- 1Neuroecology Group, School of Animal Biology and UWA Oceans Institute, The University of Western Australia, Crawley, WA, Australia
- 2Centre for Fish and Fisheries Research, School of Veterinary and Life Sciences, Murdoch University, Murdoch, WA, Australia
Very few studies have described brain scaling in vertebrates throughout ontogeny and none in lampreys, one of the two surviving groups of the early agnathan (jawless) stage in vertebrate evolution. The life cycle of anadromous parasitic lampreys comprises two divergent trophic phases, firstly filter-feeding as larvae in freshwater and secondly parasitism as adults in the sea, with the transition marked by a radical metamorphosis. We characterized the growth of the brain during the life cycle of the pouched lamprey Geotria australis, an anadromous parasitic lamprey, focusing on the scaling between brain and body during ontogeny and testing the hypothesis that the vast transitions in behavior and environment are reflected in differences in the scaling and relative size of the major brain subdivisions throughout life. The body and brain mass and the volume of six brain structures of G. australis, representing six points of the life cycle, were recorded, ranging from the early larval stage to the final stage of spawning and death. Brain mass does not increase linearly with body mass during the ontogeny of G. australis. During metamorphosis, brain mass increases markedly, even though the body mass does not increase, reflecting an overall growth of the brain, with particularly large increases in the volume of the optic tectum and other visual areas of the brain and, to a lesser extent, the olfactory bulbs. These results are consistent with the conclusions that ammocoetes rely predominantly on non-visual and chemosensory signals, while adults rely on both visual and olfactory cues.
Introduction
Lampreys are extant relatives of an early and diverse group of jawless vertebrates (Kumar and Hedges, 1998; Heimberg et al., 2008; Janvier, 2008; Smith et al., 2013). The results of early studies on the agnathan nervous system (Johnston, 1902; Heier, 1948; Nieuwenhuys, 1977) have thus been used as an indicator of the ancestral condition of the vertebrate brain (Fritzsch and Northcutt, 1993a; Butler and Hodos, 1996; Northcutt, 2002; Gilland and Baker, 2005; Khonsari et al., 2009; Suárez et al., 2014). The design or bauplan of the vertebrate brain and the developmental mechanisms that underlie their subdivisions are considered to be highly conserved (Striedter, 2005; Ota and Kuratani, 2007; Guerin et al., 2009; Charvet et al., 2011). However, it is expected that the various sensory modalities and other neural specializations will evolve, to a degree, in association with ecological niche, and that this relationship will be reflected in adapted behaviors and/or enhanced cognitive capabilities (Barton et al., 1995; Barton and Harvey, 2000; De Winter and Oxnard, 2001). Indeed, brain size and the relative development of major brain subdivisions vary at intraspecific, interspecific, and ontogenetic levels across a range of vertebrates (e.g., Kruska, 2005; Gonda et al., 2013) in relation to factors such as life style, habitat, and behavior (e.g., Pollen et al., 2007; Yopak and Montgomery, 2008; Barton and Capellini, 2011), as well as phylogenetic and developmental constraints (e.g., Finlay and Darlington, 1995; Yopak et al., 2010).
The size of the brain relative to the body (scaling) has long since been used in studies of brain development and evolution (Ariëns Kapper, 1936; Gould, 1975; Deacon, 1990; Aboitiz, 1996), in which brain mass (E) is characterized as a function of body mass (S) with Snell's formula: E = k*S∝ or log E = ∝log S + k, where ∝ = allometric slope or scaling power. It is a common assumption that encephalization (a larger than expected brain size for a given body size) reflects enhanced cognitive capabilities (Jerison, 1977; Ebbesson, 1980; Lefebvre et al., 2004), although this is still the subject of debate (Healy and Rowe, 2007; Herculano-Houzel, 2012). Previous studies have examined encephalization of the brain of jawless fishes (Platel and Delfini, 1981; Ebinger et al., 1983; Platel and Vesselkin, 1989; Wicht, 1996) and have shown that agnathans, particularly lampreys, possess a relatively small brain and some of the highest degrees of intraspecific variation in brain and body mass when compared to any other vertebrate group (Ebinger et al., 1983; Platel and Delfini, 1986). However, these data have been collected from very few species and no consideration has yet been given to changes in encephalization and brain organization that may occur throughout their life cycle. Indeed, ontogenetic studies of diverse groups of vertebrates have shown that the brain grows at different rates during their lifespan, with the rates being greatest in the embryonic and early postnatal phases (Bauchot et al., 1979; Gille and Salomon, 2000; Fu et al., 2013; Ngwenya et al., 2013). Although some studies have shown shifts in ecology and corresponding shifts in brain development occur in fishes (e.g., Brandstätter and Kotrschal, 1990; Wagner, 2003; Lisney et al., 2007; Iribarne and Castelló, 2014), there are no data on the pattern of encephalization or brain subdivision scaling during the ontogeny of lampreys.
The life cycle of lampreys is very conserved (Chang et al., 2014; Potter et al., 2015), consisting of a prolonged and sedentary larval phase, followed by metamorphosis into the free-swimming adult phase (Manzon et al., 2015), as illustrated in Figure 1. In the pouched lamprey Geotria australis, which is widely distributed in temperate regions of the southern hemisphere (Renaud, 2011), the life cycle has an approximate duration of 8 years (Potter et al., 1980, 1983; Potter and Hilliard, 1986).
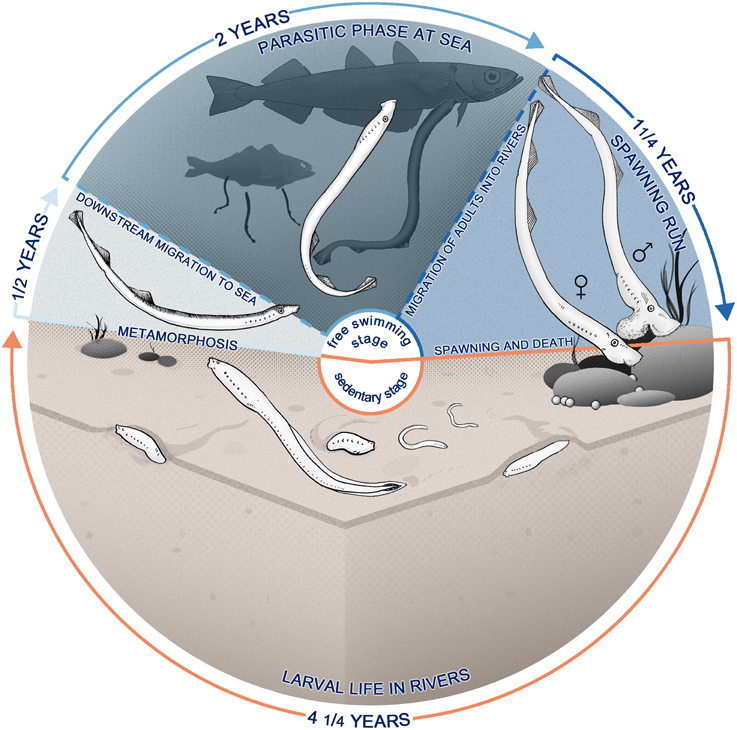
Figure 1. Life cycle of Geotria australis presenting anadromous reproductive and feeding migrations. After hatching (bottom), the larvae—also called ammocoetes—burrow in the sediments of rivers, becoming a microphagous filter-feeder for approximately 4 years. The larval phase is followed by a metamorphosis, a non-feeding transition to adult stage that lasts for approximately 6 months (left), where there is a marked transformation in most of the body systems. Animals at this stage start migrating downstream and enter the sea, where they locate a teleost host and feed on its flesh using a specialized buccal apparatus (top). G. australis return years later to the rivers, where they start a long upstream migration, subsisting only on body reserves, which are expended in developing secondary sexual characteristics and reproductive behavior. Upstream migrants finally spawn and die (right).
After hatching, the larvae (ammocoetes) burrow in the soft sediments of streams and rivers, filtering detritus, algae and other organisms from the overlying water (Piavis, 1971; Moore and Mallat, 1980; Richardson et al., 2010; Dawson et al., 2015). Ammocoetes have rudimentary eyes with a largely undifferentiated retina (Meyer-Rochow and Stewart, 1996; Villar-Cheda et al., 2008), and also a well-developed non-visual photoreceptive system, e.g., the pineal organ (García-Fernández and Foster, 1994; Deliagina et al., 1995; Melendez-Ferro et al., 2002; Vigh et al., 2002). In fact, they exhibit nocturnal habits with synchronized, seasonal downstream movements (Gritzenko, 1968; Potter, 1980), which may be controlled by circadian rhythms. An octaval lateral line system provide additional mechano-, electro-, and photo-perception, with photoreception being mediated by dermal non-visual photoreceptors located in the tail (Ronan, 1988; Ronan and Bodznick, 1991; Deliagina et al., 1995; Gelman et al., 2007). Ammocoetes also have well-developed gustatory (Baatrup, 1985; Barreiro-Iglesias et al., 2010) and olfactory (Vandenbossche et al., 1995; Zielinski et al., 2005) systems, and behavioral evidence has revealed that rotting potato haulms attracted ammocoetes when placed on the bed of freshwater streams (Enequist, 1937; Hardisty and Potter, 1971), indicating that they may actively search for food using chemosensory cues. Therefore, taste and olfaction are likely important drivers of their behavior.
The metamorphosis of anadromous parasitic species of lampreys, such as G. australis, involves major morphological and physiological changes and the development of new sensory and motor capabilities. These include the development of image-forming eyes with the potential for pentachromacy in G. australis (Meyer-Rochow and Stewart, 1996; Collin et al., 1999, 2003; Davies et al., 2007), a reduction of lateral line-mediated negative phototaxis that marks a switch from non-visual to visual perception (Binder et al., 2013), the rearrangement of the gustatory and lateral line systems (Currie and Carlsen, 1988; Jørgensen, 2005; Gelman et al., 2008; Barreiro-Iglesias et al., 2010), and the development of a tooth-bearing suctorial disc and “tongue-like” piston with the associated musculature and trigeminal motor innervation (Homma, 1978; Lethbridge and Potter, 1981). Metamorphosis also involves fundamental changes in a number of internal organs, including the intestine and gills, which enable the lamprey to osmoregulate in the sea (Youson et al., 1977; Hilliard et al., 1983; Bartels and Potter, 2004; Reis-Santos et al., 2008).
