- 1School of Nursing, State University of Campinas, Campinas, Brazil
- 2Department of Medicine, Division of Endocrinology, Diabetes, and Metabolism – Beth Israel Deaconess Medical Center, Harvard Medical School, Boston, MA, United States
- 3Laboratory of Cell Signaling, Obesity and Comorbidities Research Center, State University of Campinas, Campinas, Brazil
- 4National Institute of Science and Technology on Neuroimmunomodulation, Rio de Janeiro, Brazil
Emerging data demonstrate that microglia activation plays a pivotal role in the development of hypothalamic inflammation in obesity. Early after the introduction of a high-fat diet, hypothalamic microglia undergo morphological, and functional changes in response to excessive dietary saturated fats. Initially the resident microglia are affected; however, as diet-induced obesity persists, bone marrow-derived myeloid cells gradually replace resident microglia. Genetic and pharmacological approaches aimed at dampening the inflammatory activity in the hypothalamus of experimental models of obesity have proven beneficial to correct the obese phenotype and improve metabolic abnormalities commonly associated with obesity. These approaches provide an experimental proof-of-concept that hypothalamic inflammation is central to the pathophysiology of obesity; understanding the details of the roles played by microglia in this process may help the development of preventive and therapeutic advances in the field. In this review, we discuss the potential mechanisms underlying hypothalamic microglial activation in high-fat induced obesity.
Introduction
The mediobasal hypothalamus (MBH) is a key brain region involved in the central control of caloric intake and energy expenditure. Its function relies on a complex neuronal network that senses the energy status of the body and coordinates responses aimed at maintaining energetic homeostasis. First-order neurons in this network are located in the arcuate nucleus (ARC), sitting close to the median eminence (ME), which is richly irrigated by fenestrated capillaries that secure special permeability to the blood/spinal fluid interface (BSFI) (Rodríguez et al., 2010). The partially leaky ME-BSFI facilitates ARC neuronal and glial cells to rapidly and directly sense minimal changes in peripheral signals, such as hormones (e.g., leptin and insulin) and nutrients, essential to the regulation of whole-body energy homeostasis (Lam et al., 2005; Schaeffer et al., 2013; Timper and Brüning, 2017). Under physiological conditions, feeding leads to the activation of ARC proopiomelanocortin (POMC) and cocaine- and amphetamine-regulated transcript (CART) neurons resulting in suppressed food intake and increased energy expenditure. Conversely, fasting leads to the activation of neuropeptide Y (NPY) and agouti-related protein (AgRP) neurons, which antagonize the function of POMC neurons (Schwartz et al., 2000). Over time, the correct function of these neuronal subpopulations leads to body-mass stability. However, the excessive consumption of saturated fatty acids (SFAs) can disrupt the physiological pattern of food intake and energy expenditure control by triggering an inflammatory response in the hypothalamus, which affects the MBH at the functional and structural levels (De Souza et al., 2005; Velloso and Schwartz, 2011). Leptin resistance emerges as an early functional outcome of hypothalamic inflammation (Dragano et al., 2017; Pan and Myers, 2018), which can be overcome by reducing local inflammatory activity with genetic or pharmacological approaches, or simply by reducing the amount of SFAs in the diet. With time, chronic structural abnormalities can develop, such as changes in neuronal projections, gliosis, and eventually, neuronal death (Moraes et al., 2009; Dorfman and Thaler, 2015). The extension and chronicity of the structural and functional losses of the hypothalamus may explain the relapsing nature of obesity that can resist behavioral, pharmacological, and even surgical therapeutic measures. Thus, understanding the mechanisms involved in the evolution and persistence of hypothalamic inflammation may offer new insights into the development of strategies for the prevention and treatment of obesity.
Studies have shown that diet-induced inflammation initially and preferentially affects the MBH (Thaler et al., 2012). One of the particularities that may explain the anatomical specificity of this process is the nature of the ME-BSFI and the presence of tanycytes in this region, what makes the MBH more sensitive to dietary fats than other circumventricular organs, undergoing a rapid structural, and functional disorganization in mice fed a high-fat diet (HFD) (Ramalho et al., 2018). About the same time that structural and functional changes are occurring in the ME-BSFI, resident microglia undergo morphological and physiological alterations in the ARC region that neighbors the ME (Valdearcos et al., 2014). However, upon persistence of a HFD, bone marrow-derived myeloid cells (BMDM) migrate into the MBH, which is an important condition to secure chronic inflammation (Valdearcos et al., 2017). Approaches that inhibit the migration of BMDM cells to the MBH can reduce hypothalamic inflammation and attenuate diet-induced obesity (DIO). Despite the fact that most studies in this field were performed employing experimental models, there is evidence supporting the presence of inflammation and gliosis in the hypothalamus of obese humans as well (van de Sande-Lee et al., 2011; Thaler et al., 2012), further supporting hypothalamic inflammation as a potential pharmacological target for the obesity treatment. Here, we review the studies that provided progress in the characterization of abnormalities of MBH microglia in obesity.
Dietary Fats Modify Hypothalamic Microglial Morphology, Distribution, and Function
Microglial cells are the most abundant brain innate immune cells and are relatively quiescent under baseline conditions. However, when exposed to injury signals, microglia undergo a rapid reaction characterized by morphological and functional changes aimed at enabling them to mount an inflammatory response against the potentially existing treat (Saijo and Glass, 2011).
Studies with rodents have shown that microglia of the MBH are sensitive to SFAs (lauric, palmitic, and stearic acids) (Valdearcos et al., 2014). In the hypothalamus of rodents, consumption of large portions of SFAs promotes a rapid microglial response characterized by the appearance of a number of prolonged processes emanating from a discreetly enlarged cell body (Baufeld et al., 2016). Initially, these morphological changes are restricted to the ARC region nearby the transition between the MBH and ME and are accompanied by increased expression of inflammatory cytokines and other markers of inflammation. The hypothalamic inflammatory response to SFAs occurs in a biphasic mode. During the first week of a HFD, the mRNA and protein levels of hypothalamic cytokines such as TNF-α, IL1-β, and IL-6 increase, as well as chemokines such as MCP-1 and fractalkine (Morari et al., 2014). Upon the persistence, for 2 weeks and longer, of the exposure to SFAs in diets that are particularly rich in stearate and palmitate, the levels of inflammatory markers decrease, only to return after 4 weeks, and then persist for as long as the content of SFAs in the diet is excessive (Thaler et al., 2012).
