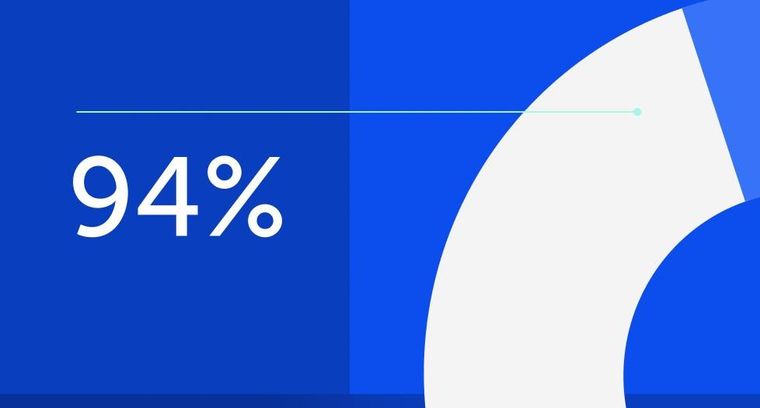
94% of researchers rate our articles as excellent or good
Learn more about the work of our research integrity team to safeguard the quality of each article we publish.
Find out more
SYSTEMATIC REVIEW article
Front. Neurosci., 05 September 2018
Sec. Neural Technology
Volume 12 - 2018 | https://doi.org/10.3389/fnins.2018.00577
This article is part of the Research Topic10 Years of Impactful, Open NeuroscienceView all 47 articles
This paper overviews the state-of-the-art in upper limb robot-supported approaches, focusing on advancements in the related mechatronic devices for the patients' rehabilitation and/or assistance. Dedicated to the technical, comprehensively methodological and global effectiveness and improvement in this inter-disciplinary field of research, it includes information beyond the therapy administrated in clinical settings-but with no diminished safety requirements. Our systematic review, based on PRISMA guidelines, searched articles published between January 2001 and November 2017 from the following databases: Cochrane, Medline/PubMed, PMC, Elsevier, PEDro, and ISI Web of Knowledge/Science. Then we have applied a new innovative PEDro-inspired technique to classify the relevant articles. The article focuses on the main indications, current technologies, categories of intervention and outcome assessment modalities. It includes also, in tabular form, the main characteristics of the most relevant mobile (wearable and/or portable) mechatronic/robotic orthoses/exoskeletons prototype devices used to assist-rehabilitate neuromotor impairments in the upper limb.
What differentiates human beings from animals is the superior psycho-cognitive activity, including the coordinated/complex, workable, actions of its highly correlated physical effecter: the upper limb, and especially the hand—as basis of our creative and modeler/draftsman kind interactions with the environment. This profound and subtle reality has been conceptualized during history by great thinkers, such as Aristotel (2005), Descartes, Newton and Kant (Lundborg, 2014).
Accordingly, finding solutions that address rehabilitation and/or functional assistance of neuromotor impairments at this level would have a remarkable positive impact: for the beneficiaries' quality of life (Frisoli et al., 2016) and from a socio-economical perspective, as well. The latter corresponds to the temporary regain/re-insertion of the productive resources lost because of the disabilities in their upper limbs. Moreover, it is to be considered, within the general context/trend of offering a reliable alternative for prolonged hospitalizations, the need for top of the range assistive/rehabilitative orthotic mobile devices. These should be capable to provide safe and of continuity rehabilitation (Loureiro et al., 2011) and/or functional assistance for the above mentioned topography, too, of neuromotor deficits including in the patient's daily life context. Such endeavors are often necessary on long term, mainly imposed, in the morbidity domain we approach, by the required duration of neuroplasticity to install/act (Muresanu et al., 2012; Basteris et al., 2014; Xiao et al., 2014; Proietti et al., 2016; Mazzoleni et al., 2017), to be (re)settled in adequate engrams for the function(s) aimed at restoring, and/or of peripheral nerves' re-growth (Guyton and Hall, 2006).
The necessity for such devices that can operate without fatigue in both clinical settings, at home and in the community is growing high (Stewart et al., 2017). This is despite of the fact that people with upper limb pathology—who do not necessarily suffer from functional issues in the lower limbs—can commonly reach clinical units (in order to receive ambulatory rehabilitative specific procedures). Another aspect is that, at the moment, there is already a “shortage” of professionals handy to deliver domiciliary physiotherapy/rehabilitation and nursing, for persons with physical impairments. This is a worrying situation, especially as it is foreseen to become more and more frequent in the years to come (Maciejasz et al., 2014).
An important related development direction consists of consolidating their wearable profile. This practically entails—subsumed to a rightful beneficiary's desire: “several hours” per day of working performance (Allotta et al., 2015)—availability for autonomous powered duty (as for easily/rapidly rechargeable facilities, too) and respectively comfortable bearing by the consumer in the daily life (Giberti et al., 2014), limitation of encumbrances, lightweight (Rocon et al., 2007; Martinez et al., 2008; Song et al., 2013, 2014; Chen et al., 2014; Giberti et al., 2014; Andrikopoulos et al., 2015; Allotta et al., 2015; Polygerinos et al., 2015; Guo et al., 2016; Nycz et al., 2016; Alavi et al., 2017; Stewart et al., 2017) and modularity (Lo et al., 2010; Pearce et al., 2012; Noveanu et al., 2013; Xiao et al., 2014; Nycz et al., 2016) and/or, in some cases, “reconfigurability” (Maciejasz et al., 2014).
Considering all the necessary technical assets for such advanced devices to be mobile (Kiguchi et al., 2008a; Lee, 2014; Nycz et al., 2016), thereby available for individual more extended use, an additional, non-technical, but derivative and decisive condition is, as well, mandatory: their cost-effectiveness (Noveanu et al., 2013).
We consider it only appropriate to iterate here a summarized idea of a previous work of ours (Onose et al., 2016) that currently there is still no such thing as an optimal, fully functional assistive-rehabilitative device (in the common sense of the term). This regards mainly: don/doff issues (Nimawat and Jailiya, 2015)–for severely disabled potential beneficiaries–, psychological acceptance (of self image/esteem kind, referring to the ensemble look of the consumer: enough miniaturization and cosmetics– thus either reaching a satisfactory clothes-like aspect or even becoming as thin as to evolve to underwear dimensions), extended power autonomy, easy and fast set-up-for professionals (Dijkers et al., 1991). Another important feature for the customers/their kin is the appropriateness for long time duty in various real life situations. One should consider also the consistent related safety, producing very low/practically imperceptible noise when in service and truly affordable/cost effective.
Despite the rigorous selection filter-classification criteria based methodology of the papers we have reviewed, some data referring to the subject matter approached, might still be overlooked. At the same time, on one hand, not all the selected articles contained aspects needing to be elicited and expressly quoted. On the other, for clarifying different notions (collateral but nevertheless, important for this paper) we also used Supplementary—to the portfolio gathered availing the below mentioned combinations of keywords—bibliographic resources.
According to the afore exposed rationale, our research considered publications from January 2001 to November 2017 from the following databases: Cochrane1, Medline/PubMed2, PMC3, Elsevier4, PEDro5, ISI Web of Knowledge/Science6.
Our search has been conducted on five stages, described by a Preferred Reporting Items for Systematic Reviews and Meta-Analyses (PRISMA)7 adapted flow diagram, but without the final meta-analysis stage (see Figure 1). Within the first stage, using the following combinations of keywords: upper limb exoskeleton, upper limb mobile robot, upper limb wearable robot, upper limb portable robot, upper limb robotic exoskeleton, upper limb robotic orthosis, upper limb robotic device, it resulted, as expected—even if overlapping—a huge amount of articles: (more than 13,000-details in Table 1).
Therefore, we still had to achieve a mandatory refinement: within the second stage, we applied contextual search, using the same above mentioned combinations of keywords, but inside quotation marks. It then resulted in over 270 articles (details in Table 2).
After eliminating all the inevitable redundancies (i.e., same article found in different queried data bases), in the next stage we have selected the most relevant articles to our targeted subject by the keywords: “mobile,” “wearable,” and respectively, “portable”—to be found in their titles and/or abstracts. This has reduced the number of results to: 3 articles for “mobile,” 29 for “wearable,” and 12 for “portable“ (but among them, 6 contained both, the “wearable” and “portable” keywords). After collecting all the respective open access articles, we had directly bought the non-available ones (with an exception: one article which was not available on the seller's platform) and then retained 37 non-redundant articles. On these we have implemented an own, customized, PEDro inspired, classification algorithm—described below.