During the marine parasitic phase, G. australis swims toward and attaches to a host, often a teleost fish, and feeds from its flesh (Hilliard et al., 1985; Renaud et al., 2009), thereby increasing in body size from approximately 100 mm and 0.75 g to 620 mm and 220 g (Potter et al., 1980, 1983). There is strong evidence that during its marine parasitic phase, G. australis occupies an epipelagic niche in the sea and exhibits diurnal habits (Potter et al., 1979; Cobley, 1996; Collin et al., 1999; Davies et al., 2007). Following the completion of the parasitic phase, the adult lamprey re-enters rivers cued mainly by pheromones that are released by the ammocoetes (Vrieze and Sorensen, 2001; Sorensen et al., 2005; Vrieze et al., 2010, 2011), where they migrate upstream at night (Jellyman et al., 2002; Binder and McDonald, 2007; Vrieze et al., 2011). Geotria australis does not feed during its exceptionally long spawning run, using body reserves accumulated during the marine phase to develop secondary sexual characters and mature gonads (Potter et al., 1983; Paton et al., 2011). The life cycle culminates in spawning and subsequent death.
During its life cycle, G. australis occupies different ecological niches and encounters diverse environmental conditions, yet there have been no comprehensive studies that have quantified the changes in brain organization corresponding to these marked changes in ecology and behavior. In this study, we assess changes in relative brain size (encephalization) and in the volume of six major brain structures (brain organization) at different phases of the life cycle in G. australis. We hypothesize that differences in brain size and organization will reflect the pronounced environmental and physiological changes that lampreys experience during ontogeny.
Methods
All the procedures described below were performed in accordance with the ethical guidelines of The University of Western Australia Animal Ethics Committee—Research Project RA/3/100/917.
Data Collection
Forty specimens of G. australis were analyzed in this study, representing six different points in their life cycle (ammocoetes of second, third, and fourth age class, downstream migrants, upstream migrants, and maturing adults). Specimens within a stage had the same fixation and preservation methods, as shown in Supplementary Table 1, and were captured in the same year (ammocoetes and downstream migrants) or in different years (upstream migrants and maturing adults). Morphometrics (body mass, body length, sex) were collected for each individual when possible. After a period of fixation, the brain was removed from the chondrocranium. The meninges were removed and the cranial nerves were cut to within 0.5 mm of the base. The brains were blotted and weighed to the nearest 0.1 mg (ammocoetes and downstream migrants) or 1 mg (upstream migrants and maturing adults). Neither brain nor body mass were corrected for shrinkage due to fixation.
Photographs of the lateral and dorsal views of each brain were taken using a Leica EC3 camera attached to a Nikon SMZ-745T dissecting microscope. Brains were submerged in a solution of 0.1 M phosphate buffer while photographed to prevent volume distortions caused by dehydration of the tissue. Measurements of length were taken for each of the six brain structures as shown in Figure 2. Brain structures were determined from previously published descriptions of the brain and the cranial nerve distribution in lampreys (Nieuwenhuys and Nicholson, 1998). The length (l), height (h), and width (w) of the olfactory bulbs (OB), telencephalic hemispheres (Te), the pineal organ (PO), the optic tectum (OT), the octaval-trigeminal area (OCT; defined as the anterior region of the rhombencephalon comprising the V–VIII nerves), and the gustatory area (GUS; defined as the posterior region of the rhombencephalon comprising the IX–XII nerves) were measured using ImageJ (Rasband, 1997) as described previously (Huber et al., 1997; Wagner, 2001; Yopak and Lisney, 2012). The pineal organ was dissected out of the brain and photographed separately, see Figure 2B.
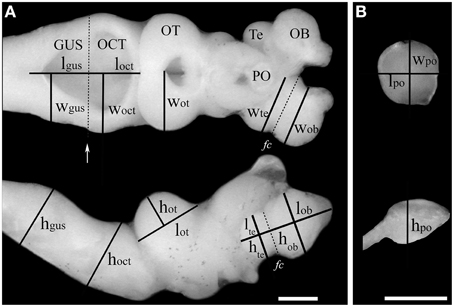
Figure 2. Estimation of the volume of brain structures using the ellipsoid method. Measurements of length (l), width (w), and height (h) of six brain structures taken from a dorsal view (A, top) or lateral view (A, bottom) of the brain of an upstream migrating G. australis. In the case of the olfactory bulbs and the telencephalic vesicles, these were defined as parallel or perpendicular lines to the Fissura circularis (fc), which is highlighted with a discontinuous line in the telencephalon. The limit of the octavo-trigeminal and gustatory areas was defined by a line running parallel to the posterior end of the head of the eighth nerve (white arrow). (B) The same measurements were performed in the pineal organ after it was dissected and separated from the remainder of the brain. OB: olfactory bulbs, Te: telencephalic vesicles, PO: pineal organ, OT: optic tectum, OCT: octavo-trigeminal area, Gus: gustatory area. Scale bars = 1 mm.
Volumes of each major brain structure were estimated using the ellipsoid method, which approximates the volume of a structure by assuming it takes the shape of an idealized ellipsoid, or a fraction of it as shown below (Huber et al., 1997; Wagner, 2001). The general formula of an ellipsoid is:
where a, b, c are the radii of the ellipsoid. Using the measurements of length (l), height (h), and width (w) shown for each structure in Figure 2, the volumes were defined as:
for the OB, Te, PO, and the OT, which were all modeled as half ellipsoids,
while the volume of the OCT and GUS were modeled as a quarter of an ellipsoid. In the case of bilateral structures (i.e., OB, Te, and TO), the values of the volumes were doubled. Volume estimates were not corrected for ventricular volume. Total brain volume was calculated from total brain mass using the estimated density of the brain tissue, d = 1.036 mg/mm3 (Stephan, 1960).
Age Determination
The approximate age of the ammocoete samples was estimated from length-frequency histograms for larval and metamorphosing representatives of G. australis (Potter et al., 1980; Potter and Hilliard, 1986). Age of adult stages was inferred from the timing of the upstream migration and sexual maturation (Potter et al., 1983).
Data Analysis
All analyses were performed using the open source software R (R Core Team, 2013). The complete dataset was divided into two subsets, one containing body and brain mass (n = 32) and the other containing total brain and brain structure volume estimates (n = 39).
Linear Models
For brain and brain structure scaling analyses, each data set was log10 transformed to improve normality prior to analysis, after being multiplied by an arbitrary factor (10 and 1000, respectively), in order to obtain positive values of the variables following log10 transformation. We conducted similar analyses on both datasets: we fitted least squares regressions within and between stages, and performed analyses of covariance (ANCOVA), with brain mass as the response variable, body mass as the covariate, and stage as a factor for the brain and body mass comparisons. In the case of the brain structures, total brain structure volume was compared to total brain volume minus total structure volume as a covariate. This was done to account for the bias that exists when a brain subdivision is scaled against total brain mass (which includes the subdivision of interest) (Deacon, 1990; Iwaniuk et al., 2010). To control for similarity within the larval or adult phases of the life cycle, stages were combined in “stage 1” (no combination), “stage 2” (all ammocoetes grouped together), “stage 3” (all adults grouped together), “stage 4” (all ammocoetes grouped together, downstream and upstream migrants grouped together), “stage 5” (all ammocoetes grouped together, upstream migrants and maturing adults grouped together), and “stage 6” (all ammocoetes grouped together, all adults grouped together) (See Supplementary Table 2). Linear models were fitted to each of these factors and the linear assumptions for each were checked using the R package glvla (Pena and Slate, 2014); valid linear models were then compared and selected using the second-order Akaike Information Criterion (AICc); If the best model had a AICc value indistinguishable from the following model(s), they were averaged using multi-model inference methods contained in the R package MuMIn (Barton, 2014), and the relative importance of the factor in the resulting model was used as a criterion for selection. Tukey Post-hoc tests were used to detect differences between groups in the selected models.
Principal Component Analysis
We also used a multivariate approach to determine the clustering of the samples in multidimensional space and characterize the patterns of brain organization of G. australis at each point of the life cycle. Principal component analysis (PCA) was performed using relative volume of each structure, calculated as a fraction of the sum of the volume of all six brain structures measured within a specimen (Wagner, 2001; Lisney et al., 2007). Structure proportions were normalized using the arcsine square root transformation previous to analysis. PCA was run using the autocovariance matrix and the singular value decomposition method for better numerical accuracy.
Results
Brain Scaling
The brain of G. australis shared similar characteristics with those of other species of lampreys (Figure 3) (Wicht, 1996; Nieuwenhuys and Nicholson, 1998). Our analysis of the scaling of brain and body mass in G. australis at successive stages of development revealed that the brain and body have different scaling patterns during ontogeny (Figure 4A). Body mass grows at a higher rate than brain mass in both the adult phase and the analyzed period of the larval phase, a trend that is interrupted during metamorphosis (Figure 4A, arrows), where body mass was similar between downstream migrants and the latest ammocoete stage (Two-tailed Welch t-test, T = 1.98, p = 0.201); however, brain mass was significantly higher in downstream migrants as compared to ammocoetes IV (One-tailed Welch t-test, T = 7.8, p = 0.037).
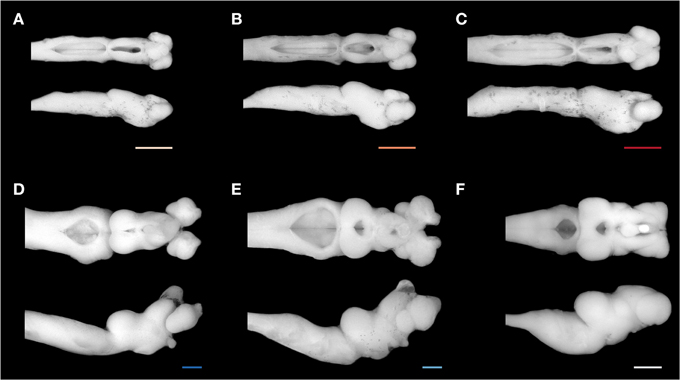
Figure 3. Brain of Geotria australis during ontogeny. A representative brain of each stage studied is shown in a dorsal (top) and lateral view (bottom): (A) second age class ammocoete, (B) third age class ammocoete, (C) fourth age class ammocoete, (D) maturing adult, (E) upstream migrant, and (F) downstream migrant. Note the marked difference between the brain of a late ammocoete and a downstream migrant (C,F). Scale bars = 1 mm.
