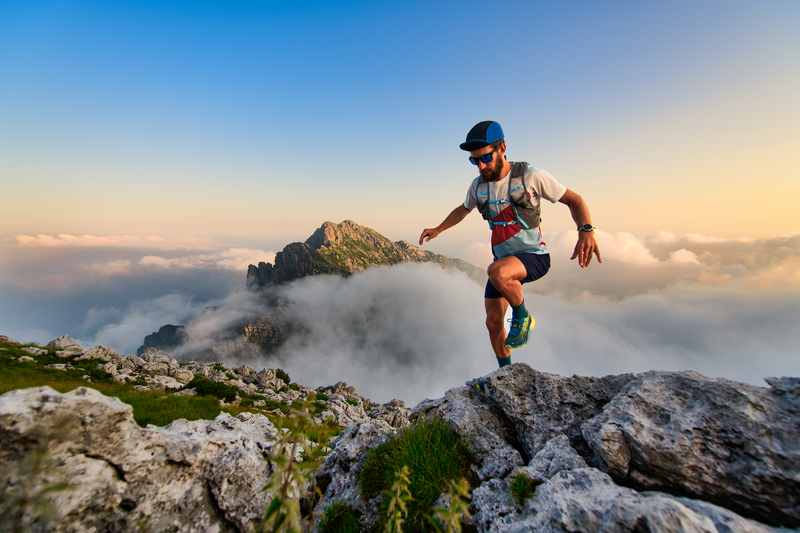
94% of researchers rate our articles as excellent or good
Learn more about the work of our research integrity team to safeguard the quality of each article we publish.
Find out more
MINI REVIEW article
Front. Neurosci. , 26 June 2018
Sec. Neural Technology
Volume 12 - 2018 | https://doi.org/10.3389/fnins.2018.00427
In the recent years numerous studies have provided encouraging results supporting the use of transcranial direct current stimulation (tDCS) as non-invasive brain stimulation technique to improve motor and cognitive functions in patients suffering from neurological and neuropsychiatric disorders as well as in healthy subjects. Among the multiple effects elicited by tDCS on cognitive functions, experimental evidence and clinical findings have highlighted the beneficial impact on long-term memory. Memory deficits occur during physiological aging as well as in neurological and neurodegenerative disorders, including Alzheimer’s disease (AD). In this scenario, non-invasive techniques for memory enhancement, such as tDCS, are receiving increasing attention. The knowledge of molecular mechanisms subtending tDCS effects is of pivotal importance for a more rationale use of this technique in clinical settings. Although we are still far from having a clear picture, recent literature on human and animal studies has pointed to the involvement of synaptic plasticity mechanisms in mediating tDCS effects on long-term memory. Here we review these studies focusing on the neurotrophin “brain-derived neurotrophic factor” (BDNF) as critical tDCS effector.
Transcranial direct current stimulation (tDCS) is a tool effectively modulating motor and cognitive functions in humans. tDCS protocols consist of low amplitude direct currents delivered to human brain via two electrodes placed over the scalp. Depending on electrode positioning (anode or cathode located over the target area), tDCS exerts opposite effects: “anodal” tDCS depolarizes membrane potential and increases excitability in stimulated neurons, whereas “cathodal” tDCS produces neuronal hyperpolarization. Different electrode configurations producing different results are used: (i) unilateral configuration (i.e., one electrode positioned over the target cortical area and the other one over the contralateral supraorbital region or, in some cases, extracephalically); (ii) bilateral configuration (i.e., one electrode positioned over the target cortical area and the other one over the contralateral side); (iii) high-definition tDCS (HD-tDCS), recently developed to improve tDCS spatial focality and based on multiple electrodes (Bikson et al., 2010). In animal models, the unilateral configuration is usually preferred to prevent current bypassing because of the limited space available for two juxtaposed electrodes. This configuration is achieved by positioning the active electrode over the target cortical area and the other one extracephalically, typically over the ventral thorax (Jackson et al., 2016).
Early tDCS studies, mainly focused on motor system, demonstrated that anodal tDCS of the human motor cortex enhanced motor cortex excitability, while cathodal stimulation decreased it (Nitsche and Paulus, 2000; Liebetanz et al., 2002; Lang et al., 2004). tDCS of motor cortex has also been used for the treatment of movement disorders, including dystonia, Parkinson’s disease and stroke (Flöel, 2014). Afterwards, multiple effects of tDCS applied over different brain areas have been described in humans and modulation of brain plasticity has been suggested to account for tDCS after-effects lasting days or weeks (Polanía et al., 2011; Pilato et al., 2012; Kuo et al., 2013; Monte-Silva et al., 2013; Di Pino et al., 2014; Table 1). tDCS applied over the dorsolateral prefrontal cortex (DLPFC) induced enhancement of high-order cognitive processes, such as working memory, attention and perception (Coffman et al., 2014), in healthy subjects as well as in patients suffering from neuropsychiatric disorders, including depression and schizophrenia (Bennabi et al., 2014). Furthermore, growing evidence suggests that tDCS has a beneficial impact on long-term memory, that is supported by synaptic plasticity and adult neurogenesis, mechanisms that are both modulated by electromagnetic stimuli (Di Lazzaro et al., 2013; Coffman et al., 2014; Leone et al., 2014, 2015; Podda et al., 2014). Specifically, clinical studies indicated that tDCS (1–2 mA; 5–30 min) of DLPFC or temporal lobe enhances both episodic and semantic memories in humans and that these effects outlast the stimulation period (Marshall et al., 2004, 2005, 2006; Boggio et al., 2009; Hammer et al., 2011; Clark et al., 2012; Falcone et al., 2012; Flöel et al., 2012; Brunyé et al., 2014; Cotelli et al., 2014; Fertonani et al., 2014; Meinzer et al., 2014; Gray et al., 2015; Chua et al., 2017; Schaal et al., 2017). In older subjects, anodal tDCS improves memory to a level comparable with younger controls (Meinzer et al., 2013) and in patients suffering from Alzheimer’s disease (AD) it enhances visual recognition memory for objects (Boggio et al., 2009).
