- 1Departamento de Fisiología (Fisiología Animal II), Facultad de Biología, Universidad Complutense de Madrid, Madrid, Spain
- 2Departamento de Fisiología de Peces y Biotecnología, Instituto de Acuicultura Torre de la Sal, Consejo Superior de Investigaciones Científicas, Castellón, Spain
- 3Laboratorio de Fisioloxía Animal, Departamento de Bioloxía Funcional e Ciencias da Saúde, Facultade de Bioloxía, Universidade de Vigo, Vigo, Spain
The regulation of food intake in fish is a complex process carried out through several different mechanisms in the central nervous system (CNS) with hypothalamus being the main regulatory center. As in mammals, a complex hypothalamic circuit including two populations of neurons: one co-expressing neuropeptide Y (NPY) and Agouti-related peptide (AgRP) and the second one population co-expressing pro-opiomelanocortin (POMC) and cocaine- and amphetamine-regulated transcript (CART) is involved in the integration of information relating to food intake control. The production and release of these peptides control food intake, and the production results from the integration of information of different nature such as levels of nutrients and hormones as well as circadian signals. The present review summarizes the knowledge and recent findings about the presence and functioning of these mechanisms in fish and their differences vs. the known mammalian model.
Introduction
Food intake is regulated through positive and negative loops acting at different locations and at different times (Langhans and Scharrer, 1992; Langhans, 1999). The positive loop (starting of food intake) results from the relationship among prior experience with nutrient availability, status of the animal and sensory qualities of the food. The negative loop relates to metabolic and gastrointestinal inputs displaying changes prior and after absorption (Langhans, 1999). Three regulatory levels have been suggested following this model: (i) short-term regulatory factors: those influenced by the size of a single meal, (ii) mid-term regulatory factors: those operating through several days; and (iii) long-term regulatory factors: those operating through longer time periods (weeks, months, and years) reflecting energy balance of the animal. These levels of regulation create a feedback loop that continuously modulate the immediate signal input from sense organs and internal sensors, which together with prior learning integrate information from the energy reserves of the body. The control determining the feeding behavior and food intake is elicited by the central nervous system (CNS) through various pathways, and hypothalamus is the main center involved in such regulation (Schwartz et al., 2000; Berthoud, 2002).
In fish is difficult to establish which factors act in the mid- or long-term, since most available data come from short-term studies. The basic mechanisms involved in regulation of food intake in fish appear to be similar to those of mammals with differences implying the existence of specific mechanisms in fish (Kulczykowska and Sánchez-Vázquez, 2010; Hoskins and Volkoff, 2012; Volkoff, 2016). In the following sections, we review the existing knowledge about hypothalamic integration in fish of metabolic, endocrine, and circadian information to elicit a coordinated feeding response, as summarized in Figure 1.
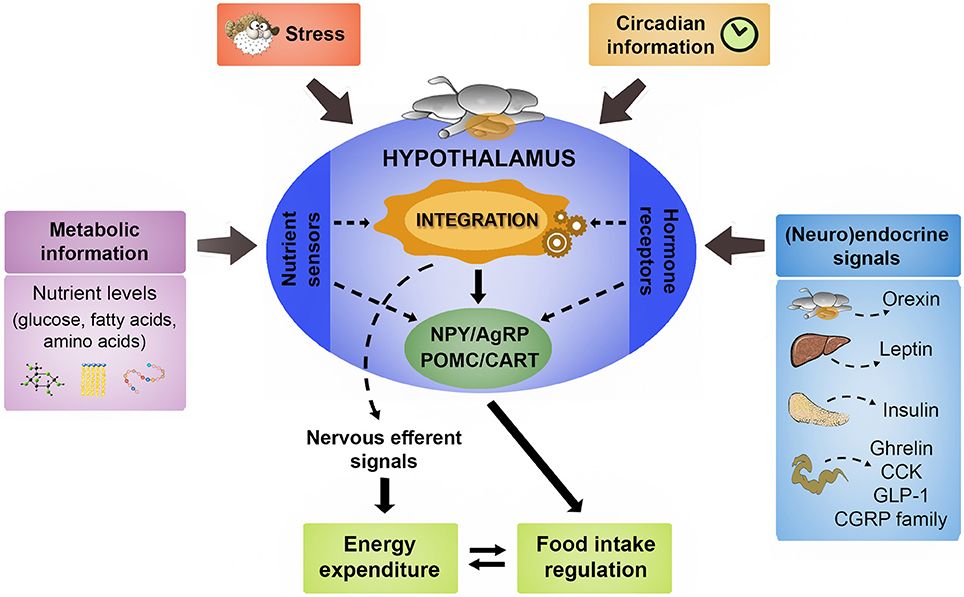
Figure 1. Schematic representation illustrating the main signaling elements involved in the hypothalamic control of food intake in fish. A comprehensive set of central and peripheral neuroendocrine messengers in a coordinated way with the metabolic information provided by nutrient levels constitute the main signaling that regulates hypothalamic neurocircuits involved in the regulation of food intake. The detailed description of such main functional neuropeptidergic circuits is shown in the text. The hypothalamus also integrates the stress response in the generation of the adaptive changes in food intake and energy expenditure according to the exposure to stressful conditions. Finally, the role of hypothalamus in the internal coordination of circadian rhythmicity related to feeding behavior is also described in the text. AgRP, agouti-related peptide; CART, cocaine- and amphetamine-related transcript; CCK, cholecystokinin; CGRP, calcitonin gene-related peptide; GLP-1, glucagon-like peptide 1; POMC, pro-opiomelanocortin; NPY, neuropeptide Y.
Hypothalamic Neuropeptides Involved in the Control of Food Intake
The integration of information involved in the control of food intake takes place in mammals through a circuit mainly localized in hypothalamic areas including arcuate (ARC), ventromedial, paraventricular, and lateral hypothalamus (Berthoud and Morrison, 2008; Zheng and Berthoud, 2008). These circuits include two populations of neurons (Mobbs et al., 2005; Blouet and Schwartz, 2010; Waterson and Horvath, 2015). The first population co-expresses neuropeptide Y (NPY) and agouti-related peptide (AgRP) and the second population co-expresses pro-opiomelanocortin (POMC) and cocaine- and amphetamine-regulated transcript (CART). Moreover, these two populations of neurons inhibit each other resulting in signaling to other higher-order neurons. The production and release of these peptides control food intake, and this occurs through integration of signals of metabolic, endocrine, and circadian nature. Other peptides such as orexins are involved in food intake modulation but they are not involved in this main integrative core.
Avaliable studies in fish (mostly through asssessment of mRNA abundance) demonstrated expression of these neuropeptides in hypothalamus. Neurons expressing NPY, AgRP, CART, and POMC in fish respond to energy challenge suggesting that the main hypothalamic pathways coordinating a response to regulate energy homeostasis are conserved throughout vertebrate evolution (Volkoff et al., 2005).
CART/POMC
Melanocortins integrate a key system in the regulation of food intake in vertebrates. These peptides, exhibiting melanotropic (melanin stimulation) and corticotropic (corticosteroid stimulation) activity encoded in the common precursor POMC. Two discrete groups of neurons in ARC and the caudal region of the nucleus of the tractus solitarius of the medulla produce POMC, mainly processed into α-melanocyte-stimulating hormone (α-MSH) and β-endorphin. Melanocortin signaling is transduced by five different G-coupled receptors (MC1R-MC5R) but only two are conspicuously expressed in the mammalian brain, i.e., MC3R and MC4R. Central activation of these receptors appears to mediate melanocortin effects on energy balance since both MC3R knockout rat and MC4R knockout mice display severe alterations in energy homeostasis (obesity, increased food intake and linear growth in MC4R; hypophagia, increased fat mass and food efficiency but reduced lean mass in MC3R). Accordingly, central administration of MC3/4Rs agonists results in a dose-dependent reduction in food intake in mice but MC4R-deficient mice do not respond to anorectic effects of agonists, suggesting that α-MSH inhibits feeding primarily by activating MC4R (reviewed by Anderson et al., 2016).
Several studies demonstrated that melanocortin system is a key point in the regulation of energy balance in fish (Cerdá-Reverter et al., 2011). Thus, in situ hybridization studies demonstrated the presence of POMC neurons in hypothalamus (Cerdá-Reverter et al., 2003a) but not in the vagal lobe (homolog of the nucleus of the tractus solitarius in mammalian brain). However, more sensitive techniques (quantitative PCR) also reported POMC-A1 expression in the hindbrain (Conde-Sieira et al., 2010b). Immunohistochemical techniques further demonstrated the presence of both α-MSH and β-endorphin in the hypothalamus of several fish species, including zebrafish and tilapia (Forlano and Cone, 2007; Chabbi and Ganesh, 2016) thus suggesting that POMC is mainly processed to these peptides. However, ACTH is also present in the preoptic area of carp (Metz et al., 1994) further suggesting an alternative POMC processing in the fish brain.
Intracerebroventricular (ICV) administration of α-MSH or α-MSH agonists inhibit food intake in a dose-dependent manner in goldfish (Cerdá-Reverter et al., 2003b) and rainbow trout (Schjolden et al., 2009), whereas administration of MC4R antagonists stimulates food intake in satiated animals (Cerdá-Reverter et al., 2003b). POMC-A1 and POMC-C levels increased post-feeding in medaka (Chisada et al., 2014), rainbow trout (Gong and Björnsson, 2014), and Atlantic halibut (Gomes et al., 2015), respectively. In contrast to that expected for a negative regulator of energy balance, POMC hypothalamic levels remained unchanged after 7 progressive fasting days in goldfish (Cerdá-Reverter et al., 2003a), but POMC-A1 mRNA decreased 50% after 28 fasting days in rainbow trout (Leder and Silverstein, 2006). Surprisingly, another study demonstrated that hypothalamic POMC A1, A2 and B levels increased after 118 fasting days in the latter species (Jørgensen et al., 2016). The lack of a consistent POMC regulation by energy balance also occurred in mammalian species. Thus, sheep with about 40% of total body-weight loss did not exhibit changes in POMC mRNA levels (Henry et al., 2001). A similar situation occurred in ewes that lost about 30% body fat (Henry et al., 2000). The constrasting effects of POMC-encoded peptides could explain the paradoxical absence of POMC regulation by energy balance. Thus, central injections of β-endorphin, the C-terminal peptide of POMC, stimulate appetite in the goldfish (De Pedro et al., 1995). It is then plausible that the effects of nutritional status might regulate post-transcriptional POMC-derived peptide levels by selective regulation of prohormone convertases. Under negative energy balance situations, when animals display an enhanced feeding drive, POMC might be processed into β-endorphin. In contrast, a positive energy balance may preferentially drive the processing of POMC into production of the food intake inhibitor α-MSH.
The melanocortin activity may be also modulated by receptor signaling. Accordingly, energy balance, in the absence of an agonist regulation, could up/down regulate the neuronal receptor density in feeding-related areas of the CNS. Studies in the sea bass suggest that hypothalamic MC4R expression remains unchanged after long-term fasting precluding this regulatory pathway (Sánchez et al., 2009). Alternatively, the melanocortin system also exhibits endogenous antagonists competing by binding and activation of the melanocortin receptors. AgRP1 is consistently upregulated by fasting in the hypothalamus of all tested vertebrates including zebrafish (Song et al., 2003), goldfish (Cerdá-Reverter and Peter, 2003), Atlantic salmon (Valen et al., 2011), and sea bass (Agulleiro et al., 2014). It is therefore conceivable that AgRP1 binding to central MCRs might regulate central melanocortinergic activity whereas POMC expression remains constant as a constitutive inhibitor.
In mammals, ARC POMC neurons also produce CART but hypothalamus is not the only brain area producing this neuropeptide (Elias et al., 1998). CART was isolated form ovine hypothalamus (Spiess et al., 1981) and its expression increased in the rat striatum after the administration of drugs such as cocaine and amphetamine (Douglass et al., 1995). Subsequent experiments revealed that CART neurons are anatomical targets for systemic leptin to induce anorexia (Kristensen et al., 1998). In fish, CART mRNA was characterized in several species (Subhedar et al., 2014) but immunohistochemical localization of the CART peptide in brain was only studied in catfish by using antibodies against rat CART (Singru et al., 2007) or by in situ hybridization in zebrafish (Nishio et al., 2012; Akash et al., 2014). CART mRNA abundance decreased with food deprivation in cod (Kehoe and Volkoff, 2007), goldfish (Volkoff and Peter, 2001a), and Atlantic salmon (Murashita et al., 2009), and increased with re-feeding in channel catfish (Kobayashi et al., 2008) whereas post-prandial changes occurred in channel catfish (Peterson et al., 2012), goldfish (Volkoff and Peter, 2001a), and dourado (Volkoff et al., 2016). As many other genes, CART is duplicated in the teleost genome and four different genes (CART1-4 or CART1, 2a, 2b, 4) encoding CART peptides are reported in zebrafish (Nishio et al., 2012) or up to seven genes in Senegalese sole (Bonacic et al., 2015). All of them are expressed in zebrafish CNS but only CART2 and 4 are produced in the hypothalamus (Akash et al., 2014). Unfortunately, CART/POMC colocalization in the neurons of the tuberal hypothalamus has not been assessed in fish yet. Both CART2 and CART4 are downregulated by fasting in ventrocaudal telencephalon (CART2) and ventral hypothalamus (CART2 and CART4) (Akash et al., 2014), as expected for a negative regulator of the energy balance and thus further suggesting their involvement in the regulation of energy balance in fish. Accordingly, ICV administration of CART peptides inhibit food intake in goldfish (Volkoff and Peter, 2000).
AgRP/NPY
Atypically, melanocortin signaling is not exclusively regulated by the binding of endogenous agonists (see above), since naturally occurring antagonists, agouti-signaling protein (ASIP) and AgRP, compete with melanocortin peptides by binding to MCRs. ASIP and AgRP molecules exhibit a cysteine-rich C-terminal domain essential for the structural and biological properties of the peptide. A basic domain and a proline-rich area precede the cysteine knot in ASIP; AgRP sequences lacks both regions but, in contrast, exhibits a processing site prior to the cysteine domain where the propeptide is cleaved (reviewed by Cerdá-Reverter et al., 2011). In mammalian species, ASIP produced in the ventral skin regulates pigment pattern and binds MC1R and MC4R with similar affinity (Cerdá-Reverter et al., 2005). In contrast, AgRP is mainly expressed in the same NPY-producing neurons in the ARC. In fact, 95% of NPY neurons co-express AgRP (Hahn et al., 1998). AgRP/NPY neurons also produce GABA (Horvath et al., 1997) and regulate the activity of hypothalamic POMC/CART neurons. In addition, the GABAergic innervation from AgRP/NPY neurons to the parabrachial nucleus in the brainstem is able to regulate feeding behavior (Wu et al., 2009). Hypothalamic AgRP sharply increases with fasting, and overexpression in transgenic mice induces overfeeding and obesity but also enhances linear growth (Ollmann et al., 1997). The selective ablation of NPY/AgRP neurons induces a rapid decrease in food intake leading to starvation (Luquet et al., 2005).
Fish exhibit two copies of AgRP named AgRP1 and AgRP2. Few studies to date have precisely localized AgRP1 mRNA in brain regions of teleost fish (Cerdá-Reverter and Peter, 2003; Song and Cone, 2007) whereas others reported AgRP-ir in fish brain (Forlano and Cone, 2007; Agulleiro et al., 2014). All studies identified AgRP1 production within the posterior region of the ventral hypothalamus of the goldfish, zebrafish and sea bass. In the zebrafish, AgRP1 and α-MSH projections strikingly match the nuclei that express MC4R (Cerdá-Reverter et al., 2003b) and MC5R mRNA (Cerdá-Reverter et al., 2003c) in the goldfish. In fact, binding studies with zebrafish receptors demonstrated that AgRP1 acts as a competitive antagonist at MC3R, MC4R, and MC5R, all of them expressed in the brain (Song and Cone, 2007). Pharmacological studies in sea bass have shown that AgRP1 works as an inverse agonist at constitutively activated MC4R (Sánchez et al., 2009). In addition, ASIP1 overexpression working at central MC4R in transgenic zebrafish model results in enhanced feeding, feed efficiency, weight and linear growth but not in obesity (Guillot et al., 2016), in a similar way to the AgRP1 transgenic zebrafish (Song and Cone, 2007). Studies in zebrafish using first developmental stages demonstrated that AgRP1 knockdown results in reduced growth suggesting that AgRP1 suppression of MC4R activity is essential for larval growth (Zhang et al., 2012). Therefore, melanocortin system seems to impose a constitutive break to feeding and growth in fish probably through constitutive activity of MC4R. AgRP1 overexpression is the soundest response to fasting reported in fish, and its hypothalamic expression is essential to counteract MC4R negative effects on energy balance by inverse agonism on the receptor thus driving fish to feeding and concomitantly enhancing fish growth both dependently and independently on feeding levels (Guillot et al., 2016). In fact, AgRP1 mRNA abundance in hypothalamus increased in food deprived zebrafish (Song et al., 2003), goldfish (Cerdá-Reverter and Peter, 2003), sea bass (Agulleiro et al., 2013), and carp (Zhong et al., 2013) but not in Atlantic salmon (Murashita et al., 2009). Post-feeding did not induce changes in AgRP1 hypothalamic mRNA abundance in medaka (Chisada et al., 2014), but the increase in food intake in the GH-transgenic carp is associated with increased values of AgRP1 mRNA (Zhong et al., 2013).
There are no studies on NPY/AgRP colocalization in hypothalamic neurons in fish brain. The NPY family of peptides consists of 36-amino-acid peptides exhibiting carboxy terminal (C-terminal) amidation (Cerdá-Reverter and Larhammar, 2000). The family comprises three different peptides, the NPY, tyrosine-tyrosine peptide, (PYY), and the pancreatic polypeptide (PP). Tetrapod species produce all three peptides, whereas non-tetrapod vertebrates have only NPY and PYY. Teleost fish synthesize two different versions of NPY and PYY but not PP (Sundström et al., 2008). Brain distribution of NPY peptides was reported in detail only in the sea bass by in situ hybridization (Cerdá-Reverter et al., 2000a,b), and only NPY1 expressed in the rostral hypothalamus, where the AgRP1 neurons are localized in this species. Concomitant coexpression in the rostral hypothalamus suggests that both NPY1 and AgRP1 colocalize also in the tuberal hypothalamus, ventral telencephalon and preoptic area of fish.
NPY is the most potent orexigenic factor in vertebrates (Stanley and Leibowitz, 1985) and many studies shown this effect after ICV administration in several fish (López-Patiño et al., 1999; Aldegunde and Mancebo, 2006; Kiris et al., 2007; Yokobori et al., 2012). The mRNA abundance of NPY decreased after meal in goldfish (Kehoe and Volkoff, 2007), zebrafish (Tian et al., 2015), and grass carp (Zhou et al., 2013), though responses were contradictory in other species, such as orange-spotted grouper (Tang et al., 2013), rainbow trout (Gong and Björnsson, 2014), and zebrafish (Chen et al., 2016). Food deprivation in rainbow trout also resulted in decreased mRNA abundance of NPY (Gong et al., 2016b) but also after NPY intraperitoneal (IP) injections in flounder (Li et al., 2016). Accordingly, brain expression is upregulated by fasting in several fish species (Matsuda et al., 2012b) but the number of NPY immunoreactive neurons increase in the hypothalamus and posterior tuberculum after seven days fasting in the goldfish but not in the thalamus (Yokobori et al., 2012). Peripheral leptin is also able to regulate hypothalamic NPY expression since IP injection of recombinant leptin inhibits AgRP1/NPY but upregulates POMC/CART expression in goldfish (Yan et al., 2016). In addition, NPY was suggested to link the reproductive system and the central circuitry regulating energy balance in sea bass (Cerdá-Reverter et al., 1999).
Hypothalamic Integration of Metabolic Information
The detection of nutrient levels in hypothalamus modulates different neurocircuits related to food intake regulation, metabolite homeostasis, energy expenditure, and status of body reserves (Morton et al., 2006; Blouet and Schwartz, 2010). The neurons coexpressing NPY/AgRP or POMC/CART are included in these circuits, and respond with decreased or increased peptide expression, respectively, to rises in circulating levels of glucose, fatty acids, or amino acids (Mobbs et al., 2005; Blouet and Schwartz, 2010; Efeyan et al., 2015). Thus, POMC/CART neurons depolarize while NPY/AgRP hyperpolarize in response to the increase in nutrient levels (Levin et al., 2004; Fioramonti et al., 2007).
The sensing of a particular nutrient may involve the direct binding of the sensed molecule to the sensor, or occur by an indirect mechanism relying on the detection of a related molecule that reflects nutrient abundance (Efeyan et al., 2015). Different organisms detect extracellular and intracellular levels of sugars, amino acids, and fatty acids. We provide a picture of the current knowledge of the systems already characterized in fish, i.e., those involved in the sensing of glucose and fatty acids (Soengas, 2014).
As for amino acids, in mammals the increase in the levels of branched-chain amino acids (BCAA) such as leucine result in decreased food intake. This effect is mediated by activation of central amino acid sensing through changes in target of rapamycin (mTOR) and/or AMP-activated protein-kinase (AMPK), and BCAA metabolism (Heeley and Blouet, 2016; Morrison et al., 2016). Furthermore, the deficiency in essential amino acids elicit an increase in food intake through central amino acids sensors mediated by general control non-depressable 2 (GCN2) and eukaryotic initiation factor 2α (eiF2α) (Fromentin et al., 2012; Maurin et al., 2014). Considering that most fish are carnivorous (strongly dependent on dietary protein/amino acid), the presence of amino acid sensors in central areas involved in the regulation of food intake like hypothalamus is likely. However, no studies are available in fish about the presence and functioning in central areas of amino acid sensors. The only available information relates to the effect of different protein levels and/or composition on food intake with contradictory responses observed (Figueiredo-Silva et al., 2012b; Wacyk et al., 2012; Tan et al., 2016).
A summary of the main findings achieved as well as the hypothetical pathways involved in nutrient sensing in fish hypothalamus is shown in Figure 2.
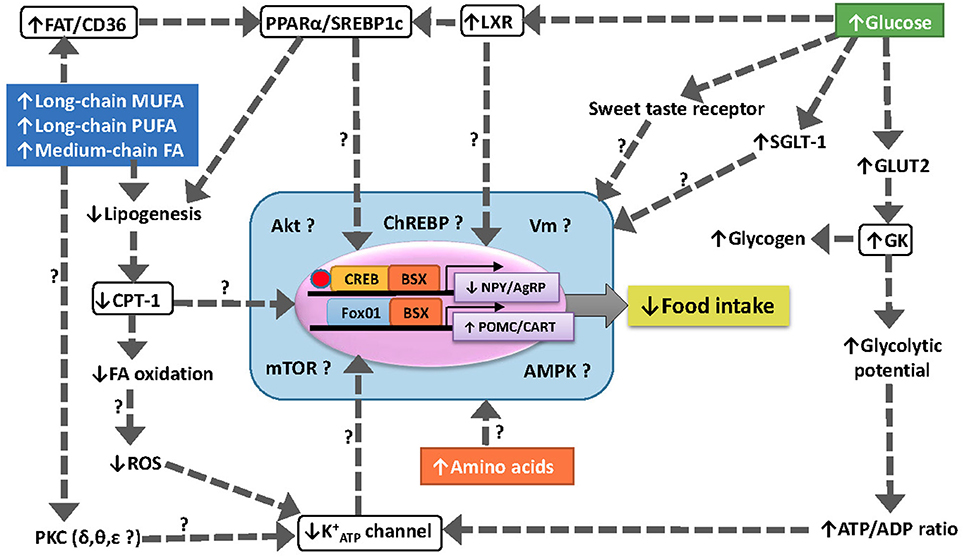
Figure 2. Schematic drawing with a hypothetical model of food intake regulation through different nutrient sensing mechanisms in fish hypothalamus. The increase in levels of specific fatty acids is sensed through several mechanisms including (1) inhibition of CPT-1 to import FA-CoA into the mitochondria for oxidation, (2) increased binding to FAT/CD36 resulting in the modulation of PPARα and SREBP1c, (3) the translocation and activation of specific isoforms of PKC, and (4) the enhanced ROS production in mitochondria by electron leakage. The increase in levels of glucose is sensed through increased GK activity, activation of LXR and modulation o sweet taste receptors. The integration of different sensing mechanisms result in changes in the expression of neuropeptides controlling food intake (NPY/AgRP and CART/POMC) through unknown mechanisms.↑, increase; ↓, decrease; ?, unknown; AgRP, agouti-related peptide; Akt, protein kinase B; AMPK, AMP-activated protein kinase; BSX, brain homeobox transcription factor; CART, cocaine- and amphetamine-related transcript; ChREBP, carbohydrate-responsive element-binding protein; CPT-1, carnitine palmitoyl transferase type 1; CREB, cAMP response-element binding protein FA, fatty acid; FAT/CD36, fatty acid translocase; FoxO1, forkhead box protein O1; K+ATP, inward rectifier ATP-dependent K+ channel; GK, glucokinase (hexokinase IV); GLUT2, facilitative glucose carrier type 2; LXR, liver X receptor; mTOR, target of rapamycin; MUFA, monounsaturated fatty acid; NPY, neuropeptide Y; POMC, pro-opio melanocortin; PPARα, peroxisome proliferator-activated receptor type α; PUFA, polyunsaturated fatty acid; SGLT-1, sodium/glucose co-transporter 1; SREBP1c, sterol regulatory element-binding protein type 1c; PKC, protein kinase C; ROS, reactive oxygen species; Vm, membrane potential.