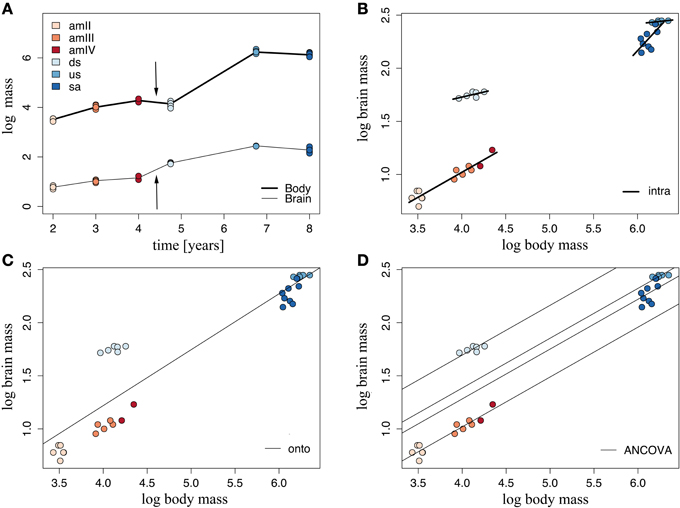
Figure 4. Brain and body growth vary during the ontogeny of Geotria australis. (A) Brain and body mass growth traced over time. Arrows mark the period of metamorphosis. (B) Intraspecific linear regressions, (C) Ontogenetic regressions, and (D) Linear regressions fitted for each stage after an ANCOVA analysis. For the values of the parameters of these regressions, refer to Supplementary Table 4. amII, second year class ammocoetes; am III, third year class ammocoetes; amIV, fourth year class ammocoetes; ds, downstream migrants; us, upstream migrants; sa, spawning adults.
According to the second-order Akaike information criterion, the best model of brain mass as a function of body mass occurred when stage 2 was used as a factor, grouping all ammocoetes together (Supplementary Table 3). We fitted stage-specific (intraspecific) regressions to each of these groups, whose slopes varied across ontogeny (Figure 4B); all groups showed intraspecific negative allometry of brain mass with body mass. The highest rate of brain growth was reached at the larval phase (α = 0.47), followed by downstream and upstream migrants (Supplementary Table 4), while the period of regression of body mass in the course of maturation was accompanied by a steep reduction of brain mass (α = 0.90). We also defined an ontogenetic linear regression as the line of best fit between all specimens, where most of the groups had large deviations from the predicted values of brain mass (Figure 4C), indicating that brain mass does not scale linearly with body mass at all stages in the life cycle of G. australis. These two sets of regressions were combined in an analysis of covariance (ANCOVA), the results of which are illustrated in Figure 4D. These data show that both stage 2 and body mass are significant when explaining the observed variance of brain mass (ANCOVA, p < 0.001), and no significant interaction between factor (stage 2) and covariate (body mass) is found, indicating no significant differences in the slopes calculated for each group in the stage-specific regressions. The ANCOVA calculated a common slope, with a similar value to the slope obtained in the intraspecific regression of ammocoetes, and different intercepts for each group (See Supplementary Table 4), which represent differences in relative brain mass between groups. The Tukey Post-hoc test showed significant differences between all groups of stage 2 (p < 0.001); downstream migrants had the highest intercept, demonstrating an increase in relative brain mass at this stage.
Scaling of Brain Structures
The analyzed brain structures showed different patterns of growth during the life cycle of G. australis. Ontogenetic regressions of total structure volume against total brain volume minus structure volume (hereafter referred to as brain volume) were fitted to each of the structures analyzed and their parameters are tabulated in Supplementary Table 5. A general trend between these regressions was the large deviations from the expected values shown by the downstream migrants, which were positive for the telencephalon and the optic tectum, but negative in the case of the pineal organ, the octavo-trigeminal area and the gustatory area.
The olfactory bulb was the only structure where the observed values fitted the expected values closely in all the stages, supporting a linear scaling of this structure with total brain throughout ontogeny (Figure 5A). Remarkably, the olfactory bulbs showed the steepest hyperallometric growth reported in this study (α = 1.27), generating highly developed olfactory bulbs in upstream migrants and maturing adults. The pineal organ and the octavo-trigeminal area also showed a significant linear fit with total brain volume, as shown in Supplementary Table 5, although this was not the best model for these structures (see below).
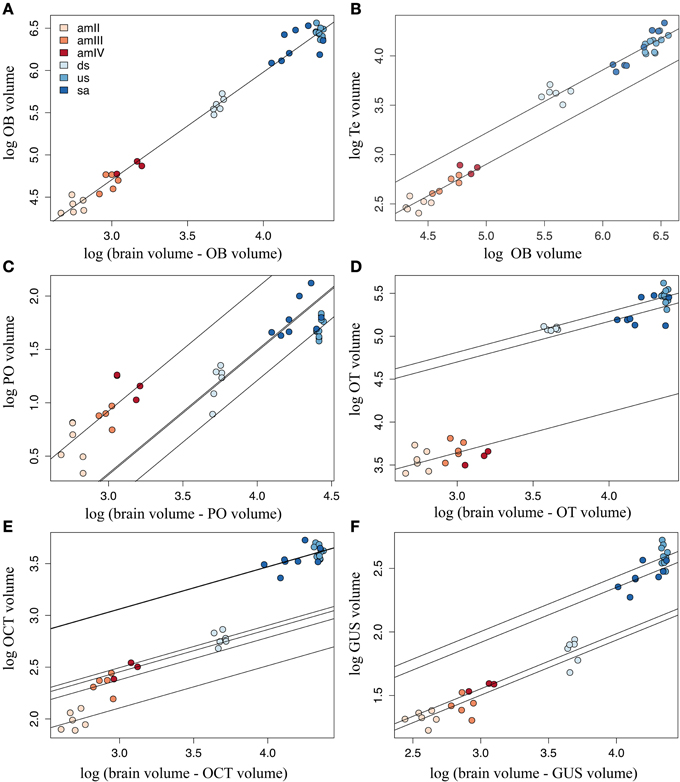
Figure 5. Calculated regression lines after ANCOVA. Best linear models are plotted for each structure, showing the differences in scaling of each structure to the rest of the brain: (A) olfactory bulbs (OB), (B) telencephalic hemispheres (Te), (C) pineal organ (PO), (D) optic tectum (OT), (E) octavo-trigeminal area (OCT), and (F) gustatory area (GUS). For the values of the parameters of these models, refer to Supplementary Table 5. amII, second age class ammocoetes; amIII, third age class ammocoetes; amIV, fourth age class ammocoetes; ds, downstream migrants; us, upstream migrants; sa, spawning adults.
Similar to the olfactory bulbs, the telencephalic hemispheres showed a close fit to brain volume in most stages, but because of the high heteroscedasticity in the values of maturing adults, the linear assumptions were violated in this case and in other tested linear models of the telencephalic hemispheres (results not shown). Nevertheless, we found that these assumptions were valid when fitting the telencephalic volume with the volume of the olfactory bulbs, and thus in this case total olfactory bulbs volume was used as covariate in the ANCOVA analysis. The best model for the telencephalic hemispheres included stage 6 as a factor (Figure 5B). This structure showed linear growth with the olfactory bulbs along the larval phase and an increase in size after metamorphosis, which is maintained throughout the adult phase of the life cycle. However, only a marginal difference was detected between ammocoetes and adults (Tukey Post-hoc test, p = 0.091).
The best models for the pineal organ and the gustatory area had stage 2 as factor, whereas for the octavo-trigeminal area it was stage 1 and for the optic tectum it was stage 4 (Supplementary Table 3). The calculated slope for the pineal organ in the ANCOVA was higher than in the ontogenetic regression, and ammocoetes had the highest intercept (Figure 5C). We found no significant differences between ammocoetes, downstream and upstream migrants, but the pineal organ in maturing adults was significantly different from that of downstream migrants, although only marginally different from upstream migrants (Tukey Post-hoc test, p = 0.017 and 0.053, respectively). The corrected slope for the optic tectum showed two markedly slow phases of growth, larval and adult, with a significant difference in size between them (Tukey Post-hoc test, p < 0.05; Figure 5D); the optic tectum of maturing adults was significantly reduced compared to downstream and upstream migrants (Tukey Post-hoc test, p < 0.05), and not different from the optic tectum of ammocoetes (Tukey Post-hoc test, p = 0.45).
The volume of the gustatory area of the downstream migrants was significantly different to the other stages (Tukey Post-hoc test, p < 0.05), with a shallow slope (α = 0.43). However, considering the value of the calculated intercepts in the ANCOVA of the gustatory area, the downstream migrants clustered with ammocoetes, whereas upstream migrants and maturing adults had higher values of intercepts (Figure 5E). This was also the case for the octavo-trigeminal area, where the volume in downstream migrants was different from all the other stages (Tukey Post-hoc test, p < 0.05) and their volume was closer to ammocoetes than to adults although, in contrast to all other structures, we found that in this area the ammocoetes were best fitted as separate groups, where the second age class ammocoetes had a smaller intercept than other larval stages (Tukey Post-hoc tests: amIII, p = 0.020; amIV, p = 0.083; Figure 5F). Some maturing adults possessed a relatively higher octavo-trigeminal area than upstream migrants, consistent with the modifications of the oral disc and the appearance of the gular sac in this period (Potter et al., 1983; Neira, 1984). However, we did not observed significant differences between these groups. Our results also showed no consistent differences between male and female lampreys in any structure (results not shown).