In this purpose, we have previously considered connected literature knowledge from the Population/ Intervention/Comparison/Outcome/Time–PICOT (Fineout-Overholt et al., 2010; Riva et al., 2012)–and Study Type–PICOS (Methley et al., 2014)–, Feasible/Interesting/Novel/ Ethic/Relevant–FINER–(Farrugia et al., 2010) and Physiotherapy Evidence Database criteria—PEDro8—(Maher et al., 2003). We had in mind some essential/defining features probable to be found in the approached domain (specifically, in our selected articles): studies using prototypes, with single (24.32%), small (21.62%), or multiple (10.81%) case/s series (details and related references in the results section). Additionally, the studies of interest for our subject matter may involve different interventions, with mobile robotic orthotic devices, where randomization “concealed/blindness” criteria are difficult to be applied/(not always found), observations on healthy subjects (63.28%)—details and related references in the results section—various assessment scales and/or end-points, used.
Consequently, we have developed [based on a Delphi kind preliminary related endeavor: brainstorming between the authors—as being a multi-/inter-disciplinary staff team9 —(Verhagen et al., 1998)] an own customized panel of criteria, using an up to 10-points, PEDro-inspired, scoring for classifying the articles assessed details in Appendix 1 within the Supplementary Material.
The articles were selected only if they had been written in English. We only kept those articles that obtained at least 4 points (“fair”/“high” quality10), considering the following grading criteria (Q1–Q4 as shown in Appendix 1 within the Supplementary Material): published in a journal within the Institute for Scientific Information (ISI) Thomson Reuters11/International Data Bases (IDB), indexed; number of citations per year, pondered (see below) through the year of publication; number of human subjects included in the study (this criterion does not apply for review articles: for such papers there have been considered, following the same calculation formula, the other three criteria); the references' quality (no reference: 0 points; 1–10: 1 point; 11–20: 2 points; 21–30: 3 points; 31–40: 4 points, 41 and over: 5 points).
For each criterion, the maximum number of points possible to be obtained was 5. In order to keep the symmetry with the other criteria regarding the PEDro inspired scoring, we have chosen to classify the articles' quality within the criterion referring to databases, such as: 3 points if the respective article is rated in minimum one IDB and 5 points if it is ISI Thomson Reuters indexed.
As announced above, in order to quantify the citation quality of an article an own customized formula was used, that takes into consideration the number of citations per year, the maximum number of citations per year for all candidate articles, and also the year in which the article was published.
First, the number of citations per year is computed using Equation (1).
where CPY stands for the citations per year, TC is the total number of citations and Y is the year in which the article was published (for article i).
In order to normalize the scores of various articles that were published in different years, a bonus scheme was developed to ensure that the number of citations for newer articles weigh more than for older ones with the same number of citations. By using Equation (2), the absolute value of the reference quality of an article is computed:
where represents the absolute value of the reference quality of article i.
Finally, all absolute values are limited to the interval [0 : 5] by using Equation 3.
where Qi is the final value of the reference quality of the evaluated article.
The article's total score is obtained as the average of each considered criterion calculated points multiplied with 2 (in order to range the maximal score up to 10). After applying this set of selection criteria, a final number of 36 articles remained. We analyzed them for this systematic review.
It is to be mentioned a sui generis situation in which, after the detailed analysis of all articles, one of them, with 6 points PEDro inspired score (Lin et al., 2013a) even if it matched the contextual search syntax “upper limb exoskeleton” within its combination of keywords, the content of the paper referred to an arm support mobile accessory—possibly just adjunct for mechatronic/robotic orthoses/exoskeletons—but without having such technical hallmark features: neither actuators nor sensors, but only mechanical passive balancing gravity components.
The most relevant mobile (wearable and/or portable) mechatronic/robotic orthoses/exoskeletons—to approach neuromotor impairments in the upper limb—prototype devices are detailed in Appendix 2 within the Supplementary Material. The equipment used in the literature we have revised is systematized in the respective table, also containing other such prototype devices met in the supplementary references (marked as italic).
Concerning the score distribution among the analyzed articles (Figure 2), it is to be emphasized its nearly Gaussian pattern (Pearson asymmetry coefficient: 0.63), thus supporting the accuracy of the selection process we have done. This reflects, at the same time, the fact that the articles were ranked as of “fair” to “high” quality, Average: 5.95; Median: 6–similar to other works (Veerbeek et al., 2017)–Dispersion: 1.81; Standard deviation: 1.5; Coefficient of variation: 25.23%. Additionally, Figure 3 displays the ascending trend of the issues per year among the articles we have selected, which is consistent with the related opinions in literature (Lo, 2012; Maciejasz et al., 2014; Proietti et al., 2016; Veerbeek et al., 2017). It is to be noted the “boom” of such articles in 2013.
Taxonomically, we have sorted the selected and qualified articles into five categories as shown in Figure 4.
As for the relevance, based on the number of cases assessed within trials evaluating the interventions done with considered mechatronic/robotic devices in upper limb neuromotor impairments, the situation determined in the analyzed articles is presented in Figures 5, 6. It may be observed that overall, there are no clinical studies with large database of cases, although in recent supplementary related literature, much larger samples can be found (Takahashi et al., 2016).
Specifically, the majority of the studies (18) assessed human-robotic device interactions with healthy individuals (totally 81 subjects) and only 3 articles included patients: 37 with stroke (15 Kim et al., 2013 and 22 Huang et al., 2012), and respectively, 10 with tremor (Rocon et al., 2007).
An important finding regards a quite promising and actual development trend: tracking for feedback and training arm (shoulder, elbow, forearm) and hand, together, produced “greater improvement” than such endeavors done separately for the respective anatomic regions (Merians et al., 2009). The methods to achieve that require the use of, including haptic-based, virtual reality (VR) facilities (Huang et al., 2012; Guo et al., 2013; Lin et al., 2013b; Wei et al., 2013; Dowling et al., 2014; Song et al., 2014; Thielbar et al., 2014; Kim and Rosen, 2015; Shull and Damian, 2015; Grimm et al., 2016; Mazzoleni et al., 2017; Maris et al., 2018). This matches with the conceptual addition of, for instance, Brain Machine Interface (BMI) and/or neuromuscular electrical stimulation (NMES)/FES or transcranial Direct Current Stimulation (tDCS), respectively repetitive transcranial magnetic stimulation (rTMS), facilities usage, too. Furthermore and more recently: some multimodal/hybrid such advanced devices may also provide compensation of gravity load by the related exoskeleton (Yu and Rosen, 2010; Kim et al., 2013; Giberti et al., 2014; Song et al., 2014; Andrikopoulos et al., 2015; Gandolla et al., 2017; Tu et al., 2017a), in order to improve robotic neurorehabiliative/assistive interventions' outcomes, including in the upper limb (Grimm et al., 2016; Tu et al., 2017a; Mazzoleni et al., 2017; Stewart et al., 2017).
At the same time, it proves to be not only actual, but also in line with the ascending trend toward “all-in-one” and/or modular kind of designs (including) for the robotic/mechatronic assistive-rehabilitative orthoses/exoskeletons. This is to be foreseen as neuroscience and technology advance, and respectively, the consumers' expectations for comfort and effectiveness increase, over time, thus supporting our option to focus this systematic synthetic review on mobile advanced systems. This type of apparatus can be divided into two categories: portable and wearable. The latter include devices using fabric integrated within smooth but robustly fit (Polygerinos et al., 2015; Nycz et al., 2015; Rus and Tolley, 2015; Onose et al., 2016). One must consider, at the same time, cosmetics: more complex, but light weight (Rocon et al., 2007; Martinez et al., 2008; Song et al., 2013, 2014; Chen et al., 2014; Giberti et al., 2014; Andrikopoulos et al., 2015; Polygerinos et al., 2015; Guo et al., 2016; Nycz et al., 2016; Alavi et al., 2017) and, at least externally, garment–like structures.
Another quite recent-justified as being a key item that synthesizes, like a top of iceberg, many of the essential mechatronic determinants is the so-called “transparency” (Kim and Rosen, 2015; Proietti et al., 2016). This actually tends to improve the overall outcome, by being underpinned including on increased intuitiveness thus matching with the user's non invasively extracted EMG and/or EEG/BCI movement purposes (Onose et al., 2012). It also provides references and adjustments (and subsequent feed forwards) within a higher level of related abstractness. All these are subsumed to its artificial intelligence/“wisdom” of not acting when not necessary. Such aspect is required for fulfilling overall enhanced (thus being more and more bionic/biomimetic, too) expected performances of robotic exoskeletons, that address also upper limb neuromotor deficits. Therefore, transparency would be, at the same time, a valuable marker for the accuracy of task-oriented achieved results, which could be obtained following human-robotic interactions (Proietti et al., 2016).
More substantiation is needed for the related quest improvement, and it mainly refers to not enough trials on extended groups of patients, complete and clear description of the research methodologies used, statistical power and—based on clinical results—data processing, randomization, (lack of) control lots, “dropout rate” reporting etc. (Lo, 2012).
Because of the mandatory necessity for an (inevitable) multi-/inter-disciplinarity domain's profile, papers can be found in the literature, well documented but based on trials with different or not always best adequate design/statistical power and/or methods used to assess the outcomes obtained. Among these, some present positive or neutral, while others, partially negative findings on robotized physiatric approaches of upper limb neuromotor impairments.
