the toxic accumulation of Mn in the brain, especially in the basal ganglia, causing hyperactivity of cortico-striatal neurons. While contradictory evidence arises from different dose regimens, in general Mn also impairs the regulation of neurotransmitters, such as dopamine, glutamate, and GABA by inhibiting the enzyme activity that regulates optimum neurotransmitter levels. High levels of glutamate and/or acetylcholine in the synaptic cleft overstimulate NMDA receptors leading to excitotoxic neuronal death. Mn may get transported into dopaminergic neurons via DAT. Excess cellular Mn2+ disrupts Ca2+ homeostasis in cells, leading to decreased dopamine production and neuronal death. Mn also causes ER and mitochondrial stress leading to neuronal apoptosis and/or gliosis. In light of the mounting evidence pointing to the deleterious effects of Mn on neurons and glia, researchers are examining the use of metal chelators and antioxidants as therapeutic interventions against manganism.
Manganese in Other Diseases
Until the last decade, Mn neurotoxicity was mainly associated with Parkinsonism, and very little attention had been given to its potential role in other neurodegenerative diseases. However, with growing interest in the neurobiology of heavy metals, Mn has now been linked to other major neurodegenerative diseases such as HD and prion diseases (Choi et al., 2010; Martin et al., 2011; Kumar et al., 2015). Furthermore, gene expression in the frontal cortex of cynomolgus macaques exposed to various Mn doses indicates that the amyloid-β (Aβ) precursor-like protein 1 (APLP1) of the amyloid precursor family was highly up-regulated, thereby linking Mn exposure to AD (Guilarte et al., 2008). Along with this gene array analysis, immunochemistry revealed the presence of Aβ plaques and αSyn aggregates, which have been linked to PD as well as AD, and which have also been seen in the gray and white matter of Mn-exposed animals (Guilarte, 2010).
In contrast to Mn-induced Parkinsonism, the pathogenesis of HD, an autosomal dominant disorder characterized by the neurodegeneration of medium spiny neurons in the striatum, appears to involve a Mn transport deficiency (Kumar et al., 2015). Recent experiments carried out with immortalized mutant HD cell lines (SThdhQ7/Q7 and SThdhQ111/Q111) show reduced TfR levels and substantial deficits in Mn uptake (Williams et al., 2010). In follow-up studies, YAC128 HD transgenic mice accumulated less Mn in their basal ganglia, including their striata, which are focal regions for both HD neuropathology and Mn accumulation (Madison et al., 2012). Furthermore, transition metal analysis of HD patients has shown significantly increased iron together with significantly decreased cortical copper and Mn (Rosas et al., 2012), further supporting the role of Mn in HD.
Prion protein (PrP) is widely known for its association with transmissible spongiform encephalopathies (TSE), a class of neurodegenerative diseases caused by the accumulation of an abnormal isoform of the prion protein known as PrPSc (Jin et al., 2015a). The cellular prion protein PrPC has a high-binding affinity for divalent metals. In fact, above-normal Mn content has been detected in the blood and brains of humans infected with Creutzfeldt–Jakob disease (CJD), in scrapie-infected mice, and in bovines infected with bovine spongiform encephalopathy (BSE) (Wong et al., 2001; Hesketh et al., 2007; Hesketh et al., 2008). The binding of Mn to prion protein mitigates Mn’s neurotoxicity during the early acute phase of Mn exposure (Choi et al., 2007). However, prolonged Mn exposure alters the stability of prion proteins without changing gene transcription (Choi et al., 2010), suggesting that Mn contributes to prion protein misfolding and prion disease pathogenesis. Interestingly, prion proteins survive significantly longer in a Mn-enriched soil matrix (Davies and Brown, 2009), a finding with important implications for the environmental transmissibility of PrPSc. The role of Mn in TSE was further validated by our lab’s discovery that it enhances the ability of the pathogenic PrPSc isoform to regulate Mn homeostasis (Martin et al., 2011) and by Davies and Brown (2009) reporting that Mn increases the infectivity of scrapie-infected cells. Therefore, deepening our understanding of how metals interact with disease-specific proteins will provide further insight into the pathogenesis and potential treatment of neurodegenerative diseases.
Future Directions
Our review of existing literature related to Mn overexposure and associated health issues has revealed genetic, sex- and age-related susceptibilities, signaling cascades involved in Mn neurotoxicity, and comparisons between PD and other motor disorders. Yet, many aspects of Mn overexposure and homeostasis remain largely understudied. For example, early childhood exposure to drinking water containing elevated Mn levels has been conclusively shown to compromise certain aspects of memory and learning; however, how absorption of excessive levels of Mn via the GIT leads to cognitive deficits (a CNS component) is still largely unknown. Secondly, given that the nasal tract and GIT are two well-known microbial environments, does overexposure to Mn via inhalation or ingestion alter the community composition of nasal or gut microbes or otherwise cause dysbiosis? Are changes in microbial populations offset by other lines of host defense against Mn toxicity or do these changes exacerbate the neuropathology? Thirdly, MRI and positron emission tomography (PET) on Mn-exposed individuals have shown changes in brain Mn accumulation and dopamine and GABA neurotransmitter levels. Yet despite the strong links between manganism and Parkinsonism, no case control study has examined the extent of elevated Mn accumulation in the brain and its associated neuropathology, including the presence of Lewy bodies/neurites and elevated phospho-α-synuclein expression. Additionally, longitudinal studies examining the immediate, intermediate and long-term effects of elevated Mn exposure on children and adults are needed to identify age- and sex-specific susceptibilities, and potential biological, psychological and cognitive biomarkers. Lastly, studies systematically identifying Mn exposure limits based on age, sex, and exposure duration as well as changes in signaling cascades associated with metal homeostasis and protein aggregation need to be conducted.
Conclusion
Chronic exposure to excessive Mn induces various neurological and psychiatric symptoms. While the body can efficiently remove excess Mn, primarily through the gut and liver, the brain cannot. Because of direct passage via the nasal neuroepithelium, inhaling large doses of Mn can lead to its accumulation in the brain’s basal ganglia. Astrocytes are particularly sensitive to Mn toxicity and may compound neuroinflammation by releasing pro-inflammatory cytokines in response to excess Mn. Mn can also bind to substrates of oxidative phosphorylation, thus inhibiting mitochondrial respiration and thereby augmenting oxidative stress. Chronic exposure to Mn causes benign α-synuclein monomers, present in all neurons, to undergo a conformational change to the oligomeric structures that are toxic to neurons. Additionally, Mn dysregulates key protein degradative and trafficking pathways including proteasomes, autophagy, and endosomal trafficking. Taken together, we entertain the notion that a ‘neurotoxic triad,’ comprising mitochondrial dysfunction and oxidative stress, protein misfolding and trafficking, and neuroinflammation, plays a major pathogenic role in Mn neurotoxicity (Figure 5). Beyond its effects on the CNS, excess Mn also interferes with the body’s iron metabolism and can cause kidney failure. Early detection and chelation therapy can effectively reverse the harmful effects caused by this metal; however, if it progresses untreated, it can cause severe neurological and physiological defects. As with any metal, the bioaccumulation and teratogenic effects of Mn remain a risk, yet this aspect has not been studied in detail. Similarly, an in-depth study of Mn’s role in protein misfolding and the upregulation of genetic markers for various neurological diseases in humans must be conducted. By combining the results of epidemiological surveys with human case studies as well as mechanistic studies done in in vitro and in animal models of Mn toxicity, we will eventually decipher the causes and symptoms of neurodegeneration caused by Mn toxicity well enough to develop effective therapeutic strategies that can be readily used against environmentally linked PD and related chronic neurodegenerative diseases.
