- 1Microbial Ecology and Nutrition Research Unit, Institute of Agrochemistry and Food Technology, National Research Council (IATA-CSIC), Valencia, Spain
- 2IMPACT Strategic Research Centre, School of Medicine, Deakin University, Geelong, VIC, Australia
Obesity continues to be one of the major public health problems due to its high prevalence and co-morbidities. Common co-morbidities not only include cardiometabolic disorders but also mood and cognitive disorders. Obese subjects often show deficits in memory, learning and executive functions compared to normal weight subjects. Epidemiological studies also indicate that obesity is associated with a higher risk of developing depression and anxiety, and vice versa. These associations between pathologies that presumably have different etiologies suggest shared pathological mechanisms. Gut microbiota is a mediating factor between the environmental pressures (e.g., diet, lifestyle) and host physiology, and its alteration could partly explain the cross-link between those pathologies. Westernized dietary patterns are known to be a major cause of the obesity epidemic, which also promotes a dysbiotic drift in the gut microbiota; this, in turn, seems to contribute to obesity-related complications. Experimental studies in animal models and, to a lesser extent, in humans suggest that the obesity-associated microbiota may contribute to the endocrine, neurochemical and inflammatory alterations underlying obesity and its comorbidities. These include dysregulation of the HPA-axis with overproduction of glucocorticoids, alterations in levels of neuroactive metabolites (e.g., neurotransmitters, short-chain fatty acids) and activation of a pro-inflammatory milieu that can cause neuro-inflammation. This review updates current knowledge about the role and mode of action of the gut microbiota in the cross-link between energy metabolism, mood and cognitive function.
Introduction
The microorganisms inhabiting the mammalian's intestinal tract (collectively termed microbiota) include bacteria, viruses, protozoa, archaea, and fungi, with bacteria representing a majority (Gill et al., 2006; Xu et al., 2007). This is an extremely complex ecosystem; in particular, the human adult gut microbiota is estimated to comprise over 1000 different bacterial species with more than 7000 strains (Ley et al., 2006a; Qin et al., 2010). The collective genome of the microbiota (termed microbiome) exceeds the human genome's size and is considered to act as a virtual organ that participates in host physiological functioning (Wang and Kasper, 2014). Gut microbes play a role in human physiology through several mechanisms including their contribution to nutrient and xenobiotic metabolism (e.g., synthesis of vitamins, digestion of oligo, and polysaccharides, drugs, etc.) and to the regulation of immune and neurodendocrine functions (Bäckhed et al., 2005). Some of these effects are mediated by products of bacterial metabolism, such as short-chain fatty acids (SCFA), including propionate, butyrate or acetate, which influence the gut barrier, the inflammatory tone and the metabolic homeostatic control in different tissues (Topping and Clifton, 2001).
A large number of cross-sectional studies report that alterations in the intestinal microbiota (termed dysbiosis) are associated not only with diseases affecting the intestine like inflammatory bowel disease (IBD) but also with extra-intestinal organs and systems. These include, but are not restricted to metabolic diseases [e.g., type-2 diabetes (T2D) and obesity], autoimmune arthritis, and psychiatric disorders (Cenit et al., 2017; Singh et al., 2017). Although it is still unclear whether the general features of a healthy microbiota can be defined at population level, disease seems to be accompanied by shifts in an individual's normal microbiota toward a dysbiotic composition which could aggravate disease pathogenesis, creating a vicious circle, though this is not completely proven. In physiological conditions, by contrast, the gut microbiota co-exist in mutualistic symbiosis with the host, contributing to the body's homeostatic control, through the regulation of immune, endocrine and neural pathways and functions (Romani-Perez et al., 2017). Obesity is a multifactorial condition that depends on intrinsic individual factors as well as on environmental variables. However, the dramatic increase in obesity over the last 40 years is considered to be a consequence of lifestyle changes such as a sedentary lifestyle and high-fat and high-carb/sugar diets. Furthermore, unhealthy dietary habits have been linked to alterations in the intestinal microbiota that could also contribute to the pathophysiology underlying obesity and its metabolic and psychological complications (Agusti et al., 2017; Portune et al., 2017). In this review, we focus on current knowledge of the role of the microbiota in regulating the gut-brain axis and its influence on mood and cognitive impairments linked to obesity. We also analyze the mechanisms through which the microbiota can alter the gut-brain communication, emphasizing effects on the hypothalamic pituitary adrenal (HPA)-axis, immune system and neurotransmission.
The Gut-Brain Axis
The gut-brain axis is a complex bidirectional communication system (see Figure 1), mediated by hormonal, immunological and neural signals, between the gut and the brain (Rhee et al., 2009). This is also a route through which the gut microbiota may impact on neurodevelopmental processes and brain functions. Dysregulation of the gut-brain axis communication is associated with metabolic diseases (Daly et al., 2011; de Lartigue et al., 2011; Grasset et al., 2017) and psychiatric and comorbid non-psychiatric disorders (Maes et al., 2007, 2008; Cryan and O'Mahony, 2011; Grenham et al., 2011; O'Mahony et al., 2011). In turn, these disorders are also frequently associated with alterations in the gut microbiota composition or function, which could also contribute to disruption of the molecular dialogue existing within the gut and brain.
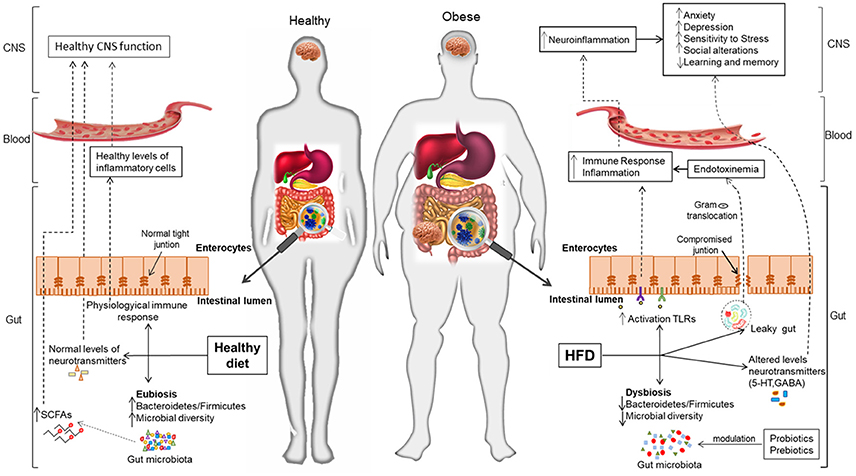
Figure 1. Interplay between the microbiota and the gut-brain axis in obesity and associated mental disorders. Gut microbiota contributes to regulating the gut-brain axis and maintaining health, while its alteration (dysbiosis) due to lifestyle factors (unhealthy diets, stress) is related to obesity and its adverse consequences on mood and cognition. A healthy dietary pattern (e.g., rich in fibers, vegetables, etc.) is thought to increase gut microbiota diversity and, thereby, contribute to epithelial gut integrity, immune homeostasis and normal CNS function through the gut-brain axis. On the contrary, Western-dietary patterns (rich in simple sugars and saturated fat) seem to reduce microbial diversity, promote inflammation and contribute to the leaky gut syndrome; this facilitates the translocation of components of Gram-negative bacteria, which increases the peripheral inflammatory tone and produces neuroinflammation and alterations in the CNS. The use of dietary strategies (e.g., probiotics, healthier diets rich in fiber, prebiotics, etc.) could beneficially impact on obesity and mental complications, via restoration of a healthy microbiota and its regulatory role in the gut-brain axis.
The gut-brain axis is formed by the central nervous system (CNS), the enteric innervation that includes extrinsic fibers of the autonomous nervous system (ANS) and intrinsic neurons of the enteric nervous system (ENS), the HPA-axis and the intestinal microbiota. The extrinsic innervations of the GI tract connect the gut with the brain through vagal and spinal fibers, while the brain sends efferent sympathetic and parasympathetic fibers to the GI tract (Grenham et al., 2011; Browning and Travagli, 2014; Foster et al., 2017). The HPA-axis is part of the limbic system and the main regulator of the stress response. Also, the HPA-axis regulates different body processes including bowel function during digestion. Corticotrophin-releasing factor (CRF) released by the HPA-axis and different members of its family (e.g., CRF, urocortin 1, urocortin 2, and urocortin 3) are known to affect gastrointestinal tract function: bowel motility (Kihara et al., 2001; Czimmer et al., 2006), bowel permeability (Söderholm et al., 2002; Zheng et al., 2013) and bowel inflammation (Dinan et al., 2006; Gill et al., 2006). Other body processes regulated by the HPA-axis are immune functions, emotions and mood (Tsigos and Chrousos, 2002). This is supported by different studies demonstrating that activation of the stress response via the HPA-axis leads to the secretion of glucocorticoids (GCs), which in turn modulate immunity (Baschant and Tuckermann, 2010; Zen et al., 2011), as well as by studies showing that mood disorders are commonly associated with dysregulation of the HPA-axis (Nemeroff et al., 1984; Rubin et al., 1996; Deuschle et al., 1997). Stress is also linked to gastrointestinal diseases like IBD or colitis (Reber, 2012). There are several mechanisms by which gut microbiota may contribute to regulating the communication and function of this axis, including the ability to modulate immune mediators (e.g., cytokines and chemokines) and vagal nerve signaling and to generate or regulate the synthesis of neuroactive metabolites and endocrine secretions (e.g., glucocorticoids, neuropeptides, etc.) or their receptors (Moloney et al., 2014). For example, Bravo et al. (2011) observed that the administration of L. rhamnosus JB-1 altered mRNA GABA receptors in different brain regions in association with attenuation of stress, anxiety and depressive-like behaviors in normal healthy animals. They identified the vagus nerve as a constitutive modulator of the communication pathway between gut microbiota and brain when vagotomized animals could not show neurochemical and behavioral effects. Desbonnet et al. (2008) treated Sprague-Dawley rats for 14 days with a strain of B. infantis. The administration of this bacterium to naive rats significantly attenuated interferon (IFN)-γ, tumor necrosis factor (TNF)-α and interleukin (IL)-6 secretion following mitogen stimulation of whole blood immune cells. They also observed a marked increase in plasma concentrations of tryptophan and kynurenic acid, as well as a reduction in 5-HIAA concentration in the frontal cortex and a decrease in DOPAC in the amygdaloid cortex in the Bifidobacterium-treated rats when compared to controls. These results indicate that gut microbiota modulation could produce changes in immune, neuroendocrine and monoaminergic activity.
The Enteric Innervation
The functionality of the GI tract (gut motility, secretory function, fluid fluxes or blood flow) is regulated by the integrated action of both CNS and the ENS. While the CNS controls gut functions through the extrinsic innervations of the ANS, the ENS may act autonomously and independently of the brain. The extrinsic innervations consist of vagal (first order cell bodies in nodose and jugular ganglia) and spinal (first order bodies in thoracolumbar and lumbosacral dorsal root ganglia) afferents which project centrally to the brainstem or spinal cord for transmitting sensory information from the gut to the CNS (Brierley and Linden, 2014). In return, the CNS sends both sympathetic efferents, which mainly induce inhibitory effects on GI tract and parasympathetic efferents that exert both inhibitory and excitatory actions (Browning and Travagli, 2014). Although the ENS receives extrinsic efferent fiber endings, it is also capable of acting as an independent nervous system. In fact, it is composed by millions of neurons including intrinsic primary afferent neurons (IPANs), which are sensory neurons, interneurons and motor neurons contained in the myenteric and submucosal plexus (Wood et al., 1999). These different neuronal populations work together to regulate several aspects of GI function (Furness et al., 2014).
The enteric innervations are separated from the intestinal luminal content, including the microbiota, by the epithelial cell barrier, the mucous layer and ion and fluid secretions (Saulnier et al., 2013). Nevertheless, study models demonstrated that the gut microbiota communicates with the enteric innervations via several possible routes that may involve intermediate interactions with immune cells and enteroendocrine cells (EECs) (Browning and Travagli, 2014). For example, EECs produce gut hormones (e.g., cholecystokinin (CCK) or glucagon-like peptide 1 (GLP-1) in response to bacterial stimuli, which also modulate enteric innervation activity; in turn, enteric neurons synapse onto EECs allowing mutual feed-back. Therefore, the microbiota could influence the ENS through its direct interaction with EEC functioning. Similarly, the ENS could sense immune signals primarily triggered by gut microbiota-immune interactions or sense the microbial molecular environment and then activate an immune response that could modify the microbiota.
Although we have limited understanding of how the intestinal microbiota influences the gut-brain axis through neural pathways and, therefore, the CNS, different study models have confirmed such interactions. The most direct evidence of the gut microbiota's role in regulating the nervous systems comes from comparing GF animals to conventionally colonized ones. Dupont et al. (1965) demonstrated that the architecture and size of the myenteric plexus were abnormal in GF rats. Anitha et al. (2012) showed that in the myenteric plexus of the colon and in the distal ileum there was a decrease in the number of nitrergic neurons in 4-week-old GF mice. The vast literature comparing GF animal models to colonized models also provides evidence that the microbiota can influence ENS development (Hyland and Cryan, 2016). Furthermore, the gut microbiota is also involved in gut barrier maintenance, since GF animals present a slower turnover of epithelial cells (Abrams et al., 1963), which could also impact on the translocation of molecules to the lamina propria and the blood stream, and the signaling process from the gut to the brain and its function.
In line with these findings, recognition of the intestinal microbiota by Toll-Like Receptors (TLR's) is essential to promote epithelial cell proliferation and regulate innate immunity (Rakoff-Nahoum et al., 2004). TLRs are also expressed by enteric neurons in the GI tract and, therefore, constitute signaling molecules that could mediate the cross-talk between the microbiota and the ENS (Koppel and Balskus, 2016). TLR2 and TLR4 play an important role in enteric innervations and small intestinal function. Cario et al. (2004) showed that redistribution of the tight junction protein zonula occludens-1 (ZO-1) was directly elevated by TLR2 activation, suggesting that TLR2 may enhance epithelial integrity. TLR2 is also involved in regulating GI physiology and enteric neurochemistry, since TLR2 knock-out mice present a reduction in the distal ileal neuron number, glial cells and myenteric ganglion area, as well as structural abnormalities in the submucosal plexus (Brun et al., 2013). Similar alterations were observed in TLR4 knock-out mice (Brun et al., 2013), showing a reduction in in vivo transit coupled with important changes in neurochemistry (Anitha et al., 2012). Recently, TLR2 has been shown to play an important role in the serotonergic system in the small intestine. Latorre et al. (2016) showed that TLR2 activation inhibits the serotonin transporter (SERT), thereby increasing serotonin (5-HT) contents in the small intestine. Dysregulation in the serotonergic system has been related to chronic inflammatory diseases such as intestinal bowel diseases (IBD) (Mawe and Hoffman, 2013) or diarrhea (Spiller, 2008). Besides, TLR2 can be activated by dietary saturated fatty acids (Hwang et al., 2016) and by HFD-induced intestinal dysbiosis, leading to overgrowth of potential pathogens like lipopolysaccharides (LPS)-producing Proteobacteria, as reported in our previous study (Moya-Perez et al., 2015; Agusti et al., 2017).