The biphasic nature of the inflammatory response in the hypothalamus of experimental models of obesity occurs similarly to the classical biphasic inflammatory response in other tissues. One reason behind the biphasic nature of obesity-associated hypothalamic inflammation is that the initial response is mounted by resident microglia; however, as dietary-fat consumption persists, bone marrow-derived myeloid cells gradually replace the MBH microglia (Valdearcos et al., 2017). Thus, at this point, it is important to understand the mechanisms that govern microglia activation, phenotype definition, and the recruitment of bone marrow-derived myeloid cells to the site of injury.
Mechanisms of Hypothalamic Microglia Activation in Obesity
Similar to the resident macrophages of tissues outside the brain, microglia of rodents are capable of sensing and responding to the presence of pathogen-associated molecular patterns (PAMPs) (Kigerl et al., 2014). The systemic injection of a single high-dose of lipopolysaccharide (LPS) promotes activation-like changes in the morphology of hypothalamic microglia, which is detected as early as 8 h after injection, peaking after 24 h and recovering after 7 days (Buttini et al., 1996). This is accompanied by increased expression of MHC-I and inflammatory cytokines (TNF-α, IL-1, and IL-6), and also by the activation of COX-1 and NF-κB (García-Bueno et al., 2009). Interestingly, the same macrophage receptor that is activated in response to LPS, TLR4, can be activated in response to SFAs (Huang et al., 2012).
Under baseline conditions, TLR4 is expressed at low levels in the brain, including the hypothalamus of rodents; however, its expression increases considerably when rodents are fed a HFD (Milanski et al., 2009). In other brain regions, TLR4 expression is involved in medical conditions such as autoimmunity (Kerfoot et al., 2004), HIV infection (Cheung et al., 2017) and Alzheimer’s disease (Ledo et al., 2016), illustrating its pleiotropic roles in brain immunity and defense. A detailed screening of dietary fats that could potentially promote the activation of hypothalamic TLR4 identified long-chain SFAs as the most potent inducers of its association with MyD88, resulting in the activation of NF-κB and JNK and leading to endoplasmic reticulum stress (Zhang et al., 2008). When dietary SFAs are substituted by similar amounts of unsaturated fatty acids (flax seed oil and olive oil), the hypothalamic inflammation is dampened in parallel to the reduced activity of JNK and NF-κB (Cintra et al., 2012). The pharmacological and the genetic inhibition of TLR4 reduce diet-induced hypothalamic inflammation and protect rodents from DIO and glucose intolerance (Milanski et al., 2012). Importantly, contact with large portions of SFAs, and not the presence of obesity per se, is required to induce hypothalamic microglial activation; this is evidenced by the fact that genetically obese mice, such as, ob/ob (leptin-deficient), db/db (leptin receptor-deficient) and MC4R-KO, do not present hypothalamic microglia activation when they are fed on chow, despite the evidence that even on this diet they become obese (Gao et al., 2014).
Cell culture approaches were used to investigate additional details involving the activation of microglia by fatty acids. Palmitate and stearate are each capable of inducing a rapid activation of BV2 microglial cells line through a mechanism dependent on TLR4 (Wang et al., 2012). Reactive morphological changes and increased production of inflammatory cytokines, nitric oxide, and reactive oxygen species (ROS) characterize the activation of microglia (Wang et al., 2012). In addition, upon exposure to palmitate, another microglial cell line, THP-1, is capable of producing inflammatory cytokines and inducing neurotoxicity (Little et al., 2012).
As a proof-of-concept for the central implication of microglia in the development of diet-induced hypothalamic inflammation, Valdearcos et al. (2014) used distinct strategies to modify the number of microglia in the hypothalamus. When microglia is increased, the intensity of hypothalamic inflammation induced by SFAs is boosted; conversely, when MBH microglia is reduced hypothalamic inflammation is abrogated. Importantly, a positive relationship exists between the number of hypothalamic microglia and the obese phenotype (Baufeld et al., 2016).
In addition to the direct effects of dietary fats to induce hypothalamic inflammation and microglial activation, some studies have shown that neural afferents from the gut can also trigger an inflammatory response in the hypothalamus (Mulders et al., 2018). The gut inflammatory signals resulting from the consumption of HFD can act through vagal connections to the nodose ganglion to increase hypothalamic microglial cells and trigger inflammation in a mechanism that can be inhibited by ghrelin (Waise et al., 2015). Furthermore, in at least one study, the gut microbial landscape changes promoted by consumption of a HFD was shown to play a role in the development of diet-induced hypothalamic microgliosis (Zhuang et al., 2017).
It is worthwhile mentioning that brain regions other than hypothalamus can also present microglial activation in response to excessive consumption of dietary fats (Erion et al., 2014; Spencer et al., 2017). However, differently of the hypothalamus, microgliosis in other regions is a late event, reinforcing the current concept that hypothalamus is the first brain region affected in response to the consumption of large amounts of dietary fats (Thaler et al., 2012; Carraro et al., 2018). Particularly, in the hippocampus, diet-induced microgliosis has been identified in both human and experimental models of Alzheimer’s disease and provides a potential mechanistic link between obesity/type 2 diabetes and the accelerated cognitive and memory decline that is present in this highly prevalent medical condition (Cope et al., 2018).
Intracellular Signaling Targets for Reducing Microglia Inflammatory Activation
Microglial cells express triggering receptor of myeloid cell-2 (TREM2), which is an important negative regulator of NF-κB. In vitro studies showed that TREM2 overexpression inhibits neuroinflammation by down-regulating PI3K/AKT and NF-κB signaling in BV2 microglia (Zhong et al., 2017; Li et al., 2018). Curiously, LPS downregulates the expression of TREM2 through the activation of JNK and NF-κB signaling pathways, resulting in a pro-inflammatory vicious cycle (Zhong et al., 2017).
Quercetin, a polyphenolic flavonoid found in many dietary sources, is a molecule studied due to its anti-inflammatory functional properties and also reduces inflammatory responses through inhibition of NF-κB activity. Quercetin can suppress the lipid content inside the palmitic acid-treated microglia through heme oxygenase (HO-1) induction (Kang et al., 2013; Yang et al., 2017a). HO-1 is an antioxidant enzyme that plays a key role in defense mechanisms against oxidative damage and inflammation. In addition, quercetin suppresses LPS-induced nitric oxide (NO) production and inducible NO synthase (iNOS) expression in BV2 cells (Kang et al., 2013).
Alpha-viniferin belongs to the same polyphenol group as quercetin and has anticancer and anti-inflammatory activity. In LPS-BV2 treated cells, α-viniferin suppresses the expression of the proinflammatory genes iNOS and COX-2 in the early stage of inflammation by inhibiting AKT/PI3K-dependent NF-κB activation and inhibits the production of proinflammatory mediators NO and PGE2 in the late stage by stimulating the Nrf2-mediated HO-1 signaling pathway (Dilshara et al., 2014). It is important to highlight that although many in vivo and in vitro studies have already shown these anti-inflammatory actions, the bioactive effects and bioavailability of these compounds in humans still need to be further investigated (Shanely et al., 2010; Pfeuffer et al., 2013; Lee et al., 2016).