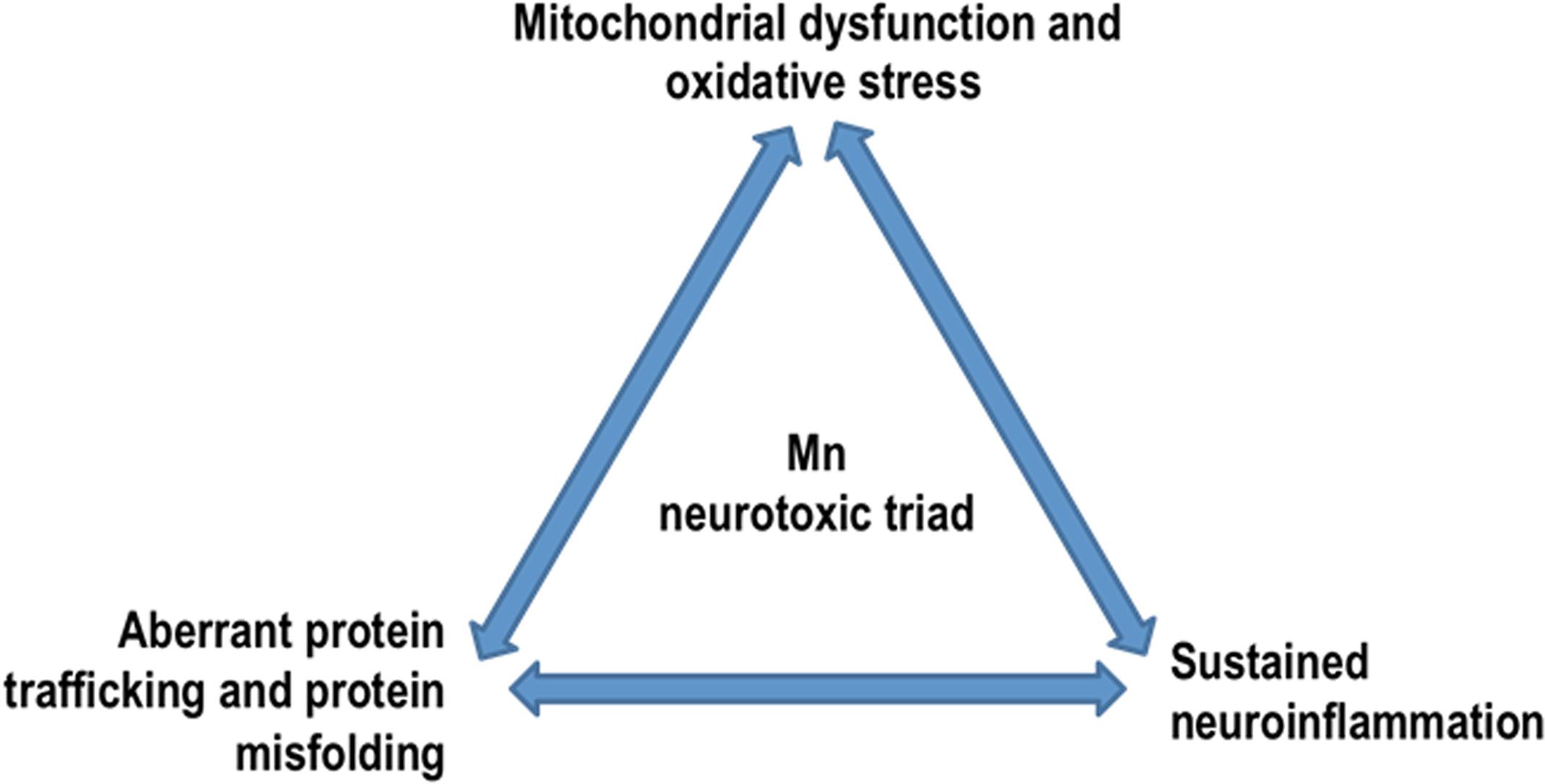
Figure 5. Mn neurotoxic triad: by dysregulating key protein degradative and trafficking pathways, Mn overexposure results in a ‘neurotoxic triad’ comprising mitochondrial dysfunction and oxidative stress, protein misfolding and trafficking, and neuroinflammation.
Author Contributions
DSH and SG conceived and wrote the article. GZ, HJ, AK, VA, and AGK provided intellectual input for review content and edited the manuscript. All authors read and approved the manuscript.
Funding
This work was supported by National Institutes of Health R01 grants ES026892, ES019267, and ES025991 to AGK and NS088206 to AK. The W. Eugene and Linda Lloyd Endowed Chair and Armbrust Endowment to AGK are also acknowledged.
Conflict of Interest Statement
AGK and VA have an equity interest in PK Biosciences Corporation located in Ames, IA, United States. The terms of this arrangement have been reviewed and approved by ISU in accordance with its conflict of interest policies.
The remaining authors declare that the research was conducted in the absence of any commercial or financial relationships that could be construed as a potential conflict of interest.
References
Aboud, A. A., Tidball, A. M., Kumar, K. K., Neely, M. D., Han, B., Ess, K. C., et al. (2014). PARK2 patient neuroprogenitors show increased mitochondrial sensitivity to copper. Neurobiol. Dis. 73C, 204–212. doi: 10.1016/j.nbd.2014.10.002
Afeseh Ngwa, H., Kanthasamy, A., Anantharam, V., Song, C., Witte, T., Houk, R., et al. (2009). Vanadium induces dopaminergic neurotoxicity via protein kinase C-delta dependent oxidative signaling mechanisms: relevance to etiopathogenesis of Parkinson’s disease. Toxicol. Appl. Pharmacol. 240, 273–285. doi: 10.1016/j.taap.2009.07.025
Afeseh Ngwa, H., Kanthasamy, A., Gu, Y., Fang, N., Anantharam, V., and Kanthasamy, A. G. (2011). Manganese nanoparticle activates mitochondrial dependent apoptotic signaling and autophagy in dopaminergic neuronal cells. Toxicol. Appl. Pharmacol. 256, 227–240. doi: 10.1016/j.taap.2011.07.018
Aguzzi, A., and Calella, A. M. (2009). Prions: protein aggregation and infectious diseases. Physiol. Rev. 89, 1105–1152. doi: 10.1152/physrev.00006.2009
Aguzzi, A., and O’Connor, T. (2010). Protein aggregation diseases: pathogenicity and therapeutic perspectives. Nat. Rev. Drug Discov. 9, 237–248. doi: 10.1038/nrd3050
Ali, M. M., Murthy, R. C., Saxena, D. K., and Chandra, S. V. (1983). Effect of low protein diet on manganese neurotoxicity: II. Brain GABA and seizure susceptibility. Neurobehav. Toxicol. Teratol. 5, 385–389.
Ali, S. F., Duhart, H. M., Newport, G. D., Lipe, G. W., and Slikker, W. Jr. (1995). Manganese-induced reactive oxygen species: comparison between Mn+2 and Mn+3. Neurodegeneration 4, 329–334. doi: 10.1016/1055-8330(95)90023-3
Aluru, N., Karchner, S. I., Franks, D. G., Nacci, D., Champlin, D., and Hahn, M. E. (2015). Targeted mutagenesis of aryl hydrocarbon receptor 2a and 2b genes in Atlantic killifish (Fundulus heteroclitus). Aquat. Toxicol. 158, 192–201. doi: 10.1016/j.aquatox.2014.11.016
Anantharam, V., Kitazawa, M., Wagner, J., Kaul, S., and Kanthasamy, A. G. (2002). Caspase-3-dependent proteolytic cleavage of protein kinase Cdelta is essential for oxidative stress-mediated dopaminergic cell death after exposure to methylcyclopentadienyl manganese tricarbonyl. J. Neurosci. 22, 1738–1751. doi: 10.1523/jneurosci.22-05-01738.2002
Andreini, C., Bertini, I., Cavallaro, G., Holliday, G. L., and Thornton, J. M. (2008). Metal ions in biological catalysis: from enzyme databases to general principles. J. Biol. Inorg. Chem. 13, 1205–1218. doi: 10.1007/s00775-008-0404-5
Aoki, I., Wu, Y. J., Silva, A. C., Lynch, R. M., and Koretsky, A. P. (2004). In vivo detection of neuroarchitecture in the rodent brain using manganese-enhanced MRI. Neuroimage 22, 1046–1059. doi: 10.1016/j.neuroimage.2004.03.031
Appendino, G., Minassi, A., and Taglialatela-Scafati, O. (2014). Recreational drug discovery: natural products as lead structures for the synthesis of smart drugs. Nat. Prod. Rep. 31, 880–904. doi: 10.1039/c4np00010b
Archibald, F. S., and Tyree, C. (1987). Manganese poisoning and the attack of trivalent manganese upon catecholamines. Arch. Biochem. Biophys. 256, 638–650. doi: 10.1016/0003-9861(87)90621-7
Aschner, J. L., and Aschner, M. (2005). Nutritional aspects of manganese homeostasis. Mol. Aspects Med. 26, 353–362. doi: 10.1016/j.mam.2005.07.003
Bak, L. K., Schousboe, A., and Waagepetersen, H. S. (2006). The glutamate/GABA-glutamine cycle: aspects of transport, neurotransmitter homeostasis and ammonia transfer. J. Neurochem. 98, 641–653. doi: 10.1111/j.1471-4159.2006.03913.x
Ballard, P. A., Tetrud, J. W., and Langston, J. W. (1985). Permanent human parkinsonism due to 1-methyl-4-phenyl-1,2,3,6-tetrahydropyridine (MPTP): seven cases. Neurology 35, 949–956.
Bell, J. G., Keen, C. L., and Lonnerdal, B. (1989). Higher retention of manganese in suckling than in adult rats is not due to maturational differences in manganese uptake by rat small intestine. J. Toxicol. Environ. Health 26, 387–398. doi: 10.1080/15287398909531263
Binolfi, A., Lamberto, G. R., Duran, R., Quintanar, L., Bertoncini, C. W., Souza, J. M., et al. (2008). Site-specific interactions of Cu(II) with alpha and beta-synuclein: bridging the molecular gap between metal binding and aggregation. J. Am. Chem. Soc. 130, 11801–11812. doi: 10.1021/ja803494v
Binolfi, A., Rasia, R. M., Bertoncini, C. W., Ceolin, M., Zweckstetter, M., Griesinger, C., et al. (2006). Interaction of alpha-synuclein with divalent metal ions reveals key differences: a link between structure, binding specificity and fibrillation enhancement. J. Am. Chem. Soc. 128, 9893–9901. doi: 10.1021/ja0618649
Bornhorst, J., Chakraborty, S., Meyer, S., Lohren, H., Brinkhaus, S. G., Knight, A. L., et al. (2014). The effects of pdr1, djr1.1 and pink1 loss in manganese-induced toxicity and the role of alpha-synuclein in C. elegans. Metallomics 6, 476–490. doi: 10.1039/c3mt00325f
Bowman, A. B., Kwakye, G. F., Herrero Hernandez, E., and Aschner, M. (2011). Role of manganese in neurodegenerative diseases. J. Trace Elem. Med. Biol. 25, 191–203. doi: 10.1016/j.jtemb.2011.08.144
Breda, C., Nugent, M. L., Estranero, J. G., Kyriacou, C. P., Outeiro, T. F., Steinert, J. R., et al. (2015). Rab11 modulates alpha-synuclein-mediated defects in synaptic transmission and behaviour. Hum. Mol. Genet. 24, 1077–1091. doi: 10.1093/hmg/ddu521
Brown, R. C., Lockwood, A. H., and Sonawane, B. R. (2005). Neurodegenerative diseases: an overview of environmental risk factors. Environ. Health Perspect. 113, 1250–1256. doi: 10.1289/ehp.7567
Cai, T., Yao, T., Zheng, G., Chen, Y., Du, K., Cao, Y., et al. (2010). Manganese induces the overexpression of alpha-synuclein in PC12 cells via ERK activation. Brain Res. 1359, 201–207. doi: 10.1016/j.brainres.2010.08.055
Calne, D. B., Chu, N. S., Huang, C. C., Lu, C. S., and Olanow, W. (1994). Manganism and idiopathic parkinsonism: similarities and differences. Neurology 44, 1583–1586.