Central Nervous System (CNS)
The communication between CNS and gut is mediated by secretion of signaling molecules by neurons, immune cells and enterochromaffin cells (ECs), all of which are regulated by the brain and strongly influence the gut microbiota (Carabotti et al., 2015). The CNS, consisting of the brain and spinal cord, is responsible for integrating and coordinating all bodily information. As explained previously, there is a bidirectional transmission of information from the gut to the CNS, and from the CNS to the gut, via afferent and efferent neural, endocrine, and immunological signals between the CNS and the GI system (Romijn et al., 2008). This communication is classically known to regulate energy balance through satiety signals, among other factors (Wang and Kasper, 2014).
Central Regulation of the Energy Balance
The CNS integrates environment and internal signals with information about energy needs and availability to produce a behavioral response including satiation signals, which induce fullness signals to stop eating (Woods and D'Alessio, 2008). Many of these satiation signals are mediated by peptides produced by EECs from the wall of the GI tract and transported from the blood to the brain, although some of these peptides are also produced in the CNS (Wang and Kasper, 2014). Many of the satiety signals are anorexigenic hormones such as peptide YY (PYY), GLP-1, gastric inhibitory neuropepetide (GIP), CCK, oxyntomodulin (OXM), and prouroguanylin (Pimentel et al., 2012). These signals together with those of neuropeptides, such as pro-opiomelanocortin (POMC) and cocaine and amphetamine regulated transcript (CART), are activated during the postprandial period. However, during the fasting period, orexigenic hormones like ghrelin are released mainly in the stomach and excreted to the peripheral circulation (Müller et al., 2015). Also, different neuropeptides like agouti-related protein (AgRP) and neuropeptide Y (NPY) are activated in hypothalamic neurons during fasting to produce a feeling of hunger and a behavioral response: i.e., to start eating (Schwartz et al., 2000; Leibowitz and Wortley, 2004; Valentino et al., 2011). Therefore, changes in ingestive behavior in response to CNS appetite control could influence the nutrient availability for the gut microbiota and subsequently their composition. In turn, modulation of gut microbiota and its activity by dietary intervention can also modify satiety signals. When gut bacteria metabolize prebiotics like oligofructose (OFS) or inulin, they produce SCFAs that can increase the gene expression of GLP-1 (Delzenne et al., 2005) and PYY (Karaki et al., 2006) in the intestinal tract inducing satiety. Of the SCFAs, butyrate acts as the main energy source for colonic cells and strengthens the gut barrier function and exerts anti-inflammatory effects (Andoh et al., 2003). Sodium butyrate administration also exerts an antidepressant effect related to the increased expression of brain-derived-neurotrophic factor (BDNF), which is diminished in mood disorders (Wei et al., 2015). Therefore, SCFAs generated by the microbial metabolic activity in the intestine are demonstrated to exert potential beneficial effects acting via the gut-brain axis in different study models. Bile acids also show a role in the regulation of the metabolic pathway through its binding to specific receptors like Farnesoid X receptor (FXR) involved in cholesterol production, glucose metabolism and bile acid synthesis or TGR5 (G-protein coupled receptor specific for bile acids) involved in energy expenditure in brown adipose tissue, obesity prevention and insulin resistance. Besides, microbiota is involved in the synthesis of bile acids and converts primary bile acid in secondary bile acids. This is evident in GF animals that show low bile acid diversity than its controls (Greiner and Backhed, 2011; Tomkin and Owens, 2016).
Central Regulation of the Peripheral Immune System
The CNS can regulate the transcription of peripheral immune response genes (Dantzer et al., 2008) via HPA-axis or via sympathetic nervous system (SNS) (McEwen, 2007). This mechanism enables the CNS to modulate the activity of internal physiological processes to optimally adapt to external conditions, like a threat in the environment. In this situation the HPA-axis produces GCs, which in the periphery modify metabolic and developmental processes, and suppress the pro-inflammatory and antiviral immune response in the short-term (Besedovsky et al., 1986; Berkenbosch et al., 1987; Sapolsky et al., 1987; Rhen and Cidlowski, 2005). The SNS uses another neural pathway that enables the CNS to modulate the innate immune system through the nerve fibers that release noradrenaline (NA) into the primary and secondary lymphoid organs, involved in haematopoiesis and interactions between antigen-presenting cells and lymphocytes (Nance and Sanders, 2007). The stored adrenaline can be released to the systemic circulation from the adrenal glandule stimulated by the nerve fibers from the SNS, suppressing type I interferon-mediated antiviral response (Collado-Hidalgo et al., 2006) and upregulating the transcription of pro-inflammatory cytokines (Cole et al., 2007).
Neural and neuroendocrine signals like serotonin, dopamine or cytokines can also be secreted into the gut lumen by neurons, immune cells and ECs. For example, an intrathecal injection of TRP (analog of thyrotropin-releasing hormone) into the cerebrospinal fluid (central injection), which regulates the stress response to cold temperatures, produces a stomach lumen secretion of serotonin, possibly mediated by vagal activation of gastric ECs (Stephens and Tache, 1989; Yang et al., 1992). Another example is the secretion of tryptase and histamine (Mast-cell products) into the human jejunum in response to stress produced by cold pain. Other mast-cell products could be secreted into the gut lumen, including serotonin and corticotrophin-release hormone (CRH) (Santos et al., 1998).
Immune Modulation of the CNS
Some studies that have associated gut infections with CNS alterations. For example, chronic infection with Helicobacter pylori produces anxiety-like behaviors in mice, induces structural and functional changes in the ENS, as well as changes in feeding patterns increasing the frequency and decreasing the quantity of food consumed. The latter are associated with a decrease in POMC expression in arcuate nucleus and increases of TNF-α in median eminence (Bercik and Collins, 2014). Campylobacter jejuni infection also produces anxiety-like behaviors without increasing inflammatory markers. In the latter case, the gut-brain communication might be mediated through activation of vagal ascending pathways (Goehler et al., 2008). Chronic infection with Trichuris muris also induces anxiety-like behavior in mice, decreased BDNF expression in the hippocampus, accompanied by a mild increase of TNF-α and IFN-γ as well as kynurenine in plasma. Administration of Bifidobacterium longum restored the behavioral alterations and BDNF but not the cytokines and kynurenine, proving that intervention in the gut modifies behavior (Bercik et al., 2010).
Gut pathogens, such as enterohaemorrhagic E. coli O157:H7, have been shown to have binding sites for enteric neurotransmitters, NA and adrenaline (ADE), on their cell surface, whose binding activates their pathogenic features and the use of adrenergic antagonists blocked this activation (Hughes and Sperandio, 2008). This suggests that the communication between enteric neurotransmitters (produced by the host) and the gut microbiota could be involved in gut infections and the inflammation associated to this process.
Impact of Gut Microbiota on CNS: Role of Neurotransmitters
Brain is the main modulator of gut homeostasis, controlling the motility, secretion of acid, bicarbonates and mucus (Carabotti et al., 2015). Studies have investigated how microbiota impacts the CNS, both, physiologically and pathologically (see Figure 1). The impact of gut microbiota on the CNS and behavior has been demonstrated in intervention studies with probiotics or antibiotics in rodents and, in a few cases, in humans. For example, some probiotic strains belonging to the genera Bifidobacterium and Lactobacillus have been reported to improve mood and reduce anxiety symptoms in patients with IBD and chronic fatigue syndrome (Logan and Katzman, 2005; Shadnoush et al., 2013). Also, intestinal dysbiosis has been linked to intestinal and systemic inflammatory tone, which is a mechanism contributing to mood disorders such as depression (Dowlati et al., 2010) in inflammatory conditions such as Irritable Bowel Syndrome (IBS) (Dai et al., 2013) and presumably in obesity. The mechanisms by which probiotics could mediate these effects include their possible immune regulatory properties explained above and their ability to modify neurotransmission. 5-HT is a monoamine neurotransmitter produced from tryptophan, ingested with the diet. This neurotransmitter is well known for its role in cognition and mood in the brain. However, 95% of 5-HT is produced in the gut, specifically by ECs of the mucosa and in the nerve terminals of ENS neurons. The classical functions assigned to 5-HT in the GI tract are related to its participation in GI motility, secretion and pain perception. 5-HT also has neuroprotective, trophic factor actions and pro-inflammatory actions in the gut (Mawe and Hoffman, 2013; Moloney et al., 2014). The regulation of mood and cognition depends on the availability of tryptophan on the CNS, which in turn depends on the availability of peripheral tryptophan, which is altered in GF mice. Clarke et al. (2013) demonstrated that the absence of gut microbiota in GF mice in early-life increased plasma tryptophan, suggesting a possible humoral pathway by which the microbiota could influence CNS serotonergic neurotransmission. Also, they observed an increase in 5-HT and its main metabolite, hydroxyindoleacetic acid, in hippocampus compared to normal animals. Furthermore, the colonization of the GF animals post weaning was not sufficient to reverse the CNS neurochemical consequences in adulthood due to a lack of microbiota in early life, even though the baseline values of tryptophan availability were restored. Nishino et al. (2013) also showed that GF mice exposed during 24 h to the SPF environment developed a normal (SPF) microbiota and that was accompanied by a decrease in the anxious-like behavior and an increase in ADE,DA and 5-HT brain levels. Other authors showed that GF animals present a significant reduction of 5-HT in serum compared to normal mice (specific pathogen-free, SPF) (Yano et al., 2015). Yano et al. (2015) also reported that indigenous spore-forming bacteria (Sp) from the mouse and human microbiota promote 5-HT biosynthesis from colonic ECs. These authors also showed that increased luminal concentration of specific microbial metabolites elevated colonic and blood 5-HT in GF mice. This study showed that host-microbiota interactions are very important in regulating essential 5-HT-related biological processes. Agusti et al. (2017) showed that 5-HT is significantly decreased in hippocampus of HFD-fed mice and this correlated with anhedonic-depressive-like behavior. The administration of B. pseudocatenulatum CECT 7765 attenuated the obesity associated depressive-like behavior and significantly increased the 5-HT concentration in hippocampus by modifying the gut microbiota. The use of probiotics in animal models of depression has shown some efficacy. For example, a strain of Bifidobacterium infantis restored the forced swimming test (FST) and decreased pro-inflammatory cytokines regulating tryptophan metabolism and the neurotransmitters in the CNS in a rat model of depression induced by maternal separation (Desbonnet et al., 2008, 2010).
GABA can also be affected by the gut microbiota. Bravo et al. (2011), as we explain briefly in section 2, observed that the administration of L. rhamnosus JB-1 altered mRNA GABA receptors in different brain regions of mice. GABA b1b receptor expression was increased in cortical cingulate and prelimbic regions, whereas it decreased in the hippocampus, amygdala, and locus coeruleus. In the prefrontal cortex (PFCx) and amygdala there was a decrease in GABA Aα2 mRNA, but an increase in the hippocampus. This was accompanied by attenuation of stress, anxiety and depressive-like behaviors in healthy animals. Results of the same study confirmed that communication between the microbiota and the brain occurred partly via the vagus nerve, which provided information from the lumen to the CNS. This was evident when vagotomized animals failed to show neurochemical and behavioral effects. Thus, the vagus nerve was identified as a constitutive modulator of the communication pathway between the gut microbiota and the brain. Other authors have also shown that components of the microbiota are able to produce molecules that act as local neurotransmitters in the ENS, for example GABA, 5-HT, acetylcholine, melatonin or histamine (Iyer et al., 2004; Portune et al., 2016).
The HPA-Axis
Gut microbiota is thought to play a role in mechanisms governing the stress response via the HPA-axis, whose deregulation has also been related to obesity. This connection was first evidence in Cushing syndrome patients who present high levels of cortisol, glucose intolerance, hypertension and upper body obesity (Bjorntorp and Rosmond, 2000; Nieuwenhuizen and Rutters, 2008).
The interconnection between the gut microbiota and the stress response via the HPA-axis was initially evidenced in GF and intentionally colonized rodents. In Sudo et al. (2004) showed that the response to stress via the HPA-axis was exacerbated in GF animals compared with SPF animals and it was accompanied by an increase in BDNF expression in cortex and hippocampus. The exaggerated response to stress was reversed by gut colonization with a strain of B. infantis and partially reversed with SPF stools at an early stage, but not later in life. This finding indicates that exposure to microbes at an early developmental stage is required for the HPA system to become completely susceptible to inhibitory neural regulation. O'Mahony et al. (2009) showed that early life stress induced by maternal separation also produces changes in fecal microbiota, supporting the idea that stress in early stages alters gut microbiota. On the other hand, modification of gut microbiota by a probiotic could also modulate the HPA-stress response. For example, Ait-Belgnaoui et al. (2012) demonstrate that the oral administration of L. Farciminis suppressed stress-induced hyperpermeability, endotoxemia and prevented HPA axis stress response and neuroinflammation.
As we indicate above, stress is an important factor in obesity and eating behavior which could also be interlinked with the microbiota. Numerous studies confirm that the HPA-axis plays an important role in the onset of metabolic alterations and obesity (Desbriere et al., 2006; Abraham et al., 2013; Champaneri et al., 2013). There is a positive association between stress, weight gain, adiposity and body mass index (BMI) (Torres and Nowson, 2007; Block et al., 2009), as well as with basal glucose, basal insulin and resistance to insulin (Sinha and Jastreboff, 2013). Furthermore, the connection between stress and metabolic dysfunction is stronger in individuals with higher BMI than in those with lower BMI (Sinha, 2008), suggesting that stress increases obesity risk especially in individuals with higher BMI. Some studies have shown that adrenal steroids increase glucose and insulin levels as well as high-caloric food intake (Sinha and Jastreboff, 2013). Chronic high levels of GCs and insulin boosted the increase in palatable food intake and abdominal fat deposition (Dallman et al., 2005; Warne, 2009). Therefore, stress could trigger metabolic dysfunction and modify eating behavior; besides, obese individuals are more sensitive to stress.
The role of the microbiota in the connection between stress and obesity has been demonstrated by, for example, the administration of potential probiotic bacteria to animal models of obesity. Thus, Agusti et al. (2017) demonstrated that basal corticosterone levels were significantly increased in mice with HFD-induced obesity. Obese animals also showed increased corticosterone levels in response to acute social stress, suggesting that obese mice were more susceptible to these stressful situations. Administration of B. pseudocatenulatum CECT 7765 reduced corticosterone levels in HDF-fed mice, indicating that an intervention primarily targeting the gut was able to reverse the anxiogenic obese profile.