Researchers have also studied other nutrients with functional properties in the context of HFD-induced microglial activation through modulation of NF-κB activity. Eicosapentaenoic acid (EPA) and docosahexaenoic acid (DHA) are the major ω-3 PUFAs of fish oil and have exhibited many anti-inflammatory effects in HFD-induced hypothalamic inflammation (Cintra et al., 2012; Nascimento et al., 2016). In the MG6 and BV2 microglial cell lines, EPA and DHA each suppressed the production of pro-inflammatory cytokines TNF-α and IL-6 throughout the inhibition of NF-κB activity and the activation of SIRT1 signaling (Inoue et al., 2017).
A few studies also investigated the potential involvement of ROS production as a mechanism of transducing inflammatory signals in microglia. Microglial inflammatory-response activation increases the production of oxidative-stress products. It is known that mitochondria constitute the major source of ROS generation in activated microglia (Park et al., 2015). ROS are capable of modifying microglial pro-inflammatory gene expression by modulating kinase cascades and activating some transcription factors, including MAPK and NF-κB (Pawate et al., 2004). LPS-treated BV2 cells show increased ROS production through NADPH oxidase, which raises the expression of TNF-α, activating NF-κB (Sumi et al., 2010). Otherwise, when these cells are treated with a NADPH oxidase inhibitor, ROS production decreases (Mander et al., 2006).
Thus, NF-κB and JNK signaling systems play central roles in the transduction of inflammatory signals in microglia. They can be either activated or inhibited by various nutrients, which make them important targets for approaches aimed at dampening HFD-induced hypothalamic inflammation. It is important to consider that due to the complex nature of human diet as compared to experimental diets offered to rodents, further clinical studies will be required in order to confirm the inflammatory nature of the hypothalamic damage in human obesity.
Microglia Polarization
Microglial cells are able to sense changes in the surrounding environment and become activated to either a pro-inflammatory (M1) or an anti-inflammatory (M2) phenotype (Kettenmann et al., 2011; Tang and Le, 2016). Polarized microglia are distinguished from polarized peripheral macrophages by protein expression, phagocytic capacity, and response to injuries (Durafourt et al., 2012; Girard et al., 2013). Considering that HFD leads to the recruitment of peripheral myeloid cells to the MBH, it is important to understand if some differences exist in the molecular mechanisms of polarization between microglial and infiltrated peripheral myeloid cells in the central nervous system (CNS).
Microglial cell polarization has an energetic demand and, thus, depends on uncoupling protein 2 (UCP2) activity, which is regulated by fatty-acid-binding proteins (FABP) (Hotamisligil and Bernlohr, 2015). Mice lacking FABP4 in the hypothalamus have increased UCP2 expression and reduced expression of TNF-α and ionized calcium-binding adapter molecule 1 (Iba1, a marker of microglial activation (Duffy et al., 2017). Pharmacological inhibition of FABP is an interesting therapeutic approach to reducing saturated-fat-induced pro-inflammatory response because it increases UCP2 expression. In addition, the inhibition of hypothalamic UCP2 increases the inflammatory damage inflicted by TNF-α, which places UCP2 as an important component in the polarization machinery of microglia (Degasperi et al., 2008).
Studies have identified a number of compounds that can reduce inflammation in the CNS by suppressing microglia M1 phenotype (Zhou et al., 2014; Orihuela et al., 2016). Conversely, only a few compounds, such as interleukin-4 and interleukin-13, can promote the polarization of microglia to the M2-like anti-inflammatory phenotype (Zhou et al., 2014). In this context, promoting an M1-M2 switch could be an interesting approach to attenuate inflammation of the neural tissue (Hu et al., 2015; Xu et al., 2015). In line, a recent study showed that betulinic acid (BA), a naturally pentacyclic triterpenoid with anti-inflammatory properties, promotes M2 polarization and inhibits M1 polarization in LPS-stimulated BV2 microglial cells and in the cerebral cortex of LPS-injected mice through calmodulin-dependent protein kinase kinase β (CaMKKβ)-dependent AMP-activated protein kinase (AMPK) activation (Li et al., 2018). In another study, Xu et al. (2015) showed that telmisartan, a potent angiotensin II receptor blocker, also prevents LPS-induced microglia activation throughout M2 microglia polarization through CaMKKβ-dependent AMPK activation. Another mechanism that leads to microglia M1/M2 polarization depends on PGC-1α inhibition, which can be induced by LPS. Some compounds, like resveratrol, a natural polyphenol with anti-inflammatory effects, has beneficial effects on microglia activation throughout PGC-1α activation, inhibiting the M1-like phenotype while stimulating the M2-like phenotype (Yang et al., 2017b).
Although a microglial switch offers a promising avenue for reducing HFD-induced microglial inflammation, further characterization of microglial phenotypic behavior, especially its temporal and spatial evolution, is critical in electing potential therapeutic approaches. Furthermore, it is important to consider that microglial polarization is a dynamic and natural reaction to saturated-fat overconsumption and its complete blockage could impair microglial activity in resolving inflammation.
The Recruitment of Peripheral Myeloid Cells to the Hypothalamus in Dio
Peripheral myeloid cells are recruited to MBH in mice fed a HFD and contribute to the development of obesity-associated hypothalamic microgliosis (Morari et al., 2014; Valdearcos et al., 2017). The fenestrated nature of the BSFI surrounding the MBH is regarded as an important factor involved in this process (Ramalho et al., 2018). Numerous studies showed that bone-marrow-derived microglia (BMDM) can infiltrate the brain parenchyma in distinct types of brain injury (Ajami et al., 2007; Davoust et al., 2008; Prinz and Mildner, 2011). Valdearcos et al. (2017) demonstrated that myeloid cells infiltrating the MBH in mice fed a HFD expressed neither typical microglial markers nor markers indicative of a bloodborne origin. Thus, it was initially suspected that these cells could represent a non-microglial population of brain-resident myeloid cells.
Under normal conditions, BMDM does not renew microglial cells, but under certain circumstances in which cells present in systemic circulation cross the BSFI, they can undergo differentiation into microglia. Thus, the adult bone marrow contains a subpopulation of cells displaying a microglial differentiation potential that can be mobilized promptly at any time (Davoust et al., 2008); however, which factors induce this specific type of monocyte chemotaxis into the MBH in the context of dietary excess is currently under investigation.