Glucosensors and Control of Food Intake
Glucosensing Mechanisms
Hypothalamic POMC/CART neurons increase and NPY/AgRP neurons decrease their firing rate in response to increased levels of glucose in mammals (Levin et al., 2004; Marty et al., 2007). This process is glucosensing. The best characterized glucosensing mechanism is that dependent on glucokinase (GK), and is similar to that existing in β-cells of endocrine pancreas (Blouet and Schwartz, 2010; Efeyan et al., 2015). Glucose is transported into the cell by glucose facilitative carrier type 2 (GLUT2) and then phosphorylated by GK. Once phosphorylated, glucose is metabolized through glycolysis then increasing intracellular ATP/ADP ratio leading to the closure of the ATP-dependent inward rectified potassium channel (K+ATP), membrane depolarization, calcium entry through L-type voltage-dependent calcium channel, and increased neuronal activity (Marty et al., 2007; De Backer et al., 2016). However, since not all glucosensing neurons rely on this mechanism (Fioramonti et al., 2004; De Backer et al., 2016), evidence for the existence of alternative glucosensing mechanisms obtained in several studies. Thus, high glucose concentrations stimulate the expression of liver X receptor (LXR) (Mitro et al., 2007) resulting in an inhibition of gluconeogenesis (Anthonisen et al., 2010; Archer et al., 2014). The stimulation by glucose of sweet taste receptors (similar to those in lingual taste cells) depending on a heterodimer of type 1 taste receptor subunits (T1Rs) formed by T1R2 + T1R3 and the G protein α-gustducin activates an intracellular signaling cascade (Ren et al., 2009). The expression of sodium/glucose co-transporter 1 (SGLT-1) increases in response to enhanced glucose levels (Díez-Sampedro et al., 2003; González et al., 2009; Herrera Moro Chao et al., 2016). Finally, another mechanism relies on mitochondrial production of reactive oxygen species leading to increased expression of uncoupling protein 2 in response to increased glucose levels (Blouet and Schwartz, 2010). In addition, several of these systems appear to be inter-connected. Thus, for instance, T1R3 and α-gustducin are necessary for increased SGLT-1 induction by dietary carbohydrates (Wauson et al., 2013).
In fish, recent studies demonstrated the presence and functioning of components of a GK-dependent glucosensing mechanism in brain areas of different species (Polakof et al., 2011d, 2012; Soengas, 2014). The response in rainbow trout hypothalamus after IP, ICV or dietary treatments inducing changes in the levels of glucose is shown in Table 1. Moreover, recent studies (Otero-Rodiño et al., 2015, 2016) provided evidence in rainbow trout for the presence and response to changes in circulating levels of glucose of glucosensing mechanisms in hypothalamus dependent on mitochondrial activity, LXR, and sweet taste receptor. No other studies attempted to elucidate the presence of glucosensing mechanisms in fish though glucose-sensing properties have been recently described in medaka hypothalamus (Hasebe et al., 2016).
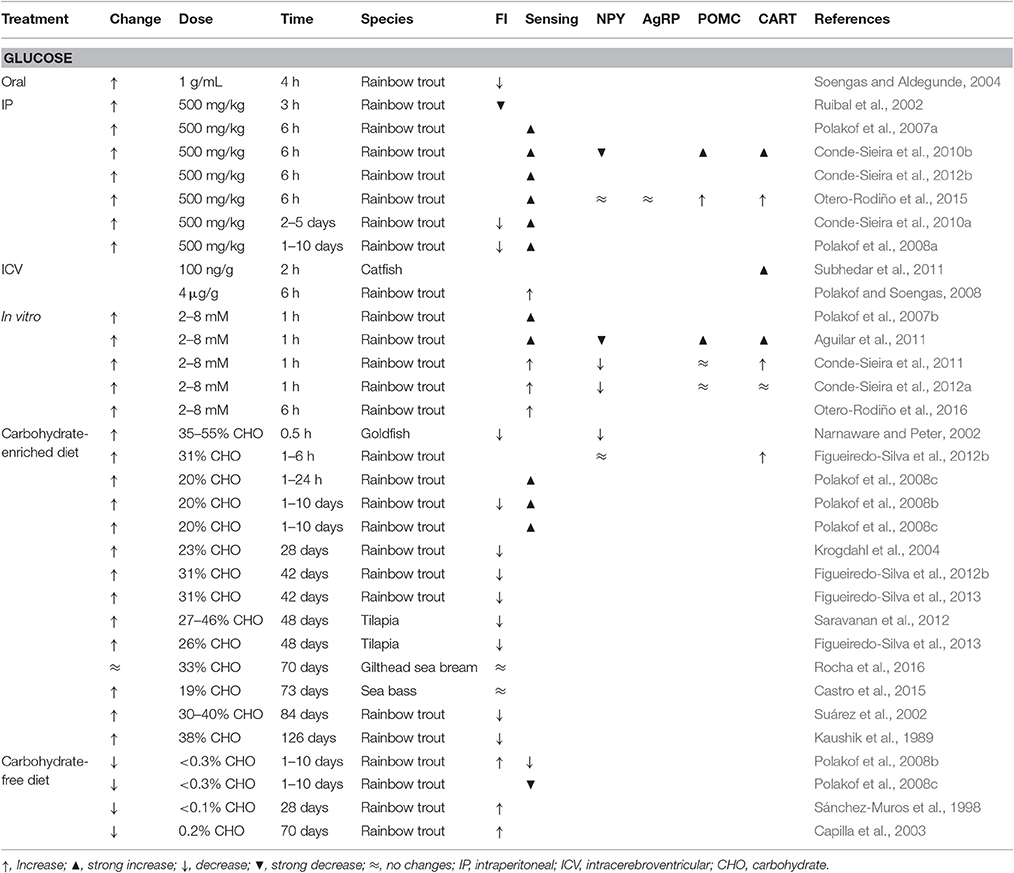
Table 1. Effects in different fish species of different treatments eliciting changes in glucose levels (change) on food intake (FI), the response of hypothalamic glucosensing systems (sensing), and the mRNA abundance in hypothalamus of orexigenic (NPY, AgRP) and anorexigenic (POMC, CART) neuropeptides.
Glucose and Food Intake Control
The detection of changes in glucose levels induces several regulatory responses allowing the animal to control glycemia, and one of these responses is food intake regulation (Marty et al., 2007). Thus, hypo- and hyperglycemia are known to increase and decrease food intake in mammals (Baird et al., 1997; Sanders et al., 2006) and birds (Seino and Miki, 2003), respectively.
Several studies carried out in fish suggest that changes in glucose levels may also modulate food intake response (Polakof et al., 2011d; Soengas, 2014), as summarized in Table 1. In general, fish fed with high-carbohydrate diets, or with glucose levels raised through IP or ICV treatments, displayed a decreased food intake, as demonstrated studies carried out in rainbow trout, goldfish, and tilapia. However, in some studies no changes occurred in food intake, as in sea bass or gilthead sea bream. Furthermore, fish fed a diet without carbohydrates or with low glucose levels resultant of IP or ICV treatments, increased food intake (Polakof et al., 2008a,b).
The mRNA of NPY, AgRP, POMC, and CART was detected in brain of different fish species in areas analogous to those of mammals (Cerdá-Reverter and Canosa, 2009), and changes in mRNA abundance related to food intake control (Volkoff et al., 2009). The presence in the same areas of rainbow trout brain of a marker of glucosesning such as GK protein (Polakof et al., 2009) and mRNA of neuropeptides involved in food intake control suggest that both findings are related. There are however few studies in fish assessing changes in hypothalamic neuropeptide mRNA abundance under conditions of altered glucose levels (Table 1). In rainbow trout NPY mRNA abundance decreased in hypothalamus after glucose treatment (Conde-Sieira et al., 2010b, 2012b; Aguilar et al., 2011; Otero-Rodiño et al., 2015) or after fish were fed with a high carbohydrate diet (Narnaware and Peter, 2002; Figueiredo-Silva et al., 2012b). Moreover, increased CART mRNA levels were observed in hypothalamus after experimental rising of glucose levels in catfish (Subhedar et al., 2011) and rainbow trout (Conde-Sieira et al., 2010b, 2012b; Otero-Rodiño et al., 2015), or in rainbow trout fed with a diet enriched with carbohydrates (Figueiredo-Silva et al., 2012b). Hypothalamic POMC-A1 mRNA abundance also increased in rainbow trout after hyperglycaemic treatment (Conde-Sieira et al., 2010b; Otero-Rodiño et al., 2015). Thus, increased glucose levels elicit increased anorectic potential whereas decreased glucose levels elicit increased orexigenic potential in agreement with the changes in food intake reported in the same species (Polakof et al., 2008a,b).
Fatty Acid Sensors and Control of Food Intake
Fatty Acid Sensing Mechanisms
Evidence in mammals supports that specialized neurons in hypothalamus detect changes in circulating levels of long-chain fatty acid (LCFA), but not short- (SCFA) or medium-chain fatty acid (MCFA) contributing to neural control of energy homeostasis (Migrenne et al., 2007; Gao et al., 2013; Duca and Yue, 2014; Efeyan et al., 2015). The most accepted mechanism is of metabolic nature. Thus, increased LCFA levels in plasma induced an increase in malonyl-CoA levels and subsequent inhibition of carnitinepalmitoyltransferase 1 (CPT-1) to import FA-CoA into the mitochondria for oxidation (López et al., 2005, 2007). Other fatty acid sensing mechanisms are present in hypothalamus. Thus, the increased binding to fatty acid translocase (FAT/CD36) induced by increased levels of LCFA results in the modulation of transcription factors like sterol regulatory element-binding protein type 1c (SREBP1c), and peroxisome proliferator-activated receptor type α (PPARα) (Le Foll et al., 2009). The translocation and activation of specific isoforms of protein kinase C (PKC) in response to enhanced LCFA levels results in PI3K inhibition (Benoit et al., 2009; Blouet and Schwartz, 2010). The enhanced production of reactive oxygen species in mitochondria in response to raised levels of LCFA results in K+ATP inhibition (Blouet and Schwartz, 2010). Finally, the activity of lipoprotein lipase related to increased levels of LCFA (Picard et al., 2013). The 18 carbon monounsaturated fatty acid oleate (C18:1 n-9) is the most studied LCFA in mammals involved in the activation of fatty acid sensing systems (López et al., 2007; Blouet and Schwartz, 2010; Duca and Yue, 2014). Fatty acid unsaturation appears to be important since the saturated fatty acid palmitate (C16:0) does not activate hypothalamic fatty acid sensing systems (Ross et al., 2010; Schwinkendorf et al., 2011; Greco et al., 2014). Moreover, the presence of more than one double bond, such as for linoleate (C18:2 n-6) or docosahexanoate (C22:6 n-3), does not activate fatty acid sensing systems in mammals (Gomez-Pinilla and Ying, 2010; Ross et al., 2010; Schwinkendorf et al., 2011; Greco et al., 2014).
In fish, lipids are main nutrients involved in metabolically sustaining relevant physiological processes (Tocher, 2003; Polakof et al., 2010). Thus, it is reasonable that lipids are involved in food intake control. In recent years, the presence and function of fatty acid sensing systems in the hypothalamus was characterized in fish (Librán-Pérez et al., 2012, 2013, 2014a,b, 2015a,b), as summarized in Table 2. IP (Librán-Pérez et al., 2012), ICV (Librán-Pérez et al., 2014a), and in vitro (Librán-Pérez et al., 2013) administration in rainbow trout of oleate or the MCFA octanoate (C8:0) induced a response in hypothalamus compatible with fatty acid sensing. This included reduced potential of lipogenesis and fatty acid oxidation, decreased potential of K+ATP, and modulation of FAT/CD36 with subsequent changes in the expression of transcription factors (Librán-Pérez et al., 2012, 2013, 2014a). This response is comparable with that of mammals with the main difference of the capacity of fish to respond to increased levels of an MCFA like octanoate (Hu et al., 2011). This could relate to the finding that body lipids in teleosts contain considerable amounts of MCFA (Davis et al., 1999; Trushenski, 2009) and that in rainbow trout there is no preferential oxidation of MCFA compared with LCFA (Figueiredo-Silva et al., 2012a), in contrast with the mammalian situation (Ooyama et al., 2009).
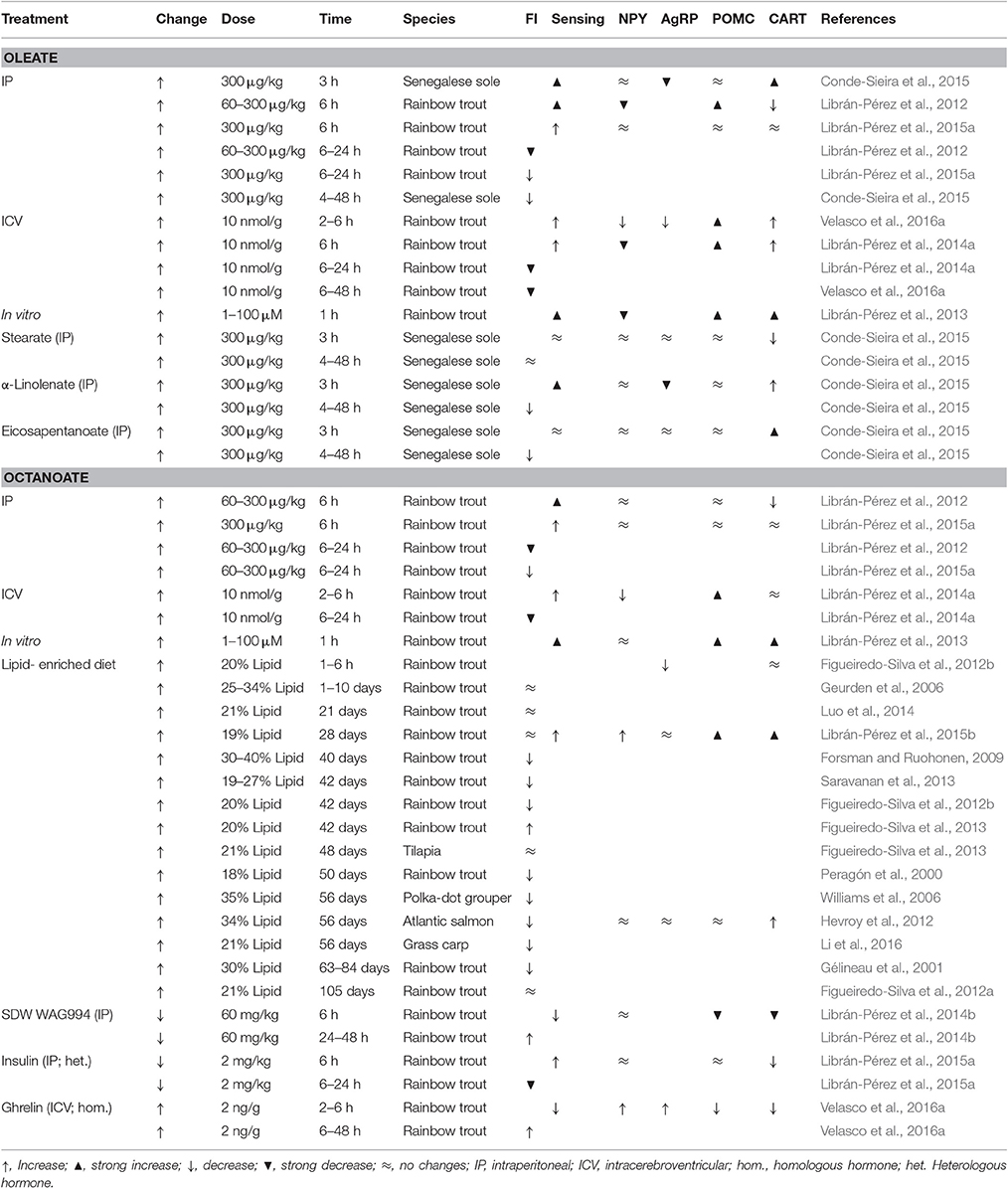
Table 2. Effects in different fish species of different treatments eliciting changes in fatty acid levels (change) on food intake (FI), the response in hypothalamus of fatty acid sensing systems (sensing), and the mRNA abundance in hypothalamus of orexigenic (NPY, AgRP) and anorexigenic (POMC, CART) neuropeptides.
All vertebrate species have dietary requirements for specific polyunsaturated fatty acid (PUFA), and diets for marine fish are particularly rich in long chain PUFA (Sargent et al., 2002). The brain of marine fish displays high levels of n-3 PUFA, mainly in α-linolenate (C18:3 n-3), eicosapentanoate (C20:5 n-3), and docosahexanoate (Tocher et al., 1992; Betancor et al., 2014). Therefore, it is reasonable to hypothesize that fish hypothalamic FA sensing systems, particularly in marine species, could differ from those of mammals in the ability to sense PUFA. Accordingly, a recent study in Senegalese sole (Conde-Sieira et al., 2015) demonstrated that its fatty acid sensing systems were activated not only by oleate but also by an n-3 PUFA such as α-linolenate. However, in the same study authors showed that another PUFA such as eicosapentanoate did not alter fatty acid sensing systems suggesting that the response might be specific to certain PUFA.
If fatty acid sensing systems activated when levels of specific fatty acid rise, what happens in those systems when levels of fatty acids fall? It is not possible to decrease the levels of a particular fatty acid. The only available studies in mammals used pharmacological treatments inhibiting lipolysis to induce a general decline in levels of all circulating fatty acids, which coincided with decreased activity of fatty acid sensing systems (Oh et al., 2012, 2014). A similar experimental approach in rainbow trout resulted in an inhibition of fatty acid sensing mechanisms in hypothalamus associated with the activation of the hypothalamus-pituitary-interrenal (HPI) axis (Librán-Pérez et al., 2014b).
Fatty Acids and Control of Food Intake
Feeding fish with diets enriched in lipids result in a decrease in food intake as observed in different species (Table 2). A comparable lower food intake also occurred in fish containing high fat stores (Shearer et al., 1997; Silverstein et al., 1999; Johansen et al., 2002, 2003). Considering the qualitative and quantitative importance of fatty acids within the lipid pool in fish diets and tissue composition, it is not surprising that the available studies are focussed on fatty acids.
In recent studies in rainbow trout, increased levels of oleate or octanoate in vivo, either after IP (Librán-Pérez et al., 2012) or ICV (Librán-Pérez et al., 2014a) treatments, resulted in decreased food intake, with more potent effects for octanoate. Moreover, also in rainbow trout fed diets with different lipid composition, the fish with the highest levels of fatty acid in plasma were those in which a decreased food intake occurred (Luo et al., 2014). Therefore, the inhibition of food intake reported in fish by feeding lipids is probably due to the action of central fatty acid sensors. This is also supported by the finding that the treatment of rainbow trout with C75 (fatty acid synthase inhibitor) resulted in a reduced food intake counteracted by TOFA (acetyl-CoA carboxylase inhibitor) (Librán-Pérez et al., 2012). In Senegalese sole, a decreased food intake occurred after IP treatment with oleate as well as different types of fatty acids including saturated fatty acids like stearate, and two types of PUFA such as α-linolenate and eicosapentanoate (Conde-Sieira et al., 2015). When levels of circulating fatty acids decreased through non-specific pharmacological treatment, a sound increase in food intake occurred in rainbow trout (Librán-Pérez et al., 2014b).
In mammals, enhanced levels of LCFA resulted in a decrease in mRNA abundance of AgRP and NPY as well as an increase of CART and POMC (López et al., 2005). However, few studies assessed in fish hypothalamus mRNA abundance of neuropeptides in response to changes in levels of fatty acids in circulation (Table 2). In rainbow trout the treatment with oleate or octanoate either IP (Librán-Pérez et al., 2012), ICV (Librán-Pérez et al., 2014a), or in vitro (Librán-Pérez et al., 2013) resulted in a decrease in mRNA abundance of NPY and an increase of CART and POMC-A1. The decrease in NPY is comparable to that described in rat hypothalamus after oleate ICV treatment (Blouet and Schwartz, 2010). Changes in mRNA abundance of neuropeptides suggest an enhancement of anorexigenic potential, which is in agreement with the decrease observed in food intake of rainbow trout after oleate or octanoate treatment (Librán-Pérez et al., 2012, 2014a). It is important to emphasize that the response to octanoate is unique to fish since in mammals octanoate is not inducing any change in mRNA abundance of neuropeptides (Hu et al., 2011). In Senegalese sole, the increase in circulating levels of oleate also resulted in decreased mRNA abundance of AgRP-2 and increased values of CART-2b again favoring increased anorectic potential (Conde-Sieira et al., 2015). In the same species the IP treatment with PUFA such as α-linolenate or eicosapentanoate (Conde-Sieira et al., 2015) increased anorectic potential based on decreased mRNA abundance of AgRP-2 (α-linolenate) and increased mRNA abundance of CART-2b (α-linolenate and eicosapentanoate). The decrease in circulating levels of fatty acid induced in rainbow trout by pharmacological treatment resulted in a fall of anorexigenic potential based on decreased mRNA abundance of POMC-A1 and CART (Librán-Pérez et al., 2014b).
Integration of Nutrient Sensing Information
The precise mechanisms connecting changes in glucose or fatty acid sensing systems and the mRNA abundance of orexigenic and anorexigenic factors are mostly unknown. Changes in neuropeptide expression have been associated with the modulation of brain homeobox transcription factor (BSX), forkhead box01 (Fox01), and phosphorylated cAMP response-element binding protein (pCREB) (Diéguez et al., 2011). The actions of these factors would result in the enhancement of CART and POMC, and the inhibition of NPY and AgRP, ultimately leading to decreased food intake (López et al., 2007; Diéguez et al., 2011). How these transcription factors relate to the activity of the different nutrient sensing systems? Several possibilities were suggested in mammals including (1) direct action of malonyl CoA or CPT1c, (2) indirect action through inhibition of CPT-1, (3) modulation by protein kinase B (Akt), AMPK, carbohydrate-responsive element-binding protein (ChREBP), or mTOR, or (4) involvement of ceramides (López et al., 2007; Diéguez et al., 2011; Gao et al., 2013).
In fish, a preliminary study in rainbow trout (Librán-Pérez et al., 2015b) showed that the cell signaling pathways that are dependent on Akt, AMPK, and mTOR activated in hypothalamus of fish fed a lipid-enriched diet. In the same species, a recent study (Velasco et al., 2016b) pointed to a possible role of ceramides in connecting hypothalamic fatty acid sensing systems and neuropeptide mRNA abundance with food intake control. Besides these studies, there is no further evidence in fish about the integration of the metabolic information of different nutrient (glucose, fatty acids, amino acids) sensing systems into a unique pathway regulating transcription factors involved in the production of neuropeptides controlling food intake. A summary of hypothetical relationships is shown in Figure 2.
Hypothalamic Integration of Endocrine Information
The hypothalamic neurons producing neuropeptides that control food intake in response to changes in the levels of nutrients are also responsive, through binding to appropriate receptors, to the effect of hormones (Levin et al., 2004; Blouet and Schwartz, 2010). These include hormones providing information regarding metabolic stores or energy status such as leptin and insulin, and gastrointestinal hormones providing information regarding absence/presence of food and its composition, including ghrelin and cholecystokinin (CCK), among others (Blouet and Schwartz, 2010). The same hormones modulate the activity of fatty acid- and glucose-sensing systems and mRNA abundance of neuropeptides related to the control of food intake in hypothalamus, as summarized in Table 2 (fatty acid) and Table 3 (glucose).
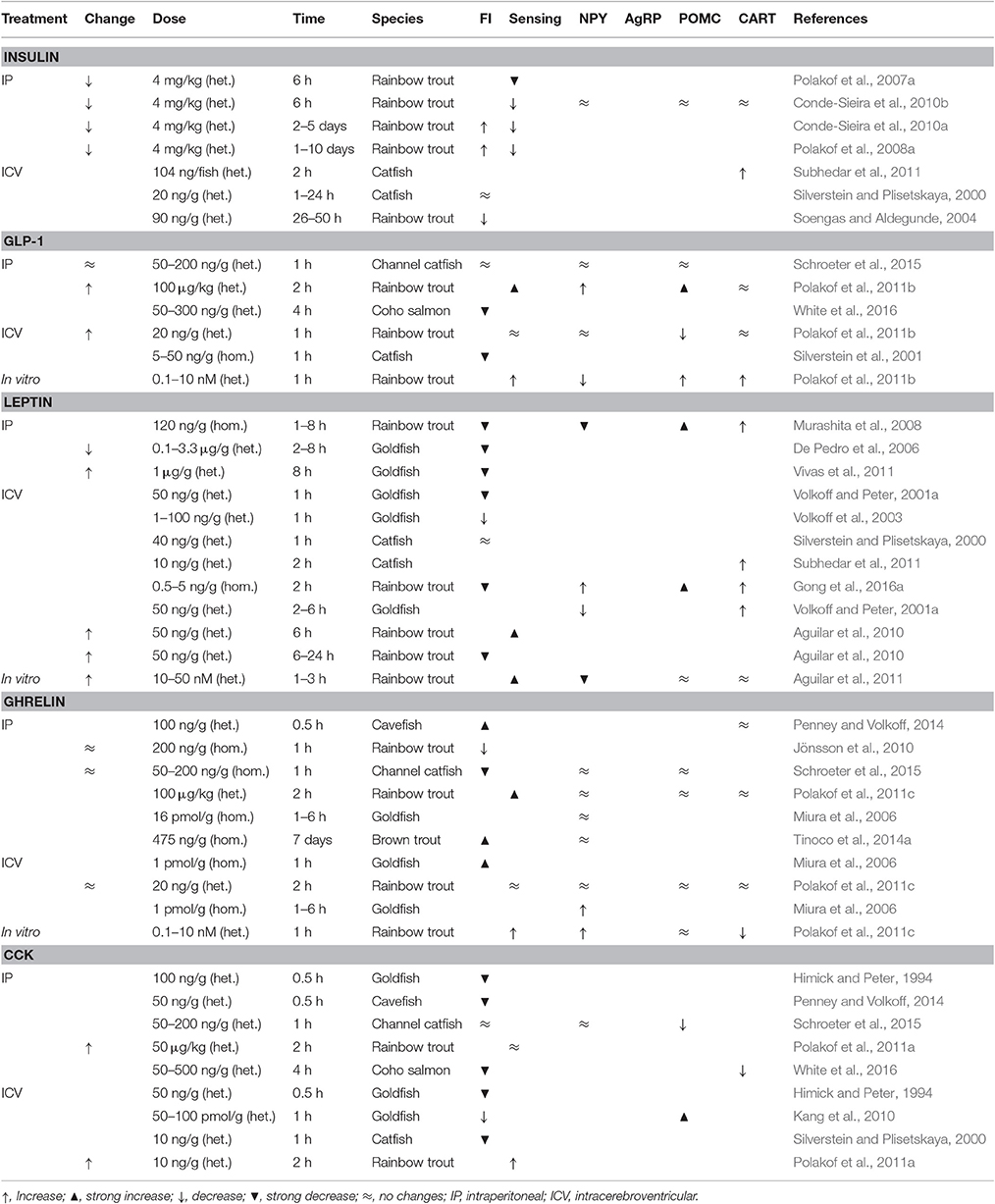
Table 3. Effects in different fish species of different hormone (either homologous: hom., or heterologous: het.) treatments eliciting changes in glucose levels (change) on food intake (FI), the response of hypothalamic glucosensing systems (sensing), and the mRNA abundance in hypothalamus of orexigenic (NPY, AgRP) and anorexigenic (POMC, CART) neuropeptides.