Possible limitations, at least in some circumstances, for (more) valid/“generic” conclusions (Proietti et al., 2016) are represented by the—intrinsic, tightly connected—particularities of various such devices and also the originality/technical advancements/contributions they bring (resulting in differences—objectively—difficult to be rigorously compared). For instance: some authors document no spectacular (if any) benefits—regarding motor function gain and cost-effectiveness—compared to classical, therapists administered, corresponding endeavors (Lo et al., 2010). Others question the effectiveness in improving ADL (divergent, i.e., beneficial Mehrholz et al., 2015) and express conflicting opinions about effects on muscle (hiper)tone states (favorable Sale et al., 2014 vs. negative Veerbeek et al., 2017). The assistance-as-need approach (rather nuanced reticent Norouzi-Gheidari et al., 2012 vs. positive Stewart et al., 2017), required dosage (Norouzi-Gheidari et al., 2012; Pollock et al., 2014; Stewart et al., 2017; Veerbeek et al., 2017) is also debated for pathologic evolution stage (better for chronic Mazzoleni et al., 2017; Veerbeek et al., 2017 vs. subacute Sale et al., 2014).
Generally, one can find discriminating opinions among the efficacy of different control strategies applied and/or between true motor rehabilitation and contextual functional compensations. The latter brings an overall added value to the patient's interaction with the environment, but cannot be clearly/specifically attributed to a certain intervention (Kwakkel et al., 2008; Marchal-Crespo and Reinkensmeyer, 2009; Veerbeek et al., 2017). Even the cost-effectiveness of such interventions remains to be proven: on mid-longer-term (time frame surveyed 36 weeks) the “total costs” of: “robot therapy,” “intensive comparison therapy” and “usual care” are “comparable” (Wagner et al., 2011). However, to balance these opinions, there can also be found in literature a quite converse overall vision on this subject: “Robotic aided therapy has shown to be more effective than traditional physical therapy in providing high intensity of exercise, better movement controllability and measurement reliability, which makes robots ideal instruments…” that “can deliver training at a much higher dosage…” (Frisoli et al., 2016).
Technological progresses are theoretically prone, on one hand, to enhance related therapeutic effectiveness and on the other, to reduce afferent costs. There are interesting divergent assertions in this respect, too: more advanced such equipments—possibly being, conversely, more expensive. However, such advances do not necessarily improve effectively the main outcomes targeted: voluntary controlled motility, muscle power and overall functionality/ADL (Veerbeek et al., 2017). But they can still offer some advantages, such as better adherence to therapy through the psychological investment. This includes patient wishful thinking, provided by novelty and equipment complexity. Also, these devices are also capable of better assistive functions and/or longer—thus more intensive—rehabilitation (Norouzi-Gheidari et al., 2012), based on much more repetitive “motor learning” (Charles et al., 2005) and task/“goal-orientated,” movements (Grimm et al., 2016).
Generally, the main risks, aside from those consequent to human involvement (for the participant and/or generated by the person—skilled or not—who aids the user in the respective man-machine interaction), related to the use of robotic exoskeletons for medical interventions in the upper limb, are: “joint misalignment, skin damage, software malfunction leading to uncontrolled behaviors, electrical and fire hazard” (He et al., 2017). Risks subsequent to possible malfunctions in pneumatic or hydraulic power systems will be emphasized further.
A limitation of our article: the strict/rigorous selection methodology we have applied resulted in rather few overall clinical cases found in the trials afferent to the works we have analyzed.
Generally, for the mechatronic orthotic devices/robotic exoskeletons—including those acting in the upper limb—there are four main applicability fields: “Military, Industry, Medical, First Responders”(Neugebauer, 2017).
In order to improve the human-robot interaction and implicitly its effect, such devices should be more appropriate with the naturalistic/biomimetic kinematics of the upper limb following the main related degrees of freedom (DOF) (Figure 7).
Figure 7. DOF for robot-assisted interventions in the upper limb (Sbenghe, 1987).
The main pathology spectra in the upper limb, approachable by robotic devices evoked in the selected articles, consists of: stroke (the majority–41.66%) (Huang et al., 2012; Song et al., 2012; Kim et al., 2013; Wei et al., 2013; Xiao et al., 2014; Nycz et al., 2015; Kim and Rosen, 2015; Guo et al., 2016; Tu et al., 2017a,b), traumatic brain injury (Giberti et al., 2014), spinal cord injury (Miller and Rosen, 2010; Frisoli et al., 2016), Parkinson's disease/tremor (Rocon et al., 2007; Nimawat and Jailiya, 2015; Shull and Damian, 2015; Freer et al., 2017), peripheral nerve lesions in the upper limb–including with carpal tunnel syndrome–(Noveanu et al., 2013; Giberti et al., 2014; Andrikopoulos et al., 2015; Shull and Damian, 2015; Frisoli et al., 2016). Aside those above mentioned, in the additional literature we have studied, there are to be found also the following indications: Cerebral Palsy, Multiple Sclerosis, Spinal Muscular Atrophy, Brachial Plexus Injury, Arthrogryposis Multiplex Congenita (Rahman et al., 2006; Haumont et al., 2011; Maciejasz et al., 2014; Lopez et al., 2014; Gilliaux et al., 2015).
“Taxonomy of these devices reflects the needs of different types of patients” (Onose et al., 2016). For instance, consider a severe (complete) paralysis of the whole upper limb [after stroke—within hemiplegia—or respectively, after a serious brachial plexus trauma, and additionally: even between different types of neural injuries that generate (morph–) functional deficit(s), there are assorted kinds of (adequate to the diagnosis) interventions]. This would demand the use of a more extended mechatronic, rehabilitative-assistive exoskeleton (as both: territory addressed—over the entire affected upper limb—and interfacing complexity needed/provided by such apparatus) than a distal lesion of the, for instance, ulnar nerve, which need such robotized orthotic interventions, basically, only over the hand region.
More detailed: conceptually analytic rehabilitation aims at (morph)–functional restoring of impairments, based on recovery and/or assistive targets (identified through clinical/para-clinical-recommended: quantitative or at least semi-quantitative-assessments). Most of the specific objectives, addressed by the assistive-rehabilitative endeavors (including) in the upper limb related pathology, basically result out of the upper motor neuron (UMN) and/or lower motor neuron (LMN) syndromes' semiological main items. In brief, these are, for the UMN syndrome: impairment/loss of voluntary movements and/or their coordination–motion force and speed and/or nicety decrease/weakness or abolishment (paresis or paralysis and/or respectively, apraxia/motor planning deficits)–, muscle hypertonia/spasticity, hyperreflexia/clonus (Reed, 2001; Basteris et al., 2014; Bryce, 2016) and for the LMN syndrome: impairment/loss of voluntary movements' control—motion force and speed decrease/weakness or abolishment (paresis or paralysis)—, hypo-/an-aesthesia, muscle hypotonia and atrophy (Purves et al., 2001; Bryce, 2016).
It is noteworthy to emphasize that mobile mechatronic/robotic orthoses/exoskeletons are—as expected with the related and general engineering advancements—also subject for actual and future construct, fabric and consequent performance, augmentations. Accordingly, such devices enable actuated controlled passive movements using VR or haptic capabilities (“wearables … untethered, ungrounded body worn devices that interact with skin directly or through clothing and can be used in natural environments outside a laboratory,” for “sensory replacement”/“augmentation” or training Shull and Damian, 2015). More interesting, for some of them, the haptic and VR facilities are coupled (Song and Guo, 2011; Huang et al., 2012; Song et al., 2013, 2014; Wei et al., 2013; Thielbar et al., 2014; Dowling et al., 2014; Grimm et al., 2016; Guo et al., 2016; Mazzoleni et al., 2017; Maris et al., 2018).
Another important technique is the electromyography (EMG)–feedbacks collected with skin surface electrodes, from the weakened muscles electrical signals. These serve, after filtered/processed into digital inputs, for the command of actuators capable to fulfill/amplify (in real-time adjustable manner) the voluntary—but otherwise impossible or impaired—movements in the “elbow/wrist/hand” (Bouzit et al., 2002; Kiguchi et al., 2008b; Stein, 2009; Frisoli et al., 2016). Additionally, it can be provided stimulation of the patient's own, biological actuators: his/her muscles, in the targeted anatomic region of the upper limb through functional electrical stimulation–FES–(Li et al., 2008) and/or, within the same paradigm, of pro-contractile mechanical input on muscle (tendons): vibratory stimulation (Lam et al., 2008; Shull and Damian, 2015).
The orthoses/exoskeletons “actuating” through FES, although not properly mechatronic–their power contribution to the respective segment's movements is not given through motors–can still be considered and discussed among the type of orthotic devices we focus on, because of their automated, robotic kind of interacting with the targeted area of upper limb with neuromotor impairment (Hu et al., 2015); the same goes for shape memory alloys (SMA–see further).
