- 1Guangdong-Hong Kong-Macau Institute of CNS Regeneration, Jinan University, Guangzhou, China
- 2Kunming Institute of Zoology, Chinese Academy of Sciences, Kunming, China
- 3Department of Biology, Huck Institutes of the Life Sciences, Pennsylvania State University, University Park, PA, United States
Adult neurogenesis has been extensively studied in rodent animals, with distinct niches found in the hippocampus and subventricular zone (SVZ). In non-human primates and human postmortem samples, there has been heated debate regarding adult neurogenesis, but it is largely agreed that the rate of adult neurogenesis is much reduced comparing to rodents. The limited adult neurogenesis may partly explain why human brains do not have self-repair capability after injury or disease. A new technology called “in vivo cell conversion” has been invented to convert brain internal glial cells in the injury areas directly into functional new neurons to replenish the lost neurons. Because glial cells are abundant throughout the brain and spinal cord, such engineered glia-to-neuron conversion technology can be applied throughout the central nervous system (CNS) to regenerate new neurons. Thus, compared to cell transplantation or the non-engineered adult neurogenesis, in vivo engineered neuroregeneration technology can provide a large number of functional new neurons in situ to repair damaged brain and spinal cord.
Introduction
Human brain has billions of neurons and even more number of glial cells. Neurons cannot divide and therefore they do not self-regenerate after injury, but glial cells can proliferate upon injury or disease. Human neurons inside the brain are believed to live most of our whole life and cannot be replaced once being damaged or degenerated. It is perhaps due to this doctrine that the first finding of adult neurogenesis in rodent animals in 1960’s (Altman and Das, 1965) was not well recognized until decades later. Nevertheless, the identification of newborn neurons in human postmortem brains (Eriksson et al., 1998) did trigger greater enthusiasm in searching for internal neural stem cells for brain repair. After two decades of research, it is found that adult neurogenesis in mammalian brains is largely restricted to a few discrete niches such as the hippocampus and the subventricular zone (Ming and Song, 2011). Some studies suggest a possibility of neuroprogenitor cells migrating toward injury sites to differentiate into neurons, but further lineage tracing studies demonstrate that the in vivo differentiated cells are mainly glial cells rather than neurons (Buffo et al., 2008; Faiz et al., 2015). Thus, a consensus is that while mammalian brains have adult neurogenesis, the number of newborn neurons is likely limited in a few regions, making it difficult to repair damaged brains.
To overcome the limitation of endogenous neurogenesis, scientists transplanted external stem cells into the brain or spinal cord in order to regenerate new neurons in any injured areas regardless whether it is close to a neurogenic niche or not. This typically involves in vitro cell cultures to expand the stem cells or even partially differentiate the stem cells toward some fate-determined neuroprogenitor cells. This in vitro cell culture followed with in vivo transplantation is referred here as “in vitro engineered neurogenesis.” Recently, a new technology called “in vivo cell conversion technology” has been invented by making use of internal glial cells to generate new neurons (Niu et al., 2013; Torper et al., 2013; Guo et al., 2014; Su et al., 2014), eliminating the in vitro steps of cell culture and the following transplantation procedures. Because glial cells are distributed throughout the brain and spinal cord, this in vivo cell conversion technology can be applied anywhere in the central nervous system (CNS), and referred here as “in vivo engineered neurogenesis.” While still in its early stage, this in vivo cell conversion technology already shows some promise in brain and spinal cord repair. This review will focus on this new emerging technology of in vivo engineered neurogenesis and compare with in vitro engineered neurogenesis as well as the endogenous neurogenesis in the hippocampus and subventricular zone.
Endogenous Neurogenesis
Adult Neurogenesis in Rodents
Half century ago, researchers provided the first evidence of neurogenesis in postnatal rats (Altman and Das, 1965). It took more than a decade to confirm neurogenesis in adult rats (Kaplan and Hinds, 1977; Bayer et al., 1982) and adult mice (Reynolds and Weiss, 1992; Lois and Alvarez-Buylla, 1993; Lois and Alvarez-Buylla, 1994). Intriguingly, the magnitude of adult-generated neurons in the rat dentate gyrus is elevated significantly by the hippocampal dependent associative learning tasks (Gould et al., 1999a). High dose BrdU injection suggested a large pool of new neurons being added in the dentate gyrus of young adult rats every day (Cameron and McKay, 2001). While the hippocampus and subventricular zone (SVZ) are two well-documented neurogenic niches in adult rodents, a small number of BrdU-labeled neurons were also found in other brain regions (Zhao et al., 2003; Dayer et al., 2005; Kokoeva et al., 2005). The adult neurogenesis can be regulated by a variety of signaling pathways including the Noggin/Bone Morphogenetic Protein (BMP) signaling (Lim et al., 2000), Wnt signaling (Kuwabara et al., 2009), the activity-induced immediate early gene Gadd45b (Ma et al., 2009), a brain-enriched microRNA miR-124 (Cheng et al., 2009), methyl-CpG–binding protein 2 (MeCP2) (Szulwach et al., 2010), and other factors (Braun and Jessberger, 2014; Goncalves et al., 2016). Furthermore, many SGZ newborn neurons undergo apoptosis during the first few days of life, leaving a small amount surviving in the adult brain (Sierra et al., 2010). Those surviving newborn neurons in adult rodents are believed to contribute to many brain functions including but not limited to olfactory- and hippocampus-dependent learning and memory (Aimone et al., 2006, 2011; Akers et al., 2014). Moreover, adult neurogenesis may play crucial roles in other cognitive functions like spatial and object recognition and fear conditioning, and correlate with disease processes such as major depression, epilepsy and major affective disorders (Cameron and Glover, 2015; Lepousez et al., 2015; Sailor et al., 2017). Recent studies using single-cell RNA-seq successfully characterized the genome-wide molecular signatures of adult NSCs in mouse dentate gyrus (Shin et al., 2015), and revealed that dormant ependymal NSCs are located in the lateral and the fourth ventricles (Luo et al., 2015). Over the past several decades, we have gained much advanced understandings on the endogenous adult neurogenesis in rodent animals (for more comprehensive review, please see Ming and Song, 2011; Goncalves et al., 2016; Lim and Alvarez-Buylla, 2016).
Adult Neurogenesis in Non-human Primates
In the 1980s, Rakic and colleagues using 3H-thymidine autoradiographic technique found newborn neurons in the SGZ of rhesus monkeys before and after birth, but could not detect newborn neurons after puberty (Rakic, 1985; Eckenhoff and Rakic, 1988). Later, via BrdU labeling method, Kornack and Rakic found some newborn neurons in the GCL in adult macaque monkeys, but the relative rate of neurogenesis is approximately 10 times less than the rodents (Kornack and Rakic, 1999). Gould and Tanapat (1999), Gould et al. (1999b) also demonstrated adult neurogenesis in the hippocampus and SVZ of both marmoset monkeys and Old-World monkeys. The rostral migratory stream (RMS), a migratory route in the brain along which neuronal precursors originated in the subventricular zone migrate to reach the olfactory bulb, has been reported in the adult monkey, similar to that in rodents (Kornack and Rakic, 2001b; Sawamoto et al., 2011). Moreover, newborn neurons are also reported in the amygdala, piriform cortex, and adjoining inferior temporal cortex in adult primates (Bernier et al., 2002), but the adult neurogenesis in monkey neocortex remains controversial (Gould and Tanapat, 1999; Gould et al., 2001; Kornack and Rakic, 2001a). Although there are only limited studies in non-human primates, there seems to be a small number of newborn neurons detectable in adult primates, but the rate of neurogenesis is greatly reduced comparing to adult rodents (Sorrells et al., 2018).
Adult Neurogenesis in Humans
It is even more controversial regarding adult neurogenesis in humans. The first evidence of human adult neurogenesis was provided in 1998, when Gage and colleagues discovered the presence of BrdU-labeled neurons in the hippocampus of cancer patients receiving BrdU for diagnosis purpose (Eriksson et al., 1998). Since then, increasing studies have documented the extent of adult neurogenesis in humans, but the overall rate of neurogenesis in humans sharply declines after birth and are nearly absent in adulthood (Sanai et al., 2011; Sorrells et al., 2018). Curtis et al. (2007) examined the brains of deceased cancer patients, who had previously been injected with BrdU, and found BrdU-positive cells in the patients’ olfactory bulbs, suggesting that RMS might exist in adult humans. However, this was challenged by Sanai et al. (2011), who used a proliferation marker Ki67 and the immature neuronal marker DCX to show that SVZ neurogenesis and migration along the RMS ceased by 2 years after birth in humans. More recent studies demonstrated that the neuroblasts generated in the human SVZ seldom migrate to the olfactory bulb, but rather to the nearby striatum (Bergmann et al., 2012; Ernst et al., 2014).
Hippocampal neurogenesis in adult human brains is also highly debated. Carbon birth-dating method implicated that hundreds of newborn neurons might be added to the adult human dentate gyrus (DG) per day with a modest decline during aging (Spalding et al., 2013), but immunocytochemistry analysis found an age-related sharp decrease of proliferating neuroblasts and putative newborn neurons (Knoth et al., 2010; Dennis et al., 2016). Recently, two prominent studies with similar data but opposite conclusions have re-ignited the debate. Sorrells et al. (2018) found that human hippocampal neurogenesis dropped sharply in children (7–13 years old) and to almost undetectable level in adults (18–77 years), whereas Boldrini et al. (2018) found existence of newborn neurons not only in young age samples but also from old patient samples (14–79 years old). Both studies used similar markers for proliferating neuroprogenitors and immature neurons but drew opposite conclusions, although the actual data was quite similar—a few thousands of newborn neurons in the adult human hippocampus. Given billions of neurons in the human brain, whether these thousands of neurons can carry out a specific function remains a question to be answered.
We have summarized the well-documented sites of adult neurogenesis in mammalian brains (Figure 1). Human brain has limited adult neurogenesis sites.