Similar results were observed in rodents, in which tDCS (100–350 μA; 20 min) applied over PFC, temporal cortex or hippocampus boosts memories (object-recognition, spatial and fear memories) in both physiological (Binder et al., 2014; Podda et al., 2016; Manteghi et al., 2017; Nasehi et al., 2017) and pathological models, including AD (Yu et al., 2015) and traumatic brain injury (TBI) (Yoon et al., 2016).
Given the constant growth of the elderly population worldwide, memory deficits associated to physiological aging and neurodegenerative disorders are rapidly increasing and, unfortunately, pharmacological strategies are poorly effective to either counteract or delay the onset of memory decline. Therefore, non-pharmacological techniques for memory enhancement, including tDCS, are receiving increasing attention. At the same time, there is urgent need to advance our knowledge of molecular mechanisms subtending neurobiological effects of tDCS for a more rational use of this technique, to maximize its benefits and minimize its potential adverse effects. Studies on animal models have been very useful to provide insights into the cellular and molecular mechanisms underlying tDCS effects on memory. Of note, convergent evidence from human and animal studies points to the neurotrophin “brain-derived neurotrophic factor” (BDNF) as critical determinant of tDCS effects.
Within this frame, here we will review recent literature concerning tDCS effects on explicit memory and the underlying molecular mechanisms, focusing on those involving synaptic plasticity and BDNF. Human and animal studies will be discussed in parallel to highlight the potential of translating information obtained from animal models to clinical practice.
The molecular determinants of tDCS-induced enhancement of memory have been recently investigated. However, so far, only few studies, mainly focused on motor cortex, examined cellular and molecular mechanisms enrolled by tDCS in humans. Specifically, a pharmacological study suggested that tDCS-induced changes in motor cortical excitability were mediated by N-methyl-D-aspartate receptors (NMDARs) and membrane depolarization (Liebetanz et al., 2002). Nitsche et al. (2003) demonstrated that sodium and calcium channels were also involved in long-term excitability changes induced by tDCS in the human motor cortex.
Magnetic resonance studies on humans also showed a polarity-dependent modulation of neurotransmitter concentration following tDCS. In particular, in both young and old adults, anodal tDCS of the motor cortex induced a significant decrease in γ-aminobutyric acid (GABA) concentration whereas cathodal stimulation led to a significant decrease in glutamate (Stagg et al., 2009; Antonenko et al., 2017). Clark et al. (2011) demonstrated that anodal tDCS applied over the right parietal cortex led to increased Glx (a combination of glutamate and glutamine) and N-acetylaspartate (NAA) in the stimulated area with respect to unstimulated contralateral hemisphere. In vivo microdialysis performed on rat models showed that dopamine levels were increased in the striatum following cathodal tDCS and these effects were seen for more than 6 h (Tanaka et al., 2013).
On the basis of these findings and considering that tDCS effects on neuronal excitability and behavior outlasted the stimulation period (Bindman et al., 1964; Reis et al., 2009; Flöel et al., 2012), it was hypothesized that long-lasting changes in synaptic strength, i.e., synaptic plasticity, might be involved in mediating tDCS after-effects (Pilato et al., 2012; Cirillo et al., 2017). Experimental evidence supported this hypothesis by showing that anodal DCS applied to mouse motor cortex slices concomitantly with synaptic activation induced NMDAR-dependent LTP, a well-established form of activity-dependent long-term changes in synaptic efficacy (Malenka and Bear, 2004; Fritsch et al., 2010). Additionally, a consistent body of evidence obtained from in vitro and in vivo studies indicates that tDCS exerts modulatory effects on LTP and LTD (Ranieri et al., 2012; Rohan et al., 2015; Podda et al., 2016; Kronberg et al., 2017; Table 1). In particular, it has been demonstrated that DCS applied to rat hippocampal slices prior to synaptic plasticity induction protocol, modulated LTP in a polarity-dependent manner, with anodal DCS enhancing LTP and cathodal DCS decreasing it. Anodal and cathodal DCS also induced the expression of immediate early genes (IEGs), such as c-fos and zif268, in the rat hippocampus (Ranieri et al., 2012). Further in vivo studies showed that tDCS exerted polarity-dependent modulatory action on hippocampal LTP. In particular, anodal tDCS (100–250 μA; 30 min) applied in vivo over the hippocampus of freely moving rats enhanced hippocampal LTP and paired-pulse facilitation. Anodal tDCS effects on hippocampal LTP were intensity-dependent, persisted 24 h after the end of tDCS protocol and were mediated by NMDARs (Rohan et al., 2015).
Parallel studies by Podda et al. (2016) demonstrated that anodal tDCS (350 μA; 20 min) applied over the hippocampus of freely moving mice enhanced hippocampal LTP and this effect was seen up to 1 week after stimulation. Reduced hippocampal LTP was instead observed in slices obtained from mice subjected to cathodal tDCS (Podda et al., 2016). Moreover, it has been shown that DCS applied to rat hippocampal slices concomitantly with synaptic plasticity induction protocol exerts different effects on LTP, likely related to dendritic location. Specifically, in apical dendrites cathodal DCS enhanced hippocampal LTP, while anodal DCS had no significant effects. Conversely, in basal dendrites anodal DCS enhanced LTP while cathodal DCS did not exert significant effects. Both anodal and cathodal DCS reduced hippocampal LTD in apical dendrites, indicating that DCS effects on LTD are not polarity-dependent. The different effects of DCS on LTP have been attributed to modifications in membrane potential occurring in apical and basal dendrites. In particular, cathodal DCS (i.e., cathode closer to CA1 apical dendrites) would hyperpolarize somas and basal dendrites and depolarize apical dendrites, whereas anodal DCS (i.e., anode closer to CA1 apical dendrites) would exert opposite effects (Kronberg et al., 2017). Although the mechanisms underlying the effects of DCS on LTP were not investigated by this study, it can be hypothesized that downstream effectors, including BDNF, are similarly affected in apical and basal dendrites (i.e., where LTP enhancement was observed) receiving depolarizing current.