Regarding mobile devices, innovative systems are proposed in several articles (Yu and Rosen, 2010; Lenzi et al., 2011; Lin et al., 2013b; Chen et al., 2014; Giberti et al., 2014; Tageldeen et al., 2016; Xiao et al., 2017). The respective approaches differ by their mechanisms to generate rotational DOF:
• Electric: using gear rings (Chen et al., 2014) cable transmission systems (Xiao et al., 2017; Nycz et al., 2015); moving cylinders (Rocon et al., 2007; Lenzi et al., 2011; Wei et al., 2013).
• Pneumatic: (Balasubramanian et al., 2008; Yu and Rosen, 2010; Chen et al., 2014; Dowling et al., 2014; Lee, 2014; Andrikopoulos et al., 2015; Nycz et al., 2016; Tu et al., 2017a,b; Xiao et al., 2017) including pneumatic muscle actuators (Caldwell et al., 2007; Andrikopoulos et al., 2015).
• Hydraulic/“hydropneumatic” (Mistry et al., 2005; Noveanu et al., 2013; Lee, 2014; Polygerinos et al., 2015), including “flexible fluidic actuators” (Schill et al., 2011) or those using electro and magneto rheological fluids (ERF-MRF).
• “Shape memory alloys”–SMA– (Rocon et al., 2007; Pittaccio et al., 2013, 2015; Kyrylova, 2015).
Aside the above described mechatronic infrastructure composition of the robotic orthoses/exoskeletons and their consequent actions interfering with the user, an equally important item underpinning the usefulness of such devices is the control loop closing, based on complex acquired inputs (Rocon et al., 2007; Yu and Rosen, 2010; Song and Guo, 2011; Song et al., 2012, 2013, 2014; Chen et al., 2014; Dowling et al., 2014; Andrikopoulos et al., 2015; Nimawat and Jailiya, 2015; Polygerinos et al., 2015; Nycz et al., 2016; Tageldeen et al., 2016):
• Signals for controlling the device (with the related sensoristics): measurement of interaction with the resistance opposed by the user and/or respectively, by the device and/or motion parameters (Giberti et al., 2014; Guo et al., 2016), including with spatial position of different segments of the upper limb/device (Powell and O'Malley, 2011).
• Signals to trigger an action (provided by different types of “switches”): manual commands and/or EMG/electroencephalography (EEG–non invasive brain computer/machine interface– BCI/BMI) and/or the contra-lateral, healthy limb movements and/or intervener forces between the user and the device (Ding et al., 2008; Kiguchi et al., 2008a; Marchal-Crespo and Reinkensmeyer, 2009; Guo et al., 2013; Basteris et al., 2014; Dowling et al., 2014; Maciejasz et al., 2014; Xiao et al., 2014; Frisoli et al., 2016; Tageldeen et al., 2016; Freer et al., 2017; Tu et al., 2017a,b).
• Signals used to quantify the parameters' evolution (mainly Range of Motion–ROM–, motor control, muscle strength and even, possibly, some current items regarding functionality–ADL type) give supplemental capability dimensions to modern more complex devices (Gilliaux et al., 2015; Maris et al., 2018).
• VR capabilities (Lin et al., 2013b) are another type of apparatus/software facility and consequent (mainly as adjunct) therapeutic rehabilitative method, especially higher motivating and credited as a moderate contributive treatment in improving rehabilitative outcomes (Lohse et al., 2014; Thielbar et al., 2014; Palma et al., 2017), some of them using ADL inspired “serious” gaming12 (Huang et al., 2012; Kim et al., 2013; Kim and Rosen, 2015; Frisoli et al., 2016; Tageldeen et al., 2016; Maris et al., 2018).
Newer and very challenging technological developments are expected to be implemented in the field we approached. If this would result in effectively mobile and completely functional, well tolerated, wearable such devices, this could represent a real breakthrough. Specifically, “soft body robots” (Nycz et al., 2015; Polygerinos et al., 2015) are bionic/biomimetic inspired, possibly actuated by “variable length tendons in the form of tension cables or shaped-memory alloy actuators” (as already afore exposed) or based on the expansion properties of elastomer structures, that can be powered either pneumatically or hydraulically (Dowling et al., 2014; Rus and Tolley, 2015; Polygerinos et al., 2015). Briefly resumed here, there are other two very important and useful features of such devices following the general trend of being more and more wearable and effective: anatomical/functional and or technical/structural modularity (Ding et al., 2008; Lo et al., 2010; Schill et al., 2011; Pearce et al., 2012; Noveanu et al., 2013; Lee, 2014; Xiao et al., 2014; Nycz et al., 2016) and complexity of “all-in-one”/hybrid kind (Giberti et al., 2014; Looned et al., 2014; Gandolla et al., 2017; Resquin et al., 2017; Tu et al., 2017a,b).
Other strong candidates for such a revolutionary advancement are: the (still) promising electroactive polymers (Rocon et al., 2007; Onose et al., 2016) and the (also still) near futuristic soft and stretchable electronics (Rus and Tolley, 2015). These may be suitable for incorporation within “sophisticated embodiments” if produced “…in microstructured and nanostructured forms, intimately integrated with elastomeric substrates …” - for instance: polydimethylsiloxane - and eventually maybe even resulting in “tissue-like” devices (Rogers et al., 2010).
Regarding the main categories of related interventions provided, there are asserted as principal elements supporting “motor recovery,” the following: timely approach, adequate dosage, goal-settled exercise and patient's collaborative—if possible—involvement (Frisoli et al., 2016). Furthermore, these can be taxonomically systematized as shown in Figure 8.
Figure 8. Taxonomy of main category interventions (Marchal-Crespo and Reinkensmeyer, 2009).
There doesn't seem to be a consensus in the literature about the consistency—both quantitative and qualitative—of the clinical research studies, deployed to date in the field (especially during the time frame considered, basically, by us, too—as afore specified). For example some authors consider that there are many studies using standardized scales (Marchal-Crespo and Reinkensmeyer, 2009). Others consider their number (including with related results) to be “sparse,” especially of clinical type (Lo, 2012; Maciejasz et al., 2014; Proietti et al., 2016). This partially explains the “slowly” growing recognition of the mechatronic-assisted therapies usefulness. But, however all of them acknowledge as being, numerically, on an ascendant trend.
To be noted that some of the measurement tools frequently used are–as known–comprehensive, being conceived to evaluate in detail, thoroughly the functioning (from the International Classification of Functioning, Disability and Health -ICF-perspective)13,14 aspects they are designated to quantify and hence, are very laborious and–inevitably–chronophagic. Thereby, the use of advanced, multimodal assistive-rehabilitative mechatronic/ robotic orthoses/ exoskeletons, provided including with (automated–so user friendly and investigators' time/ effort saver, and at the same time, standardized/ more prone to non-altered repeatability in “observational” and tracking use) assessment facilities, might represent a noteworthy alternative/ “Complement to Clinical Scales” (Mazzoleni et al., 2017).
In the 36 articles we have selected, there have been met the following assessment scales:
• Fugl–Meyer Assessment–FM(A)15 (APTA, 2011, pages: 51, 55–67, 70–74; Huang et al., 2012; Kim et al., 2013);
• Wolf Motor Function Test-WMFT (Taub et al., 2011; Huang et al., 2012; Takahashi et al., 2016);
• Active Range of Motion–AROM16 (Huang et al., 2012);
• Mini-Mental State Exam–MMSE17 (Kim et al., 2013);
• Peg-in-hole task18 (Miller and Rosen, 2010; Kim et al., 2012a,b; Kim and Rosen, 2015)-is a virtual or physical testing modality, resembling, in principle, to the standardized “9 Hole Peg Test” (APTA, 2011 page: 5).
In the supplementary literature we have studied, there have also been found—aside the above mentioned ones—around twenty assessment scales (at least about half of them very often mentioned/availed in different studies) (details in19).
According to the reasoning illustrated throughout this overview, the tenacious pursuing of basic—neuro-physiological, clinical and technological—but also translational research is warranted and we consider it has potential to reach the afore-mentioned therapeutic/rehabilitative and/or assistive goals. All these aim to improve the needing persons' overall functionality and consequent quality of life, additionally targeting, in the actual and future context, a more efficient skilled human resources use, within a globally improved related cases management approach paradigm. This is, at the same time, consistent with the fact that there are currently no available related spectacular bio-/pharmacological solutions for the central nervous system lesions' repair (Talley Watts et al., 2015).
Also important: as the industry of robotics with medical designations, including with the mobile—portable and/or wearable—orthoses/exoskeletons personally used to rehabilitate and/or assist neuromotor impairments in upper limbs, is progressing, “the regulatory science of powered exoskeletons is still developing.” Its utility regards such devices, too, despite the fact that, compared to the ones designated to be used in the lower limbs, these are safer, including: “free from the dangers associated with falling” (He et al., 2017). Therefore, although this might slow the advancement in the domain, more and more rigor is necessary and–on the long run–beneficial, in terms of safety for the users and emulator. At the same time, focus should also be maintained on a improving human-machine interaction through better technological and translational solutions.