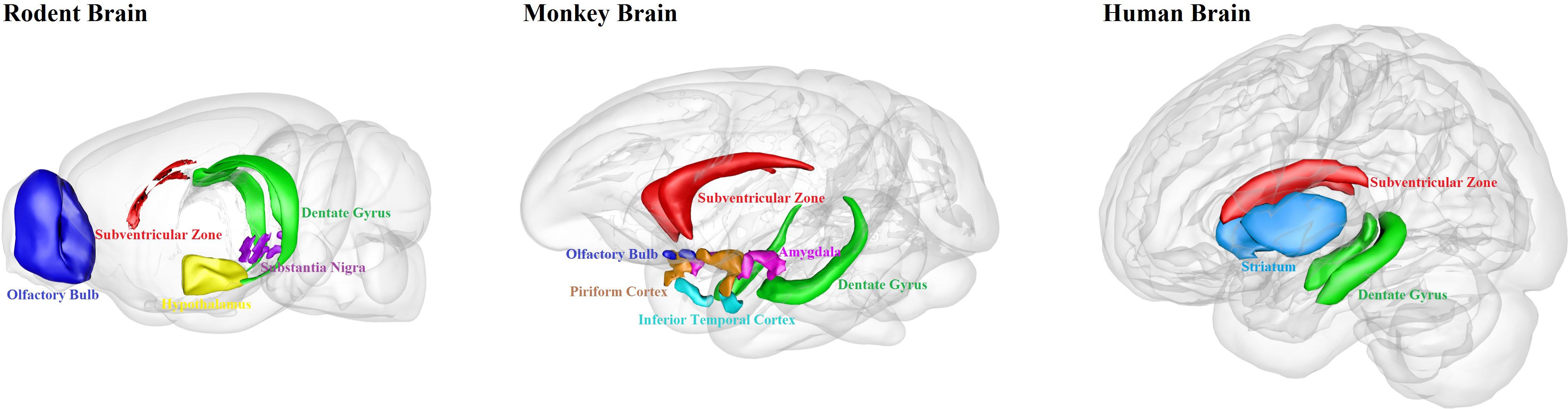
Figure 1. Schematic illustration of adult neurogenesis sites in the brains of rodent, monkey and human. Neurogenesis takes place throughout life in the hippocampal dentate gyrus and the subventricular zone in rodent, monkey and human brains. Newborn cells from the subventricular zone migrate to the olfactory bulb in rodents and monkeys, but maybe not in humans. Adult neurogenesis has also been found in some other brain regions, such as hypothalamus and substantia nigra in rodents; amygdala, piriform cortex and inferior temporal cortex in monkeys; and striatum in humans. This figure is inspired by the Scalable Brain Atlas website and its 3-D Composer.
When comparing adult neurogenesis among rodents, primates, and humans, one can find that hippocampal neurogenesis is conserved among mammals, but there are still differences between rodents and primates. The distribution of cell population and the cytoarchitecture of the SVZ is different between rodents and primates (Sanai et al., 2004, 2011). In primates, the SVZ have a three-layer organization, with an ependymal layer, a hypocellular gap layer, and an astrocyte ribbon layer; in contrast, adult mice do not have the hypocellular gap layer, and the mouse SVZ is composed of neuroblasts, glial cells, and precursor cells (Brus et al., 2013). Moreover, there are large differences between humans and other mammals in the output of new neurons from the SVZ. In rodents and non-human primates, neuroblasts coming from the SVZ migrate to the OB. However, humans do not have obvious addition of new neurons in the OB after 2 years of birth (Bergmann et al., 2012). Lastly, only a few new neuroblasts appear to be produced in the SVZ in humans (Ernst and Frisen, 2015). In humans dentate gyrus, most of the neurons might have been replaced after birth, compared to only ∼10% replaced in mice at birth (Spalding et al., 2013). On the other hand, the maturation of newborn neurons takes much longer time in primates, making the adult-born neurons more difficult to survive than the neurons born during embryonic development (Kohler et al., 2011). Together, we should be cautious in extrapolating the findings on adult neurogenesis from rodents to primates, and in particular not to imply any direct clinical applications for humans.
In Vitro Engineered Neurogenesis
Besides relying upon endogenous neural stem cells to generate new neurons, transplantation of external cells into the brain and spinal cord has been explored as an alternative approach for neuroregeneration. The major external cell source for transplantation includes embryonic stem cells (ESCs), mesenchymal stem cells (MSCs), induced pluripotent stem cells (iPSCs), organoids, and other in vitro differentiated or trans-differentiated cells (Yang et al., 2011; Trounson and McDonald, 2015). Transplantation of external cells into the brain has faced tremendous technical challenges such as immunorejection, long-term survival and functional integration, as well as tumorigenesis (Trounson and McDonald, 2015; Goldman, 2016). This review will briefly summarize the recent progress on in vitro engineered neurogenesis, putting more emphasis on in vivo engineered neurogenesis in the next section.
ESC/iPSC Technology
Embryonic stem cells are expandable in vitro and able to differentiate into functional neuronal subtypes in defined medium. Their efficacy to alleviate neurological abnormalities has been proven in a number of animal models, and clinical trials using ESC-derived neural cells have been initiated to treat several neurological disorders such as macular degeneration, Parkinson’s disease and spinal cord injury (Trounson and McDonald, 2015). iPSCs are reprogrammed from somatic cells (Takahashi and Yamanaka, 2006; Takahashi et al., 2007) with defined factors or small molecules, and can be differentiated into a variety of neuronal subtypes but circumvent the immunorejection and ethical issues faced by ESCs (Martin, 2017). The first clinical trial using iPSC-derived retinal pigment epithelium was conducted in Japan on a patient suffering from age-related macular degeneration (Cyranoski, 2014). Recently, new clinical trial using iPSC-derived dopaminergic neurons to treat Parkinson’s patients has been announced in Japan after successful preclinical studies in monkeys (Kikuchi et al., 2017). Engrafted stem cell-derived neurons expressing channel rhodopsin or DREADDs (designer receptors exclusively activated by designer drugs) can be activated in an optical or chemical-controllable fashion to modulate mouse motor functions (Bryson et al., 2014; Steinbeck et al., 2015; Chen et al., 2016), suggesting that some of the transplanted cells have integrated into the preexisting circuits. On the other hand, it should be cautioned that for wide-spreading neurodegenerative disorders such as Alzheimer’s disease, it is more difficult for cell transplantation to reach every corner of the degenerating brain.
In vitro Neuronal Trans-Differentiation
The idea of using transcriptional factors to reprogram somatic cells into stem cells (Takahashi and Yamanaka, 2006; Takahashi et al., 2007) has inspired a new field called “cell trans-differentiation,” where almost any cell type can be reprogrammed or trans-differentiated into a different cell type (Vierbuchen et al., 2010; Tsunemoto et al., 2015, 2018). Vierbuchen et al. (2010) first reported a groundbreaking work in converting skin fibroblast cells into induced neurons (iNs) using three transcription factors Ascl1, Brn2, and Myt1l. Since then, various subtypes of iNs including glutamatergic, GABAergic, dopaminergic, motor neurons, and retinal neurons have been successfully trans-differentiated from a number of initial somatic cells such as fibroblasts, hepatocytes, pericytes, astrocytes, and peripheral T cells (Heinrich et al., 2010; Addis et al., 2011; Caiazzo et al., 2011; Marro et al., 2011; Pfisterer et al., 2011; Son et al., 2011; Yoo et al., 2011; Giorgetti et al., 2012; Karow et al., 2012; Ladewig et al., 2012; Liu et al., 2013; Xue et al., 2013; Victor et al., 2014; Colasante et al., 2015; Li et al., 2015; Zhang et al., 2015; Tanabe et al., 2018). iNs bypass the process of iPSCs and have minimal tumorigenic risk. In terms of cell replacement, there are already a few reports on the transplantation of iNs in vivo (Kim et al., 2011; Son et al., 2011; Colasante et al., 2015; Hu et al., 2015; Mertens et al., 2015), but more comprehensive evaluations are required before clinical translation. One interesting study found that iNs converted from fibroblasts of elder donor preserved the cellular signature of aging, suggesting that iNs may be a better in vitro model than iPSCs to study aging (Mertens et al., 2015).
Brain Organoids
Neuroprogenitors differentiated from iPSCs can self-organize in vitro to form 3D complex structures containing areas resembling diverse regions of the brain (Di Lullo and Kriegstein, 2017; Amin and Pasca, 2018; Pasca, 2018). These structures are named “brain organoids.” Different region-specific organoids can fuse together to develop brain assembloids (Di Lullo and Kriegstein, 2017; Pasca, 2018). Brain organoids hold promise to accelerate our understanding of human brain development and brain disorders due to their several distinct advantages. First, human brain organoids display specific features of human brain development that are absent in rodent models, such as the presence of outer radial glial cells (Di Lullo and Kriegstein, 2017). Second, brain organoids generated from patient-derived iPSCs can better recapitulate the structural and functional complexity of the human brain than the 2D monolayer systems, and thus more precisely modeling neurological disorders for mechanistic studies or for drug screening (Amin and Pasca, 2018). Third, brain organoids can be manipulated with genome editing techniques using CRISPR-Cas9 before being transplanted into animals to further study in vivo development (Pasca, 2018). On the other hand, brain organoids also have limitations. They are typically in an early phase of development, and thus may fail to model some later developmental events or late-onset disease phenotypes (Di Lullo and Kriegstein, 2017). Additionally, the extent of diverse cell types in the organoids so far has not been fully validated and the reproducibility of compositions is poor (Amin and Pasca, 2018). Moreover, the transcriptional identity of some cells in the organoids is not closely correlated to an in vivo counterpart (Di Lullo and Kriegstein, 2017). Finally, brain organoids currently lack some of the cell types such as endothelial cells and microglia, which are involved in normal brain development and circuit function (Di Lullo and Kriegstein, 2017; Pasca, 2018). Future works are required to overcome these limitations.
In Vivo Engineered Neurogenesis
Engineered Adult Neurogenesis Through Glia-to-Neuron Conversion
To overcome the limitation of endogenous neurogenesis and the disadvantages of external cell transplantation, in vivo engineered neurogenesis has been brought to the front stage in recent years by making use of internal glial cells to generate neurons through in vivo glia-to-neuron conversion technology. Importantly, unlike the endogenous neurogenesis that is restricted in certain niches, in vivo engineered neurogenesis can be induced in any places throughout the brain and spinal cord (Li and Chen, 2016). Moreover, different subtypes of neurons have been regenerated in different brain regions as discussed below.
In vivo Reprogramming Glial Cells Into Glutamatergic Neurons
Neuroprogenitor cells (NPCs) are known to express certain glial markers such as GFAP, and astrocytes after injury can express certain NPC markers such as Nestin and Sox2. Such observation once confused some scientists that glial cells might be a kind of NPC-like cells, but lineage-tracing studies found that glial cells rarely generate neurons in vivo (Buffo et al., 2008; Faiz et al., 2015). However, overexpression of a mutant transcription factor Olig2 in the stab-wound mouse cortex (Buffo et al., 2005) or overexpressing neurogenin 2 (Ngn2) and Ascl1 (Mash1) in injured spinal cord (Ohori et al., 2006) both generated immature neurons, suggesting that it might be possible to directly engineer adult neurogenesis in vivo. At that time, it was not clear whether new neurons were generated from NPCs or glial cells, because they share some common properties (Gotz et al., 2015). Later, Berninger et al. (2007) demonstrated that single transcription factor neurogenin-2 (Ngn2) could convert cultured postnatal cortical astroglia into glutamatergic neurons in vitro. After repeating the conversion of cultured astrocytes into neurons induced by Ngn2 in cell cultures, we further tested whether it is possible to directly convert astrocytes into neurons inside mouse brains in vivo. It was a bit disappointing that injecting retroviruses expressing Ngn2 alone in adult mouse cortex in vivo yielded very few newborn neurons. However, after replacing Ngn2 with a different transcription factor NeuroD1, we observed high efficiency conversion (90%) of reactive astrocytes into glutamatergic neurons in adult mouse cortex (Guo et al., 2014). More importantly, unlike immature neurons reported earlier, NeuroD1-converted neurons are fully functional, showing repetitive action potentials and robust synaptic responses that are similar to their neighboring neurons (Guo et al., 2014). NeuroD1 can also convert NG2 cells into mostly glutamatergic neurons, with a small population being GABAergic neurons (Guo et al., 2014). A most recent study using lentivirus and CD68 promoter to drive NeuroD1 overexpression in striatal microglia also converted them into neurons (Matsuda et al., 2019), whereas we previously used retrovirus and CAG promoter to express NeuroD1 but could not convert cortical microglia into neurons (Guo et al., 2014), raising an interesting question regarding different viruses and promoters in affecting in vivo cell conversion in different brain regions. Another surprising finding about NeuroD1 is that even in 14-month old Alzheimer’s disease mouse model (5xFAD), NeuroD1 can still efficiently convert reactive astrocytes into functional neurons, characterized by highly functional electrophysiological properties (Guo et al., 2014). Obtaining functional neurons in the adult brain is critical, because immature neurons often cannot survive well in the adult brain, and this is especially true for neurons in an injured or diseased environment (Buss et al., 2006). Generating a large number of functional new neurons in injured brains is the first step toward functional repair.