Fractalkine (CX3CL1), a chemokine that signals through the CX3CR1 receptor, is involved in this earlier activation of hypothalamic inflammation in HFD-fed mice (Morari et al., 2014). Another chemokine axis, MCP-1/CCR2, has also been described as an important potential mediator of the migration of monocytes into affected areas in brain diseases (Deshmane et al., 2009). Under chronic stress, e.g., a long period of high-fat feeding, the BMDM are recruited to the paraventricular nucleus of the hypothalamus (PVN) throughout, after the activation of the MCP-1/CCR2 axis (Ataka et al., 2013). In this study, the authors blocked the recruitment by peripheral administration of a CCR2 antagonist, which resulted in a reduction of local inflammation, providing evidence of an important role of the MCP-1/CCR2 axis in diet-induced hypothalamic inflammation.
Although the origin and identity of myeloid cells that migrate to the MBH after HFD consumption are unknown, these cells displayed morphological features of resident microglia (Valdearcos et al., 2017). The BMDM, as resident microglia, can be clearly distinguished from other CNS-infiltrating macrophages, based on its CD45 expression (Priller et al., 2001). In addition, BMDM can proliferate in the neural tissue when inflammation is induced, whereas infiltrating macrophages do not (Wirenfeldt et al., 2007).
Another important question still unanswered refers to the mechanism that induces the recruitment of BMDM into the peripheral circulation because it precedes recruitment to the CNS. SDF-1/CXCR4 interaction is required to recruit BMDM out of the bone marrow toward systemic circulation (Katayama et al., 2006). SDF-1 expression, in turn, is regulated by brain stimuli through sympathetic pathways, throughout β3-adrenergic receptors on bone marrow stromal cells (Lilly et al., 2011). Thus, it is possible that the signal that triggers the whole process is mediated by autonomic connections between the hypothalamus and the bone marrow; however, further studies are needed to clarify this important issue.
In conclusion, the current data are reshaping the concept that resident microglia constitutes a stable brain-cell population that is not affected by the recruitment of BMDM (Matsumoto and Fujiwara, 1987; Lassmann et al., 1993). Certain types of brain injury, including the damage caused in the hypothalamus by the excessive consumption of SFAs, can recruit BMDM, which seem to be an important event to modulate the inflammatory response. Particularly, after consumption of a HFD, it seems the recruitment of peripheral myeloid cells to the hypothalamus occurs in an attempt to help the resident microglia smother the inflammatory response. As this response happens at the beginning of HFD overconsumption, exploring which mechanisms are involved and how it alters hypothalamic neurocircuits involved in metabolic control represents a new frontier for proposing a viable therapeutic approach before the damage is established. Figure 1 provides a summarized view of the mechanisms involved in the regulation of hypothalamic microglia in obesity.
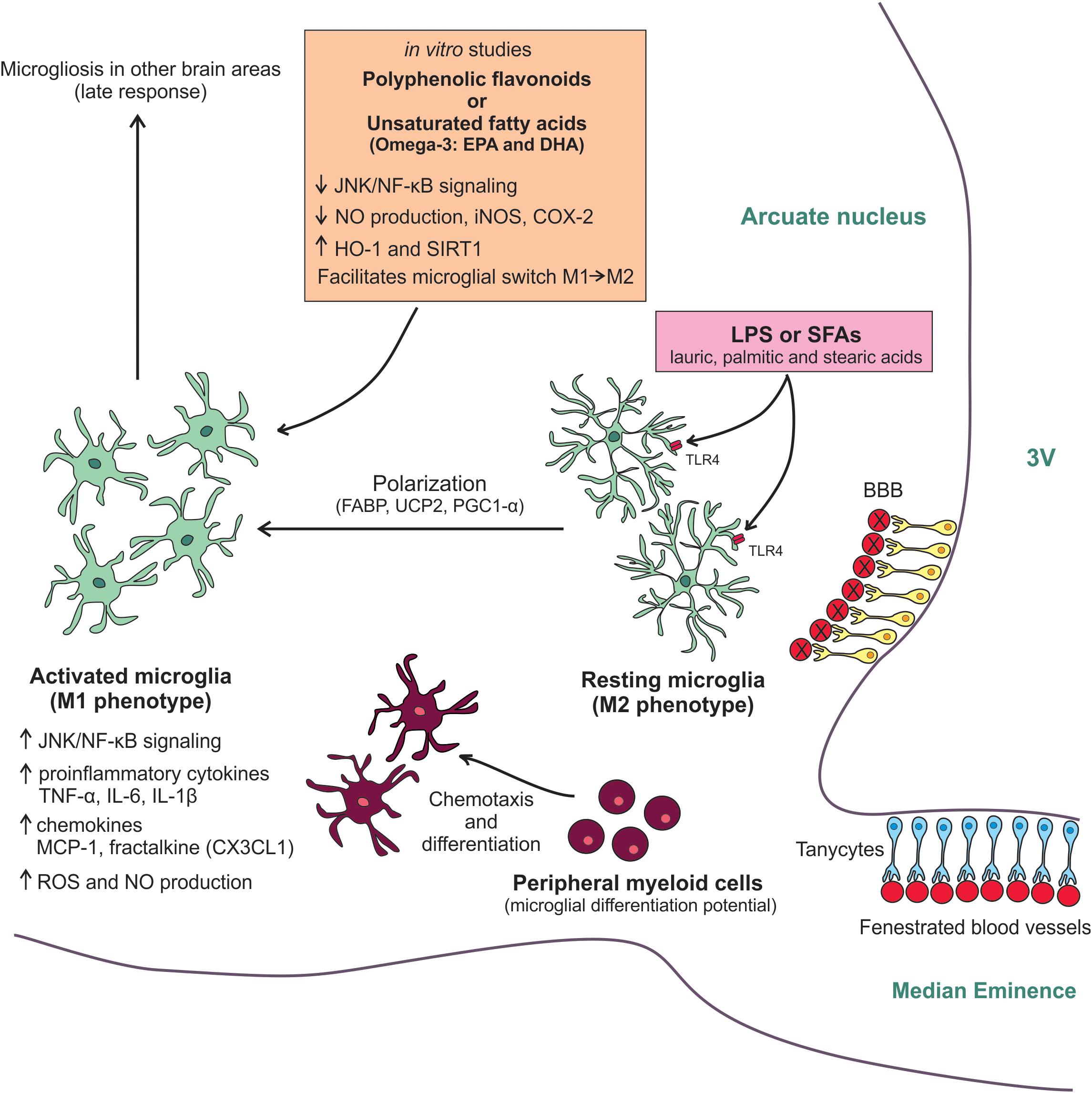
FIGURE 1. Molecular mechanisms involved in the HFD-induced hypothalamic microgliosis. Diet-induced inflammation initially affects the MBH. Due to its anatomic location, microglial cells of arcuate nucleus are sensitive to LPS and SFAs from the diet. Both molecules activate TLR4 in the microglia membrane resulting in the switching to M1-like pro-inflammatory phenotype. This M2/M1 polarization has an energetic demand and depends on UCP2 activity, which is regulated by FABP, and on PGC-1α activation. The microglia activation results in an increased JNK/NF-kB activity, raised expression of pro-inflammatory cytokines and chemokines, as well as increased ROS and NO production. With the persistence of the high-fat feeding, peripheral myeloid cells are recruited to the neural tissue. These cells have a microglial differentiation potential and can quickly differentiate in activated cells helping resident microglia smother the hypothalamic inflammatory response. The chemotaxis of these peripheral cells is mainly controlled by MCP-1/CCR2 axis and fractalkine. As a late response, the microgliosis can also affect other non-hypothalamic brains areas. Some anti-inflammatory and antioxidants molecules, such as polyphenolic flavonoids and unsaturated fatty acids, have been tested in vitro for attenuating the microglial activation. The main benefits found in these studies were the reduction of JNK/NF-kB activity, decreased NO production, as well as iNOS and COX-2 expression, and increased HO-1 and SIRT-1 expression. Besides, these molecules also seem to facilitate the microglial switch M1/M2, reducing the inflammatory response. Further studies are still needed to further test these mechanisms in experimental models.