Insulin
Insulin and insulin receptors are present in fish hypothalamus (Gutiérrez and Plisetskaya, 1994; Leibush et al., 1996; Caruso et al., 2008). The effects of insulin treatment on food intake in fish are however contradictory. Thus, in rainbow trout IP administration of insulin either resulted in inhibition (Librán-Pérez et al., 2015a) or activation (Polakof et al., 2008a; Conde-Sieira et al., 2010b) of food intake, whereas ICV treatment did not affect food intake in catfish (Silverstein and Plisetskaya, 2000) but induced a decrease in rainbow trout (Soengas and Aldegunde, 2004). The anorectic effects in rainbow trout agree with the increased anorexigenic potential (increased CART and decreased NPY mRNA abundance) observed in hypothalamus after insulin treatment (Librán-Pérez et al., 2015a). Moreover, insulin administration in rainbow trout inhibits the glucosensing system dependent on GK (Polakof et al., 2007a, 2008a; Conde-Sieira et al., 2010b) whereas no clear effects were noted on fatty acid sensing systems (Librán-Pérez et al., 2015a).
Leptin
Multiple forms of leptin exist in fishes, with the major form, leptin A, being the most likely candidate for food intake regulation and energy signaling (Gorissen and Flik, 2014). The treatment with either fish or recombinant human leptin in fish usually results in an anorectic response (De Pedro et al., 2006; Murashita et al., 2008; Kling et al., 2009; Vivas et al., 2011; Won et al., 2012; Gong et al., 2016a). This anorexigenic action of leptin appears to be mediated by brain leptin receptors, particularly in the hypothalamus (Kurokawa et al., 2008; Tinoco et al., 2012; Angotzi et al., 2016), where leptin exerts its anorectic action through regulation of neuropeptide expression. Accordingly, the leptin receptor-deficient medaka shows increased food intake accompanied by increased NPYa and AgRP mRNA abundance, and decreased POMC1 mRNA abundance (Chisada et al., 2014). Moreover, ICV injection of leptin reduced NPY mRNA levels in hypothalamus and telencephalon of goldfish (Volkoff et al., 2003), and in the whole brain of grass carp (Li et al., 2010). Peripheral treatment with recombinant salmon leptin A1 in rainbow trout induced a hypothalamic transient reduction and elevation of NPY and POMC-A1 mRNA levels, respectively (Murashita et al., 2011). Moreover, central injection of leptin increased CART-I mRNA levels in hypothalamus of goldfish (Volkoff and Peter, 2001a), and POMC-A1, A2 and B, and CART mRNA levels in rainbow trout (Gong et al., 2016a). On the other hand, the leptin anorectic effects also related in rainbow trout to the activation of central glucosensing systems (Aguilar et al., 2010, 2011).
The action of leptin on food intake regulation depends on the time of hormone administration, indicating a circadian dependence of leptin anorexigenic effects (Vivas et al., 2011). In this sense, leptin-aI and leptin-aII mRNAs in hypothalamus of goldfish show 24-h rhythms under light–dark cycle and scheduled feeding conditions (Tinoco et al., 2014b). Post-prandial increases of hypothalamic and liver leptin-aI expression (Huising et al., 2006; Moen and Finn, 2013; Zhang et al., 2013) are in agreement with the anorexigenic role of this hormone in fish. As expected for an anorexigenic hormone, feeding changes and nutritional status modifies the leptin system in fish, but different responses occurred depending on the feeding regime and species. Thus, leptin genes expression or circulating leptin levels rise with food restriction or starvation, when energy stores decline, and decrease during refeeding in some species (Gorissen and Flik, 2014; Johansson and Björnsson, 2015), but not in others (Huising et al., 2006; Tinoco et al., 2012). These, and other recent studies (Chisada et al., 2014; Londraville et al., 2014; Salmerón et al., 2015; Jørgensen et al., 2016) support that leptin in fish should not be considered as a lipostat signal in contrast to mammals. In this way, zebrafish knockout for leptin suggest the involvement of leptin in glucose homeostasis but not in adipostasis (Michel et al., 2016). Nevertheless, the existence of different leptin paralogs in fish differentially involved in the regulation on energy resources in a species/tissue dependent manner might explain the variety of results.
Gastrointestinal Hormones
Ghrelin
Ghrelin is a peptide mainly synthesized in the stomach or its equivalent in stomachless fish species (Kaiya et al., 2008), although its gene expression is also detected in other peripheral locations and in brain (Kojima et al., 1994; Unniappan et al., 2002; Feng et al., 2013). Some studies point to the endocrine cells in the digestive mucosa as the peripheral primary synthesizing site of ghrelin in fish (Jönsson, 2013). Ghrelin is also widely expressed in fish brain, particularly in hypothalamus (Jönsson and Holmgren, 2012; Sánchez-Bretaño et al., 2015b). Ghrelin requires a post-translational acylation (catalyzed by ghrelin O-acyltransferase, GOAT) before binding to its receptor (Yang et al., 2008). Due to tetraploidization experienced by some fish species, two paralog genes of ghrelin receptor and four or eight receptor subtypes are present in some fish (Kaiya et al., 2013). The wide distribution of transcripts of these receptors, and particularly its presence in gastrointestinal tract, liver, brain sensory areas, and pituitary may indicate multiple targets for the regulation of energy balance by this hormone (Jönsson, 2013). Particularly, the GHS-R1a subtype (specifically involved in energy balance, Kaiya et al., 2010) exhibits a dense expression in discrete hypothalamic nucleus, such as the lateral recessus nucleus, in support of the orexigenic role of the ghrelinergic system (Sánchez-Bretaño et al., 2015b).
Ghrelin stimulates feeding in all mammalian species studied so far, primarily through increased release of NPY and AgRP (Patton and Mistlberger, 2013). However, this generalized orexigenic effect is not so evident in fish. Ghrelin is an orexigenic peptide in many fishes (Miura et al., 2006; Kaiya et al., 2008; Picha et al., 2009; Kang et al., 2011; Jönsson, 2013; Penney and Volkoff, 2014; Tinoco et al., 2014a). However, this peptide exerts anorexigenic actions in tilapia (Peddu et al., 2009) and both anorexigenic (Jönsson et al., 2010) and orexigenic (Velasco et al., 2016a,b) effects in rainbow trout. The orexigenic action of ghrelin in goldfish is mediated by hypothalamic activation of NPY (Miura et al., 2006) and orexin-A (Miura et al., 2007; Nisembaum et al., 2014b) mRNA via vagus nerve (Matsuda et al., 2006b), as peripheral administration of ghrelin does not modify NPY mRNA expression in goldfish hypothalamus (Nisembaum et al., 2014b). Interestingly, the expression of ghs-r1 ghrelin receptor (Sánchez-Bretaño et al., 2015b) and GOAT (Blanco et al., 2016b) in hypothalamic nuclei that also express orexin-A and NPY in goldfish reinforces the suggested mechanism for the orexigenic action of ghrelin in this teleost. On the other hand, the post-feeding decrease of circulating ghrelin levels (Unniappan et al., 2004) and the preprandial rise of circulating acyl-ghrelin and GOAT (Blanco et al., 2016a) support the role of this peptide as a meal initiator in goldfish. In agreement with this action of ghrelin, the hypothalamic mRNA expression of GH secretagogue receptors (sbGHSR-1a and sbGHSR-1b) was higher in fasted than in fed seabream (Zhang et al., 2008).
In addition to the effects of ghrelin on the feeding regulatory neurons in hypothalamus, some studies suggest that the effects of ghrelin on food intake are also mediated by gastrointestinal motility in the periphery, as in zebrafish (Olsson et al., 2008). However, this is not the rule in fish, since ghrelin does not modify intestinal motility in other teleost (Kitazawa et al., 2012). Localization studies agree with this lack of ghrelin effect on gut motility since ghrelin receptor expressing cells are not present in the muscle layer of gut (Sánchez-Bretaño et al., 2015b).
Feeding and nutritional status are the main factors involved in the regulation of ghrelinergic system in mammals, but this is controversial in fish. Different responses to food deprivation occurred depending on the species, tissue, and duration of food deprivation. Accordingly, different effects are found on ghrelin release, its expression in different central and peripheral tissues, and GOAT activity (Unniappan et al., 2004; Jönsson et al., 2007; Fox et al., 2009; Hevrøy et al., 2011; Blanco et al., 2016b).
The effect of ghrelin treatment on central nutrient sensing systems was assessed in rainbow trout, and glucosensing systems appear to be activated by ghrelin treatment (Polakof et al., 2011c), an effect opposed to that addressed in mammals (Wang et al., 2008). In agreement with the well-known effect in mammals, fatty acid sensing systems are inhibited in rainbow trout by ICV ghrelin treatment (Velasco et al., 2016a,b). These changes agree with the increased mRNA abundance of AgRP/NPY and decreased mRNA abundance of POMC-A1/CART observed simultaneously (Velasco et al., 2016a,b).
CCK
CCK is a member of the CCK-gastrin family that exerts a key role in digestive physiology of vertebrates, including fish (Olsson and Holmgren, 2011). Partial and complete mRNA sequences of CCK are available for a number of fish species, and CCK-like-ir is present in gut and nervous system of several teleost species (Jönsson et al., 1987; Micale et al., 2012, 2014; Ji et al., 2015). CCK-like peptides are potent anorexigenic signals in fish (Himick and Peter, 1994; Volkoff et al., 2005; Rubio et al., 2008; MacDonald and Volkoff, 2009; White et al., 2016). Moreover, these hormones are involved in many digestive functions and glucose homeostasis (Rajjo et al., 1988; Aldman and Holmgren, 1995; Einarsson et al., 1997; Polakof et al., 2011a; Tinoco et al., 2014b). CCK-8 may be involved in the seasonal changes of food intake experienced by salmonids (White et al., 2016), and starvation challenge experiments support the anorexigenic action of CCK-8 in fish. The expression levels of CCK mRNA decreased in brain and intestine after starvation in different fish species (Murashita et al., 2006; Feng et al., 2012; Ji et al., 2015), while CCK is released after feeding in intestine (Aldman and Holmgren, 1995). The mechanisms underlying the anorexigenic effect of CCK-like peptides are unknown, but the lack of effect after peripheral administration in channel catfish (Schroeter et al., 2015) suggests that anorexigenic action occur at the central level. Interactions with other feeding regulators, as the hypothalamic expression of amylin, have been described in goldfish (Thavanathan and Volkoff, 2006), but the mechanisms involved appear to be different when comparing peripheral and central administration (Hoskins and Volkoff, 2012). Accordingly, a differential distribution pattern of CCK receptors subtypes is present in goldfish with high expression of CCKAR subtype in the intestine, whereas the CCKBR subtype predominantly expressed in hypothalamus and vagal lobe (Tinoco et al., 2015). Finally, CCK-8 treatment activated glucosensing capacity in hypothalamus and hindbrain of rainbow trout (Polakof et al., 2011a).
Other Gastrointestinal Hormones
Glucagon-like peptide 1 (GLP-1) appears to be anorectic in fish (Silverstein et al., 2001; White et al., 2016). In rainbow trout GLP-1 treatment activated hypothalamic glucosensing systems (Polakof et al., 2011b) in parallel with altered mRNA abundance of several neuropeptides including increased values for CART and POMC-A1 and decreased values for NPY (Polakof et al., 2011b).
Other gastrointestinal peptides also show anorexigenic properties in fish including those peptides belonging to the calcitonin gene-related peptide family, such as the calcitonin gene-related peptide (CGRP), intermedin (or adrenomedulin) and amylin (or islet amyloid polypeptide). These peptides act through a calcitonin receptor-like receptor and show a broad distribution in brain and peripheral tissues in fish (Ogoshi et al., 2003; Martínez-Álvarez et al., 2008). CGRP mRNA is widely expressed in the central and peripheral nervous system, intermedin-ir is present in brain, pituitary and most peripheral tissues, including endocrine pancreas (López et al., 1999). Amylin is present in Brockmann bodies, thus suggesting that once produced in the periphery transported into brain where it has central actions (Westermark et al., 2002). ICV injections of these three peptides in goldfish induced a decrease in food intake (Martínez-Álvarez et al., 2009) mediated at the central level through unknown mechanisms. The presence of CGRP fibers innervating ventromedial hypothalamic nucleus (Batten and Cambre, 1989) support a direct effect on hypothalamus probably in a paracrine or autocrine manner (Martínez-Álvarez et al., 2009).
Hypothalamic Integration of Circadian Information
Hypothalamus and Peripheral Circadian Clocks
Feeding shows a rhythmic pattern in a widespread of fish (Madrid et al., 2001), occurring periodically through the 24-h light/dark cycle, but tidal, lunar and seasonal feeding rhythms are also been described in some species (López-Olmeda and Sánchez-Vázquez, 2010). These feeding rhythms, as many other aspects of energy metabolism and homeostatic balance, are coordinated by the phylogenetically well-conserved circadian system, which generates an internal timing that allow animals to anticipate cyclic environmental and endogenous variations, and then, prepare the physiology for upcoming challenges. In the functional organization of circadian system, multiple endogenous clocks, synchronized by environmental and endogenous cues, generate an internal rhythmicity close to 24 h (Albrecht, 2012). The hypothalamus plays a key role in the circadian timekeeping system of vertebrates, acting as an integrative neuronal network. In mammals, a bilateral structure in the anterior hypothalamus, the suprachiasmatic nucleus (SCN), is an autonomously rhythmic nucleus that drives overt circadian rhythms in behavior and physiology, including food intake (Partch et al., 2014). The SCN is formed by clusters of interconnected neurons with individual molecular clock mechanisms inside each cell that function as an autonomous oscillator, and the intercelular coupling among these individual cells is critical for the coordination of endogenous rhythmicity and the outputs to downstream tissues (Evans and Gorman, 2016). Additional neuronal clocks are also present in ARC, ventromedial hypothalamus, and the dorsomedial hypothalamus, and in a variety of peripheral tissues, including liver, adipose tissue, adrenal and muscle (Dibner et al., 2010). In mammals, it is widely accepted that the internal coordination of circadian rhythmicity is established by the temporal signals provided by the master SCN to other hypothalamic nuclei and peripheral organs through a wide variety of direct and indirect pathways that form a network of oscillators. Fish hypothalamus also contains circadian oscillators (Velarde et al., 2009; Patiño et al., 2011; Vatine et al., 2011; Idda et al., 2012; Martín-Robles et al., 2012; Nisembaum et al., 2012; Vera et al., 2013), but no master clock has been yet identified. In contrast, the functional relevance of endogenous clocks in a variety of fish tissues supports the existence of a multiple network of endogenous oscillators in the brain (hypothalamus, diencephalon, pineal, retina, optic tectum, pituitary) and peripheral (liver, gut, gonads, head kidney) locations (Isorna et al., 2017). A summary of the main components in the fish circadian organization is shown in Figure 3.
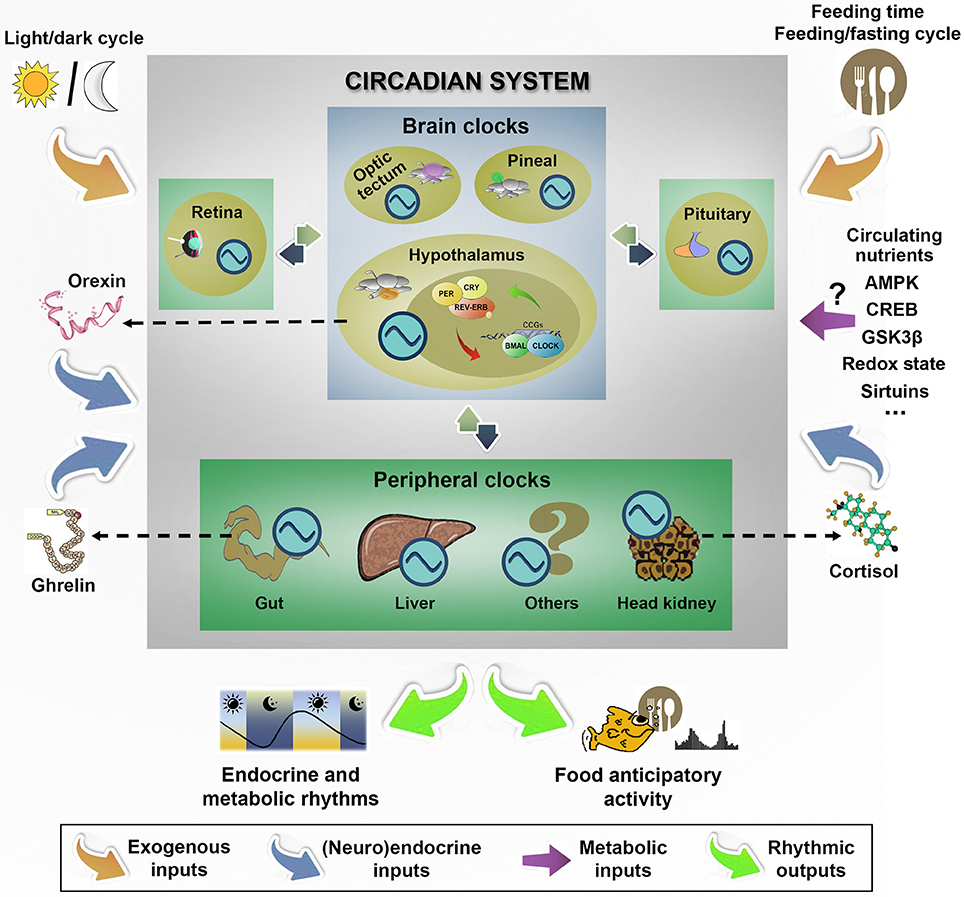
Figure 3. Hallmarks of functional organization of circadian system in fish emphasizing the main external and endogenous inputs that synchronizes brain and peripheral clocks to generate rhythmic outputs, and particularly, the daily food anticipatory activity and food intake. In fish, the lack of a master clock in the functional circadian system organization shapes a more flexible model with different intercommunicated clocks located in many tissues throughout the organism. As external inputs, the light/dark cycle is one of the best characterized, but other time cues (such as feeding time and feeding/fasting cycle) are the dominant exogenous synchronizers of peripheral clocks. The possible role of circulating nutrients and some central and peripheral regulatory signals (such as orexin, ghrelin or cortisol) is discussed in the text. ?, Unknown; AMPK, AMP-activated protein kinase; CREB, cAMP response-element binding protein; GSK3β, Glycogen synthase kinase 3 beta. Clock genes: PER, CRY, REV-ERB, BMAL, CLOCK, CCG. Circadian oscillator: .
The key molecules that establish the functional core of self-sustained circadian oscillators clocks are highly conserved from invertebrates to mammals, including fish (Partch et al., 2014), although important differences are found in fish regarding the additional number of copies of the clock genes resulting from the 3R teleost-specific genome duplication. The generation of cellular circadian oscillations is based on autoregulatory 24-h transcriptional/translational feedback loops of a set of clock genes (Ko and Takahashi, 2006). The proteins BMAL1, CLOCK and its analog NPAS2 act as transcriptional activators via E-box sequences driving the expression of clock controlled genes, some of them encoding other core clock protein repressors (PER and CRY). These PER and CRY proteins form complexes that are translocated into the nucleus where they shut down their own expression by removing the CLOCK(NPAS2)/BMAL1 complexes, defining a main negative loop. Some reinforcing loops are formed by the orphan nuclear receptors from the REV-ERBα-β and RORα-β families, to modulate the rhythmic transcription of Bmal1, Npas and Clock (Guillaumond et al., 2005; Schibler et al., 2015). Recent studies demonstrated that such oscillations in clock genes expression paralleled critical events of chromatin remodeling (Coomans et al., 2015; Masri et al., 2015).
Feeding as an Entrainment Cue for the Circadian Clocks
The light/dark cycle is the most effective signal that entrains the brain circadian clocks (Schibler et al., 2015). However, feeding related cues, including feeding time, nutritional inputs, feeding/fasting cycle and diet composition are the dominant synchronizers of peripheral clocks. Such so-called food-entrained oscillators provide animals the adaptive advantage of anticipate periodic meals within a circadian period of entrainment (Stephan, 2002; Mendoza, 2006). The food-entrained oscillators constitute the basis of the food anticipatory activity, an increase in locomotor activity occurring just before feeding time in anticipation of a predictable daily meal. This activity was evidenced in fish (López-Olmeda and Sánchez-Vázquez, 2010; Feliciano et al., 2011; Vera et al., 2013) and mammals (Mistlberger, 2009), and appears to be independent on hypothalamic SCN in mammals (Stephan, 2002) and hypothalamic clocks in fish (Velarde et al., 2009). To date, the endogenous control of the food anticipatory activity is largely unknown, but recent studies suggest that some orexigenic hormones, such as ghrelin, may be involved both in fish (Nisembaum et al., 2014b) and in mammals (Patton and Mistlberger, 2013).
The location of food-entrained oscillators is not yet certain. Neuronal feeding-synchronized clocks are suggested to reside in ventromedial and dorsomedial hypothalamus (Acosta-Galvan et al., 2011), or even in extrahypothalamic brain areas including nucleus accumbens and amygdala in mammals. The search and identification of feeding entrained clocks is a topical subject in fish, and both, peripheral (Nisembaum et al., 2012; Sánchez-Bretaño et al., 2015a) and brain (Feliciano et al., 2011; Vera et al., 2013; Sánchez-Bretaño et al., 2015b) are possible targets. This complex variety of locations of oscillators requires a functional organization among them to generate coordinated responses, and recent data suggest that nutritional cues may act as time givers (Dattolo et al., 2016).
Food intake is a potent phase-resetting agent for peripheral and central clocks, by entraining a number of overt rhythms in animals. The molecular mechanism underlying feeding entrainment remains largely unknown. In mammals, feeding modifies the phase of molecular oscillations in ARC and dorsomedial hypothalamic nuclei independently on the hypothalamic SCN (Feillet et al., 2008). In fish, a daily feeding schedule synchronizes rhythmicity of central (hypothalamus and optic tectum) and peripheral (gut and liver) clock genes (Feliciano et al., 2011; Nisembaum et al., 2012; Vera et al., 2013). The nature of the endogenous signals associated with feeding that may be capable of resetting peripheral clocks is not fully characterized yet, but metabolites, nutrient sensors, hormones, and possibly neuronal signals transmitted from nutrient-sensing areas to peripheral organs are good candidates.
Metabolic Signals and Circadian Clocks
The circadian system controls the expression of essential genes in numerous metabolic pathways (Ribas-Latre and Eckel-Mahan, 2016), but, in turns, it can be synchronized by its own effectors, generating a bidirectional relationship among the circadian clocks and metabolic signals (Challet, 2013).
Little is known about how specific diet components can modulate the circadian clocks, although it appears that periodic availability of circulating nutrients induces the feeding dependent resetting of peripheral and central circadian networks (Asher and Sassone-Corsi, 2015). In this context, ARC is an important target for such metabolic feedback (Uchida et al., 2016). Certain populations of neurons in ARC show rhythmicity in mammals (Ellis et al., 2008), with circadian rhythms of PER2 in vitro (Guilding et al., 2009). The circadian modulation of ARC neurons by circulating metabolites support the timing activation of this center for sensory metabolic information (Van den Top and Spanswick, 2006). The fact that ARC is also able to influence the functionality of the SCN (Yi et al., 2006), suggest that this ARC-SCN reciprocal interaction is essential to maintain a well-balanced metabolic circadian pattern.
Nutrient sensors evolved as time-giving signals by exerting different actions on the circadian clocks. This is the case of AMPK, regulated by glucose and fatty acids, which directly regulates CRYs proteins (Lamia et al., 2009), CREB, glycogen synthase kinase 3 beta, PPARs, and the redox state (Oosterman et al., 2015; Ribas-Latre and Eckel-Mahan, 2016). One interesting link between sensing of cellular energy status and circadian clocks is the control of clock genes expression and deacetylation of clock proteins by the sirtuins (SIRT), a (NAD+)-dependent class III of histone deacetylases that are well characterized by their numerous effects on intracellular metabolism. Particularly, the SIRT1 and SIRT6 establish functional links between cellular metabolism and circadian clocks physiology in mammals (Masri et al., 2014; Orozco-Solis et al., 2015). The activity of SIRT1 fluctuates through the feeding/fasting cycle (Cakir et al., 2009) and is involved in the cyclic control of cofactors and peptides of circadian clocks by deacetylating BMAL1 and PER2 in liver (Nakahata et al., 2008) and activates the hypothalamic SCN pacemaker in mice (Chang and Guarente, 2013). The high expression of this enzyme in the ventromedial hypothalamus support the role of this brain area on monitoring metabolic signals through SIRT1, at least in mice. Interestingly, these ventromedial neurons expressing SIRT1 project POMC neurons in the ARC, and innervate the outer shell of SCN, providing a basis to integrate and communicate metabolic signals in the hypothalamus (Orozco-Solis et al., 2015). SIRT6 appears to be involved in the cyclic synthesis of lipids and carbohydrates (Masri et al., 2014), which may reflect differential responses to distinct nutritional intakes. Whether these molecular sensors modulate brain and peripheral clocks in fish remains to be elucidated. Preliminary studies in rainbow trout assessed changes in mRNA abundance of SIRT-1 in parallel with the functioning of fatty acid sensing systems (Velasco et al., 2016a,b). The clearly adaptive significance of circadian clocks entrainment by nutrient status is supported in fishes by the feeding-related rhythms driven by the circadian system in digestive enzymes (Vera et al., 2007; Montoya et al., 2010; Nisembaum et al., 2014b). Furthermore, changes described in parameters related to glucose, fatty acid, and amino acid metabolism in brain (Polakof et al., 2007c) and peripheral tissues (Polakof et al., 2007d; Hernández-Pérez et al., 2015) also support the involvement of nutrient status.