Different from the NeuroD1-mediated direct glia-to-neuron conversion, Zhang and colleagues reported successful neuroregeneration from astrocytes using a neural stem cell factor Sox2 to reprogram astrocytes back to neuroblasts and then further differentiate into neurons in both brain and spinal cord (Niu et al., 2013, 2015; Su et al., 2014; Islam et al., 2015; Wang et al., 2016). Importantly, these converted neurons are also electrophysiologically functional (Niu et al., 2013). The advantage of this approach is the potential of generating multiple neurons by reversing astrocytes back to neuroblast cells. The disadvantage is a two-step approach with additional factors needed for further neuronal differentiation such as BDNF and Noggin, VPA, or silencing p53. It is worth to note that the successful production of a significant number of mature and functional neurons in the adult spinal cord may provide a potential regeneration-based treatment for spinal cord injury. Further studies are warranted to generate subtype-specific neurons such as motor neurons to facilitate motor functional recovery after spinal cord injury.
The expression of Ascl1 was shown to convert astrocytes into functional glutamatergic and GABAergic neurons in dorsal midbrain, striatum, and somatosensory cortex of postnatal and adult mice in vivo (Liu et al., 2015). A combination of three transcription factors Ascl1, Lmx1a, and Nurr1 could turn striatal NG2 glia into mainly GABAergic neurons and a small proportion of glutamatergic neurons (Torper et al., 2015). Combining Ascl1 with Sox2 has been reported to transform NG2 glia into relatively immature neurons (mostly Doublecortin+) in the stab-injured cortex of adult mice in vivo (Heinrich et al., 2014). Ngn2 alone showed low conversion efficiency in vivo, but addition of Bcl-2 and anti-oxidative treatment (such as vitamin E and α-Tocotrienol) together with Ngn2 was reported to enhance conversion efficiency significantly, suggesting that oxidative stress may act as a major hurdle for cell conversion (Gascon et al., 2016).
It is worth noting that neurons not only can be reprogrammed from non-neuronal cells but also from other subtypes of neurons. For instance, overexpression of transcription factor Fezf2 can differentiate GABAergic progenitors in the mouse striatum into glutamatergic corticofugal projection neurons (Rouaux and Arlotta, 2010). Similarly, overexpression of Fezf2 in cortical layer IV neurons (De la Rossa et al., 2013) or layer II/III neurons (Rouaux and Arlotta, 2013) can change their identity into layer-V/VI corticofugal neurons. A recent study by Zhang and colleagues converted mouse striatal GABAergic neurons into dopaminergic neurons using a cocktail of factors including SOX2, Nurr1, Lmx1a, and Foxa2, and VPA (Niu et al., 2018). Changing one neuron subtype into another might cause unwanted outcome such as tilting the excitation-inhibition (E-I) balance (Ye et al., 2015). Because neurons cannot divide but glial cells can, it is ideal to use glial cells as conversion source instead of changing preexisting neurons.
In vivo Reprogramming Glial Cells Into GABAergic Neurons
GABAergic interneurons (INs) (γ-aminobutyric acid secreting neurons), constituting around 10–20% of all neurons in the brain, are critical for balancing the excitatory and inhibitory tone in neural networks (Caputi et al., 2013). The breakdown of E-I balance causes epilepsy, depression, schizophrenia, and other neurological disorders (Caputi et al., 2013). Using various pro-neuronal transcriptional factors (TFs), GABAergic INs have been in vivo converted from different types of non-neuronal cells. We reported that about 10% of glia-converted neurons after ectopic expression of NeuroD1 in reactive glial cells were GABAergic neurons (Guo et al., 2014). Neurogenin-2 combined with growth factors (FGF2 and EGF) were able to covert proliferative non-neuronal cells residing in stab-injured rat striatum into mainly GABAergic neurons, but the total reprogramming efficiency was quite low (∼4%) (Grande et al., 2013). To increase the reprogramming efficiency, other TFs or TFs combined with small molecules were explored. Niu et al. (2015) found that transiently over-expressing Sox2 in the striatal resident astrocytes and subsequently treating with VPA could induce calretinin+ INs. Besides astrocytes, striatal NG2 glia cells could also be targeted. Ascl1, Lmx1a, and Nurr1 could successfully convert NG2 glial cells into GAD65/67+ neurons in intact striatum (Torper et al., 2015), and predominately fast-spiking parvalbumin+ (PV) neurons in the striatum (Pereira et al., 2017). In addition, oligodendrocytes in the striatum were reported to be reprogrammed into striatal neurons (Weinberg et al., 2017). The converted GABAergic neurons are functional, with mature electrophysiological firings and integrations (Grande et al., 2013; Niu et al., 2015; Pereira et al., 2017; Weinberg et al., 2017). However, compared with the diversity of GABAergic subtypes in the brain, the reprogrammed GABAergic neurons so far are very limited in subtypes. The conversion of GABAergic medium-spiny neurons (MSN, DARPP32+) and somatostatin expressing INs (SST+) are of less efficiency (Niu et al., 2015; Pereira et al., 2017). MSNs, the major projection neurons residing in the striatum and responsible for initiating and controlling movements, can be impaired when suffering from Huntington’s disease or stroke. SST neurons represent around 30% of the total IN population in many brain regions and their dysfunctions have been associated with seizure disorders (Urban-Ciecko and Barth, 2016). Thus, to generate specific GABAergic subtypes in specific brain areas under specific neurological disorders is a fundamental issue to be addressed for in vivo glia-to-neuron conversion approach.
In vivo Reprogramming Glial Cells Into Dopaminergic Neurons
Parkinson’s disease (PD) is a common neurodegenerative disorder mainly caused by degeneration of dopaminergic neurons in the substantia nigra. Cell replacement therapy has been tested as a potential therapy to restore motor functions in PD patients. Researchers have provided evidence that dopaminergic neurons, derived from the human fetal ventral midbrain, are able to partially improve the clinical outcome in a few PD patients (Kefalopoulou et al., 2014; Li et al., 2016). But, there are still ethical concerns and technical difficulties in obtaining and standardizing human fetal tissue for transplantation, as well as the risk of graft-induced dyskinesia (Olanow et al., 2003; Politis et al., 2010). An alternative approach for generating dopaminergic neurons is to trans-differentiate glial cells into dopaminergic neurons directly inside the brains in vivo, eliminating the risk of external cell transplantation. Caiazzo et al. (2011) first reported in cell cultures that three transcription factors, Ascl1 (Mash1), Lmx1a/b, and Nurr1 (together referred as ALN) can reprogram mouse fibroblasts or glial cells into dopaminergic neurons in vitro (Addis et al., 2011; Torper et al., 2013). However, in mouse brain in vivo, the ALN combination fails to convert astrocytes or NG2 glia into TH+ dopaminergic neurons (Torper et al., 2015), but rather into GABAergic neurons. More recently, a study found that by combining three transcription factors, NeuroD1, Ascl1, and Lmx1a, together with microRNA 218, mouse astrocytes can be converted into dopaminergic neurons in vivo (Rivetti di Val Cervo et al., 2017), although the efficiency is relatively low (below 20%). Interestingly, a different combination of factors including SOX2, Nurr1, Lmx1a, and Foxa2, and VPA can convert striatal GABAergic neurons into dopaminergic neurons (Niu et al., 2018). More studies are necessary to find efficient conversion of glial cells into dopaminergic neurons for effective treatment of PD.
In vivo Reprogramming Glial Cells Into Retinal Neurons
Unlike fish or chicken that can functionally regenerate their retina after injury (Fischer and Reh, 2001; Nagashima et al., 2013; Lenkowski and Raymond, 2014; Gallina et al., 2016), the adult mammalian retina has a restricted ability for self-regeneration. Previous work showed that Ascl1 is a key factor involved in Müller glia (MG) de-differentiation and retina regeneration in the fish (Fausett et al., 2008; Ramachandran et al., 2010, 2012; Wan et al., 2012). Pollak et al. (2013) further demonstrated that forced expression of Ascl1 in mouse MG induces a neurogenic state in in vitro condition. In mouse retina in vivo, overexpression of Ascl1 alone can only convert MG into immature neurons in young mice (<1 month old) with NMDA (N-methyl-D-aspartate) damage (Ueki et al., 2015). After combining Ascl1 with the histone deacetylase inhibitor trichostatin-A (TSA), Müller glia in adult mice with NMDA damage can be converted into more mature inner retinal neurons, including bipolar cells and amacrine cells, but rarely ganglion neurons (Jorstad et al., 2017). A different approach is to convert adult rods into cones, via knockdown of the rod photoreceptor determinant Nrl or Nr2e3, which appeared to preserve retinal tissue and restore visual function (Montana et al., 2013; Zhu et al., 2017). Most recently, Yao et al. (2018) reported that following gene transfer of β-catenin, cell-cycle-reactivated MG can be reprogrammed in vivo to generate new rod photoreceptors that integrate into retinal circuits and restore vision in mice by subsequent gene transfer of transcription factors Otx2, Crx, and Nrl. The next challenging task is to regenerate functional ganglion neurons in the adult mammalian retina.
To help the readers quickly grasp the rapid advancement of the emerging new field of engineered adult neurogenesis through glia-to-neuron conversion, we have compiled a comprehensive list of studies reporting on adult neurogenesis via in vivo reprogramming (Table 1). It sums up how different subtypes of neurons can be generated from different subtypes of glial cells from different brain regions through different transcription factors and compound treatments.