Outstanding Questions
(1) How do different subsets of microglia participate in the HFD-induced hypothalamic inflammation?
(2) Could microglia from other brain areas also migrate to the MBH contributing to the local inflammation?
(3) Is the recruitment of BMDM triggered by hypothalamic outputs? If so, which mechanisms are involved?
(4) Is there inflammation and microglial activation in the hypothalamus of obese humans? If so, is there any possibility of pharmacologically targeting this inflammation to treat human obesity?
Author Contributions
All authors wrote the paper and approved the final version of the manuscript.
Funding
The writing of this article was supported by Fundação de Amparo à Pesquisa do Estado de São Paulo (FAPESP, 2013/07607-8, and 2017/02983-2) and by the National Institutes of Health (R01DK106076 to Y-BK) NM is a recipient of a doctorate fellowship provided by São Paulo Research Foundation to (FAPESP, 2016/17810-3, and 2017/22511-8). The Laboratory of Cell Signaling belongs to the Obesity and Comorbidities Research Center and the National Institute of Science and Technology – Diabetes and Obesity.
Conflict of Interest Statement
The authors declare that the research was conducted in the absence of any commercial or financial relationships that could be construed as a potential conflict of interest.
References
Ajami, B., Bennett, J. L., Krieger, C., Tetzlaff, W., and Rossi, F. M. (2007). Local selfrenewal can sustain CNS microglia maintenance and function throughout adult life. Nat. Neurosci. 10, 1538–1543. doi: 10.1038/nn2014
Ataka, K., Asakawa, A., Nagaishi, K., Kaimoto, K., Sawada, A., Hayakawa, Y., et al. (2013). Bone marrow-derived microglia infiltrate into the paraventricular nucleus of chronic psychological stress-loaded mice. PLoS One 8:e81744. doi: 10.1371/journal.pone.0081744
Baufeld, C., Osterloh, A., Prokop, S., Miller, K. R., and Heppner, F. L. (2016). High-fat diet-induced brain region-specific phenotypic spectrum of CNS resident microglia. Acta Neuropathol. 132, 361–375. doi: 10.1007/s00401-016-1595-4
Buttini, M., Limonta, S., and Boddeke, H. W. (1996). Peripheral administration of lipopolysaccharide induces activation of microglial cells in rat brain. Neurochem. Int. 29, 25–35.
Carraro, R. S., Souza, G. F., Solon, C., Razolli, D. S., Chausse, B., Barbizan, R., et al. (2018). Hypothalamic mitochondrial abnormalities occur downstream of inflammation in diet-induced obesity. Mol. Cell. Endocrinol. 460, 238–245. doi: 10.1016/j.mce.2017.07.029
Cheung, A. K. L., Kwok, H. Y., Huang, Y., Chen, M., Mo, Y., Wu, X., et al. (2017). Gut-homing Δ42PD1+Vδ2 T cells promote innate mucosal damage via TLR4 during acute HIV type 1 infection. Nat. Microbiol. 2, 1389–1402. doi: 10.1038/s41564-017-0006-5
Cintra, D. E., Ropelle, E. R., Moraes, J. C., Pauli, J. R., Morari, J., and Souza, C. T. (2012). Unsaturated fatty acids revert diet-induced hypothalamic inflammation in obesity. PLoS One 7:e30571. doi: 10.1371/journal.pone.0030571
Cope, E. C., LaMarca, E. A., Monari, P. K., Olson, L. B., Martinez, S., Zych, A. D., et al. (2018). Microglia play an active role in obesity-associated cognitive decline. J. Neurosci. 38, 8889–8904. doi: 10.1523/JNEUROSCI.0789-18.2018
Davoust, N., Vuaillat, C., Androdias, G., and Nataf, S. (2008). From bone marrow to microglia: barriers and avenues. Trends Immunol. 29, 227–234. doi: 10.1016/j.it.2008.01.010
De Souza C. T., Araujo, E. P., Bordin, S., Ashimine, R., Zollner, R. L., Boschero, A. C., et al. (2005). Consumption of a fat-rich diet activates a proinflammatory response and induces insulin resistance in the hypothalamus. Endocrinology 146, 4192–4199. doi: 10.1210/en.2004-1520
Degasperi, G. R., Romanatto, T., Denis, R. G., Araújo, E. P., Moraes, J. C., Inada, N. M., et al. (2008). UCP2 protects hypothalamic cells from TNF-alpha-induced damage. FEBS Lett. 582, 3103–3110. doi: 10.1016/j.febslet.2008.08.006
Deshmane, S. L., Kremlev, S., Amini, S., and Sawaya, B. E. (2009). Monocyte chemoattractant protein-1 (MCP-1): an overview. J. Interferon Cytokine Res. 29, 313–326. doi: 10.1089/jir.2008.0027
Dilshara, M. G., Lee, K. T., Kim, H. J., Lee, H. J., Choi, Y. H., Lee, C. M., et al. (2014). Anti-inflammatory mechanism of α-viniferin regulates lipopolysaccharide-induced release of proinflammatory mediators in BV2 microglial cells. Cell. Immunol. 290, 21–29. doi: 10.1016/j.cellimm.2014.04.009
Dorfman, M. D., and Thaler, J. P. (2015). Hypothalamic inflammation and gliosis in obesity. Curr. Opin. Endocrinol. Diabetes Obes. 22, 325–330. doi: 10.1097/MED.0000000000000182
Dragano, N. R., Haddad-Tovolli, R., and Velloso, L. A. (2017). Leptin, neuroinflammation and obesity. Front. Horm. Res. 48, 84–96. doi: 10.1159/000452908
Duffy, C. M., Xu, H., Nixon, J. P., Bernlohr, D. A., and Butterick, T. A. (2017). Identification of a fatty acid binding protein4-UCP2 axis regulating microglial mediated neuroinflammation. Mol. Cell. Neurosci. 80, 52–57. doi: 10.1016/j.mcn.2017.02.004
Durafourt, B. A., Moore, C. S., Zammit, D. A., Johnson, T. A., Zaguia, F., and Guiot, M. C. (2012). Comparison of polarization properties of human adult microglia and blood-derived macrophages. Glia 60, 717–727. doi: 10.1002/glia.22298
