- 1Neuroscience Institute Cavalieri Ottolenghi, Orbassano, Italy
- 2Department of Veterinary Sciences, University of Turin, Torino, Italy
After five decades of research in adult neurogenesis (AN) it is far from easy to make a balance. If this field was a movie genre, brain repair goals would be a dreary mystery (with cell replacement therapies approaching fantasy), opportunities would be high quality science fiction, and limits could well belong to a hopeless thriller. Though apparently depicting a pessimistic screenplay, these aspects actually represent very exciting plots in which the only pitfall had been the attitude of those main characters (the scientists) who, starting with the re-discovery of AN (Paton and Nottebohm, 1984; Lois and Alvarez-Buylla, 1994), looked for neuronal cell replacement. The chimera of regenerative outcomes led to an exponential burst of studies: more than 7500 articles on PubMed with the keyword “adult neurogenesis.” Why such an interest many years after the first demonstration of AN (Altman and Das, 1965)? Maybe because the first isolation of neural stem cells (NSCs) took place in the same period (Reynolds and Weiss, 1992), thus making it possible to figure out continuous replenishment of new neurons throughout a brain's life (Gage, 2000; Alvarez-Buylla et al., 2001). At the same time, the possibility to play in vitro with the NSC plasticity (Galli et al., 2003) might explain why the AN articles in PubMed become 23,000 when the keyword “neural stem cell” is employed.
Revisiting the History of AN
Most AN review articles start with Altman's pioneering studies, disregarded at the time by most neurobiologists and then upgraded to the death of a dogma (Gross, 2000). What is more difficult to find is a critical evaluation of what happened after the nineties. Briefly, an intense phase of AN characterization contributed to persuade the scientific community that stem cells actually persist in the adult mammalian brain (Palmer et al., 1997; Doetsch et al., 1999), making the integration of new neurons a real phenomenon producing anatomical and functional changes (Gage, 2000; Alvarez-Buylla et al., 2001; Lledo et al., 2006). The stem cell niches of two main neurogenic sites (subventricular zone and hippocampal dentate gyrus) were identified and progressively defined in their structure and regulation (Figure 1). On these solid bases, a sort of gold rush-like fever aiming at demonstrating new sites of AN grew exponentially (Gould et al., 1999, 2001; Zhao et al., 2003; Dayer et al., 2005; Shapiro et al., 2007). Yet, some of the “alternative” neurogenic regions were subsequently denied by independent studies (references in Bonfanti and Peretto, 2011; Nacher and Bonfanti, 2015). In parallel, it was shown that neurogenesis can be induced by different types of injury or disease (lesion-induced, reactive neurogenesis), either by mobilization of cells from the neurogenic sites (Arvidsson et al., 2002) or by local activation of parenchymal progenitors (Magnusson et al., 2014; Nato et al., 2015; Figure 1). Nevertheless, though large numbers of neuroblasts can be produced in response to stroke or inflammation (Arvidsson et al., 2002; Ohira et al., 2010; Magnusson et al., 2014; Nato et al., 2015), the mechanisms of such responses as well as the ultimate fate of the newborn cells remain largely unknown, as acknowledged by leading experts in the field (Lindvall and Kokaia, 2015). In addition, only limited spontaneous recovery occurs (Sohur et al., 2006; Bonfanti, 2011) and some promising results published on megahit journals have not been reproduced (Magavi et al., 2000; Nakatomi et al., 2002). Finally, the huge effort for obtaining regenerative outcomes by using exogenous sources of stem/progenitor cells has also led, until now, to scarce results in terms of reliability and effectiveness (Li et al., 2010), although some therapeutic perspectives might come from the use of stem cell-derived dopaminergic cells in Parkinson disease (Barker et al., 2015).