Carson, M. J., Bilousova, T. V., Puntambekar, S. S., Melchior, B., Doose, J. M., and Ethell, I. M. (2007). A rose by any other name? The potential consequences of microglial heterogeneity during CNS health and disease. Neurotherapeutics 4, 571–579. doi: 10.1016/j.nurt.2007.07.002
Cerchiaro, G., Bolin, C., and Cardozo-Pelaez, F. (2009). Hydroxyl radical oxidation of guanosine 5’-triphosphate (GTP): requirement for a GTP-Cu(II) complex. Redox Rep. 14, 82–92. doi: 10.1179/135100009X392520
Chen, C. J., and Liao, S. L. (2002). Oxidative stress involves in astrocytic alterations induced by manganese. Exp. Neurol. 175, 216–225. doi: 10.1006/exnr.2002.7894
Chen, M. T., Cheng, G. W., Lin, C. C., Chen, B. H., and Huang, Y. L. (2006). Effects of acute manganese chloride exposure on lipid peroxidation and alteration of trace metals in rat brain. Biol. Trace Elem. Res. 110, 163–178. doi: 10.1385/bter:110:2:163
Chen, M. T., Sheu, J. Y., and Lin, T. H. (2000). Protective effects of manganese against lipid peroxidation. J. Toxicol. Environ. Health A 61, 569–577.
Choi, C. J., Anantharam, V., Martin, D. P., Nicholson, E. M., Richt, J. A., Kanthasamy, A., et al. (2010). Manganese upregulates cellular prion protein and contributes to altered stabilization and proteolysis: relevance to role of metals in pathogenesis of prion disease. Toxicol. Sci. 115, 535–546. doi: 10.1093/toxsci/kfq049
Choi, C. J., Anantharam, V., Saetveit, N. J., Houk, R. S., Kanthasamy, A., and Kanthasamy, A. G. (2007). Normal cellular prion protein protects against manganese-induced oxidative stress and apoptotic cell death. Toxicol. Sci. 98, 495–509. doi: 10.1093/toxsci/kfm099
Chung, C. Y., Khurana, V., Yi, S., Sahni, N., Loh, K. H., Auluck, P. K., et al. (2017). In situ peroxidase labeling and mass-spectrometry connects alpha-synuclein directly to endocytic trafficking and mRNA metabolism in neurons. Cell Syst. 4, 242–250.e4. doi: 10.1016/j.cels.2017.01.002
Ciccocioppo, F., Lanuti, P., Marchisio, M., Gambi, F., Santavenere, E., Pierdomenico, L., et al. (2008). Expression and phosphorylation of protein kinase C isoforms in Abeta(1-42) activated T lymphocytes from Alzheimers disease. Int. J. Immunopathol. Pharmacol. 21, 23–33. doi: 10.1177/039463200802100104
Claus Henn, B., Ettinger, A. S., Schwartz, J., Tellez-Rojo, M. M., Lamadrid-Figueroa, H., Hernandez-Avila, M., et al. (2010). Early postnatal blood manganese levels and children’s neurodevelopment. Epidemiology 21, 433–439. doi: 10.1097/ede.0b013e3181df8e52
Conway, K. A., Rochet, J. C., Bieganski, R. M., and Lansbury, P. T. Jr. (2001). Kinetic stabilization of the alpha-synuclein protofibril by a dopamine-alpha-synuclein adduct. Science 294, 1346–1349. doi: 10.1126/science.1063522
Cook, D. G., Fahn, S., and Brait, K. A. (1974). Chronic manganese intoxication. Arch. Neurol. 30, 59–64. doi: 10.1001/archneur.1974.00490310061010
Cooke, M. S., Evans, M. D., Dizdaroglu, M., and Lunec, J. (2003). Oxidative DNA damage: mechanisms, mutation, and disease. FASEB J. 17, 1195–1214. doi: 10.1096/fj.02-0752rev
Cooper, A. A., Gitler, A. D., Cashikar, A., Haynes, C. M., Hill, K. J., Bhullar, B., et al. (2006). Alpha-synuclein blocks ER-Golgi traffic and Rab1 rescues neuron loss in Parkinson’s models. Science 313, 324–328. doi: 10.1126/science.1129462
Cordova, F. M., Aguiar, A. S. Jr., Peres, T. V., Lopes, M. W., Gonçalves, F. M., Pedro, D. Z., et al. (2013). Manganese-exposed developing rats display motor deficits and striatal oxidative stress that are reversed by Trolox. Arch. Toxicol. 87, 1231–1244. doi: 10.1007/s00204-013-1017-5
Couper, J. (1837). On the effects of black oxide of manganese when inhaled into the lungs. Br. Ann. Med. Pharmacol. 1, 41–42.
Crossgrove, J. S., Allen, D. D., Bukaveckas, B. L., Rhineheimer, S. S., and Yokel, R. A. (2003). Manganese distribution across the blood-brain barrier. I. Evidence for carrier-mediated influx of managanese citrate as well as manganese and manganese transferrin. Neurotoxicology 24, 3–13. doi: 10.1016/s0161-813x(02)00089-x
Crossgrove, J. S., and Yokel, R. A. (2005). Manganese distribution across the blood-brain barrier. IV. Evidence for brain influx through store-operated calcium channels. Neurotoxicology 26, 297–307. doi: 10.1016/j.neuro.2004.09.004
Davidsson, L., Lonnerdal, B., Sandstrom, B., Kunz, C., and Keen, C. L. (1989). Identification of transferrin as the major plasma carrier protein for manganese introduced orally or intravenously or after in vitro addition in the rat. J. Nutr. 119, 1461–1464. doi: 10.1093/jn/119.10.1461
Davies, P., and Brown, D. R. (2009). Manganese enhances prion protein survival in model soils and increases prion infectivity to cells. PLoS One 4:e7518. doi: 10.1371/journal.pone.0007518
Davis, M. Y., Keene, C. D., Jayadev, S., and Bird, T. (2014). The co-occurrence of Alzheimer’s disease and Huntington’s disease: a neuropathological study of 15 elderly Huntington’s disease subjects. J. Huntingtons Dis. 3, 209–217. doi: 10.3233/JHD-140111
Dawson, T. M., Ko, H. S., and Dawson, V. L. (2010). Genetic animal models of Parkinson’s disease. Neuron 66, 646–661. doi: 10.1016/j.neuron.2010.04.034
Dendle, P. (2001). Lupines, manganese, and devil-sickness: an Anglo-Saxon medical response to epilepsy. Bull. Hist. Med. 75, 91–101. doi: 10.1353/bhm.2001.0009
Desole, M. S., Serra, P. A., Esposito, G., Delogu, M. R., Migheli, R., Fresu, L., et al. (2000). Glutathione deficiency potentiates manganese-induced increases in compounds associated with high-energy phosphate degradation in discrete brain areas of young and aged rats. Aging 12, 470–477. doi: 10.1007/bf03339879
Desplats, P., Lee, H. J., Bae, E. J., Patrick, C., Rockenstein, E., Crews, L., et al. (2009). Inclusion formation and neuronal cell death through neuron-to-neuron transmission of alpha-synuclein. Proc. Natl. Acad. Sci. U.S.A. 106, 13010–13015. doi: 10.1073/pnas.0903691106
Dick, F. D., De Palma, G., Ahmadi, A., Scott, N. W., Prescott, G. J., Bennett, J., et al. (2007). Environmental risk factors for Parkinson’s disease and parkinsonism: the Geoparkinson study. Occup. Environ. Med. 64, 666–672. doi: 10.1136/oem.2006.027003
Du, H. N., Li, H. T., Zhang, F., Lin, X. J., Shi, J. H., Shi, Y. H., et al. (2006). Acceleration of alpha-synuclein aggregation by homologous peptides. FEBS Lett. 580, 3657–3664.