Erion, J. R., Wosiski-Kuhn, M., Dey, A., Hao, S., Davis, C. L., Pollock, N. K., et al. (2014). Obesity elicits interleukin 1-mediated deficits in hippocampal synaptic plasticity. J. Neurosci. 34, 2618–2631. doi: 10.1523/JNEUROSCI.4200-13.2014
Gao, Y., Ottaway, N., Schriever, S. C., Legutko, B., García-Cáceres, C., de la Fuente, E., et al. (2014). Hormones and diet, but not body weight, control hypothalamic microglial activity. Glia 62, 17–25. doi: 10.1002/glia.22580
García-Bueno, B., Serrats, J., and Sawchenko, P. E. (2009). Cerebrovascular cyclooxygenase-1 expression, regulation, and role in hypothalamic-pituitary-adrenal axis activation by inflammatory stimuli. J. Neurosci. 29, 12970–12981. doi: 10.1523/JNEUROSCI.2373-09.2009
Girard, S., Brough, D., Lopez-Castejon, G., Giles, J., Rothwell, N. J., and Allan, S. M. (2013). Microglia and macrophages differentially modulate cell death after brain injury caused by oxygen-glucose deprivation in organotypic brain slices. Glia 61, 813–824. doi: 10.1002/glia.22478
Hotamisligil, G. S., and Bernlohr, D. A. (2015). Metabolic functions of FABPs–mechanisms and therapeutic implications. Nat. Rev. Endocrinol. 11, 592–605. doi: 10.1038/nrendo.2015.122
Hu, X., Leak, R. K., Shi, Y., Suenaga, J., Gao, Y., Zheng, P., et al. (2015). Microglial and macrophage polarization-new prospects for brain repair. Nat. Rev. Neurol. 11, 56–64. doi: 10.1038/nrneurol.2014.207
Huang, S., Rutkowsky, J. M., Snodgrass, R. G., Ono-Moore, K. D., Schneider, D. A., and Newman, J. W. (2012). Saturated fatty acids activate TLR-mediated proinflammatory signaling pathways. J. Lipid Res. 53, 2002–2013. doi: 10.1194/jlr.D029546
Inoue, T., Tanaka, M., Masuda, S., Ohue-Kitano, R., Yamakage, H., Muranaka, K., et al. (2017). Omega-3 polyunsaturated fatty acids suppress the inflammatory responses of lipopolysaccharide-stimulated mouse microglia by activating SIRT1 pathways. Biochim. Biophys. Acta 1862, 552–560. doi: 10.1016/j.bbalip.2017.02.010
Kang, C. H., Choi, Y. H., Moon, S. K., Kim, W. J., and Kim, G. Y. (2013). Quercetin inhibits lipopolysaccharide-induced nitric oxide production in BV2 microglial cells by suppressing the NF-κB pathway and activating the Nrf2-dependent HO-1 pathway. Int. Immunopharmacol. 17, 808–813. doi: 10.1016/j.intimp.2013.09.009
Katayama, Y., Battista, M., Kao, W. M., Hidalgo, A., Peired, A. J., Thomas, S. A., et al. (2006). Signals from the sympathetic nervous system regulate hematopoietic stem cell egress from bone marrow. Cell 124, 407–421. doi: 10.1016/j.cell.2005.10.041
Kerfoot, S. M., Long, E. M., Hickey, M. J., Andonegui, G., Lapointe, B. M., Zanardo, R. C., et al. (2004). TLR4 contributes to disease-inducing mechanisms resulting in central nervous system autoimmune disease. J. Immunol. 173, 7070–7077. doi: 10.4049/jimmunol.173.11.7070
Kettenmann, H., Hanisch, U. K., Noda, M., and Verkhratsky, A. (2011). Physiology of microglia. Physiol. Rev. 91, 461–553. doi: 10.1152/physrev.00011.2010
Kigerl, K. A., de Rivero Vaccari, J. P., Dietrich, W. D., Popovich, P. G., and Keane, R. W. (2014). Pattern recognition receptors and central nervous system repair. Exp. Neurol. 258, 5–16. doi: 10.1016/j.expneurol.2014.01.001
Lam, T. K., Schwartz, G. J., and Rossetti, L. (2005). Hypothalamic sensing of fatty acids. Nat. Neurosci. 8, 579–584. doi: 10.1038/nn1456
Lassmann, H., Schmied, M., Vass, K., and Hickey, W. F. (1993). Bone marrow derived elements and resident microglia in brain inflammation. Glia 7, 19–24.
Ledo, J. H., Azevedo, E. P., Beckman, D., Ribeiro, F. C., Santos, L. E., Razolli, D. S., et al. (2016). Cross talk between brain innate immunity and serotonin signaling underlies depressive-like behavior induced by Alzheimer’s amyloid-β oligomers in mice. J. Neurosci. 36, 12106–12116. doi: 10.1523/JNEUROSCI.1269-16.2016
Lee, J., Cha, Y., Lee, K., and Yim, J. (2016). Onion peel extract reduces the percentage of body fat in overweight and obese subjects: a 12-week, randomized, double-blind, placebo-controlled study. Nutr. Res. Pract. 10, 175–181. doi: 10.4162/nrp.2016.10.2.175
Li, C., Zhang, C., Zhou, H., Feng, Y., Tang, F., Hoi, M. P. M., et al. (2018). Inhibitory effects of betulinic acid on LPS-induced neuroinflammation involve M2 microglial polarization via CaMKKβ-dependent AMPK activation. Front. Mol. Neurosci. 11:98. doi: 10.3389/fnmol.2018.00098
Lilly, A. J., Johnson, W. E., and Bunce, C. M. (2011). The haematopoietic stem cell niche: new insights into the mechanisms regulating haematopoietic stem cell behaviour. Stem Cells Int. 2011:274564. doi: 10.4061/2011/274564
Little, J. P., Madeira, J. M., and Klegeris, A. (2012). The saturated fatty acid palmitate induces human monocytic cell toxicity toward neuronal cells: exploring a possible link between obesity-related metabolic impairments and neuroinflammation. J. Alzheimers Dis. 2, S179–S183. doi: 10.3233/JAD-2011-111262
Mander, P. K., Jekabsone, A., and Brown, G. C. (2006). Microglia proliferation is regulated by hydrogen peroxide from NADPH oxidase. J. Immunol. 76, 1046–1052.