Multivariate Analysis and Stage Clustering
The principal component analysis performed on the correlation matrix of the relative size of the six brain structures measured in this study provided a clear separation in the multidimensional space of the two phases of the life cycle of G. australis. The relative loadings of the first four components and their relative importance are given in Table 1. The first two components explained 93.3% of the overall variance and their scores are plotted in Figure 6. The first component (PC1) reflects the high loadings for the optic tectum and gustatory area, and secondarily in the olfactory bulbs, separating larvae, which had a relatively large gustatory area and pineal organ, from adults, which had relatively larger optic tecta, olfactory bulbs and telencephalic hemispheres. Similarly, the second component (PC2) separated younger and older individuals within a phase, where older individuals had relatively larger olfactory bulbs and octavo-trigeminal areas than younger individuals in both phases of the life cycle.
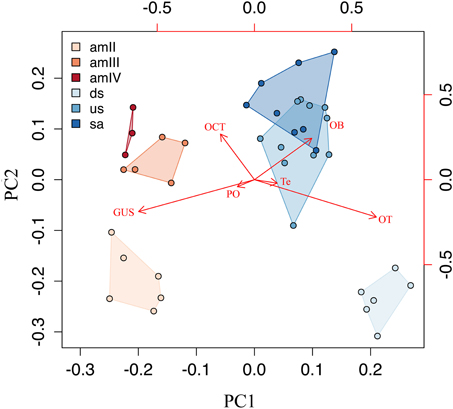
Figure 6. A scatterplot of principal components PC1 and PC2. Principal component analysis, representing the major changes in the composition of the brain during the life cycle. OB, olfactory bulbs; Te, telencephalic hemispheres; PO, pineal organ; OT, optic tectum; OCT, octaval-trigeminal area; GUS, gustatory area; amII, second age class ammocoetes; amIII, third age class ammocoetes; amIV, fourth age class ammocoetes; ds, downstream migrants; us, upstream migrants; sa, spawning adults.
Discussion
Lampreys experience very different behavioral phases during the life cycle, from a microphagous sedentary mode to an active parasitic mode. This study characterized the growth of the brain (encephalization) during the life cycle of G. australis, focusing on the scaling between brain and body throughout ontogeny and testing the hypotheses that the vast transitions in behavior and environment are reflected in differences in both encephalization and the relative development of major brain subdivisions.
The changes occurring in the nervous system of lampreys during ontogeny have attracted the attention of many comparative neurobiologists, who have shown extensive morphological and physiological modifications of the peripheral and central nervous system, such as the development of the visual system (Kennedy and Rubinson, 1977; Kosareva, 1980; De Miguel and Anadon, 1987; Rubinson, 1990; Fritzsch and Northcutt, 1993b; Pombal et al., 1994; Davies et al., 2007; Villar-Cheda et al., 2008). However, in spite of the multiple studies quantifying these changes throughout the life cycle (Tamotsu and Morita, 1986; De Miguel and Anadon, 1987; Currie and Carlsen, 1988; Melendez-Ferro et al., 2003; Vidal Pizarro et al., 2004; Antri et al., 2006), an overall view of the pattern of development of the brain and its organization, including larval and adult phases, has been absent until now.
Brain Scaling
The description of the changes in encephalization during the life cycle of jawless fishes will improve our current understanding brain development at multiple levels. Previous interspecific studies in agnathans have differed on the scaling relationship between brain size and body size of lampreys, ranging from 0.23 (Ebinger et al., 1983) to 0.56 (Platel and Vesselkin, 1988). In addition to discrepancies in the value of the scaling exponent, both studies suffered from low sample sizes, with data on only three (Ebinger et al., 1983) and two (Platel and Vesselkin, 1988) species, out of 41 currently recognized species of lampreys (Potter et al., 2015). This discrepancy in the scaling exponent requires improved resolution, as one value classifies lampreys as being far less encephalized than other gnathostomes, with a slow rate of growth of the brain in relation to the body (α = 0.23), while the other places this group within the known range of the interspecific variation in the scaling exponent between most vertebrate groups (α = 0.56), which usually falls between 0.5 and 0.6 (Striedter, 2005). Similarly, no consensus has been reached with regards to the intraspecific scaling exponent in the sea lamprey Petromyzon marinus, which ranges from −0.04 (Ebinger et al., 1983) to 0.56 (Platel and Delfini, 1986). However, given the dramatic shifts that occur throughout the life history of lampreys, these published values for brain scaling are likely to be highly dependent on when in the life cycle the brains were sampled. In fact, this study shows that, as lampreys advance in their upstream migration, they lose both body and brain mass at different rates, which is reflected in a higher intraspecific scaling factor in maturing adults (Figure 4). This variation between early and late upstream migrants may explain previously reported discrepancies in the intraspecific allometric slope in P. marinus. Nonetheless, it is possible that the observed differences in relative brain mass may also be related to intraspecific variation between separate populations (Gonda et al., 2011) or according to mating strategies (Kolm et al., 2009), which have also been described in lampreys (Hume et al., 2013).
The ontogenetic scaling of brain and body mass in other basal vertebrate groups, such as teleost fishes, has shown that the larvae of both metamorphic (Bauchot et al., 1979; Tomoda and Uematsu, 1996; Wagner, 2003; Sala et al., 2005) and non-metamorphic fishes (Iribarne and Castelló, 2014) exhibit allometric scaling between brain and body size in the early post-hatching development phase, which may be equivalent to the linear phase of growth reported for ammocoetes in this study. However, in the case of metamorphic fishes, such as the rainbow trout Oncorhyncus mykiss or the Japanese eel Anguilla japonica, there is no clear evidence of an increase of encephalization associated with metamorphosis (Bauchot et al., 1979; Tomoda and Uematsu, 1996), as our results suggest for lampreys, but constitutes an interesting point that warrants further investigation and should be an area of future study.
Teleost fishes possess continuous growth of both the body and the nervous system throughout life (Bauchot et al., 1979; Leyhausen et al., 1987), as opposed to amniotes where brain growth plateaus before the animal reach its final body size (reviewed in Striedter, 2005), although there are some exceptions (Ngwenya et al., 2013). Yet in lampreys, our results and previous records on P. marinus (Ebinger et al., 1983) suggest that, in early upstream migrants (end of the parasitic phase), brain growth may have actually reached a plateau, given the low intraspecific scaling factor found at this point of the life cycle (Figure 4B: α = 0.09 for G. australis, α = −0.04 for P. marinus), although these values were not statistically significant in either study. In addition, we found evidence that a relative reduction in brain mass occurs in parallel with the typical reduction of body mass in maturing lampreys (Potter et al., 1983; Paton et al., 2011), which has not been previously shown in other ontogenetic studies of brain scaling in vertebrates. Even though complex behavior is generally associated with larger brains (reviewed by Striedter, 2005), lampreys still exhibit sophisticated behaviors, such as nest construction, in this period (Hardisty and Potter, 1971; Sousa et al., 2012; Johnson et al., 2015).
Brain growth in vertebrates has been described as the result of several processes, including cell growth and the addition and elimination of cells (Pirlot and Bernier, 1991; Candal et al., 2005; Bandeira et al., 2009; Fu et al., 2013; Boyd et al., 2015). In lampreys, neuro- and glio-genesis are restricted to ventricular proliferative zones in late embryos and early to mid larval stages (Vidal Pizarro et al., 2004; Villar-Cheda et al., 2006; Guerin et al., 2009) and, although adult neurogenesis is widespread in other basal vertebrate groups (Kaslin et al., 2008), it is considered mostly absent in lampreys (Villar-Cheda et al., 2006; Kempermann, 2012). Taken together, these results suggest that brain growth from late ammocoetes onwards is mainly due to the addition of glia, cell growth, and the establishment of new synapses that contribute to the formation of plexiform tissue or neuropil, as suggested previously for lampreys (Rovainen, 1979, 1996).
Scaling of Brain Structures
Transitions in habitat and behavior are common during the development of aquatic vertebrates, even if they do not undergo a metamorphic stage, such as recruitment of fish larvae (Kingsford et al., 2002; Kotrschal et al., 2012; McMenamin and Parichy, 2013) and the use of nursery areas in sharks (e.g., Bethea et al., 2004; Heupel and Simpfendorfer, 2011). Usually these transitions are accompanied by ad-hoc sensorimotor specializations (Brandstätter and Kotrschal, 1990; Montgomery and Sutherland, 1997; Lisney et al., 2007; Lecchini et al., 2014). Similarly, adults of both bony and cartilaginous fishes, as well as other vertebrates, possess well-developed adaptations to their ecological niche, which are generally reflected in their nervous system as a variation in the relative size of brain subdivisions (Kotrschal and Palzenberger, 1992; Gonzalez-Voyer et al., 2009; Gonzalez-Voyer and Kolm, 2010; Yopak, 2012). Surprisingly, the relative size of these brain subdivisions appear to be constant between species of parasitic lampreys, despite the diverse aquatic niches in which they inhabit (Renaud, 2011; Potter et al., 2015). We found that the optic tectum and olfactory bulbs in adults of G. australis comprise similar proportions of the brain to that of P. marinus (Platel and Delfini, 1986) and other species of lampreys (Platel and Vesselkin, 1989), concordant with the lack of appreciable neuroanatomical differences in the brain between lamprey species, as reported previously (Platel and Vesselkin, 1989; Nieuwenhuys and Nicholson, 1998). However, we consider that more species of lampreys needs to be examined, including those with alternative life style strategies, such as parasitic and non-parasitic paired species of lampreys, to have a wider perspective of the diversity found in the nervous system of extant agnathans.
Olfactory Bulbs
It has been suggested that the level of variation in the relative size of the major brain subdivisions may occur in particular structure in a modular or mosaic fashion (Barton and Harvey, 2000), or with a concerted pattern of allometric scaling (Finlay and Darlington, 1995). It has recently been shown that most major brain areas in cartilaginous fishes scale with a characteristic slope that may be conserved across other vertebrates, including mammals (Yopak et al., 2010). One notable exception is found in the olfactory bulbs, which maintain a level of statistical independence from total brain size in a range of vertebrate groups (Finlay and Darlington, 1995; Gonzalez-Voyer et al., 2009; Yopak et al., 2010, 2015). At the ontogenetic level, however, our analysis of the scaling of the olfactory bulbs shows the opposite pattern, whereby the olfactory bulbs scale very tightly with total brain size, with a highly hyperallometric growth (Figure 5A).