Further evidence supports the view that tDCS elicits LTP-like mechanisms, showing that a single session of stimulation (250 μA; 20 min) affects hippocampal α-Amino-3-hydroxy-5-methyl-4-isoxazole propionic acid receptors (AMPARs). In particular, tDCS promotes both translocation of GluA1-containing AMPARs from cytosol to synaptic membrane and GluA1 subunit phosphorylation at Ser831 (Stafford et al., 2017; Figure 1).
FIGURE 1. Schematic representation of molecular cascades involved in anodal transcranial direct current stimulation (tDCS)-induced enhancement of synaptic plasticity and memory, in animal models. Experimental evidence suggests that tDCS-induced enhancement of explicit memory is mediated by a molecular cascade including: (i) transient increase in intraneuronal Ca2+; (ii) increased activation of CREB by Ser133 phosphorylation; (iii) CREB binding to brain-derived neurotrophic factor (BDNF) promoter I; (iv) recruitment of CBP; (v) H3K9 acetylation of BDNF promoter I by CBP; (vi) persistent increase in BDNF expression; (vii) activation of TrkB receptor; (viii) inhibition of GSK-3β by Ser9 phosphorylation. The multiple levels of regulation of BDNF expression are numbered (1–5) and listed on the right; those engaged by anodal tDCS are indicated in black. The same regulatory systems might also be engaged by tDCS to enhance the expression of other plasticity-related genes, such as CREB, synapsin I and IEGs. Increased phosphorylation of AMPAR GluA1 subunit at Ser831 in the hippocampus has also been documented following anodal tDCS. Moreover, tDCS was shown to target astrocytes by inducing: (i) noradrenaline (NA) release from noradrenergic fibers or boutons and (ii) NA-induced astrocytic Ca2+ elevation thorough the α1 adrenergic receptor (A1AR). The Ca2+-mediated release of gliotransmitters (glutamate, ATP, D-serine, etc.) from astrocytes might contribute to the enhancement of NMDAR-dependent plasticity induced by tDCS.
Consistent with the notion that LTP-like plasticity mechanisms are involved in the consolidation of memories (Lynch, 2004), it was found that anodal tDCS of the hippocampus improved spatial and recognition memory in mice, as revealed by Morris Water Maze and Novel Object Recognition tasks. Interestingly, both LTP and memory enhancement induced by anodal tDCS persisted 1 week after stimulation (Podda et al., 2016).
Studies discussed so far on both humans and animal models strongly suggest that memory enhancement induced by different tDCS protocols might be mediated by common molecular mechanisms involving NMDAR-dependent synaptic plasticity events. Afterwards, numerous studies have been performed to further investigate the molecular cascade enrolled by tDCS that might explain long-lasting effects on explicit memory.
Literature reports provided convincing evidence on the role of BDNF in synaptic plasticity, learning and memory (Kowiański et al., 2018). In particular, spatial memory training enhanced the expression of both pro-BDNF and BDNF “tyrosine receptor kinase B” (TrkB) receptors in the hippocampus (Silhol et al., 2007). The activity-dependent increase of BDNF is mediated by NMDAR stimulation and subsequent Ca2+ influx. This event leads to activation of the transcription factor “cAMP response-element-binding protein” (CREB), which binds to BDNF promoter, thus triggering BDNF transcription (Zafra et al., 1991; Ghosh et al., 1994; Tao et al., 1998, 2002). It is also well known that BDNF plays a key role in both the early phase and the late phase of LTP (Woo and Lu, 2009).
Given the critical role of BDNF in synaptic plasticity, its involvement in mediating tDCS effects on motor and cognitive functions has been thoroughly investigated. BDNF gene is characterized by several single nucleotide polymorphisms (Liu et al., 2005) and one of these, causing a substitution of valine to methionine at position 66 (Val66Met), has been linked to a reduced responsiveness to tDCS. In particular, Fritsch and collaborators reported that tDCS-induced motor skill enhancement was greater in individuals homozygous for the Val allele than in Met allele carriers (Fritsch et al., 2010). Moreover, in subjects with Val66Met polymorphism cathodal tDCS did not exert any pre-conditioning effect on the response to a subsequent TMS protocol (Cheeran et al., 2008). These effects may be associated to mechanistic differences in the regulation of BDNF expression between Val/Val and Met allele carriers, affecting crucial sites for tDCS action. Indeed, in the hippocampus of BDNFMet/Met mice compared to BDNFV al/V al mice was found: (i) reduced expression of BDNF exon IV and VI transcripts; (ii) increased trimethylation of histone 3 at lysine 27 at BDNF promoters V, VI and VIII; (iii) impaired trafficking of BDNF VI transcript to dendrites; (iv) reduced levels of BDNF protein (Mallei et al., 2015). Furthermore, decreased hippocampal volume and impaired hippocampal-dependent explicit memory have been described in BDNFMet/Met subjects (Chaieb et al., 2014).
These studies, which mainly refer to tDCS effects on motor cortex, provide a rationale for further investigating the involvement of BDNF in tDCS-induced modulation of motor and cognitive functions. This issue has been addressed by using animal models. Specifically, it has been demonstrated that potentiation of post-synaptic responses observed in motor cortex slices following DCS was not elicited in slices obtained from BDNF and TrkB mutant mice (Fritsch et al., 2010). Podda et al. (2016) also demonstrated a causal-link between BDNF and tDCS effects on memory by showing that pharmacological inhibition of TrkB receptors with ANA-12 hindered tDCS effects on memory. Likewise, Kim et al. (2017) showed that repetitive anodal tDCS (250 μA; 20 min; once per day for seven consecutive days) applied over the right sensorimotor cortex of healthy rats enhanced mRNA levels of plasticity-associated genes, including BDNF, CREB, CaMKII, synapsin I, and IEGs, such as c-fos (Figure 1).