Overall, to date, a certain multi-plane related diversity/“heterogeneity” (Basteris et al., 2014) can still be confirmed. This refers to: devices availed, trials carried out [as designs, participants (biometric and/or pathology data–and moreover, within each, on possible different evolution stages/respectively consequent onset of the robotic intervention(s), and/or severity degree of the neuromotor impairment at baseline), outcomes determined and evaluation methods used to measure them, different components of the administration dose, period(s) surveyed] and—partially—the related point wise and/or general conclusions on the subject matter, derived in various reviews/overviews, encompassing systematic ones, some of them with afferent meta-analyses fulfilled. Yet, this must not be so worrying, but rather motivating for perseverance, with constantly improved—mainly more unitary—research methodology. As long as the trend of growing interest for the domain of robotic assistive-rehabilitative approaches will continue, it will entail inherent—actual and very probable, future, too—controversies over different specific aspects; but more important, we reckon, it will leave the door open for progress as well.
All authors listed have made a substantial, direct and intellectual contribution to the work, and approved it for publication.
The authors declare that the research was conducted in the absence of any commercial or financial relationships that could be construed as a potential conflict of interest.
This work has received the approval (number 2637/26.01.2018) of the Ethics Commission of the Teaching Emergency Hospital Bagdasar–Arseni (TEHBA), in Bucharest, Romania.
The Supplementary Material for this article can be found online at: https://www.frontiersin.org/articles/10.3389/fnins.2018.00577/full#supplementary-material
2. ^https://www.ncbi.nlm.nih.gov
3. ^https://www.ncbi.nlm.nih.gov
5. ^http://search.pedro.org.au/search
6. ^https://apps.webofknowledge.com
7. ^http://prisma-statement.org/documents/PRISMA%202009%20flow%20diagram.pdf
8. ^Data, 2017, from: http://www.pedro.org.au/english/downloads/pedro-scale/
9. ^Data, 2017, from: https://cris.maastrichtuniversity.nl/portal/files/549625/guid-964a90f5-d8aa-4c7f-9d74-b4b70552d067-ASSET1.0
10. ^Data, 2017, from: https://www.strokengine.ca/glossary/pedro-score
12. ^Individualized Technology and Robot-Assisted Virtual Learning Environment (I-TRAVLE) project– https://kenniscentrum.adelante-zorggroep.nl/en/research-programme/projects-2014/individualised-technology-and-robot-assisted-virtual-learning-environment-(i-travle)-www-i-travle-eu/
13. ^Available online at: https://www.cdc.gov/nchs/data/icd/icfoverview_finalforwho10sept.pdf
14. ^Available online at: http://apps.who.int/classifications/icfbrowser/
15. ^http://www.neuropt.org/docs/edge-documents/stroke-edge-compendium-of-instructions2A86360E9D57.pdf?sfvrsn=2
16. ^https://www.dshs.wa.gov/sites/default/files/FSA/forms/pdf/13-585a.pdf
17. ^http://enotes.tripod.com/MMSE.pdf
Alavi, N., Zampierin, S., Komeili, M., Cocuzza, S., Debei, S., and Menon, C. (2017). A preliminary investigation into the design of pressure cushions and their potential applications for forearm robotic orthoses. BioMed. Eng. OnLine 16:54. doi: 10.1186/s12938-017-0345-8
Allotta, B., Conti, R., Governi, L., Meli, E., and Ridolfi, A. (2015). “Development and testing of a low cost wearable and portable hand exoskeleton based on a parallel mechanism,” in ASME. International Design Engineering Technical Conferences and Computers and Information in Engineering Conference, Vol 5A: 39th Mechanisms and Robotics Conference (Boston, MA).
Andrikopoulos, G., Nikolakopoulos, G., and Manesis, S. (2015). “Motion control of a novel robotic wrist exoskeleton via pneumatic muscle actuators,” in 2015 IEEE 20th Conference on Emerging Technologies Factory Automation (ETFA) (Luxembourg), 1–8.
APTA (2011). Compendium of Instructions for Outcome Measures - StrokEDGE Taskforce. New Orleans:APTA Neurology.
Aristotel (2005). About Soul - Translation From Greek and Notes by Baumgarten A. Bucharest: Humanitas.
Balasubramanian, S., Wei, R., Perez, M., Shepard, B., Koeneman, E., Koeneman, J., et al. (2008). “Rupert: an exoskeleton robot for assisting rehabilitation of arm functions,” in 2008 Virtual Rehabilitation (Vancouver, BC), 163–167.
Basteris, A., Nijenhuis, S. M., Stienen, A. H., Buurke, J. H., Prange, G. B., and Amirabdollahian, F. (2014). Training modalities in robot-mediated upper limb rehabilitation in stroke: a framework for classification based on a systematic review. J. Neuroeng. Rehabil. 11:111. doi: 10.1186/1743-0003-11-111
Bouzit, M., Burdea, G., Popescu, G., and Boian, R. (2002). The rutgers master ii-new design force-feedback glove. IEEE/ASME Trans. Mechatr. 7, 256–263. doi: 10.1109/TMECH.2002.1011262
Bryce, T. (2016). “Braddom's Physical Medicine and Rehabilitation,” in Spinal Cord Injury (Philadelphia, PA: Elsevier), 1095–1136.
Caldwell, D. G., Tsagarakis, N. G., Kousidou, S., Costa, N., and Sarakoglu, I. (2007). Soft exosckeletons for upper and lower body rehabilitation - design, control and testing. Int. J. Humanoid Robot. 4, 549–573. doi: 10.1142/S0219843607001151
Charles, S. K., Krebs, H. I., Volpe, B. T., Lynch, D., and Hogan, N. (2005). “Wrist rehabilitation following stroke: initial clinical results,” in 9th International Conference on Rehabilitation Robotics, 2005. ICORR 2005 (Chicago, IL), 13–16.
Chen, Y., Li, G., Zhu, Y., Zhao, J., and Cai, H. (2014). Design of a 6-DOF upper limb rehabilitation exoskeleton with parallel actuated joints. Biomed. Mater. Eng. 24, 2527–2535. doi: 10.3233/BME-141067
Dijkers, M. P., deBear, P. C., Erlandson, R. F., Kristy, K., Geer, D. M., and Nichols, A. (1991). Patient and staff acceptance of robotic technology in occupational therapy: a pilot study. J. Rehabil. Res. Dev. 28, 33–44. doi: 10.1682/JRRD.1991.04.0033
Ding, M., Ueda, J., and Ogasawara, T. (2008). “Pinpointed muscle force control using a power-assisting device: System configuration and experiment,” in 2008 2nd IEEE RAS EMBS International Conference on Biomedical Robotics and Biomechatronics (Scottsdale), 181–186.
Dowling, A. V., Barzilay, O., Lombrozo, Y., and Wolf, A. (2014). An adaptive home-use robotic rehabilitation system for the upper body. IEEE J. Transl. Eng. Health Med. 2:2100310. doi: 10.1109/JTEHM.2014.2314097
Farrugia, P., Petrisor, B. A., Farrokhyar, F., and Bhandari, M. (2010). Practical tips for surgical research: Research questions, hypotheses and objectives. Can. J. Surg. 53, 278–281.
Fineout-Overholt, E., Melnyk, B. M., Stillwell, S. B., and Williamson, K. M. (2010). Evidence-based practice step by step: Critical appraisal of the evidence: part I. Am. J. Nurs. 110, 47–52. doi: 10.1097/01.NAJ.0000383935.22721.9c
Freer, D. R., Liu, J., and Yang, G. Z. (2017). “Optimization of emg movement recognition for use in an upper limb wearable robot,” in 2017 IEEE 14th International Conference on Wearable and Implantable Body Sensor Networks (BSN) (Eindhoven), 202–205.
Frisoli, A., Solazzi, M., Loconsole, C., and Barsotti, M. (2016). New generation emerging technologies for neurorehabilitation and motor assistance. Acta Myol. 35, 141–144.
Gandolla, M., Costa, A., Aquilante, L., Gfoehler, M., Puchinger, M., Braghin, F., and Pedrocchi, A. (2017). “Bridge - behavioural reaching interfaces during daily antigravity activities through upper limb exoskeleton: preliminary results,” in 2017 International Conference on Rehabilitation Robotics (ICORR) (London), 1007–1012.
Giberti, H., Bertoni, V., and Coppola, G. (2014). “Conceptual design and feasibility study of a novel upper-limb exoskeleton,” in 2014 IEEE/ASME 10th International Conference on Mechatronic and Embedded Systems and Applications (MESA) (Ancona), 1–6.