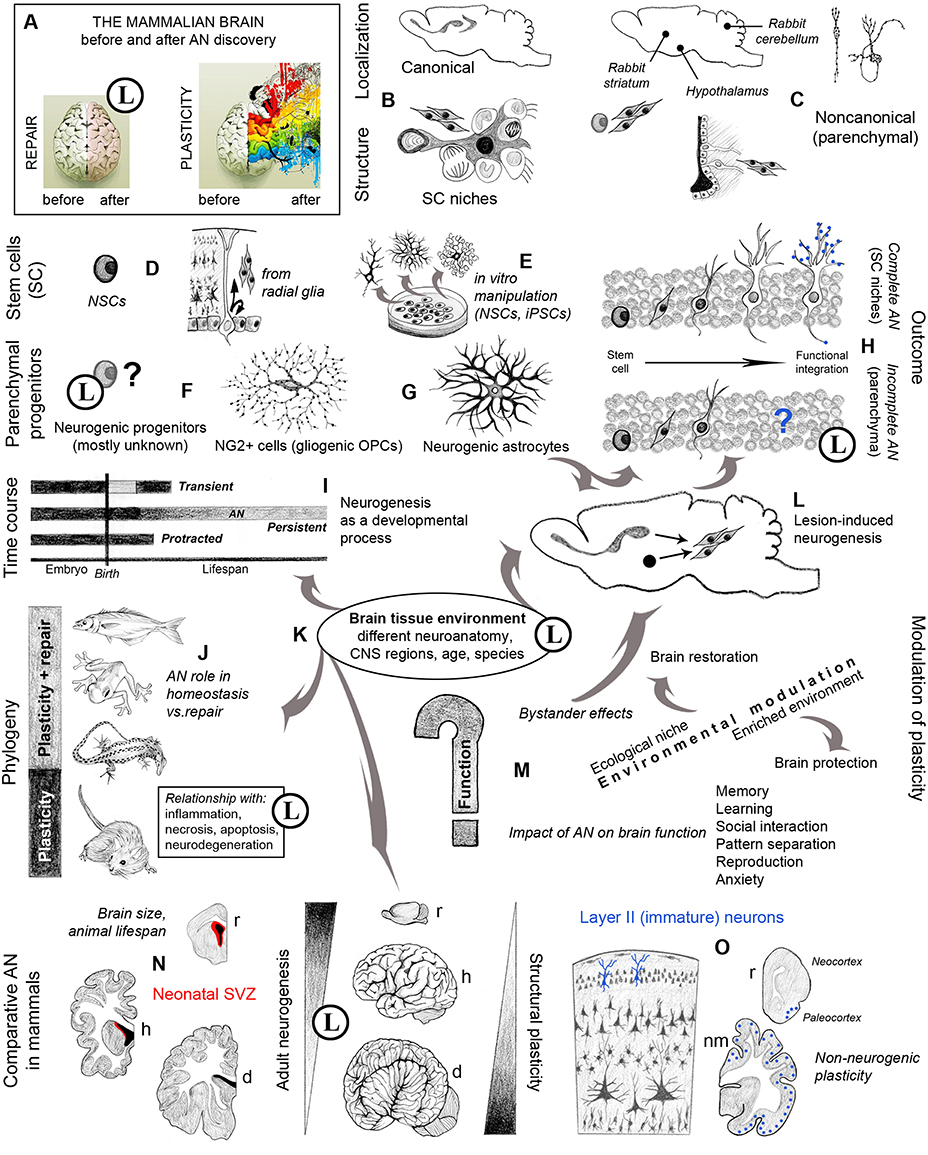
Figure 1. Graphic representation of multifaceted aspects and new opportunities arisen in neurobiology by the study of adult neurogenesis (AN). Circled L indicate when substantial limits are also present. (A) An image originally referring to brain hemisphere asymmetry (http://thebiointernet.org/training-of-right-brain-hemisphere-and-intuitive-information-sight-in-bratislava/) is used here to represent the new vision of brain plasticity after AN discoveries; beside remarkable limits still existing in brain repair (pale pink), most opportunities involve new forms of structural plasticity with respect to the old dogma of a static brain (rainbow colors). (B) Canonical sites of AN, harboring well characterized stem cell niches (Tong and Alvarez-Buylla, 2014; Vadodaria and Gage, 2014). (C) Different types and locations of non-canonical neurogenesis do occur in various brain regions, depending on the species (Luzzati et al., 2006; Ponti et al., 2008; Feliciano et al., 2015). (D) NSCs are astrocytes originating from bipotent radial glia cells (Kriegstein and Alvarez-Buylla, 2009); (E) the occurrence of stem cells in the brain gives rise to (theoretically endless) in vitro manipulations. (F) Parenchymal progenitors are less known; most of them are gliogenic, yet some are responsible for species-specific/region-specific, non-canonical neurogenesis, and some others can be activated after lesion (G) (Nishiyama et al., 2009; Feliciano et al., 2015; Nato et al., 2015). (H) The outcome of canonical and non-canonical neurogenesis is different, only the former leading to functional integration of the newborn neurons (Bonfanti and Peretto, 2011); blue dots: synaptic contacts between the new neurons and the pre-existing neural circuits. (I) Strictly speaking, AN should be restricted to the continuous, “persistent” genesis of new neurons, which is different from “protracted” neurogenesis (delayed developmental processes, e.g., postnatal genesis of cerebellar granule cells, postnatal streams of neuroblasts directed to the cortex; Luzzati et al., 2003; Ponti et al., 2006, 2008), and “transient” genesis of neuronal populations within restricted temporal windows (e.g., striatal neurogenesis in guinea pig; Luzzati et al., 2014). (L) Reactive neurogenesis can be observed in different injury/disease states both as a cell mobilization from neurogenic sites and as a local activation of parenchymal progenitors (Arvidsson et al., 2002; Magnusson et al., 2014; Nato et al., 2015). (J) Evolutionary constraints have dramatically reduced the reparative role of AN, involving tissue reactions far more deleterious than in non-mammalian vertebrates (Weil et al., 2008; Bonfanti, 2011). (K) Failure in mammalian CNS repair/regeneration is likely linked to mature tissue environment, clearly refractory to new neuron integration outside the two canonical NSC niches and relative neural systems; this fact confines AN to physiological/homeostatic roles, which remain undefined in terms of “function.” (M) The role of AN strictly depends on the animal species, evolutionary history and ecological niche; its rate and outcome is affected by different internal and external cues; although not being strictly a function, AN can impact several brain functions (Voss et al., 2013; Aimone et al., 2014; Amrein, 2015). (N) Different anatomy, physiology, and lifespan in mammals do affect AN rate and outcome; periventricular AN is highly reduced in large-brained mammals (Sanai et al., 2011; Paredes et al., 2015; Parolisi et al., 2015). (O) Studies on AN carried out by using markers of immaturity (e.g., DCX and PSA-NCAM) have revealed other forms of plasticity (non-neurogenic), being well represented in large-brained mammals (Gomez-Climent et al., 2008; Bonfanti and Nacher, 2012). r, rodents; h, humans; d, dolphins; nm, non-rodent mammals. Drawings by the Author.