Immune Mediators in the Gut-Brain Axis
The immune system plays an important role in the gut-brain axis, considering that the GI tract contains the highest concentration of immune cells in the body. In GF animal models, immune defects have been observed at both levels: cellular and structural. One the one hand, Round and Mazmanian (2009) showed that there was a reduction in B-cell production of secretory IgA (sIgA) and a decrease in the intestinal T helper 17 (Th 17), CD4+ and CD8+ immune cells. They also found defects at the structural level with a decrease in Peyer's patches, lamina propria and isolated lymphoid follicles. On the other hand, the re-colonization of GF mice with a main commensal component of the gut microbiota, Bacteroides fragilis, proved efficient in restoring the immune maturation in gut associated lymphoid tissues.
The immune system also plays an important role in obesity (see Figure 1). The chronic nature of obesity produces prolonged low-grade activation (Gregor and Hotamisligil, 2011; Lumeng and Saltiel, 2011). Both the increase of the permeability of the gut barrier and the usual fat intake, that increases LPS absorption, contribute to this endotoxaemia (Delzenne et al., 2011; Torres-Fuentes et al., 2017). However, this endotoxaemia could be prevented by some gut bacteria which preserve the integrity of the gut barrier (Rooks and Garrett, 2016). Obesity is also associated with neuro-inflammation as described in other pathologies, including Alzheimer disease (AD) or depression (Whitmer et al., 2007; Mayeux and Stern, 2012). Obese patients are more prone to develop these types of pathologies and neuro-inflammation may underpin these associations (Guillemot-Legris and Muccioli, 2017).
Peripheral inflammation associated with obesity may lead to neuro-inflammation and could be due to the activation of components of innate immunity like Toll-like receptors (TLRs) and increased intestinal permeability (known as a leaky gut). Leaky gut is a loss of intestinal barrier integrity making it less able to protect the internal environment. This loss of integrity allows bacteria, toxins and other molecules to arrive to the bloodstream. In recent studies it has been demonstrated that gut compositional changes and inflammation related with leaky gut may contribute to the pathophysiology of several diseases like depression, chronic fatigue syndrome, obesity, Type-2 diabetes or IBS (Slyepchenko et al., 2017). Dietary saturated fatty acids (SFAs) can induce inflammatory responses in different organs like liver, pancreas, adipose tissue and muscle (Guillemot-Legris and Muccioli, 2017). SFAs are able to activate the TLRs expressed in intestinal epithelial and innate immune cells, specifically TLR2 and TLR4 (Hwang et al., 2016). TLR2 activation can mediate signaling cascades through myeloid differentiation factor-88 (MyD-88) and NF-κB, (Kim et al., 2013; Hayward and Lee, 2014) and TLR4 activation can activate NF-κB as well as activator protein 1 (AP-1), triggering cerebral inflammation by the upregulation of proinflammatory cytokines (Guillemot-Legris and Muccioli, 2017). The dysbiotic microbiota associated with obesity can also contribute to increasing the inflammatory tone via activation of TLRs and the subsequent production of inflammatory cytokines (Sanz and Moya-Perez, 2014).
In addition, several environmental factors such as diets rich in fats and poor in fibers, alcohol, stress, and intestinal dysbiosis observed in obese patients (Ley et al., 2006a; Riva et al., 2017) and animal models (Lin et al., 2014; Schneeberger et al., 2015) can alter the gut epithelial barrier, possibly as a secondary consequence of the inflammatory process (de Melo et al., 2017). Disruption of the intestinal barrier facilitates the translocation of components of mainly Gram-negative bacteria (e.g., LPS) from the lumen to the mesenteric lymph nodes (MNLs) and peripheral circulation (de Kort et al., 2011; Leonard and Maes, 2012). There, bacterial motifs activate the immune system through TLR2 and TLR4-biding, boosting the release of pro-inflammatory pathways (NF-κB and Mitogen-Activated Protein Kinases [MAPK]) and cytokines (e.g., TNF-α) (Chan and Riches, 2001; Wischmeyer, 2006) and increasing IgA and IgM responses to Gram-negative bacteria (Maes et al., 2007, 2008). Proinflammatory cytokines can also contribute to tight junction disruption leading to bacterial translocation (Slyepchenko et al., 2017). In addition, hypertrophy of adipose tissue increases pro-inflammatory signaling, creating a vicious circle. Similar processes may also disrupt the BBB allowing leukocyte infiltration into CNS, contributing to the development of mood disorders (de Melo et al., 2017) and producing neuroinflammation. The translocation of Gram-negative bacteria from the gut induce oxidative and nitrosative stress (O&N stress) processes that produce redox-derived DAMPs (damage-associated molecular patterns), which may activate TLR-2 and 4, leading to a vicious cycle known as the TLR2/4 radical cycle (Lucas and Maes, 2013). Both, endotoxemia (increased serum LPS), consequence of leaky gut, and adipose tissue-related inflammation could lead to insulin resistance and hyperglycemia (Fandriks, 2017). Peripheral insulin resistance may be essential to initiate a sequence of pathophysiological events in obesity. Leaky gut and consequent translocation of Gram-negative bacteria are also detected in MDD. This is reflected in increased IgA and IgM levels against Gram-negative bacteria, including Hafnia alvei, Pseudomonas aeruginosa, Morganella morganii, Pseudomonas putida, Citrobacter koseri, and Klebsiella pneumoniae (Maes et al., 2007, 2008). Furthermore, in MDD, these IgA/IgM levels directed to commensal bacteria are related with immune activation and O&NS (Maes et al., 2013).
Effects of Obesity on Cognitive Functions and Mood
Obesity may contribute to cognitive damage (Johnson et al., 2016) and behavioral alterations (see Figure 2), which may partly be due to obesity-associated neuro-inflammatory processes (Guillemot-Legris and Muccioli, 2017). Obesity in childhood and adolescence could have a particularly relevant impact since these are critical periods for neurodevelopment and neuronal plasticity (Spear, 2000; Boitard et al., 2012), where negative experiences can alter brain functions, behaviors and mood states in adulthood (García-Pardo et al., 2015).
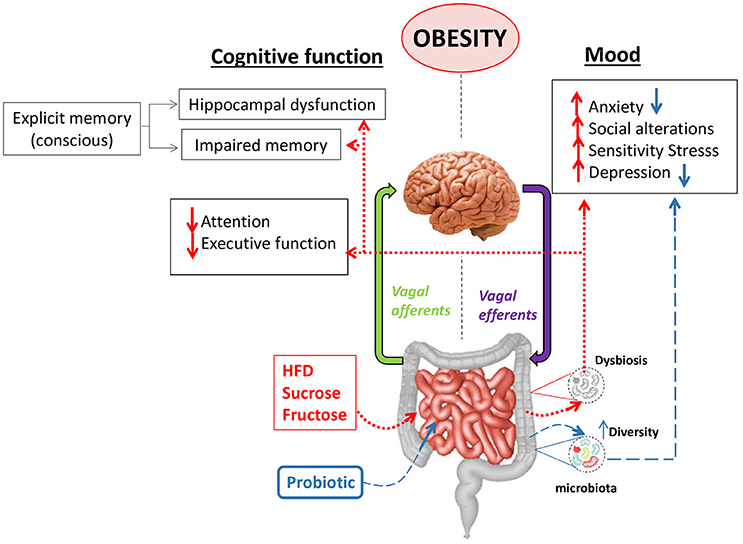
Figure 2. Mood and cognitive alterations in obesity: the role of the gut-brain axis. The diversity and stability of the gut microbiota can be affected by high-fat diets (HFD) or high carb diets leading to dysbiosis, which is a typical alteration observed in obesity. A dysbiotic microbiota is thought to alter the communication between the gut and the brain axis contributing to mood alterations like anxiety, depression, sensitivity to stress, social behavioral alterations and cognitive alterations like hippocampal dysfunction, impaired memory and reduction of attention or the executive function. The use of some probiotics has demonstrated to ameliorate some of the mood alterations like anxiety or depression through different mechanisms in animal models.
Learning and Memory in Obesity
Obesity, microbiota and diet may affect episodic and semantic memory (Cheke et al., 2016; Noble et al., 2017). The explicit memory helps us to store information intentionally and consciously in order to remember past experiences. Using this type of memory we can remember autobiographic and episodic information, historical dates, vocabulary or different types of language. Various authors have demonstrated the effect of HFD or high-sugar diet on memory. For example, overfeeding in the neonatal period can lead to hippocampus damage (the main structure involved in memory tasks), causing microgliosis in this area after only 14 days of overfeeding, which can persist into adulthood (De Luca et al., 2016). In adolescents, memory impairments have been described after 4 weeks of HFD (Del Rio et al., 2016). Administration of sucrose to rats when they were progressing through puberty and adolescence may cause deficits in recognition memory tasks (Jurdak and Kanarek, 2009; Reichelt et al., 2015).
However, other data are controversial, probably due to methodological differences. Heyward et al. (2012) found that mice fed with HFD over 23 weeks exhibited intact novel object recognition but cognitive impairment in object location memory task (OLM). Also, Krishna et al. (2015) showed that novel object recognition memory was unaffected after HFD in female mice. Underwood and Thompson (2016) showed that both sexes fed HFD for 12 weeks were equally impaired in cognitive tasks.
The influence of diets rich in saturated fat on spatial learning and memory has also been reported in animal models. Collison et al. (2010) fed adult mice with a trans-fatty-acid (TFA)-enriched diet, monosodium glutamate (MSG) or a combination of both (TFA+MSG). TFA+MSG caused impairment in locating the hidden platform in the Morris Water Maze (MWM), showing a reduction in spatial cognition. Similar results were found by Guimarães et al. (2017) using the novel object recognition memory test in adult rats injected with MSG subcutaneously during the first 5 days of life to induce obesity. In adolescent animals, various authors also evidenced the relationship between juvenile HFD and spatial cognitive deficits (Boitard et al., 2012; Wang et al., 2015). Interestingly, this type of diet did not affect spatial performance in adulthood (Boitard et al., 2014) and these cognitive deficits could be reversed by exercise (Klein et al., 2016). In adult animals, caloric restriction (CR) protected CNS. Spatial memory was significantly increased in the MWM in the CR group and significantly decreased in the high-calorie (HC) group demonstrating that long-term high caloric intake induces autophagy in the hippocampus, which may increase risk to cognitive impairments (Dong et al., 2015). Mielke et al. (2006), on the other hand, found that HFD did not affect spatial memory in the MWM. Other authors have questioned whether cognitive impairment precedes obesity or is a consequence of obesity (Gurung et al., 2016). In order to test this hypothesis, obesity sensitive and resistant strain rats were fed with a standard chow diet from 4 through 20 weeks of age. At 12 weeks of age sensitive rats showed significant memory impairment on MWM compared to resistant rats. Alterations in implicit memory associated with obesity have also been investigated. Implicit or unconscious memory refers to non-intentional acts that we keep in our brain. In the rodent, additional amounts of cholesterol in the diet (0.5 % dry weight) significantly improved short-term and long-term memory (Apryatin et al., 2017). However, addition of fructose, including in combination with HFD, significantly worsened short and long-term memory in mice. Furthermore, the effects of HFD on fear conditioning may differ in female and male mice. Hwang et al. (2010) demonstrated that HFD produces more cognitive impairment in male mice than females evaluated with PAT.
Animal studies have revealed a relationship between obesity, neuro-inflammation and memory impairments. For example, Wang et al. (2016) used an anti-inflammatory agent to show that chronic rhein (the main ingredient of rhubarb plant with anti-inflammatory properties) treatment prevented HFD-induced recognition memory impairment. The rhubarb-exposed rats also had an increased bacterial diversity in the ileum, which could also contribute or reflect the beneficial effects of the treatment (Peng et al., 2014). Wang et al. (2017) demonstrated that teasaponin, an active component of tea with anti-inflammatory effects, limit unfavorable gut microbiota alterations, reduced body weight, improved glucose tolerance and prevent recognition memory impairment in HFD-fed mice; it also improved neuroinflammation, gliosis and BDNF deficits in hippocampus. Bruce-Keller et al. (2015) reported that mice receiving HFD microbiota had significant disruptions in cognitive, exploratory and stereotypical behavior compared with mice receiving control-diet microbiota in the absence of significant differences in body weight. Maternal Western diet during gestation and lactation was also shown to modify offspring's microbiota activity and cognitive responses in Yucatan pigs with modifications in hippocampal neurogenesis (Val-Laillet et al., 2017).
In humans, several studies demonstrate that obesity is associated with cognitive decline and elevated risk of neurodegenerative diseases during aging (Mazon et al., 2017). Gunstad et al. (2007) demonstrated that obese adults, with a BMI greater than 25 in an age range between 22 and 82 years old, showed a poorer executive function test performance than normal weight adults (BMI, 18.5–24.9 kg/m2) and some differences in the attention test performance, suggesting an association between elevated BMI and lower cognitive performance, independently of age. Several studies with obese children showed alterations in attention and attentional shifting compared to normal children (Cserjési et al., 2007; Davis and Cooper, 2011; Maayan et al., 2011; Wirt et al., 2015) and visuospatial abilities (Li et al., 2008; Jansen et al., 2011; Martin et al., 2016).
Research reports that fat and sugar intake at different life periods may also impair cognition. For example, Francis and Stevenson (2011) showed that healthy undergraduate students with high (self-reported) fat and refined sugar intake presented impaired memory tasks related to hippocampal function. Similarly, Beilharz et al. (2015) showed that hippocampal-dependent memory is sensitive to high-energy diet and they found differences between chronic and acute exposure to high-energy diets. Cournot et al. (2006) showed that higher body mass index was associated with lower cognition scores in healthy, middle-aged, non-demented participants. Another study in an Australian cohort of school-aged children, reported that higher intake of a Western diet at age 14 was associated with worse cognitive performance 3 years later (Nyaradi et al., 2014). Crichton et al. (2012) showed that the daily consumption of low-fat foods may improve cognitive performance.
SFAs have also been associated with cognitive impairments. SFA intake in young adulthood, mid and later life, increases vulnerability to cognitive deficits and even neurological diseases (Solfrizzi et al., 2011; Okereke et al., 2012). Likewise, a higher intake of carbohydrates, particularly simple sugars, has been associated with impaired cognitive functions (Roberts et al., 2012), although these associations were not detected in all studies (Halyburton et al., 2007; Brinkworth et al., 2009; Beilharz et al., 2015). Higher intake of polyunsaturated fatty acids (PUFA) and higher PUFA to SFA ratios have been associated with improved memory functions (also in children) and decreased risk of memory impairments (Kalmijn et al., 2004; Morris et al., 2004; Devore et al., 2009).