Gilliaux, M., Renders, A., Dispa, D., Holvoet, D., Sapin, J., Dehez, B., et al. (2015). Upper limb robot-assisted therapy in cerebral palsy: a single-blind randomized controlled trial. Neurorehabil. Neural Repair 29, 183–192. doi: 10.1177/1545968314541172
Grimm, F., Walter, A., Spuler, M., Naros, G., Rosenstiel, W., and Gharabaghi, A. (2016). Hybrid neuroprosthesis for the upper limb: combining brain-controlled neuromuscular stimulation with a multi-joint arm exoskeleton. Front. Neurosci. 10:367. doi: 10.3389/fnins.2016.00367
Guo, S., Gao, J., Guo, J., Zhang, W., and Hu, Y. (2016). “Design of the structural optimization for the upper limb rehabilitation robot,” in 2016 IEEE International Conference on Mechatronics and Automation (Beijing), 1185–1190.
Guo, S., Zhang, F., Wei, W., Guo, J., and Ge, W. (2013). “Development of force analysis-based exoskeleton for the upper limb rehabilitation system,” in 2013 ICME International Conference on Complex Medical Engineering (Harbin), 285–289.
Guyton, A., and Hall, J. (2006). Textbook of Medical Physiology. Philadelphia, PA: Elsevier Saunders.
Haumont, T., Rahman, T., Sample, W., M King, M., Church, C., Henley, J., et al. (2011). Wilmington robotic exoskeleton: a novel device to maintain arm improvement in muscular disease. J. Pediatr. Orthop. 31, e44–e49. doi: 10.1097/BPO.0b013e31821f50b5
He, Y., Eguren, D., Luu, T. P., and Contreras-Vidal, J. L. (2017). Risk management and regulations for lower limb medical exoskeletons: a review. Med. Dev. (Auckl) 10, 89–107. doi: 10.2147/MDER.S107134
Hu, X. L., Tong, R. K., Ho, N. S., Xue, J. J., Rong, W., and Li, L. S. (2015). Wrist rehabilitation assisted by an electromyography-driven neuromuscular electrical stimulation robot after stroke. Neurorehabil. Neural Repair. 29, 767–776. doi: 10.1177/1545968314565510
Huang, S., Luo, C., Ye, S., Liu, F., Xie, B., Wang, C., et al. (2012). Motor impairment evaluation for upper limb in stroke patients on the basis of a microsensor. Int. J. Rehabil. Res. 35, 161–169. doi: 10.1097/MRR.0b013e328353053a
Kiguchi, K., Liyanage, M., and Kose, Y. (2008a). “Intelligent perception assist with optimum force vector modification for an upper-limb power-assist exoskeleton,” in 2008 2nd IEEE RAS EMBS International Conference on Biomedical Robotics and Biomechatronics (Scottsdale), 175–180.
Kiguchi, K., Rahman, M. H., Sasaki, M., and Teramoto, K. (2008b). Development of a 3dof mobile exoskeleton robot for human upper-limb motion assist. Robot. Auton. Syst. 56, 678–691. doi: 10.1016/j.robot.2007.11.007
Kim, H., Miller, L. M., Byl, N., Abrams, G. M., and Rosen, J. (2012a). Redundancy resolution of the human arm and an upper limb exoskeleton. IEEE Trans. Biomed. Eng. 59, 1770–1779. doi: 10.1109/TBME.2012.2194489
Kim, H., Miller, L. M., Fedulow, I., Simkins, M., Abrams, G. M., Byl, N., et al. (2013). Kinematic data analysis for post-stroke patients following bilateral versus unilateral rehabilitation with an upper limb wearable robotic system. IEEE Trans. Neural Syst. Rehabil. Eng. 21, 153–164. doi: 10.1109/TNSRE.2012.2207462
Kim, H., Miller, L. M., Li, Z., Roldan, J. R., and Rosen, J. (2012b). “Admittance control of an upper limb exoskeleton - reduction of energy exchange,” in 2012 Annual International Conference of the IEEE Engineering in Medicine and Biology Society (San Diego, CA), 6467–6470.
Kim, H., and Rosen, J. (2015). Predicting redundancy of a 7 dof upper limb exoskeleton toward improved transparency between human and robot. J. Intell. Robotics Syst. 80, 99–119. doi: 10.1007/s10846-015-0212-4
Kwakkel, G., Kollen, B. J., and Krebs, H. I. (2008). Effects of robot-assisted therapy on upper limb recovery after stroke: a systematic review. Neurorehabil. Neural Repair. 22, 111–121. doi: 10.1177/1545968307305457
Kyrylova, A. (2015). Development of a Wearable Mechatronic Elbow Brace for Postoperative Motion Rehabilitation. PhD thesis, The University of Western Ontario - Electronic Thesis and Dissertation Repository.
Lam, P., Hebert, D., Boger, J., Lacheray, H., Gardner, D., Apkarian, J., and Mihailidis, A. (2008). A haptic-robotic platform for upper-limb reaching stroke therapy: preliminary design and evaluation results. J. Neuroeng. Rehabil. 5:15. doi: 10.1186/1743-0003-5-15
Lee, Y. K. (2014). “Design of exoskeleton robotic hand/arm system for upper limbs rehabilitation considering mobility and portability,” in 2014 11th International Conference on Ubiquitous Robots and Ambient Intelligence (URAI) (Kuala Lumpur), 540–544.
Lenzi, T., Vitiello, N., Rossi, S. M. M. D., Persichetti, A., Giovacchini, F., Roccella, S., et al. (2011). Measuring human-robot interaction on wearable robots: a distributed approach. Mechatronics 21, 1123–1131. doi: 10.1016/j.mechatronics.2011.04.003
Li, R., Hu, X. L., and Tong, K. Y. (2008). “Combined electromyography(emg)-driven system with functional electrical stimulation (fes) for poststroke rehabilitation,” in 2008 2nd IEEE RAS EMBS International Conference on Biomedical Robotics and Biomechatronics (Scottsdale), 642–646.
Lin, P.-Y., Shieh, W.-B., and Chen, D.-Z. (2013a). A theoretical study of weight-balanced mechanisms for design of spring assistive mobile arm support (mas). Mechanism Mach. Theory 61(Suppl. C), 156–167. doi: 10.1016/j.mechmachtheory.2012.11.003
Lin, Y.-C., Jog, S., and Chang, J.-Y. J. (2013b). “Pose estimation and simulation of upper limb exoskeleton,” in ASME 2013 Conference on Information Storage and Processing Systems (Santa Clara).
Lo, A. C. (2012). Clinical designs of recent robot rehabilitation trials. Am. J. Phys. Med. Rehabil. 91(11 Suppl. 3):S204–S216. doi: 10.1097/PHM.0b013e31826bcfa3
Lo, A. C., Guarino, P. D., Richards, L. G., Haselkorn, J. K., Wittenberg, G. F., Federman, D. G., et al. (2010). Robot-assisted therapy for long-term upper-limb impairment after stroke. N. Engl. J. Med. 362, 1772–1783. doi: 10.1056/NEJMoa0911341
Lohse, K. R., Hilderman, C. G., Cheung, K. L., Tatla, S., and Van der Loos, H. F. (2014). Virtual reality therapy for adults post-stroke: a systematic review and meta-analysis exploring virtual environments and commercial games in therapy. PLoS ONE 9:e93318. doi: 10.1371/journal.pone.0093318
Looned, R., Webb, J., Xiao, Z. G., and Menon, C. (2014). Assisting drinking with an affordable bci-controlled wearable robot and electrical stimulation: a preliminary investigation. J. NeuroEng. Rehabilit. 11:51. doi: 10.1186/1743-0003-11-51
Lopez, N. M., de Diego, N., Hernandez, R., Perez, E., Ensinck, G., and Valentinuzzi, M. E. (2014). Customized device for pediatric upper limb rehabilitation in obstetric brachial palsy. Am. J. Phys. Med. Rehabil. 93, 263–266. doi: 10.1097/PHM.0b013e3182a51c95
Loureiro, R. C., Harwin, W. S., Nagai, K., and Johnson, M. (2011). Advances in upper limb stroke rehabilitation: a technology push. Med. Biol. Eng. Comput. 49, 1103–1118. doi: 10.1007/s11517-011-0797-0
Lundborg, G. (2014). The Hand and the Brain. From Lucy's Thumb to the Thought-Controlled Robotic Hand. London; Heidelberg; New York, NY; Dordrecht: Springer.