Mechanisms of in vivo Glia-to-Neuron Conversion
The mechanisms that govern the in vivo reprogramming of non-neuron cells into neurons are mostly unknown and understudied, and the mechanisms for in vitro reprogramming may not be readily applicable to in vivo conditions, given multiple discrepancies between in vitro and in vivo reprogramming (Kim et al., 2003; Heinrich et al., 2010; Caiazzo et al., 2011; Grande et al., 2013; Torper et al., 2013, 2015; Gascon et al., 2016). In general, the master transcription factors such as NeuroD1, Sox2, Ngn2, and Ascl1 play a major role in guiding the glia-converted neuronal fate, but the intrinsic lineages of the glial cells and local environmental cues both contribute to the final cell fate of in vivo reprogramming (Berninger and Jessberger, 2016; Li and Chen, 2016). The forced expression of NeuroD1 converts reactive astrocytes and NG2 cells into neurons (Guo et al., 2014), and microglia into neurons as well (Matsuda et al., 2019), potentially through the activation of downstream neural transcription factors (Gao et al., 2009; Kuwabara et al., 2009; Boutin et al., 2010), epigenetic modulation (Matsuda et al., 2019), and regulation of the Sonic hedgehog (SHH) signaling pathway (Sirko et al., 2013). Sox2 may prime the epigenetic landscape (Amador-Arjona et al., 2015) and directly regulates Tlx enhancer activity during in vivo reprogramming of astrocytes (Islam et al., 2015). Interestingly, Ngn2-mediated in vivo reprogramming appears to require the co-expression of Bcl-2 to inhibit ferroptosis, and anti-oxidative treatments also improve Ngn2 conversion efficiency (Gascon et al., 2016). For Ascl1-induced conversion into neurons in vivo, one possible mechanism relies on the capacity of accessing closed chromatin by Ascl1, when these chromatin loci are in a specific histone modification state (Wapinski et al., 2013). Reprogramming of Müller glia in mouse retina provides more insight into the mechanisms for in vivo neuronal conversion. For example, reduced chromatin accessibility leads to loss of neurogenic capacity in mature Müller glia (Ueki et al., 2015), and histone deacetylase inhibitor allows more effective reprogramming by promoting accessibility at key gene loci in the Müller glia (Jorstad et al., 2017). The cell cycle of Müller glia can be reactivated by gene transfer of β-catenin, and then these Müller glia can be reprogrammed into rod photoreceptors (Yao et al., 2018).
Similar transcription factors might convert glial cells into different subtypes of neurons in different brain regions, likely because glial cells in different brain regions arise from different lineages during development. For instance, glial cells in cortex and striatum can be reprogrammed into glutamatergic and GABAergic neurons by Ngn2, respectively (Grande et al., 2013; Gascon et al., 2016). Likewise, Ascl1 can convert glial cells in the striatum and midbrain into GABAergic neurons, but it converts retina Müller glia into retinal neurons (Liu et al., 2015; Torper et al., 2015; Ueki et al., 2015; Jorstad et al., 2017; Pereira et al., 2017). The impact of the local microenvironment on in vivo reprogramming is also strong. For example, the adult neocortex and striatum respond differently to Ngn2 and growth factors and generate new neurons with distinct molecular phenotypes (Grande et al., 2013). Brain or spinal cord injury can promote neurogenesis by rendering cells more permissive to chromatin remodeling (Dimou and Gotz, 2014; Heinrich et al., 2014), or unleashing the neurogenic potential restrained by Notch signaling (Magnusson et al., 2014). Lastly, chemical reprogramming may act through epigenetic silencing of glial genes (e.g., GFAP and ALDH1L1) and transcriptional activation of neural transcription factors and neuron-enriched genes (e.g., NeuroD1, Neurogenin2, MAP2, and NEUN) (Zhang et al., 2015; Gao et al., 2017). Small-molecule treatment increases DNA methylation in the GFAP promoter region but decreases H3K27 methylation at the Ngn2 transcription start site, therefore promoting glia-to-neuron conversion (Zhang et al., 2015).
Endogenous adult neurogenesis is a natural process similar to embryonic neurogenesis, both of which involve NSC differentiation due to intrinsic transcriptional regulation. In contrast, in vivo reprogramming involves an engineered cell fate change through ectopic expression of neural transcription factors that are normally expressed very low in glial cells (Li and Chen, 2016). The intrinsic NSCs or NPCs are limited, whereas glial cells can provide unlimited cell source for in vivo reprogramming, because astrocytes and NG2 cells are present throughout the CNS and not as easily exhaustible as NSCs in adult animals (Li and Chen, 2016). On the other hand, for both adult neurogenesis and in vivo cell conversion, newborn neurons are surrounded by an adult environment that may be full of neuroinhibitory factors and immune cells, particularly after injury or diseases. Unless there are sufficient number of newborn neurons that can support each other and quickly establish synaptic connections with preexisting neurons, the newly generated neurons may not survive well (Gascon et al., 2016). Therefore, the total number of newborn neurons generated in an adult environment is critical for their long-term survival and functional integration.
Advantages and Challenges of in vivo Glia-to-Neuron Conversion
Compared to in vivo cell conversion, in vitro engineered neurogenesis currently dominates the field of regenerative medicine, largely because stem cells are easy to culture in an in vitro environment than to manipulate in an adult animal. Past several decades have gained much insight into the molecular mechanisms of stem cell self-renewal and differentiation, including neural differentiation of embryonic stem cells and iPSCs. However, despite some success of cell transplantation in animal models showing symptomatic relief or even partial functional improvement, there still lacks a widely applicable stem cell therapy to treat severe neurological disorders in a disease-modifying manner. The potential reasons for this gap between basic research and clinical applications regarding in vitro engineered neurogenesis might include the efficacy of neural differentiation, the purity of differentiated neuronal subtypes, the potential of tumorigenesis associated with stem cells, the potential gene mutations accumulated during multi-generation subcultures, the potential immunorejection after transplantation, and ethical concerns associated with embryonic stem cells and human aborted fetal tissues. In contrast, all the cell culture-associated drawbacks are automatically overcome by in vivo cell conversion approach.
In addition, the engineered in vivo cell conversion technology also has a number of advantages over endogenous adult neurogenesis. Using endogenous glial cells that are nearby the lost neurons for regeneration is perhaps the most economical way to replenish the lost neurons and restore the lost functions. Because glial cells are widespread in the CNS, in vivo glia-to-neuron conversion technology can regenerate a large number of new neurons throughout the CNS, whereas the endogenous adult neurogenesis is rather limited in mammalian brains (Arvidsson et al., 2002; Sun et al., 2012; Magnusson et al., 2014; Tobin et al., 2014). Another potential advantage is that converting dividing reactive glial cells into non-dividing neurons not only reduces the number of reactive glial cells but also decreases the tumor risk associated with dividing glial cells. Therefore, we predict that a highly efficient glia-to-neuron conversion technology will be the next-generation therapy for brain and spinal cord repair.
Like any new groundbreaking technology, the in vivo glia-to-neuron conversion approach also faces many challenges ahead before potential translation into clinical therapies. One big challenge is how to convert local glial cells into neurons with identity similar to the regional neurons. This may require screening an optimal combination of transcription factors (TFs) that can mimic the early neuronal specification process. The recent advent of algorithms to predict TFs involved in cell-cell conversion might help to accelerate this process (Rackham et al., 2016; Ronquist et al., 2017). Another challenge is to convert glial cells into fully functional neurons and achieve long-term survival in the adult brains. Many published studies have shown successful glia-to-neuron conversion in vivo, but only a few studies demonstrate highly functional properties of the glia-converted neurons using electrophysiological recordings. The third challenge is to demonstrate the integration of newly converted neurons not only into the local neural circuits but also into the global neural circuits by performing anterograde and retrograde tracing experiments. One concern is whether the newly generated neurons might form wrong targets with neighboring or distant cells. This is certainly possible but according to the developmental principles of “wiring together, firing together; firing together, wiring together,” those wrongly formed connections might be eliminated if there is not enough experience-dependent activity to support their existence. Another concern is whether too many neurons might be generated through in vivo glia conversion? This is again not completely excludable, but a simple solution is to find a suitable dose of viral vectors so that neurons will not be overproduced. In fact, direct glia-to-neuron conversion has not been able to generate 100% of lost neurons to fully reverse the neuronal loss yet. Interestingly, we found that NeuroD1 conversion efficiency in reactive astrocytes is very high (90%) (Guo et al., 2014), but another group reported low efficiency in non-reactive astrocytes (Brulet et al., 2017), suggesting that reactive state might facilitate conversion. Similarly, Sox2 was found to convert reactive NG2 cells into neurons in injured adult cortex, but not resting NG2 cells without injury (Heinrich et al., 2014). From neural repair point of view, such high conversion efficiency in reactive glial cells but low efficiency in resting glial cells is ideal, because one may not want to disturb too much the resting glial cells. It is the reactive glial cells in the injured areas that are the best cell source for in vivo conversion. On the other hand, we also noticed that some reactive glial cells in the lesion core might be more difficult to convert than the reactive glial cells in the penumbra areas, suggesting that the degree of injury also affects conversion efficiency. Last but not least, we have never observed glial depletion in NeuroD1-converted areas even though its conversion efficiency is very high, because glial cells have intrinsic proliferation capability. This is very important because glial cells play important roles in supporting neuronal functions including promoting synapse formation and providing nutrients to neurons. It is important to note that in vivo cell conversion typically converts a subset of reactive glial cells into neurons, and the remaining glial cells can further divide to repopulate themselves to avoid being depleted.
Besides challenges, there are certainly some limitations of in vivo cell conversion. One obvious limitation is that when injury or degeneration is too severe, such as massive tissue loss at late stage, in vivo cell conversion might not be sufficient to generate enough cells. Perhaps grafting external cells or artificial tissue might be necessary to repair the tissue loss. Another limitation is that if neurodegeneration is caused by gene mutation, converted neurons would still carry the mutation and might still degenerate later. Although for neurodegenerative disorders, the newborn neurons would survive for a significant time before start to degenerate again, the ideal strategy may be to combine cell conversion together with gene editing technology to correct the mutation so that converted neurons can survive even longer. Finally, while most of the neural transcription factors used for in vivo cell conversion, such as NeuroD1, Ngn2, and Ascl1, are endogenous proteins expressed in neurons during brain development, the potential impact of their constitutive expression in adult neurons needs to be fully assessed.
The advantage of overexpressing TFs through viral vectors is that it can be precisely delivered into the brain or spinal cord where injury occurs. However, such precision viral delivery requires high technical skill of neurosurgeons and well-equipped hospitals. For rural areas with scarce medical facilities, gene therapy will be difficult to carry out. To overcome such limitation of gene therapy, we have tested the possibility of drug therapy and achieved the first step of success. Using a combination of small molecules, we are capable to transform cultured human astrocytes into functional glutamatergic neurons, at least in in vitro condition (Zhang et al., 2015). Following our success, Gao et al. (2017) also reported that human adult astrocytes isolated from glioma patients can be chemically converted into glutamatergic neurons in cell cultures. Our most recent study has successfully achieved chemical conversion of human astrocytes into neurons using 3–4 small molecules, making it one step closer for drug development (Yin et al., 2019). These studies paved the way toward future drug therapy, but in vivo studies on direct chemical reprogramming and efficient drug delivery across blood–brain-barrier are yet to be achieved before potential clinical translations. Nevertheless, it is worth the great effort to develop such drug therapy that can regenerate new neurons in patients’ brains not only to slow down the progression but also to reverse the course of neurodegenerative disorders.
Author Contributions
WLe, WLi, LG, and GC contributed toward writing the review article. WLe and GC finalized the manuscript.