Multiple functional hypotheses have been proposed to explain the relative size of the olfactory bulbs (reviewed in Yopak et al., 2015), including the relationship of olfactory cues with navigation, which may play an important role in lampreys while finding a host or on their way back to rivers for the spawning run (Siefkes et al., 2003; Johnson et al., 2005, 2009; Sorensen et al., 2005; Wagner et al., 2009). The olfactory spatial hypothesis predicts that the size of the olfactory bulbs should covary with navigational ability, which is supported by the olfactory input to the hippocampus (Jacobs, 2012). The statistical independence of the olfactory bulbs is then substantiated by the fact that the olfactory bulbs, the hippocampus, and other associated areas of the telencephalon do not scale as tightly with brain size as do other brain subdivisions (Finlay and Darlington, 1995; Finlay et al., 2001; Gonzalez-Voyer et al., 2009; Yopak et al., 2010) and can vary across mammalian taxa depending on the influence of olfactory cues in their behavior (Reep et al., 2007). If these theories can be applied in the context of the lamprey life cycle, we would therefore expect that, should homologous olfactory areas exist in the telencephalon of G. australis, they would also scale isometrically with the rest of the brain in this group during ontogeny. Early descriptions of the telencephalon of the lamprey and later hodological evidence have suggested the presence of a hippocampal primordium or medial pallium (Johnston, 1912; Northcutt and Puzdrowski, 1988; Polenova and Vesselkin, 1993; Northcutt and Wicht, 1997). However, scaling of these telencephalic structures have not been studied in agnathans at any level, and even the existence of a medial pallium is disputed by neuroanatomical descriptions based on molecular markers (Pombal and Puelles, 1999; Weigle and Northcutt, 1999; Pombal et al., 2011). Considering that interspecific scaling of the olfactory bulbs has not yet been described in jawless fishes, the available evidence does not permit any definitive conclusions to be made with regard to differences found in the scaling of the olfactory bulbs between lampreys and other vertebrates.
An alternative explanation of the involvement of olfaction in navigation in lampreys is the hypothesis of dual olfaction, which assumes parallel processing of distinct sets of molecules or environmental odors by the main olfactory system and pheromones by the vomeronasal system, following independent pathways in the brain, and acting synergistically in the regulation of olfactory-guided behaviors (reviewed in Suárez et al., 2012). In lampreys, two anatomically distinct sets of olfactory epithelia have been described that show different patterns of central projections, which suggests the existence of a precursor of the vomeronasal system in this group (Ren et al., 2009; Chang et al., 2013). This accessory olfactory system is tightly coupled to motor areas of the brain, constituting an unusual motor system in vertebrates, which is capable of eliciting swimming movements after olfactory stimulation with both naturally occurring odors and pheromones (Derjean et al., 2010). Since lampreys can detect very low (subpicomolar) concentrations of pheromones (Sorensen et al., 2005), this system may be employed in navigation and other behaviors involving pheromone perception, such as searching for a natal river environment to spawn (Siefkes et al., 2003; Johnson et al., 2005, 2009; Sorensen et al., 2005; Wagner et al., 2009). However, whether these differential central projections vary interspecifically and affect the relative size of the olfactory bulbs and/or a tight coupling between development of the olfactory bulbs and motor areas in the brain is unknown and requires further research.
The Telencephalic Hemispheres
Interspecific studies of the scaling of major brain subdivisions have shown that areas of the brain associated with behavioral and motor complexity, e.g., telencephalon and cerebellum, enlarge disproportionately as brain size increases in a range of vertebrates (Finlay and Darlington, 1995; Finlay et al., 2001; Pollen et al., 2007; Yopak et al., 2010). In lampreys, the everted portion of the telencephalon considered in this study (the cerebral hemispheres or telencephalic hemispheres) can be regarded as the multimodal sensorimotor integration center of the telencephalon, providing a neural substrate for orientation movements of the eyes, trunk, and oral movements, due to direct efferent projections to brainstem motor centers and the optic tectum, in a similar fashion to motor control systems of amniote vertebrates (Ericsson et al., 2013; Grillner and Robertson, 2015; Ocaña et al., 2015). The telencephalic hemispheres are also the main target of secondary olfactory projections from the lateral olfactory bulb, which, in turn, receives its primary afferents from the main olfactory epithelium (Northcutt and Puzdrowski, 1988; Northcutt and Wicht, 1997; Ren et al., 2009; Derjean et al., 2010). Therefore, it is not surprising to find a tight scaling relationship between this structure and the olfactory bulbs (R2 = 0.987). In addition, this telencephalic area receives afferent fibers from the dorsal thalamus, possibly relaying visual and other sensory input that converge on this thalamic area (Polenova and Vesselkin, 1993; Northcutt and Wicht, 1997). Although not significant, there is some evidence of differences in the size of the telencephalic hemispheres between larvae and adults (Tukey Post-hoc test, p = 0.091), which may be due to the increase of secondary sensory fibers terminating in this area, as both the primary olfactory system (Vandenbossche et al., 1995; Villar-Cheda et al., 2006) and the primary visual projections to the dorsal thalamus (Kennedy and Rubinson, 1977; Kosareva, 1980) develop during metamorphosis. Despite the various studies on the pallial telencephalon of lampreys, no consensus has been achieved yet in relation to the homology of this area with the pallium of other vertebrates (Northcutt and Puzdrowski, 1988; Nieuwenhuys and Nicholson, 1998; Pombal et al., 2009).
The Pineal Organ
The pineal complex in lampreys is formed by the pineal and the parapineal organs (Eddy and Strahan, 1970; Puzdrowski and Northcutt, 1989; Pombal et al., 1999; Yáñez et al., 1999), which participate in non-visual photo-perception and neuroendocrine control of the circadian rhythms in these animals, as it does in a range of vertebrates (Ekström and Meissl, 1997, 2003; Vernadakis et al., 1998). The pineal organ has also been documented in extinct agnathans, where it was similar in relative size to that of contemporary ammocoetes (Gai et al., 2011), suggesting that non-visual light perception was also highly developed in these extinct groups. The observed morphological and physiological variability of this organ in tetrapods has been linked to latitudinal distribution of the species (Ralph, 1975), nocturnality (Bhatnagar et al., 1986; Haldar and Bishnupuri, 2001), and habitat depth in demersal fishes (Wagner and Mattheus, 2002; Bowmaker and Wagner, 2004), although none of these factors fully explained the variability found in the size and morphology of this organ across species.
The best model for the pineal organ described three distinctive periods of growth in the life cycle of G. australis. First, there was consistent hyperallometric growth throughout the larval phase; in the second period, during early adult life, including the marine parasitic phase, we observed that the growth of this organ plateaus after metamorphosis, where the size of the pineal organ of ammocoetes was not significantly different to that of downstream or upstream migrants, opposite to what was observed in the other brain structures; and third, we found a relative increase in the size of the pineal organ during sexual maturation. A similar pattern of growth has been documented in the pineal organ of the arctic lamprey Lethenteron camtschaticum (Tamotsu and Morita, 1986). The larval phase and sexual maturation periods anticipate important milestones in the ontogeny of lampreys, such as the onset of metamorphosis and spawning, both of which likely depend on the timing of circadian rhythms (Freamat and Sower, 2013). In this regard, it was shown that metamorphosis was prevented with pinealoctomy in G. australis and other species (Eddy and Strahan, 1968; Cole and Youson, 1981), and maturation was delayed in adults of the river lamprey Lampetra fluviatilis after the same procedure (Eddy, 1971).
The Optic Tectum
In lampreys and other non-mammalian vertebrates, the optic tectum is the main primary visual center of the brain, receiving extensive topographic retinal (retinotopic) projections to the superficial layers (Butler and Hodos, 1996; Iwahori et al., 1999; De Arriba and Pombal, 2007; Jones et al., 2009). Electroreceptive and other sensory input also converge onto this tectal map (Bodznick and Northcutt, 1981; Ronan and Northcutt, 1990; Robertson et al., 2006), where the relevance of salient stimuli can be assessed, as in other vertebrates (Karamian et al., 1966, 1984; Pombal et al., 2001; Gruberg et al., 2006; Kardamakis et al., 2015), leading to orienting movements of the eye, head and trunk (Saitoh et al., 2007; Ocaña et al., 2015).
Ontogenetic comparisons of the relative size of the optic tectum have been documented in several species of elasmobranchs (Lisney et al., 2007) and teleost fishes (Brandstätter and Kotrschal, 1990; Kotrschal et al., 1990; Wagner, 2003), and have shown a shift from an initially well-developed visual system, followed by a relative reduction in the size of the optic tectum and a corresponding increase in other sensory brain areas, such as those that process olfactory or lateral line input, as the animal matures. This change in brain organization has been associated with shifts in ecological niche, from a well-lit environment in epipelagic fish larvae or nurseries of juvenile elasmobranchs to a different primary habitat as adults. In contrast to these groups, we report an opposite shift in brain organization. In ammocoetes of G. australis, the optic tectum underwent moderate growth with total brain size (α = 0.47; Figure 5D). In fact, this structure remains mostly undifferentiated and poorly layered during most of the larval phase in lampreys (Kennedy and Rubinson, 1977; De Miguel and Anadon, 1987; De Miguel et al., 1990) and only the central retina is differentiated (Meyer-Rochow and Stewart, 1996; Villar-Cheda et al., 2008). The major growth of the optic tectum occurs in conjunction with the development of the adult eye, in a rapid process that starts at the end of the larval phase and continues during the initial stages of metamorphosis (Potter et al., 1980; De Miguel and Anadon, 1987). Indeed, it is only at the end of the larval phase that the typical retinotopic projections found in adults reach the optic tectum (Jones et al., 2009; Cornide-Petronio et al., 2011). Soon after metamorphosis (downstream migrants), the relative size of the optic tectum is more similar to that of adults than ammocoetes (Supplementary Table 5, Figure 5D).