How can we find an explanation for recurrent failures in obtaining cell replacement from AN? Maybe the answer resides in a psychological attitude: the initial burst of optimism affecting scientists with the biased vision that “new neurons equals brain repair” persisted too long under translational pressures, in forgetfulness of a basic fact: the mammalian central nervous system (CNS) evolved to be substantially nonrenewable, relatively hardwired, non-self repairing (Weil et al., 2008). Further proof come from examples of spontaneous “parenchymal” (non-canonical) neurogenesis detectable in other mammals: the outcome of these newly-produced neurons is quite different from that performed in canonical NSC niches (Feliciano et al., 2015) since “transient” neural cells are mostly produced (Gould et al., 2001; Luzzati et al., 2014). More recently, some neurogenic activity has been shown in the hypothalamus, starting from tanycytes harbored within a germinal layer-derived zone, linked with feeding regulation and energy balance, and responding to external stimuli (Migaud et al., 2010). Yet, low levels of neurons are generated in basal conditions, and their final outcome is far from clear.
Hence, if regarding AN as a “full biological process” (from NSC activation to neuronal integration), all neurogenic phenomena occurring out of the hippocampus and olfactory bulb should be classified as “incomplete” (Bonfanti and Peretto, 2011), both spontaneously-occurring and reactive neurogenic events appearing as “unwanted hosts” in the mature brain tissue (Figure 1K).
The Big Questions in AN
By putting together data learned over 50 years of AN research with CNS evolutionary history, it appears clear that: (i) AN has lost most of its capacity for brain repair in mammals with respect to other vertebrates (Grandel and Brand, 2013), its role being largely restricted to physiological plasticity of specific systems (Peretto and Bonfanti, 2014); (ii) this feature might not primarily depend on the availability of stem cells (AN does exist in mammals!) rather on CNS structural, cellular, molecular organization, as a result of its postnatal development and immunological responses (Bonfanti, 2011). Hence, one big question concerns the intermix of biological events leading to such a loss of regenerative capacity.
Many scientists working in the field focus on the question: how NSCs divide and regulate their quiescent/active state in vivo? (in the perspective of modulating—usually intended as “increasing”—their mitotic activity and neuronal fate). These actually are crucial points in NSC basic biology. Yet, beside the common viewpoint considering the neurogenic potential of NSCs to be beneficial, the fact is emerging that having more new neurons or synapses is not always better (Tang et al., 2014; e.g., hippocampal AN can be implicated in memory erasure, Akers et al., 2014; Kitamura and Inokuchi, 2014). By contrast, I consider as essential questions: whether, how, when different types of progenitor cells can produce a progeny which can actually survive and functionally integrate in the brain regions in which they are needed, out of the two canonical niches. Even within the niches, specific subsets of progenitors occupying precise topographical subregions produce only selected neuronal types for selected tissue domains (Obernier et al., 2014), thus confirming that mature brain neurogenic plasticity occurs only within restricted bounds. Also in gliogenesis, the amount of oligodendrocyte precursor cells (OPCs) generated daily in the adult CNS (Young et al., 2013; Boda and Buffo, 2014) clashes with the slow rate of myelin turnover, suggesting that only a small fraction of them actually integrate. Moreover, they appear able to sustain remyelination after acute lesion or disease but not in chronic phases (Franklin, 2002).
A fundamental issue regards the molecular and cellular features which make the mature mammalian brain environment refractory to substantial reshaping or repair, both in physiological and pathological states, with respect to the permissive conditions existing in non-mammalian vertebrates (Kyritsis et al., 2014; Figures 1J,K). Unfortunately, the tools at present available to address such aspect are scarce. One possible way could reside in neurodevelopmental studies aimed at unraveling how the embryonic, permissive tissue environment shifts to mature, more restrictive conditions (Peretto et al., 2005), taking into account that a regulated balance of stability and plasticity is required for optimal functioning of neuronal circuits (Abraham and Robins, 2005; Akers et al., 2014). This approach could open new landscapes from the re-expression of developmental programs (Sohur et al., 2012) to the cutting edge frontier of homeosis (Arlotta and Hobert, 2015).