Maciejasz, P., Eschweiler, J., Gerlach-Hahn, K., Jansen-Troy, A., and Leonhardt, S. (2014). A survey on robotic devices for upper limb rehabilitation. J. Neuroeng. Rehabilit. 11:3. doi: 10.1186/1743-0003-11-3
Maher, C. G., Sherrington, C., Herbert, R. D., Moseley, A. M., and Elkins, M. (2003). Reliability of the PEDro scale for rating quality of randomized controlled trials. Phys. Ther. 83, 713–721. doi: 10.1093/ptj/83.8.713
Marchal-Crespo, L., and Reinkensmeyer, D. J. (2009). Review of control strategies for robotic movement training after neurologic injury. J. NeuroEng. Rehabilit. 6:20. doi: 10.1186/1743-0003-6-20
Maris, A., Coninx, K., Seelen, H., Truyens, V., De Weyer, T., Geers, R., et al. (2018). The impact of robot-mediated adaptive I-TRAVLE training on impaired upper limb function in chronic stroke and multiple sclerosis. Disabil. Rehabil. Assist. Technol. 13, 1–9. doi: 10.1080/17483107.2016.1278467
Martinez, F., Retolaza, I., Pujana-Arrese, A., Cenitagoya, A., Basurko, J., and Landaluze, J. (2008). “Design of a five actuated dof upper limb exoskeleton oriented to workplace help,” in 2008 2nd IEEE RAS EMBS International Conference on Biomedical Robotics and Biomechatronics (Scottsdale), 169–174.
Mazzoleni, S., Duret, C., Grosmaire, A. G., and Battini, E. (2017). Combining upper limb robotic rehabilitation with other therapeutic approaches after stroke: current status, rationale, and challenges. Biomed. Res. Int. 2017:8905637. doi: 10.1155/2017/8905637
Mehrholz, J., Pohl, M., Platz, T., Kugler, J., and Elsner, B. (2015). Electromechanical and robot-assisted arm training for improving activities of daily living, arm function, and arm muscle strength after stroke. Cochr. Datab. Syst. Rev. 11:CD006876. doi: 10.1002/14651858.CD006876.pub4
Merians, A. S., Tunik, E., Fluet, G. G., Qiu, Q., and Adamovich, S. V. (2009). Innovative approaches to the rehabilitation of upper extremity hemiparesis using virtual environments. Eur. J. Phys. Rehabil. Med. 45, 123–133.
Methley, A. M., Campbell, S., Chew-Graham, C., McNally, R., and Cheraghi-Sohi, S. (2014). PICO, PICOS and SPIDER: a comparison study of specificity and sensitivity in three search tools for qualitative systematic reviews. BMC Health Serv. Res. 14:579. doi: 10.1186/s12913-014-0579-0
Miller, L. M., and Rosen, J. (2010). “Comparison of multi-sensor admittance control in joint space and task space for a seven degree of freedom upper limb exoskeleton,” in 2010 3rd IEEE RAS EMBS International Conference on Biomedical Robotics and Biomechatronics (Tokyo), 70–75.
Mistry, M., Mohajerian, P., and Schaal, S. (2005). “An exoskeleton robot for human arm movement study,” in 2005 IEEE/RSJ International Conference on Intelligent Robots and Systems (Edmonton, BC), 4071–4076.
Muresanu, D. F., Buzoianu, A., Florian, S. I., and von Wild, T. (2012). Towards a roadmap in brain protection and recovery. J. Cell. Mol. Med. 16, 2861–2871. doi: 10.1111/j.1582-4934.2012.01605.x
Neugebauer, J. (2017). Arl-Wearable Robotics/Exoskeleton/Augmentation Definitions and Taxonomy. Technical Report, Integrated Capability Enhancement Branch Human Research and Engineering Directorate.
Nimawat, D., and Jailiya, P. R. S. (2015). Requirement of wearable robots in current scenario. Eur. J. Adv. Eng. Technol. 2, 19–23. Available online at: https://www.semanticscholar.org/paper/Requirement-of-Wearable-Robots-in-Current-Scenario-Nimawat-Jailiya/92f555844eab04bfb623b56d8e251b13fadfe0c8
Norouzi-Gheidari, N., Archambault, P. S., and Fung, J. (2012). Effects of robot-assisted therapy on stroke rehabilitation in upper limbs: systematic review and meta-analysis of the literature. J. Rehabil. Res. Dev. 49, 479–496. doi: 10.1682/JRRD.2010.10.0210
Noveanu, S., Chetran, B., Tatar, O., Raducanu, G., and Mândru, D. (2013). “Structural synthesis of the upper limb modular wearable exercisers,” in 2013 17th International Conference on System Theory, Control and Computing (ICSTCC) (Sinaia), 693–698.
Nycz, C. J., Bützer, T., Lambercy, O., Arata, J., Fischer, G. S., and Gassert, R. (2016). Design and characterization of a lightweight and fully portable remote actuation system for use with a hand exoskeleton. IEEE Robot. Automat. Lett. 1, 976–983. doi: 10.1109/LRA.2016.2528296
Nycz, C. J., Delph, M. A., and Fischer, G. S. (2015). “Modeling and design of a tendon actuated soft robotic exoskeleton for hemiparetic upper limb rehabilitation,” in 2015 37th Annual International Conference of the IEEE Engineering in Medicine and Biology Society (EMBC) (Milano), 3889–3892.
Onose G, Cârdei V, Crăciunoiu S. T., Avramescu, V, Opriş, I, Lebedev, M. A., et al. (2016). Mechatronic wearable exoskeletons for bionic bipedal standing and walking: a new synthetic approach. Front. Neurosci. 10:343. doi: 10.3389/fnins.2016.00343
Onose, G., Grozea, C., Anghelescu, A., Daia, C., Sinescu, C. J., Ciurea, A. V., et al. (2012). On the feasibility of using motor imagery EEG-based brain-computer interface in chronic tetraplegics for assistive robotic arm control: a clinical test and long-term post-trial follow-up. Spinal Cord 50, 599–608. doi: 10.1038/sc.2012.14
Palma, G. C., Freitas, T. B., Bonuzzi, G. M., Soares, M. A., Leite, P. H., Mazzini, N. A., et al. (2017). Effects of virtual reality for stroke individuals based on the international classification of functioning and health: a systematic review. Top Stroke Rehabil. 24, 269–278. doi: 10.1080/10749357.2016.1250373
Pearce, A. J., Adair, B., Miller, K., Ozanne, E., Said, C., Santamaria, N., et al. (2012). Robotics to enable older adults to remain living at home. J. Aging Res. 2012:10. doi: 10.1155/2012/538169
Pittaccio, S., Garavaglia, L., Ceriotti, C., and Passaretti, F. (2015). Applications of shape memory alloys for neurology and neuromuscular rehabilitation. J. Funct. Biomater. 6, 328–344. doi: 10.3390/jfb6020328
Pittaccio, S., Garavaglia, L., Viscuso, S., Beretta, E., and Strazzer, S. (2013). Implementation, testing and pilot clinical evaluation of superelastic splints that decrease joint stiffness. Ann. Biomed. Eng. 41, 2003–2017. doi: 10.1007/s10439-013-0848-9
Pollock, A., Farmer, S. E., Brady, M. C., Langhorne, P., Mead, G. E., Mehrholz, J., et al. (2014). Interventions for improving upper limb function after stroke. Cochrane Database Syst. Rev. 11:CD010820. doi: 10.1002/14651858.CD010820.pub2
Polygerinos, P., Wang, Z., Galloway, K. C., Wood, R. J., and Walsh, C. J. (2015). Soft robotic glove for combined assistance and at-home rehabilitation. Robot. Auton. Syst. 73, 135–143. doi: 10.1016/j.robot.2014.08.014
Powell, D., and O'Malley, M. K. (2011). “Efficacy of shared-control guidance paradigms for robot-mediated training,” in 2011 IEEE World Haptics Conference (Istanbul), 427–432.
Proietti, T., Crocher, V., Roby-Brami, A., and Jarrasse, N. (2016). Upper-limb robotic exoskeletons for neurorehabilitation: review on control strategies. IEEE Rev. Biomed. Eng. 9, 4–14. doi: 10.1109/RBME.2016.2552201
Purves, D., Augustine, G. J., Fitzpatrick, D., Katz, L. C., LaMantia, A.-S., McNamara, J. O., et al. (2001). Neuroscience, 2nd Edn. Sunderland: Sinauer Associates.
Rahman, T., Sample, W., Jayakumar, S., King, M. M., Wee, J. Y., Seliktar, R., et al. (2006). Passive exoskeletons for assisting limb movement. J. Rehabil. Res. Dev. 43, 583–590. doi: 10.1682/JRRD.2005.04.0070
Resquin, F., Gonzalez-Vargas, J., Ibanez, J., Brunetti, F., Dimbwadyo, I., Carrasco, L., et al. (2017). Adaptive hybrid robotic system for rehabilitation of reaching movement after a brain injury: a usability study. J. Neuroeng. Rehabil. 14:104. doi: 10.1186/s12984-017-0312-4
Riva, J. J., Malik, K. M., Burnie, S. J., Endicott, A. R., and Busse, J. W. (2012). What is your research question? An introduction to the PICOT format for clinicians. J. Can. Chiropr. Assoc. 56, 167–171.