Ducic, T., Carboni, E., Lai, B., Chen, S., Michalke, B., Lazaro, D. F., et al. (2015). Alpha-synuclein regulates neuronal levels of manganese and calcium. ACS Chem. Neurosci. 6, 1769–1779. doi: 10.1021/acschemneuro.5b00093
Dukhande, V. V., Malthankar-Phatak, G. H., Hugus, J. J., Daniels, C. K., and Lai, J. C. (2006). Manganese-induced neurotoxicity is differentially enhanced by glutathione depletion in astrocytoma and neuroblastoma cells. Neurochem. Res. 31, 1349–1357. doi: 10.1007/s11064-006-9179-7
Dunning, C. J., George, S., and Brundin, P. (2013). What’s to like about the prion-like hypothesis for the spreading of aggregated alpha-synuclein in Parkinson disease? Prion 7, 92–97. doi: 10.4161/pri.23806
El-Agnaf, O. M., Salem, S. A., Paleologou, K. E., Cooper, L. J., Fullwood, N. J., Gibson, M. J., et al. (2003). Alpha-synuclein implicated in Parkinson’s disease is present in extracellular biological fluids, including human plasma. FASEB J. 17, 1945–1947. doi: 10.1096/fj.03-0098fje
Emmanouilidou, E., Melachroinou, K., Roumeliotis, T., Garbis, S. D., Ntzouni, M., Margaritis, L. H., et al. (2010). Cell-produced alpha-synuclein is secreted in a calcium-dependent manner by exosomes and impacts neuronal survival. J. Neurosci. 30, 6838–6851. doi: 10.1523/JNEUROSCI.5699-09.2010
Ensing, J. G. (1985). Bazooka: cocaine-base and manganese carbonate. J. Anal. Toxicol. 9, 45–46. doi: 10.1093/jat/9.1.45
Erikson, K. M., and Aschner, M. (2006). Increased manganese uptake by primary astrocyte cultures with altered iron status is mediated primarily by divalent metal transporter. Neurotoxicology 27, 125–130. doi: 10.1016/j.neuro.2005.07.003
Erikson, K. M., John, C. E., Jones, S. R., and Aschner, M. (2005). Manganese accumulation in striatum of mice exposed to toxic doses is dependent upon a functional dopamine transporter. Environ. Toxicol. Pharmacol. 20, 390–394. doi: 10.1016/j.etap.2005.03.009
Erikson, K. M., Suber, R. L., and Aschner, M. (2002). Glutamate/aspartate transporter (GLAST), taurine transporter and metallothionein mRNA levels are differentially altered in astrocytes exposed to manganese chloride, manganese phosphate or manganese sulfate. Neurotoxicology 23, 281–288. doi: 10.1016/s0161-813x(02)00041-4
Esterbauer, H., Schaur, R. J., and Zollner, H. (1991). Chemistry and biochemistry of 4-hydroxynonenal, malonaldehyde and related aldehydes. Free Radic. Biol. Med. 11, 81–128. doi: 10.1016/0891-5849(91)90192-6
Farina, M., Avila, D. S., Da Rocha, J. B., and Aschner, M. (2013). Metals, oxidative stress and neurodegeneration: a focus on iron, manganese and mercury. Neurochem. Int. 62, 575–594. doi: 10.1016/j.neuint.2012.12.006
Farooqui, A. A., and Horrocks, L. A. (2007). Glycerophospholipids in Brain: Phospholipase A2 in Neurological Disorders. New York, NY: Springer.
Farooqui, T., and Farooqui, A. A. (2011). Lipid-mediated oxidative stress and inflammation in the pathogenesis of Parkinson’s disease. Parkinsons Dis. 2011:247467. doi: 10.4061/2011/247467
Farrell, N. (2003). “Metal complexes as drugs and chemotherapeutic agents,” in Comprehensive Coordination Chemistry II, eds J. A. McCleverty and T. J. Meyer (Oxford: Pergamon), 809–840.
Finley, J. W., Johnson, P. E., and Johnson, L. K. (1994). Sex affects manganese absorption and retention by humans from a diet adequate in manganese. Am. J. Clin. Nutr. 60, 949–955. doi: 10.1093/ajcn/60.6.949
Fitsanakis, V. A., Au, C., Erikson, K. M., and Aschner, M. (2006). The effects of manganese on glutamate, dopamine and gamma-aminobutyric acid regulation. Neurochem. Int. 48, 426–433. doi: 10.1016/j.neuint.2005.10.012
Fitsanakis, V. A., Piccola, G., Marreilha Dos Santos, A. P., Aschner, J. L., and Aschner, M. (2007). Putative proteins involved in manganese transport across the blood-brain barrier. Hum. Exp. Toxicol. 26, 295–302. doi: 10.1177/0960327107070496
Gavin, C. E., Gunter, K. K., and Gunter, T. E. (1990). Manganese and calcium efflux kinetics in brain mitochondria. Relevance to manganese toxicity. Biochem. J. 266, 329–334. doi: 10.1042/bj2660329
Gavin, C. E., Gunter, K. K., and Gunter, T. E. (1999). Manganese and calcium transport in mitochondria: implications for manganese toxicity. Neurotoxicology 20, 445–453.
Ghosh, A., Roy, A., Liu, X., Kordower, J. H., Mufson, E. J., Hartley, D. M., et al. (2007). Selective inhibition of NF-kappaB activation prevents dopaminergic neuronal loss in a mouse model of Parkinson’s disease. Proc. Natl. Acad. Sci. U.S.A. 104, 18754–18759. doi: 10.1073/pnas.0704908104
Ghosh, A., Roy, A., Matras, J., Brahmachari, S., Gendelman, H. E., and Pahan, K. (2009). Simvastatin inhibits the activation of p21ras and prevents the loss of dopaminergic neurons in a mouse model of Parkinson’s disease. J. Neurosci. 29, 13543–13556. doi: 10.1523/JNEUROSCI.4144-09.2009
Ghosh, A., Saminathan, H., Kanthasamy, A., Anantharam, V., Jin, H., Sondarva, G., et al. (2013). The peptidyl-prolyl isomerase Pin1 up-regulation and proapoptotic function in dopaminergic neurons: relevance to the pathogenesis of Parkinson disease. J. Biol. Chem. 288, 21955–21971. doi: 10.1074/jbc.M112.444224
Girijashanker, K., He, L., Soleimani, M., Reed, J. M., Li, H., Liu, Z., et al. (2008). Slc39a14 gene encodes ZIP14, a metal/bicarbonate symporter: similarities to the ZIP8 transporter. Mol. Pharmacol. 73, 1413–1423. doi: 10.1124/mol.107.043588
Girotto, S., Sturlese, M., Bellanda, M., Tessari, I., Cappellini, R., Bisaglia, M., et al. (2012). Dopamine-derived quinones affect the structure of the redox sensor DJ-1 through modifications at Cys-106 and Cys-53. J. Biol. Chem. 287, 18738–18749. doi: 10.1074/jbc.M111.311589
Gitler, A. D., Chesi, A., Geddie, M. L., Strathearn, K. E., Hamamichi, S., Hill, K. J., et al. (2009). Alpha-synuclein is part of a diverse and highly conserved interaction network that includes PARK9 and manganese toxicity. Nat. Genet. 41, 308–315. doi: 10.1038/ng.300
Goncalves, S. A., Macedo, D., Raquel, H., Simoes, P. D., Giorgini, F., Ramalho, J. S., et al. (2016). shRNA-based screen identifies endocytic recycling pathway components that act as genetic modifiers of alpha-synuclein aggregation, secretion and toxicity. PLoS Genet. 12:e1005995. doi: 10.1371/journal.pgen.1005995
Graham, D. G. (1978). Oxidative pathways for catecholamines in the genesis of neuromelanin and cytotoxic quinones. Mol. Pharmacol. 14, 633–643.
Grant, B. D., and Donaldson, J. G. (2009). Pathways and mechanisms of endocytic recycling. Nat. Rev. Mol. Cell Biol. 10, 597–608. doi: 10.1038/nrm2755
Guilarte, T. R. (2010). APLP1, Alzheimer’s-like pathology and neurodegeneration in the frontal cortex of manganese-exposed non-human primates. Neurotoxicology 31, 572–574. doi: 10.1016/j.neuro.2010.02.004
Guilarte, T. R., Burton, N. C., Verina, T., Prabhu, V. V., Becker, K. G., Syversen, T., et al. (2008). Increased APLP1 expression and neurodegeneration in the frontal cortex of manganese-exposed non-human primates. J. Neurochem. 105, 1948–1959. doi: 10.1111/j.1471-4159.2008.05295.x
Harischandra, D. S., Ghaisas, S., Rokad, D., and Kanthasamy, A. G. (2017). Exosomes in toxicology: relevance to chemical exposure and pathogenesis of environmentally linked diseases. Toxicol. Sci. 158, 3–13. doi: 10.1093/toxsci/kfx074
Harischandra, D. S., Ghaisas, S., Rokad, D., Zamanian, M., Jin, H., Anantharam, V., et al. (2018). Environmental neurotoxicant manganese regulates exosome-mediated extracellular miRNAs in cell culture model of Parkinson’s disease: relevance to alpha-synuclein misfolding in metal neurotoxicity. Neurotoxicology 64, 267–277. doi: 10.1016/j.neuro.2017.04.007
Harischandra, D. S., Jin, H., Anantharam, V., Kanthasamy, A., and Kanthasamy, A. G. (2015a). alpha-Synuclein protects against manganese neurotoxic insult during the early stages of exposure in a dopaminergic cell model of Parkinson’s disease. Toxicol. Sci. 143, 454–468. doi: 10.1093/toxsci/kfu247
Harischandra, D. S., Lawana, V., Rokad, D., Jin, H., Anantharam, V., Kanthasamy, A., et al. (2015b). Lysosomal dysfunction caused by the environmental neurotoxicant manganese increases exosome-mediated cell-to-cell transfer of α-synuclein by a prion-like mechanism. Neurotoxicol. Teratol. 49:109. doi: 10.1016/j.ntt.2015.04.034
Harischandra, D. S., Kondru, N., Martin, D. P., Kanthasamy, A., Jin, H., Anantharam, V., et al. (2014). Role of proteolytic activation of protein kinase Cdelta in the pathogenesis of prion disease. Prion 8, 143–153. doi: 10.4161/pri.28369
Harischandra, D. S., Rokad, D., Neal, M. L., Ghaisas, S., Manne, S., Sarkar, S., et al. (2019). Manganese promotes the aggregation and prion-like cell-to-cell exosomal transmission of alpha-synuclein. Sci. Signal. 12:eaau4543. doi: 10.1126/scisignal.aau4543
Hazell, A. S., and Norenberg, M. D. (1998). Ammonia and manganese increase arginine uptake in cultured astrocytes. Neurochem. Res. 23, 869–873.