Funding
This article writing was supported by internal funds from both Jinan University and Penn State University.
Conflict of Interest Statement
GC is a founder of NeuExcell Therapeutics Inc.
The remaining authors declare that the research was conducted in the absence of any commercial or financial relationships that could be construed as a potential conflict of interest.
References
Addis, R. C., Hsu, F. C., Wright, R. L., Dichter, M. A., Coulter, D. A., and Gearhart, J. D. (2011). Efficient conversion of astrocytes to functional midbrain dopaminergic neurons using a single polycistronic vector. PLoS One 6:e28719. doi: 10.1371/journal.pone.0028719
Aimone, J. B., Deng, W., and Gage, F. H. (2011). Resolving new memories: a critical look at the dentate gyrus, adult neurogenesis, and pattern separation. Neuron 70, 589–596. doi: 10.1016/j.neuron.2011.05.010
Aimone, J. B., Wiles, J., and Gage, F. H. (2006). Potential role for adult neurogenesis in the encoding of time in new memories. Nat. Neurosci. 9, 723–727. doi: 10.1038/nn1707
Akers, K. G., Martinez-Canabal, A., Restivo, L., Yiu, A. P., De Cristofaro, A., Hsiang, H. L., et al. (2014). Hippocampal neurogenesis regulates forgetting during adulthood and infancy. Science 344, 598–602. doi: 10.1126/science.1248903
Altman, J., and Das, G. D. (1965). Autoradiographic and histological evidence of postnatal hippocampal neurogenesis in rats. J. Comp. Neurol. 124, 319–335. doi: 10.1002/cne.901240303
Amador-Arjona, A., Cimadamore, F., Huang, C. T., Wright, R., Lewis, S., Gage, F. H., et al. (2015). SOX2 primes the epigenetic landscape in neural precursors enabling proper gene activation during hippocampal neurogenesis. Proc. Natl. Acad. Sci. U.S.A. 112, E1936–E1945. doi: 10.1073/pnas.1421480112
Amin, N. D., and Pasca, S. P. (2018). Building models of brain disorders with three-dimensional organoids. Neuron 100, 389–405. doi: 10.1016/j.neuron.2018.10.007
Arvidsson, A., Collin, T., Kirik, D., Kokaia, Z., and Lindvall, O. (2002). Neuronal replacement from endogenous precursors in the adult brain after stroke. Nat. Med. 8, 963–970. doi: 10.1038/nm747
Bayer, S. A., Yackel, J. W., and Puri, P. S. (1982). Neurons in the rat dentate gyrus granular layer substantially increase during juvenile and adult life. Science 216, 890–892. doi: 10.1126/science.7079742
Bergmann, O., Liebl, J., Bernard, S., Alkass, K., Yeung, M. S., Steier, P., et al. (2012). The age of olfactory bulb neurons in humans. Neuron 74, 634–639. doi: 10.1016/j.neuron.2012.03.030
Bernier, P. J., Bedard, A., Vinet, J., Levesque, M., and Parent, A. (2002). Newly generated neurons in the amygdala and adjoining cortex of adult primates. Proc. Natl. Acad. Sci. U.S.A. 99, 11464–11469. doi: 10.1073/pnas.172403999
Berninger, B., Costa, M. R., Koch, U., Schroeder, T., Sutor, B., Grothe, B., et al. (2007). Functional properties of neurons derived from in vitro reprogrammed postnatal astroglia. J. Neurosci. 27, 8654–8664. doi: 10.1523/JNEUROSCI.1615-07.2007
Berninger, B., and Jessberger, S. (2016). Engineering of adult neurogenesis and gliogenesis. Cold Spring Harb. Perspect. Biol 8:a018861. doi: 10.1101/cshperspect.a018861
Boldrini, M., Fulmore, C. A., Tartt, A. N., Simeon, L. R., Pavlova, I., Poposka, V., et al. (2018). Human hippocampal neurogenesis persists throughout aging. Cell Stem Cell 22, 589–599.e5. doi: 10.1016/j.stem.2018.03.015
Boutin, C., Hardt, O., de Chevigny, A., Core, N., Goebbels, S., Seidenfaden, R., et al. (2010). NeuroD1 induces terminal neuronal differentiation in olfactory neurogenesis. Proc. Natl. Acad. Sci. U.S.A. 107, 1201–1206. doi: 10.1073/pnas.0909015107
Braun, S. M., and Jessberger, S. (2014). Adult neurogenesis: mechanisms and functional significance. Development 141, 1983–1986. doi: 10.1242/dev.104596
Brulet, R., Matsuda, T., Zhang, L., Miranda, C., Giacca, M., Kaspar, B. K., et al. (2017). NEUROD1 instructs neuronal conversion in non-reactive astrocytes. Stem Cell Rep. 8, 1506–1515. doi: 10.1016/j.stemcr.2017.04.013
Brus, M., Keller, M., and Levy, F. (2013). Temporal features of adult neurogenesis: differences and similarities across mammalian species. Front. Neurosci. 7:135. doi: 10.3389/fnins.2013.00135
Bryson, J. B., Machado, C. B., Crossley, M., Stevenson, D., Bros-Facer, V., Burrone, J., et al. (2014). Optical control of muscle function by transplantation of stem cell-derived motor neurons in mice. Science 344, 94–97. doi: 10.1126/science.1248523
Buffo, A., Rite, I., Tripathi, P., Lepier, A., Colak, D., Horn, A. P., et al. (2008). Origin and progeny of reactive gliosis: a source of multipotent cells in the injured brain. Proc. Natl. Acad. Sci. U.S.A. 105, 3581–3586. doi: 10.1073/pnas.0709002105
Buffo, A., Vosko, M. R., Erturk, D., Hamann, G. F., Jucker, M., Rowitch, D., et al. (2005). Expression pattern of the transcription factor Olig2 in response to brain injuries: implications for neuronal repair. Proc. Natl. Acad. Sci. U.S.A. 102, 18183–18188. doi: 10.1073/pnas.0506535102
Buss, R. R., Sun, W., and Oppenheim, R. W. (2006). Adaptive roles of programmed cell death during nervous system development. Annu. Rev. Neurosci. 29, 1–35. doi: 10.1146/annurev.neuro.29.051605.112800
Caiazzo, M., Dell’Anno, M. T., Dvoretskova, E., Lazarevic, D., Taverna, S., Leo, D., et al. (2011). Direct generation of functional dopaminergic neurons from mouse and human fibroblasts. Nature 476, 224–227. doi: 10.1038/nature10284
Cameron, H. A., and Glover, L. R. (2015). Adult neurogenesis: beyond learning and memory. Annu. Rev. Psychol. 66, 53–81. doi: 10.1146/annurev-psych-010814-015006
Cameron, H. A., and McKay, R. D. (2001). Adult neurogenesis produces a large pool of new granule cells in the dentate gyrus. J. Comp. Neurol. 435, 406–417. doi: 10.1002/cne.1040
Caputi, A., Melzer, S., Michael, M., and Monyer, H. (2013). The long and short of GABAergic neurons. Curr. Opin. Neurobiol. 23, 179–186. doi: 10.1016/j.conb.2013.01.021
Chen, Y., Xiong, M., Dong, Y., Haberman, A., Cao, J., Liu, H., et al. (2016). Chemical control of grafted human PSC-derived neurons in a mouse model of Parkinson’s Disease. Cell Stem Cell 18, 817–826. doi: 10.1016/j.stem.2016.03.014
Cheng, L. C., Pastrana, E., Tavazoie, M., and Doetsch, F. (2009). miR-124 regulates adult neurogenesis in the subventricular zone stem cell niche. Nat. Neurosci. 12, 399–408. doi: 10.1038/nn.2294
Colasante, G., Lignani, G., Rubio, A., Medrihan, L., Yekhlef, L., Sessa, A., et al. (2015). Rapid conversion of fibroblasts into functional forebrain GAB aergic interneurons by direct genetic reprogramming. Cell Stem Cell 17, 719–734. doi: 10.1016/j.stem.2015.09.002
Curtis, M. A., Kam, M., Nannmark, U., Anderson, M. F., Axell, M. Z., Wikkelso, C., et al. (2007). Human neuroblasts migrate to the olfactory bulb via a lateral ventricular extension. Science 315, 1243–1249. doi: 10.1126/science.1136281
Cyranoski, D. (2014). Japanese Woman is First Recipient of next-generation stem cells. Nature News. London: Nature Publishing Group.