There are also some studies reporting beneficial effects of probiotics on cognitive functions in humans. For example, a cocktail of different probiotics (Bifidobacterium bifidum W23, Bifidobacterium lactis W52, Lactobacillus acidophilus W37, Lactobacillus brevis W63, Lactobacillus casei W56, Lactobacillus salivarius W24 and L. lactis W19 and W58) improved cognitive reactivity to sad mood in normal individuals (Steenbergen et al., 2015). A study of healthy woman treated with probiotic-fermented milk showed that probiotics were able to modulate brain activity (measured using fMRI) in brain regions involved in mediating cognitive performance (Tillisch et al., 2013).
Anxiety and Depression in Obesity
Anxiety and depression are the most prevalent mental disorders in developed societies (World Health Organization, 2017). These often originate as a consequence of adverse experiences and maladaptation to stress. In addition, they show bidirectional associations with obesity and related metabolic disorders (e.g., type-2 diabetes, cardiovascular disease), as reported in both animal and human studies, summarized below.
Animal studies have investigated the effects of stress and Western diets leading to obesity on mood, and investigated the possible interactions and mediating mechanism. Santos et al. (2016) demonstrated that a high carbohydrate-containing diet administered for 12 weeks was anxiogenic and induced depressive symptoms after exposure to different stress paradigms. Chronic stress can also increase the consumption of food containing sugar and fat ingredients as a compensatory mechanism (comfort food) to reduce stress-related anxiety, which could lead to overeating and obesity long-term (Oliveira et al., 2015). In support of this hypothesis, other studies have shown that HFD administration to adult rats reduced anxiety (Leffa et al., 2015; McNeilly et al., 2015). A recent study also indicates that the combination of obesity (induced by diet) with chronic unpredictable mild stress (induced by unpredictable mild stressors like 8 h of food or water deprivation, confusing day and night, soaking the cage with water or horizontal oscillation for 20 min) induces depression and anxiety-like behaviors and the down-regulation of leptin/LepRb signaling (Yang et al., 2016). This is suggested as a possible mechanism mediating the negative consequences of obesity and stress on mood (Yang et al., 2016). In this regard, Haque et al. (2013) also indicate that leptin is important for mood and emotion regulation. They observed that animals exposed to stress (2 h immobilization) exhibited behavioral deficits, but these were reversed by exogenous leptin in a dose-dependent manner. Finger et al. (2010) showed that leptin-deficient (ob/ob) mice displayed higher levels of anxiety.
The connection between obesity and behavioral deficits is further supported by evidence showing that some anti-obesity therapies exert anxiolytic effects. Thus, several drugs used to treat obesity have attenuated behavioral alterations in mice, such as sibutramine (Santos et al., 2014), duloxetine (Chudasama and Bhatt, 2009), pioglitazone (Kurhe and Mahesh, 2016), celecoxib (Kurhe et al., 2014a), ondansetron (Kurhe and Mahesh, 2015), 3-methoxy-N-p-tolylquinoxalin-2-carboxamide (QCM-4) (Kurhe et al., 2014b, 2015), GPR120 agonist (Auguste et al., 2016). These findings suggest that obesity and emotional disorders, such as anxiety and depression, could share a common base. Recent works propose that part of this common base could be the gut microbiota coupled with its role in the regulation of the inflammatory tone. In support of this notion, some probiotic strains with anti-depressive effects also showed anti-obesity effects, suggesting that modulation of microbiota composition or their function could be beneficial for obesity-related depression (Schachter et al., 2017). Thus, Ohland et al. (2013) showed that administration of a strain of Lactobacillus helveticus restored the Firmicutes/Bacteroidetes ratio altered in HFD-fed mice and reduced anxiety-like behaviors. Abildgaard et al. (2017) showed that a mix of eight different strains of Bifidobacterium and Lactobacillus reduced depressive-like behaviors in HFD-fed mice in association with lowered IL-6 and TNF-α levels in serum. Therefore, the beneficial effects of some of these bacterial strains could be partly mediated by their ability to reduce the inflammatory tone affecting both obesity and mood disorders.
Epidemiological and clinical research studies in humans also support bi-directional associations between obesity, dietary patterns, and mood related disorders (see Figure 2). Systematic reviews and meta-analysis of longitudinal studies indicate that obesity increases the risk for onset of depression by 55% (de Wit et al., 2010) and depression increases the risk for obesity onset by 58% (Luppino et al., 2010). Although not all results are consistent, epidemiological data also suggest that a higher quality diet lowers the risk of onset of depressive symptoms (Molendijk et al., 2018) while consumption of high-sugar and high-saturated-fat diets is associated with greater depressive symptoms and depressed mood (Vermeulen et al., 2017). A couple of intervention studies also show that improvements in dietary quality (Mediterranean-style diet) led to improvements in depressive symptoms in adults (Opie et al., 2017; Parletta et al., 2017), further supporting the role of diet in depression. In addition, depression has been associated with alterations in the gut microbiota in a few cross-sectional studies (Cenit et al., 2017). Some of these studies also propose possible mediating mechanisms by which gut dysbiosis could contribute to mood alterations, such as changes in the tryptophan metabolism (Kelly et al., 2016).
Social Behavior and Obesity
Research shows a link between obesity and social behavioral alterations in animal models but evidence in humans is very limited. In rodents, Takase et al. (2016) observed that social interactions were altered by HFD consumption in adulthood, independently of obesity, since both experimental groups, the HFD-induced obesity group and a non-obese HFD-fed group, presented social alteration. Changes in social interaction during the prepuberty period, which could cause cognitive deficits in adulthood, are modulated by diet type in rats. For example, Arcego et al. (2016) demonstrated that rats exposed to 7 days of social isolation showed memory impairment and reduced BDNF, Na+, K+, ATPase activity, MAPK, AKT and phospho-AKT levels in PFCx; however, rats exposed to this stress situation but fed with HFD showed restored memory impairment as well as the aforementioned biochemical alterations. However, Choi et al. (2017) demonstrated that sugar-sweetened beverages consumed by young mice significantly promoted social aggression in the adult life, which represents one of the most detrimental long-term outcomes of neurodevelopmental disorders (Lesch et al., 2012). Maged1-deficient mice (MAGE family genes, related with Prader-Willi syndrome (PWS), which includes hyperphagia, repetitive and compulsive behaviors, and cognitive impairment) developed progressive obesity associated with hyperphagia and a complex behavioral syndrome including reduced social interactions, memory impairments, deficient sexual behaviors, as well as increased anxiety, suggesting a connection between metabolic and behavioral outcomes (Dombret et al., 2012). They also observed a reduction in oxytocin (important hormone involved in social behavior) but not its precursor in the hypothalamus of Mage1-defficient mutants, indicating that decreased oxytocine could be responsible for the changes in social behavior.
Microbiota may also be involved in diet-related social behavioral alterations. (Buffington et al., 2016) showed a link between maternal HFD (MHFD), gut microbial imbalance, ventral tegmental area plasticity and social behavior alterations in descendants. They also observed a fewer oxytocin immunoreactive neurons in the hypothalamus of the offspring. Administration of a strain of Lactobacillus reuteri (reduced in microbiota of both, MHFD and their descendants) to the offspring, corrected the oxytocin levels and social deficits in MHFD progeny, suggesting that probiotics may relieve abnormalities associated with neurodevelopmental disorders. Other studies have suggested that obesity-related inflammatory processes, originating either from the adipose tissue or gut microbiota, affect the brain, leading to substantial changes in different neuro circuitry, neuroendocrine activity (impaired feedback response to cortisol), neurotransmitter metabolism and activity (alteration in basal ganglia and dopamine system), and neurogenesis (impaired in the hippocampus) (Castanon et al., 2014). The findings indicate that obesity is related to neuropsychiatric comorbidities like fatigue, anhedonia, psychomotor slowing, decreased motivation, and depressed mood (Castanon et al., 2014) as well as to social disorders like autism spectrum disorder (Gareau, 2016).
Conclusions
The associations established between obesity and mental disorders (cognitive impairment and mood and social behavioral alterations) in epidemiological and experimental studies point to shared contributing factors and pathophysiological mechanisms. These associations could be related to dietary-induced alterations in the intestinal microbiota that, in turn, may contribute to (neuro) inflammation and dysregulation of the neuroendocrine system associated with obesity comorbid with mental impairments. This hypothesis has been confirmed to some extent in experimental models, which have investigated the effectiveness of some probiotics or compounds altering the gut microbiota, in both attenuating obesity and associated mental disorders. These studies, however, do not help us discern whether interventions affecting the gut ecosystem play a primary or secondary role in alleviating obesity-associated mental impairments. Fecal transplants have, however, provided more direct evidence for the role of dysbiotic microbiota in neurobehavioral alterations, since the colonization of lean mice with the HFD-induced microbiota of obese mice led to the neurologic complications of obesity. Although we still lack a full understanding of the mechanisms by which microbiota may influence the gut-brain axis and, thereby, brain function and behavior, studies have shed light on numerous factors, including: regulation of the gut barrier, inflammation and signaling through TLR that recognize bacterial motifs and mediate in the communication with ENS, regulation of enteroendocrine secretions and the HPA stress response, and production and regulation of host neurotransmitter levels and their receptors. However, further translational and functional studies are needed to progress in the identification of molecular targets/pathways that could be favorable modulated by microbiota-based interventions to help reduce obesity associated complications.
Author Contributions
All authors listed, have made substantial, direct and intellectual contribution to the work, and approved it for publication.
Conflict of Interest Statement
The authors declare that the research was conducted in the absence of any commercial or financial relationships that could be construed as a potential conflict of interest.
Acknowledgments
This work and the contract of AA were supported by grant AGL2014-52101-P from the Spanish Ministry of Economy and Competitiveness (MINECO, Spain). The PTA contract of IC and the pre-doctoral scholarship of IL-A from MINECO (Spain) are also fully acknowledged. The contract of MR-P was supported by the EU Project MyNewGut (No. 613979) from the 7th Framework Program.
References
Abildgaard, A., Elfving, B., Hokland, M., Wegener, G., and Lund, S. (2017). Probiotic treatment reduces depressive-like behaviour in rats independently of diet. Psychoneuroendocrinology 79, 40–48. doi: 10.1016/j.psyneuen.2017.02.014
Abraham, S. B., Rubino, D., Sinaii, N., Ramsey, S., and Nieman, L. K. (2013). Cortisol, obesity, and the metabolic syndrome: a cross-sectional study of obese subjects and review of the literature. Obesity (Silver Spring) 21, E105–E117. doi: 10.1002/oby.20083
Abrams, G. D., Bauer, H., and Sprinz, H. (1963). Influence of the normal flora on mucosal morphology and cellular renewal in the ileum. A comparison of germ-free and conventional mice. Lab Invest 12, 355–364.
Agusti, A., Moya-Perez, A., Campillo, I., Montserrat-de la Paz, S., Cerrudo, V., Perez-Villalba, A., et al. (2017). Bifidobacterium pseudocatenulatum cect 7765 ameliorates neuroendocrine alterations associated with an exaggerated stress response and anhedonia in obese mice. Mol. Neurobiol. doi: 10.1007/s12035-017-0768-z. [Epub ahead of print].