Another fundamental question remains substantially unanswered (and often skipped by scientists hurrying in search for reparative roles of AN): concerns the function of AN (Figure 1M). It seems clear that AN can play a physiological role in memory and learning, yet rapid adaptation of hippocampal neurogenesis to experimental challenges appears to be a characteristic of laboratory rodents, whereas low or missing AN in bats and dolphins argues against a critical role in spatial learning (Amrein and Lipp, 2009). Wild mammals show species-specific, rather stable hippocampal neurogenesis, which appears related to demands that characterize the niche exploited by a species rather than to acute events in the life of its members (Amrein, 2015). It is worthwhile to remember that AN itself should not be considered as a “function,” rather a tool the brain can use to perform different functions (see also Hersman et al., 2016). As stated by Anderson and Finlay (2014), “Mounting evidence from allometric, developmental, comparative, systems-physiological, neuroimaging, and neurological studies suggests that brain elements are used and reused in multiple functional systems.” They suggest that “this variable allocation can be seen in neuroplasticity over the life span,” and that “the same processes are evident in brain evolution (interaction between evolutionary and developmental mechanisms to produce distributed and overlapping functional architectures in the brain).” That is to say: brain evolution is an ultimate expression of neuroplasticity, and more systematic information about evolutionary perspectives is needed to set out the question of the normal functionality of new neurons.
Astrocytes and Other, Widely Ramified, Opportunities
The most counterintuitive discovery in half a century of AN research concerned the central role of astrocytes as primary progenitors for neuron production (Alvarez-Buylla et al., 2001). Across the years, new roles for these glial cells progressively emerged in different steps of the AN process, from maintenance of the NSC niche, through substrate for migration and functional integration of the newlyborn neurons (Sultan et al., 2015), to that of parenchymal progenitors activated by lesion (Magnusson et al., 2014; Nato et al., 2015). The regional and temporal heterogeneity of astrocytes should be among the big issues for future investigation of brain plasticity (Bayraktar et al., 2015), but this is only one example indicating how deeply different is our vision of brain structure and function before and after AN discovery. More recent breakthroughs concern the modulatory effects of lifestyle on AN (e.g., how exercise protects and restores the brain; Voss et al., 2013), and many emerging roles of the new neurons in impacting brain functions such as social interaction, reproduction, memory, learning, pattern separation, overgeneralization of sensory stimuli, and anxiety disorders (Leuner and Gould, 2010; Sahay et al., 2011; Feierstein, 2012; Kheirbek et al., 2012; Figure 1M). Furthermore, a vast range of “bystander effects” acting through paracrine or immunemodulatory mechanisms can exert beneficial effects by modifying the microenvironment at the injury site through the release of chemokines/cytokines (Martino et al., 2011; Kokaia et al., 2012; Pluchino and Cossetti, 2013). Other ramifications involve the big chapter of widespread gliogenesis (Nishiyama et al., 2009), whose effects are not limited to glial cell renewal, since bystander functions are also emerging for OPCs (Boda and Buffo, 2014; Birey et al., 2015). Yet, in the complex intermix of interactions involved in AN, most processes remain ill-defined as “ghost outcomes” of the stem cell activity (including the transient existence of the progeny), thus being worthwhile of further investigation.
Finally, unexpected trends are emerging from comparative studies showing how the spatial and temporal extent of AN dramatically decreases in large-brained, long-living species (e.g., humans and dolphins; Sanai et al., 2011; Parolisi et al., 2015; Patzke et al., 2015) with respect to small-brained, short-living rodents (Paredes et al., 2015; Figure 1N). The use of markers usually expressed in newly born neurons (e.g., doublecortin) led to reveal the existence of immature, non-newly generated cells (Gomez-Climent et al., 2008) which are more abundant in large-brained species (Luzzati et al., 2009; Bonfanti and Nacher, 2012; Figure 1O). This fact opens new hypothesis about the evolutionary choices in terms of structural plasticity among mammals, again underlining the importance of comparative studies (Lindsey and Tropepe, 2006; Bonfanti et al., 2011).
Conclusion
Even if we are still far from healing most brain lesions and neurodegenerative diseases, we have gained a fully new vision of brain plasticity (Figure 1A). In AN history, it seems that scientists have made serious sins in their approach. Yet, there are many reasons for forgiveness linked to the extremely innovative character of their work aimed at unraveling the dynamic nature of a brain tissue constrained within limits of invariability imposed by evolution. Five decades after the first demonstration of AN we still need to place it in the domain of basic research aimed at unraveling cellular, molecular, and evolutionary aspects of an extremely complex biological process. Maintaining a substantial independence from translational pressures (what implies hard work of teaching the values of fundamental research to grantmakers) could lead to higher achievements: the understanding of brain function and plasticity.
Looking back to its origin and forward to its future, the AN research field is maybe one of the best movies ever shot in the neurosciences, with passion and love for the unknown prevailing at the beginning of the story, then gradually shifting to magical realism toward the end.
Author Contributions
The author confirms being the sole contributor of this work and approved it for publication.
Conflict of Interest Statement
The author declares that the research was conducted in the absence of any commercial or financial relationships that could be construed as a potential conflict of interest.
Acknowledgments
The author wishes to thank Barbara Finlay and Enrica Boda for helpful discussion, and Christopher Pullen for English revision.