Dayer, A. G., Cleaver, K. M., Abouantoun, T., and Cameron, H. A. (2005). New GABAergic interneurons in the adult neocortex and striatum are generated from different precursors. J. Cell Biol. 168, 415–427. doi: 10.1083/jcb.200407053
De la Rossa, A., Bellone, C., Golding, B., Vitali, I., Moss, J., Toni, N., et al. (2013). In vivo reprogramming of circuit connectivity in postmitotic neocortical neurons. Nat. Neurosci. 16, 193–200. doi: 10.1038/nn.3299
Dennis, C. V., Suh, L. S., Rodriguez, M. L., Kril, J. J., and Sutherland, G. T. (2016). Human adult neurogenesis across the ages: an immunohistochemical study. Neuropathol. Appl. Neurobiol. 42, 621–638. doi: 10.1111/nan.12337
Di Lullo, E., and Kriegstein, A. R. (2017). The use of brain organoids to investigate neural development and disease. Nat. Rev. Neurosci. 18, 573–584. doi: 10.1038/nrn.2017.107
Dimou, L., and Gotz, M. (2014). Glial cells as progenitors and stem cells: new roles in the healthy and diseased brain. Physiol. Rev. 94, 709–737. doi: 10.1152/physrev.00036.2013
Eckenhoff, M. F., and Rakic, P. (1988). Nature and fate of proliferative cells in the hippocampal dentate gyrus during the life span of the rhesus monkey. J. Neurosci. 8, 2729–2747. doi: 10.1523/JNEUROSCI.08-08-02729.1988
Eriksson, P. S., Perfilieva, E., Bjork-Eriksson, T., Alborn, A. M., Nordborg, C., Peterson, D. A., et al. (1998). Neurogenesis in the adult human hippocampus. Nat. Med. 4, 1313–1317. doi: 10.1038/3305
Ernst, A., Alkass, K., Bernard, S., Salehpour, M., Perl, S., Tisdale, J., et al. (2014). Neurogenesis in the striatum of the adult human brain. Cell 156, 1072–1083. doi: 10.1016/j.cell.2014.01.044
Ernst, A., and Frisen, J. (2015). Adult neurogenesis in humans- common and unique traits in mammals. PLoS Biol. 13:e1002045. doi: 10.1371/journal.pbio.1002045
Faiz, M., Sachewsky, N., Gascon, S., Bang, K. W., Morshead, C. M., and Nagy, A. (2015). Adult neural stem cells from the subventricular zone give rise to reactive astrocytes in the cortex after stroke. Cell Stem Cell 17, 624–634. doi: 10.1016/j.stem.2015.08.002
Fausett, B. V., Gumerson, J. D., and Goldman, D. (2008). The proneural basic helix-loop-helix gene ascl1a is required for retina regeneration. J. Neurosci. 28, 1109–1117. doi: 10.1523/JNEUROSCI.4853-07.2008
Fischer, A. J., and Reh, T. A. (2001). Muller glia are a potential source of neural regeneration in the postnatal chicken retina. Nat. Neurosci. 4, 247–252. doi: 10.1038/85090
Gallina, D., Palazzo, I., Steffenson, L., Todd, L., and Fischer, A. J. (2016). Wnt/beta-catenin-signaling and the formation of Muller glia-derived progenitors in the chick retina. Dev. Neurobiol. 76, 983–1002. doi: 10.1002/dneu.22370
Gao, L., Guan, W., Wang, M., Wang, H., Yu, J., Liu, Q., et al. (2017). Direct generation of human neuronal cells from adult astrocytes by small molecules. Stem Cell Rep. 8, 538–547. doi: 10.1016/j.stemcr.2017.01.014
Gao, Z., Ure, K., Ables, J. L., Lagace, D. C., Nave, K. A., Goebbels, S., et al. (2009). Neurod1 is essential for the survival and maturation of adult-born neurons. Nat. Neurosci. 12, 1090–1092. doi: 10.1038/nn.2385
Gascon, S., Murenu, E., Masserdotti, G., Ortega, F., Russo, G. L., Petrik, D., et al. (2016). Identification and successful negotiation of a metabolic checkpoint in direct neuronal reprogramming. Cell Stem Cell 18, 396–409. doi: 10.1016/j.stem.2015.12.003
Giorgetti, A., Marchetto, M. C., Li, M., Yu, D., Fazzina, R., Mu, Y., et al. (2012). Cord blood-derived neuronal cells by ectopic expression of Sox2 and c-Myc. Proc. Natl. Acad. Sci. U.S.A. 109, 12556–12561. doi: 10.1073/pnas.1209523109
Goldman, S. A. (2016). Stem and progenitor cell-based therapy of the central nervous system: hopes, hype, and wishful thinking. Cell Stem Cell 18, 174–188. doi: 10.1016/j.stem.2016.01.012
Goncalves, J. T., Schafer, S. T., and Gage, F. H. (2016). Adult neurogenesis in the hippocampus: from stem cells to behavior. Cell 167, 897–914. doi: 10.1016/j.cell.2016.10.021
Gotz, M., Sirko, S., Beckers, J., and Irmler, M. (2015). Reactive astrocytes as neural stem or progenitor cells: in vivo lineage, In vitro potential, and Genome-wide expression analysis. Glia 63, 1452–1468. doi: 10.1002/glia.22850
Gould, E., Beylin, A., Tanapat, P., Reeves, A., and Shors, T. J. (1999a). Learning enhances adult neurogenesis in the hippocampal formation. Nat. Neurosci. 2, 260–265.
Gould, E., Reeves, A. J., Fallah, M., Tanapat, P., Gross, C. G., and Fuchs, E. (1999b). Hippocampal neurogenesis in adult Old World primates. Proc. Natl. Acad. Sci. U.S.A. 96, 5263–5267.
Gould, E., and Tanapat, P. (1999). Stress and hippocampal neurogenesis. Biol. Psychiatry 46, 1472–1479. doi: 10.1016/S0006-3223(99)00247-4
Gould, E., Vail, N., Wagers, M., and Gross, C. G. (2001). Adult-generated hippocampal and neocortical neurons in macaques have a transient existence. Proc. Natl. Acad. Sci. U.S.A. 98, 10910–10917. doi: 10.1073/pnas.181354698
Grande, A., Sumiyoshi, K., Lopez-Juarez, A., Howard, J., Sakthivel, B., Aronow, B., et al. (2013). Environmental impact on direct neuronal reprogramming in vivo in the adult brain. Nat. Commun. 4:2373. doi: 10.1038/ncomms3373
Guo, Z., Zhang, L., Wu, Z., Chen, Y., Wang, F., and Chen, G. (2014). In vivo direct reprogramming of reactive glial cells into functional neurons after brain injury and in an Alzheimer’s disease model. Cell Stem Cell 14, 188–202. doi: 10.1016/j.stem.2013.12.001
Heinrich, C., Bergami, M., Gascon, S., Lepier, A., Vigano, F., Dimou, L., et al. (2014). Sox2-mediated conversion of NG2 glia into induced neurons in the injured adult cerebral cortex. Stem Cell Rep. 3, 1000–1014. doi: 10.1016/j.stemcr.2014.10.007
Heinrich, C., Blum, R., Gascon, S., Masserdotti, G., Tripathi, P., Sanchez, R., et al. (2010). Directing astroglia from the cerebral cortex into subtype specific functional neurons. PLoS Biol. 8:e1000373. doi: 10.1371/journal.pbio.1000373
Hu, W., Qiu, B., Guan, W., Wang, Q., Wang, M., Li, W., et al. (2015). Direct conversion of normal and Alzheimer’s disease human fibroblasts into neuronal cells by small molecules. Cell Stem Cell 17, 204–212. doi: 10.1016/j.stem.2015.07.006
Islam, M. M., Smith, D. K., Niu, W., Fang, S., Iqbal, N., Sun, G., et al. (2015). Enhancer analysis unveils genetic interactions between TLX and SOX2 in neural stem cells and in vivo reprogramming. Stem Cell Rep. 5, 805–815. doi: 10.1016/j.stemcr.2015.09.015
Jorstad, N. L., Wilken, M. S., Grimes, W. N., Wohl, S. G., VandenBosch, L. S., Yoshimatsu, T., et al. (2017). Stimulation of functional neuronal regeneration from Muller glia in adult mice. Nature 548, 103–107. doi: 10.1038/nature23283
Kaplan, M. S., and Hinds, J. W. (1977). Neurogenesis in the adult rat: electron microscopic analysis of light radioautographs. Science 197, 1092–1094. doi: 10.1126/science.887941
Karow, M., Sanchez, R., Schichor, C., Masserdotti, G., Ortega, F., Heinrich, C., et al. (2012). Reprogramming of pericyte-derived cells of the adult human brain into induced neuronal cells. Cell Stem Cell 11, 471–476. doi: 10.1016/j.stem.2012.07.007
Kefalopoulou, Z., Politis, M., Piccini, P., Mencacci, N., Bhatia, K., Jahanshahi, M., et al. (2014). Long-term clinical outcome of fetal cell transplantation for Parkinson disease: two case reports. JAMA Neurol. 71, 83–87. doi: 10.1001/jamaneurol.2013.4749
Kikuchi, T., Morizane, A., Doi, D., Magotani, H., Onoe, H., Hayashi, T., et al. (2017). Human iPS cell-derived dopaminergic neurons function in a primate Parkinson’s disease model. Nature 548, 592–596. doi: 10.1038/nature23664
Kim, J., Su, S. C., Wang, H., Cheng, A. W., Cassady, J. P., Lodato, M. A., et al. (2011). Functional integration of dopaminergic neurons directly converted from mouse fibroblasts. Cell Stem Cell 9, 413–419. doi: 10.1016/j.stem.2011.09.011
Kim, J. Y., Koh, H. C., Lee, J. Y., Chang, M. Y., Kim, Y. C., Chung, H. Y., et al. (2003). Dopaminergic neuronal differentiation from rat embryonic neural precursors by Nurr1 overexpression. J. Neurochem. 85, 1443–1454. doi: 10.1046/j.1471-4159.2003.01780.x
Knoth, R., Singec, I., Ditter, M., Pantazis, G., Capetian, P., Meyer, R. P., et al. (2010). Murine features of neurogenesis in the human hippocampus across the lifespan from 0 to 100 years. PLoS One 5:e8809. doi: 10.1371/journal.pone.0008809
Kohler, S. J., Williams, N. I., Stanton, G. B., Cameron, J. L., and Greenough, W. T. (2011). Maturation time of new granule cells in the dentate gyrus of adult macaque monkeys exceeds six months. Proc. Natl. Acad. Sci. U.S.A. 108, 10326–10331. doi: 10.1073/pnas.1017099108
Kokoeva, M. V., Yin, H., and Flier, J. S. (2005). Neurogenesis in the hypothalamus of adult mice: potential role in energy balance. Science 310, 679–683. doi: 10.1126/science.1115360
Kornack, D. R., and Rakic, P. (1999). Continuation of neurogenesis in the hippocampus of the adult macaque monkey. Proc. Natl. Acad. Sci. U.S.A. 96, 5768–5773. doi: 10.1073/pnas.96.10.5768
Kornack, D. R., and Rakic, P. (2001a). Cell proliferation without neurogenesis in adult primate neocortex. Science 294, 2127–2130. doi: 10.1126/science.1065467
Kornack, D. R., and Rakic, P. (2001b). The generation, migration, and differentiation of olfactory neurons in the adult primate brain. Proc. Natl. Acad. Sci. U.S.A. 98, 4752–4757.