The role of BDNF in mediating tDCS effects on memory has also emerged from studies on animal models of disease. Indeed, anodal tDCS of the frontal cortex rescued short-term memory deficits in spontaneous hypertensive rats (SHR) that are the most widely used animal models of attention-deficit/hyperactivity disorder. tDCS also increased dopamine levels in the striatum and hippocampus of SHR rats and, importantly, enhanced BDNF levels in the striatum (Leffa et al., 2016). Moreover, it has been reported that anodal tDCS applied over the motor perilesional cortex of rat models of TBI for 2 weeks post-injury improved spatial memory and increased BDNF levels (Yoon et al., 2016).
As a growing body of evidence implicates BDNF in tDCS effects on synaptic plasticity and memory under both physiological and pathological conditions, the BDNF signaling pathway activated by tDCS has been put into focus.
BDNF expression is highly regulated at several levels. The structure of BDNF, consisting of eight 5′ non-coding exons and a 3′ coding exon (i.e., exon IX) under the control of different promoters, allows a temporal and spatial regulation of BDNF expression by multiple stimuli. Among the different stimuli regulating BDNF expression, neuronal activity has been described as the most potent one (Aid et al., 2007; Chen and Chen, 2017). In addition to the binding of sequence-specific transcription factors to different promoters, transcription of BDNF is modulated by stimulus-dependent changes in chromatin structure, i.e., epigenetic modifications and, in particular, histone acetylation at BDNF promoter I has been shown to affect LTP and long-term memories (Alarcón et al., 2004). Other BDNF regulation systems are proteolytic processing, binding to distinct receptors and activation of different signaling cascades (Benarroch, 2015; Figure 1).
As for the intracellular messengers linking tDCS to the downstream molecular cascades leading to BDNF regulation, involvement of increased Ca2+ levels has been documented. Indeed, tDCS effects have been associated with membrane depolarization-dependent increases in intracellular Ca2+ levels via NMDAR and voltage-gated calcium channel activation (Pelletier and Cicchetti, 2014). Of note, increased Ca2+ levels could initiate molecular pathways leading to enhanced acetylation via CREB/CBP (Kornhauser et al., 2002). Activation of Ca2+ signaling by tDCS has also been reported in cortical astrocytes as a result of increased noradrenaline release that might conceivably contribute to tDCS-induced neural plasticity and epigenetic modifications (Monai and Hirase, 2016; Monai et al., 2016; Figure 1).
Interestingly, our recent study showed that tDCS induced: (i) enhanced phosphorylation of CREB at Ser133; (ii) increased binding of activated CREB to the BDNF promoter I; (iii) the recruitment to BDNF promoter I of the transcriptional coactivator CBP, which acts as a histone acetyltransferase (Podda et al., 2016). tDCS-induced epigenetic modifications at BDNF promoter I consisted in increased acetylation of histone 3 at lysine 9 (H3K9ac) (Podda et al., 2016; Figure 1). This event has been considered the main molecular mechanism whereby anodal tDCS enhanced BDNF exon I and IX mRNA and protein expression up to 1 week after stimulation. As a proof-of principle, we treated mice with the p300/CBP HAT inhibitor, curcumin (Zhu et al., 2014), before subjecting them to tDCS and demonstrated that this treatment occluded tDCS effects on learning and memory, LTP and BDNF expression.
The BDNF downstream signaling pathways activated by tDCS have been also investigated. In particular, glycogen synthase kinase-3β (GSK-3β) is a key substrate of TrkB receptors and is mostly involved in synaptic plasticity (Peineau et al., 2008). Data provided by Podda et al. (2016) demonstrated that tDCS enhanced GSK-3β phosphorylation at Ser9 (pGSK-3βSer9), which is known to promote GSK-3β inhibition resulting in LTP enhancement (Figure 1).
In the last few years, the impact of tDCS on cognitive domains, especially on memory, has been thoroughly studied. In this context, animal models have provided several insights into neuroplasticity changes and molecular events occurring following tDCS that fit well with the results of clinical studies. In particular, it has been demonstrated that BDNF has a crucial role in mediating the beneficial effects of tDCS on explicit memory. Recent findings also showed increased acetylation levels at BDNF promoter I soon after as well as 1 week after tDCS, thus providing a possible molecular mechanism responsible for long-lasting tDCS effects.
Data accrued so far about tDCS effects on memory clearly indicate the need of more in-depth analyses of tDCS action on cognitive functions by combining clinical studies with basic research that, despite the limits of animal models, has the advantage of providing mechanistic insights on effects observed in humans. The results of these studies might pave the way to the identification of promising strategies for personalized medicine to treat cognitive impairment in neuropsychiatric disorders.
Toward this effort, it should be pointed out that, whereas most studies on explicit memory in humans have been performed targeting DLPFC, the majority of data from animal models refers to the hippocampus. Therefore, one possible direction of future studies is to determine the impact of tDCS in tuning hippocampal-prefrontal interactions given the well-established role of this circuit in memory and high-order cognitive functions (Sigurdsson and Duvarci, 2016).
SC, MVP, and CG conceived the review and reviewed the literature. SC wrote the manuscript. MVP and CG revised the manuscript. All authors finalized the manuscript and approved the current version of this paper.
This work was supported by ONR Global (N62909-15-1-2002), Italian Ministry of Health (RF-2013-02356444 and GR-2011-02349998), and D1 Intramural Funds from Università Cattolica del Sacro Cuore.
The authors declare that the research was conducted in the absence of any commercial or financial relationships that could be construed as a potential conflict of interest.