This rapid development of the visual system explains the lack of a linear fit of the optic tectum in the ontogenetic scaling of this structure with the rest of the brain. We expect that this fast switch from non-visual to visual perception will also affect the scaling of other visual areas of the brain receiving primary retinal input, such as the dorsal thalamus, and that it may be less pronounced in non-visual areas receiving retinal projections, such as the hypothalamus and pretectal area, which are already developed in ammocoetes, where they participate, for example, in non-visual reflexes (De Miguel and Anadon, 1987; Ullen et al., 1995, 1997; Jones et al., 2009). Nevertheless, the scaling of these visual and non-visual areas of the brain has yet to be studied.
Our results suggest that vision may be important during the parasitic phase, reflected in the high development of the optic tectum during metamorphosis. However, the significant reduction in the size of the optic tectum in maturing adults, which is corroborated with reports of eye degeneration during the spawning run (Applegate, 1950), supports previous evidence that vision is not important in lampreys during their upstream migration (Binder and McDonald, 2007; Johnson et al., 2015).
Medulla Oblongata
Interspecies comparisons in gnathostomes and agnathans have shown that the size of the rhombencephalon, i.e., the medulla oblongata plus the cerebellum, is well-predicted from total brain size in both groups (Ebinger et al., 1983; Yopak et al., 2010), although in lampreys only cerebellum-like structures can be identified (Weigle and Northcutt, 1998; Northcutt, 2002; Montgomery et al., 2012). When comparing brain subdivisions, the medulla oblongata had the lowest scaling factor in cartilaginous fishes (Yopak et al., 2010), whereas it was the highest in agnathans (Ebinger et al., 1983). Indeed, the medulla accounts for approximately half of the total brain size in adult lampreys (this study, Platel and Vesselkin, 1989), and even more in early larvae (Scott, 1887), although this is not as obvious in downstream migrants (see below). The medulla is the first to develop cranial nerves in lampreys (Kuratani et al., 1997; Barreiro-Iglesias et al., 2008) and maintains a relatively stable scaling relationship with total brain size during the later larval phase and even throughout metamorphosis (Figures 5E, F). However, there was a significant difference in the size of the octavo-trigeminal area between the second-age class ammocoetes and older stages (see intercepts in Supplementary Table 5), which may be related to the development of a number of the diverse sensory and motor systems located in this brain structure, as discussed previously.
The growth of the medulla oblongata during metamorphosis maintains a tight scaling relationship with total brain size in late ammocoetes, which supports previous findings that the motoneurons of the trigeminal nucleus in lampreys are conserved through metamorphosis, in spite of the massive replacement of muscle in the head during this period (Homma, 1978; Rovainen, 1996). This has also been documented in other metamorphic vertebrates, such as frogs (Alley and Omerza, 1998).
However, while several brain structures, e.g., the olfactory bulbs and the optic tectum, exhibit greater rate of growth during metamorphosis, both the octavo-trigeminal and gustatory areas grow with a slower rate during this phase, which is expressed as a lower proportion of this area compared to total brain volume in downstream migrants. Nonetheless, our results show a later growth phase of this subdivision during the parasitic phase, particularly of the octavo-trigeminal area, which may be associated with the development of the musculature of the ventilatory branchial basket and the oropharyngeal region, and to the scaling of other somatic and sensory functions as body size enlarges during the marine parasitic phase (Aboitiz, 1996; Rovainen, 1996; De Winter and Oxnard, 2001).
Neuroecology of the Life Cycle
Growth of the central nervous system in lampreys is a discontinuous process, with a variable rate of growth of both total brain and its subdivisions throughout life, which was expressed in the relative size of diverse brain structures in each phase of the life cycle (Figure 6). These patterns of brain organization may be interpreted as “cerebrotypes” (Clark et al., 2001; Iwaniuk and Hurd, 2005; Willemet, 2012, 2013), whereby similar patterns of brain organization exist in species that share certain lifestyle characteristics. In this case, different cerebrotypes may in fact exist within a species at different phases of the life cycle.
The ammocoetes of G. australis are less encephalized compared to young adults (downstream migrants), with brains that are characterized by a relatively large gustatory area and a highly developed pineal organ (Figures 3, 6). The relative size of the octavo-trigeminal area is increased in late ammocoetes (Figure 5E), whereas the olfactory bulbs, telencephalic hemispheres and optic tectum were relatively small during the whole larval phase (this study, Scott, 1887). It is possible that these characteristics are related to the requirements of a sessile, burrower lifestyle and/or to filter-feeding specializations in this group. Patterns of brain organization of other filter-feeding vertebrates has been described previously, such as the basking shark Cetorhinus maximus and the whale shark Rhincodon typus (Kruska, 1988; Yopak and Frank, 2009), and mobulid rays (Ari, 2011), which similarly possess a relatively small telencephalon and mesencephalon (Kruska, 1988; Yopak and Frank, 2009). However, given the drastic differences in the ethology between filter feeding jawless and cartilaginous fishes, it is impossible to draw parallels between patterns of brain organization in these groups. Further research is required to determine the existence of common characteristics in brain organization associated with a filter-feeding lifestyle in lampreys.
In contrast to ammocoetes, adult parasitic lampreys are active swimmers who are highly encephalized and possess a battery of well-developed sensory systems during the adult phase, including vision and olfaction. Correspondingly, they also possess a relatively large telencephalon and olfactory bulbs, structures that may be important in navigation (Derjean et al., 2010; Ocaña et al., 2015), and a relatively large optic tectum, which participates in orientation movements and plays a role in visual processing (Saitoh et al., 2007; Kardamakis et al., 2015). Interestingly, some of these features, such high levels of encephalization and a well-developed optic tectum, have also been observed in many coastal-oceanic and pelagic species of both cartilaginous and bony fishes (Lisney and Collin, 2006; Yopak, 2012; Yopak et al., 2015), which may be related to the sensory requirements of the open water habitat across both jawed and jawless fishes.
Conclusions
We have employed a widely-used volumetric approach (Huber et al., 1997; Wagner, 2001; Gonzalez-Voyer et al., 2009; Yopak and Lisney, 2012; Lecchini et al., 2014) to quantify differences in the relative size of major brain structures during the ontogeny of lampreys. Our results demonstrate shifts in encephalization between larvae and adults, as well as considerable differences in the relative size of brain subdivisions. Taken together, these shifts in brain organization may reflect the sensory requirements of this species at each stage of the life cycle. The inclusion of data of the growth of the brain and its subdivisions in embryonic, prolarva, and early larval stages of ammocoetes, metamorphic, as well as individuals sampled during the parasitic phase, will provide a more comprehensive insight of the growth of the brain and body during the life cycle of lampreys and eventually allow the use of alternative mathematical functions to describe the process of growth in each phase (i.e., Gompertz models, e.g., Calabrese et al., 2013).
It is yet to be determined whether this pattern of brain development is conserved in other species of lampreys, but we anticipate that it is, based on how conserved the life cycle is in this group (Potter et al., 2015), which could explain the reported homogeneity of the central nervous system between species of lampreys. Further studies on the changes in the brain of lampreys throughout ontogeny will contribute to the understanding of the evolution of the brain in agnathans and across vertebrates.
Author Contributions
SC, NH, IP, and CS contributed to the conception, RW and CS acquired the data, KY and CS designed the analyses and interpreted the data. CS drafted the article, and all authors collaborated in its revision.
Conflict of Interest Statement
The Reviewer Thomas Lisney declares that, despite being affiliated to the same institution as authors Carlos A. Salas, Kara E. Yopak, Rachael E. Warrington, Nathan S. Hart and Shaun P. Collin, the review process was handled objectively and no conflict of interest exists. The authors declare that the research was conducted in the absence of any commercial or financial relationships that could be construed as a potential conflict of interest.
Acknowledgments
This work was supported by an Australian Research Council Discovery Grant (DP120102327) to SC, UWA SIRF, UIS, and UIS Top Up Scholarships to RW, and UWA SIRF and CONICYT Becas Chile Scholarships to CS. We thank Dr. Maik Kschischo from University of Applied Sciences Koblenz for his advice on growth curves, and Dr. Evelyn Habit from the University of Concepcion for kindly donating samples for this study. We also thank Dr. Macarena Faunes from the University of Auckland and Dr. Howard Gill from Murdoch University for their critical review of the work, and the reviewers who helped to improve a previous version of this article. We are also grateful to Mr. Helios Lara for adapting the illustration on the life cycle of Geotria australis.
Supplementary Material
The Supplementary Material for this article can be found online at: http://journal.frontiersin.org/article/10.3389/fnins.2015.00251
References
Aboitiz, F. (1996). Does bigger mean better? Evolutionary determinants of brain size and structure. Brain Behav. Evol. 47, 225–235. doi: 10.1159/000113243
Alley, K. E., and Omerza, F. F. (1998). Reutilization of trigeminal motoneurons during amphibian metamorphosis. Brain Res. 813, 187–190. doi: 10.1016/S0006-8993(98)00980-9
Antri, M., Cyr, A., Auclair, F., and Dubuc, R. (2006). Ontogeny of 5-HT neurons in the brainstem of the lamprey, Petromyzon marinus. J. Comp. Neurol. 495, 788–800. doi: 10.1002/cne.20910
Applegate, V. C. (1950). Natural history of the sea lamprey, Petromyzon marinus, in Michigan. U. S. Fish Wildlife Serv. Spec. Sci. Rep. Fish. 55, 1–237.
Ari, C. (2011). Encephalization and brain organization of mobulid rays (Myliobatiformes, Elasmobranchii) with ecological perspectives. TOANATJ 3, 1–13. doi: 10.2174/1877609401103010001
Ariëns Kapper, J. (1936). Brain-bodyweight relation in human ontogenesis and the “indice de valeur cérébrale” of Anthony and Coupin. Proc. R. Neth. Acad. Arts Sci. 39, 1019–1028.