References
Abraham, W. C., and Robins, A. (2005). Memory retention - the synaptic stability versus plasticity dilemma. Trends Neurosci. 28, 73–78. doi: 10.1016/j.tins.2004.12.003
Aimone, J. B., Li, Y., Lee, S. W., Clemenson, G. D., Deng, W., and Gage, F. H. (2014). Regulation and function of adult neurogenesis: from genes to cognition. Physiol. Rev. 94, 1991–1026. doi: 10.1152/physrev.00004.2014
Akers, K. G., Martinez-Canabal, A., Restivo, L., Yiu, A. P., De Cristofaro, A., Hsiang, H. L., et al. (2014). Hippocampal neurogenesis regulates forgetting during adulthood and infancy. Science 344, 598–602. doi: 10.1126/science.1248903
Altman, J., and Das, G. D. (1965). Post-natal origin of microneurones in the rat brain. Nature 207, 953–956. doi: 10.1038/207953a0
Alvarez-Buylla, A., Garcia-Verdugo, J. M., and Tramontin, A. D. (2001). A unified hypothesis on the lineage of neural stem cells. Nat. Rev. Neurosci. 2, 287–293. doi: 10.1038/35067582
Amrein, I. (2015). Adult hippocampal neurogenesis in natural populations of mammals. Cold Spring Harb. Perspect. Biol. 7:a021295. doi: 10.1101/cshperspect.a021295
Amrein, I., and Lipp, H. P. (2009). Adult hippocampal neurogenesis of mammals: evolution and life history. Biol. Lett. 5, 141–144. doi: 10.1098/rsbl.2008.0511
Anderson, M. L., and Finlay, B. L. (2014). Allocating structure to function: the strong links between neuroplasticity and natural selection. Front. Hum. Neurosci. 7:918. doi: 10.3389/fnhum.2013.00918
Arlotta, P., and Hobert, O. (2015). Homeotic transformations of neuronal cell identities. Trends Neurosci. 38, 751–762. doi: 10.1016/j.tins.2015.10.005
Arvidsson, A., Collin, T., Kirik, D., Kokaia, Z., and Lindvall, O. (2002). Neuronal replacement from endogenous precursors in the adult brain after stroke. Nat. Med. 8, 963–970. doi: 10.1038/nm747
Barker, R. A., Drouin-Ouellet, J., and Parmar, M. (2015). Cell-based therapies for Parkinson disease-past insights and future perspectives. Nat. Rev. Neurol. 11, 492–503. doi: 10.1038/nrneurol.2015.123
Bayraktar, O. A., Fuentealba, L. C., Alvarez-Buylla, A., and Rowitch, D. H. (2015). Astrocyte development and heterogeneity. Cold Spring Harb. Perspect. Biol. 7:a020362. doi: 10.1101/cshperspect.a020362
Birey, F., Kloc, M., Chavali, M., Hussein, I., Wilson, M., Christoffel, D. J., et al. (2015). Genetic and stress-induced loss of NG2 glia triggers emergence of depressive-like behaviors through reduced secretion of FGF2. Neuron 88, 941–956. doi: 10.1016/j.neuron.2015.10.046
Boda, E., and Buffo, A. (2014). Beyond cell replacement: unresolved roles of NG2- expressing progenitors. Front. Neurosci. 8:122. doi: 10.3389/fnins.2014.00122
Bonfanti, L. (2011). From hydra regeneration to human brain structuralplasticity: a long trip through narrowing roads. ScientificWorldJournal 11, 1270–1299. doi: 10.1100/tsw.2011.113
Bonfanti, L., and Nacher, J. (2012). New scenarios for neuronal structural plasticity in non-neurogenic brain parenchyma: The case of cortical layer II immature neurons. Prog. Neurobiol. 98, 1–15. doi: 10.1016/j.pneurobio.2012.05.002
Bonfanti, L., and Peretto, P. (2011). Adult neurogenesis in mammals–a theme with many variations. Eur. J. Neurosci. 34, 930–950. doi: 10.1111/j.1460-9568.2011.07832.x
Bonfanti, L., Rossi, F., and Zupanc, G. K. (2011). Towards a comparative understanding of adult neurogenesis. Eur. J. Neurosci. 34, 845–846. doi: 10.1111/j.1460-9568.2011.07816.x
Dayer, A. G., Cleaver, K. M., Abouantoun, T., and Cameron, H. A. (2005). New GABAergic interneurons in the adult neocortex and striatum are generated from different precursors. J. Cell Biol. 168, 415–427. doi: 10.1083/jcb.200407053
Doetsch, F., Caille, I., Lim, D. A., Garcia-Verdugo, J. M., and Alvarez-Buylla, A. (1999). Subventricular zone astrocytes are neural stem cells in the adult mammalian brain. Cell 97, 703–716. doi: 10.1016/S0092-8674(00)80783-7
Feierstein, C. E. (2012). Linking adult olfactory neurogenesis to social behavior. Front. Neurosci. 6:173. doi: 10.3389/fnins.2012.00173
Feliciano, D. M., Bordey, A., and Bonfanti, L. (2015). Noncanonical sites of adult neurogenesis in the mammalian brain. Cold Spring Harb. Perspect. Biol. 7:a018846 doi: 10.1101/cshperspect.a018846