Endocrine Signals in the Entrainment of Circadian Clocks
It is widely accepted that daily endocrine rhythms are outputs of the circadian system, but recent reports suggest that several hormones may act as inputs in the timing signalization of hypothalamic and peripheral clocks (Challet, 2015; Coomans et al., 2015).
Ghrelin may prove to be an endocrine signal that participates in the integration of gastrointestinal signals by the central clocks. The rhythmic expression of ghrelin transcript in hypothalamus, pituitary and gut (Sánchez-Bretaño et al., 2015b), and the periprandial variations in plasma of goldfish (Blanco et al., 2016a), and in hypothalamus of tilapia, zebrafish and goldfish (Peddu et al., 2009; Blanco et al., 2016b) indicate that ghrelin may work as an output of food-entrained oscillators. Ghrelin receptors are widely expressed in fish brain (Chen et al., 2008; Sánchez-Bretaño et al., 2015b), including those hypothalamic areas involved in the integration of food intake control, as well as the preoptic region and the anterior periventricular nucleus (Kaiya et al., 2010; Sánchez-Bretaño et al., 2015b). The expression of clock genes in these hypothalamic areas (Mazurais et al., 2000; Sánchez-Bretaño et al., 2015c) and the induction of per genes by ghrelin (Nisembaum et al., 2014b) support the role of ghrelin as an input of food-entrained clocks. In vivo (Nisembaum et al., 2014b) and in vitro (Sánchez-Bretaño et al., 2016b) studies carried out in goldfish reinforce the role of ghrelin in the feeding and metabolism-related signaling in hypothalamic and liver clocks. Furthermore, some reports indicate that this hormone may also participate in the regulation of food anticipatory activity in goldfish, as it modifies locomotor activity (Matsuda et al., 2006a), and this locomotor activity is blocked by a ghrelin antagonist (Nisembaum et al., 2014b).
The orexin/hypocretin system is involved in the coordination of rhythmic daily functions, such as feeding and energy balance in fish (Matsuda et al., 2012a). Taking in mind the orexigenic role of this regulator and its stimulatory effect on daily activity rhythms in the absence of other inputs (Nisembaum et al., 2014a), the possible role of orexin system in the physiology of certain brain areas involved in food intake and circadian regulation is intriguing. Orexin fibers found in some central oscillators, such as the pineal and the SCN, and other brain regions related to the regulation of activity/rest cycles (Wong et al., 2011; López et al., 2014). As a rule, orexins exert excitatory effects on various brain nuclei with the exception of a direct hyperpolarisation of clock cells in SCN during the night (Belle et al., 2014).
The orexin system is under the control of the molecular clock, as orexin neurons projecting into the pineal show circadian daily rhythmicity driven by the clock (Appelbaum et al., 2010), and daily variations in the hypothalamic expression of orexin in goldfish related to clock genes oscillations (Hoskins and Volkoff, 2012). Nevertheless, recent studies suggest that this peptidergic system also acts as an input to the clockworks. Particularly, this peptide seems to play an important role in food intake anticipation in fish, as its central administration up-regulates per genes in hypothalamus (central) and foregut (peripheral) clocks (Nisembaum et al., 2014a). The induction of per genes by orexin, as produced ghrelin, questions the existence of synergy between both orexigenic regulators in their actions on the food-entrained clocks.
Glucocorticoids display strong daily rhythms in vertebrates including fish. Cortisol daily rhythms are clear outputs of the circadian system, which are synchronized by the feeding-fasting cycle and feeding time in fish (Isorna et al., 2017). However, little is known about the possible role of glucocorticoids as inputs to the circadian system in fish. Recent reports in goldfish show that cortisol induces per1a and per1b expression, and represses the positive elements (bmal1a and clock) in liver clockwork (Sánchez-Bretaño et al., 2016a). Interestingly, such actions of cortisol on the liver, one of the most sensitive peripheral clockwork, link the entrainment of food-entrained clocks by both, metabolic signals and hormones.
Hypothalamic Integration of Other Factors
Stress
The endocrine stress response in fish is mediated by both the hypothalamic-sympathetic chromaffin cell and the HPI axes. The activation of both systems restores homeostasis by mobilizing fuel to make energy available to cope with increased metabolic demand (Wenderlar Bonga, 1997; Mommsen et al., 1999). A disruption of the feeding behavior is a common feature of the behavioral response to stress in fish (Bernier and Peter, 2001; Bernier, 2006). The activation of HPI in response to stress involves the synthesis of corticotrophin relasing factor (CRF) in the neurons of the preotic area of the CNS that, in turn, stimulates release of adrenocorticotropic hormone (ACTH) from the corticotrophic cells in the adenohypophysis. ACTH binds to the MC2R in the surface of the interrenal cells of the head kidney to stimulate glucocorticoid release into the blood (Wenderlar Bonga, 1997; Mommsen et al., 1999). Cortisol, the main glucocorticoid in fish mediates many effects of stressors on metabolic and behavioral processes (Barton, 2002; Bernier, 2006; Aluru and Vijayan, 2009).
In the fish brain, CRF is abundantly expressed within the dorsal telencephalic structures but also in the preoptic area and tuberal hypothalamus of zebrafish (Alderman and Bernier, 2007). ICV administration induces a dose-dependent reduction in food intake levels in goldfish (De Pedro et al., 1993; Bernier, 2006) that is reverted by the injection of receptor antagonist (De Pedro et al., 1997; Bernier and Peter, 2001). Goldfish treated with glucocorticoid antagonists or inhibitors of cortisol synthesis, display increased CRF mRNA brain levels and reduced feeding, which again can be reverted by CRF receptor antagonist (Bernier and Peter, 2001). It is suggested that CRF is a major physiological transductor of stress effects on food intake in fish (Bernier, 2006). In addition, CRF seems also to regulate social effects since its increased expression is responsible of the reduced feeding levels in subordinate fish (Doyon et al., 2003).
Recent studies demonstrated that MC4R is able to bind ACTH in the presence of the melanocortin receptor accessory protein 2 in zebrafish. In addition, ACTH administration inhibits food intake in zebrafish but only in animals carrying a functional copy of MC4R (Agulleiro et al., 2013). Together with the ACTH production in carp brain (Metz et al., 1994) this suggests that ACTH can also transduce stress information into feeding circuitry downstream of the melanocortin system. In addition, MC4R is highly expressed in the magnocellular neurons of the preoptic area where CRF is synthesized thus suggesting that central melanocortins could modulate the activity of the CRF neurons (Cerdá-Reverter et al., 2003a; Sánchez et al., 2009). Stress conditions not only regulate CRF and melanocortinergic pathways in fish as the activity of many other central systems vary with stressful conditions and/or increased glucocorticoid signaling. Changes in the activity of central monoaminergic systems including serotoninergic and dopaminergic systems occurred after stressful conditions. These central monoaminergic systems can play a key role in the integration of information during the exposition to the stressor but also in organizing the coordinated stress response (Lanfumey et al., 2008). Studies in rainbow trout demonstrated that the severity of the stressors were able to produce a rated stress response, but serotonin central levels were not accordingly gradated suggesting that some other systems must be integrating the magnitude of the stress response (Gesto et al., 2015). Chronic treatment with fluoxetine (inhibitor of serotonin recapture) is able to reduce whole body cortisol levels and display anxiolytic effects in zebrafish (Egan et al., 2009) but, on the contrary, acute stress induced a rapid increase of serotoninergic and dopaminergic activity in the forebrain of rainbow trout (Gesto et al., 2013). Accordingly, both dopamine (Leal et al., 2009) and serotonin (Ortega et al., 2013) inhibit food intake in fish supporting both dopaminergic and serotoninergic pathways as neuroanatomical substrates for the integration of stress effects on behavioral pathways regulating food intake.
The reduced food intake observed in response to stress in fish could also relate to changes in the ability of nutrient sensing systems to modulate food intake. In rainbow trout chronic stress induced a readjustment in the activity of hypothalamic glucosensing mechanisms (Conde-Sieira et al., 2010a; Otero-Rodiño et al., 2015). The readjustment resulted in an inability of the fish to compensate with changes in food intake those of circulating glucose levels as observed in non-stressed fish. The response of hypothalamic mRNA abundance of CART, POMC, and NPY to glucose changed under stress conditions (Conde-Sieira et al., 2010a; Otero-Rodiño et al., 2015). CRF might be involved in the mechanism through which stress influences food intake control (Evans et al., 2004; McCrimmon et al., 2006). Accordingly, CRF treatment of rainbow trout hypothalamus in vitro readjusted glucosensing mechanisms (Conde-Sieira et al., 2011) in a way similar to that observed under stress conditions (Conde-Sieira et al., 2010a).
Orexins
The orexins/hypocretins are neuropeptides belonging to the incretin gene family of peptides and are involved in many physiological processes in fish, including feeding (Hoskins and Volkoff, 2012), locomotion/rest cycles (Volkoff, 2012; Nakamachi et al., 2014; Nisembaum et al., 2014a) and reproduction (Wong et al., 2011). The cDNA sequences encoding for preproorexin in fish contains orexin A and orexin B, with a high degree of homology with other orexins in vertebrates (Kaslin et al., 2004). Orexins are present in numerous fish species with a broad distribution. Thus, orexin ir-cell bodies, preproorexin mRNA, and orexin proteins are abundant in hypothalamus and preoptic area of most species so far studied (Volkoff, 2015), areas associated with the control of food intake in fish.
Orexins stimulate food intake in all fishes investigated so far. Earlier studies demonstrated this effect in goldfish (Volkoff et al., 1999; Facciolo et al., 2011; Matsuda et al., 2012a), and zebrafish (Novak et al., 2005; Yokobori et al., 2012). Currently, it is generally accepted that orexins are potent orexigenic regulators in fish (Hoskins and Volkoff, 2012; Matsuda et al., 2012a; Penney and Volkoff, 2014). The stimulation of appetite by orexin injections in many of the species studied is consistent with increases in preproorexin brain mRNA expression levels displayed after different periods of food deprivation (Novak et al., 2005; Abott and Volkoff, 2011; Volkoff, 2014). In goldfish, the presence of orexin-like-ir in the hypothalamic nucleus recessus lateralis is induced by fasting condition (Nakamachi et al., 2006). These stimulations of the hypothalamic orexin system by fasting suggest that this peptide play a role in long-term regulation of feeding in fish. Thus, short-term periprandial changes in the expression of orexin occurred in fishes. Orexin expression in the brain increases 1 h before the scheduled mealtime in Mexican blind cavefish (Wall and Volkoff, 2013); and hypothalamic preproorexin expression peaks around mealtime, and decreases after feeding in Atlantic cod (Xu and Volkoff, 2007) and orange-spotted grouper (Yan et al., 2011). These results suggest that orexin may be a signal preceding food supply under scheduled feeding conditions. Recently, it has been shown that orexin-A enhances locomotor activity in goldfish (Nakamachi et al., 2014), and synchronizes locomotor activity in goldfish maintained under 24 L and fasting conditions (Nisembaum et al., 2014a), which allowed authors to suggest that orexin might mediate the locomotor activity entrainment by food schedule in this teleost.
Orexin system is a good target to investigate possible interactions among food intake regulators. Central administration of orexin increases the expression of hypothalamic NPY in goldfish (Volkoff and Peter, 2001b; Nisembaum et al., 2014a), and in the orange spotted grouper (Yan et al., 2011). Desensitizing the orexin system by treatment with high doses of orexin A results in a decrease in NPY-induced feeding (Volkoff and Peter, 2001b), while the blocking of NPY receptors reduces feeding induced by orexin A (Kojima et al., 2009). In addition, ICV co-administration of orexin-A and NPY results in a synergistic orexigenic effect in goldfish (Volkoff and Peter, 2001b; Volkoff et al., 2003). Thus, a functional interdependence may exist between orexin-A and NPY in the stimulation of food intake. On the other hand, ICV orexin-A treatment stimulates ghrelin mRNA 1 h post-injection in goldfish foregut. This agrees with the increase of diencephalic ghrelin induced by orexin-A in this teleost (Miura et al., 2007), and demonstrates that the interaction between these two peptides takes place not only into the hypothalamus, but also suggests a brain-gut communication (Unniappan et al., 2004; Nakamachi et al., 2006). Neuroanatomical evidences support the interactions above described among orexin-A and other appetite-regulating peptides, as NPY and ghrelin (Volkoff et al., 2003; Miura et al., 2007). Recent studies suggested a role of tyrosine hydroxylase in the regulation of food intake and locomotor activity in vertebrates (Wall and Volkoff, 2013). The increased mRNA expression levels of this enzyme induced by fasting or orexin in cavefish may suggest the involvement of catecholamines in the actions of orexin (Abott and Volkoff, 2011).
Melanin-Concentrating Hormone (MCH)
Melanin-concentrating hormone (MCH) characterized in chum salmon as a circulating-cyclic heptadecapeptide involved in color change (Kawauchi et al., 1983). MCH is mainly produced within the tuberal hypothalamus, stored in the pituitary neural lobe, and released under adaptation to a light-colored background (Cerdá-Reverter and Canosa, 2009). MCH is a potent orexigenic factor inducing weight gain in mammals (Qu et al., 1996). In fish, however, contradictory results were obtained, such as in goldfish (Matsuda et al., 2006c). Data reported in barfin flounder showed increased hypothalamic MCH expression after food deprivation (Takahasi et al., 2004) and enhanced somatic growth after white background adaptation (Yamanome et al., 2005). Transgenic medaka overexpressing MCH exhibit lightened body color, but development, growth, feeding behavior and reproduction do not remarkably differ from non-transgenic siblings (Kinoshita et al., 2001).
Conclusions
We aimed with this review to show readers the existing information about the way in which fish hypothalamus integrates information of metabolic, endocrine, and circadian nature to elicit a coordinated food intake response. Compared with the mammalian models, the available studies in fish are more limited resulting in the lack of knowledge in several important aspects of hypothalamic integration. Thus, we have almost no idea about how mechanisms governing food intake operate in the long-term since the available information in fish refers to mechanisms operating at short-term periods. The absence of knowledge is also relevant in terms of characterizing the hypothalamic specific nuclei involved in the process of integration of information as well as in the relationship among cells in those nuclei. In this way, almost all available studies in fish assessed the whole hypothalamus, and this may result in differences in the amount and type of neurons assessed. The large diversity of fish species is not sufficiently covered in the available studies, which in some cases (metabolic control) rely on a handful of species. The possible presence and functioning of amino acid sensing systems in fish, as well as the elucidation of signaling pathways linking activity of sensors with the effectors controlling homeostasis are also open questions demanding further research.
Even in the short-term mechanisms comparable to those known in mammals regarding the existence of neurons involved, presence of nutrient sensing systems and modulatory effects of hormones, the specific responses obtained are not identical to those observed in mammals. In some cases, these differences may relate to the limited available studies in fish precluding us to formulate clear hypothesis. Moreover, other factors might be involved, and we can only speculate about some of them. For instance, the activation of fatty acid sensing systems in mammalian hypothalamus relates to the thermogenic capacity of brown adipose tissue (Contreras et al., 2016), i.e., one of the mechanisms involved in thermoregulation that is not present in fish. Accordingly, the reduced energy expenditure in fish may relate to differential food intake regulation as suggested in a recent review (Van de Pol et al., 2017). A second reason might relate to the gene duplication present in actinopterygians resulting in multiple isoforms of neuropeptides, transporters, enzymes, etc. Accordingly, different subfunctions or different functions may attribute to different isoforms in fish, and this might explain some of the differential effects of hormones when comparing fish and mammals, but this has been evaluated only in a few cases. A third reason may relate to the fact that since fish live in a great variety of habitats they also display many species-specific adaptations such as those related to feeding behavior. Fish eating habits (carnivores, omnivores or herbivores) may be responsible of specific differences in gastrointestinal morphology and hormone functions, and this could be responsible (at least in part) of the differences observed when comparing responses among fish species. Finally, many fish species, including several of the models studied such as rainbow trout, are carnivorous, which is in contrast with the mammalian models studied so far (almost all of them omnivorus/herbivorous species). Accordingly, several of the differences in food intake regulation might relate to this fact. However, this is probably not so simple since the scarce data available in carnivorous mammals (Batchelor et al., 2011) also display differential responses compared with carnivorous fish.
The research carried out in the field in recent years provided evidence for several of the mechanisms involved, though certainly more research is needed, especially to ascertain the interactions among different regulatory mechanisms as well as to establish what are the intracellular common mechanisms through which the information is integrated to elicit a food intake response. Ongoing research in our groups, as well as in many others, will provide responses in the near future.
Author Contributions
All authors listed, have made substantial, direct and intellectual contribution to the work, and approved it for publication.
Funding
The authors acknowledge grants from Spanish Agencia Estatal de Investigación (AEI) and European Fund of Regional Development (FEDER) to MD (AGL2016-74857-C3-2-R), JC (AGL2016-74857-C3-3-R), and JS (AGL2016-74857-C3-1-R).
Conflict of Interest Statement
The authors declare that the research was conducted in the absence of any commercial or financial relationships that could be construed as a potential conflict of interest.
Acknowledgments
The authors acknowledge all present and past members of their research groups for their scientific and personal inputs throughout years of hard work.
References
Abott, M., and Volkoff, H. (2011). Thyrotropin releasing hormone (TRH) in goldfish (Carassius auratus): role in the regulation of feeding and locomotor behaviors and interactions with the orexin system and cocaine- and amphetamine regulated transcript (CART). Horm. Behav. 59, 236–245. doi: 10.1016/j.yhbeh.2010.12.008
Acosta-Galvan, G., Yi, C. X., van der Vliet, J., Jhamandas, J. H., Panula, P., Angeles-Castellanos, M., et al. (2011). Interaction between hypothalamic dorsomedial nucleus and the suprachiasmatic nucleus determines intensity of food anticipatory behavior. Proc. Natl. Acad. Sci. U.S.A. 108, 5813–5818. doi: 10.1073/pnas.1015551108
Aguilar, A. J., Conde-Sieira, M., López-Patiño, M. A., Míguez, J. M., and Soengas, J. L. (2011). In vitro leptin treatment of rainbow trout hypothalamus and hindbrain affects glucosensing and gene expression of neuropeptides involved in food intake regulation. Peptides 32, 232–240. doi: 10.1016/j.peptides.2010.11.007
Aguilar, A. J., Conde-Sieira, M., Polakof, S., Míguez, J. M., and Soengas, J. L. (2010). Central leptin treatment modulates brain glucosensing function and peripheral energy metabolism of rainbow trout. Peptides 31, 1044–1054. doi: 10.1016/j.peptides.2010.02.026
Agulleiro, M. J., Cortés, R., Fernández-Durán, B., Guillot, R., Navarro, S., Meimaridou, E., et al. (2013). Melanocortin 4 receptor becomes an ACTH receptor by coexpression of melanocortin receptor accessory protein 2. Mol. Endocrinol. 27, 1934–1945. doi: 10.1210/me.2013-1099
Agulleiro, M. J., Cortés, R., Leal, E., Ríos, D., Sánchez, E., and Cerdá-Reverter, J. M. (2014). Characterization, tissue distribution and regulation by fasting of the agouti family of peptides in the sea bass (Dicentrarchus labrax). Gen. Comp. Endocrinol. 205, 251–259. doi: 10.1016/j.ygcen.2014.02.009
Akash, G., Kaniganti, T., Tiwari, N. K., Subhedar, N. K., and Ghose, A. (2014). Differential distribution and energy status-dependent regulation of the four CART neuropeptide genes in the zebrafish brain. J. Comp. Neurol. 522, 2266–2285. doi: 10.1002/cne.23532
Albrecht, U. (2012). Timing to perfection: the biology of central and peripheral circadian clocks. Neuron 74, 246–260. doi: 10.1016/j.neuron.2012.04.006
Aldegunde, M., and Mancebo, M. (2006). Effects of neuropeptide Y on food intake and brain biogenic amines in the rainbow trout (Oncorhynchus mykiss). Peptides. 27, 719–727. doi: 10.1016/j.peptides.2005.09.014
Alderman, S. L., and Bernier, N. J. (2007). Localization of corticotropin-releasing factor, urotensin I, and CRF-binding protein gene expression in the brain of the zebrafish, Danio rerio. J. Comp. Neurol. 502, 783–793. doi: 10.1002/cne.21332
Aldman, G., and Holmgren, S. (1995). Intraduodenal fat and amino acids activate gallbladder motility in the rainbow trout, Oncorhynchus mykiss. Gen. Comp. Endocrinol. 100, 27–32. doi: 10.1006/gcen.1995.1128
Aluru, N., and Vijayan, M. M. (2009). Stress transcriptomics in fish: a role for genomic cortisol signaling. Gen. Comp. Endocrinol. 164, 142–150. doi: 10.1016/j.ygcen.2009.03.020
Anderson, E. J., Çakir, I., Carrington, S. J., Cone, R. D., Ghamari-Langroudi, M., Gillyard, T., et al. (2016). 60 YEARS OF POMC: regulation of feeding and energy homeostasis by α-MSH. J. Mol. Endocrinol. 56, T157–T174. doi: 10.1530/jme-16-0014
Angotzi, A. R., Stefansson, S. O., Nilsen, T. O., Øvrebø, J. I., Andersson, E., Taranger, G. L., et al. (2016). Identification of a novel leptin receptor duplicate in Atlantic salmon: expression analyses in different life stages and in response to feeding status. Gen. Comp. Endocrinol. 235, 108–119. doi: 10.1016/j.ygcen.2016.06.004
Anthonisen, E. H., Berven, L., Holm, S., Nygård, M., Nebb, H. I., and Grønning-Wang, L. M. (2010). Nuclear receptor liver X receptor is O-GlcNAc-modified in response to glucose. J. Biol. Chem. 285, 1607–1615. doi: 10.1074/jbc.M109.082685
Appelbaum, L., Wang, G., Yokogawa, T., Skariah, G. M., Smith, S. J., Mourrain, P., et al. (2010). Circadian and homeostatic regulation of structural synaptic plasticity in hypocretin neurons. Neuron 68, 87–98. doi: 10.1016/j.neuron.2010.09.006
Archer, A., Laurencikiene, J., Ahmed, O., Steffensen, K. R., Parini, P., Gustafsson, J.-A., et al. (2014). Skeletal muscle as a target of LXR agonist after long-term treatment: focus on lipid homeostasis. Am. J. Physiol. Endocrinol. Metab. 306, E494–E502. doi: 10.1152/ajpendo.00410.2013
Asher, G., and Sassone-Corsi, P. (2015). Time for food: the intimate interplay between nutrition, metabolism, and the circadian clock. Cell 161, 84–92. doi: 10.1016/j.cell.2015.03.015
Baird, J.-P., Grill, H. J., and Kaplan, J. M. (1997). Intake suppression after hepatic portal glucose infusion: all-or-none effect and its temporal threshold. Am. J. Physiol. Regul. Integr. Comp. Physiol. 272, R1454–R1460.