Baatrup, E. (1985). Physiological studies on the pharyngeal terminal buds in the larval brook lamprey, Lampetra planeri (Bloch). Chem. Senses 10, 549–558. doi: 10.1093/chemse/10.4.549
Bandeira, F., Lent, R., and Herculano-Houzel, S. (2009). Changing numbers of neuronal and non-neuronal cells underlie postnatal brain growth in the rat. Proc. Natl. Acad. Sci. U.S.A. 106, 14108–14113. doi: 10.1073/pnas.0804650106
Barreiro-Iglesias, A., Anadon, R., and Rodicio, M. C. (2010). The gustatory system of lampreys. Brain Behav. Evol. 75, 241–250. doi: 10.1159/000315151
Barreiro-Iglesias, A., Villar-Cheda, B., Abalo, X.-M., Anadón, R., and Rodicio, M. C. (2008). The early scaffold of axon tracts in the brain of a primitive vertebrate, the sea lamprey. Brain. Res. Bull. 75, 42–52. doi: 10.1016/j.brainresbull.2007.07.020
Bartels, H., and Potter, I. C. (2004). Cellular composition and ultrastructure of the gill epithelium of larval and adult lampreys: implications for osmoregulation in fresh and seawater. J. Exp. Biol. 207, 3447–3462. doi: 10.1242/jeb.01157
Barton, K. (2014). MuMIn: Multi-model Inference. R Package Version 1.10.15. Available online at: http://CRAN.R-project.org/package=MuMIn
Barton, R. A., and Capellini, I. (2011). Maternal investment, life histories, and the costs of brain growth in mammals. Proc. Natl. Acad. Sci. U.S.A. 108, 6169–6174. doi: 10.1073/pnas.1019140108
Barton, R. A., and Harvey, P. H. (2000). Mosaic evolution of brain structure in mammals. Nature 405, 1055–1058. doi: 10.1038/35016580
Barton, R. A., Purvis, A., and Harvey, P. H. (1995). Evolutionary radiation of visual and olfactory brain systems in primates, bats and insectivores. Philos. Trans. R. Soc. B 348, 381–392. doi: 10.1098/rstb.1995.0076
Bauchot, R., Diagne, M., and Ribet, J. M. (1979). Post-hatching growth and allometry of the teleost brain. J. Hirnforsch. 20, 29–34.
Bethea, D. M., Buckel, J. A., and Carlson, J. K. (2004). Foraging ecology of the early life stages of four sympatric shark species. Mar. Ecol. Prog. Ser. 268, 245–264. doi: 10.3354/meps268245
Bhatnagar, K. P., Frahm, H. D., and Stephan, H. (1986). The pineal organ of bats: a comparative morphological and volumetric investigation. J. Anat. 147, 143–161.
Binder, T. R., and McDonald, D. G. (2007). Is there a role for vision in the behaviour of sea lampreys (Petromyzon marinus) during their upstream spawning migration? Can. J. Fish. Aquat. Sci. 64, 1403–1412. doi: 10.1139/f07-102
Binder, T. R., McDonald, D. G., and Wilkie, M. P. (2013). Reduced dermal photosensitivity in juvenile sea lampreys (Petromyzon marinus) reflects life-history-dependent changes in habitat and behaviour. Can. J. Zool. 91, 635–639. doi: 10.1139/cjz-2013-0041
Bodznick, D., and Northcutt, R. G. (1981). Electroreception in lampreys - evidence that the earliest vertebrates were electroreceptive. Science 212, 465–467. doi: 10.1126/science.7209544
Bowmaker, J. K., and Wagner, H.-J. (2004). Pineal organs of deep-sea fish: photopigments and structure. J. Exp. Biol. 207, 2379–2387. doi: 10.1242/jeb.01033
Boyd, J. L., Skove, S. L., Rouanet, J. P., Pilaz, L.-J., Bepler, T., Gordân, R., et al. (2015). Human-chimpanzee differences in a FZD8 enhancer alter cell-cycle dynamics in the developing neocortex. Curr. Biol. 25, 772–779. doi: 10.1016/j.cub.2015.01.041
Brandstätter, R., and Kotrschal, K. (1990). Brain growth patterns in 4 European cyprinid fish species (Cyprinidae, Teleostei): roach (Rutilus rutilus), bream (Abramis brama), common carp (Cyprinus carpio) and sabre carp (Pelecus cultratus). Brain Behav. Evol. 35, 195–211. doi: 10.1159/000115867
Butler, A. B., and Hodos, W. (1996). Comparative Vertebrate Neuroanatomy: Evolution and Adaptation. New York, NY: Wiley-Liss.
Calabrese, E., Badea, A., Watson, C., and Johnson, G. A. (2013). A quantitative magnetic resonance histology atlas of postnatal rat brain development with regional estimates of growth and variability. Neuroimage 71, 196–206. doi: 10.1016/j.neuroimage.2013.01.017
Candal, E., Anadon, R., Bourrat, F., and Rodriguez-Moldes, I. (2005). Cell proliferation in the developing and adult hindbrain and midbrain of trout and medaka (teleosts): a segmental approach. Brain Res. Dev. Brain. Res. 160, 157–175. doi: 10.1016/j.devbrainres.2005.08.009
Chang, M.-M., Wu, F., Miao, D., and Zhang, J. (2014). Discovery of fossil lamprey larva from the Lower Cretaceous reveals its three-phased life cycle. Proc. Natl. Acad. Sci. U.S.A. 111, 15486–15490. doi: 10.1073/pnas.1415716111
Chang, S., Chung-Davidson, Y. W., Libants, S. V., Nanlohy, K. G., Kiupel, M., Brown, C. T., et al. (2013). The sea lamprey has a primordial accessory olfactory system. BMC Evol. Biol. 13:172. doi: 10.1186/1471-2148-13-172
Charvet, C. J., Striedter, G. F., and Finlay, B. L. (2011). Evo-devo and brain scaling: candidate developmental mechanisms for variation and constancy in vertebrate brain evolution. Brain Behav. Evol. 78, 248–257. doi: 10.1159/000329851
Clark, D. A., Mitra, P. P., and Wang, S. S. (2001). Scalable architecture in mammalian brains. Nature 411, 189–193. doi: 10.1038/35075564
Cobley, N. D. (1996). An observation of live prey capture by black-browed albatross Diomedea melanophrys. Mar. Ornithol. 24, 45–46.
Cole, W. C., and Youson, J. H. (1981). The effect of pinealectomy, continuous light, and continuous darkness on metamorphosis of anadromous sea lampreys, Petromyzon marinus L. J. Exp. Biol. 218, 397–404. doi: 10.1002/jez.1402180311
Collin, S. P., Hart, N. S., Shand, J., and Potter, I. C. (2003). Morphology and spectral absorption characteristics of retinal photoreceptors in the southern hemisphere lamprey (Geotria australis). Vis. Neurosci. 20, 119–130. doi: 10.1017/S0952523803202030
Collin, S. P., Potter, I. C., and Braekevelt, C. R. (1999). The ocular morphology of the southern hemisphere lamprey Geotria australis gray, with special reference to optical specialisations and the characterisation and phylogeny of photoreceptor types. Brain Behav. Evol. 54, 96–118. doi: 10.1159/000006616
Cornide-Petronio, M. E., Barreiro-Iglesias, A., Anadon, R., and Rodicio, M. C. (2011). Retinotopy of visual projections to the optic tectum and pretectum in larval sea lamprey. Exp. Eye Res. 92, 274–281. doi: 10.1016/j.exer.2011.01.011
Currie, S. N., and Carlsen, R. C. (1988). Cranial components of startle behavior in larval and adult lampreys. Neuroscience 24, 709–718. doi: 10.1016/0306-4522(88)90363-6
Davies, W. L., Cowing, J. A., Carvalho, L. S., Potter, I. C., Trezise, A. E., Hunt, D. M., et al. (2007). Functional characterization, tuning, and regulation of visual pigment gene expression in an anadromous lamprey. FASEB J. 21, 2713–2724. doi: 10.1096/fj.06-8057com
Dawson, H. A., Quintella, B. R., Almeida, P. R., Treble, A. J., and Jolley, J. C. (2015). “The ecology of larval and metamorphosing lampreys,” in Lampreys: Biology, Conservation and Control, ed M. F. Docker (New York, NY: Springer), 75–137.
Deacon, T. W. (1990). Problems of ontogeny and phylogeny in brain-size evolution. Int. J. Primatol. 11, 237–282. doi: 10.1007/BF02192870
De Arriba, M. D., and Pombal, M. A. (2007). Afferent connections of the optic tectum in lampreys: an experimental study. Brain Behav. Evol. 69, 37–68. doi: 10.1159/000095272
Deliagina, T. G., Ullen, F., Gonzalez, M. J., Efson, H. H., Orlovsky, G. N., and Grillner, S. (1995). Initiation of locomotion by lateral-line photoreceptors in lamprey - behavioral and neurophysiological studies. J. Exp. Biol. 198, 2581–2591.
De Miguel, E., and Anadon, R. (1987). The development of retina and the optic tectum of Petromyzon marinus, L. A light microscopic study. J. Hirnforsch. 28, 445–456.
De Miguel, E., Rodicio, C., and Anadon, R. (1990). Organization of the visual system in larval lampreys: an HRP study. J. Comp. Neurol. 302, 529–542. doi: 10.1002/cne.903020309
Derjean, D., Moussaddy, A., Atallah, E., St-Pierre, M., Auclair, F., Chang, S., et al. (2010). A novel neural substrate for the transformation of olfactory inputs into motor output. PLoS Biol. 8:e1000567. doi: 10.1371/journal.pbio.1000567
De Winter, W., and Oxnard, C. E. (2001). Evolutionary radiations and convergences in the structural organization of mammalian brains. Nature 409, 710–714. doi: 10.1038/35055547
Ebbesson, S. E. (1980). The parcellation theory and its relation to interspecific variability in brain organization, evolutionary and ontogenetic development, and neuronal plasticity. Cell Tissue Res. 213, 179–212. doi: 10.1007/BF00234781
Ebinger, P., Wächtler, K., and Stähler, S. (1983). Allometrical studies in the brain of cyclostomes. J. Hirnforsch. 24, 545–550.
Eddy, J. M. P. (1971). “The pineal complex,” in The Biology of Lampreys, eds M. W. Hardisty and I. C. Potter (London: Academic Press), 91–103.