Matsumoto, Y., and Fujiwara, M. (1987). Absence of donor-type major histocompatibility complex class I antigen-bearing microglia in the rat central nervous system of radiation bone marrow chimeras. J. Neuroimmunol. 17, 71–82.
Milanski, M., Arruda, A. P., Coope, A., Ignacio-Souza, L. M., Nunez, C. E., Roman, E. A., et al. (2012). Inhibition of hypothalamic inflammation reverses diet-induced insulin resistance in the liver. Diabetes 61, 1455–1462. doi: 10.2337/db11-0390
Milanski, M., Degasperi, G., Coope, A., Morari, J., Denis, R., Cintra, D. E., et al. (2009). Saturated fatty acids produce an inflammatory response predominantly through the activation of TLR4 signaling in hypothalamus: implications for the pathogenesis of obesity. J. Neurosci. 29, 359–370. doi: 10.1523/JNEUROSCI.2760-08.2009
Moraes, J. C., Coope, A., Morari, J., Cintra, D. E., Roman, E. A., Pauli, J. R., et al. (2009). High-fat diet induces apoptosis of hypothalamic neurons. PLoS One 4:e5045. doi: 10.1371/journal.pone.0005045
Morari, J., Anhe, G. F., Nascimento, L. F., de Moura, R. F., Razolli, D., Solon, C., et al. (2014). Fractalkine (CX3CL1) is involved in the early activation of hypothalamic inflammation in experimental obesity. Diabetes 63, 3770–3784. doi: 10.2337/db13-1495
Mulders, R. J., de Git, K. C. G., Schéle, E., Dickson, S. L., Sanz, Y., and Adan, R. A. H. (2018). Microbiota in obesity: interactions with enteroendocrine, immune and central nervous systems. Obes. Rev. 19, 435–451. doi: 10.1111/obr.12661
Nascimento, L. F., Souza, G. F., Morari, J., Barbosa, G. O., Solon, C., Moura, R. F., et al. (2016). n-3 fatty acids induce neurogenesis of predominantly POMC-expressing cells in the hypothalamus. Diabetes 65, 673–686. doi: 10.2337/db15-0008
Orihuela, R., McPherson, C. A., and Harry, G. J. (2016). Microglial M1/M2 polarization and metabolic states. Br. J. Pharmacol. 173, 649–665. doi: 10.1111/bph.13139
Pan, W. W., and Myers, M. G. Jr. (2018). Leptin and the maintenance of elevated body weight. Nat. Rev. Neurosci. 19, 95–105. doi: 10.1038/nrn.2017.168
Park, J., Min, J. S., Kim, B., Chae, U. B., Yun, J. W., Choi, M. S., et al. (2015). Mitochondrial ROS govern the LPS-induced pro-inflammatory response in microglia cells by regulating MAPK and NF-κB pathways. Neurosci. Lett. 584, 191–196. doi: 10.1016/j.neulet.2014.10.016
Pawate, S., Shen, Q., Fan, F., and Bhat, N. R. (2004). Redox regulation of glial inflammatory response to lipopolysaccharide and interferongamma. J. Neurosci. Res. 77, 540–551. doi: 10.1002/jnr.20180
Pfeuffer, M., Auinger, A., Bley, U., Kraus-Stojanowic, I., Laue, C., Winkler, P., et al. (2013). Effect of quercetin on traits of the metabolic syndrome, endothelial function and inflammation in men with different APOE isoforms. Nutr. Metab. Cardiovasc. Dis. 23, 403–409. doi: 10.1016/j.numecd.2011.08.010
Priller, J., Flügel, A., Wehner, T., Boentert, M., Haas, C. A., and Prinz, M. (2001). Targeting gene-modified hematopoietic cells to the central nervous system: use of green fluorescent protein uncovers microglial engraftment. Nat. Med. 7, 1356–1361. doi: 10.1038/nm1201-1356
Prinz, M., and Mildner, A. (2011). Microglia in the CNS: immigrants from another world. Glia. 59, 177–187. doi: 10.1002/glia.21104
Ramalho, A. F., Bombassaro, B., Dragano, N. R., Solon, C., Morari, J., and Fioravante, M. (2018). Dietary fats promote functional and structural changes in the median eminence blood/spinal fluid interface-the protective role for BDNF. J. Neuroinflammation 15:10. doi: 10.1186/s12974-017-1046-8
Rodríguez, E. M., Blázquez, J. L., and Guerra, M. (2010). The design of barriers in the hypothalamus allows the median eminence and the arcuate nucleus to enjoy private milieus: the former opens to the portal blood and the latter to the cerebrospinal fluid. Peptides. 31, 757–776. doi: 10.1016/j.peptides.2010.01.003
Saijo, K., and Glass, C. K. (2011). Microglial cell origin and phenotypes in health and disease. Nat. Rev. Immunol. 11, 775–787. doi: 10.1038/nri3086
Schaeffer, M., Langlet, F., Lafont, C., Molino, F., Hodson, D. J., Roux, T., et al. (2013). Rapid sensing of circulating ghrelin by hypothalamic appetite-modifying neurons. Proc. Natl. Acad. Sci. U.S.A. 110, 1512–1517. doi: 10.1073/pnas.1212137110
Schwartz, M. W., Woods, S. C., Porte, D. Jr., Seeley, R. J., and Baskin, D. G. (2000). Central nervous system control of food intake. Nature 404, 661–671. doi: 10.1038/35007534
Shanely, R. A., Knab, A. M., Nieman, D. C., Jin, F., McAnulty, S. R., and Landram, M. J. (2010). Quercetin supplementation does not alter antioxidant status in humans. Free Radic. Res. 44, 224–231. doi: 10.3109/10715760903407293
Spencer, S. J., D’Angelo, H., Soch, A., Watkins, L. R., Maier, S. F., and Barrientos, R. M. (2017). High-fat diet and aging interact to produce neuroinflammation and impair hippocampal- and amygdalar-dependent memory. Neurobiol. Aging 58, 88–101. doi: 10.1016/j.neurobiolaging.2017.06.014