Ait-Belgnaoui, A., Durand, H., Cartier, C., Chaumaz, G., Eutamene, H., Ferrier, L., et al. (2012). Prevention of gut leakiness by a probiotic treatment leads to attenuated hpa response to an acute psychological stress in rats. Psychoneuroendocrinology 37, 1885–1895. doi: 10.1016/j.psyneuen.2012.03.024
Andoh, A., Tsujikawa, T., and Fujiyama, Y. (2003). Role of dietary fiber and short-chain fatty acids in the colon. Curr. Pharm. Des. 9, 347–358. doi: 10.2174/1381612033391973
Anitha, M., Vijay-Kumar, M., Sitaraman, S. V., Gewirtz, A. T., and Srinivasan, S. (2012). Gut microbial products regulate murine gastrointestinal motility via toll-like receptor 4 signaling. Gastroenterology 143, 1006–16 e4. doi: 10.1053/j.gastro.2012.06.034
Apryatin, S. A., Sidorova, Y. S., Shipelin, V. A., Balakina, A., Trusov, N. V., and Mazo, V. K. (2017). Neuromotor activity, anxiety and cognitive function in the in vivo model of alimentary hyperlipidemia and obesity. Bull. Exp. Biol. Med. 163, 37–41. doi: 10.1007/s10517-017-3732-z
Arcego, D. M., Krolow, R., Lampert, C., Toniazzo, A. P., Berlitz, C., Lazzaretti, C., et al. (2016). Early life adversities or high fat diet intake reduce cognitive function and alter Bdnf signaling in adult rats: interplay of these factors changes these effects. Int. J. Dev. Neurosci. 20, 16–25. doi: 10.1016/j.ijdevneu.2016.03.001
Auguste, S., Fisette, A., Fernandes, M. F., Hryhorczuk, C., Poitout, V., Alquier, T., et al. (2016). Central agonism of Gpr120 acutely inhibits food intake and food reward and chronically suppresses anxiety-like behavior in mice. Int. J. Neuropsychopharmacol. 19:pyw014. doi: 10.1093/ijnp/pyw014
Bäckhed, F., Ley, R. E., Sonnenburg, J. L., Peterson, D. A., and Gordon, J. I. (2005). Host-bacterial mutualism in the human intestine. Science 307, 1915–1920. doi: 10.1126/science.1104816
Baschant, U., and Tuckermann, J. (2010). The role of the glucocorticoid receptor in inflammation and immunity. The Role of the Glucocorticoid Receptor in Inflammation and Immunity. J. Steroid Biochem. Mol. Biol. 120, 69–75. doi: 10.1016/j.jsbmb.2010.03.058
Beilharz, J. E., Maniam, J., and Morris, M. J. (2015). Diet-induced cognitive deficits: the role of fat and sugar, potential mechanisms and nutritional interventions. Nutrients 7, 6719–6738. doi: 10.3390/nu7085307
Bercik, P., and Collins, S. M. (2014). The effects of inflammation, infection and antibiotics on the microbiota-gut-brain axis. Adv. Exp. Med. Biol. 817, 279–289. doi: 10.1007/978-1-4939-0897-4_13
Bercik, P., Verdu, E. F., Foster, J. A., Macri, J., Potter, M., Huang, X., et al. (2010). Chronic gastrointestinal inflammation induces anxiety-like behavior and alters central nervous system biochemistry in mice. Gastroenterology 139, 2102–2112 e1. doi: 10.1053/j.gastro.2010.06.063
Berkenbosch, F., van Oers, J., del Rey, A., Tilders, F., and Besedovsky, H. (1987). Corticotropin-releasing factor-producing neurons in the rat activated by interleukin-1. Science 238, 524–526. doi: 10.1126/science.2443979
Besedovsky, H., del Rey, A., Sorkin, E., and Dinarello, C. A. (1986). Immunoregulatory feedback between interleukin-1 and glucocorticoid hormones. Science 233, 652–654. doi: 10.1126/science.3014662
Bjorntorp, P., and Rosmond, R. (2000). Obesity and cortisol. Nutrition 16, 924–936. doi: 10.1016/S0899-9007(00)00422-6
Block, J. P., He, Y., Zaslavsky, A. M., Ding, L., and Ayanian, J. Z. (2009). Psychosocial stress and change in weight among us adults. Am. J. Epidemiol. 170, 181–192. doi: 10.1093/aje/kwp104
Boitard, C., Cavaroc, A., Sauvant, J., Aubert, A., Castanon, N., Layé, S., et al. (2014). Impairment of hippocampal-dependent memory induced by juvenile high-fat diet intake is associated with enhanced hippocampal inflammation in rats. Brain Behav. Immun. 40, 9–17. doi: 10.1016/j.bbi.2014.03.005
Boitard, C., Etchamendy, N., Sauvant, J., Aubert, A., Tronel, S., Marighetto, A., et al. (2012). Juvenile, but not adult exposure to high-fat diet impairs relational memory and hippocampal neurogenesis in mice. Hippocampus, 22, 2095–2100. doi: 10.1002/hipo.22032
Bravo, J. A., Forsythe, P., Chew, M. V., Escaravage, E., Savignac, H. M., Dinan, T. G., et al. (2011). Ingestion of lactobacillus strain regulates emotional behavior and central gaba receptor expression in a mouse via the vagus nerve. Proc. Natl. Acad. Sci. U.S.A. 108, 16050–16055. doi: 10.1073/pnas.1102999108
Brierley, S. M., and Linden, D. R. (2014). Neuroplasticity and Dysfunction after Gastrointestinal Inflammation. Nat. Rev. Gastroenterol. Hepatol. 11, 611–627. doi: 10.1038/nrgastro.2014.103
Brinkworth, G. D., Buckley, J. D., Noakes, M., Clifton, P. M., and Wilson, C. J. (2009). Long-term effects of a very low-carbohydrate diet and a low-fat diet on mood and cognitive function. Arch. Inter. Med. 169, 1873–1880. doi: 10.1001/archinternmed.2009.329
Browning, K. N., and Travagli, R. A. (2014). Central nervous system control of gastrointestinal motility and secretion and modulation of gastrointestinal functions. Compr. Physiol. 4, 1339–1368. doi: 10.1002/cphy.c130055
Bruce-Keller, A. J., Salbaum, J. M., Luo, M., Blanchard, E., Taylor, C. M., Welsh, D. A., et al. (2015). Obese-type gut microbiota induce neurobehavioral changes in the absence of obesity. Biol. Psychiatry 77, 607–615. doi: 10.1016/j.biopsych.2014.07.012
Brun, P., Giron, M. C., Qesari, M., Porzionato, A., Caputi, V., Zoppellaro, C., et al. (2013). Toll-like receptor 2 regulates intestinal inflammation by controlling integrity of the enteric nervous system. Gastroenterology 145, 1323–1333. doi: 10.1053/j.gastro.2013.08.047
Buffington, S. A., Di Prisco, G. V., Auchtung, T. A., Ajami, N. J., Petrosino, J. F., and Costa-Mattioli, M. (2016). Microbial reconstitution reverses maternal diet-induced social and synaptic deficits in offspring. Cell 165, 1762–1775. doi: 10.1016/j.cell.2016.06.001
Carabotti, M., Scirocco, A., Maselli, M. A., and Severi, C. (2015). The gut-brain axis: interactions between enteric microbiota, central and enteric nervous systems. Ann. Gastroenterol. 28, 203–209.
Cario, E., Gerken, G., and Podolsky, D. K. (2004). Toll-like receptor 2 enhances Zo-1-associated intestinal epithelial barrier integrity via protein kinase C. Gastroenterology 127, 224–238. doi: 10.1053/j.gastro.2004.04.015
Castanon, N., Lasselin, J., and Capuron, L. (2014). Neuropsychiatric comorbidity in obesity: role of inflammatory processes. Front. Endocrinol. 5:74. doi: 10.3389/fendo.2014.00074
Cenit, M. C., Sanz, Y., and Codoner-Franch, P. (2017). Influence of gut microbiota on neuropsychiatric disorders. World J. Gastroenterol. 23, 5486–5498. doi: 10.3748/wjg.v23.i30.5486
Champaneri, S., Xu, X., Carnethon, M. R., Bertoni, A. G., Seeman, T., DeSantis, A. S., et al. (2013). Diurnal salivary cortisol is associated with body mass index and waist circumference: the multiethnic study of atherosclerosis. Obesity (Silver Spring) 21, E56–E63. doi: 10.1002/oby.20047
Chan, E., and Riches, D. W. (2001). Ifn-Γ+ Lps induction of inos is modulated by Erk, Jnk/Sapk, and P38 Mapk in a mouse macrophage cell line. Am. J. Physiol. Cell Physiol. 280, C441–C450. doi: 10.1152/ajpcell.2001.280.3.C441
Cheke, L. G., Simons, J. S., and Clayton, N. S. (2016). Higher body mass index is associated with episodic memory deficits in young adults. Q. J. Exp. Psychol. 69, 2305–2316. doi: 10.1080/17470218.2015.1099163
Choi, J. Y., Park, M. N., Kim, C. S., Lee, Y. K., Choi, E. Y., Chun, W. Y., et al. (2017). Long-term consumption of sugar-sweetened beverage during the growth period promotes social aggression in adult mice with proinflammatory responses in the brain. Sci. Rep. 7:45693. doi: 10.1038/srep45693
Chudasama, H. P., and Bhatt, P. A. (2009). Evaluation of anti-obesity activity of duloxetine in comparison with sibutramine along with its anti-depressant activity: an experimental study in obese rats. Can. J. Physiol. Pharmacol. 87, 900–907. doi: 10.1139/Y09-080
Clarke, G., Grenham, S., Scully, P., Fitzgerald, P., Moloney, R. D., Shanahan, F., et al. (2013). The microbiome-gut-brain axis during early life regulates the hippocampal serotonergic system in a sex-dependent manner. Mol. Psychiatry 18, 666–673. doi: 10.1038/mp.2012.77
Cole, S. W., Hawkley, L. C., Arevalo, J. M., Sung, C. Y., Rose, R. M., and Cacioppo, J. T. (2007). Social regulation of gene expression in human leukocytes. Genome Biol. 8:R189. doi: 10.1186/gb-2007-8-9-r189
Collado-Hidalgo, A., Sung, C., and Cole, S. (2006). Adrenergic inhibition of innate anti-viral response: pka blockade of type i interferon gene transcription mediates catecholamine support for HIV-1 replication. Brain Behav. Immun. 20, 552–563. doi: 10.1016/j.bbi.2006.01.005
Collison, K. S., Makhoul, N. J., Inglis, A., Al-Johi, M., Zaidi, M. Z., Maqbool, Z., et al. (2010). Dietary trans-fat combined with monosodium glutamate induces dyslipidemia and impairs spatial memory. Physiol. Behav. 99, 334–342. doi: 10.1016/j.physbeh.2009.11.010
Cournot, M., Marquie, J. C., Ansiau, D., Martinaud, C., Fonds, H., Ferrieres, J., et al. (2006). Relation between body mass index and cognitive function in healthy middle-aged men and women. Neurology 67, 1208–1214. doi: 10.1212/01.wnl.0000238082.13860.50
Crichton, G. E., Murphy, K. J., Howe, P. R., Buckley, J. D., and Bryan, J. (2012). Dairy consumption and working memory performance in overweight and obese adults. Appetite 59, 34–40. doi: 10.1016/j.appet.2012.03.019
Cryan, J. F., and O'Mahony, S. M. (2011). The microbiome-gut-brain axis: from bowel to behavior. Neurogastroenterol. Motil. 23, 187–192. doi: 10.1111/j.1365-2982.2010.01664.x
Cserjési, R., Molnár, D., Luminet, O., and Lénárd, L. (2007). Is there any relationship between obesity and mental flexibility in children? Appetite 49, 675–678. doi: 10.1016/j.appet.2007.04.001
Czimmer, J., Million, M., and Taché, Y. (2006). Urocortin 2 acts centrally to delay gastric emptying through sympathetic pathways while CRF and urocortin 1 inhibitory actions are vagal dependent in rats. Am. J. Physiol. Gastrointest. Liver Physiol. 290, G511–G518. doi: 10.1152/ajpgi.00289.2005
Dai, C., Zheng, C. Q., Jiang, M., Ma, X. Y., and Jiang, L. J. (2013). Probiotics and irritable bowel syndrome. World J. Gastroenterol. 19, 5973–5980. doi: 10.3748/wjg.v19.i36.5973
Dallman, M. F., Pecoraro, N. C., and la Fleur, S. E. (2005). Chronic stress and comfort foods: self-medication and abdominal obesity. Brain Behav. Immun. 19, 275–280. doi: 10.1016/j.bbi.2004.11.004
Daly, D. M., Park, S. J., Valinsky, W. C., and Beyak, M. J. (2011). Impaired intestinal afferent nerve satiety signalling and vagal afferent excitability in diet induced obesity in the mouse. J. Physiol. 589(Pt 11), 2857–2870. doi: 10.1113/jphysiol.2010.204594
Dantzer, R., O'Connor, J. C., Freund, G. G., Johnson, R. W., and Kelley, K. W. (2008). From inflammation to sickness and depression: when the immune system subjugates the brain. Nat. Rev. Neurosci. 9, 46–56. doi: 10.1038/nrn2297
Davis, C. L., and Cooper, S. (2011). Fitness, fatness, cognition, behavior, and academic achievement among overweight children: do cross-sectional associations correspond to exercise trial outcomes? Prev. Med. 52(Suppl. 1), S65–S69. doi: 10.1016/j.ypmed.2011.01.020
de Kort, S., Keszthelyi, D., and Masclee, A. A. (2011). Leaky gut and diabetes mellitus: what is the link? Obes Rev. 12, 449–458. doi: 10.1111/j.1467-789X.2010.00845.x
de Lartigue, G., de La Serre, C. B., and Raybould, H. E. (2011). Vagal afferent neurons in high fat diet-induced obesity; intestinal microflora, gut inflammation and cholecystokinin. Physiol. Behav. 105, 100–105. doi: 10.1016/j.physbeh.2011.02.040
De Luca, S. N., Ziko, I., Sominsky, L., Nguyen, J. C., Dinan, T., Miller, A. A., et al. (2016). Early life overfeeding impairs spatial memory performance by reducing microglial sensitivity to learning. J. Neuroinflammation. 13:112. doi: 10.1186/s12974-016-0578-7
de Melo, L. G. P., Nunes SOV1Anderson, G., Vargas, H. O., Barbosa, D. S., Galecki, P., Carvalho, A. F., et al. (2017). Shared metabolic and immune-inflammatory, oxidative and nitrosative stress pathways in the metabolic syndrome and mood disorders. Prog. Neuropsychopharmacol. Biol. Psychiatry. 78, 34–50. doi: 10.1016/j.pnpbp.2017.04.027
de Wit, L., Luppino, F., van Straten, A., Penninx, B., Zitman, F., and Cuijpers, P. (2010). Depression and obesity: a meta-analysis of community-based studies. Psychiatry Res. 178, 230–235. doi: 10.1016/j.psychres.2009.04.015
Del Rio, D., Morales, L., Ruiz-Gayo, M., and Del Olmo, N. (2016). Effect of high-fat diets on mood and learning performance in adolescent mice. Behav. Brain Res. 311, 167–172. doi: 10.1016/j.bbr.2016.04.052
Delzenne, N. M., Cani, P. D., Daubioul, C., and Neyrinck, A. M. (2005). Impact of inulin and oligofructose on gastrointestinal peptides. Br. J. Nutr. 93(Suppl. 1), S157–S161. doi: 10.1079/BJN20041342
Delzenne, N. M., Neyrinck, A. M., Backhed, F., and Cani, P. D. (2011). Targeting gut microbiota in obesity: effects of prebiotics and probiotics. Nat. Rev. Endocrinol. 7, 639–646. doi: 10.1038/nrendo.2011.126
Desbonnet, L., Garrett, L., Clarke, G., Bienenstock, J., and Dinan, T. G. (2008). The probiotic bifidobacteria infantis: an assessment of potential antidepressant properties in the rat. J. Psychiatr. Res. 43, 164–174. doi: 10.1016/j.jpsychires.2008.03.009
Desbonnet, L., Garrett, L., Clarke, G., Kiely, B., Cryan, J. F., and Dinan, T. G. (2010). Effects of the probiotic bifidobacterium infantis in the maternal separation model of depression. Neuroscience 170, 1179–1188. doi: 10.1016/j.neuroscience.2010.08.005
Desbriere, R., Vuaroqueaux, V., Achard, V., Boullu-Ciocca, S., Labuhn, M., Dutour, A., et al. (2006). 11beta-hydroxysteroid dehydrogenase type 1 mrna is increased in both visceral and subcutaneous adipose tissue of obese patients. Obesity 14, 794–798. doi: 10.1038/oby.2006.92
Deuschle, M., Schweiger, U., Weber, B., Gotthardt, U., Korner, A., Schmider, J., et al. (1997). Diurnal activity and pulsatility of the hypothalamus-pituitary-adrenal system in male depressed patients and healthy controls. J. Clin. Endocrinol. Metab. 82, 234–238. doi: 10.1210/jcem.82.1.3689
Devore, E. E., Grodstein, F., van Rooij, F. J., Hofman, A., Rosner, B., Stampfer, M. J., et al. (2009). Dietary intake of fish and omega-3 fatty acids in relation to long-term dementia risk. Am. J. Clin. Nutr. 90, 170–176. doi: 10.3945/ajcn.2008.27037
Dinan, T. G., Quigley, E. M., Ahmed, S. M., Scully, P., O'Brien, S., O'Mahony, L., et al. (2006). Hypothalamic-pituitary-gut axis dysregulation in irritable bowel syndrome: plasma cytokines as a potential biomarker? Gastroenterology 130, 304–311. doi: 10.1053/j.gastro.2005.11.033
Dombret, C., Nguyen, T., Schakman, O., Michaud, J. L., Hardin-Pouzet, H., Bertrand, M. J., et al. (2012). Loss of Maged1 results in obesity, deficits of social interactions, impaired sexual behavior and severe alteration of mature oxytocin production in the hypothalamus. Hum. Mol. Genet. 21, 4703–4717. doi: 10.1093/hmg/dds310
Dong, W., Wang, R., Ma, L. N., Xu, B. L., Zhang, J. S., Zhao, Z. W., et al. (2015). Autophagy involving age-related cognitive behavior and hippocampus injury is modulated by different caloric intake in mice. Int. J. Clin. Exp. Med. 8, 11843–11853.