Barton, B. A. (2002). Stress in fishes: a diversity of responses with particular reference to changes in circulating corticosteroids. Integr. Comp. Biol. 42, 517–525. doi: 10.1093/icb/42.3.517
Batchelor, D. J., Al-Rammahi, M., Moran, A. W., Brand, J. G., Li, X., Haskins, M., et al. (2011). Sodium/glucose cotransporter-1, sweet receptor, and disaccharidase expression in the intestine of the domestic dog and cat: two species of different dietary habit. Am. J. Physiol. Regul. Integr. Comp. Physiol. 300, R67–R75. doi: 10.1152/ajpregu.00262.2010
Batten, T. F. C., and Cambre, M. L. (1989). Calcitonin gene-related peptide-like immunoreactive fibres innervating the hypothalamic inferior lobes of teleost fishes. Neurosci. Lett. 98, 1–7. doi: 10.1016/0304-3940(89)90363-7
Belle, M. D. C., Hughes, A. T. L., Bechtold, D. A., Cunningham, P., Pierucci, M., Burdakov, D., et al. (2014). Acute suppressive and long-term phase modulation actions of orexin on the mammalian circadian clock. J. Neurosci. 34, 3607–3621. doi: 10.1523/jneurosci.3388-13.2014
Benoit, S. C., Kemp, C. J., Elias, C. F., Abplanalp, W., Herman, J. P., Migrenne, S., et al. (2009). Palmitic acid mediates hypothalamic insulin resistance by altering PKC-O subcellular localization in rodents. J. Clin. Invest. 119, 2577–2589. doi: 10.1172/JCI36714
Bernier, N. J. (2006). The corticotrophin-releasing factor system as mediator of the appetite-suppressing effects of stress in fish. Gen. Comp. Endocrinol. 144, 45–55 doi: 10.1016/j.ygcen.2005.11.016
Bernier, N. J., and Peter, R. E. (2001). The hypothalamic-pituitary-interrenal axis and the control of food intake in teleost fish. Comp. Biochem. Physiol. 129, 639–644. doi: 10.1016/S1096-4959(01)00360-8
Berthoud, H. R. (2002). Multiple neural systems controlling food intake and body weight. Neurosci. Biobehav. Rev. 26, 393–428. doi: 10.1016/S0149-7634(02)00014-3
Berthoud, H.-R., and Morrison, C. (2008). The brain, appetite, and obesity. Annu. Rev. Psychol. 59, 55–92. doi: 10.1146/annurev.psych.59.103006.093551
Betancor, M. B., Howarth, F. J. E., Glencross, B. D., and Tocher, D. R. (2014). Influence of dietary docosahexanoic acid in combination with other long-chain polyunsaturated fatty acids on expression of biosynthesis genes and phospholipid fatty acid compositions in tissues of post-smolt Atlantic salmon (Salmo salar). Comp. Biochem. Physiol. B. 172, 74–89. doi: 10.1016/j.cbpb.2014.04.007
Blanco, A. M., Gómez-Boronat, M., Redondo, I., Valenciano, A. I., and Delgado, M. J. (2016a). Periprandial changes and effects of short- and long-term fasting on ghrelin, GOAT, and ghrelin receptors in goldfish (Carassius auratus). J. Comp. Physiol. B 186, 727–738. doi: 10.1007/s00360-016-0986-0
Blanco, A. M., Sánchez-Bretaño, A., Delgado, M. J., and Valenciano, A. I. (2016b). Brain mapping of ghrelin O-acyltransferase in goldfish (Carassius auratus): novel roles for the ghrelinergic system in fish? Anat. Rec. 299, 748–758. doi: 10.1002/ar.23346
Blouet, C., and Schwartz, G. J. (2010). Hypothalamic nutrient sensing in the control of energy homeostasis. Behav. Brain Res. 209, 1–12. doi: 10.1016/j.bbr.2009.12.024
Bonacic, K., Martínez, A., Martín-Robles, A. J., Muñoz-Cueto, J. A., and Morais, S. (2015). Characterization of seven cocaine- and amphetamine-regulated transcripts (CARTs) differentially expressed in the brain and peripheral tissues of Solea senegalensis (Kaup). Gen. Comp. Endocrinol. 224, 260–272. doi: 10.1016/j.ygcen.2015.08.017
Cakir, I., Perello, M., Lansari, O., Messier, N. J., Vaslet, C. A., and Nillni, E. A. (2009). Hypothalamic Sirt1 regulates food intake in a rodent model system. PLoS ONE 4:e8322. doi: 10.1371/journal.pone.0008322
Capilla, E., Médale, F., Navarro, I., Panserat, S., Vachot, C., Kaushik, S., et al. (2003). Muscle insulin binding and plasma levels in relation to liver glucokinase activity, glucose metabolism and dietary carbohydrates in rainbow trout. Reg. Peptides 110, 123–132. doi: 10.1016/S0167-0115(02)00212-4
Caruso, M. A., Kittilson, J. D., Raine, J., and Sheridan, M. A. (2008). Rainbow trout (Oncorhynchus mykiss) possess two insulin-encoding mRNAs that are differentially expressed. Gen. Comp. Endocrinol. 155, 695–704. doi: 10.1016/j.ygcen.2007.09.006
Castro, C., Corraze, G., Pérez-Jimenez, A., Larroquet, L., Cluzeaud, M., Panserat, S., et al. (2015). Dietary carbohydrate and lipid source affect cholesterol metabolism of European sea bass (Dicentrarchus labrax) juveniles. Br. J. Nutr. 114, 1584–1593. doi: 10.1017/S0007114515003360
Cerdá-Reverter, J. M., Agulleiro, M. J., Guillot, R., Sánchez, E., Ceinos, R., and Rotllant, J. (2011). Fish melanocortin system. Eur. J. Pharm. 660, 53–60. doi: 10.1016/j.ejphar.2010.10.108
Cerdá-Reverter, J. M., Anglade, I., Martínez-Rodríguez, G., Mazurais, D., Muñoz-Cueto, J. A., Carrillo, M., et al. (2000b). Characterization of neuropeptide Y expression in the brain of a perciform fish, the sea bass (Dicentrarchus labrax). J. Chem. Neuroanat. 19, 197–210. doi: 10.1016/S0891-0618(00)00063-6
Cerdá-Reverter, J. M., and Canosa, L. F. (2009). “Neuroendocrine systems of the fish brain,” in Fish Neuroendocrinology, eds N. J. Bernier, G. J. Van der Kraak, A. P. Farrell, and C. J. Brauner (Amsterdam: Elsevier), 3–74.
Cerdá-Reverter, J. M., Haitina, T., Schioth, H. B., and Peter, R. E. (2005). Gene structure of the goldfish agouti-signaling protein: a putative role in the dorsal-ventral pigment pattern of fish. Endocrinology 146, 1597–1610. doi: 10.1210/en.2004-1346
Cerdá-Reverter, J. M., and Larhammar, D. (2000). Neuropeptide Y family of peptides: structure, anatomical expression, function and molecular evolution. Biochem. Cell Biol. 78, 371–392. doi: 10.1139/o00-004
Cerdá-Reverter, J. M., Martínez-Rodríguez, G., Zanuy, S., Carrillo, M., and Larhammar, D. (2000a). Molecular evolution of the neuropeptide Y (NPY) family of peptides: cloning of three NPY-related peptides from the sea bass (Dicentrarchus labrax). Regul. Pept. 95, 25–34. doi: 10.1016/S0167-0115(00)00132-4
Cerdá-Reverter, J. M., Ling, M. K., Schiöth, H. B., and Peter, R. E. (2003c). Molecular cloning, characterization and brain mapping of the melanocortin 5 receptor in the goldfish. J. Neurochem. 87, 1354–1367. doi: 10.1046/j.1471-4159.2003.02107.x
Cerdá-Reverter, J. M., and Peter, R. E. (2003). Endogenous melanocortin antagonist in fish: structure, brain mapping, and regulation by fasting of the goldfish agouti-related protein gene. Endocrinology 144, 3552–4561. doi: 10.1210/en.2003-0453
Cerdá-Reverter, J. M., Ringholm, A., Schiöth, H. B., and Peter, R. E. (2003b). Molecular cloning, pharmacological characterization, and brain mapping of the melanocortin 4 receptor in the goldfish: involvement in the control of food intake. Endocrinology 144, 2336–2349. doi: 10.1210/en.2002-0213
Cerdá-Reverter, J. M., Schiöth, H. B., and Peter, R. E. (2003a). The central melanocortin system regulates food intake in goldfish. Regul. Peptides 115, 101–113. doi: 10.1016/S0167-0115(03)00144-7
Cerdá-Reverter, J. M., Sorbera, L. A., Carrillo, M., and Zanuy, S. (1999). Energetic dependence of NPY-induced LH secretion in a teleost fish (Dicentrarchus labrax). Am. J. Physiol. Regul. Integr. Comp. Physiol. 277, R1627–R1634.
Chabbi, A., and Ganesh, C. B. (2016). Neuroanatomical evidence for the involvement of β-endorphin during reproductive stress response in the fish Oreochromis mossambicus. J. Chem. Neuroanat. 77, 161–168. doi: 10.1016/j.jchemneu.2016.07.002
Challet, E. (2013). Circadian clocks, food intake, and metabolism. Prog. Mol. Biol. Transl. Sci. 119, 105–135. doi: 10.1016/B978-0-12-396971-2.00005-1
Challet, E. (2015). Keeping circadian time with hormones. Diabetes Obes. Metab. 17(Suppl. 1), 76–83. doi: 10.1111/dom.12516
Chang, H.-C., and Guarente, L. (2013). SIRT1 mediates central circadian control in the SCN by a mechanism that decays with aging. Cell 153, 1448–1460. doi: 10.1016/j.cell.2013.05.027
Chen, H., Huang, H., Chen, X., Deng, S., Zhu, C., Huang, H., et al. (2016). Structural and functional characterization of neuromedin S in the teleost fish, zebrafish (Danio rerio). Comp. Biochem. Physiol. B. 191, 76–83. doi: 10.1016/j.cbpb.2015.09.007
Chen, T., Tang, Z., Yan, A., Li, W., and Lin, H. (2008). Molecular cloning and mRNA expression analysis of two GH secretagogue receptor transcripts in orange-spotted grouper (Epinephelus coioides). J. Endocrinol. 199, 253–265. doi: 10.1677/JOE-08-0325
Chisada, S.-I., Kurokawa, T., Murashita, K., Rønnestad, I., Taniguchi, Y., Toyoda, A., et al. (2014). Leptin receptor-deficient (knockout) medaka, Oryzias latipes, show chronical up-regulated levels of orexigenic neuropeptides, elevated food intake and stage specific effects on growth and fat allocation. Gen. Comp. Endocrinol. 195, 9–20. doi: 10.1016/j.ygcen.2013.10.008
Conde-Sieira, M., Aguilar, A. J., López-Patiño, M. A., Míguez, J. M., and Soengas, J. L. (2010a). Stress alters food intake and glucosensing response in hypothalamus, hindbrain, liver, and Brockmann bodies of rainbow trout. Physiol. Behav. 101, 483–493. doi: 10.1016/j.physbeh.2010.07.016
Conde-Sieira, M., Agulleiro, M. J., Aguilar, A. J., Míguez, J. M., Cerdá-Reverter, J. M., and Soengas, J. L. (2010b). Effect of different glycaemic conditions on gene expression of neuropeptides involved in control of food intake in rainbow trout; interaction with stress. J. Exp. Biol. 213, 3858–3865. doi: 10.1242/jeb.048439
Conde-Sieira, M., Bonacic, K., Velasco, C., Valente, L. M. P., Morais, S., and Soengas, J. L. (2015). Hypothalamic fatty acid sensing in Senegalese sole (Solea senegalensis): response to long-chain saturated, monounsaturated, and polyunsaturated (n-3) fatty acids. Am. J. Physiol. Regul. Integr. Comp. Physiol. 309, R1521–R1531. doi: 10.1152/ajpregu.00386.2015
Conde-Sieira, M., Librán-Pérez, M., López Patiño, M. A., Míguez, J. M., and Soengas, J. L. (2011). CRF treatment indices a readjustment in glucosensing capacity in the hypothalamus and hindbrain of rainbow trout. J. Exp. Biol. 214, 3887–3894. doi: 10.1242/jeb.061564
Conde-Sieira, M., Librán-Pérez, M., López Patiño, M. A., Soengas, J. L., and Míguez, J. M. (2012a). Melatonin treatment alters glucosensing capacity and mRNA expression levels of peptides related to food intake control in rainbow trout hypothalamus. Gen. Comp. Endocrinol. 178, 131–138. doi: 10.1016/j.ygcen.2012.04.011
Conde-Sieira, M., López-Patiño, M. A., Míguez, J. M., and Soengas, J. L. (2012b). Glucosensing capacity in rainbow trout liver displays day-night variations possibly related to melatonin action. J. Exp. Biol. 215, 3112–3119. doi: 10.1242/jeb.069740
Contreras, C., Nogueiras, R., Diéguez, C., Medina-Gómez, G., and López, M. (2016). Hypothalamus and thermogenesis: heating the BAT, browning the WAT. Mol. Cell Endocrinol. 438, 107–115. doi: 10.1016/j.mce.2016.08.002
Coomans, C. E., Lucassen, E. A., Kooijman, S., Fifel, K., Deboer, D., Rensen, P. C. N., et al. (2015). Plasticity of circadian clocks and consequences for metabolism. Diab. Obes. Metab. 17(Suppl. 1), 65–75. doi: 10.1111/dom.12513
Dattolo, T., Coomans, C. P., Van diepen, H. C., Patton, D. F., Power, S., Antle, M. C., et al. (2016). A neural activity in the suprachiasmatic circadian clock of nocturnal mice anticipating a daytime meal. Neuroscience 315, 91–103. doi: 10.1016/j.neuroscience.2015.12.014
Davis, D. A., Lazo, J. P., and Arnold, C. R. (1999). Response of juvenile red drum (Sciaenops ocellatus) to practical diets supplemented with medium chain triglycerides. Fish Physiol. Biochem. 21, 235–247. doi: 10.1023/A:1007836612376
De Backer, I., Hussain, S. S., Bloom, S. R., and Gardiner, J. V. (2016). Insights into the role of neuronal glucokinase. Am. J. Physiol. Endocrinol. Metab. 311, E42–E55. doi: 10.1152/ajpendo.00034.2016
De Pedro, N., Alonso-Gomez, A. L., Gancedo, B., Delgado, M. J., and Alonso-Bedate, M. (1993). Role of corticotropin-releasing factor (CRF) as a food intake regulator in goldfish. Physiol. Behav. 53, 517–520. doi: 10.1016/0031-9384(93)90146-7
De Pedro, N., Alonso-Gomez, A. L., Gancedo, B., Valenciano, A. I., Delgado, M. J., and Alonso-Bedate, M. (1997). Effect of α-helical-CRF (9–41) on feeding in goldfish: involvement of cortisol and catecholamines. Behav. Neurosci. 111, 398–403. doi: 10.1037/0735-7044.111.2.398
De Pedro, N., Delgado, M. J., and Alonso-Bedate, M. (1995). Central administration of β-endorphin increases food intake in goldfish: pretreatment with the opioid antagonist naloxone. Regul. Peptides 55, 189–195. doi: 10.1016/0167-0115(95)92305-V
De Pedro, N., Martínez-Álvarez, R., and Delgado, M. J. (2006). Acute and chronic leptin reduces food intake and body weight in goldfish (Carassius auratus). J. Endocrinol. 188, 513–520. doi: 10.1677/joe.1.06349
Dibner, C., Schibler, U., and Albrecht, U. (2010). The mammalian circadian timing system: organization and coordination of central and peripheral clocks. Annu. Rev. Physiol. 72, 517–549. doi: 10.1146/annurev-physiol-021909-135821
Diéguez, C., Vazquez, M. J., Romero, A., López, M., and Nogueiras, R. (2011). Hypothalamic control of lipid metabolism: focus on leptin, ghrelin and melanocortins. Neuroendocrinology 94, 1–11. doi: 10.1159/000328122
Díez-Sampedro, A., Hirayama, B. A., Osswald, C., Gorboulev, V., Baumgarten, K., Volk, C., et al. (2003). A glucose sensor hiding in a family of transporters. Proc. Natl. Acad. Sci. U.S.A. 100, 11753–11758. doi: 10.1073/pnas.1733027100
Douglass, J., McKinzie, A. A., and Couceyro, P. (1995). PCR differential display identifies a rat brain mRNA that is transcriptionally regulated by cocaine and amphetamine. J. Neurosci. 15, 2471–2481.
Doyon, C., Gilmour, K. M., Trudeau, V. L., and Moon, T. W. (2003). Corticotropin-releasing factor and neuropeptide Y mRNA levels are elevated in the preoptic area of socially subordinate rainbow trout. Gen. Comp. Endocrinol. 133, 260–271. doi: 10.1016/S0016-6480(03)00195-3
Duca, F. A., and Yue, J. T. Y. (2014). Fatty acid sensing in the gut and the hypothalamus: in vivo and in vitro perspectives. Mol. Cell Endocrinol. 397, 23–33. doi: 10.1016/j.mce.2014.09.022
Efeyan, A., Comb, W. C., and Sabatini, D. M. (2015). Nutrient sensing mechanisms and pathways. Nature 517, 302–310. doi: 10.1038/nature14190
Egan, R. J., Bergner, C. L., Hart, P. C., Cachat, J. M., Canavello, P. R., Elegante, M. F., et al. (2009). Understanding behavioral and physiological phenotypes of stress and anxiety in zebrafish. Behav. Brain Res. 205, 38–44. doi: 10.1016/j.bbr.2009.06.022
Einarsson, S., Davies, P. S., and Talbot, C. (1997). Effect of exogenous cholecystokinin on the discharge of the gallbladder and the secretion of trypsin and chymotrypsin from the pancreas of the Atlantic salmon, Salmo salar L. Comp. Biochem. Physiol. C. 117, 63–67. doi: 10.1016/s0742-8413(96)00226-5
Elias, C. F., Lee, C., Kelly, J., Aschkenasi, C., Ahima, R. S., Couceyro, P. R., et al. (1998). Leptin activates hypothalamic CART neurons projecting to the spinal cord. Neuron 21, 1375–1385. doi: 10.1016/S0896-6273(00)80656-X
Ellis, C., Moar, K. M., Logie, T. J., Ross, A. W., Morgan, P. J., and Mercer, J. G. (2008). Diurnal profiles of hypothalamic energy balance gene expression with photoperiod manipulation in the Siberian hamster, Phodopus sungorus. Am. J. Physiol. Regul. Integr. Comp. Physiol. 294, R1148–R1153. doi: 10.1152/ajpregu.00825.2007
Evans, J. A., and Gorman, M. R. (2016). In synch but not in step: circadian clock circuits regulating plasticity in daily rhythms. Neuroscience 320, 259–280. doi: 10.1016/j.neuroscience.2016.01.072
Evans, M. L., McCrimmon, R. J., Flanagan, D. E., Keshavarz, T., Fan, X., McNay, E., et al. (2004). Hypothalamic ATP-sensitive K+ channels play a key role in sensing hypoglycemia and triggering counter-regulatory epinephrine and glucagon responses. Diabetes 53, 2542–2551. doi: 10.2337/diabetes.53.10.2542
Facciolo, R. M., Crudo, M., Zizza, M., Giusi, G., and Canonaco, M. (2011). Feeding behaviors and ORXR–β-GABAAR subunit interactions in Carassius auratus. Neurotoxicol. Teratol. 33, 641–650. doi: 10.1016/j.ntt.2011.09.008
Feillet, C. A., Mendoza, J., Albrecht, U., Pevet, P., and Challet, E. (2008). Forebrain oscillators ticking with different clock hands. Mol. Cell Neurosci. 37, 209–221. doi: 10.1016/j.mcn.2007.09.010
Feliciano, A., Vivas, Y., De Pedro, N., Delgado, M. J., Velarde, E., and Isorna, E. (2011). Feeding time synchronizes clock gene rhythmic expression in brain and liver of goldfish (Carassius auratus). J. Biol. Rhythms 26, 24–33. doi: 10.1177/0748730410388600
Feng, K., Zhang, G.-R., Wei, K.-J., and Xiong, B.-X. (2013). Molecular cloning, tissue distribution, and ontogenetic expression of ghrelin and regulation of expression by fasting and refeeding in the grass carp (Ctenopharyngodon idellus). J. Exp. Zool. 319, 202–212. doi: 10.1002/jez.1784
Feng, K., Zhang, G. R., Wei, K. J., Xiong, B. X., Liang, T., and Ping, H. C. (2012). Molecular characterization of cholecystokinin in grass carp (Ctenopharyngodon idellus): cloning, localization, developmental profile, and effect of fasting and refeeding on expression in the brain and intestine. Fish Physiol. Biochem. 38, 1825–1834. doi: 10.1007/s10695-012-9679-0
Figueiredo-Silva, A. C., Saravanan, S., Schrama, J. W., Kaushik, S., and Geurden, I. (2012b). Macronutrient-induced differences in food intake relate with hepatic oxidative metabolism and hypothalamic regulatory neuropeptides in rainbow trout (Oncorhynchus mykiss). Physiol. Behav. 106, 499–505. doi: 10.1016/j.physbeh.2012.03.027
Figueiredo-Silva, A. C., Saravanan, S., Schrama, J. W., Panserat, S., Kaushik, S., and Geurden, I. (2013). A comparative study of the metabolic response in rainbow trout and Nile tilapia to changes in dietary macronutrient composition. Br. J. Nutr. 109, 816–826. doi: 10.1017/S000711451200205X
Figueiredo-Silva, A. C., Kaushik, S., Terrier, F., Schrama, J. W., Médale, F., and Geurden, I. (2012a). Link between lipid metabolism and voluntary food intake in rainbow trout fed coconut oil rich in medium-chain TAG. Br. J. Nutr. 107, 1714–1725. doi: 10.1017/S0007114511004739
Fioramonti, X., Conti,é, S., Song, Z., Routh, V. H., Lorsignol, A., and Pénicaud, L. (2007). Characterization of glucosensing neuron subpopulations in the arcuate nucleus. Integration in neuropeptide Y and pro-opio melanocortin networs? Diabetes 56, 1219–1227. doi: 10.2337/db06-0567
Fioramonti, X., Lorsignol, A., Taupignon, A., and Pénicaud, L. (2004). A new ATP-sensitive K+ channel-independent mechanism is involved in glucose-excited neurons of mouse arcuate nucleus. Diabetes 53, 2767–2775. doi: 10.2337/diabetes.53.11.2767
Forlano, P. M., and Cone, R. D. (2007). Conserved neurochemical pathways involved in hypothalamic control of energy homeostasis. J. Comp. Neurol. 505, 235–248. doi: 10.1002/cne.21447
Forsman, A., and Ruohonen, K. (2009). Dynamics of protein and lipid intake regulation of rainbow trout studied with a wide lipid range of encapsulated diets and self-feeders. Physiol. Behav. 96, 85–90. doi: 10.1016/j.physbeh.2008.08.018
Fox, B. K., Breves, J. P., Hirano, T., and Grau, E. G. (2009). Effects of short- and long-term fasting on plasma and stomach ghrelin, and the growth hormone/insulin-like growth factor I axis in the tilapia, Oreochromis mossambicus. Domest. Anim. Endocrinol. 37, 1–11. doi: 10.1016/j.domaniend.2009.01.001
Fromentin, G., Darcel, N., Chaumontet, C., Marsset-Baglieri, A., Nadkarni, N., and Tomé, D. (2012). Peripheral and central mechanisms involved in the control of food intake by dietary amino acids and proteins. Nutr. Res. Rev. 25, 29–39. doi: 10.1017/S0954422411000175
Gao, S., Moran, T. H., Lopaschuk, G. D., and Butler, A. A. (2013). Hypothalamic malonyl-CoA and the control of food intake. Physiol. Behav. 122, 17–24. doi: 10.1016/j.physbeh.2013.07.014
Gélineau, A., Corraze, G., Boujard, T., Larroquet, L., and Kaushik, S. (2001). Relation between dietary lipid level and voluntary feed intake, growth, nutrient gain, lipid deposition and hepatic lipogenesis in rainbow trout. Reprod. Nutr. Dev. 41, 487–503. doi: 10.1051/rnd:2001103
Gesto, M., López-Patiño, M. A., Hernández, J., Soengas, J. L., and Míguez, J. M. (2013). The response of brain serotonergic and dopaminergic systems to an acute stressor in rainbow trout: a time course study. J. Exp. Biol. 216, 4435–4442. doi: 10.1242/jeb.091751
Gesto, M., López-Patiño, M. A., Hernández, J., Soengas, J. L., and Míguez, J. M. (2015). Gradation of the stress response in rainbow trout exposed to stressors of different severity: the role of brain serotonergic and dopaminergic systems. J. Neuroendocrinol. 27, 131–141. doi: 10.1111/jne.12248
Geurden, I., Gondouin, E., Rimbach, M., Koppe, W., Kaushik, S., and Boujard, T. (2006). The evaluation of energy intake adjustments and preferences in juvenile rainbow trout fed increasing amounts of lipid. Physiol. Behav. 88, 325–332. doi: 10.1016/j.physbeh.2006.03.033
Gomes, A. S., Olderbakk Jordal, A.-E., Olsen, K., Harboe, T., Power, D. M., and Ronnestad, I. (2015). Neuroendocrine control of appetite in Atlantic halibut (Hippoglossus hippoglossus): changes during metamorphosis and effects of feeding. Comp. Biochem. Physiol. A. 183, 116–125. doi: 10.1016/j.cbpa.2015.01.009
Gomez-Pinilla, F., and Ying, Z. (2010). Differential effects of exercise and dietary docosahexanoic acid on molecular systems associated with control of allostasis in the hypothalamus and hippocampus. Neuroscience 168, 130–137. doi: 10.1016/j.neuroscience.2010.02.070
Gong, N., and Björnsson, B. T. (2014). Leptin signaling in the rainbow trout central nervous system is modulated by a truncated leptin receptor isoform. Endocrinology 155, 2445–2455. doi: 10.1210/en.2013-2131
Gong, N., Johansson, M., and Björnsson, B. T. (2016b). Impaired central leptin signaling and sensitivity in rainbow trout with high muscle adiposity. Gen. Comp. Endocrinol. 235, 48–56. doi: 10.1016/j.ygcen.2016.06.013
Gong, N., Jönsson, E., and Björnsson, B. T. (2016a). Acute anorexigenic action of leptin in rainbow trout is mediated by the hypothalamic Pi3k pathway. J. Mol. Endocrinol. 56, 227–238. doi: 10.1530/JME-15-0279
González, J. A., Reimann, F., and Burdakov, D. (2009). Dissociation between sensing and metabolism of glucose in sugar sensing neurones. J. Physiol. 587, 41–48. doi: 10.1113/jphysiol.2008.163410
Gorissen, M., and Flik, G. (2014). Leptin in teleostean fish, towards the origins of leptin physiology. J. Chem. Neuroanat. 61–62, 200–206. doi: 10.1016/j.jchemneu.2014.06.005
Greco, J. A., Oosterman, J. E., and Belsham, D. D. (2014). Differential effects of omega-3 fatty acid docosahexanoic acid and palmitate on the circadian transcriptional profile of clock genes in immortalized hypothalamic neurons. Am. J. Physiol. Regul. Integr. Comp. Physiol. 307, R1049–R1060. doi: 10.1152/ajpregu.00100.2014
Guilding, C., Hughes, A. T., Brown, T. M., Namvar, S., and Piggins, H. D. (2009). A riot of rhythms: neuronal and glial circadian oscillators in the mediobasal hypothalamus. Mol. Brain. 2:28. doi: 10.1186/1756-6606-2-28
Guillaumond, F., Dardente, H., Giguère, V., and Cermakian, N. (2005). Differential control of Bmal1 circadian transcription by REV-ERB and ROR nuclear receptors. J. Biol. Rhythms 20, 391–400. doi: 10.1177/0748730405277232
Guillot, R., Cortés, R., Navarro, S., Mischitelli, M., García-Herranz, V., Sánchez, E., et al. (2016). Behind melanocortin antagonist overexpression in the zebrafish brain: a behavioral and transcriptomic approach. Horm Behav. 82, 87–100. doi: 10.1016/j.yhbeh.2016.04.011
Gutiérrez, J., and Plisetskaya, E. M. (1994). “Peptide receptor assay: insulin receptors” in Biochemistry and Molecular Biology of Fishes, Vol. 3, eds P. W. Hochachka and T. P. Mommsen (Amsterdam: Elsevier), 429–444.