Aid, T., Kazantseva, A., Piirsoo, M., Palm, K., and Timmusk, T. (2007). Mouse and rat BDNF gene structure and expression revisited. J. Neurosci. Res. 85, 525–535. doi: 10.1002/jnr.21139
Alarcón, J. M., Malleret, G., Touzani, K., Vronskaya, S., Ishii, S., Kandel, E. R., et al. (2004). Chromatin acetylation, memory, and LTP are impaired in CBP+/- mice: a model for the cognitive deficit in Rubinstein-Taybi syndrome and its amelioration. Neuron 42, 947–959. doi: 10.1016/j.neuron.2004.05.021
Antonenko, D., Schubert, F., Bohm, F., Ittermann, B., Aydin, S., Hayek, D., et al. (2017). tDCS-induced modulation of GABA levels and resting-state functional connectivity in older adults. J. Neurosci. 37, 4065–4073. doi: 10.1523/JNEUROSCI.0079-17.2017
Benarroch, E. E. (2015). Brain-derived neurotrophic factor: regulation, effects, and potential clinical relevance. Neurology 84, 1693–1704. doi: 10.1212/WNL.0000000000001507
Bennabi, D., Pedron, S., Haffen, E., Monnin, J., Peterschmitt, Y., and Van Waes, V. (2014). Transcranial direct current stimulation for memory enhancement: from clinical research to animal models. Front. Syst. Neurosci. 8:159. doi: 10.3389/fnsys.2014.00159
Bikson, M., Datta, A., Rahman, A., and Scaturro, J. (2010). Electrode montages for tDCS and weak transcranial electrical stimulation: role of “return” electrode’s position and size. Clin. Neurophysiol. 121, 1976–1978. doi: 10.1016/j.clinph.2010.05.020
Binder, S., Berg, K., Gasca, F., Lafon, B., Parra, L. C., Born, J., et al. (2014). Transcranial slow oscillation stimulation during sleep enhances memory consolidation in rats. Brain Stimul. 7, 508–515. doi: 10.1016/j.brs.2014.03.001
Bindman, L. J., Lippold, O. C., and Redfearn, J. W. (1964). The action of brief polarizing currents on the cerebral cortex of the rat (1) during current flow and (2) in the production of long-lasting after-effects. J. Physiol. 172, 369–382.
Boggio, P. S., Khoury, L. P., Martins, D. C., Martins, O. E., de Macedo, E. C., and Fregni, F. (2009). Temporal cortex direct current stimulation enhances performance on a visual recognition memory task in Alzheimer disease. J. Neurol. Neurosurg. Psychiatry 80, 444–447. doi: 10.1136/jnnp.2007.141853
Brunyé, T. T., Holmes, A., Cantelon, J., Eddy, M. D., Gardony, A. L., Mahoney, C. R., et al. (2014). Direct current brain stimulation enhances navigation efficiency in individuals with low spatial sense of direction. Neuroreport 25, 1175–1179. doi: 10.1097/WNR.0000000000000214
Chaieb, L., Antal, A., Ambrus, G. G., and Paulus, W. (2014). Brain-derived neurotrophic factor: its impact upon neuroplasticity and neuroplasticity inducing transcranial brain stimulation protocols. Neurogenetics 15, 1–11. doi: 10.1007/s10048-014-0393-1
Cheeran, B., Talelli, P., Mori, F., Koch, G., Suppa, A., Edwards, M., et al. (2008). A common polymorphism in the brain-derived neurotrophic factor gene (BDNF) modulates human cortical plasticity and the response to rTMS. J. Physiol. 586, 5717–5725. doi: 10.1113/jphysiol.2008.159905
Chen, K. W., and Chen, L. (2017). Epigenetic regulation of BDNF gene during development and diseases. Int. J. Mol. Sci. 18:E571. doi: 10.3390/ijms18030571
Chua, E. F., Ahmed, R., and Garcia, S. M. (2017). Effects of HD-tDCS on memory and metamemory for general knowledge questions that vary by difficulty. Brain Stimul. 10, 231–241. doi: 10.1016/j.brs.2016.10.013
Cirillo, G., Di Pino, G., Capone, F., Ranieri, F., Florio, L., Todisco, V., et al. (2017). Neurobiological after-effects of non-invasive brain stimulation. Brain Stimul. 10, 1–18. doi: 10.1016/j.brs.2016.11.009
Clark, V. P., Coffman, B. A., Mayer, A. R., Weisend, M. P., Lane, T. D., Calhoun, V. D., et al. (2012). TDCS guided using fMRI significantly accelerates learning to identify concealed objects. Neuroimage 59, 117–128. doi: 10.1016/j.neuroimage.2010.11.036
Clark, V. P., Coffman, B. A., Trumbo, M. C., and Gasparovic, C. (2011). Transcranial direct current stimulation (tDCS) produces localized and specific alterations in neurochemistry: a 1H magnetic resonance spectroscopy study. Neurosci. Lett. 500, 67–71. doi: 10.1016/j.neulet.2011.05.244
Coffman, B. A., Clark, V. P., and Parasuraman, R. (2014). Battery powered thought: enhancement of attention, learning, and memory in healthy adults using transcranial direct current stimulation. Neuroimage 85, 895–908. doi: 10.1016/j.neuroimage.2013.07.083
Cotelli, M., Manenti, R., Brambilla, M., Petesi, M., Rosini, S., Ferrari, C., et al. (2014). Anodal tDCS during face-name associations memory training in Alzheimer’s patients. Front. Aging Neurosci. 6:38. doi: 10.3389/fnagi.2014.00038
Di Lazzaro, V., Capone, F., Apollonio, F., Borea, P. A., Cadossi, R., Fassina, L., et al. (2013). A consensus panel review of central nervous system effects of the exposure to low-intensity extremely low-frequency magnetic fields. Brain Stimul. 6, 469–476. doi: 10.1016/j.brs.2013.01.004
Di Pino, G., Pellegrino, G., Assenza, G., Capone, F., Ferreri, F., Formica, D., et al. (2014). Modulation of brain plasticity in stroke: a novel model for neurorehabilitation. Nat. Rev. Neurol. 10, 597–608. doi: 10.1038/nrneurol.2014.162
Falcone, B., Coffman, B. A., Clark, V. P., and Parasuraman, R. (2012). Transcranial direct current stimulation augments perceptual sensitivity and 24-hour retention in a complex threat detection task. PLoS One 7:e34993. doi: 10.1371/journal.pone.0034993
Fertonani, A., Brambilla, M., Cotelli, M., and Miniussi, C. (2014). The timing of cognitive plasticity in physiological aging: a tDCS study of naming. Front. Aging Neurosci. 6:131. doi: 10.3389/fnagi.2014.00131
Flöel, A. (2014). tDCS-enhanced motor and cognitive function in neurological diseases. Neuroimage 85, 934–947. doi: 10.1016/j.neuroimage.2013.05.098
Flöel, A., Suttorp, W., Kohl, O., Kürten, J., Lohmann, H., Breitenstein, C., et al. (2012). Non-invasive brain stimulation improves object-location learning in the elderly. Neurobiol. Aging 33, 1682–1689. doi: 10.1016/j.neurobiolaging.2011.05.007
Fritsch, B., Reis, J., Martinowich, K., Schambra, H. M., Ji, Y., Cohen, L. G., et al. (2010). Direct current stimulation promotes BDNF-dependent synaptic plasticity: potential implications for motor learning. Neuron 66, 198–204. doi: 10.1016/j.neuron.2010.03.035
Ghosh, A., Carnahan, J., and Greenberg, M. E. (1994). Requirement for BDNF in activity dependent survival of cortical neurons. Science 263, 1618–1623.