Kuwabara, T., Hsieh, J., Muotri, A., Yeo, G., Warashina, M., Lie, D. C., et al. (2009). Wnt-mediated activation of NeuroD1 and retro-elements during adult neurogenesis. Nat. Neurosci. 12, 1097–1105. doi: 10.1038/nn.2360
Ladewig, J., Mertens, J., Kesavan, J., Doerr, J., Poppe, D., Glaue, F., et al. (2012). Small molecules enable highly efficient neuronal conversion of human fibroblasts. Nat. Methods 9, 575–578. doi: 10.1038/nmeth.1972
Lenkowski, J. R., and Raymond, P. A. (2014). Muller glia: stem cells for generation and regeneration of retinal neurons in teleost fish. Prog. Retin. Eye Res. 40, 94–123. doi: 10.1016/j.preteyeres.2013.12.007
Lepousez, G., Nissant, A., and Lledo, P. M. (2015). Adult neurogenesis and the future of the rejuvenating brain circuits. Neuron 86, 387–401. doi: 10.1016/j.neuron.2015.01.002
Li, H., and Chen, G. (2016). In vivo reprogramming for CNS repair: regenerating neurons from endogenous glial cells. Neuron 91, 728–738. doi: 10.1016/j.neuron.2016.08.004
Li, W., Englund, E., Widner, H., Mattsson, B., van Westen, D., Latt, J., et al. (2016). Extensive graft-derived dopaminergic innervation is maintained 24 years after transplantation in the degenerating parkinsonian brain. Proc. Natl. Acad. Sci. U.S.A. 113, 6544–6549. doi: 10.1073/pnas.1605245113
Li, X., Zuo, X., Jing, J., Ma, Y., Wang, J., Liu, D., et al. (2015). Small-molecule-driven direct reprogramming of mouse fibroblasts into functional neurons. Cell Stem Cell 17, 195–203. doi: 10.1016/j.stem.2015.06.003
Lim, D. A., and Alvarez-Buylla, A. (2016). The adult ventricular-subventricular zone (V-SVZ) and Olfactory Bulb (OB) Neurogenesis. Cold Spring Harb. Perspect. Biol. 8:a018820. doi: 10.1101/cshperspect.a018820
Lim, D. A., Tramontin, A. D., Trevejo, J. M., Herrera, D. G., Garcia-Verdugo, J. M., and Alvarez-Buylla, A. (2000). Noggin antagonizes BMP signaling to create a niche for adult neurogenesis. Neuron 28, 713–726. doi: 10.1016/S0896-6273(00)00148-3
Liu, M. L., Zang, T., Zou, Y., Chang, J. C., Gibson, J. R., Huber, K. M., et al. (2013). Small molecules enable neurogenin 2 to efficiently convert human fibroblasts into cholinergic neurons. Nat. Commun. 4:2183. doi: 10.1038/ncomms3183
Liu, Y., Miao, Q., Yuan, J., Han, S., Zhang, P., Li, S., et al. (2015). Ascl1 converts dorsal midbrain astrocytes into functional neurons in vivo. J. Neurosci. 35, 9336–9355. doi: 10.1523/JNEUROSCI.3975-14.2015
Lois, C., and Alvarez-Buylla, A. (1993). Proliferating subventricular zone cells in the adult mammalian forebrain can differentiate into neurons and glia. Proc. Natl. Acad. Sci. U.S.A. 90, 2074–2077. doi: 10.1073/pnas.90.5.2074
Lois, C., and Alvarez-Buylla, A. (1994). Long-distance neuronal migration in the adult mammalian brain. Science 264, 1145–1148. doi: 10.1126/science.8178174
Luo, Y., Coskun, V., Liang, A., Yu, J., Cheng, L., Ge, W., et al. (2015). Single-cell transcriptome analyses reveal signals to activate dormant neural stem cells. Cell 161, 1175–1186. doi: 10.1016/j.cell.2015.04.001
Ma, D. K., Jang, M. H., Guo, J. U., Kitabatake, Y., Chang, M. L., Pow-Anpongkul, N., et al. (2009). Neuronal activity-induced Gadd45b promotes epigenetic DNA demethylation and adult neurogenesis. Science 323, 1074–1077. doi: 10.1126/science.1166859
Magnusson, J. P., Goritz, C., Tatarishvili, J., Dias, D. O., Smith, E. M., Lindvall, O., et al. (2014). A latent neurogenic program in astrocytes regulated by Notch signaling in the mouse. Science 346, 237–241. doi: 10.1126/science.346.6206.237
Marro, S., Pang, Z. P., Yang, N., Tsai, M. C., Qu, K., Chang, H. Y., et al. (2011). Direct lineage conversion of terminally differentiated hepatocytes to functional neurons. Cell Stem Cell 9, 374–382. doi: 10.1016/j.stem.2011.09.002
Martin, U. (2017). Therapeutic application of pluripotent stem cells: challenges and risks. Front. Med. 4:229. doi: 10.3389/fmed.2017.00229
Matsuda, T., Irie, T., Katsurabayashi, S., Hayashi, Y., Nagai, T., Hamazaki, N., et al. (2019). Pioneer Factor NeuroD1 rearranges transcriptional and epigenetic profiles to execute microglia-neuron conversion. Neuron doi: 10.1016/j.neuron.2018.12.010 [Epub ahead of print].
Mertens, J., Paquola, A. C. M., Ku, M., Hatch, E., Bohnke, L., Ladjevardi, S., et al. (2015). Directly reprogrammed human neurons retain aging-associated transcriptomic signatures and reveal age-related nucleocytoplasmic defects. Cell Stem Cell 17, 705–718. doi: 10.1016/j.stem.2015.09.001
Ming, G. L., and Song, H. (2011). Adult neurogenesis in the mammalian brain: significant answers and significant questions. Neuron 70, 687–702. doi: 10.1016/j.neuron.2011.05.001
Montana, C. L., Kolesnikov, A. V., Shen, S. Q., Myers, C. A., Kefalov, V. J., and Corbo, J. C. (2013). Reprogramming of adult rod photoreceptors prevents retinal degeneration. Proc. Natl. Acad. Sci. U.S.A. 110, 1732–1737. doi: 10.1073/pnas.1214387110
Nagashima, M., Barthel, L. K., and Raymond, P. A. (2013). A self-renewing division of zebrafish Muller glial cells generates neuronal progenitors that require N-cadherin to regenerate retinal neurons. Development 140, 4510–4521. doi: 10.1242/dev.090738
Niu, W., Zang, T., Smith, D. K., Vue, T. Y., Zou, Y., Bachoo, R., et al. (2015). SOX2 reprograms resident astrocytes into neural progenitors in the adult brain. Stem Cell Rep. 4, 780–794. doi: 10.1016/j.stemcr.2015.03.006
Niu, W., Zang, T., Wang, L. L., Zou, Y., and Zhang, C. L. (2018). Phenotypic reprogramming of striatal neurons into dopaminergic neuron-like cells in the adult mouse brain. Stem Cell Rep. 11, 1156–1170. doi: 10.1016/j.stemcr.2018.09.004
Niu, W., Zang, T., Zou, Y., Fang, S., Smith, D. K., Bachoo, R., et al. (2013). In vivo reprogramming of astrocytes to neuroblasts in the adult brain. Nat. Cell Biol. 15, 1164–1175. doi: 10.1038/ncb2843
Ohori, Y., Yamamoto, S., Nagao, M., Sugimori, M., Yamamoto, N., Nakamura, K., et al. (2006). Growth factor treatment and genetic manipulation stimulate neurogenesis and oligodendrogenesis by endogenous neural progenitors in the injured adult spinal cord. J. Neurosci. 26, 11948–11960. doi: 10.1523/JNEUROSCI.3127-06.2006
Olanow, C. W., Goetz, C. G., Kordower, J. H., Stoessl, A. J., Sossi, V., Brin, M. F., et al. (2003). A double-blind controlled trial of bilateral fetal nigral transplantation in Parkinson’s disease. Ann. Neurol. 54, 403–414. doi: 10.1002/ana.10720
Pasca, S. P. (2018). The rise of three-dimensional human brain cultures. Nature 553, 437–445. doi: 10.1038/nature25032
Pereira, M., Birtele, M., Shrigley, S., Benitez, J. A., Hedlund, E., Parmar, M., et al. (2017). Direct reprogramming of resident NG2 Glia into neurons with properties of fast-spiking parvalbumin-containing interneurons. Stem Cell Rep. 9, 742–751. doi: 10.1016/j.stemcr.2017.07.023
Pfisterer, U., Kirkeby, A., Torper, O., Wood, J., Nelander, J., Dufour, A., et al. (2011). Direct conversion of human fibroblasts to dopaminergic neurons. Proc. Natl. Acad. Sci. U.S.A. 108, 10343–10348. doi: 10.1073/pnas.1105135108
Politis, M., Wu, K., Loane, C., Quinn, N. P., Brooks, D. J., Rehncrona, S., et al. (2010). Serotonergic neurons mediate dyskinesia side effects in Parkinson’s patients with neural transplants. Sci. Transl. Med. 2:38ra46. doi: 10.1126/scitranslmed.3000976
Pollak, J., Wilken, M. S., Ueki, Y., Cox, K. E., Sullivan, J. M., Taylor, R. J., et al. (2013). ASCL1 reprograms mouse Muller glia into neurogenic retinal progenitors. Development 140, 2619–2631. doi: 10.1242/dev.091355
Rackham, O. J., Firas, J., Fang, H., Oates, M. E., Holmes, M. L., Knaupp, A. S., et al. (2016). A predictive computational framework for direct reprogramming between human cell types. Nat. Genet. 48, 331–335. doi: 10.1038/ng.3487
Rakic, P. (1985). Limits of neurogenesis in primates. Science 227, 1054–1056. doi: 10.1126/science.3975601
Ramachandran, R., Fausett, B. V., and Goldman, D. (2010). Ascl1a regulates Muller glia dedifferentiation and retinal regeneration through a Lin-28-dependent, let-7 microRNA signalling pathway. Nat. Cell Biol. 12, 1101–1107. doi: 10.1038/ncb2115
Ramachandran, R., Zhao, X. F., and Goldman, D. (2012). Insm1a-mediated gene repression is essential for the formation and differentiation of Muller glia-derived progenitors in the injured retina. Nat. Cell Biol. 14, 1013–1023. doi: 10.1038/ncb2586
Reynolds, B. A., and Weiss, S. (1992). Generation of neurons and astrocytes from isolated cells of the adult mammalian central nervous system. Science 255, 1707–1710. doi: 10.1126/science.1553558
Rivetti, di Val, Cervo, P., Romanov, R. A., Spigolon, G., Masini, D., et al. (2017). Induction of functional dopamine neurons from human astrocytes in vitro and mouse astrocytes in a Parkinson’s disease model. Nat. Biotechnol. 35, 444–452. doi: 10.1038/nbt.3835
Ronquist, S., Patterson, G., Muir, L. A., Lindsly, S., Chen, H., Brown, M., et al. (2017). Algorithm for cellular reprogramming. Proc. Natl. Acad. Sci. U.S.A. 114, 11832–11837. doi: 10.1073/pnas.1712350114
Rouaux, C., and Arlotta, P. (2010). Fezf2 directs the differentiation of corticofugal neurons from striatal progenitors in vivo. Nat. Neurosci. 13, 1345–1347. doi: 10.1038/nn.2658
Rouaux, C., and Arlotta, P. (2013). Direct lineage reprogramming of post-mitotic callosal neurons into corticofugal neurons in vivo. Nat. Cell Biol. 15, 214–221. doi: 10.1038/ncb2660
Sailor, K. A., Schinder, A. F., and Lledo, P. M. (2017). Adult neurogenesis beyond the niche: its potential for driving brain plasticity. Curr. Opin. Neurobiol. 42, 111–117. doi: 10.1016/j.conb.2016.12.001
Sanai, N., Nguyen, T., Ihrie, R. A., Mirzadeh, Z., Tsai, H. H., Wong, M., et al. (2011). Corridors of migrating neurons in the human brain and their decline during infancy. Nature 478, 382–386. doi: 10.1038/nature10487