Hahn, T. M., Breininger, J. F., Baskin, D. G., and Schwartz, M. W. (1998). Coexpression of Agrp and NPY in fasting-activated hypothalamic neurons. Nat. Neurosci. 1, 271–272. doi: 10.1038/1082
Hasebe, M., Kanda, S., and Oka, Y. (2016). Female specific glucose-sensitivity of GhRH neurons leads to sexually dimorphic inhibition of reproduction in medaka. Endocrinology 157, 4318–4329. doi: 10.1210/en.2016-1352
Heeley, N., and Blouet, C. (2016). Central amino acid sensing in the control of feeding behavior. Front. Endocrinol. 7:148. doi: 10.3389/fendo.2016.00148
Henry, B. A., Rao, A., Ikenasio, B. A., Mountjoy, K. G., Tilbrook, A. J., and Clarke, I. J. (2001). Differential expression of cocaine- and amphetamine-regulated transcript and agouti-related protein in chronically food-restricted sheep. Brain. Res. 918, 40–50. doi: 10.1016/S0006-8993(01)02918-3
Henry, B. A., Tilbrook, A. J., Dunshea, F. R., Rao, A., Blache, D., Martin, G. B., et al. (2000). Long-term alterations in adiposity affect expression of melanin concentrating hormone and enkephalin but not proopiomelanocortin in the hypothalamus of ovarectomized ewes. Endocrinology 141, 1506–1514. doi: 10.1210/endo.141.4.7434
Hernández-Pérez, J., Míguez, J. M., Librán-Pérez, M., Otero-Rodiño, C., Naderi, F., Soengas, J. L., et al. (2015). Daily rhythms in activity and mRNA abundance of enzymes involved in glucose and lipid metabolism in liver of rainbow trout, Oncorhynchus mykiss. Influence of light and food availability. Chronobiol. Int. 32, 1391–1408. doi: 10.3109/07420528.2015.1100633
Herrera Moro Chao, D., Argmann, C., Van Eijk, M., Boot, R. G., Ottenhoff, R., Van Roomen, C., et al. (2016). Impact of obesity on taste receptor expression in extra-oral tissues: emphasis on hypothalamus and brainstem. Sci. Rep. 6:29094. doi: 10.1038/srep29094
Hevrøy, E. M., Azpeleta, C., Shimizu, M., Lanzén, A., Kaiya, H., Espe, M., et al. (2011). Effects of short-term starvation on ghrelin, GH-IGF system, and IGF-binding proteins in Atlantic salmon. Fish Physiol. Biochem. 37, 217–232. doi: 10.1007/s10695-010-9434-3
Hevroy, E. M., Waagbo, R., Torstensen, B. E., Takle, H., Stubhaug, I., Jorgensen, S. M., et al. (2012). Ghrelin is involved in voluntary anorexia in Atlantic salmon raised at elevated sea temperatures. Gen. Comp. Endocrinol. 175, 118–134. doi: 10.1016/j.ygcen.2011.10.007
Himick, B. A., and Peter, R. E. (1994). CCK/gastrin-like immunoreactivity in brain and gut, and CCK suppression of feeding in goldfish. Am. J. Physiol. Regul. Integr. Comp. Physiol. 267, R841–R851.
Horvath, T. L., Bechmann, I., Naftolin, F., Kalra, S. P., and Leranth, C. (1997). Heterogeneity in the neuropeptide Y-containing neurons of the rat arcuate nucleus: GABAergic and non-GABAergic subpopulations. Brain Res. 756, 283–286. doi: 10.1016/S0006-8993(97)00184-4
Hoskins, L. J., and Volkoff, H. (2012). The comparative endocrinology of feeding in fish: insights and challenges. Gen. Comp. Endocrinol. 176, 327–335. doi: 10.1016/j.ygcen.2011.12.025
Hu, Z., Cha, S. H., Chohnan, S., and Lane, M. D. (2011). Hypothalamic malonyl-CoA as a mediator of feeding behavior. Proc. Natl. Acad. Sci. U.S.A. 100, 12624–12629. doi: 10.1073/pnas.1834402100
Huising, M. O., Geven, E. J. W., Kruiswijk, C. P., Nabuurs, S. B., Stolte, E. H., Spanings, F. A. T., et al. (2006). Increased leptin expression in common carp (Cyprinus carpio) after food intake but not after fasting or feeding to satiation. Endocrinology 147, 5786–5797. doi: 10.1210/en.2006-0824
Idda, M. L., Bertolucci, C., Vallone, D., Gothilf, Y., Sánchez-Vázquez, F. J., and Foulkes, N. S. (2012). Circadian clocks: lessons from fish. Prog. Brain Res. 199, 41–57. doi: 10.1016/B978-0-444-59427-3.00003-4
Isorna, E., de Pedro, N., Valenciano, A. I., Alonso-Gómez, A. L., and Delgado, M. J. (2017). Interplay between the endocrine and circadian systems in fishes. J. Endocrinol. 232, R141–R159. doi: 10.1530/joe-16-0330
Ji, W., Ping, H.-C., Wei, K.-J., Zhang, G.-R., Shi, Z.-C., Yang, R. B., et al. (2015). Ghrelin, neuropeptide Y (NPY) and cholecystokinin (CCK) in blunt snout bream (Megalobrama amblycephala): cDNA cloning, tissue distribution and mRNA expression changes responding to fasting and refeeding. Gen. Comp. Endocrinol. 223, 108–119. doi: 10.1016/j.ygcen.2015.08.009
Johansen, S. J. S., Ekli, M., and Jobling, M. (2002). Is there lipostatic regulation of feed intake in Atlantic salmon Salmo salar L.? Aquacult. Res. 33, 515–524. doi: 10.1046/j.1365-2109.2002.00736.x
Johansen, S. J. S., Sveier, H., and Jobling, M. (2003). Lipostatic regulation of feed intake in Atlantic salmon Salmo salar L. defending adiposity at the expense of growth? Aquacult. Res. 34, 317–331. doi: 10.1046/j.1365-2109.2003.00821.x
Johansson, M., and Björnsson, B. T. (2015). Elevated plasma leptin levels of fasted rainbow trout decrease rapidly in response to feed intake. Gen. Comp. Endocrinol. 214, 24–29. doi: 10.1016/j.ygcen.2015.02.020
Jönsson, A. C., Holmgren, S., and Holstein, B. (1987). Gastrin/CCK-like immunoreactivity in endocrine cells and nerves in the gastrointestinal tract of the cod, Gadus morhua, and the effect of peptides of the gastrin/CCK family on cod gastrointestinal smooth muscle. Gen. Comp. Endocrinol. 66, 190–202. doi: 10.1016/0016-6480(87)90267-X
Jönsson, E. (2013). The role of ghrelin in energy balance regulation in fish. Gen. Comp. Endocrinol. 187, 79–85. doi: 10.1016/j.ygcen.2013.03.013
Jönsson, E., Forsman, A., Einarsdottir, I. E., Kaiya, H., Ruohonen, K., and Björnsson, B. T. (2007). Plasma ghrelin levels in rainbow trout in response to fasting, feeding and food composition, and effects of ghrelin on voluntary food intake. Comp. Biochem. Physiol. A 147, 1116–1124. doi: 10.1016/j.cbpa.2007.03.024
Jönsson, E., and Holmgren, S. (2012). “The endocrine system of the gut,” in Encyclopedia of Fish Physiology: From Gene to Environment, ed A. P. Farrell (Amsterdam: Elsevier), 1341–1347.
Jönsson, E., Kaiya, H., and Björnsson, B. T. (2010). Ghrelin decreases food intake in juvenile rainbow trout (Oncorhynchus mykiss) through the central anorexigenic corticotropin-releasing factor system. Gen. Comp. Endocrinol. 166, 39–46. doi: 10.1016/j.ygcen.2009.11.001
Jørgensen, E. H., Bernier, N. J., Maule, A. G., and Vijayan, M. M. (2016). Effect of long-term fasting and a subsequent meal on mRNA abundances of hypothalamic appetite regulators, central and peripheral leptin expression and plasma leptin levels in rainbow trout. Peptides 86, 162–170. doi: 10.1016/j.peptides.2015.08.010
Kaiya, H., Kangawa, K., and Miyazato, M. (2013). Ghrelin receptors in nonmammalian vertebrates. Front. Endocrinol. 4:81. doi: 10.3389/fendo.2013.00081
Kaiya, H., Miura, T., Matsuda, K., Miyazato, M., and Kangawa, K. (2010). Two functional growth hormone secretagogue receptor (ghrelin receptor) type 1a and 2a in goldfish, Carassius auratus. Mol. Cell. Endocrinol. 327, 25–39. doi: 10.1016/j.mce.2010.06.004
Kaiya, H., Miyazato, M., Kangawa, K., Peter, R. E., and Unniappan, S. (2008). Ghrelin: a multifunctional hormone in non-mammalian vertebrates. Comp. Biochem. Physiol. A. 149, 109–128. doi: 10.1016/j.cbpa.2007.12.004
Kang, K. S., Yahashi, S., Azuma, M., and Matsuda, K. (2010). The anorexigenic effect of cholecystokinin octapeptide in a goldfish model is mediated by the vagal afferent and subsequently through the melanocortin- and corticotropin-releasing hormone-signaling pathways. Peptides 31, 2130–2134. doi: 10.1016/j.peptides.2010.07.019
Kang, K. S., Yahashi, S., and Matsuda, K. (2011). Central and peripheral effects of ghrelin on energy balance, food intake and lipid metabolism in teleost fish. Peptides 32, 2242–2247. doi: 10.1016/j.peptides.2011.05.006
Kaslin, J., Nystedt, J. M., Ostergard, M., Peitsaro, N., and Panula, P. (2004). The orexin/hypocretin system in zebrafish is connected to the aminergic and cholinergic systems. J. Neurosci. 24, 2678–2689. doi: 10.1523/JNEUROSCI.4908-03.2004
Kaushik, S., Médale, F., Fauconneau, B., and Blanc, D. (1989). Effect of digestible carbohydrates on protein - Energy utilization and on glucose metabolism in rainbow trout (Salmo gairdneri R). Aquaculture 79, 63–74. doi: 10.1016/0044-8486(89)90446-8
Kawauchi, H., Kawazoe, I., Tsubokawa, M., Kishida, M., and Baker, B. I. (1983). Characterization of melanin-concentrating hormone in chum salmon pituitaries. Nature 305, 321–323 doi: 10.1038/305321a0
Kehoe, A. S., and Volkoff, H. (2007). Cloning and characterization of neuropeptide Y (NPY) and cocaine and amphetamine regulated transcript (CART) in Atlantic cod (Gadus morhua). Comp. Biochem. Physiol. A. 146, 451–461. doi: 10.1016/j.cbpa.2006.12.026
Kinoshita, M., Morita, T., Toyohara, H., Hirata, T., Sakaguchi, M., Ono, M., et al. (2001). Transgenic medaka overexpressing a melanin-concentrating hormone exhibit lightened body color but no remarkably abnormality. Mar. Biotechnol. 3, 536–543. doi: 10.1007/s10126-001-0061-Y
Kiris, G. A., Kumlu, M., and Dikel, S. (2007). Stimulatory effects of neuropeptide Y on food intake and growth of Oreochromis niloticus. Aquaculture 264, 383–389. doi: 10.1016/j.aquaculture.2006.12.004
Kitazawa, T., Itoh, K., Yaosak, N., Maruyama, K., Matsuda, K., Teraoka, H., et al. (2012). Ghrelin does not affect gastrointestinal contractility in rainbow trout and goldfish in vitro. Gen. Comp. Endocrinol. 178, 539–545. doi: 10.1016/j.ygcen.2012.06.025
Kling, P., Ronnestad, I., Stefansson, S. O., Murashita, K., Kurokawa, T., and Björnsson, B. T. (2009). A homologous salmonid leptin radioimmunoassay indicates elevated plasma leptin levels during fasting of rainbow trout. Gen. Comp. Endocrinol. 162, 307–312. doi: 10.1016/j.ygcen.2009.04.003
Ko, C. H., and Takahashi, J. S. (2006). Molecular components of the mammalian circadian clock. Hum. Mol. Genet. 15, R271–R277. doi: 10.1093/hmg/ddl207
Kobayashi, Y., Peterson, B. C., and Waldbieser, G. C. (2008). Association of cocaine- and amplhetamine-regulated transcript (CART) messenger RNA level, food intake, and growth in channel catfish. Comp. Biochem. Physiol. A. 151, 219–225. doi: 10.1016/j.cbpa.2008.06.029
Kojima, K., Kamijo, M., Kageyama, H., Uchiyama, M., Shioda, S., and Matsuda, S. (2009). Neuronal relationship between orexin-A- and neuropeptide Y-induced orexigenic actions in goldfish. Neuropeptides 43, 63–71. doi: 10.1016/j.npep.2009.01.004
Kojima, M., Hosoda, H., Date, Y., Nakazato, M., Matsuo, H., and Kangawa, K. (1994). Ghrelin is a growth hormone- releasing acylated peptide from stomach. Nature 402, 656–660. doi: 10.1038/45230
Kristensen, P., Judge, M. E., Thim, L., Ribel, U., Christjansen, K. N., Wulff, B. S., et al. (1998). Hypothalamic CART is a new anorectic peptide regulated by leptin. Nature. 393, 72–76. doi: 10.1038/29993
Krogdahl, A., Sundby, A., and Olli, J. J. (2004). Atlantic salmon (Salmo salar) and rainbow trout (Oncorhynchus mykiss) digest and metabolize nutrients differently. Effects of water salinity and dietary starch level. Aquaculture 229, 335–360. doi: 10.1016/S0044-8486(03)00396-X
Kulczykowska, E., and Sánchez-Vázquez, F. J. (2010). Neurohormonal regulation of feed intake and response to nutrients in fish: aspects of feeding rhythm and stress. Aquacul. Res. 41, 654–657. doi: 10.1111/j.1365-2109.2009.02350.x
Kurokawa, T., Murashita, K., Suzuki, T., and Uji, S. (2008). Genomic characterization and tissue distribution of leptin receptor and leptin receptor overlapping transcript genes in the pufferfish, Takifugu rubripes. Gen. Comp. Endocrinol. 158, 108–114. doi: 10.1016/j.ygcen.2008.06.003
Lamia, K. A., Sachdeva, U. M., DiTacchio, L., Williams, E. C., Alvarez, J. G., Egan, D. F., et al. (2009). AMPK regulates the circadian clock by cryptochrome phosphorylation and degradation. Science 326, 437–440. doi: 10.1126/science.1172156
Lanfumey, L., Mongeau, R., Cohen-Salmon, C., and Hamon, M. (2008). Corticosteroid–serotonin interactions in the neurobiological mechanisms of stress-related disorders. Neurosci. Biobehav. Rev. 32, 1174–1184. doi: 10.1016/j.neubiorev.2008.04.006
Langhans, W. (1999). “Appetite regulation,” in Protein Metabolism and Nutrition, eds G. E. Lobley, A. White, and J. C. MacRae (Wageningen: Wageningen Press), 225–251.
Langhans, W., and Scharrer, E. (1992). Metabolic control of eating. World Rev. Nutr. Diet. 70, 1–67. doi: 10.1159/000421670
Leal, E., Sánchez, E., Muriach, B., and Cerdá-Reverter, J. M. (2009). Sex steroid-induced inhibition of food intake in sea bass (Dicentrarchus labrax). J. Comp. Physiol. B. 179, 77–86. doi: 10.1007/s00360-008-0285-5
Leder, E. H., and Silverstein, J. T. (2006). The pro-opiomelanocortin genes in rainbow trout (Oncorhynchus mykiss): duplications, splice variants, and differential expression. J Endocrinol. 188, 355–363. doi: 10.1677/joe.1.06283
Le Foll, C., Irani, B. G., Magnan, C., Dunn-Meynell, A. A., and Levin, B. E. (2009). Characteristics and mechanisms of hypothalamic neuronal fatty acid sensing. Am. J. Physiol. Regul. Integr. Comp. Physiol. 297, R655–R664. doi: 10.1152/ajpregu.00223.2009
Leibush, B., Parrizas, I., Navarro, I., Lappova, M. A., Maestro, M., Encinas, M., et al. (1996). Insulin and insulin-like growth factor-I receptors in fish brain. Regul. Peptides 61, 155–161. doi: 10.1016/0167-0115(95)00154-9
Levin, B. E., Routh, V. H., Kang, L., Sanders, N. M., and Dunn-Meynell, A. A. (2004). Neuronal glucosensing. What do we know after 50 years? Diabetes 53, 2521–2528. doi: 10.2337/diabetes.53.10.2521
Li, A., Yuan, X., Liang, X.-F., Liu, L., Li, J., Li, B., et al. (2016). Adaptations of lipid metabolism and food intake in response to low and high fat diets in juvenile grass carp (Ctenopharyngodon idellus). Aquaculture 457, 43–49. doi: 10.1016/j.aquaculture.2016.01.014
Li, G.-G., Liang, X.-F., Xie, Q., Li, G., Yu, Y., and Lai, K. (2010). Gene structure, recombinant expression and functional characterization of grass carp leptin. Gen. Comp. Endocrinol. 166, 117–127. doi: 10.1016/j.ygcen.2009.10.009
Librán-Pérez, M., Geurden, I., Dias, K., Corraze, G., Panserat, S., and Soengas, J. L. (2015b). Feeding rainbow trout with a lipid-enriched diet: effects on fatty acid sensing, regulation of food intake and cellular signaling pathways. J. Exp. Biol. 218, 2610–2619. doi: 10.1242/jeb.123802
Librán-Pérez, M., López-Patiño, M. A., Míguez, J. M., and Soengas, J. L. (2013). Oleic acid and octanoic acid sensing capacity in rainbow trout Oncorhynchus mykiss is direct in hypothalamus and Brockmann bodies. PLoS ONE 8:e59507. doi: 10.1371/journal.pone.0059507
Librán-Pérez, M., Otero-Rodiño, C., López-Patiño, M. A., Míguez, J. M., and Soengas, J. L. (2014a). Central administration of oleate or octanoate activates hypothalamic fatty acid sensing and inhibits food intake in rainbow trout. Physiol. Behav. 129, 272–279. doi: 10.1016/j.physbeh.2014.02.061
Librán-Pérez, M., Polakof, S., López-Patiño, M. A., Míguez, J. M., and Soengas, J. L. (2012). Evidence of a metabolic fatty-acid sensing system in the hypothalamus and Brockmann bodies of rainbow trout: implications in food intake regulation. Am. J. Physiol. Regul. Integr. Comp. Physiol. 302, R1340–R1350. doi: 10.1152/ajpregu.00070.2012
Librán-Pérez, M., Velasco, C., López-Patiño, M. A., Míguez, J. M., and Soengas, J. L. (2014b). Counter-regulatory response to a fall in circulating fatty acid levels in rainbow trout. Possible involvement of the hypothalamus-pituitary-interrenal axis. PLoS ONE 9:e113291. doi: 10.1371/journal.pone.0113291
Librán-Pérez, M., Velasco, C., Otero-Rodiño, C., López-Patiño, M. A., Míguez, J. M., and Soengas, J. L. (2015a). Effects of insulin treatment on the response to oleate and octanoate of food intake and fatty acid-sensing systems in rainbow trout. Domestic Anim. Endocrinol. 53, 124–135. doi: 10.1016/j.domaniend.2015.06.004
Londraville, R. L., Macotela, Y., Duff, R. J., Easterling, M. R., Liu, Q., and Crespi, E. J. (2014). Comparative endocrinology of leptin: assessing function in a phylogenetic context. Gen. Comp. Endocrinol. 203, 146–157. doi: 10.1016/j.ygcen.2014.02.002
López, J., Cuesta, N., Cuttitta, F., and Martinez, A. (1999). Adrenomedullin in nonmammalian vertebrate pancreas: an immunocytochemical study. Gen. Comp. Endocrinol. 115, 309–322. doi: 10.1006/gcen.1999.7335
López, J. M., Sanz-Morello, B., and González, A. (2014). Organization of the orexin/hypocretin system in the brain of two basal actinopterygian fishes, the cladistians Polypterus senegalus and Erpetoichthys calabaricus. Peptides 61, 23–37. doi: 10.1016/j.peptides.2014.08.011
López, M., Lelliott, C. J., and Vidal-Puig, A. (2007). Hypothalamic fatty acid metabolism: a housekeeping pathway that regulates food intake. BioEssays 29, 248–261. doi: 10.1002/bies.20539
López, M., Tovar, S., Vázquez, M. J., Nogueiras, R., Señarís, R., and Diéguez, C. (2005). Sensing the fat: fatty acid metabolism in the hypothalamus and the melanocortin system. Peptides 26, 1753–1758. doi: 10.1016/j.peptides.2004.11.025
López-Olmeda, J. F., and Sánchez-Vázquez, F. J. (2010). “Feeding rhythms in fish: from behavioral to molecular approach,” in Biological Clock in Fish, eds E. Kulczykowska, W. Popek, and B. G. Kapoor (Enfield: Science Pub CRC Press), 155–183.
López-Patiño, M. A., Guijarro, A. I., Isorna, E., Delgado, M. J., Alonso-Bedate, M., and De Pedro, N. (1999). Neuropeptide Y has a stimulatory action on feeding behavior in goldfish (Carassius auratus). Eur. J. Pharmacol. 377, 147–153.
Luo, L., Xue, M., Vachot, C., Geurden, I., and Kaushik, S. (2014). Dietary medium chain fatty acids from coconut oil have little effects on postprandial plasma metabolite profiles in rainbow trout (Oncorhynchus mykiss). Aquaculture 420–421, 24–31. doi: 10.1016/j.aquaculture.2013.10.024
Luquet, S., Perez, F. A., Hnasko, T. S., and Palmiter, R. D. (2005). NPY/AgRP neurons are essential for feeding in adult mice but can be ablated in neonates. Science 310, 683–685. doi: 10.1126/science.1115524
MacDonald, E., and Volkoff, H. (2009). Cloning, distribution and effects of season and nutritional status on the expression of neuropeptide Y (NPY), cocaine and amphetamine regulated transcript (CART) and cholecystokinin (CCK) in winter flounder (Pseudopleuronectes americanus). Horm. Behav. 56, 58–65. doi: 10.1016/j.yhbeh.2009.03.002
Madrid, J. A., Boujard, T., and Sánchez-Vázquez, F. J. (2001). “Feeding rhythms in fish,” in Food Intake in Fish, eds D. F. Houlihan, T. Boujard, and M. Jobling (Oxford: Blackwell Science), 189–215.
Martínez-Álvarez, R. M., Volkoff, H., Muñoz-Cueto, J. A., and Delgado, M. J. (2008). Molecular characterization of calcitonin gene-related peptide (CGRP) related peptides (CGRP, amylin, adrenomedullin and adrenomedullin-2/intermedin) in goldfish (Carassius auratus): cloning and distribution. Peptides 29, 1534–1543. doi: 10.1016/j.peptides.2008.04.013
Martínez-Álvarez, R. M., Volkoff, H., Muñoz-Cueto, J. A., and Delgado, M. J. (2009). Effect of calcitonin gene-related peptide (CGRP), adrenomedullin and adrenomedullin-2/intermedin on food intake in goldfish (Carassius auratus). Peptides 30, 803–807. doi: 10.1016/j.peptides.2008.12.015
Martín-Robles, A. J., Whitmore, D., Sánchez-Vázquez, F. J., Pendón, C., and Muñoz-Cueto, J. A. (2012). Cloning, tissue expression pattern and daily rhythms of Period1, Period2, and Clock transcripts in the flatfish Senegalese sole, Solea senegalensis. J. Comp. Physiol. B 182, 673–685. doi: 10.1007/s00360-012-0653-z
Marty, N., Dallaporta, M., and Thorens, B. (2007). Brain glucose sensing, counteregulation, and energy homeostasis. Physiology 22, 241–251. doi: 10.1152/physiol.00010.2007
Masri, S., Orozco-Solis, R., Aguilar-Arnal, L., Cervantes, M., and Sassone-Corsi, P. (2015). Coupling circadian rhythms of metabolism and chromatin remodelling. Diab. Obes. Metab. 17(Suppl. 1), 17–22. doi: 10.1111/dom.12509
Masri, S., Rigor, P., Cervantes, M., Ceglia, N., Sebastian, C., Xiao, C., et al. (2014). Partitioning circadian transcription by SIRT6 leads to segregated control of cellular metabolism. Cell 158, 659–672. doi: 10.1016/j.cell.2014.06.050
Matsuda, K., Azuma, M., and Kang, K. S. (2012a). “Orexin system in teleost fish,” in Vitamins and Hormones, ed L. Gerald (New York, NY: Academic Press), 341–361.