Gray, S. J., Brookshire, G., Casasanto, D., and Gallo, D. A. (2015). Electrically stimulating prefrontal cortex at retrieval improves recollection accuracy. Cortex 73, 188–194. doi: 10.1016/j.cortex.2015.09.003
Hammer, A., Mohammadi, B., Schmicker, M., Saliger, S., and Münte, T. F. (2011). Errorless and errorful learning modulated by transcranial direct current stimulation. BMC Neurosci. 12:72. doi: 10.1186/1471-2202-12-72
Jackson, M. P., Rahman, A., Lafon, B., Kronberg, G., Ling, D., Parra, L. C., et al. (2016). Animal models of transcranial direct current stimulation: methods and mechanisms. Clin. Neurophysiol. 127, 3425–3454. doi: 10.1016/j.clinph.2016.08.016
Kim, M. S., Koo, H., Han, S. W., Paulus, W., Nitsche, M. A., Kim, Y. H., et al. (2017). Repeated anodal transcranial direct current stimulation induces neural plasticity-associated gene expression in the rat cortex and hippocampus. Restor. Neurol. Neurosci. 35, 137–146. doi: 10.3233/RNN-160689
Kornhauser, J. M., Cowan, C. W., Shaywitz, A. J., Dolmetsch, R. E., Griffith, E. C., Hu, L. S., et al. (2002). CREB transcriptional activity in neurons is regulated by multiple, calcium-specific phosphorylation events. Neuron 34, 221–233.
Kowiański, P., Lietzau, G., Czuba, E., Waśkow, M., Steliga, A., and Moryś, J. (2018). BDNF: a key factor with multipotent impact on brain signaling and synaptic plasticity. Cell Mol. Neurobiol. 38, 579–593. doi: 10.1007/s10571-017-0510-4
Kronberg, G., Bridi, M., Abel, T., Bikson, M., and Parra, L. C. (2017). Direct current stimulation modulates LTP and LTD: activity dependence and dendritic effects. Brain Stimul. 10, 51–58. doi: 10.1016/j.brs.2016.10.001
Kuo, H. I., Bikson, M., Datta, A., Minhas, P., Paulus, W., Kuo, M. F., et al. (2013). Comparing cortical plasticity induced by conventional and high-definition 4 x 1 ring tDCS: a neurophysiological study. Brain Stimul. 6, 644–648. doi: 10.1016/j.brs.2012.09.010
Lang, N., Nitsche, M. A., Paulus, W., Rothwell, J. C., and Lemon, R. N. (2004). Effects of transcranial direct current stimulation over the human motor cortex on corticospinal and transcallosal excitability. Exp. Brain Res. 156, 439–443. doi: 10.1007/s00221-003-1800-2
Leffa, D. T., de Souza, A., Scarabelot, V. L., Medeiros, L. F., de Oliveira, C., Grevet, E. H., et al. (2016). Transcranial direct current stimulation improves short-term memory in an animal model of attention-deficit/hyperactivity disorder. Eur. Neuropsychopharmacol. 26, 368–377. doi: 10.1016/j.euroneuro.2015.11.012
Leone, L., Fusco, S., Mastrodonato, A., Piacentini, R., Barbati, S. A., Zaffina, S., et al. (2014). Epigenetic modulation of adult hippocampal neurogenesis by extremely low-frequency electromagnetic fields. Mol. Neurobiol. 49, 1472–1486. doi: 10.1007/s12035-014-8650-8
Leone, L., Podda, M. V., and Grassi, C. (2015). Impact of electromagnetic fields on stem cells: common mechanisms at the crossroad between adult neurogenesis and osteogenesis. Front. Cell. Neurosci. 9:228. doi: 10.3389/fncel.2015.00228
Liebetanz, D., Nitsche, M. A., Tergau, F., and Paulus, W. (2002). Pharmacological approach to the mechanisms of transcranial DC-stimulation-induced after-effects of human motor cortex excitability. Brain 125, 2238–2247.