Sumi, N., Nishioku, T., Takata, F., Matsumoto, J., Watanabe, T., and Shuto, H. (2010). Lipopolysaccharide-activated microglia induce dysfunction of the blood-brain barrier in rat microvascular endothelial cells co-cultured with microglia. Cell Mol. Neurobiol. 30, 247–253. doi: 10.1007/s10571-009-9446-7
Tang, Y., and Le, W. (2016). Differential roles of M1 and M2 microglia in neurodegenerative diseases. Mol. Neurobiol. 53, 1181–1194. doi: 10.1007/s12035-014-9070-5
Thaler, J. P., Yi, C. X., Schur, E. A., Guyenet, S. J., Hwang, B. H., Dietrich, M. O., et al. (2012). Obesity is associated with hypothalamic injury in rodents and humans. J. Clin. Invest. 122, 153–162. doi: 10.1172/JCI59660
Timper, K., and Brüning, J. C. (2017). Hypothalamic circuits regulating appetite and energy homeostasis: pathways to obesity. Dis. Model Mech. 10, 679–689. doi: 10.1242/dmm.026609
Valdearcos, M., Douglass, J. D., Robblee, M. M., Dorfman, M. D., Stifler, D. R., and Bennett, M. L. (2017). Microglial inflammatory signaling orchestrates the hypothalamic immune response to dietary excess and mediates obesity susceptibility. Cell Metab. 26, 185–197. doi: 10.1016/j.cmet.2017.05.015
Valdearcos, M., Robblee, M. M., and Benjamin, D. I. (2014). Microglia dictate the impact of saturated fat consumption on hypothalamic inflammation and neuronal function. Cell Rep. 9, 2124–2138. doi: 10.1016/j.celrep.2014.11.018
van de Sande-Lee, S., Pereira, F. R., Cintra, D. E., Fernandes, P. T., Cardoso, A. R., Garlipp, C. R., et al. (2011). Partial reversibility of hypothalamic dysfunction and changes in brain activity after body mass reduction in obese subjects. Diabetes 60, 1699–1704. doi: 10.2337/db10-1614
Velloso, L. A., and Schwartz, M. W. (2011). Altered hypothalamic function in diet-induced obesity. Int. J. Obes. 35, 1455–1465. doi: 10.1038/ijo.2011.56
Waise, T. M. Z., Toshinai, K., Naznin, F., NamKoong, C., Md Moin, A. S., Sakoda, H., et al. (2015). One-day high-fat diet induces inflammation in the nodose ganglion and hypothalamus of mice. Biochem. Biophys. Res. Commun. 464, 1157–1162. doi: 10.1016/j.bbrc.2015.07.097
Wang, Z., Liu, D., Wang, F., Liu, S., Zhao, S., Ling, E. A., et al. (2012). Saturated fatty acids activate microglia via Toll-like receptor 4/NF-kappaB signalling. Br. J. Nutr. 107, 229–241. doi: 10.1017/S0007114511002868
Wirenfeldt, M., Dissing-Olesen, L., Babcock, A. A., Nielsen, M., Meldgaard, M., and Zimmer, J. (2007). Population control of resident and immigrant microglia by mitosis and apoptosis. Am. J. Pathol. 171, 617–631. doi: 10.2353/ajpath.2007.061044
Xu, H., Xiao, T., Chen, C. H., Li, W., Meyer, C. A., and Wu, Q. (2015). Sequence determinants of improved CRISPR sgRNA design. Genome Res. 25, 1147–1157. doi: 10.1101/gr.191452.115
Yang, J., Kim, C., Tu, T., Kim, M., Goto, T., Kawada, T., et al. (2017a). Quercetin protects Obesity-Induced hypothalamic inflammation by reducing microglia-mediated inflammatory responses via HO-1 induction. Nutrients 9:650. doi: 10.3390/nu9070650
Yang, X., Xu, S., Qian, Y., and Xiao, Q. (2017b). Resveratrol regulates microglia M1/M2 polarization via PGC-1α in conditions of neuroinflammatory injury. Brain Behav. Immun. 64, 162–172. doi: 10.1016/j.bbi.2017.03.003
Zhang, X., Zhang, G., Zhang, H., Karin, M., Bai, H., and Cai, D. (2008). Hypothalamic IKKβ/NF-κB and ER stress link overnutrition to energy imbalance and obesity. Cell 135, 61–73. doi: 10.1016/j.cell.2008.07.043
Zhong, L., Zhang, Z. L., Li, X., Liao, C., Mou, P., Wang, T., et al. (2017). TREM2/DAP12 complex regulates inflammatory responses in microglia via the JNK signaling pathway. Front. Aging Neurosci. 9:204. doi: 10.3389/fnagi.2017.00204
Zhou, D., Huang, C., Lin, Z., Zhan, S., Kong, L., Fang, C., et al. (2014). Macrophage polarization and function with emphasis on the evolving roles of coordinated regulation of cellular signaling pathways. Cell. Signal. 26, 192–197. doi: 10.1016/j.cellsig.2013.11.004
Keywords: inflammation, chemokine, cytokine, brain, metabolism
Citation: Mendes NF, Kim Y-B, Velloso LA and Araújo EP (2018) Hypothalamic Microglial Activation in Obesity: A Mini-Review. Front. Neurosci. 12:846. doi: 10.3389/fnins.2018.00846
Received: 29 August 2018; Accepted: 29 October 2018;
Published: 15 November 2018.
Edited by:
Alexandra Latini, Universidade Federal de Santa Catarina, BrazilReviewed by:
Gisela Helfer, University of Bradford, United KingdomAlex Rafacho, Universidade Federal de Santa Catarina, Brazil
Aline Pertile Remor, University of West of Santa Catarina, Brazil
Roberta De Paula Martins, Universidade Federal de Santa Catarina, Brazil
Copyright © 2018 Mendes, Kim, Velloso and Araújo. This is an open-access article distributed under the terms of the Creative Commons Attribution License (CC BY). The use, distribution or reproduction in other forums is permitted, provided the original author(s) and the copyright owner(s) are credited and that the original publication in this journal is cited, in accordance with accepted academic practice. No use, distribution or reproduction is permitted which does not comply with these terms.
*Correspondence: Eliana P. Araújo, ZWFyYXVqb0B1bmljYW1wLmJy; cGEuZWxpYW5hQGdtYWlsLmNvbQ==