Matsuda, K., Miura, T., Kaiya, H., Maruyama, K., Shimakura, S. I., Uchiyama, M., et al. (2006b). Regulation of food intake by acyl and des-acyl-ghrelins in the goldfish. Peptides 27, 2321–2325. doi: 10.1016/j.peptides.2006.03.028
Matsuda, K., Miura, T., Kaiya, H., Maruyama, K., Uchiyama, M., Kangawa, K., et al. (2006a). Stimulatory effect of n-octanoylated ghrelin on locomotor activity in the goldfish, Carassius auratus. Peptides 27, 1335–1340. doi: 10.1016/j.peptides.2005.10.011
Matsuda, K., Sakashita, A., Yokobori, E., and Azuma, M. (2012b). Neuroendocrine control of feeding behavior and psychomotor activity by neuropeptide Y in fish. Neuropeptides 46, 275–283. doi: 10.1016/j.npep.2012.09.006
Matsuda, K., Shimakura, S. I., Maruyama, K., Miura, T., Uchiyama, M., Kawauchi, H., et al. (2006c). Central administration of melanin-concentrating hormone (MCH) suppresses food intake but not locomotor activity, in the goldfish, Carassius auratus. Neurosci. Lett. 399, 259–263. doi: 10.1016/j.neulet.2006.02.005
Maurin, A.-C., Benani, A., Lorsignol, A., Brenachot, X., Parry, L., Carraro, V., et al. (2014). Hypothalamic elF2α signaling regulates food intake. Cell Rep. 6, 438–444. doi: 10.1016/j.celrep.2014.01.006
Mazurais, D., Le Dréan, G., Brierley, I., Anglade, I., Bromage, N., Williams, L. M., et al. (2000). Expression of clock gene in the brain of rainbow trout: comparison with the distribution of melatonin receptors. J. Comp. Neurol. 422, 612–620. doi: 10.1002/1096-9861(20000710)422:4<612::AID-CNE9>3.0.CO;2-Y
McCrimmon, R. J., Song, Z., Cheng, H., McNay, E. C., Weickart-Yeckel, C., Routh, V. H., et al. (2006). Corticotrophin-releasing factor receptors within the ventromedial hypothalamus regulate hypoglycemia-induced hormonal counterregulation. J. Clin. Invest. 116, 1723–1730. doi: 10.1172/JCI27775
Mendoza, J. (2006). Circadian clocks: setting time by food. J. Neuroendocrinol. 19, 127–137. doi: 10.1111/j.1365-2826.2006.01510.x
Metz, J. R., Huising, M. O., Meek, J., Taverne-Thiele, A. J., Wendelaar Bonga, S. E., and Flik, G. (1994). Localization, expression and control of adrenocorticotropic hormone in the nucleus preopticus and pituitary gland of common carp (Cyprinus carpio L.). J. Endocrinol. 182, 23–31. doi: 10.1677/joe.0.1820023
Micale, V., Campo, S., D'Ascola, A., Guerrera, C., Levanti, C., Germanà, A., et al. (2014). Cholecystokinin: how many functions? Observations in seabreams. Gen. Comp. Endocrinol. 205, 166–167. doi: 10.1016/j.ygcen.2014.02.019
Micale, V., Campo, S., D'Ascola, A., Guerrera, M. C., Levanti, M. B., German,à, A., et al. (2012). Cholecystokinin in white sea bream: molecular cloning, regional expression, and immunohistochemical localization in the gut after feeding and fasting. PLoS ONE 7:e52428. doi: 10.1371/journal.pone.0052428
Michel, M., Page-McCaw, P. S., Chen, W., and Cone, R. D. (2016). Leptin signaling regulates glucose homeostasis, but not adipostasis, in the zebrafish. Proc. Natl. Acad. Sci. U.S.A. 113, 3084–3089. doi: 10.1073/pnas.1513212113
Migrenne, S., Magnan, C., and Cruciani-Guglielmacci, C. (2007). Fatty acid sensing and nervous control of energy homeostasis. Diabetes Metab. 33, 177–182. doi: 10.1016/j.diabet.2007.01.006
Mistlberger, R. E. (2009). Food anticipatory circadian rhythms: concepts and methods. Eur. J. Neurosci. 30, 1718–1729. doi: 10.1111/j.1460-9568.2009.06965.x
Mitro, N., Mak, P. A., Vargas, L., Godio, C., Hampton, E., Molteni, V., et al. (2007). The nuclear receptor LXR is a glucose sensor. Nature 445, 219–223. doi: 10.1038/nature05449
Miura, T., Maruyama, K., Shimakura, S., Kaiya, H., Uchiyama, M., Kangawa, K., et al. (2006). Neuropeptide y mediates ghrelin-induced feeding in the goldfish, Carassius auratus. Neurosci. Lett. 407, 279–283. doi: 10.1016/j.neulet.2006.08.071
Miura, T., Maruyama, K., Shimakura, S., Kaiya, H., Uchiyama, M., Kangawa, K., et al. (2007). Regulation of food intake in the goldfish by interaction between ghrelin and orexin. Peptides 28, 1207–1213. doi: 10.1016/j.peptides.2007.03.023
Mobbs, C. V., Isoda, F., Makimura, H., Mastaitis, J., Mizuno, T., Shu, I.-W., et al. (2005). Impaired glucose signaling as a cause of obesity and the metabolic syndrome: the glucoadipostatic hypothesis. Physiol. Behav. 85, 2–23. doi: 10.1016/j.physbeh.2005.04.005
Moen, A. G., and Finn, R. N. (2013). Short-term, but not long-term feed restriction causes differential expression of leptins in Atlantic salmon. Gen. Comp. Endocrinol. 183, 83–88. doi: 10.1016/j.ygcen.2012.09.027
Mommsen, T. P., Vijayan, M. M., and Moon, T. W. (1999). Cortisol in teleosts: dynamics, mechanisms of action, and metabolic regulation. Rev. Fish Biol. Fish. 9, 211–268. doi: 10.1023/A:1008924418720
Montoya, A., López-Olmeda, J. F., Yúfera, M., Sánchez-Muros, M. J., and Sánchez-Vázquez, F. J. (2010). Feeding time synchronises daily rhythms of behaviour and digestive physiology in gilthead seabream (Sparus auratus). Aquaculture 306, 315–321. doi: 10.1016/j.aquaculture.2010.06.023
Morrison, C. D., Xi, X., White, C. L., Ye, J., and Martin, R. J. (2016). Amino acids inhibit Agrp gene expression via an mTOR-dependent mechanism. Am. J. Physiol. Endocrinol. Metab. 293, E165–E171. doi: 10.1152/ajpendo.00675.2006
Morton, G. J., Cummings, D. E., Baskin, D. G., Barsh, G. S., and Schwartz, M. W. (2006). Central nervous system control of food intake and body weight. Nature 443, 289–295. doi: 10.1038/nature05026
Murashita, K., Fukada, H., Hosokawa, H., and Masumoto, T. (2006). Cholecystokinin and peptide y in yellowtail (Seriola quinqueradiata): molecular cloning, real-time quantitative RT-PCR, and response to feeding and fasting. Gen. Comp. Endocrinol. 145, 287–297. doi: 10.1016/j.ygcen.2005.09.008
Murashita, K., Jordal, A.-E. O., Nilsen, T. O., Stefansson, S. O., Kurokawa, T., Björnsson, B. T. H., et al. (2011). Leptin reduces Atlantic salmon growth through the central pro-opiomelanocortin pathway. Comp. Biochem. Physiol. A. 158, 79–86. doi: 10.1016/j.cbpa.2010.09.001
Murashita, K., Kurokawa, T., Ebbesson, L. O. E., Stefansson, S. O., and Ronnestad, I. (2009). Characterization, tissue distribution, and regulation of agouti-related protein (AgRP), cocaine- and amphetamine-regulated transcript (CART) and neuropeptide Y (NPY) in Atlantic salmon (Salmo salar). Gen. Comp. Endocrinol. 162, 160–171. doi: 10.1016/j.ygcen.2009.03.015
Murashita, K., Uji, S., Yamamoto, T., Ronnestad, I., and Kurokawa, T. (2008). Production of recombinant leptin and its effects on food intake in rainbow trout (Oncorhynchus mykiss). Comp. Biochem. Physiol. B. 150, 377–384. doi: 10.1016/j.cbpb.2008.04.007
Nakahata, Y., Kaluzova, M., Grimaldi, B., Sahar, S., Hirayama, J., Chen, D., et al. (2008). The NAD+−dependent deacetylase SIRT1 modulates CLOCK-mediated chromatin remodeling and circadian control. Cell 134, 329–340. doi: 10.1016/j.cell.2008.07.002
Nakamachi, T., Matsuda, K., Maruyama, K., Miura, T., Uchiyama, M., Funahashi, H., et al. (2006). Regulation by orexin of feeding behaviour and locomotor activity in the goldfish. J. Neuroendocrinol. 18, 290–297. doi: 10.1111/j.1365-2826.2006.01415.x
Nakamachi, T., Shibata, H., Sakashita, A., Iinuma, N., Wada, K., Konno, N., et al. (2014). Orexin A enhances locomotor activity and induces anxiogenic-like action in the goldfish, Carassius auratus. Horm. Behav. 66, 317–323. doi: 10.1016/j.yhbeh.2014.06.004
Narnaware, Y., and Peter, R. E. (2002). Influence of diet composition on food intake and neuropeptide Y (NPY) gene expression in goldfish brain. Reg. Peptides 103, 75–83. doi: 10.1016/S0167-0115(01)00342-1
Nisembaum, L. G., de Pedro, N., Delgado, M. J., and Isorna, E. (2014b). Crosstalking between the “gut-brain” hormone ghrelin and the circadian system in the goldfish. Effects on clock gene expression and food anticipatory activity. Gen. Comp. Endocrinol. 205, 287–295. doi: 10.1016/j.ygcen.2014.03.016
Nisembaum, L. G., de Pedro, N., Delgado, M. J., Sánchez-Bretaño, A., and Isorna, E. (2014a). Orexin as an input of circadian system in goldfish: effects on clock gene expression and locomotor activity rhythms. Peptides 52, 29–37. doi: 10.1016/j.peptides.2013.11.014
Nisembaum, L. G., Velarde, E., Tinoco, A. B., Azpeleta, C., de Pedro, N., Alonso-Gómez, A. L., et al. (2012). Light-dark cycle and feeding time differentially entrains the gut molecular clock of the goldfish (Carassius auratus). Chronobiol. Int. 29, 665–673. doi: 10.3109/07420528.2012.686947
Nishio, S., Gibert, Y., Berekelya, L., Bernard, L., Brunet, F., Guillot, E., et al. (2012). Fasting induces CART down-regulation in the zebrafish nervous system in a cannabinoid receptor 1-dependent manner. Mol. Endocrinol. 26, 1316–1326. doi: 10.1210/me.2011-1180
Novak, C. M., Jiang, X., Wang, C., Teske, J. A., Kotz, C. M., and Levine, J. A. (2005). Calorie restriction and physical activity in zebrafish (Danio rerio). Neurosci. Lett. 383, 99–104. doi: 10.1016/j.neulet.2005.03.048
Ogoshi, M., Inoue, K., and Takei, Y. (2003). Identification of a novel adrenomedullin gene family in teleost fish. Biochem. Biophys. Res. Commun. 311, 1072–1077. doi: 10.1016/j.bbrc.2003.10.111
Oh, Y. T., Kim, J., Kang, I., and Youn, J. H. (2014). Regulation of hypothalamic-pituitary-adrenal axis by circulating free fatty acids in male wistar rats: role of individual free fatty acids. Endocrinology 155, 923–931. doi: 10.1210/en.2013-1700
Oh, Y. T., Oh, K.-S., Kang, I., and Youn, J. H. (2012). A fall in plasma free fatty acid (FFA) level activates the hypothalamic-pituitary-adrenal axis independent of plasma glucose: evidence for brain sensing of circulating FFA. Endocrinology 153, 3587–3592. doi: 10.1210/en.2012-1330
Ollmann, M. M., Wilson, B. D., Yang, Y. K., Kerns, J. A., Chen, Y., Gantz, I., et al. (1997). Antagonism of central melanocortin receptors in vitro and in vivo by agouti-related protein. Science 278, 135–138. doi: 10.1126/science.278.5335.135
Olsson, C., Holbrook, J. D., Bompadre, G., Jönsson, E., Hoyle, C. H. V., Sanger, G. J., et al. (2008). Identification of genes for the ghrelin and motilin receptors and a novel related gene in fish, and stimulation of intestinal motility in zebrafish (Danio rerio) by ghrelin and motilin. Gen. Comp. Endocrinol. 155, 217–226. doi: 10.1016/j.ygcen.2007.05.016
Olsson, C., and Holmgren, S. (2011). Autonomic control of gut motility: a comparative view. Auton. Neurosci. 165, 80–101. doi: 10.1016/j.autneu.2010.07.002
Oosterman, J. E., Kalsbeek, A., la Fleur, S. E., and Belsham, D. D. (2015). Impact of nutrients on circadian rhythmicity. Am. J. Physiol. Regul. Integr. Comp. Physiol. 308, R337–R350. doi: 10.1152/ajpregu.00322.2014
Ooyama, Y., Kojima, K., Aoyama, T., and Takeuchi, H. (2009). Decrease of food intake in rats after ingestion of medium-chain triacylglycerol. J. Nutr. Sci. Vitaminol. 55, 423–427. doi: 10.3177/jnsv.55.423
Orozco-Solis, R., Ramadori, G., Coppari, R., and Sassone-Corsi, P. (2015). SIRT1 relays nutritional inputs to the circadian clock through the Sf1 neurons of the ventromedial hypothalamus. Endocrinology 156, 2174–2184. doi: 10.1210/en.2014-1805
Ortega, V. A., Lovejoy, D. A., and Bernier, N. J. (2013). Appetite-suppressing effects and interactions of centrally administered corticotropin-releasing factor, urotensin I and serotonin in rainbow trout (Oncorhynchus mykiss). Front. Neurosci. 7:196. doi: 10.3389/fnins.2013.00196
Otero-Rodiño, C., Librán-Pérez, M., Velasco, C., López-Patiño, M. A., Míguez, J. M., and Soengas, J. L. (2015). Evidence for the presence of glucosensor mechanisms not dependent on glucokinase in hypothalamus and hindbrain of rainbow trout (Oncorhynchus mykiss). PLoS ONE 10:e0128603. doi: 10.1371/journal.pone.0128603
Otero-Rodiño, C., Velasco, C., Álvarez-Otero, R., López-Patiño, M. A., Míguez, J. M., and Soengas, J. L. (2016). In vitro evidence supports the presence of glucokinase-independent glucosensing mechanisms in hypothalamus and hindbrain of rainbow trout. J. Exp. Biol. 219, 1750–1759. doi: 10.1242/jeb.137737
Partch, C. L., Green, C. B., and Takahashi, J. S. (2014). Molecular architecture of the mammalian circadian clock. Trends Cell. Biol. 24, 90–99. doi: 10.1016/j.tcb.2013.07.002
Patiño, M. A. L., Rodríguez-Illamola, A., Conde-Sieira, M., Soengas, J. L., and Míguez, J. M. (2011). Daily rhythmic expression patterns of clock1a, bmal1, and per1 genes in retina and hypothalamus of the rainbow trout, Oncorhynchus mykiss. Chronobiol. Int. 28, 381–389. doi: 10.3109/07420528.2011.566398
Patton, D. F., and Mistlberger, R. E. (2013). Circadian adaptations to meal timing; neuroendocrine mechanisms. Front. Neurosci. 7:185. doi: 10.3389/fnins.2013.00185
Peddu, S. C., Breves, J. P., Kaiya, H., Grau, E. G., and Riley, L. G. Jr. (2009). Pre- and postprandial effects on ghrelin signaling in the brain and on the GH/IGF-I axis in the Mozambique tilapia (Oreochromis mossambicus). Gen. Comp. Endocrinol. 161, 412–418. doi: 10.1016/j.ygcen.2009.02.008
Penney, C. C., and Volkoff, H. (2014). Peripheral injections of cholecystokinin, apelin, ghrelin and orexin in cavefish (Astyanax fasciatus mexicanus): effects on feeding and on the brain expression levels of tyrosine hydroxylase, mechanistic target of rapamycin and appetite-related hormones. Gen. Comp. Endocrinol. 196, 34–40. doi: 10.1016/j.ygcen.2013.11.015
Peragón, J., Barroso, J. B., García-Salguero, L., de la Higuera, M., and Lupiáñez, J. A. (2000). Dietary alterations in protein, carbohydrates and fat increase liver protein-turnover rate and decrease overall growth rate in the rainbow trout (Oncorhynchus mykiss). Mol. Cell. Biochem. 209, 97–104. doi: 10.1023/A:1007130906365
Peterson, B. C., Waldbieser, G. C., Riley, L. G. Jr., Upton, K. R., Kobayashi, Y., and Small, B. C. (2012). Pre- and postprandial changes in orexigenic and anorexigenic factors in channel catfish (Ictalurus punctatus). Gen. Comp. Endocrinol. 176, 231–239. doi: 10.1016/j.ygcen.2012.01.022
Picard, A., Rouch, C., Kassis, N., Moullé, V. S., Croizier, S., Denis, R. G., et al. (2013). Hippocampal lipoprotein lipase regulates energy balance in rodents. Mol. Metab. 3, 167–176. doi: 10.1016/j.molmet.2013.11.002
Picha, M. E., Strom, C. N., Riley, L. G., Walker, A. A., Won, E. T., Johnstone, W. M., et al. (2009). Plasma ghrelin and growth hormone regulation in response to metabolic state in hybrid striped bass: effects of feeding, ghrelin and insulin-like growth factor-I on in vivo and in vitro GH secretion. Gen. Comp. Endocrinol. 161, 365–372. doi: 10.1016/j.ygcen.2009.01.026
Polakof, S., Ceinos, R. M., Fernández-Durán, B., Míguez, J. M., and Soengas, J. L. (2007c). Daily changes in parameters of energy metabolism in brain of rainbow trout: dependence on feeding. Comp. Biochem. Physiol. A. 146, 265–273. doi: 10.1016/j.cbpa.2006.10.026
Polakof, S., Médale, F., Skiba-Cassy, S., Corraze, G., and Panserat, S. (2010). Molecular regulation of lipid metabolism in liver and muscle of rainbow trout subjected to acute and chronic insulin treatments. Domestic Anim. Endocrinol. 39, 26–33. doi: 10.1016/j.domaniend.2010.01.003
Polakof, S., Míguez, J. M., Moon, T. W., and Soengas, J. L. (2007a). Evidence for the presence of a glucosensor in hypothalamus, hindbrain, and Brockmann bodies of rainbow trout. Am. J. Physiol. Regul. Integr. Comp. Physiol. 292, R1657–R1666. doi: 10.1152/ajpregu.00525.2006
Polakof, S., Míguez, J. M., and Soengas, J. L. (2007b). In vitro evidences for glucosensing capacity and mechanisms in hypothalamus, hindbrain, and Brockmann bodies of rainbow trout. Am. J. Physiol. Regul. Integr. Comp. Physiol. 293, R1410–R1420. doi: 10.1152/ajpregu.00283.2007
Polakof, S., Míguez, J. M., and Soengas, J. L. (2007d). Daily changes in parameters of energy metabolism in liver, white muscle, and gills of rainbow trout: dependence on feeding. Comp. Biochem. Physiol. A. 147, 363–374. doi: 10.1016/j.cbpa.2007.01.009
Polakof, S., Míguez, J. M., and Soengas, J. L. (2008a). Changes in food intake and glucosensing function of hypothalamus and hindbrain in rainbow trout subjected to hyperglycemic or hypoglycemic conditions. J. Comp. Physiol. A. 194, 829–839. doi: 10.1007/s00359-008-0354-y
Polakof, S., Míguez, J. M., and Soengas, J. L. (2008b). Dietary carbohydrates induce changes in glucosensing capacity and food intake in rainbow trout. Am. J. Physiol. Regul. Integr. Comp. Physiol. 295, R478–R489. doi: 10.1152/ajpregu.00176.2008
Polakof, S., Míguez, J. M., and Soengas, J. L. (2011a). Cholecystokinin impact on rainbow trout glucose homeostasis: possible involvement of central glucosensors. Reg. Peptides 172, 23–29. doi: 10.1016/j.regpep.2011.08.002
Polakof, S., Míguez, J. M., and Soengas, J. L. (2011b). Evidence for a gut-brain axis used by glucagon-like peptide-1 to elicit hyperglycaemia in fish. J. Neuroendocrinol. 23, 508–518. doi: 10.1111/j.1365-2826.2011.02137.x
Polakof, S., Míguez, J. M., and Soengas, J. L. (2011c). Ghrelin effects on central glucosensing ad energy homeostasis-related peptides in rainbow trout. Domestic Anim. Endocrinol. 41, 126–136. doi: 10.1016/j.domaniend.2011.05.006
Polakof, S., Mommsen, T. P., and Soengas, J. L. (2011d). Glucosensing and glucose homeostasis: from fish to mammals. Comp. Biochem. Physiol. B. 160, 123–149. doi: 10.1016/j.cbpb.2011.07.006
Polakof, S., Panserat, S., Plagnes-Juan, E., and Soengas, J. L. (2008c). Altered dietary carbohydrates significantly affect gene expression of the major glucosensing components in Brockmannn bodies and hypothalamus of rainbow trout. Am. J. Physiol. Regul. Integr. Comp. Physiol. 295, R1077–R1088. doi: 10.1152/ajpregu.90476.2008
Polakof, S., Panserat, S., Soengas, J. L., and Moon, T. W. (2012). Glucose metabolism in fish: a review. J. Comp. Physiol. B. 182, 1015–1045. doi: 10.1007/s00360-012-0658-7
Polakof, S., Rodríguez-Alonso, M., and Soengas, J. L. (2009). Immunohistochemical localization of glucokinase in rainbow trout brain. Comp. Biochem. Physiol. A. 153, 352–358. doi: 10.1016/j.cbpa.2009.03.015
Polakof, S., and Soengas, J. L. (2008). Involvement of lactate in glucose metabolism and glucosensing function in selected tissues of rainbow trout. J. Exp. Biol. 211, 1075–1086. doi: 10.1242/jeb.014050
Qu, D., Ludwig, D. S., Gammeltoft, S., Piper, M., Pelleymounter, M. A., Cullen, M. J., et al. (1996). A role for melanin-concentrating hormone in the central regulation of feeding behaviour. Nature. 380, 243–247. doi: 10.1038/380243a0
Rajjo, I. M., Vigna, S. R., and Crim, J. W. (1988). Actions of cholecystokinin-related peptides on the gallbladder of bony fishes in vitro. Comp. Biochem. Physiol. C. 90, 267–273. doi: 10.1016/0742-8413(88)90132-6
Ren, X., Zhou, L., Terwiliger, R., Newton, S. S., and de Araujo, I. E. (2009). Sweet taste signaling functions as a hypothalamic glucose sensor. Front. Integr. Neurosci. 3:12. doi: 10.3389/neuro.07.012.2009
Ribas-Latre, A., and Eckel-Mahan, A. (2016). Interdependence of nutrient metabolism and the circadian clock system: importance for metabolic health. Molec. Metab. 5, 133–152. doi: 10.1016/j.molmet.2015.12.006
Rocha, F., Dias, J., Geurden, I., Dinis, M. T., Panserat, S., and Engrola, S. (2016). Dietary glucose stimulus at larval stage modifies the carbohydrate metabolic pathway in gilthead seabream (Sparus aurata) juveniles: an in vivo approach using 14C-starch. Comp. Biochem. Physiol. A. 201, 189–199. doi: 10.1016/j.cbpa.2016.07.016
Ross, R. A., Rossetti, L., Lam, T. K. T., and Schwartz, G. J. (2010). Differential effects of hypothalamic long-chain fatty acid infusions on suppression of hepatic glucose production. Am. J. Physiol. Endocrinol. Metab. 299, E633–E639. doi: 10.1152/ajpendo.00190.2010
Rubio, V. C., Sánchez-Vázquez, F. J., and Madrid, J. A. (2008). Role of cholecystokinin and its antagonist proglumide on macronutrient selection in European sea bass Dicentrarchus labrax, L. Physiol. Behav. 93, 862–869. doi: 10.1016/j.physbeh.2007.12.001
Ruibal, C., Soengas, J. L., and Aldegunde, M. (2002). Brain serotonin and the control of food intake in rainbow trout (Oncorhynchus mykiss): effects of changes in plasma glucose levels. J. Comp. Physiol. A. 188, 479–484. doi: 10.1007/s00359-002-0320-z
Salmerón, C., Johansson, M., Asaad, M., Angotzi, A. R., Ronnestad, I., Stefansson, S. O., et al. (2015). Roles of leptin and ghrelin in adipogenesis and lipid metabolism of rainbow trout adipocytes in vitro. Comp. Biochem. Physiol. A. 188, 40–48. doi: 10.1016/j.cbpa.2015.06.017
Sánchez, E., Rubio, V. C., Thompson, D., Metz, J., Flik, G., Millhauser, G. L., et al. (2009). Phosphodiesterase inhibitor-dependent inverse agonism of agouti-related protein (AGRP) on melanocortin 4 receptor in sea bass (Dicentrarchus labrax). Am. J. Physiol. Regul. Integr. Comp. Physiol. 296, R1293–R1306. doi: 10.1152/ajpregu.90948.2008
Sánchez-Bretaño, A., Alonso-Gómez, A. L., Delgado, M. J., and Isorna, E. (2015a). The liver of goldfish as a component of the circadian system: integrating a network of signals. Gen. Comp. Endocrinol. 221, 213–216. doi: 10.1016/j.ygcen.2015.05.001
Sánchez-Bretaño, A., Alonso-Gómez, A. L., Delgado, M. J., and Isorna, E. (2016a). Clock genes expression rhythms in liver of goldfish in vivo and in vitro. Possible role of glucocorticoids as a synchronizer. J. Comp. Physiol. B. 186, 73–82.
Sánchez-Bretaño, A., Blanco, A. M., Unniappan, S., Kah, O., Gueguen, M. M., Bertucci, J. I., et al. (2015b). In situ localization and rhythmic expression of ghrelin and ghs-r1 ghrelin receptor in the brain and gastrointestinal tract of goldfish (Carassius auratus). PLoS ONE 10:e0141043. doi: 10.1371/journal.pone.0141043
Sánchez-Bretaño, A., Callejo, M., Montero, M., Alonso-Gómez, A. L., Delgado, M. J., and Isorna, E. (2016b). Performing a hepatic timing signal: glucocorticoids induce gper1a and gper1b expression and repress gclock1a and gbmal1a in the liver of goldfish. J. Comp. Physiol. B 186, 73–82. doi: 10.1007/s00360-015-0936-2
Sánchez-Bretaño, A., Gueguen, M. M., Cano-Nicolau, J., Kah, O., Alonso-Gómez, A. L., Delgado, M. J., et al. (2015c). Anatomical distribution and daily profile of gper1b gene expression in brain and peripheral structures of goldfish (Carassius auratus). Chronobiol. Int. 32, 889–902. doi: 10.3109/07420528.2015.1049615
Sánchez-Muros, M. J., García-Rejón, L., García-Salguero, L., de la Higuera, M., and Lupiáñez, J. A. (1998). Long-term nutritional effects on the pimary liver and kidney metabolism in rainbow trout. Adaptative response to starvation and high-protein, carbohydrate-free diet to glutamate dehydrogenase and alanine aminotransferase kinetics. Int. J. Biochem. 30, 55–63.