Liu, Q. R., Walther, D., Drgon, T., Polesskaya, O., Lesnick, T. G., Strain, K. J., et al. (2005). Human brain derived neurotrophic factor (BDNF) genes, splicing patterns, and assessments of associations with substance abuse and Parkinson’s Disease. Am. J. Med. Genet. B Neuropsychiatr. Genet. 134B, 93–103. doi: 10.1002/ajmg.b.30109
Lynch, M. A. (2004). Long-term potentiation and memory. Physiol. Rev. 84, 87–136. doi: 10.1152/physrev.00014.2003
Malenka, R. C., and Bear, M. F. (2004). LTP and LTD: an embarrassment of riches. Neuron 44, 5–21. doi: 10.1016/j.neuron.2004.09.012
Mallei, A., Baj, G., Ieraci, A., Corna, S., Musazzi, L., Lee, F. S., et al. (2015). Expression and dendritic trafficking of BDNF-6 splice variant are impaired in knock-in mice carrying human BDNF Val66Met polymorphism. Int. J. Neuropsychopharmacol. 18:pyv069. doi: 10.1093/ijnp/pyv069
Manteghi, F., Nasehi, M., and Zarrindast, M. R. (2017). Precondition of right frontal region with anodal tDCS can restore the fear memory impairment induced by ACPA in male mice. EXCLI J. 16, 1–13. doi: 10.17179/excli2016-693
Marshall, L., Helgadóttir, H., Mölle, M., and Born, J. (2006). Boosting slow oscillations during sleep potentiates memory. Nature 444, 610–613. doi: 10.1038/nature05278
Marshall, L., Mölle, M., Hallschmid, M., and Born, J. (2004). Transcranial direct current stimulation during sleep improves declarative memory. J. Neurosci. 24, 9985–9992. doi: 10.1523/JNEUROSCI.2725-04.2004
Marshall, L., Mölle, M., Siebner, H. R., and Born, J. (2005). Bifrontal transcranial direct current stimulation slows reaction time in a working memory task. BMC Neurosci. 6:23. doi: 10.1186/1471-2202-6-23
Meinzer, M., Jähnigen, S., Copland, D. A., Darkow, R., Grittner, U., Avirame, K., et al. (2014). Transcranial direct current stimulation over multiple days improves learning and maintenance of a novel vocabulary. Cortex 50, 137–147. doi: 10.1016/j.cortex.2013.07.013
Meinzer, M., Lindenberg, R., Antonenko, D., Flaisch, T., and Flöel, A. (2013). Anodal transcranial direct current stimulation temporarily reverses age-associated cognitive decline and functional brain activity changes. J. Neurosci. 33, 12470–12478. doi: 10.1523/JNEUROSCI.5743-12.2013
Monai, H., and Hirase, H. (2016). Astrocytic calcium activation in a mouse model of tDCS-extended discussion. Neurogenesis 3:e1240055. doi: 10.1080/23262133.2016.1240055
Monai, H., Ohkura, M., Tanaka, M., Oe, Y., Konno, A., Hirai, H., et al. (2016). Calcium imaging reveals glial involvement in transcranial direct current stimulation-induced plasticity in mouse brain. Nat. Commun. 7:11100. doi: 10.1038/ncomms11100
Monte-Silva, K., Kuo, M. F., Hessenthaler, S., Fresnoza, S., Liebetanz, D., Paulus, W., et al. (2013). Induction of late LTP-like plasticity in the human motor cortex by repeated non-invasive brain stimulation. Brain Stimul. 6, 424–432. doi: 10.1016/j.brs.2012.04.011
Nasehi, M., Khani-Abyaneh, M., Ebrahimi-Ghiri, M., and Zarrindast, M. R. (2017). The effect of left frontal transcranial direct-current stimulation on propranolol-induced fear memory acquisition and consolidation deficits. Behav. Brain Res. 331, 76–83. doi: 10.1016/j.bbr.2017.04.055
Nitsche, M. A., Fricke, K., Henschke, U., Schlitterlau, A., Liebetanz, D., Lang, N., et al. (2003). Pharmacological modulation of cortical excitability shifts induced by transcranial direct current stimulation in humans. J. Physiol. 553, 293–301. doi: 10.1113/jphysiol.2003.049916
Nitsche, M. A., and Paulus, W. (2000). Excitability changes induced in the human motor cortex by weak transcranial direct current stimulation. J. Physiol. 527, 633–639.
Peineau, S., Bradley, C., Taghibiglou, C., Doherty, A., Bortolotto, Z. A., Wang, Y. T., et al. (2008). The role of GSK-3 in synaptic plasticity. Br. J. Pharmacol. 153, S428–S437. doi: 10.1038/bjp.2008.2
Pelletier, S. J., and Cicchetti, F. (2014). Cellular and molecular mechanisms of action of transcranial direct current stimulation: evidence from in vitro and in vivo models. Int. J. Neuropsychopharmacol. 18:pyu047. doi: 10.1093/ijnp/pyu047
Pilato, F., Profice, P., Ranieri, F., Capone, F., Di Iorio, R., Florio, L., et al. (2012). Synaptic plasticity in neurodegenerative diseases evaluated and modulated by in vivo neurophysiological techniques. Mol. Neurobiol. 46, 563–571. doi: 10.1007/s12035-012-8302-9
Podda, M. V., Cocco, S., Mastrodonato, A., Fusco, S., Leone, L., Barbati, S. A., et al. (2016). Anodal transcranial direct current stimulation boosts synaptic plasticity and memory in mice via epigenetic regulation of BDNF expression. Sci. Rep. 6:22180. doi: 10.1038/srep22180
Podda, M. V., Leone, L., Barbati, S. A., Mastrodonato, A., Li Puma, D. D., Piacentini, R., et al. (2014). Extremely low-frequency electromagnetic fields enhance the survival of newborn neurons in the mouse hippocampus. Eur. J. Neurosci. 39, 893–903. doi: 10.1111/ejn.12465
Polanía, R., Paulus, W., Antal, A., and Nitsche, M. A. (2011). Introducing graph theory to track for neuroplastic alterations in the resting human brain: a transcranial direct current stimulation study. Neuroimage 54, 2287–2296. doi: 10.1016/j.neuroimage.2010.09.085