Hermida-Ameijeiras, A., Mendez-Alvarez, E., Sanchez-Iglesias, S., Sanmartin-Suarez, C., and Soto-Otero, R. (2004). Autoxidation and MAO-mediated metabolism of dopamine as a potential cause of oxidative stress: role of ferrous and ferric ions. Neurochem. Int. 45, 103–116. doi: 10.1016/j.neuint.2003.11.018
Hesketh, S., Sassoon, J., Knight, R., and Brown, D. R. (2008). Elevated manganese levels in blood and CNS in human prion disease. Mol. Cell. Neurosci. 37, 590–598. doi: 10.1016/j.mcn.2007.12.008
Hesketh, S., Sassoon, J., Knight, R., Hopkins, J., and Brown, D. R. (2007). Elevated manganese levels in blood and central nervous system occur before onset of clinical signs in scrapie and bovine spongiform encephalopathy. J. Anim. Sci. 85, 1596–1609. doi: 10.2527/jas.2006-714
Holm, R. H., Kennepohl, P., and Solomon, E. I. (1996). Structural and functional aspects of metal sites in biology. Chem. Rev. 96, 2239–2314. doi: 10.1021/cr9500390
Huang, C. C. (2007). Parkinsonism induced by chronic manganese intoxication–an experience in Taiwan. Chang Gung Med. J. 30, 385–395.
Huang, C. C., Chu, N. S., Lu, C. S., Wang, J. D., Tsai, J. L., Tzeng, J. L., et al. (1989). Chronic manganese intoxication. Arch. Neurol. 46, 1104–1106.
Huang, E., Ong, W. Y., and Connor, J. R. (2004). Distribution of divalent metal transporter-1 in the monkey basal ganglia. Neuroscience 128, 487–496. doi: 10.1016/j.neuroscience.2004.06.055
Ingersoll, R. T., Montgomery, E. B. Jr., and Aposhian, H. V. (1999). Central nervous system toxicity of manganese. II: cocaine or reserpine inhibit manganese concentration in the rat brain. Neurotoxicology 20, 467–476.
Isobe, C., Abe, T., and Terayama, Y. (2010). Levels of reduced and oxidized coenzyme Q-10 and 8-hydroxy-2’-deoxyguanosine in the cerebrospinal fluid of patients with living Parkinson’s disease demonstrate that mitochondrial oxidative damage and/or oxidative DNA damage contributes to the neurodegenerative process. Neurosci. Lett. 469, 159–163. doi: 10.1016/j.neulet.2009.11.065
Jin, H., Harischandra, D. S., Choi, C., Martin, D., Anantharam, V., Kanthasamy, A., et al. (2015a). “Chapter 23 manganese and prion disease,” in Manganese in Health and Disease, ed. D. R. Brown (London: The Royal Society of Chemistry), 574–603. doi: 10.1039/9781782622383-00574
Jin, H., Kanthasamy, A., Harischandra, D. S., Anantharam, V., Rana, A., and Kanthasamy, A. (2015b). Targeted toxicants to dopaminergic neuronal cell death. Methods Mol. Biol. 1254, 239–252. doi: 10.1007/978-1-4939-2152-2_18
Jin, H., Kanthasamy, A., Anantharam, V., Rana, A., and Kanthasamy, A. G. (2011). Transcriptional regulation of pro-apoptotic protein kinase Cdelta: implications for oxidative stress-induced neuronal cell death. J. Biol. Chem. 286, 19840–19859. doi: 10.1074/jbc.M110.203687
Kannurpatti, S. S., Joshi, P. G., and Joshi, N. B. (2000). Calcium sequestering ability of mitochondria modulates influx of calcium through glutamate receptor channel. Neurochem. Res. 25, 1527–1536.
Kanthasamy, A. G., Anantharam, V., Zhang, D., Latchoumycandane, C., Jin, H., Kaul, S., et al. (2006). A novel peptide inhibitor targeted to caspase-3 cleavage site of a proapoptotic kinase protein kinase C delta (PKCdelta) protects against dopaminergic neuronal degeneration in Parkinson’s disease models. Free Radic. Biol. Med. 41, 1578–1589. doi: 10.1016/j.freeradbiomed.2006.08.016
Kanthasamy, A. G., Choi, C., Jin, H., Harischandra, D. S., Anantharam, V., and Kanthasamy, A. (2012). Effect of divalent metals on the neuronal proteasomal system, prion protein ubiquitination and aggregation. Toxicol. Lett. 214, 288–295. doi: 10.1016/j.toxlet.2012.09.008
Kanthasamy, A. G., Kitazawa, M., Yang, Y., Anantharam, V., and Kanthasamy, A. (2008). Environmental neurotoxin dieldrin induces apoptosis via caspase-3-dependent proteolytic activation of protein kinase C delta (PKCdelta): implications for neurodegeneration in Parkinson’s disease. Mol. Brain 1:12. doi: 10.1186/1756-6606-1-12
Kenche, V. B., and Barnham, K. J. (2011). Alzheimer’s disease & metals: therapeutic opportunities. Br. J. Pharmacol. 163, 211–219.
Kett, L. R., and Dauer, W. T. (2016). Endolysosomal dysfunction in Parkinson’s disease: recent developments and future challenges. Mov. Disord. 31, 1433–1443. doi: 10.1002/mds.26797
Kim, Y., Kim, J. M., Kim, J. W., Yoo, C. I., Lee, C. R., Lee, J. H., et al. (2002). Dopamine transporter density is decreased in Parkinsonian patients with a history of manganese exposure: what does it mean? Mov. Disord. 17, 568–575. doi: 10.1002/mds.10089
Kordower, J. H., Chu, Y., Hauser, R. A., Freeman, T. B., and Olanow, C. W. (2008). Lewy body-like pathology in long-term embryonic nigral transplants in Parkinson’s disease. Nat. Med. 14, 504–506. doi: 10.1038/nm1747
Kotzbauer, P. T., Trojanowsk, J. Q., and Lee, V. M. (2001). Lewy body pathology in Alzheimer’s disease. J. Mol. Neurosci. 17, 225–232.
Kumar, K. K., Goodwin, C. R., Uhouse, M. A., Bornhorst, J., Schwerdtle, T., Aschner, M., et al. (2015). Untargeted metabolic profiling identifies interactions between Huntington’s disease and neuronal manganese status. Metallomics 7, 363–370. doi: 10.1039/c4mt00223g
Kwakye, G. F., Paoliello, M. M., Mukhopadhyay, S., Bowman, A. B., and Aschner, M. (2015). Manganese-induced Parkinsonism and Parkinson’s disease: shared and distinguishable features. Int. J. Environ. Res. Public Health 12, 7519–7540. doi: 10.3390/ijerph120707519
Lai, J. C., Minski, M. J., Chan, A. W., Leung, T. K., and Lim, L. (1999). Manganese mineral interactions in brain. Neurotoxicology 20, 433–444.