Sanders, N. M., Figlewicz, D. P., Taborsky, G. J. Jr., Wilkinson, C. W., Daumen, W., and Levin, B. E. (2006). Feeding and neuroendocrine responses after recurrent insulin-induced hypoglycemia. Physiol. Behav. 87, 700–706. doi: 10.1016/j.physbeh.2006.01.007
Saravanan, S., Geurden, I., Figueiredo-Silva, A. C., Kaushik, S., Haidar, M. N., Verreth, J. A. J., et al. (2012). Control of voluntary feed intake in fish: a role for dietary oxygen demand in Nile tilapia (Oreochromis niloticus) fed diets with different macronutrient profiles. Br. J. Nutr. 108, 1519–1529. doi: 10.1017/S0007114511006842
Saravanan, S., Geurden, I., Figueiredo-Silva, A. C., Kaushik, S., Verreth, J. A. J., and Schrama, J. W. (2013). Voluntary feed intake in rainbow trout is regulated by diet-induced diferences in oxygen use. J. Nutr. 143, 781–787. doi: 10.3945/jn.112.173062
Sargent, J. R., Tocher, D. R., and Bell, J. G. (2002). “The lipids,” in Fish Nutrition, eds J. E. Halver and R. W. Hardy (San Diego, CA: Academic Press), 182–258.
Schibler, U., Gotic, I., Saini, C., Gos, P., Curie, T., Emmenegger, Y., et al. (2015). Clock-talk: interactions between central and peripheral circadian oscillators in mammals. Old Spring Harb. Symp. Quant. Biol. 80, 223–232. doi: 10.1101/sqb.2015.80.027490
Schjolden, J., Schiöth, H. B., Larhammar, D., Winberg, S., and Larson, E. T. (2009). Melanocortin peptides affect the motivation to feed in rainbow trout (Oncorhynchus mykiss). Gen. Comp. Endocrinol. 160, 134–138. doi: 10.1016/j.ygcen.2008.11.003
Schroeter, J. C., Fenn, C. M., and Small, B. C. (2015). Elucidating the roles of gut neuropeptides on channel catfish feed intake, glycemia, and hypothalamic NPY and POMC expression. Comp. Biochem. Physiol. A. 188, 168–174. doi: 10.1016/j.cbpa.2015.06.031
Schwartz, M. W., Woods, S. C., Porte, D. Jr., Seeley, R. J., and Baskin, D. G. (2000). Central nervous system control of food intake. Nature 404, 661–671. doi: 10.1038/35007534
Schwinkendorf, D. R., Tsatsos, N. G., Gosnell, B. A., and Mashek, D. G. (2011). Effects of central administration of distinct fatty acids on hypothalamic neuropeptide expression and energy metabolism. Int. J. Obes. 35, 336–344. doi: 10.1038/ijo.2010.159
Seino, S., and Miki, T. (2003). Physiological and pathophysiological roles of ATP-sensitive K+ channels. Prog. Biophys. Mol. Biol. 81, 133–176. doi: 10.1016/S0079-6107(02)00053-6
Shearer, K. D., Silverstein, J., and Plisetskaya, E. M. (1997). Role of adiposity in food intake control of juvenile chinook salmon (Oncorhynchus tshawytscha). Comp. Biochem. Physiol. A. 118, 1209–1215. doi: 10.1016/S0300-9629(97)86801-6
Silverstein, J. T., Bondareva, V. M., Leonard, J. B. K., and Plisetskaya, E. M. (2001). Neuropeptide regulation of feeding in catfish, Ictalurus punctatus: a role for glucagon-like peptide-1 (GLP-1)? Comp. Biochem. Physiol. B. 129, 623–631. doi: 10.1016/S1096-4959(01)00357-8
Silverstein, J. T., and Plisetskaya, E. M. (2000). The effects of NPY and insulin on food intake regulation in fish. Am. Zool. 40, 296–308. doi: 10.1093/icb/40.2.296
Silverstein, J. T., Shearer, K. D., Dickhoff, W. W., and Plisetskaya, E. M. (1999). Regulation of nutrient intake and energy balance in salmon. Aquaculture 177, 161–169. doi: 10.1016/S0044-8486(99)00076-9
Singru, P. S., Mazumdar, M., Sakharkar, A. J., Lechan, R. M., Thim, L., Clausen, J. T., et al. (2007). Immunohistochemical localization of cocaine- and amphetamine regulated transcript peptide in the brain of the catfish, Clarias batrachus (Linn.). J. Comp. Neurol. 502, 215–235. doi: 10.1002/cne.21295
Soengas, J. L. (2014). Contribution of glucose- and fatty acid sensing systems to the regulation of food intake in fish. A review. Gen. Comp. Endocrinol. 205, 36–48. doi: 10.1016/j.ygcen.2014.01.015
Soengas, J. L., and Aldegunde, M. (2004). Brain glucose and insulin: effects on food intake and brain biogenic amines of rainbow trout. J. Comp. Physiol. A. 190, 641–649. doi: 10.1007/s00359-004-0524-5
Song, Y., and Cone, R. D. (2007). Creation of a genetic model of obesity in a teleost. FASEB J. 21, 2042–2049. doi: 10.1096/fj.06-7503com
Song, Y., Golling, G., Thacker, T. L., and Cone, R. D. (2003). Agouti-related protein (AGRP) is conserved and regulated by metabolic state in the zebrafish, Danio rerio. Endocrine 22, 257–265. doi: 10.1385/ENDO:22:3:257
Spiess, J., Villarreal, J., and Vale, W. (1981). Isolation and sequence analysis of a somatostatin-like polypeptide from ovine hypothalamus. Biochemistry 20, 1982–1988. doi: 10.1021/bi00510a038
Stanley, B. G., and Leibowitz, S. F. (1985). Neuropeptide Y injected in the paraventricular hypothalamus: a powerful stimulant of feeding behavior. Proc. Natl. Acad. Sci. U.S.A. 82, 3940–3943. doi: 10.1073/pnas.82.11.3940
Stephan, F. K. (2002). The ‘other’ circadian system: food as a zeitgeber. J. Biol. Rhythms 17, 284–292. doi: 10.1177/074873002129002591
Suárez, M. D., Sanz, A., Bazoco, J., and García-Gallego, M. (2002). Metabolic effects of changes in the dietary protein:carbohydrate ratio in eel (Anguilla anguilla) and trout (Oncorhynchus mykiss). Aquaculture Int. 10, 143–156. doi: 10.1023/A:1021371104839
Subhedar, N. K., Nakhate, K. T., Upadhya, M. A., and Kokare, D. M. (2014). CART in the brain of vertebrates: circuits, functions and evolution. Peptides 54, 108–130 doi: 10.1016/j.peptides.2014.01.004
Subhedar, N., Varsagade, V. G., Singru, P. S., Thim, L., and Clausen, J. T. (2011). Cocaine- and amphetamine-regulated transcript peptide (CART) in the telencephalon of the catfish, Clarias gariepinus: distribution and response to fasting, 2-deoxy-D-glucose, glucose, insulin, and leptin treatments. J. Comp. Neurol. 519, 1281–1300. doi: 10.1002/cne.22569
Sundström, G., Larsson, T. A., Brenner, S., Venkatesh, B., and Larhammar, D. (2008). Evolution of neuropeptide y family: new genes by chromosome duplications in early vertebrate and in teleost fishes. Gen. Comp. Endocrinol. 155, 705–716. doi: 10.1016/j.ygcen.2007.08.016
Takahasi, A., Tsuchicha, K., Yamanome, T., Amano, M., Yasuda, A., Yamamori, K., et al. (2004). Possible involvement of melanin-concentrating hormone in food intake in a teleost fish, barfin flounder. Peptides 25, 1613–1622. doi: 10.1016/j.peptides.2004.02.022
Tan, X., Lin, H., Huang, Z., Zhou, C., Wang, A., Qi, C., et al. (2016). Effects of dietary leucine on growth performance, feed utilization, non-specific immune responses and gut morphology of juvenile golden pompano Trachinotus ovatus. Aquaculture 465, 100–107. doi: 10.1016/j.aquaculture.2016.08.034
Tang, Z., Sun, C., Yan, A., Wu, S., Qin, C., Zhang, Y., et al. (2013). Genes involved in fatty acid metabolism: molecular characterization and hypothalamic mRNA response to energy status and neuropeptide Y treatment in the orange-spotted grouper Epinephelus coioides. Mol. Cell Endocrinol. 376, 114–124. doi: 10.1016/j.mce.2013.06.020
Thavanathan, P., and Volkoff, H. (2006). Effects of amylin on feeding of goldfish: interactions with CCK. Regul. Pept. 133, 90–96. doi: 10.1016/j.regpep.2005.09.025
Tian, J., He, G., Mai, K., and Liu, C. (2015). Effects of postprandial starvation on mRNA expression of endocrine-, amino acid and peptide transporter-, and metabolic enzyme-related genes in zebrafish (Danio rerio). Fish Physiol. Biochem. 41, 773–787. doi: 10.1007/s10695-015-0045-x
Tinoco, A. B., Näslund, J., Delgado, M. J., De Pedro, N., Johnsson, J. I., and Jönsson, E. (2014a). Ghrelin increases food intake, swimming activity and growth in juvenile brown trout (Salmo trutta). Physiol. Behav. 124, 15–22. doi: 10.1016/j.physbeh.2013.10.034
Tinoco, A. B., Nisembaum, L. G., de Pedro, N., Delgado, M. J., and Isorna, E. (2014b). Leptin expression is rhythmic in brain and liver of goldfish (Carassius auratus). Role of feeding time. Gen. Comp. Endocrinol. 204, 239–247. doi: 10.1016/j.ygcen.2014.06.006
Tinoco, A. B., Nisembaum, L. G., Isorna, E., Delgado, M. J., and de Pedro, N. (2012). Leptins and leptin receptor expression in the goldfish (Carassius auratus). Regulation by food intake and fasting/overfeeding conditions. Peptides 34, 329–335. doi: 10.1016/j.peptides.2012.02.001
Tinoco, A. I., Valenciano, A. I., Gómez-Boronat, M., Blanco, A. M., Nisembaum, L. G., De Pedro, N., et al. (2015). Two cholecystokinin receptor subtypes are identified in goldfish, being the CCKAR involved in the regulation of intestinal motility. Comp. Biochem. Physiol. A. 187, 193–201. doi: 10.1016/j.cbpa.2015.05.027
Tocher, D. R. (2003). Metabolism and functions of lipids and fatty acids in teleost fish. Rev. Fish. Sci. 11, 107–184. doi: 10.1080/713610925
Tocher, D. R., Mourente, G., and Sargent, J. R. (1992). Metabolism of [1-14C]Docosahexaenoate (22:6n-3), [1-14C]Eicosapentaenoate (20:5n-3) and [1-14C]Linolenate (18:3n-3) in brain cells from juvenile turbot Scophthalmus maximus. Lipids 27, 494–499. doi: 10.1007/BF02536129
Trushenski, J. T. (2009). Saturated lipid sources in feeds for sunshine bass: alterations in production performance and tissue fatty acid composition. North Am. J. Aquac. 71, 363–373. doi: 10.1577/A09-001.1
Uchida, H., Nakamura, T. J., Takasu, N. N., Todo, T., Sakai, T., and Nakamura, W. (2016). Cryptochrome-dependent circadian periods in the arcuate nucleus. Neurosci. Lett. 610, 123–128. doi: 10.1016/j.neulet.2015.10.071
Unniappan, S., Canosa, L. F., and Peter, R. E. (2004). Orexigenic actions of ghrelin in goldfish: feeding-induced changes in brain and gut mRNA expression and serum levels, and responses to central and peripheral injections. Neuroendocrinology 79, 100–108. doi: 10.1159/000076634
Unniappan, S., Lin, X., Cervini, L., Rivier, J., Kaiya, H., Kangawa, K., et al. (2002). Goldfish ghrelin: molecular characterization of the complementary deoxyribonucleic acid, partial gene structure and evidence for its stimulatory role in food intake. Endocrinology 143, 4143–4146. doi: 10.1210/en.2002-220644
Valen, R., Jordal, A. E., Murashita, K., and Rønnestad, I. (2011). Postprandial effects on appetite-related neuropeptide expression in the brain of Atlantic salmon, Salmo salar. Gen. Comp. Endocrinol. 171, 359–366. doi: 10.1016/j.ygcen.2011.02.027
Van den Top, M., and Spanswick, D. (2006). Integration of metabolic stimuli in the hypothalamic arcuate nucleus. Prog Brain Res. 153, 141–154. doi: 10.1016/S0079-6123(06)53008-0
Van de Pol, I., Flik, G., and Gorissen, M. (2017). Comparative physiology of energy metabolism: fishing for endocrine signals in the early vertebrate pool. Front. Endocrinol. 8:36. doi: 10.3389/fendo.2017.00036
Vatine, G., Vallone, D., Gothilf, Y., and Foulkes, N. S. (2011). It's time to swim! Zebrafish and the circadian clock. FEBS Lett. 585, 1485–1494. doi: 10.1016/j.febslet.2011.04.007
Velarde, E., Haque, R., Iuvone, P. M., Azpeleta, C., Alonso-Gómez, A. L., and Delgado, M. J. (2009). Circadian clock genes of goldfish, Carassius auratus: cDNA cloning and rhythmic expression of period and cryptochrome transcripts in retina, liver, and gut. J. Biol. Rhythms 24, 104–113. doi: 10.1177/0748730408329901
Velasco, C., Librán-Pérez, M., Otero-Rodiño, C., López-Patiño, M. A., Míguez, J. M., Cerdá-Reverter, J. M., et al. (2016a). Ghrelin modulates hypothalamic fatty acid-sensing and control of food intake in rainbow trout. J. Endocrinol. 228, 25–37. doi: 10.1530/JOE-15-0391
Velasco, C., Librán-Pérez, M., Otero-Rodiño, C., López-Patiño, M. A., Míguez, J. M., and Soengas, J. L. (2016b). Ceramides are involved in regulation of food intake in rainbow trout (Oncorhynchus mykiss). Am. J. Physiol. Regul. Integr. Comp. Physiol. 311, R658–R668. doi: 10.1152/ajpregu.00201.2016
Vera, L. M., De Pedro, N., Gómez-Milán, E., Delgado, M. J., Sánchez-Muros, M. J., Madrid, J. A., et al. (2007). Feeding entrainment of locomotor activity rhythms, digestive enzymes and neuroendocrine factors in goldfish. Physiol. Behav. 90, 518–524. doi: 10.1016/j.physbeh.2006.10.017
Vera, L. M., Negrini, P., Zagatti, C., Frigato, E., Sánchez-Vázquez, F. J., and Bertolucci, C. (2013). Light and feeding entrainment of the molecular circadian clock in a marine teleost (Sparus aurata). Chronobiol. Int. 30, 649–661. doi: 10.3109/07420528.2013.775143
Vivas, Y., Azpeleta, C., Feliciano, A., Velarde, E., Isorna, E., Delgado, M. J., et al. (2011). Time-dependent effects of leptin on food intake and locomotor activity in goldfish. Peptides 32, 989–995. doi: 10.1016/j.peptides.2011.01.028
Volkoff, H. (2012). “Sleep and orexins in nonmammalian vertebrates,” in Vitamins and Hormones, ed L. Gerald (San Diego, CA: Academic Press), 315–339.
Volkoff, H. (2014). Appetite regulating peptides in red-bellied piranha, Pygocentrus nattereri: cloning, tissue distribution and effect of fasting on mRNA expression levels. Peptides 56, 116–124. doi: 10.1016/j.peptides.2014.03.022
Volkoff, H. (2015). Cloning and tissue distribution of appetite-regulating peptides in pirapitinga (Piaractus brachypomus). J. Anim. Physiol. Anim. Nutr. 99, 987–1001. doi: 10.1111/jpn.12318
Volkoff, H. (2016). The neuroendocrine regulation of food intake in fish: a review of current knowledge. Front. Neurosci. 10:540. doi: 10.3389/fnins.2016.00540
Volkoff, H., Bjorklund, J. M., and Peter, R. E. (1999). Stimulation of feeding behavior and food consumption in the goldfish, Carassius auratus, by orexin-A and orexin-B. Brain Res. 846, 204–209. doi: 10.1016/S0006-8993(99)02052-1
Volkoff, H., Canosa, L. F., Unniappan, S., Cerdá-Reverter, J. M., Bernier, N. J., Kelly, S. P., et al. (2005). Neuropeptides and the control of food intake in fish. Gen. Comp. Endocrinol. 142, 3–19. doi: 10.1016/j.ygcen.2004.11.001
Volkoff, H., Eykelbosh, A. J., and Peter, R. E. (2003). Role of leptin in the control of feeding of goldfish Carassius auratus: interactions with cholecystokinin, neuropeptide Y and orexin A, and modulation by fasting. Brain Res. 972, 90–109. doi: 10.1016/S0006-8993(03)02507-1
Volkoff, H., Hoskins, L. J., and Tuziak, S. M. (2009). Influence of intrinsic signals and environmental cue on the endocrine control of feeding in fish: potential application in aquaculture. Gen. Comp. Endocrinol. 167, 352–359. doi: 10.1016/j.ygcen.2009.09.001
Volkoff, H., and Peter, R. E. (2000). Effects of CART peptides on food consumption, feeding and associated behaviors in the goldfish, Carassius auratus: actions on neuropeptide Y- and orexin A-induced feeding. Brain Res. 887, 125–133. doi: 10.1016/S0006-8993(00)03001-8
Volkoff, H., and Peter, R. E. (2001a). Characterization of two forms of cocaine- and amphetamine-regulated transcript (CART) peptide precursors in goldfish: molecular cloning and distribution, modulation of expression by nutritional status, and interactions with leptin. Endocrinology 142, 5076–5088. doi: 10.1210/endo.142.12.8519
Volkoff, H., and Peter, R. E. (2001b). Interactions between orexin A, NPY and galanin in the control of food intake of the goldfish, Carassius auratus. Regul. Pept. 101, 59–72. doi: 10.1016/S0167-0115(01)00261-0
Volkoff, H., Sabioni, R. E., and Cyrino, J. E. P. (2016). Appetite regulating factors in dourado, Salminus brasiliensis: cDNA cloning and effects of fasting and feeding on gene expression. Gen. Comp. Endocrinol. 237, 34–42. doi: 10.1016/j.ygcen.2016.07.022
Wacyk, J., Powell, M., Rodnick, K. J., Overturf, K., Hill, R. A., and Hardy, R. (2012). Dietary protein source significantly alters growth performance, plasma variables and hepatic gene expression in rainbow trout (Oncorhynchus mykiss) fed amino acid balanced diets. Aquaculture 356–357, 223–234. doi: 10.1016/j.aquaculture.2012.05.013
Wall, A., and Volkoff, H. (2013). Effects of fasting and feeding on the brain mRNA expressions of orexin, tyrosine hydroxylase (TH), PYY and CCK in the Mexican blind cavefish (Astyanax fasciatus mexicanus). Gen. Comp. Endocrinol. 183, 44–52. doi: 10.1016/j.ygcen.2012.12.011
Wang, W. G., Chen, X., Jiang, H., and Jiang, Z. Y. (2008). Effects of ghrelin on glucose-sensing and gastric distension sensitive neurons in rat dorsal vagal complex. Regul. Peptides 146, 169–175. doi: 10.1016/j.regpep.2007.09.007
Waterson, M. J., and Horvath, T. L. (2015). Neuronal regulation of energy homeostasis: beyond the hypothalamus and feeding. Cell Metab. 22, 962–970. doi: 10.1016/j.cmet.2015.09.026
Wauson, E. M., Lorente-Rodríguez, A., and Cobb, M. H. (2013). Minireview: nutrient sensing by G protein-couple receptors. Mol. Endocrinol. 27, 1188–1197. doi: 10.1210/me.2013-1100
Westermark, G. T., Falkmer, S., Steiner, D. F., Chan, S. J., Engstrom, U., and Westermark, P. (2002). Islet amyloid polypeptide is expressed in the pancreatic islet parenchyma of the teleostean fish, Myoxocephalus (cottus) scorpius. Comp. Biochem. Physiol. B. 133, 119–125. doi: 10.1016/S1096-4959(02)00113-6
White, S. L., Volkoff, H., and Devlin, R. H. (2016). Regulation of feeding behavior and food intake by appetite-regulating peptides in wild-type and growth hormone-transgenic coho salmon. Hormone. Behav. 84, 18–28. doi: 10.1016/j.yhbeh.2016.04.005
Williams, I., Williams, K. C., Smith, D. M., and Jones, M. (2006). Polka-dot grouper, Cromileptes altivelis, can utilize dietary fat efficiently. Aquac. Nutr. 12, 379–387. doi: 10.1111/j.1365-2095.2006.00437.x
Won, E. T., Baltzegar, D. A., Picha, M. E., and Borski, R. J. (2012). Cloning and characterization of leptin in a Perciform fish, the striped bass (Morone saxatilis): control of feeding and regulation by nutritional state. Gen. Comp. Endocrinol. 178, 98–107. doi: 10.1016/j.ygcen.2012.04.019
Wong, K. K., Ng, S. Y., Lee, L. T., Ng, H. K., and Chow, B. K. (2011). Orexins and their receptors from fish to mammals: a comparative approach. Gen. Comp. Endocrinol. 171, 124–130. doi: 10.1016/j.ygcen.2011.01.001
Wu, Q., Boyle, M. P., and Palmiter, R. D. (2009). Loss of GABAergic signaling by AgRP neurons to the parabrachial nucleus leads to starvation. Cell. 137, 1225–1234. doi: 10.1016/j.cell.2009.04.022
Xu, M., and Volkoff, H. (2007). Molecular characterization of prepro-orexin in Atlantic cod (Gadus morhua): cloning, localization, developmental profile and role in food intake regulation. Mol. Cell. Endocrinol. 271, 28–37. doi: 10.1016/j.mce.2007.03.003
Yamanome, T., Amano, M., and Takahasi, A. (2005). White background reduces the occurrence of staining, activates melanin-concentrating hormone and promotes somatic growth in barfin flounder. Aquaculture 244, 323–329. doi: 10.1016/j.aquaculture.2004.11.020
Yan, A. F., Chen, T., Chen, S., Ren, C. H., Hu, C. Q., Cai, Y. M., et al. (2016). Goldfish leptin-AI and leptin-AII: function and central mechanism in feeding control. Int. J. Mol. Sci. 17:783. doi: 10.3390/ijms17060783
Yan, A., Zhang, L., Tang, Z., Zhang, Y., Qin, C., Li, B., et al. (2011). Orange spotted grouper (Epinephelus coioides) orexin: molecular cloning, tissue expression, ontogeny, daily rhythm and regulation of NPY gene expression. Peptides 32, 1363–1370. doi: 10.1016/j.peptides.2011.05.004
Yang, J., Brown, M. S., Liang, G., Grishin, N. V., and Goldstein, J. L. (2008). Identification of the acyltransferase that octanoylates ghrelin, an appetite-stimulating peptide hormone. Cell 132, 387–396. doi: 10.1016/j.cell.2008.01.017
Yi, C. X., van der Vliet, J., Dai, J., Yin, G., Ru, L., and Buijs, R. M. (2006). Ventromedial arcuate nucleus communicates peripheral metabolic information to the suprachiasmatic nucleus. Endocrinology 147, 283–294. doi: 10.1210/en.2005-1051
Yokobori, E., Azuma, M., Nishiguchi, R., Kang, K. S., Kamijo, M., Uchiyama, M., et al. (2012). Neuropeptide Y stimulates food intake in the zebrafish, Danio rerio. J. Neuroendocrinol. 24, 766–773. doi: 10.1111/j.1365-2826.2012.02281.x
Zhang, C., Forlano, P. M., and Cone, R. D. (2012). AgRP and POMC neurons are hypophysiotropic and coordinately regulate multiple endocrine axes in a larval teleost. Cell Metab. 15, 256–264. doi: 10.1016/j.cmet.2011.12.014
Zhang, H., Chen, H., Zhang, Y., Li, S., Lu, D., Zhang, H., et al. (2013). Molecular cloning, characterization and expression profiles of multiple leptin genes and a leptin receptor gene in orange-spotted grouper (Epinephelus coioides). Gen. Comp. Endocrinol. 181, 295–305. doi: 10.1016/j.ygcen.2012.09.008
Zhang, Y., Liu, Y., Huang, X., Liu, X., Jiao, B., Meng, Z., et al. (2008). Two alternatively spliced GPR39 transcripts in seabream: molecular cloning, genomic organization, and regulation of gene expression by metabolic signals. J. Endocrinol. 199, 457–470. doi: 10.1677/JOE-07-0608
Zheng, H., and Berthoud, H.-R. (2008). Neural systems controlling the drive to eat: mind versus metabolism. Physiology 23, 75–83. doi: 10.1152/physiol.00047.2007
Zhong, C., Song, Y., Wang, Y., Zhang, T., Duan, M., Li, Y., et al. (2013). Increased food intake in growth hormone-transgenic common carp (Cyprinus carpio L.) may be mediated by upregulating Agouti-related protein (AgRP). Gen. Comp. Endocrinol. 192, 81–88. doi: 10.1016/j.ygcen.2013.03.024
Keywords: food intake, fish, hypothalamus, review, nutrient sensing, circadian rhythm, leptin, ghrelin
Citation: Delgado MJ, Cerdá-Reverter JM and Soengas JL (2017) Hypothalamic Integration of Metabolic, Endocrine, and Circadian Signals in Fish: Involvement in the Control of Food Intake. Front. Neurosci. 11:354. doi: 10.3389/fnins.2017.00354
Received: 08 October 2016; Accepted: 07 June 2017;
Published: 26 June 2017.
Edited by:
Hubert Vaudry, University of Rouen, FranceReviewed by:
Kouhei Matsuda, University of Toyama, JapanLidy Verburg-van Kemenade, Wageningen University and Research, Netherlands
Copyright © 2017 Delgado, Cerdá-Reverter and Soengas. This is an open-access article distributed under the terms of the Creative Commons Attribution License (CC BY). The use, distribution or reproduction in other forums is permitted, provided the original author(s) or licensor are credited and that the original publication in this journal is cited, in accordance with accepted academic practice. No use, distribution or reproduction is permitted which does not comply with these terms.
*Correspondence: José L. Soengas, anNvZW5nYXNAdXZpZ28uZXM=