Dowlati, Y., Herrmann, N., Swardfager, W., Liu, H., Sham, L., Reim, E. K., et al. (2010). A meta-analysis of cytokines in major depression. Biol. Psychiatry 67, 446–457. doi: 10.1016/j.biopsych.2009.09.033
Dupont, J. R., Jervis, H. R., and Sprinz, H. (1965). Auerbach's plexus of the rat cecum in relation to the germfree state. J. Comp. Neurol. 125, 11–18. doi: 10.1002/cne.901250103
Fandriks, L. (2017). Roles of the gut in the metabolic syndrome: an overview. J. Intern. Med. 281, 319–336. doi: 10.1111/joim.12584
Finger, B. C., Dinan, T. G., and Cryan, J. F. (2010). Leptin-deficient mice retain normal appetitive spatial learning yet exhibit marked increases in anxiety-related behaviours. Psychopharmacology 210, 559–568. doi: 10.1007/s00213-010-1858-z
Foster, J. A., Rinaman, L., and Cryan, J. F. (2017). Stress & the gut-brain axis: regulation by the microbiome. Neurobiol. Stress 7, 124–136. doi: 10.1016/j.ynstr.2017.03.001
Francis, H. M., and Stevenson, R. J. (2011). Higher reported saturated fat and refined sugar intake is associated with reduced hippocampal-dependent memory and sensitivity to interoceptive signals. Behav. Neurosci. 125, 943–955. doi: 10.1037/a0025998
Furness, J. B., Callaghan, B. P., Rivera, L. R., and Cho, H. J. (2014). The enteric nervous system and gastrointestinal innervation: integrated local and central control. Adv. Exp. Med. Biol. 817, 39–71. doi: 10.1007/978-1-4939-0897-4_3
García-Pardo, M. P., Blanco-Gandía, M. C., Valiente-Lluch, M., Rodríguez-Arias, M., Miñarro, J., and Aguilar, M. A. (2015). Long-term effects of repeated social stress on the conditioned place preference induced by mdma in mice. Prog. Neuro-Psychopharmacol. Biol. Psychiatry 63, 98–109. doi: 10.1016/j.pnpbp.2015.06.006
Gareau, M. G. (2016). Chapter eleven-cognitive function and the microbiome. Int. Rev. Neurobiol. 131, 227–246. doi: 10.1016/bs.irn.2016.08.001
Gill, S. R., Pop, M., Deboy, R. T., Eckburg, P. B., Turnbaugh, P. J., Samuel, B. S., et al. (2006). Metagenomic analysis of the human distal gut microbiome. Science 312, 1355–1359. doi: 10.1126/science.1124234
Goehler, L. E., Park, S. M., Opitz, N., Lyte, M., and Gaykema, R. P. (2008). Campylobacter jejuni infection increases anxiety-like behavior in the holeboard: possible anatomical substrates for viscerosensory modulation of exploratory behavior. Brain Behav. Immun. 22, 354–366. doi: 10.1016/j.bbi.2007.08.009
Grasset, E., Puel, A., Charpentier, J., Collet, X., Christensen, J. E., Tercé, F., et al. (2017). A specific gut microbiota dysbiosis of type 2 diabetic mice induces glp-1 resistance through an enteric no-dependent and gut-brain axis mechanism. Cell Metab. 25, 1075–1090. doi: 10.1016/j.cmet.2017.04.013
Gregor, M. F., and Hotamisligil, G. S. (2011). Inflammatory mechanisms in obesity. Annu. Rev. Immunol. 29, 415–445. doi: 10.1146/annurev-immunol-031210-101322
Greiner, T., and Backhed, F. (2011). Effects of the gut microbiota on obesity and glucose homeostasis. Trends Endocrinol. Metab. 22, 117–123. doi: 10.1016/j.tem.2011.01.002
Grenham, S., Clarke, G., Cryan, J. F., and Dinan, T. G. (2011). Brain-gut-microbe communication in health and disease. Front. Physiol. 2:94. doi: 10.3389/fphys.2011.00094
Guillemot-Legris, O., and Muccioli, G. G. (2017). Obesity-induced neuroinflammation: beyond the hypothalamus. Trends Neurosci. 40, 237–253. doi: 10.1016/j.tins.2017.02.005
Guimarães, E. D., de Caires Júnior, L. C., Musso, C. M., Macedo de Almeida, M., Gonçalves, C. F., Pettersen, K. G., et al. (2017). Altered behavior of adult obese rats by monosodium l-glutamate neonatal treatment is related to hypercorticosteronemia and activation of hypothalamic Erk1 and Erk2. Nutr. Neurosci. 20, 153–160. doi: 10.1179/1476830515Y.0000000004.
Gunstad, J., Paul, R. H., Cohen, R. A., Tate, D. F., Spitznagel, M. B., and Gordon, E. (2007). Elevated body mass index is associated with executive dysfunction in otherwise healthy adults. Compr. Psychiatry 48, 57–61. doi: 10.1016/j.comppsych.2006.05.001
Gurung, S., Agbaga, M. P., and Myers, D. A. (2016). Cognitive differences between sprague-dawley rats selectively bred for sensitivity or resistance to diet induced obesity. Behav. Brain Res. 311, 122–130. doi: 10.1016/j.bbr.2016.05.018
Halyburton, A. K., Brinkworth, G. D., Wilson, C. J., Noakes, M., Buckley, J. D., Keogh, J. B., et al. (2007). Low-and high-carbohydrate weight-loss diets have similar effects on mood but not cognitive performance. Am. J. Clin. Nutr. 86, 580–587. doi: 10.1093/ajcn/86.3.580
Haque, Z., Akbar, N., Yasmin, F., Haleem, M. A., and Haleem, D. J. (2013). Inhibition of immobilization stress-induced anorexia, behavioral deficits, and plasma corticosterone secretion by injected leptin in rats. Stress 16, 353–362. doi: 10.3109/10253890.2012.736047
Hayward, J. H., and Lee, S. J. (2014). A decade of research on Tlr2 discovering its pivotal role in glial activation and neuroinflammation in neurodegenerative diseases. Exp. Neurobiol. 23, 138–147. doi: 10.5607/en.2014.23.2.138
Heyward, F. D., Walton, R. G., Carle, M. S., Coleman, M. A., Garvey, W. T., and Sweatt, J. D. (2012). Adult mice maintained on a high-fat diet exhibit object location memory deficits and reduced hippocampal sirt1 gene expression. Neurobiol. Learn. Mem. 98, 25–32. doi: 10.1016/j.nlm.2012.04.005
Hughes, D. T., and Sperandio, V. (2008). Inter-kingdom signalling: communication between bacteria and their hosts. Nat. Rev. Microbiol. 6, 111–120. doi: 10.1038/nrmicro1836
Hwang, D. H., Kim, J. A., and Lee, J. Y. (2016). Mechanisms for the activation of toll-like receptor 2/4 by saturated fatty acids and inhibition by docosahexaenoic acid. Eur. J. Pharmacol. 785, 24–35. doi: 10.1016/j.ejphar.2016.04.024
Hwang, L. L., Wang, C. H., Li, T. L., Chang, S. D., Lin, L. C., Chen, C. P., et al. (2010). Sex differences in high-fat diet-induced obesity, metabolic alterations and learning, and synaptic plasticity deficits in mice. Obesity 18, 463–469. doi: 10.1038/oby.2009.273
Hyland, N. P., and Cryan, J. F. (2016). Microbe-host interactions: influence of the gut microbiota on the enteric nervous system. Dev. Biol. 417, 182–187. doi: 10.1016/j.ydbio.2016.06.027
Iyer, L. M., Aravind, L., Coon, S. L., Klein, D. C., and Koonin, E. V. (2004). Evolution of cell–cell signaling in animals: did late horizontal gene transfer from bacteria have a role?. Trends Genet. 20, 292–299. doi: 10.1016/j.tig.2004.05.007
Jansen, P., Schmelter, A., Kasten, L., and Heil, M. (2011). Impaired mental rotation performance in overweight children. Appetite 56, 766–769. doi: 10.1016/j.appet.2011.02.021
Johnson, L. A., Zuloaga, K. L., Kugelman, T. L., Mader, K. S., Morré, J. T., Zuloaga, D. G., et al. (2016). Amelioration of metabolic syndrome-associated cognitive impairments in mice via a reduction in dietary fat content or infusion of non-diabetic plasma. EBioMedicine 3, 26–42. doi: 10.1016/j.ebiom.2015.12.008
Jurdak, N., and Kanarek, R. B. (2009). Sucrose-induced obesity impairs novel object recognition learning in young rats. Physiol. Behav. 96, 1–5. doi: 10.1016/j.physbeh.2008.07.023
Kalmijn, S., van Boxtel, M. P., Ocke, M., Verschuren, W. M., Kromhout, D., and Launer, L. J. (2004). Dietary intake of fatty acids and fish in relation to cognitive performance at middle age. Neurology 62, 275–280. doi: 10.1212/01.WNL.0000103860.75218.A5
Karaki, S., Mitsui, R., Hayashi, H., Kato, I., Sugiya, H., Iwanaga, T., et al. (2006). Short-chain fatty acid receptor, Gpr43, is expressed by enteroendocrine cells and mucosal mast cells in rat intestine. Cell Tissue Res. 324, 353–360. doi: 10.1007/s00441-005-0140-x
Kelly, J. R., Borre, Y., O' Brien, C., Patterson, E., El Aidy, S., Deane, J., et al. (2016). Transferring the blues: depression-associated gut microbiota induces neurobehavioural changes in the rat. J. Psychiatr. Res. 82, 109–118. doi: 10.1016/j.jpsychires.2016.07.019
Kihara, N., Fujimura, M., Yamamoto, I., Itoh, E., Inui, A., and Fujimiya, M. (2001). Effects of central and peripheral urocortin on fed and fasted gastroduodenal motor activity in conscious rats. Am. J. Physiol. Gastrointest Liver Physiol. 280, G406–G419. doi: 10.1152/ajpgi.2001.280.3.G406
Kim, C., Ho, D. H., Suk, J. E., You, S., Michael, S., Kang, J., et al. (2013). Neuron-released oligomeric alpha-synuclein is an endogenous agonist of tlr2 for paracrine activation of microglia. Nat. Commun. 4:1562. doi: 10.1038/ncomms2534
Klein, C., Jonas, W., Iggena, D., Empl, L., Rivalan, M., Wiedmer, P., et al. (2016). Exercise prevents high-fat diet-induced impairment of flexible memory expression in the water maze and modulates adult hippocampal neurogenesis in mice. Neurobiol. Learn. Mem. 131, 26–35. doi: 10.1016/j.nlm.2016.03.002
Koppel, N., and Balskus, E. P. (2016). Exploring and Understanding the biochemical diversity of the human microbiota. Cell Chem. Biol. 23, 18–30. doi: 10.1016/j.chembiol.2015.12.008
Krishna, S., Keralapurath, M. M., Lin, Z., Wagner, J. J., de La Serre, C. B., Harn, D. A., et al. (2015). Neurochemical and electrophysiological deficits in the ventral hippocampus and selective behavioral alterations caused by high-fat diet in female C57bl/6 mice. Neuroscience 297:170. doi: 10.1016/j.neuroscience.2015.03.068
Kurhe, Y., and Mahesh, R. (2015). Ondansetron attenuates co-morbid depression and anxiety associated with obesity by inhibiting the biochemical alterations and improving serotonergic neurotransmission. Pharmacol. Biochem. Behav. 136, 107–116. doi: 10.1016/j.pbb.2015.07.004
Kurhe, Y., and Mahesh, R. (2016). Pioglitazone, a Pparγ agonist rescues depression associated with obesity using chronic unpredictable mild stress model in experimental mice. Neurobiol. Stress 3, 114–121. doi: 10.1016/j.ynstr.2016.05.001
Kurhe, Y., Mahesh, R., and Devadoss, T. (2015). Qcm-4, a 5-Ht 3 receptor antagonist ameliorates plasma hpa axis hyperactivity, leptin resistance and brain oxidative stress in depression and anxiety-like behavior in obese mice. Biochem. Biophys. Res. Commun. 456, 74–79. doi: 10.1016/j.bbrc.2014.11.036
Kurhe, Y., Mahesh, R., and Gupta, D. (2014a). Effect of a selective cyclooxygenase type 2 inhibitor celecoxib on depression associated with obesity in mice: an approach using behavioral tests. Neurochem. Res. 39, 1395–1402. doi: 10.1007/s11064-014-1322-2
Kurhe, Y., Mahesh, R., Gupta, D., and Devadoss, T. (2014b). Qcm-4, a serotonergic type 3 receptor modulator attenuates depression co-morbid with obesity in mice: an approach based on behavioral and biochemical investigations. Eur. J. Pharmacol. 740, 611–618. doi: 10.1016/j.ejphar.2014.06.020
Latorre, E., Layunta, E., Grasa, L., Castro, M., Pardo, J., Gomollon, F., et al. (2016). Intestinal serotonin transporter inhibition by toll-like receptor 2 activation. a feedback modulation. PLoS ONE 11:e0169303. doi: 10.1371/journal.pone.0169303
Leffa, D. D., Valvassori, S. S., Varela, R. B., Lopes-Borges, J., Daumann, F., Longaretti, L. M., et al. (2015). Effects of palatable cafeteria diet on cognitive and noncognitive behaviors and brain neurotrophins' levels in mice. Metab. Brain Dis. 30, 1073–1082. doi: 10.1007/s11011-015-9682-0.