Franklin, R. J. (2002). Why does remyelination fail in multiple sclerosis? Nat. Rev. Neurosci. 3, 705–714. doi: 10.1038/nrn917
Gage, F. H. (2000). Mammalian neural stem cells. Science 287, 1433–1438. doi: 10.1126/science.287.5457.1433
Galli, R., Gritti, A., Bonfanti, L., and Vescovi, A. L. (2003). Neural stem cells: an overview. Circ. Res. 92, 598–608. doi: 10.1161/01.RES.0000065580.02404.F4
Gomez-Climent, M. A., Castillo-Gomez, E., Varea, E., Guirado, R., Blasco-Ibanez, J. M., Crespo, C., et al. (2008). A population of prenatallygenerated cells in the rat paleocortex maintains an immature neuronal phenotypeinto adulthood. Cereb. Cortex 18, 2229–2240. doi: 10.1093/cercor/bhm255
Gould, E., Reeves, A. J., Graziano, M. S., and Gross, C. G. (1999). Neurogenesis in the neocortex of adult primates. Science 286, 548–552. doi: 10.1126/science.286.5439.548
Gould, E., Vail, N., Wagers, M., and Gross, C. G. (2001). Adult-generated hippocampal and neocortical neurons in macaques have a transient existence. Proc. Natl. Acad. Sci. U.S.A. 98, 10910–10917. doi: 10.1073/pnas.181354698
Grandel, H., and Brand, M. (2013). Comparative aspects of adult neural stem cell activity in vertebrates. Dev. Genes Evol. 223, 131–147. doi: 10.1007/s00427-012-0425-5
Gross, C. G. (2000). Neurogenesis in the adult brain: death of a dogma. Nat. Rev. Neurosci. 1, 67–73. doi: 10.1038/35036235
Hersman, S., Rodriguez Barrera, V., and Fanselow, M. (2016). Assigning functions to adult-born neurons: a theoretical framework for characterizing neural manipulation of learning. Front. Syst. Neurosci. 9:182. doi: 10.3389/fnsys.2015.00182
Kheirbek, M. A., Klemenhagen, K. C., Sahay, A., and Hen, R. (2012). Neurogenesis and generalization: a new approach to stratify and treat anxiety disorders. Nat. Neurosci. 15, 1613–1620. doi: 10.1038/nn.3262
Kitamura, T., and Inokuchi, K. (2014). Role of adult neurogenesis in hippocampal-cortical memory consolidation. Mol. Brain 7:13. doi: 10.1186/1756-6606-7-13
Kokaia, Z., Martino, G., Schwartz, M., and Lindvall, O. (2012). Cross-talk between neural stem cells and immune cells: the key to better brain repair? Nat. Neurosci. 15, 1078–1087. doi: 10.1038/nn.3163
Kriegstein, A., and Alvarez-Buylla, A. (2009). The glial nature of embryonic and adult neural stem cells. Annu. Rev. Neurosci. 32, 149–184. doi: 10.1146/annurev.neuro.051508.135600
Kyritsis, N., Kizil, C., and Brand, M. (2014). Neuroinflammation and central nervous system regeneration in vertebrates. Trends Cell Biol. 24, 128–135. doi: 10.1016/j.tcb.2013.08.004
Leuner, B., and Gould, E. (2010). Structural plasticity and hippocampal function. Annu. Rev. Psychol. 61, 111–140. doi: 10.1146/annurev.psych.093008.100359
Li, L., Jiang, Q., Ding, G., Zhang, L., Zhang, Z. G., Li, Q., et al. (2010). Effects of administration route on migration and distribution of neural progenitor cells transplanted into rats with focal cerebral ischemia, an MRI study. J. Cereb. Blood Flow Metab. 30, 653–662. doi: 10.1038/jcbfm.2009.238
Lindsey, B. W., and Tropepe, V. (2006). A comparative framework for understanding the biological principles of adult neurogenesis. Prog. Neurobiol. 80, 281–307. doi: 10.1016/j.pneurobio.2006.11.007
Lindvall, O., and Kokaia, Z. (2015). Neurogenesis following stroke affecting the adult brain. Cold Spring Harb. Perspect. Biol. 7:a019034. doi: 10.1101/cshperspect.a019034
Lledo, P. M., Alonso, M., and Grubb, M. S. (2006). Adult neurogenesis and functional plasticity in neuronal circuits. Nat. Rev. Neurosci. 7, 179–193. doi: 10.1038/nrn1867
Lois, C., and Alvarez-Buylla, A. (1994). Long-distance neuronal migration in the adult mammalian brain. Science 264, 1145–1148. doi: 10.1126/science.8178174
Luzzati, F., Bonfanti, L., Fasolo, A., and Peretto, P. (2009). DCX and PSA-NCAM expression identifies a population of neurons preferentially distributed in associative areas of different pallial derivatives and vertebrate species. Cereb. Cortex 19, 1028–1041. doi: 10.1093/cercor/bhn145
Luzzati, F., De Marchis, S., Fasolo, A., and Peretto, P. (2006). Neurogenesis in the caudate nucleus of the adult rabbit. J. Neurosci. 26, 609–621. doi: 10.1523/JNEUROSCI.4371-05.2006