Langston, J. W., Ballard, P., Tetrud, J. W., and Irwin, I. (1983). Chronic Parkinsonism in humans due to a product of meperidine-analog synthesis. Science 219, 979–980. doi: 10.1126/science.6823561
Latchoumycandane, C., Anantharam, V., Jin, H., Kanthasamy, A., and Kanthasamy, A. (2011). Dopaminergic neurotoxicant 6-OHDA induces oxidative damage through proteolytic activation of PKCdelta in cell culture and animal models of Parkinson’s disease. Toxicol. Appl. Pharmacol. 256, 314–323. doi: 10.1016/j.taap.2011.07.021
Latchoumycandane, C., Anantharam, V., Kitazawa, M., Yang, Y., Kanthasamy, A., and Kanthasamy, A. G. (2005). Protein kinase Cdelta is a key downstream mediator of manganese-induced apoptosis in dopaminergic neuronal cells. J. Pharmacol. Exp. Ther. 313, 46–55. doi: 10.1124/jpet.104.078469
LaVoie, M. J., Ostaszewski, B. L., Weihofen, A., Schlossmacher, M. G., and Selkoe, D. J. (2005). Dopamine covalently modifies and functionally inactivates parkin. Nat. Med. 11, 1214–1221. doi: 10.1038/nm1314
Lee, H. J., Suk, J. E., Bae, E. J., Lee, J. H., Paik, S. R., and Lee, S. J. (2008). Assembly-dependent endocytosis and clearance of extracellular alpha-synuclein. Int. J. Biochem. Cell Biol. 40, 1835–1849. doi: 10.1016/j.biocel.2008.01.017
Leitch, S., Feng, M., Muend, S., Braiterman, L. T., Hubbard, A. L., and Rao, R. (2011). Vesicular distribution of Secretory Pathway Ca2+-ATPase isoform 1 and a role in manganese detoxification in liver-derived polarized cells. Biometals 24, 159–170. doi: 10.1007/s10534-010-9384-3
Leyva-Illades, D., Chen, P., Zogzas, C. E., Hutchens, S., Mercado, J. M., Swaim, C. D., et al. (2014). SLC30A10 is a cell surface-localized manganese efflux transporter, and parkinsonism-causing mutations block its intracellular trafficking and efflux activity. J. Neurosci. 34, 14079–14095. doi: 10.1523/JNEUROSCI.2329-14.2014
Li, J. Y., Englund, E., Holton, J. L., Soulet, D., Hagell, P., Lees, A. J., et al. (2008). Lewy bodies in grafted neurons in subjects with Parkinson’s disease suggest host-to-graft disease propagation. Nat. Med. 14, 501–503. doi: 10.1038/nm1746
Li, Y., Sun, L., Cai, T., Zhang, Y., Lv, S., Wang, Y., et al. (2010). alpha-Synuclein overexpression during manganese-induced apoptosis in SH-SY5Y neuroblastoma cells. Brain Res. Bull. 81, 428–433. doi: 10.1016/j.brainresbull.2009.11.007
Liu, G., Aliaga, L., and Cai, H. (2012). alpha-synuclein, LRRK2 and their interplay in Parkinson’s disease. Future Neurol. 7, 145–153. doi: 10.2217/fnl.12.2
Liu, J., Zhang, J. P., Shi, M., Quinn, T., Bradner, J., Beyer, R., et al. (2009). Rab11a and HSP90 regulate recycling of extracellular alpha-synuclein. J. Neurosci. 29, 1480–1485. doi: 10.1523/JNEUROSCI.6202-08.2009
Lotharius, J., and Brundin, P. (2002). Pathogenesis of Parkinson’s disease: dopamine, vesicles and alpha-synuclein. Nat. Rev. Neurosci. 3, 932–942. doi: 10.1038/nrn983
Luan, X., Sansanaphongpricha, K., Myers, I., Chen, H., Yuan, H., and Sun, D. (2017). Engineering exosomes as refined biological nanoplatforms for drug delivery. Acta Pharmacol. Sin. 38, 754–763. doi: 10.1038/aps.2017.12
Maddirala, Y., Tobwala, S., and Ercal, N. (2015). N-acetylcysteineamide protects against manganese-induced toxicity in SHSY5Y cell line. Brain Res. 1608, 157–166. doi: 10.1016/j.brainres.2015.02.006
Madejczyk, M. S., and Ballatori, N. (2012). The iron transporter ferroportin can also function as a manganese exporter. Biochim. Biophys. Acta 1818, 651–657. doi: 10.1016/j.bbamem.2011.12.002
Madison, J. L., Wegrzynowicz, M., Aschner, M., and Bowman, A. B. (2012). Disease-toxicant interactions in manganese exposed Huntington disease mice: early changes in striatal neuron morphology and dopamine metabolism. PLoS One 7:e31024. doi: 10.1371/journal.pone.0031024
Martin, D. P., Anantharam, V., Jin, H., Witte, T., Houk, R., Kanthasamy, A., et al. (2011). Infectious prion protein alters manganese transport and neurotoxicity in a cell culture model of prion disease. Neurotoxicology 32, 554–562. doi: 10.1016/j.neuro.2011.07.008
Martinez-Finley, E. J., Chakraborty, S., Fretham, S. J., and Aschner, M. (2012). Cellular transport and homeostasis of essential and nonessential metals. Metallomics 4, 593–605. doi: 10.1039/c2mt00185c
McKeith, I., Mintzer, J., Aarsland, D., Burn, D., Chiu, H., Cohen-Mansfield, J., et al. (2004). Dementia with Lewy bodies. Lancet Neurol. 3, 19–28.
Menezes-Filho, J. A., Novaes Cde, O., Moreira, J. C., Sarcinelli, P. N., and Mergler, D. (2011). Elevated manganese and cognitive performance in school-aged children and their mothers. Environ. Res. 111, 156–163. doi: 10.1016/j.envres.2010.09.006
Miklossy, J., Doudet, D. D., Schwab, C., Yu, S., Mcgeer, E. G., and Mcgeer, P. L. (2006). Role of ICAM-1 in persisting inflammation in Parkinson disease and MPTP monkeys. Exp. Neurol. 197, 275–283. doi: 10.1016/j.expneurol.2005.10.034
Milatovic, D., and Aschner, M. (2009). Measurement of isoprostanes as markers of oxidative stress in neuronal tissue. Curr. Protoc. Toxicol. 2009, 12.14.1–12.14.12. doi: 10.1002/0471140856.tx1214s39
Milatovic, D., Gupta, R. C., and Aschner, M. (2006). Anticholinesterase toxicity and oxidative stress. ScientificWorldJournal 6, 295–310. doi: 10.1100/tsw.2006.38
Milatovic, D., Gupta, R. C., Yu, Y., Zaja-Milatovic, S., and Aschner, M. (2011). Protective effects of antioxidants and anti-inflammatory agents against manganese-induced oxidative damage and neuronal injury. Toxicol. Appl. Pharmacol. 256, 219–226. doi: 10.1016/j.taap.2011.06.001
Milatovic, D., Yin, Z., Gupta, R. C., Sidoryk, M., Albrecht, J., Aschner, J. L., et al. (2007). Manganese induces oxidative impairment in cultured rat astrocytes. Toxicol. Sci. 98, 198–205. doi: 10.1093/toxsci/kfm095
Milatovic, D., Zaja-Milatovic, S., Gupta, R. C., Yu, Y., and Aschner, M. (2009). Oxidative damage and neurodegeneration in manganese-induced neurotoxicity. Toxicol. Appl. Pharmacol. 240, 219–225. doi: 10.1016/j.taap.2009.07.004
Mogi, M., Harada, M., Kondo, T., Riederer, P., Inagaki, H., Minami, M., et al. (1994). Interleukin-1 beta, interleukin-6, epidermal growth factor and transforming growth factor-alpha are elevated in the brain from Parkinsonian patients. Neurosci. Lett. 180, 147–150. doi: 10.1016/0304-3940(94)90508-8
Morello, M., Canini, A., Mattioli, P., Sorge, R. P., Alimonti, A., Bocca, B., et al. (2008). Sub-cellular localization of manganese in the basal ganglia of normal and manganese-treated rats An electron spectroscopy imaging and electron energy-loss spectroscopy study. Neurotoxicology 29, 60–72. doi: 10.1016/j.neuro.2007.09.001
Moreno, J. A., Sullivan, K. A., Carbone, D. L., Hanneman, W. H., and Tjalkens, R. B. (2008). Manganese potentiates nuclear factor-kappaB-dependent expression of nitric oxide synthase 2 in astrocytes by activating soluble guanylate cyclase and extracellular responsive kinase signaling pathways. J. Neurosci. Res. 86, 2028–2038. doi: 10.1002/jnr.21640
Munson, P., Lam, Y. W., Macpherson, M., Beuschel, S., and Shukla, A. (2018). Mouse serum exosomal proteomic signature in response to asbestos exposure. J. Cell. Biochem. 119, 6266–6273. doi: 10.1002/jcb.26863
Murphy, V. A., Wadhwani, K. C., Smith, Q. R., and Rapoport, S. I. (1991). Saturable transport of manganese(II) across the rat blood-brain barrier. J. Neurochem. 57, 948–954. doi: 10.1111/j.1471-4159.1991.tb08242.x
Ng, E., Lind, P. M., Lindgren, C., Ingelsson, E., Mahajan, A., Morris, A., et al. (2015). Genome-wide association study of toxic metals and trace elements reveals novel associations. Hum. Mol. Genet. 24, 4739–4745. doi: 10.1093/hmg/ddv190