Rocon, E., Belda-Lois, J. M., Ruiz, A. F., Manto, M., Moreno, J. C., and Pons, J. L. (2007). Design and validation of a rehabilitation robotic exoskeleton for tremor assessment and suppression. IEEE Trans. Neural Syst. Rehabilit. Eng. 15, 367–378. doi: 10.1109/TNSRE.2007.903917
Rogers, J. A., Someya, T., and Huang, Y. (2010). Materials and mechanics for stretchable electronics. Science 327, 1603–1607. doi: 10.1126/science.1182383
Rus, D., and Tolley, M. T. (2015). Design, fabrication and control of soft robots. Nature 521, 467–475. doi: 10.1038/nature14543
Sale, P., Franceschini, M., Mazzoleni, S., Palma, E., Agosti, M., and Posteraro, F. (2014). Effects of upper limb robot-assisted therapy on motor recovery in subacute stroke patients. J. Neuroeng. Rehabilit. 11:104. doi: 10.1186/1743-0003-11-104
Sbenghe, T. (1987). Kinetologie Profilactică, Terapeutică şi de Recuperare. Bucharest: Editura Medicală.
Schill, O., Wiegand, R., Schmitz, B., Matthies, R., Eck, U., Pylatiuk, C., et al. (2011). OrthoJacket: an active FES-hybrid orthosis for the paralysed upper extremity. Biomed. Tech. (Berl.) 56, 35–44. doi: 10.1515/bmt.2010.056
Shull, P. B., and Damian, D. D. (2015). Haptic wearables as sensory replacement, sensory augmentation and trainer – a review. J. Neuroeng. Rehabilit. 12:59. doi: 10.1186/s12984-015-0055-z
Song, Z., and Guo, S. (2011). “Development of a real-time upper limb's motion tracking exoskeleton device for active rehabilitation using an inertia sensor,” in 2011 9th World Congress on Intelligent Control and Automation (Taipei), 1206–1211.
Song, Z., Guo, S., Pang, M., Zhang, S., Xiao, N., Gao, B., et al. (2014). Implementation of resistance training using an upper-limb exoskeleton rehabilitation device for elbow joint. J. Med. Biol. Eng. 34, 188–196. doi: 10.5405/jmbe.1337
Song, Z., Guo, S., Xiao, N., Gao, B., and Shi, L. (2012). Implementation of human-machine synchronization control for active rehabilitation using an inertia sensor. Sensors (Basel) 12, 16046–16059. doi: 10.3390/s121216046
Song, Z., Wang, Z., Guo, S., and Gao, B. (2013). “Study on resistance training for upper-limb rehabilitation using an exoskeleton device,” in 2013 IEEE International Conference on Mechatronics and Automation (Taipei), 932–938.
Stein, J. (2009). e100 NeuroRobotic system. Expert Rev. Med. Dev. 6, 15–19. doi: 10.1586/17434440.6.1.15
Stewart, A. M., Pretty, C. G., Adams, M., and Chen, X. (2017). Review of upper limb hybrid exoskeletons. IFAC-PapersOnLine 50, 15169–15178. doi: 10.1016/j.ifacol.2017.08.2266
Tageldeen, M. K., Elamvazuthi, I., and Perumal, N. (2016). “Motion control for a multiple input rehabilitation wearable exoskeleton using fuzzy logic and pid,” in 2016 IEEE 14th International Workshop on Advanced Motion Control (AMC) (Auckland), 473–478.
Takahashi, K., Domen, K., Sakamoto, T., Toshima, M., Otaka, Y., Seto, M., et al. (2016). Efficacy of upper extremity robotic therapy in subacute poststroke hemiplegia: an exploratory randomized trial. Stroke 47, 1385–1388. doi: 10.1161/STROKEAHA.115.012520
Talley Watts, L., Long, J. A., Manga, V. H., Huang, S., Shen, Q., and Duong, T. Q. (2015). Normobaric oxygen worsens outcome after a moderate traumatic brain injury. J. Cereb. Blood Flow Metab. 35, 1137–1144. doi: 10.1038/jcbfm.2015.18
Taub, E., Morris, D., Crago, J., and King, D. (2011). Wolf Motor Function Test (wmft) Manual. Technical report, University of Alabama at Birmingham (UAB - Constraint-Induced Movement Therapy Research Group).
Thielbar, K. O., Lord, T. J., Fischer, H. C., Lazzaro, E. C., Barth, K. C., Stoykov, M. E., et al. (2014). Training finger individuation with a mechatronic-virtual reality system leads to improved fine motor control post-stroke. J. Neuroeng. Rehabil. 11:171. doi: 10.1186/1743-0003-11-171
Tu, X., Han, H., Huang, J., Li, J., Su, C., Jiang, X., et al. (2017a). Upper limb rehabilitation robot powered by PAMs Cooperates with FES Arrays to Realize Reach-to-Grasp Trainings. 2017:1282934. J. Health. Eng. 2017:1282934. doi: 10.1155/2017/1282934
Tu, X., Zhou, X., Li, J., Su, C., Sun, X., Han, H., et al. (2017b). Iterative learning control applied to a hybrid rehabilitation exoskeleton system powered by pam and fes. Cluster Comput. 20, 2855–2868. doi: 10.1007/s10586-017-0880-x
Veerbeek, J. M., Langbroek-Amersfoort, A. C., van Wegen, E. E., Meskers, C. G., and Kwakkel, G. (2017). Effects of robot-assisted therapy for the upper limb after stroke. Neurorehabil. Neural Repair 31, 107–121. doi: 10.1177/1545968316666957
Verhagen, A. P., de Vet, H. C., de Bie, R. A., Kessels, A. G., Boers, M., Bouter, L. M., et al. (1998). The Delphi list: a criteria list for quality assessment of randomized clinical trials for conducting systematic reviews developed by Delphi consensus. J. Clin. Epidemiol. 51, 1235–1241. doi: 10.1016/S0895-4356(98)00131-0
Wagner, T. H., Lo, A. C., Peduzzi, P., Bravata, D. M., Huang, G. D., Krebs, H. I., et al. (2011). An economic analysis of robot-assisted therapy for long-term upper-limb impairment after stroke. Stroke 42, 2630–2632. doi: 10.1161/STROKEAHA.110.606442
Wei, W., Guo, S., Zhang, F., Guo, J., Ji, Y., and Wang, Y. (2013). “A novel upper limb rehabilitation system with hand exoskeleton mechanism,” in 2013 IEEE International Conference on Mechatronics and Automation (Takamatsu), 285–290.
Xiao, F., Gao, Y., Wang, Y., Zhu, Y., and Zhao, J. (2017). Design of a wearable cable-driven upper limb exoskeleton based on epicyclic gear trains structure. Technol. Health Care 25, 3–11. doi: 10.3233/THC-171300
Xiao, Z. G., Elnady, A. M., Webb, J., and Menon, C. (2014). “Towards a brain computer interface driven exoskeleton for upper extremity rehabilitation,” in 5th IEEE RAS/EMBS International Conference on Biomedical Robotics and Biomechatronics (São Paulo), 432–437.
Keywords: upper limb rehabilitation, robotic exoskeletons, mobile robotic orthotic devices, mechatronic wearable orthoses, systematic and synthetic review
Citation: Onose G, Popescu N, Munteanu C, Ciobanu V, Sporea C, Mirea M-D, Daia C, Andone I, Spînu A and Mirea A (2018) Mobile Mechatronic/Robotic Orthotic Devices to Assist–Rehabilitate Neuromotor Impairments in the Upper Limb: A Systematic and Synthetic Review. Front. Neurosci. 12:577. doi: 10.3389/fnins.2018.00577
Received: 22 February 2018; Accepted: 30 July 2018;
Published: 05 September 2018.
Edited by:
Laura Ballerini, Scuola Internazionale Superiore di Studi Avanzati (SISSA), ItalyReviewed by:
Józef Alfons Opara, Jerzy Kukuczka Academy of Physical Education in Katowice, PolandCopyright © 2018 Onose, Popescu, Munteanu, Ciobanu, Sporea, Mirea, Daia, Andone, Spînu and Mirea. This is an open-access article distributed under the terms of the Creative Commons Attribution License (CC BY). The use, distribution or reproduction in other forums is permitted, provided the original author(s) and the copyright owner(s) are credited and that the original publication in this journal is cited, in accordance with accepted academic practice. No use, distribution or reproduction is permitted which does not comply with these terms.
*Correspondence: Gelu Onose, Z2VsdW9ub3NlQGdtYWlsLmNvbQ==
Disclaimer: All claims expressed in this article are solely those of the authors and do not necessarily represent those of their affiliated organizations, or those of the publisher, the editors and the reviewers. Any product that may be evaluated in this article or claim that may be made by its manufacturer is not guaranteed or endorsed by the publisher.
Research integrity at Frontiers
Learn more about the work of our research integrity team to safeguard the quality of each article we publish.