Luzzati, F., Nato, G., Oboti, L., Vigna, E., Rolando, C., Armentano, M., et al. (2014). Quiescent neuronal progenitors are activated in the juvenile guinea pig lateral striatum and give rise to transient neurons. Development 141, 4065–4075. doi: 10.1242/dev.107987
Luzzati, F., Peretto, P., Aimar, P., Ponti, G., Fasolo, A., and Bonfanti, L. (2003). Glia-independent chains of neuroblasts through the subcortical parenchyma of the adult rabbit brain. Proc. Natl. Acad. Sci. U.S.A. 100, 13036–13041. doi: 10.1073/pnas.1735482100
Magavi, S. S., Leavitt, B. R., and Macklis, J. D. (2000). Induction of neurogenesis in the neocortex of adult mice. Nature 405, 951–955. doi: 10.1038/35016083
Magnusson, J. P., Göritz, C., Tatarishvili, J., Dias, D. O., Smith, E. M. K., Lindvall, O. et al. (2014). A latent neurogenic program in astrocytes regulated by Notch signaling in the mouse. Science 346, 237–241. doi: 10.1126/science.346.6206.237
Martino, G. V., Pluchino, S., Bonfanti, L., and Schwartz, M. (2011). Brain regeneration in physiology and pathology: the immune signature driving therapeutic plasticity of neural stem cells. Phys. Rev. 91, 1281–1304. doi: 10.1152/physrev.00032.2010
Migaud, M., Batailler, M., Segura, S., Duittoz, A., Franceschini, I., and Pillon, D. (2010). Emerging new sites for adult neurogenesis in the mammalian brain: a comparative study between the hypothalamus and the classical neurogenic zones. Eur. J. Neurosci. 32, 2042–2052. doi: 10.1111/j.1460-9568.2010.07521.x
Nacher, J., and Bonfanti, L. (2015). New neurons from old beliefs in the adult piriform cortex? Front. Neuroanat. 9:62. doi: 10.3389/fnana.2015.00062
Nakatomi, H., Kuriu, T., Okabe, S., Yamamoto, S., Hatano, O., Kawahara, N., et al. (2002). Regeneration of hippocampal pyramidal neurons after ischemic brain injury by recruitment of endogenous neural progenitors. Cell 110, 429–441. doi: 10.1016/S0092-8674(02)00862-0
Nato, G., Caramello, A., Trova, S., Avataneo, V., Rolando, C., Taylor, V., et al. (2015). Striatal astrocytes produce neuroblasts in an excitotoxic model of Huntington disease. Development 142, 840–845. doi: 10.1242/dev.116657
Nishiyama, A., Komitova, M., Suzuki, R., and Zhu, X. (2009). Polydendrocytes (NG2 cells): multifunctional cells with lineage plasticity. Nat. Rev. Neurosci. 10, 9–22. doi: 10.1038/nrn2495
Obernier, K., Tong, C. K., and Alvarez-Buylla, A. (2014). Restricted nature of adult neural stem cells: re-evaluation of their potential for brain repair. Front. Neurosci. 8:162. doi: 10.3389/fnins.2014.00162
Ohira, K., Furuta, T., Hioki, H., Nakamura, K. C., Kuramoto, E., Tanaka, Y., et al. (2010). Ischemia-induced neurogenesis of neocortical layer 1 progenitor cells. Nat. Neurosci. 13, 173–179. doi: 10.1038/nn.2473
Palmer, T. D., Takahashi, J., and Gage, F. H. (1997). The adult rat hippocampus contains primordial neural stem cells. Mol. Cell. Neurosci. 8, 389–404. doi: 10.1006/mcne.1996.0595
Paredes, M. F., Sorrells, S. F., Garcia-Verdugo, J. M., and Alvarez-Buylla, A. (2015). Brain sixe and limits to adult neurogenesis. J. Comp. Neurol. 524, 646–664. doi: 10.1002/cne.23896
Parolisi, R., Peruffo, A., Messina, S., Panin, M., Montelli, S., Giurisato, M., et al. (2015). Forebrain neuroanatomy of the neonatal and juvenile dolphin (T. truncatus & S. coeruloalba). Front. Neuroanat. 9:140. doi: 10.3389/fnana.2015.00140
Paton, J. A., and Nottebohm, F. (1984). Neurons generated in adult brain are recruited into functional circuits. Science 225, 1046–1048. doi: 10.1126/science.6474166
Patzke, N., Spocter, M. A., Karlsson, K. Æ., Bertelsen, M. F., Haagensen, M., Chawana, R., et al. (2015). In contrast to many other mammals, cetaceans have relatively small hippocampi that appear to lack adult neurogenesis. Brain Struct. Funct. 220, 361–383. doi: 10.1007/s00429-013-0660-1
Peretto, P., and Bonfanti, L. (2014). Major unsolved points in adult neurogenesis: doors open on a translational future? Front. Neurosci. 8:154. doi: 10.3389/fnins.2014.00154
Peretto, P., Giachino, C., Aimar, P., Fasolo, A., and Bonfanti, L. (2005). Chain formation and glial tube assembly in the shift from neonatal to adult subventricular zone of the rodent forebrain. J. Comp. Neurol. 487, 407–427.