Ngalame, N. N. O., Luz, A. L., Makia, N., and Tokar, E. J. (2018). Arsenic alters exosome quantity and cargo to mediate stem cell recruitment into a cancer stem cell-like phenotype. Toxicol. Sci. 165, 40–49. doi: 10.1093/toxsci/kfy176
Oikawa, S., Hirosawa, I., Tada-Oikawa, S., Furukawa, A., Nishiura, K., and Kawanishi, S. (2006). Mechanism for manganese enhancement of dopamine-induced oxidative DNA damage and neuronal cell death. Free Radic. Biol. Med. 41, 748–756. doi: 10.1016/j.freeradbiomed.2006.05.018
Perl, D. P., and Olanow, C. W. (2007). The neuropathology of manganese-induced Parkinsonism. J. Neuropathol. Exp. Neurol. 66, 675–682. doi: 10.1097/nen.0b013e31812503cf
Perrett, R. M., Alexopoulou, Z., and Tofaris, G. K. (2015). The endosomal pathway in Parkinson’s disease. Mol. Cell. Neurosci. 66, 21–28. doi: 10.1016/j.mcn.2015.02.009
Pfeffer, S. R. (2010). Two Rabs for exosome release. Nat. Cell Biol. 12, 3–4. doi: 10.1038/ncb0110-3
Pfeiffer, R. F. (2009). Parkinson disease. Nonmotor symptoms in Parkinson disease: the PRIAMO study. Nat. Rev. Neurol. 5, 531–532. doi: 10.1038/nrneurol.2009.156
Poehler, A. M., Xiang, W., Spitzer, P., May, V. E., Meixner, H., Rockenstein, E., et al. (2014). Autophagy modulates SNCA/alpha-synuclein release, thereby generating a hostile microenvironment. Autophagy 10, 2171–2192. doi: 10.4161/auto.36436
Pohanka, M. (2012). Alpha7 nicotinic acetylcholine receptor is a target in pharmacology and toxicology. Int. J. Mol. Sci. 13, 2219–2238. doi: 10.3390/ijms13022219
Poulopoulos, M., Levy, O. A., and Alcalay, R. N. (2012). The neuropathology of genetic Parkinson’s disease. Mov. Disord. 27, 831–842. doi: 10.1002/mds.24962
Prabhakaran, K., Chapman, G. D., and Gunasekar, P. G. (2011). alpha-Synuclein overexpression enhances manganese-induced neurotoxicity through the NF-kappaB-mediated pathway. Toxicol. Mech. Methods 21, 435–443. doi: 10.3109/15376516.2011.560210
Priyadarshi, A., Khuder, S. A., Schaub, E. A., and Priyadarshi, S. S. (2001). Environmental risk factors and Parkinson’s disease: a metaanalysis. Environ. Res. 86, 122–127. doi: 10.1006/enrs.2001.4264
Przedborski, S., and Vila, M. (2003). The 1-methyl-4-phenyl-1,2,3,6-tetrahydropyridine mouse model: a tool to explore the pathogenesis of Parkinson’s disease. Ann. N. Y. Acad. Sci. 991, 189–198. doi: 10.1111/j.1749-6632.2003.tb07476.x
Racette, B. A., Antenor, J. A., Mcgee-Minnich, L., Moerlein, S. M., Videen, T. O., Kotagal, V., et al. (2005). [18F]FDOPA PET and clinical features in Parkinsonism due to manganism. Mov. Disord. 20, 492–496. doi: 10.1002/mds.20381
Rama Rao, K. V., Reddy, P. V., Hazell, A. S., and Norenberg, M. D. (2007). Manganese induces cell swelling in cultured astrocytes. Neurotoxicology 28, 807–812. doi: 10.1016/j.neuro.2007.03.001
Ramirez, A., Heimbach, A., Grundemann, J., Stiller, B., Hampshire, D., Cid, L. P., et al. (2006). Hereditary Parkinsonism with dementia is caused by mutations in ATP13A2, encoding a lysosomal type 5 P-type ATPase. Nat. Genet. 38, 1184–1191. doi: 10.1038/ng1884
Rasia, R. M., Bertoncini, C. W., Marsh, D., Hoyer, W., Cherny, D., Zweckstetter, M., et al. (2005). Structural characterization of copper(II) binding to alpha-synuclein: insights into the bioinorganic chemistry of Parkinson’s disease. Proc. Natl. Acad. Sci. U.S.A. 102, 4294–4299. doi: 10.1073/pnas.0407881102
Rodier, J. (1955). Manganese poisoning in Moroccan miners. Br. J. Ind. Med. 12, 21–35. doi: 10.1136/oem.12.1.21
Rosas, H. D., Chen, Y. I., Doros, G., Salat, D. H., Chen, N. K., Kwong, K. K., et al. (2012). Alterations in brain transition metals in Huntington disease: an evolving and intricate story. Arch. Neurol. 69, 887–893.
Ross, C. A., and Poirier, M. A. (2004). Protein aggregation and neurodegenerative disease. Nat. Med. 10(Suppl.), S10–S17.
Roth, J. A. (2014). Correlation between the biochemical pathways altered by mutated Parkinson-related genes and chronic exposure to manganese. Neurotoxicology 44, 314–325. doi: 10.1016/j.neuro.2014.08.006
Roth, J. A., Li, Z., Sridhar, S., and Khoshbouei, H. (2013). The effect of manganese on dopamine toxicity and dopamine transporter (DAT) in control and DAT transfected HEK cells. Neurotoxicology 35, 121–128. doi: 10.1016/j.neuro.2013.01.002
Salazar, J., Mena, N., Hunot, S., Prigent, A., Alvarez-Fischer, D., Arredondo, M., et al. (2008). Divalent metal transporter 1 (DMT1) contributes to neurodegeneration in animal models of Parkinson’s disease. Proc. Natl. Acad. Sci. U.S.A. 105, 18578–18583. doi: 10.1073/pnas.0804373105
Santos, D., Milatovic, D., Andrade, V., Batoreu, M. C., Aschner, M., and Marreilha Dos Santos, A. P. (2012). The inhibitory effect of manganese on acetylcholinesterase activity enhances oxidative stress and neuroinflammation in the rat brain. Toxicology 292, 90–98. doi: 10.1016/j.tox.2011.11.017
Sarban, S., Isikan, U. E., Kocabey, Y., and Kocyigit, A. (2007). Relationship between synovial fluid and plasma manganese, arginase, and nitric oxide in patients with rheumatoid arthritis. Biol. Trace Elem. Res. 115, 97–106. doi: 10.1007/bf02686022
Sarkar, S., Rokad, D., Malovic, E., Luo, J., Harischandra, D. S., Jin, H., et al. (2019). Manganese activates NLRP3 inflammasome signaling and propagates exosomal release of ASC in microglial cells. FASEB J. 12:eaat9900. doi: 10.1126/scisignal.aat9900
Sarko, D. K., and McKinney, C. E. (2017). Exosomes: origins and therapeutic potential for neurodegenerative disease. Front. Neurosci. 11:82. doi: 10.3389/fnins.2017.00082
Schapira, A. H. V., Chaudhuri, K. R., and Jenner, P. (2017). Non-motor features of Parkinson disease. Nat. Rev. Neurosci. 18, 435–450. doi: 10.1038/nrn.2017.62
Sengupta, A., Mense, S. M., Lan, C., Zhou, M., Mauro, R. E., Kellerman, L., et al. (2007). Gene expression profiling of human primary astrocytes exposed to manganese chloride indicates selective effects on several functions of the cells. Neurotoxicology 28, 478–489. doi: 10.1016/j.neuro.2006.10.005
Sepulveda, M. R., Vanoevelen, J., Raeymaekers, L., Mata, A. M., and Wuytack, F. (2009). Silencing the SPCA1 (secretory pathway Ca2+-ATPase isoform 1) impairs Ca2+ homeostasis in the Golgi and disturbs neural polarity. J. Neurosci. 29, 12174–12182. doi: 10.1523/JNEUROSCI.2014-09.2009
Shoji, M., Harigaya, Y., Sasaki, A., Ueda, K., Ishiguro, K., Matsubara, E., et al. (2000). Accumulation of NACP/alpha-synuclein in lewy body disease and multiple system atrophy. J. Neurol. Neurosurg. Psychiatry 68, 605–608. doi: 10.1136/jnnp.68.5.605
Shukla, G. S., and Chandra, S. V. (1981). Manganese toxicity: lipid peroxidation in rat brain. Acta Pharmacol. Toxicol. 48, 95–100. doi: 10.1111/j.1600-0773.1981.tb01594.x
Sidoryk-Wegrzynowicz, M., and Aschner, M. (2013). Manganese toxicity in the central nervous system: the glutamine/glutamate-gamma-aminobutyric acid cycle. J. Intern. Med. 273, 466–477. doi: 10.1111/joim.12040
Stephenson, A. P., Schneider, J. A., Nelson, B. C., Atha, D. H., Jain, A., Soliman, K. F., et al. (2013). Manganese-induced oxidative DNA damage in neuronal SH-SY5Y cells: attenuation of thymine base lesions by glutathione and N-acetylcysteine. Toxicol. Lett. 218, 299–307. doi: 10.1016/j.toxlet.2012.12.024
Su, X., Maguire-Zeiss, K. A., Giuliano, R., Prifti, L., Venkatesh, K., and Federoff, H. J. (2008). Synuclein activates microglia in a model of Parkinson’s disease. Neurobiol. Aging 29, 1690–1701. doi: 10.1016/j.neurobiolaging.2007.04.006
Takagi, H., Uehara, M., Sakurai, S., Yamada, S., Takayama, H., Saitoh, S., et al. (1990). [Evaluation of non-surgical treatments of hepatocellular carcinoma–investigation of the cases with long and short survivals after treatment]. Nihon Gan Chiryo Gakkai Shi 25, 757–769.