Sanai, N., Tramontin, A. D., Quinones-Hinojosa, A., Barbaro, N. M., Gupta, N., Kunwar, S., et al. (2004). Unique astrocyte ribbon in adult human brain contains neural stem cells but lacks chain migration. Nature 427, 740–744. doi: 10.1038/nature02301
Sawamoto, K., Hirota, Y., Alfaro-Cervello, C., Soriano-Navarro, M., He, X., Hayakawa-Yano, Y., et al. (2011). Cellular composition and organization of the subventricular zone and rostral migratory stream in the adult and neonatal common marmoset brain. J. Comp. Neurol. 519, 690–713. doi: 10.1002/cne.22543
Shin, J., Berg, D. A., Zhu, Y., Shin, J. Y., Song, J., Bonaguidi, M. A., et al. (2015). Single-Cell RNA-Seq with waterfall reveals molecular cascades underlying adult neurogenesis. Cell Stem Cell 17, 360–372. doi: 10.1016/j.stem.2015.07.013
Sierra, A., Encinas, J. M., Deudero, J. J., Chancey, J. H., Enikolopov, G., Overstreet-Wadiche, L. S., et al. (2010). Microglia shape adult hippocampal neurogenesis through apoptosis-coupled phagocytosis. Cell Stem Cell 7, 483–495. doi: 10.1016/j.stem.2010.08.014
Sirko, S., Behrendt, G., Johansson, P. A., Tripathi, P., Costa, M., Bek, S., et al. (2013). Reactive glia in the injured brain acquire stem cell properties in response to sonic hedgehog [corrected]. Cell Stem Cell 12, 426–439. doi: 10.1016/j.stem.2013.01.019
Son, E. Y., Ichida, J. K., Wainger, B. J., Toma, J. S., Rafuse, V. F., Woolf, C. J., et al. (2011). Conversion of mouse and human fibroblasts into functional spinal motor neurons. Cell Stem Cell 9, 205–218. doi: 10.1016/j.stem.2011.07.014
Sorrells, S. F., Paredes, M. F., Cebrian-Silla, A., Sandoval, K., Qi, D., Kelley, K. W., et al. (2018). Human hippocampal neurogenesis drops sharply in children to undetectable levels in adults. Nature 555, 377–381. doi: 10.1038/nature25975
Spalding, K. L., Bergmann, O., Alkass, K., Bernard, S., Salehpour, M., Huttner, H. B., et al. (2013). Dynamics of hippocampal neurogenesis in adult humans. Cell 153, 1219–1227. doi: 10.1016/j.cell.2013.05.002
Steinbeck, J. A., Choi, S. J., Mrejeru, A., Ganat, Y., Deisseroth, K., Sulzer, D., et al. (2015). Optogenetics enables functional analysis of human embryonic stem cell-derived grafts in a Parkinson’s disease model. Nat. Biotechnol. 33, 204–209. doi: 10.1038/nbt.3124
Su, Z., Niu, W., Liu, M. L., Zou, Y., and Zhang, C. L. (2014). In vivo conversion of astrocytes to neurons in the injured adult spinal cord. Nat. Commun. 5:3338. doi: 10.1038/ncomms4338
Sun, X., Zhang, Q. W., Xu, M., Guo, J. J., Shen, S. W., Wang, Y. Q., et al. (2012). New striatal neurons form projections to substantia nigra in adult rat brain after stroke. Neurobiol. Dis. 45, 601–609. doi: 10.1016/j.nbd.2011.09.018
Szulwach, K. E., Li, X., Smrt, R. D., Li, Y., Luo, Y., Lin, L., et al. (2010). Cross talk between microRNA and epigenetic regulation in adult neurogenesis. J. Cell Biol. 189, 127–141. doi: 10.1083/jcb.200908151
Takahashi, K., Tanabe, K., Ohnuki, M., Narita, M., Ichisaka, T., Tomoda, K., et al. (2007). Induction of pluripotent stem cells from adult human fibroblasts by defined factors. Cell 131, 861–872. doi: 10.1016/j.cell.2007.11.019
Takahashi, K., and Yamanaka, S. (2006). Induction of pluripotent stem cells from mouse embryonic and adult fibroblast cultures by defined factors. Cell 126, 663–676. doi: 10.1016/j.cell.2006.07.024
Tanabe, K., Ang, C. E., Chanda, S., Olmos, V. H., Haag, D., Levinson, D. F., et al. (2018). Transdifferentiation of human adult peripheral blood T cells into neurons. Proc. Natl. Acad. Sci. U.S.A. 115, 6470–6475. doi: 10.1073/pnas.1720273115
Tobin, M. K., Bonds, J. A., Minshall, R. D., Pelligrino, D. A., Testai, F. D., and Lazarov, O. (2014). Neurogenesis and inflammation after ischemic stroke: what is known and where we go from here. J. Cereb. Blood Flow Metab. 34, 1573–1584. doi: 10.1038/jcbfm.2014.130
Torper, O., Ottosson, D. R., Pereira, M., Lau, S., Cardoso, T., Grealish, S., et al. (2015). In vivo reprogramming of striatal NG2 Glia into functional neurons that integrate into local host circuitry. Cell Rep. 12, 474–481. doi: 10.1016/j.celrep.2015.06.040
Torper, O., Pfisterer, U., Wolf, D. A., Pereira, M., Lau, S., Jakobsson, J., et al. (2013). Generation of induced neurons via direct conversion in vivo. Proc. Natl. Acad. Sci. U.S.A. 110, 7038–7043. doi: 10.1073/pnas.1303829110
Trounson, A., and McDonald, C. (2015). Stem cell therapies in clinical trials: progress and challenges. Cell Stem Cell 17, 11–22. doi: 10.1016/j.stem.2015.06.007
Tsunemoto, R., Lee, S., Szucs, A., Chubukov, P., Sokolova, I., Blanchard, J. W., et al. (2018). Diverse reprogramming codes for neuronal identity. Nature 557, 375–380. doi: 10.1038/s41586-018-0103-5
Tsunemoto, R. K., Eade, K. T., Blanchard, J. W., and Baldwin, K. K. (2015). Forward engineering neuronal diversity using direct reprogramming. EMBO J. 34, 1445–1455. doi: 10.15252/embj.201591402
Ueki, Y., Wilken, M. S., Cox, K. E., Chipman, L., Jorstad, N., Sternhagen, K., et al. (2015). Transgenic expression of the proneural transcription factor Ascl1 in Muller glia stimulates retinal regeneration in young mice. Proc. Natl. Acad. Sci. U.S.A. 112, 13717–13722. doi: 10.1073/pnas.1510595112
Urban-Ciecko, J., and Barth, A. L. (2016). Somatostatin-expressing neurons in cortical networks. Nat. Rev. Neurosci. 17, 401–409. doi: 10.1038/nrn.2016.53
Victor, M. B., Richner, M., Hermanstyne, T. O., Ransdell, J. L., Sobieski, C., Deng, P. Y., et al. (2014). Generation of human striatal neurons by microRNA-dependent direct conversion of fibroblasts. Neuron 84, 311–323. doi: 10.1016/j.neuron.2014.10.016
Vierbuchen, T., Ostermeier, A., Pang, Z. P., Kokubu, Y., Sudhof, T. C., and Wernig, M. (2010). Direct conversion of fibroblasts to functional neurons by defined factors. Nature 463, 1035–1041. doi: 10.1038/nature08797
Wan, J., Ramachandran, R., and Goldman, D. (2012). HB-EGF is necessary and sufficient for Muller glia dedifferentiation and retina regeneration. Dev. Cell 22, 334–347. doi: 10.1016/j.devcel.2011.11.020
Wang, L. L., Su, Z., Tai, W., Zou, Y., Xu, X. M., and Zhang, C. L. (2016). The p53 pathway controls SOX2-mediated reprogramming in the adult mouse spinal cord. Cell Rep. 17, 891–903. doi: 10.1016/j.celrep.2016.09.038
Wapinski, O. L., Vierbuchen, T., Qu, K., Lee, Q. Y., Chanda, S., Fuentes, D. R., et al. (2013). Hierarchical mechanisms for direct reprogramming of fibroblasts to neurons. Cell 155, 621–635. doi: 10.1016/j.cell.2013.09.028
Weinberg, M. S., Criswell, H. E., Powell, S. K., Bhatt, A. P., and McCown, T. J. (2017). Viral vector reprogramming of adult resident striatal oligodendrocytes into functional neurons. Mol. Ther. 25, 928–934. doi: 10.1016/j.ymthe.2017.01.016
Xue, Y., Ouyang, K., Huang, J., Zhou, Y., Ouyang, H., Li, H., et al. (2013). Direct conversion of fibroblasts to neurons by reprogramming PTB-Regulated MicroRNA circuits. Cell 152, 82–96. doi: 10.1016/j.cell.2012.11.045
Yang, N., Ng, Y. H., Pang, Z. P., Sudhof, T. C., and Wernig, M. (2011). Induced neuronal cells: how to make and define a neuron. Cell Stem Cell 9, 517–525. doi: 10.1016/j.stem.2011.11.015
Yao, K., Qiu, S., Wang, Y. V., Park, S. J. H., Mohns, E. J., Mehta, B., et al. (2018). Restoration of vision after de novo genesis of rod photoreceptors in mammalian retinas. Nature 560, 484–488. doi: 10.1038/s41586-018-0425-3
Ye, Z., Mostajo-Radji, M. A., Brown, J. R., Rouaux, C., Tomassy, G. S., Hensch, T. K., et al. (2015). Instructing perisomatic inhibition by direct lineage reprogramming of neocortical projection neurons. Neuron 88, 475–483. doi: 10.1016/j.neuron.2015.10.006
Yin, J.-C., Zhang, L., Ma, N.-X., Wang, Y., Lee, G., Hou, X.-Y., et al. (2019). Chemical conversion of human fetal astrocytes into neurons through modulation of multiple signaling pathways. Stem Cell Reports. doi: 10.1016/j.stemcr.2019.01.003 [Epub ahead of print].
Yoo, A. S., Sun, A. X., Li, L., Shcheglovitov, A., Portmann, T., Li, Y., et al. (2011). MicroRNA-mediated conversion of human fibroblasts to neurons. Nature 476, 228–231. doi: 10.1038/nature10323
Zhang, L., Yin, J. C., Yeh, H., Ma, N. X., Lee, G., Chen, X. A., et al. (2015). Small molecules efficiently reprogram human astroglial cells into functional neurons. Cell Stem Cell 17, 735–747. doi: 10.1016/j.stem.2015.09.012
Zhao, M., Momma, S., Delfani, K., Carlen, M., Cassidy, R. M., Johansson, C. B., et al. (2003). Evidence for neurogenesis in the adult mammalian substantia nigra. Proc. Natl. Acad. Sci. U.S.A. 100, 7925–7930. doi: 10.1073/pnas.1131955100
Keywords: neurogenesis, in vivo reprogramming, glia-to-neuron conversion, brain repair, neuron, astrocyte
Citation: Lei W, Li W, Ge L and Chen G (2019) Non-engineered and Engineered Adult Neurogenesis in Mammalian Brains. Front. Neurosci. 13:131. doi: 10.3389/fnins.2019.00131
Received: 06 October 2018; Accepted: 05 February 2019;
Published: 21 February 2019.
Edited by:
Paolo Peretto, University of Turin, ItalyReviewed by:
Lorraine Iacovitti, Thomas Jefferson University, United StatesJorge Valero, Achucarro Basque Center for Neuroscience, Spain
Copyright © 2019 Lei, Li, Ge and Chen. This is an open-access article distributed under the terms of the Creative Commons Attribution License (CC BY). The use, distribution or reproduction in other forums is permitted, provided the original author(s) and the copyright owner(s) are credited and that the original publication in this journal is cited, in accordance with accepted academic practice. No use, distribution or reproduction is permitted which does not comply with these terms.
*Correspondence: Wenliang Lei, bGVpd2VubGlhbmdAam51LmVkdS5jbg==; bGVpd2VubGlhbmcxOTgzQGdtYWlsLmNvbQ== Gong Chen, Z29uZ2NoZW5AcHN1LmVkdQ==