Leibowitz, S. F., and Wortley, K. E. (2004). Hypothalamic control of energy balance: different peptides, different functions. Peptides 25, 473–504. doi: 10.1016/j.peptides.2004.02.006
Leonard, B., and Maes, M. (2012). Mechanistic explanations how cell-mediated immune activation, inflammation and oxidative and nitrosative stress pathways and their sequels and concomitants play a role in the pathophysiology of unipolar depression. Neurosci. Biobehav. Rev. 36, 764–785. doi: 10.1016/j.neubiorev.2011.12.005
Lesch, K. P., Araragi, N., Waider, J., van den Hove, D., and Gutknecht, L. (2012). Targeting brain serotonin synthesis: insights into neurodevelopmental disorders with long-term outcomes related to negative emotionality, aggression and antisocial behaviour. Philos. Trans. R. Soc. B 367, 2426–2443. doi: 10.1098/rstb.2012.0039
Ley, R. E., Turnbaugh, P. J., Klein, S., and Gordon, J. I. (2006a). Microbial ecology: human gut microbes associated with obesity. Nature 444, 1022–1023. doi: 10.1038/4441022a
Li, Y., Dai, Q., Jackson, J. C., and Zhang, J. (2008). Overweight is associated with decreased cognitive functioning among school-age children and adolescents. Obesity (Silver Spring) 16, 1809–1815. doi: 10.1038/oby.2008.296
Lin, C. S., Chang, C. J., Lu, C. C., Martel, J., Ojcius, D. M., Ko, Y. F., et al. (2014). Impact of the gut microbiota, prebiotics, and probiotics on human health and disease. Biomed. J. 37, 259–268. doi: 10.4103/2319-4170.138314
Logan, A. C., and Katzman, M. (2005). Major depressive disorder: probiotics may be an adjuvant therapy. Med. Hypotheses 64, 533–538. doi: 10.1016/j.mehy.2004.08.019
Lucas, K., and Maes, M. (2013). Role of the toll like receptor (Tlr) radical cycle in chronic inflammation: possible treatments targeting the Tlr4 pathway. Mol. Neurobiol. 48, 190–204. doi: 10.1007/s12035-013-8425-7
Lumeng, C. N., and Saltiel, A. R. (2011). Inflammatory links between obesity and metabolic disease. J. Clin. Invest. 121, 2111–2117. doi: 10.1172/JCI57132
Luppino, F. S., de Wit, L. M., Bouvy, P. F., Stijnen, T., Cuijpers, P., Penninx, B. W., et al. (2010). Overweight, obesity, and depression: a systematic review and meta-analysis of longitudinal studies. Arch. Gen. Psychiatry 67, 220–229. doi: 10.1001/archgenpsychiatry.2010.2
Maayan, L., Hoogendoorn, C., Sweat, V., and Convit, A. (2011). Disinhibited eating in obese adolescents is associated with orbitofrontal volume reductions and executive dysfunction. Obesity (Silver Spring). 19, 1382–1387. doi: 10.1038/oby.2011.15
Maes, M., Kubera, M., and Leunis, J. C. (2008). The gut-brain barrier in major depression: intestinal mucosal dysfunction with an increased translocation of lps from gram negative enterobacteria (leaky gut) plays a role in the inflammatory pathophysiology of depression. Neuro Endocrinol. Lett. 29, 117–124.
Maes, M., Kubera, M., Mihaylova, I., Geffard, M., Galecki, P., Leunis, J. C., et al. (2013). Increased autoimmune responses against auto-epitopes modified by oxidative and nitrosative damage in depression: implications for the pathways to chronic depression and neuroprogression. J. Affect. Disord. 149, 23–29. doi: 10.1016/j.jad.2012.06.039
Maes, M., Mihaylova, I., and Leunis, J. C. (2007). Increased serum iga and igm against lps of enterobacteria in chronic fatigue syndrome (Cfs): indication for the involvement of gram-negative enterobacteria in the etiology of cfs and for the presence of an increased gut-intestinal permeability. J. Affect. Disord. 99, 237–240. doi: 10.1016/j.jad.2006.08.021
Martin, A., Booth, J. N., Young, D., Revie, M., Boyter, A. C., Johnston, B., et al. (2016). Associations between obesity and cognition in the pre-school years. Obesity (Silver Spring). 24, 207–214. doi: 10.1002/oby.21329
Mawe, G. M., and Hoffman, J. M. (2013). Serotonin signalling in the gut–functions, dysfunctions and therapeutic targets. Nat. Rev. Gastroenterol. Hepatol. 10, 473–486. doi: 10.1038/nrgastro.2013.105
Mayeux, R., and Stern, Y. (2012). Epidemiology of Alzheimer disease. Cold Spring Harb. Perspect. Med. 2:8. doi: 10.1101/cshperspect.a006239
Mazon, J. N., de Mello, A. H., Ferreira, G. K., and Rezin, G. T. (2017). The impact of obesity on neurodegenerative diseases. Life Sci. 182, 22–28. doi: 10.1016/j.lfs.2017.06.002
McEwen, B. S. (2007). Physiology and neurobiology of stress and adaptation: central role of the brain. Physiol. Rev. 87, 873–904. doi: 10.1152/physrev.00041.2006
McNeilly, A. D., Stewart, C. A., Sutherland, C., and Balfour, D. J. (2015). High fat feeding is associated with stimulation of the hypothalamic-pituitary-adrenal axis and reduced anxiety in the rat. Psychoneuroendocrinology 52, 272–280. doi: 10.1016/j.psyneuen.2014.12.002
Mielke, J. G., Nicolitch, K., Avellaneda, V., Earlam, K., Ahuja, T., Mealing, G., et al. (2006). Longitudinal study of the effects of a high-fat diet on glucose regulation, hippocampal function, and cerebral insulin sensitivity in C57bl/6 mice. Behav. Brain Res. 175, 374–382. doi: 10.1016/j.bbr.2006.09.010
Molendijk, M., Molero, P., Ortuno Sanchez-Pedreno, F., Van der Does, W., and Angel Martinez-Gonzalez, M. (2018). Diet quality and depression risk: a systematic review and dose-response meta-analysis of prospective studies. J. Affect. Disord. 226, 346–354. doi: 10.1016/j.jad.2017.09.022
Moloney, R. D., Desbonnet, L., Clarke, G., Dinan, T. G., and Cryan, J. F. (2014). The microbiome: stress, health and disease. Mamm. Genome 25, 49–74. doi: 10.1007/s00335-013-9488-5
Morris, M. C., Evans, D. A., Bienias, J. L., Tangney, C. C., and Wilson, R. S. (2004). Dietary fat intake and 6-year cognitive change in an older biracial community population. Neurology 62, 1573–1579. doi: 10.1212/01.WNL.0000123250.82849.B6
Moya-Perez, A., Neef, A., and Sanz, Y. (2015). Bifidobacterium pseudocatenulatum Cect 7765 reduces obesity-associated inflammation by restoring the lymphocyte-macrophage balance and gut microbiota structure in high-fat diet-fed mice. PLoS ONE 10:e0126976. doi: 10.1371/journal.pone.0126976
Müller, T. D., Nogueiras, R., Andermann, M. L., Andrews, Z. B., Anker, S. D., Argente, J., et al. (2015). Ghrelin. Mol. Metab. 4, 437–460. doi: 10.1016/j.molmet.2015.03.005
Nance, D. M., and Sanders, V. M. (2007). Autonomic innervation and regulation of the immune system (1987-2007). Brain Behav. Immun. 21, 736–745. doi: 10.1016/j.bbi.2007.03.008
Nemeroff, C. B., Widerlov, E., Bissette, G., Walleus, H., Karlsson, I., Eklund, K., et al. (1984). Elevated concentrations of csf corticotropin-releasing factor-like immunoreactivity in depressed patients. Science 226, 1342–1344. doi: 10.1126/science.6334362
Nieuwenhuizen, A. G., and Rutters, F. (2008). The hypothalamic-pituitary-adrenal-axis in the regulation of energy balance. Physiol. Behav. 94, 169–177. doi: 10.1016/j.physbeh.2007.12.011
Nishino, R., Mikami, K., Takahashi, H., Tomonaga, S., Furuse, M., Hiramoto, T., et al. (2013). Commensal microbiota modulate murine behaviors in a strictly contamination-free environment confirmed by culture-based methods. Neurogastroenterol. Motil. 25, 521–528. doi: 10.1111/nmo.12110
Noble, E. E., Hsu, T. M., and Kanoski, S. E. (2017). Gut to brain dysbiosis: mechanisms linking western diet consumption, the microbiome, and cognitive impairment. Front. Behav. Neurosci. 11:9. doi: 10.3389/fnbeh.2017.00009
Nyaradi, A., Foster, J. K., Hickling, S., Li, J., Ambrosini, G. L., Jacques, A., et al. (2014). Prospective associations between dietary patterns and cognitive performance during adolescence. J. Child Psychol. Psychiatry 55, 1017–1024. doi: 10.1111/jcpp.12209
O'Mahony, S. M., Hyland, T. G., Dinan, J., and Cryan, F. (2011). Maternal separation as a model of brain–gut axis dysfunction. Psychopharmacology 214, 71–88.
Ohland, C. L., Kish, L., Bell, H., Thiesen, A., Hotte, N., Pankiv, E., et al. (2013). Effects of lactobacillus helveticus on murine behavior are dependent on diet and genotype and correlate with alterations in the gut microbiome. Psychoneuroendocrinology 38, 1738–1747. doi: 10.1016/j.psyneuen.2013.02.008
Okereke, O. I., Rosner, B. A., Kim, D. H., Kang, J. H., Cook, N. R., Manson, J. E., et al. (2012). dietary fat types and 4-year cognitive change in community-dwelling older women. Ann. Neurol. 72, 124–134. doi: 10.1002/ana.23593
Oliveira, C. D., Oliveira, C. M. D., de Macedo, I. C., Quevedo, A. S., Filho, P. R. M., Silva, F. R. D., et al. (2015). Hypercaloric diet modulates effects of chronic stress: a behavioral and biometric study on rats. Stress 18, 514–523. doi: 10.3109/10253890.2015.1079616
O'Mahony, S. M., Marchesi, J. R., Scully, P., Codling, C., Ceolho, A. M., Quigley, E. M., et al. (2009). Early life stress alters behavior, immunity, and microbiota in rats: implications for irritable bowel syndrome and psychiatric illnesses. Biol. Psychiatry 65, 263–267. doi: 10.1016/j.biopsych.2008.06.026
Opie, R. S., O'Neil, A., Jacka, F. N., Pizzinga, J., and Itsiopoulos, C. (2017). A modified mediterranean dietary intervention for adults with major depression: dietary protocol and feasibility data from the smiles trial. Nutr. Neurosci. doi: 10.1080/1028415X.2017.1312841. [Epub ahead of print].
Parletta, N., Zarnowiecki, D., Cho, J., Wilson, A., Bogomolova, S., Villani, A., et al. (2017). A mediterranean-style dietary intervention supplemented with fish oil improves diet quality and mental health in people with depression: a randomized controlled trial (HELFIMED). Nutr. Neurosci. doi: 10.1080/1028415X.2017.1411320. [Epub ahead of print].
Peng, Y., Wu, C., Yang, J., and Li, X. (2014). Gut microbial diversity in rat model induced by rhubarb. Exp. Anim. 63, 415–422. doi: 10.1538/expanim.13-0104
Pimentel, G. D., Micheletti, T. O., Pace, F., Rosa, J. C., Santos, V. T., and Lira, F. S. (2012). Gut-central nervous system axis is a target for nutritional therapies. Nutr. J. 11:22. doi: 10.1186/1475-2891-11-22
Portune, K. J., Beaumont, M., Davila, A. M., Tomé, D., Blachier, F., and Sanz, Y. (2016). Gut microbiota role in dietary protein metabolism and health-related outcomes: the two sides of the coin. Trends Food Sci. Technol. 57b, 213–232. doi: 10.1016/j.tifs.2016.08.011
Portune, K. J., Benitez-Paez, A., Del Pulgar, E. M., Cerrudo, V., and Sanz, Y. (2017). Gut microbiota, diet, and obesity-related disorders-the good, the bad, and the future challenges. Mol. Nutr. Food Res. 61:1600252. doi: 10.1002/mnfr.201600252
Qin, J., Li, R., Raes, J., Arumugam, M., Burgdorf, K. S., Manichanh, C., et al. (2010). A human gut microbial gene catalogue established by metagenomic sequencing. Nature 464, 59–65. doi: 10.1038/nature08821
Rakoff-Nahoum, S., Paglino, J., Eslami-Varzaneh, F., Edberg, S., and Medzhitov, R. (2004). Recognition of commensal Microflora by toll-like receptors is required for intestinal homeostasis. Cell 118, 229–241. doi: 10.1016/j.cell.2004.07.002
Reber, S. O. (2012). Stress and animal models of inflammatory bowel disease–an update on the role of the hypothalamo-pituitary-adrenal axis. Psychoneuroendocrinology 37, 1–19. doi: 10.1016/j.psyneuen.2011.05.014
Reichelt, A. C., Killcross, S., Hambly, L. D., Morris, M. J., and Westbrook, R. F. (2015). Impact of adolescent sucrose access on cognitive control, recognition memory, and parvalbumin immunoreactivity. Learn. Mem. 22, 215–224. doi: 10.1101/lm.038000.114
Rhee, S. H., Pothoulakis, C., and Mayer, E. A. (2009). Principles and clinical implications of the brain-gut-enteric microbiota axis. Nat. Rev. Gastroenterol. Hepatol. 6, 306–314. doi: 10.1038/nrgastro.2009.35
Rhen, T., and Cidlowski, J. A. (2005). Antiinflammatory action of glucocorticoids–new mechanisms for old drugs. N. Engl. J. Med. 353, 1711–1723. doi: 10.1056/NEJMra050541
Riva, A., Borgo, F., Lassandro, C., Verduci, E., Morace, G., and Borghi, E. (2017). Pediatric obesity is associated with an altered gut microbiota and discordant shifts in firmicutes populations. Environ. Microbiol 19, 95–105. doi: 10.1111/1462-2920.13463
Roberts, R. O., Roberts, L. A., Geda, Y. E., Cha, R. H., Pankratz, V. S., O'Connor, H. M., et al. (2012). Relative intake of macronutrients impacts risk of mild cognitive impairment or dementia. J. Alzheimers Dis. 32, 329–339. doi: 10.3233/JAD-2012-120862
Romani-Perez, M., Agusti, A., and Sanz, Y. (2017). Innovation in microbiome-based strategies for promoting metabolic health. Curr. Opin. Clin. Nutr. Metab. Care 20, 484–491. doi: 10.1097/MCO.0000000000000419
Romijn, J. A., Corssmit, E. P., Havekes, L. M., and Pijl, H. (2008). Gut-brain axis. Curr. Opin. Clin. Nutr. Metab. Care 11, 518–521. doi: 10.1097/MCO.0b013e328302c9b0
Rooks, M. G., and Garrett, W. S. (2016). Gut microbiota, metabolites and host immunity. Nat. Rev. Immunol. 16, 341–352. doi: 10.1038/nri.2016.42
Round, J. L., and Mazmanian, S. K. (2009). The gut microbiota shapes intestinal immune responses during health and disease. Nat. Rev. Immunol. 9, 313–323. doi: 10.1038/nri2515
Rubin, R. T., Phillips, J. J., McCracken, J. T., and Sadow, T. F. (1996). Adrenal gland volume in major depression: relationship to basal and stimulated pituitary-adrenal cortical axis function. Biol. Psychiatry 40, 89–97. doi: 10.1016/0006-3223(95)00358-4
Santos, J., Saperas, E., Nogueiras, C., Mourelle, M., Antolín, M., Cadahia, A., et al. (1998). Release of mast cell mediators into the jejunum by cold pain stress in humans. Gastroenterology 114, 640–648. doi: 10.1016/S0016-5085(98)70577-3
Santos, R. O., de Assunção, G. L., de Medeiros, D. M., de Sousa Pinto, I. A., de Barros, K. S., Soares, B. L., et al. (2014). Evaluation of the effect of acute sibutramine in female rats in the elevated t-maze and elevated plus-maze tests. Basic Clin. Pharmacol. Toxicol. 114, 181–187. doi: 10.1111/bcpt.12131
Santos, R. O., Trindade, S. C., Maurer, L. H., Bersch, A. M., Sautter, C. K., and Penna, N. G. (2016). Physicochemical, antioxidant and sensory quality of brazilian blueberry wine. An. Acad. Bras. Cienc. 88, 1557–1568. doi: 10.1590/0001-3765201620140491
Sanz, Y., and Moya-Perez, A. (2014). Microbiota, inflammation and obesity. Adv. Exp. Med. Biol. 817, 291–317. doi: 10.1007/978-1-4939-0897-4_14
Sapolsky, R., Rivier, C., Yamamoto, G., Plotsky, P., and Vale, W. (1987). Interleukin-1 stimulates the secretion of hypothalamic corticotropin-releasing factor. Science 238, 522–524. doi: 10.1126/science.2821621
Saulnier, D. M., Ringel, Y., Heyman, M. B., Foster, J. A., Bercik, P., Shulman, R. J., et al. (2013). The intestinal microbiome, probiotics and prebiotics in neurogastroenterology. Gut Microb. 4, 17–27. doi: 10.4161/gmic.22973
Schachter, J., Martel, J., Lin, C. S., Chang, C. J., Wu, T. R., Lu, C. C., et al. (2017). Effects of obesity on depression: a role for inflammation and the gut microbiota. Brain Behav. Immun. doi: 10.1016/j.bbi.2017.08.026. [Epub ahead of print].