Ranieri, F., Podda, M. V., Riccardi, E., Frisullo, G., Dileone, M., Profice, P., et al. (2012). Modulation of LTP at rat hippocampal CA3-CA1 synapses by direct current stimulation. J. Neurophysiol. 107, 1868–1880. doi: 10.1152/jn.00319.2011
Reis, J., Schambra, H. M., Cohen, L. G., Buch, E. R., Fritsch, B., Zarahn, E., et al. (2009). Noninvasive cortical stimulation enhances motor skill acquisition over multiple days through an effect on consolidation. Proc. Natl. Acad. Sci. U.S.A. 106, 1590–1595. doi: 10.1073/pnas.0805413106
Rohan, J. G., Carhuatanta, K. A., McInturf, S. M., Miklasevich, M. K., and Jankord, R. (2015). Modulating hippocampal plasticity with in vivo brain stimulation. J. Neurosci. 35, 12824–12832. doi: 10.1523/JNEUROSCI.2376-15.2015
Schaal, N. K., Pollok, B., and Banissy, M. J. (2017). Hemispheric differences between left and right supramarginal gyrus for pitch and rhythm memory. Sci. Rep. 7:42456. doi: 10.1038/srep42456
Sigurdsson, T., and Duvarci, S. (2016). Hippocampal-prefrontal interactions in cognition, behavior and psychiatric disease. Front. Syst. Neurosci. 9:190. doi: 10.3389/fnsys.2015.00190
Silhol, M., Arancibia, S., Maurice, T., and Tapia-Arancibia, L. (2007). Spatial memory training modifies the expression of brain-derived neurotrophic factor tyrosine kinase receptors in young and aged rats. Neuroscience 146, 962–973. doi: 10.1016/j.neuroscience.2007.02.013
Stafford, J., Brownlow, M. L., Qualley, A., and Jankord, R. (2017). AMPA receptor translocation and phosphorylation are induced by transcranial direct current stimulation in rats. Neurobiol. Learn. Mem. 150, 36–41. doi: 10.1016/j.nlm.2017.11.002
Stagg, C. J., Best, J. G., Stephenson, M. C., O’Shea, J., Wylezinska, M., Kincses, Z. T., et al. (2009). Polarity-sensitive modulation of cortical neurotransmitters by transcranial stimulation. J. Neurosci. 29, 5202–5206. doi: 10.1523/JNEUROSCI.4432-08.2009
Tanaka, T., Takano, Y., Tanaka, S., Hironaka, N., Kobayashi, K., Hanakawa, T., et al. (2013). Transcranial direct-current stimulation increases extracellular dopamine levels in the rat striatum. Front. Syst. Neurosci. 7:6. doi: 10.3389/fnsys.2013.00006
Tao, X., Finkbeiner, S., Arnold, D. B., Shaywitz, A. J., and Greenberg, M. E. (1998). Ca2+ influx regulates BDNF transcription by a CREB family transcription factor-dependent mechanism. Neuron 20, 709–726.
Tao, X., West, A. E., Chen, W. G., Corfas, G., and Greenberg, M. E. (2002). A calcium responsive transcription factor, CaRF, that regulates neuronal activity dependent expression of BDNF. Neuron 33, 383–395.
Woo, N. H., and Lu, B. (2009). “BDNF in synaptic plasticity and memory,” in Intercellular Communication in the Nervous System, ed. R. Malenka (London: Academic Press), 590–598.
Yoon, K. J., Lee, Y. T., Chae, S. W., Park, C. R., and Kim, D. Y. (2016). Effects of anodal transcranial direct current stimulation (tDCS) on behavioral and spatial memory during the early stage of traumatic brain injury in the rats. J. Neurol. Sci. 362, 314–320. doi: 10.1016/j.jns.2016.02.005
Yu, X., Li, Y., Wen, H., Zhang, Y., and Tian, X. (2015). Intensity-dependent effects of repetitive anodal transcranial direct current stimulation on learning and memory in a rat model of Alzheimer’s disease. Neurobiol. Learn. Mem. 123, 168–178. doi: 10.1016/j.nlm.2015.06.003
Zafra, F., Castren, E., Thoenen, H., and Lindholm, D. (1991). Interplay between glutamate and gamma-aminobutyric acid transmitter systems in the physiological regulation of brain-derived neurotrophic factor and nerve growth factor synthesis in hippocampal neurons. Proc. Natl. Acad. Sci. U.S.A. 88, 10037–10041.
Keywords: tDCS, memory, synaptic plasticity, BDNF, epigenetics, personalized medicine
Citation: Cocco S, Podda MV and Grassi C (2018) Role of BDNF Signaling in Memory Enhancement Induced by Transcranial Direct Current Stimulation. Front. Neurosci. 12:427. doi: 10.3389/fnins.2018.00427
Received: 06 March 2018; Accepted: 06 June 2018;
Published: 26 June 2018.
Edited by:
Ioan Opris, University of Miami, United StatesReviewed by:
Filippo Brighina, Università degli Studi di Palermo, ItalyCopyright © 2018 Cocco, Podda and Grassi. This is an open-access article distributed under the terms of the Creative Commons Attribution License (CC BY). The use, distribution or reproduction in other forums is permitted, provided the original author(s) and the copyright owner are credited and that the original publication in this journal is cited, in accordance with accepted academic practice. No use, distribution or reproduction is permitted which does not comply with these terms.
*Correspondence: Maria V. Podda, bWFyaWF2aXR0b3JpYS5wb2RkYUB1bmljYXR0Lml0
Disclaimer: All claims expressed in this article are solely those of the authors and do not necessarily represent those of their affiliated organizations, or those of the publisher, the editors and the reviewers. Any product that may be evaluated in this article or claim that may be made by its manufacturer is not guaranteed or endorsed by the publisher.
Research integrity at Frontiers
Learn more about the work of our research integrity team to safeguard the quality of each article we publish.