Tan, J., Zhang, T., Jiang, L., Chi, J., Hu, D., Pan, Q., et al. (2011). Regulation of intracellular manganese homeostasis by Kufor-Rakeb syndrome-associated ATP13A2 protein. J. Biol. Chem. 286, 29654–29662. doi: 10.1074/jbc.M111.233874
Tansey, M. G., Frank-Cannon, T. C., Mccoy, M. K., Lee, J. K., Martinez, T. N., Mcalpine, F. E., et al. (2008). Neuroinflammation in Parkinson’s disease: is there sufficient evidence for mechanism-based interventional therapy? Front. Biosci. 13, 709–717. doi: 10.1007/s11481-009-9176-0
Taylor, C. A., Hutchens, S., Liu, C., Jursa, T., Shawlot, W., Aschner, M., et al. (2019). SLC30A10 transporter in the digestive system regulates brain manganese under basal conditions while brain SLC30A10 protects against neurotoxicity. J. Biol. Chem. 294, 1860–1876. doi: 10.1074/jbc.RA118.005628
Thery, C., Zitvogel, L., and Amigorena, S. (2002). Exosomes: composition, biogenesis and function. Nat. Rev. Immunol. 2, 569–579. doi: 10.1038/nri855
Thompson, K., Molina, R. M., Donaghey, T., Schwob, J. E., Brain, J. D., and Wessling-Resnick, M. (2007). Olfactory uptake of manganese requires DMT1 and is enhanced by anemia. FASEB J. 21, 223–230. doi: 10.1096/fj.06-6710com
Tjalve, H., Henriksson, J., Tallkvist, J., Larsson, B. S., and Lindquist, N. G. (1996). Uptake of manganese and cadmium from the nasal mucosa into the central nervous system via olfactory pathways in rats. Pharmacol. Toxicol. 79, 347–356. doi: 10.1111/j.1600-0773.1996.tb00021.x
Tottey, S., Waldron, K. J., Firbank, S. J., Reale, B., Bessant, C., Sato, K., et al. (2008). Protein-folding location can regulate manganese-binding versus copper- or zinc-binding. Nature 455, 1138–1142. doi: 10.1038/nature07340
Troadec, M. B., Ward, D. M., Lo, E., Kaplan, J., and De Domenico, I. (2010). Induction of FPN1 transcription by MTF-1 reveals a role for ferroportin in transition metal efflux. Blood 116, 4657–4664. doi: 10.1182/blood-2010-04-278614
Tuschl, K., Clayton, P. T., Gospe, S. M. Jr., Gulab, S., Ibrahim, S., Singhi, P., et al. (2012). Syndrome of hepatic cirrhosis, dystonia, polycythemia, and hypermanganesemia caused by mutations in SLC30A10, a manganese transporter in man. Am. J. Hum. Genet. 90, 457–466. doi: 10.1016/j.ajhg.2012.01.018
Uchikado, H., Lin, W. L., Delucia, M. W., and Dickson, D. W. (2006). Alzheimer disease with amygdala Lewy bodies: a distinct form of alpha-synucleinopathy. J. Neuropathol. Exp. Neurol. 65, 685–697. doi: 10.1097/01.jnen.0000225908.90052.07
Uversky, V. N., Li, J., and Fink, A. L. (2001). Metal-triggered structural transformations, aggregation, and fibrillation of human alpha-synuclein. A possible molecular NK between Parkinson’s disease and heavy metal exposure. J. Biol. Chem. 276, 44284–44296. doi: 10.1074/jbc.m105343200
Verina, T., Kiihl, S. F., Schneider, J. S., and Guilarte, T. R. (2011). Manganese exposure induces microglia activation and dystrophy in the Substantia nigra of non-human primates. Neurotoxicology 32, 215–226. doi: 10.1016/j.neuro.2010.11.003
Verina, T., Schneider, J. S., and Guilarte, T. R. (2013). Manganese exposure induces alpha-synuclein aggregation in the frontal cortex of non-human primates. Toxicol. Lett. 217, 177–183. doi: 10.1016/j.toxlet.2012.12.006
Wang, C. Y., Jenkitkasemwong, S., Duarte, S., Sparkman, B. K., Shawki, A., Mackenzie, B., et al. (2012). ZIP8 is an iron and zinc transporter whose cell-surface expression is up-regulated by cellular iron loading. J. Biol. Chem. 287, 34032–34043. doi: 10.1074/jbc.M112.367284
Wang, T., Li, X., Yang, D., Zhang, H., Zhao, P., Fu, J., et al. (2015). ER stress and ER stress-mediated apoptosis are involved in manganese-induced neurotoxicity in the rat striatum in vivo. Neurotoxicology 48, 109–119. doi: 10.1016/j.neuro.2015.02.007
Wedler, F. C., and Denman, R. B. (1984). Glutamine synthetase: the major Mn(II) enzyme in mammalian brain. Curr. Top. Cell. Regul. 24, 153–169.
Wedler, F. C., Vichnin, M. C., Ley, B. W., Tholey, G., Ledig, M., and Copin, J. C. (1994). Effects of Ca(II) ions on Mn(II) dynamics in chick glia and rat astrocytes: potential regulation of glutamine synthetase. Neurochem. Res. 19, 145–151. doi: 10.1007/bf00966809
Whittaker, V. P. (1990). The contribution of drugs and toxins to understanding of cholinergic function. Trends Pharmacol. Sci. 11, 8–13. doi: 10.1016/0165-6147(90)90034-6
Williams, B. B., Kwakye, G. F., Wegrzynowicz, M., Li, D., Aschner, M., Erikson, K. M., et al. (2010). Altered manganese homeostasis and manganese toxicity in a Huntington’s disease striatal cell model are not explained by defects in the iron transport system. Toxicol. Sci. 117, 169–179. doi: 10.1093/toxsci/kfq174
Wittung-Stafshede, P. (2002). Role of cofactors in protein folding. Acc. Chem. Res. 35, 201–208. doi: 10.1021/ar010106e
Wong, B. S., Chen, S. G., Colucci, M., Xie, Z., Pan, T., Liu, T., et al. (2001). Aberrant metal binding by prion protein in human prion disease. J. Neurochem. 78, 1400–1408. doi: 10.1046/j.1471-4159.2001.00522.x
Xu, B., Wu, S. W., Lu, C. W., Deng, Y., Liu, W., Wei, Y. G., et al. (2013). Oxidative stress involvement in manganese-induced alpha-synuclein oligomerization in organotypic brain slice cultures. Toxicology 305, 71–78. doi: 10.1016/j.tox.2013.01.006
Yan, D. Y., Liu, C., Tan, X., Ma, Z., Wang, C., Deng, Y., et al. (2019). Mn-induced neurocytes injury and autophagy dysfunction in alpha-synuclein wild-type and knock-out mice: highlighting the role of alpha-synuclein. Neurotox. Res. doi: 10.1007/s12640-019-00016-y [Epub ahead of print].
Yin, Z., Jiang, H., Lee, E. S., Ni, M., Erikson, K. M., Milatovic, D., et al. (2010). Ferroportin is a manganese-responsive protein that decreases manganese cytotoxicity and accumulation. J. Neurochem. 112, 1190–1198. doi: 10.1111/j.1471-4159.2009.06534.x
Zhang, D., Kanthasamy, A., Anantharam, V., and Kanthasamy, A. (2011). Effects of manganese on tyrosine hydroxylase (TH) activity and TH-phosphorylation in a dopaminergic neural cell line. Toxicol. Appl. Pharmacol. 254, 65–71. doi: 10.1016/j.taap.2010.03.023
Zhang, J., Perry, G., Smith, M. A., Robertson, D., Olson, S. J., Graham, D. G., et al. (1999). Parkinson’s disease is associated with oxidative damage to cytoplasmic DNA and RNA in Substantia nigra neurons. Am. J. Pathol. 154, 1423–1429. doi: 10.1016/s0002-9440(10)65396-5
Zhang, S., Zhou, Z., and Fu, J. (2003). Effect of manganese chloride exposure on liver and brain mitochondria function in rats. Environ. Res. 93, 149–157. doi: 10.1016/s0013-9351(03)00109-9
Keywords: manganese neurotoxicity, Parkinson’s disease, protein aggregation, exosome, cell-to-cell transmission and neuroinflammation
Citation: Harischandra DS, Ghaisas S, Zenitsky G, Jin H, Kanthasamy A, Anantharam V and Kanthasamy AG (2019) Manganese-Induced Neurotoxicity: New Insights Into the Triad of Protein Misfolding, Mitochondrial Impairment, and Neuroinflammation. Front. Neurosci. 13:654. doi: 10.3389/fnins.2019.00654
Received: 10 April 2019; Accepted: 06 June 2019;
Published: 26 June 2019.
Edited by:
Krishnan Prabhakaran, Norfolk State University, United StatesReviewed by:
Aaron B. Bowman, Purdue University, United StatesMaria Xilouri, Biomedical Research Foundation of the Academy of Athens, Greece
Copyright © 2019 Harischandra, Ghaisas, Zenitsky, Jin, Kanthasamy, Anantharam and Kanthasamy. This is an open-access article distributed under the terms of the Creative Commons Attribution License (CC BY). The use, distribution or reproduction in other forums is permitted, provided the original author(s) and the copyright owner(s) are credited and that the original publication in this journal is cited, in accordance with accepted academic practice. No use, distribution or reproduction is permitted which does not comply with these terms.
*Correspondence: Anumantha G. Kanthasamy, YWthbnRoYXNAaWFzdGF0ZS5lZHU=
†Present address: Dilshan S. Harischandra, Covance Greenfield Laboratories, Greenfield, IN, United States Shivani Ghaisas, Department of Cancer Biology, University of Pennsylvania, Philadelphia, PA, United States