Schneeberger, M., Everard, A., Gomez-Valades, A. G., Matamoros, S., Ramirez, S., Delzenne, N. M., et al. (2015). Akkermansia muciniphila inversely correlates with the onset of inflammation, altered adipose tissue metabolism and metabolic disorders during obesity in mice. Sci. Rep. 5:16643. doi: 10.1038/srep16643
Schwartz, M. W., Woods, S. C., Porte, D. Jr., Seeley, R. J., and Baskin, D. G. (2000). Central nervous system control of food intake. Nature 404, 661–671. doi: 10.1038/35007534
Shadnoush, M., Shaker Hosseini, R., Mehrabi, Y., Delpisheh, A., Alipoor, E., Faghfoori, Z., et al. (2013). Probiotic yogurt affects pro- and anti-inflammatory factors in patients with inflammatory bowel disease. Iran. J. Pharm. Res. 12, 929–936.
Singh, R. K., Chang, H. W., Yan, D., Lee, K. M., Ucmak, D., Wong, K., et al. (2017). Influence of diet on the gut microbiome and implications for human health. J. Transl. Med. 15:73. doi: 10.1186/s12967-017-1175-y
Sinha, R. (2008). Chronic stress, drug use, and vulnerability to addiction. Ann. N. Y. Acad. Sci. 1141, 105–130. doi: 10.1196/annals.1441.030
Sinha, R., and Jastreboff, A. M. (2013). Stress as a common risk factor for obesity and addiction. Biol. Psychiatry. 73, 827–835. doi: 10.1016/j.biopsych.2013.01.032
Slyepchenko, A., Maes, M., Jacka, F. N., Köhler, C. A., Barichello, T., McIntyre, R. S., et al. (2017). Gut microbiota, bacterial translocation, and interactions with diet: pathophysiological links between major depressive disorder and non-communicable medical comorbidities. Psychother. Psychosom. 86, 31–46. doi: 10.1159/000448957
Söderholm, J. D., Yates, D. A., Gareau, M. G., Yang, P. C., MacQueen, G., and Perdue, M. H. (2002). Neonatal maternal separation predisposes adult rats to colonic barrier dysfunction in response to mild stress. Am. J. Physiol. Gastrointest Liver Physiol. 283, G1257–G1263. doi: 10.1152/ajpgi.00314.2002
Solfrizzi, V., Scafato, E., Capurso, C., D'Introno, A., Colacicco, A. M., Frisardi, V., et al. (2011). Metabolic syndrome, mild cognitive impairment, and progression to dementia. The italian longitudinal study on aging. Neurobiol Aging 32, 1932–1941. doi: 10.1016/j.neurobiolaging.2009.12.012
Spear, L. P. (2000). The adolescent brain and age-related behavioral manifestations. Neurosci. Biobehav. Rev. 24, 417–463. doi: 10.1016/S0149-7634(00)00014-2
Spiller, R. (2008). Serotonin and Gi clinical disorders. Neuropharmacology 55, 1072–1080. doi: 10.1016/j.neuropharm.2008.07.016
Steenbergen, L., Sellaro, R., van Hemert, S., Bosch, J. A., and Colzato, L. S. (2015). A randomized controlled trial to test the effect of multispecies probiotics on cognitive reactivity to sad mood. Brain Behav. Immun. 48, 258–264. doi: 10.1016/j.bbi.2015.04.003
Stephens, R. L., and Tache, Y. (1989). Intracisternal injection of a Trh analogue stimulates gastric luminal serotonin release in rats. Am. J. Physiol. 256(Pt 1), G377–G3783. doi: 10.1152/ajpgi.1989.256.2.G377
Sudo, N., Chida, Y., Aiba, Y., Sonoda, J., Oyama, N., Yu, X. N., et al. (2004). Postnatal microbial colonization programs the hypothalamic-pituitary-adrenal system for stress response in mice. J. Physiol. 558(Pt 1), 263–275. doi: 10.1113/jphysiol.2004.063388
Takase, K., Tsuneoka, Y., Oda, S., Kuroda, M., and Funato, H. (2016). High-fat diet feeding alters olfactory-, social-, and reward-related behaviors of mice independent of obesity. Obesity 24, 886–894. doi: 10.1002/oby.21441
Tillisch, K., Labus, J., Kilpatrick, L., Jiang, Z., Stains, J., Ebrat, B., et al. (2013). Consumption of fermented milk product with probiotic modulates brain activity. Gastroenterology 144, 1394–1401. doi: 10.1053/j.gastro.2013.02.043
Tomkin, G. H., and Owens, D. (2016). Obesity diabetes and the role of bile acids in metabolism. J. Transl. Int. Med. 4, 73–80. doi: 10.1515/jtim-2016-0018
Topping, D. L., and Clifton, P. M. (2001). Short-chain fatty acids and human colonic function: roles of resistant starch and nonstarch polysaccharides. Physiol. Rev. 81, 1031–1064. doi: 10.1152/physrev.2001.81.3.1031
Torres, S. J., and Nowson, C. A. (2007). Relationship between stress, eating, behavior and obesity. Nutrition 23, 887–894. doi: 10.1016/j.nut.2007.08.008
Torres-Fuentes, C., Schellekens, H., Dinan, T. G., and Cryan, J. F. (2017). The microbiota-gut-brain axis in obesity. Lancet Gastroenterol. Hepatol. 2, 747–756. doi: 10.1016/S2468-1253(17)30147-4
Tsigos, C., and Chrousos, G. P. (2002). Hypothalamic-pituitary-adrenal axis, neuroendocrine factors and stress. J. Psychosom. Res. 53, 865–871. doi: 10.1016/S0022-3999(02)00429-4
Underwood, E. L., and Thompson, L. T. (2016). A high-fat diet causes impairment in hippocampal memory and sex-dependent alterations in peripheral metabolism. Neural Plast. 2016:7385314. doi: 10.1155/2016/7385314
Valentino, M. A., Lin, J. E., Snook, A. E., Li, P., Kim, G. W., Marszalowicz, G., et al. (2011). A uroguanylin-Gucy2c endocrine axis regulates feeding in mice. J. Clin. Invest. 121, 3578–3588. doi: 10.1172/JCI57925
Val-Laillet, D., Besson, M., Guérin, S., Coquery, N., Randuineau, G., Kanzari, A., et al. (2017). A maternal western diet during gestation and lactation modifies offspring's microbiota activity, blood lipid levels, cognitive responses, and hippocampal neurogenesis in yucatan pigs. FASEB J. 31, 2037–2049. doi: 10.1096/fj.201601015R
Vermeulen, E., Stronks, K., Snijder, M. B., Schene, A. H., Lok, A., de Vries, J. H., et al. (2017). A combined high-sugar and high-saturated-fat dietary pattern is associated with more depressive symptoms in a multi-ethnic population: the helius (healthy life in an urban setting) study. Public Health Nutr. 20, 2374–2382. doi: 10.1017/S1368980017001550
Wang, D., Yan, J., Chen, J., Wu, W., Zhu, X., and Wang, Y. (2015). Naringin improves neuronal insulin signaling, brain mitochondrial function, and cognitive function in high-fat diet-induced obese mice. Cell. Mol. Neurobiol. 35, 1061–1071. doi: 10.1007/s10571-015-0201-y
Wang, S., Huang, X. F., Zhang, P., Newell, K. A., Wang, H., Zheng, K., et al. (2017). Dietary teasaponin ameliorates alteration of gut microbiota and cognitive decline in diet-induced obese mice. Sci. Rep. 7:12203. doi: 10.1038/s41598-017-12156-2
Wang, S., Huang, X. F., Zhang, P., Wang, H., Zhang, Q., Yu, S., et al. (2016). Chronic rhein treatment improves recognition memory in high-fat diet-induced obese male mice. J. Nutr. Biochem. 36, 42–50. doi: 10.1016/j.jnutbio.2016.07.008
Wang, Y., and Kasper, L. H. (2014). The role of microbiome in central nervous system disorders. Brain Behav. Immun. 38, 1–12. doi: 10.1016/j.bbi.2013.12.015
Warne, J. P. (2009). Shaping the stress response: interplay of palatable food choices, glucocorticoids, insulin and abdominal obesity. Mol. Cell. Endocrinol. 300, 137–146. doi: 10.1016/j.mce.2008.09.036
Wei, Y. B., Melas, P. A., Wegener, G., Mathé, A. A., and Lavebratt, C. (2015). Antidepressant-like effect of sodium butyrate is associated with an increase in tet1 and in 5-hydroxy-methylation levels in the Bdnf gene. Intern. J. Neuropsychopharmacol. 18:pyu032. doi: 10.1093/ijnp/pyu032
Whitmer, R. A., Gunderson, E. P., Quesenberry, C. P. Jr., Zhou, J., and Yaffe, K. (2007). Body mass index in midlife and risk of Alzheimer disease and vascular dementia. Curr. Alzheimer Res. 4, 103–109. doi: 10.2174/156720507780362047
Wirt, T., Schreiber, A., Kesztyüs, D., and Steinacker, J. M. (2015). Early life cognitive abilities and body weight: cross-sectional study of the association of inhibitory control, cognitive flexibility, and sustained attention with bmi percentiles in primary school children. J. Obes. 2015:534651. doi: 10.1155/2015/534651
Wischmeyer, P. E. (2006). Glutamine: role in gut protection in critical illness. Curr. Opin. Clin. Nutr. Metab. Care 9, 607–612. doi: 10.1097/01.mco.0000241672.09676.03
Wood, J. D., Alpers, D. H., and Andrews, P. L. (1999). Fundamentals of neurogastroenterology. Gut 45(Suppl. 2), II6–II16. doi: 10.1136/gut.45.2008.ii6
Woods, S. C., and D'Alessio, D. A. (2008). Central control of body weight and appetite. J. Clin. Endocrinol. Metab. 93(Suppl. 1), S37–S50. doi: 10.1210/jc.2008-1630
World Health Organization. (2017). Depression and Other Common Mental Disorders: Global Health Estimates. Geneva.
Xu, J., Mahowald, M. A., Ley, R. E., Lozupone, C. A., Hamady, M., Martens, E. C., et al. (2007). Evolution of symbiotic bacteria in the distal human intestine. PLoS Biol. 5:e156. doi: 10.1371/journal.pbio.0050156
Yang, H., Stephens, R. L., and Taché, Y. (1992). Trh analogue microinjected into specific medullary nuclei stimulates gastric serotonin secretion in rats. Am. J. Physiol. 262(2 Pt 1), G216–G222. doi: 10.1152/ajpgi.1992.262.2.G216
Yang, J. L., De Xiang Liu, H. J., Pan, F., Ho, C. S., and Ho, R. C. (2016). The effects of high-fat-diet combined with chronic unpredictable mild stress on depression-like behavior and Leptin/Leprb in male rats. Sci. Rep. 6:35239. doi: 10.1038/srep35239
Yano, J. M., Yu, K., Donaldson, G. P., Shastri, G. G., Ann, P., Ma, L., et al. (2015). Indigenous bacteria from the gut microbiota regulate host serotonin biosynthesis. Cell 161, 264–276. doi: 10.1016/j.cell.2015.02.047
Zen, M., Canova, M., Campana, C., Bettio, S., Nalotto, L., Rampudda, M., et al. (2011). The kaleidoscope of glucorticoid effects on immune system. Autoimmun. Rev. 10, 305–310. doi: 10.1016/j.autrev.2010.11.009
Keywords: microbiota, cognition, mood, behavior, obesity
Citation: Agustí A, García-Pardo MP, López-Almela I, Campillo I, Maes M, Romaní-Pérez M and Sanz Y (2018) Interplay Between the Gut-Brain Axis, Obesity and Cognitive Function. Front. Neurosci. 12:155. doi: 10.3389/fnins.2018.00155
Received: 10 November 2017; Accepted: 26 February 2018;
Published: 16 March 2018.
Edited by:
Antonio Benítez-Burraco, Universidad de Sevilla, SpainReviewed by:
Francesco Marotta, ReGenera Research Group, ItalyMaite Solas, Universidad de Navarra, Spain
Cristina Torres Fuentes, University of California, Davis, United States
Copyright © 2018 Agustí, García-Pardo, López-Almela, Campillo, Maes, Romaní-Pérez and Sanz. This is an open-access article distributed under the terms of the Creative Commons Attribution License (CC BY). The use, distribution or reproduction in other forums is permitted, provided the original author(s) and the copyright owner are credited and that the original publication in this journal is cited, in accordance with accepted academic practice. No use, distribution or reproduction is permitted which does not comply with these terms.
*Correspondence: Ana Agustí, aagusti@iata.csic.es