Pluchino, S., and Cossetti, C. (2013). How stem cells speak with host immune cells in inflammatory brain diseases. Glia 61, 1379–1401. doi: 10.1002/glia.22500
Ponti, G., Aimar, P., and Bonfanti, L. (2006). Cellular composition and cytoarchitecture of the rabbit subventricular zone (SVZ) and its extensions in the forebrain. J. Comp. Neurol. 498, 491–507. doi: 10.1002/cne.21043
Ponti, G., Peretto, P., and Bonfanti, L. (2008). Genesis of neuronal and glial progenitors in the cerebellar cortex of peripuberal and adult rabbits. PLoS ONE 3:e2366. doi: 10.1371/journal.pone.0002366
Reynolds, B. A., and Weiss, S. (1992). Generation of neurons and astrocytes from isolated cells of the adult mammalian central nervous system. Science 255, 1707–1710. doi: 10.1126/science.1553558
Sahay, A., Wilson, D. A., and Hen, R. (2011). Pattern separation: a common function for new neurons in hippocampus and olfactory bulb. Neuron 70, 582–588. doi: 10.1016/j.neuron.2011.05.012
Sanai, N., Nguyen, T., Ihrie, R. A., Mirzadeh, Z., Tsai, H. H., Wong, M., et al. (2011). Corridors of migrating neurons in the human brain and their decline during infancy. Nature 478, 382–386. doi: 10.1038/nature10487
Shapiro, L. A., Ng, K. L., Kinyamu, R., Whitaker-Azmitia, P., Geisert, E. E., Blurton-Jones, M., et al. (2007). Origin, migration and fate of newly generated neurons in the adult rodent piriform cortex. Brain Struct. Funct. 212, 133–148. doi: 10.1007/s00429-007-0151-3
Sohur, U. S., Arlotta, P., and MacKlis, J. D. (2012). Developmental controls are re-expressed during induction of neurogenesis in the neocortex of young adult mice. Front. Neurosci. 6:12. doi: 10.3389/fnins.2012.00012
Sohur, U. S., Emsley, J. G., Mitchell, B. D., and MacKlis, J. D. (2006). Adult neurogenesis and repair of the adult CNS with neural progenitors, precursors, and stem cells. Philos. Trans. R. Soc. Lond. B Biol. Sci. 361, 1477–1497. doi: 10.1098/rstb.2006.1887
Sultan, S., Li, L., Moss, J., Petrelli, F., Casse, F., Gebara, E., et al. (2015). Synaptic integration of adult-born hippocampal neurons is locally controlled by astrocytes. Neuron 88, 1–16. doi: 10.1016/j.neuron.2015.10.037
Tang, G., Gudsnuk, K., Kuo, S.-H., Cotrina, M. L., Rosoklija, G., Sosunov, A., et al. (2014). Loss of mTOR-dependent macroautophagy causes autistic-like synaptic pruning deficits. Neuron 83, 1131–1143. doi: 10.1016/j.neuron.2014.07.040
Tong, C. K., and Alvarez-Buylla, A. (2014). SnapShot: adult neurogenesis in the V-SVZ. Neuron 81, 220–220. doi: 10.1016/j.neuron.2013.12.004
Vadodaria, K. C., and Gage, F. H. (2014). SnapShot: adult hippocampal neurogenesis. Cell 156, 1114–1114. doi: 10.1016/j.cell.2014.02.029
Voss, M. W., Vivar, C., Kramer, A. F., and van Praag, H. (2013). Bridging animal and human models of exercise-induced brain plasticity. Trends Cogn. Sci. 17, 525–544. doi: 10.1016/j.tics.2013.08.001
Weil, Z. M., Norman, G. J., DeVries, A. C., and Nelson, R. J. (2008). The injured nervous system: A Darwinian perspective. Prog. Neurobiol. 86, 48–59. doi: 10.1016/j.pneurobio.2008.06.001
Young, K. M., Psachoulia, K., Tripathi, R. B., Dunn, S.-J., Cossell, L., Attwell, D., et al. (2013). Oligodendrocyte dynamics in the healthy adult CNS: evidence for myelin remodeling. Neuron 77, 873–885. doi: 10.1016/j.neuron.2013.01.006
Keywords: neural stem cells, brain repair, parenchymal progenitors, astrocytes, comparative neurogenesis, mammalian brain
Citation: Bonfanti L (2016) Adult Neurogenesis 50 Years Later: Limits and Opportunities in Mammals. Front. Neurosci. 10:44. doi: 10.3389/fnins.2016.00044
Received: 04 January 2016; Accepted: 01 February 2016;
Published: 19 February 2016.
Edited by:
Alino Martinez-Marcos, Universidad de Castilla, SpainReviewed by:
Juan Manuel Encinas, Ikerbasque - the Basque Foundation for Science, SpainDavid Díaz, Universidad de Salamanca, Spain
Copyright © 2016 Bonfanti. This is an open-access article distributed under the terms of the Creative Commons Attribution License (CC BY). The use, distribution or reproduction in other forums is permitted, provided the original author(s) or licensor are credited and that the original publication in this journal is cited, in accordance with accepted academic practice. No use, distribution or reproduction is permitted which does not comply with these terms.
*Correspondence: Luca Bonfanti, bHVjYS5ib25mYW50aUB1bml0by5pdA==