Eddy, J. M. P., and Strahan, R. (1968). The role of the pineal complex in the pigmentary effector system of the lampreys, Mordacia mordax (Richardson) and Geotria australis (Gray). Gen. Comp. Endocrinol. 11, 528–534. doi: 10.1016/0016-6480(68)90067-1
Eddy, J. M. P., and Strahan, R. (1970). The structure of the epiphyseal complex of Mordacia mordax and Geotria australis (Petromyzonidae). Acta Zool. 51, 67–84. doi: 10.1111/j.1463-6395.1970.tb00418.x
Ekström, P., and Meissl, H. (1997). The pineal organ of teleost fishes. Rev. Fish. Biol. Fish. 7, 199–284. doi: 10.1023/A:1018483627058
Ekström, P., and Meissl, H. (2003). Evolution of photosensory pineal organs in new light: the fate of neuroendocrine photoreceptors. Philos. Trans. R. Soc. Lond. B 358, 1679–1700. doi: 10.1098/rstb.2003.1303
Enequist, P. (1937). Das bachneunauge als ökologische modifikation des flussneunauges. Über die fluss – und bachneunaugen schwedens; vorläufige mitteilung. Ark. Zool. 29, 1–22.
Ericsson, J., Stephenson-Jones, M., Kardamakis, A., Robertson, B., Silberberg, G., and Grillner, S. (2013). Evolutionarily conserved differences in pallial and thalamic short-term synaptic plasticity in striatum. J. Physiol. 591, 859–874. doi: 10.1113/jphysiol.2012.236869
Finlay, B. L., and Darlington, R. B. (1995). Linked regularities in the development and evolution of mammalian brains. Science 268, 1578–1584. doi: 10.1126/science.7777856
Finlay, B. L., Darlington, R. B., and Nicastro, N. (2001). Developmental structure in brain evolution. Behav. Brain Sciences 24, 263–278. doi: 10.1017/S0140525X01003958
Freamat, M., and Sower, S. A. (2013). Integrative neuro-endocrine pathways in the control of reproduction in lamprey: a brief review. Front. Endocrinol. 4:151. doi: 10.3389/fendo.2013.00151
Fritzsch, B., and Northcutt, R. G. (1993a). Cranial and spinal nerve organization in amphioxus and lampreys: evidence for an ancestral craniate pattern. Acta Anat. 148, 96–109. doi: 10.1159/000147529
Fritzsch, B., and Northcutt, R. G. (1993b). Origin and migration of trochlear, oculomotor and abducent motor neurons in Petromyzon marinus L. Brain Res. Dev. Brain Res. 74, 122–126. doi: 10.1016/0165-3806(93)90091-N
Fu, Y., Rusznak, Z., Herculano-Houzel, S., Watson, C., and Paxinos, G. (2013). Cellular composition characterizing postnatal development and maturation of the mouse brain and spinal cord. Brain Struct. Funct. 218, 1337–1354. doi: 10.1007/s00429-012-0462-x
Gai, Z. K., Donoghue, P. C. J., Zhu, M., Janvier, P., and Stampanoni, M. (2011). Fossil jawless fish from China foreshadows early jawed vertebrate anatomy. Nature 476, 324–327. doi: 10.1038/nature10276
García-Fernández, J. M., and Foster, R. G. (1994). Immunocytochemical identification of photoreceptor proteins in hypothalamic cerebrospinal fluid-contacting neurons of the larval lamprey (Petromyzon marinus). Cell Tissue Res. 275, 319–326. doi: 10.1007/BF00319430
Gelman, S., Ayali, A., Kiemel, T., Sanovich, E., and Cohen, A. H. (2008). Metamorphosis-related changes in the lateral line system of lampreys, Petromyzon marinus. J. Comp. Physiol. A. 194, 945–956. doi: 10.1007/s00359-008-0367-6
Gelman, S., Ayali, A., Tytell, E. D., and Cohen, A. H. (2007). Larval lampreys possess a functional lateral line system. J. Comp. Physiol. A 193, 271–277. doi: 10.1007/s00359-006-0183-9
Gilland, E., and Baker, R. (2005). Evolutionary patterns of cranial nerve efferent nuclei in vertebrates. Brain Behav. Evol. 66, 234–254. doi: 10.1159/000088128
Gille, U., and Salomon, F. V. (2000). Brain growth in mallards, Pekin and Muscovy ducks (Anatidae). J. Zool. 252, 399–404. doi: 10.1111/j.1469-7998.2000.tb00635.x
Gonda, A., Herczeg, G., and Merila, J. (2011). Population variation in brain size of nine-spined sticklebacks (Pungitius pungitius)–local adaptation or environmentally induced variation? BMC Evol. Biol. 11:75. doi: 10.1186/1471-2148-11-75
Gonda, A., Herczeg, G., and Merilä, J. (2013). Evolutionary ecology of intraspecific brain size variation: a review. Ecol. Evol. 3, 2751–2764. doi: 10.1002/ece3.627
Gonzalez-Voyer, A., and Kolm, N. (2010). Sex, ecology and the brain: evolutionary correlates of brain structure volumes in Tanganyikan cichlids. PLoS ONE 5:e14355. doi: 10.1371/journal.pone.0014355
Gonzalez-Voyer, A., Winberg, S., and Kolm, N. (2009). Brain structure evolution in a basal vertebrate clade: evidence from phylogenetic comparative analysis of cichlid fishes. BMC Evol. Biol. 9:238. doi: 10.1186/1471-2148-9-238
Gould, S. J. (1975). Allometry in primates, with emphasis on scaling and the evolution of the brain. Contrib. Primatol. 5, 244–292.
Grillner, S., and Robertson, B. (2015). The basal ganglia downstream control of brainstem motor centres—an evolutionarily conserved strategy. Curr. Opin. Neurobiol. 33, 47–52. doi: 10.1016/j.conb.2015.01.019
Gritzenko, O. F. (1968). On the question of an ecological paralIelism between lampreys and salmon (in Russian). Izv. Tikhookean. Nauchno-lssled. Inst. Rybn. Khoz. Okeanogr. 65, 157–169.
Gruberg, E., Dudkin, E., Wang, Y., Marin, G., Salas, C., Sentis, E., et al. (2006). Influencing and interpreting visual input: the role of a visual feedback system. J. Neurosci. 26, 10368–10371. doi: 10.1523/JNEUROSCI.3288-06.2006
Guerin, A., D'aubenton-Carafa, Y., Marrakchi, E., Da Silva, C., Wincker, P., Mazan, S., et al. (2009). Neurodevelopment genes in lampreys reveal trends for forebrain evolution in craniates. PLoS ONE 4:e5374. doi: 10.1371/journal.pone.0005374
Haldar, C., and Bishnupuri, K. S. (2001). Comparative view of pineal gland morphology of nocturnal and diurnal birds of tropical origin. Microsc. Res. Tech. 53, 25–32. doi: 10.1002/jemt.1065
Healy, S. D., and Rowe, C. (2007). A critique of comparative studies of brain size. Proc. R. Soc. Lond. B 274, 453–464. doi: 10.1098/rspb.2006.3748
Heier, P. (1948). Fundamental principles in the structure of the brain of Petromyzon fluviatilis. Acta Anat. 5, 1–213.
Heimberg, A. M., Sempere, L. F., Moy, V. N., Donoghue, P. C. J., and Peterson, K. J. (2008). MicroRNAs and the advent of vertebrate morphological complexity. Proc. Natl. Acad. Sci. U.S.A. 105, 2946–2950. doi: 10.1073/pnas.0712259105
Herculano-Houzel, S. (2012). The remarkable, yet not extraordinary, human brain as a scaled-up primate brain and its associated cost. Proc. Natl. Acad. Sci. U.S.A. 109, 10661–10668. doi: 10.1073/pnas.1201895109
Heupel, M. R., and Simpfendorfer, C. A. (2011). Estuarine nursery areas provide a low-mortality environment for young bull sharks Carcharhinus leucas. Mar. Ecol. Prog. Ser. 433, 237–244. doi: 10.3354/meps09191
Hilliard, R. W., Bird, D. J., and Potter, I. C. (1983). Metamorphic changes in the intestine of three species of lampreys. J. Morphol. 176, 181–196. doi: 10.1002/jmor.1051760207
Hilliard, R. W., Potter, I. C., and Macey, D. J. (1985). The dentition and feeding mechanisms in adults of the Southern Hemisphere lamprey Geotria australis Gray. Acta Zool. 66, 159–170. doi: 10.1111/j.1463-6395.1985.tb00834.x
Homma, S. (1978). Organization of the trigeminal motor nucleus before and after metamorphosis in lampreys. Brain Res. 140, 33–42. doi: 10.1016/0006-8993(78)90236-6
Huber, R., van Staaden, M. J., Kaufman, L. S., and Liem, K. F. (1997). Microhabitat use, trophic patterns, and the evolution of brain structure in African cichlids. Brain Behav. Evol. 50, 167–182. doi: 10.1159/000113330
Hume, J. B., Adams, C. E., Mable, B., and Bean, C. W. (2013). Sneak male mating tactics between lampreys (Petromyzontiformes) exhibiting alternative life-history strategies. J. Fish Biol. 82, 1093–1100. doi: 10.1111/jfb.12047
Iribarne, L., and Castelló, M. E. (2014). Postnatal brain development of the pulse type, weakly electric gymnotid fish Gymnotus omarorum. J. Physiol. Paris 108, 47–60. doi: 10.1016/j.jphysparis.2014.05.001
Iwahori, N., Kawawaki, T., and Baba, J. (1999). Neuronal organization of the optic tectum in the river lamprey, Lampetra japonica: a Golgi study. J. Hirnforsch. 39, 409–424.
Iwaniuk, A. N., Gutierrez-Ibanez, C., Pakan, J. M., and Wylie, D. R. (2010). Allometric scaling of the tectofugal pathway in birds. Brain Behav. Evol. 75, 122–137. doi: 10.1159/000311729
Iwaniuk, A. N., and Hurd, P. L. (2005). The evolution of cerebrotypes in birds. Brain Behav. Evol. 65, 215–230. doi: 10.1159/000084313
Jacobs, L. F. (2012). From chemotaxis to the cognitive map: the function of olfaction. Proc. Natl. Acad. Sci. U.S.A. 109, 10693–10700. doi: 10.1073/pnas.1201880109
Janvier, P. (2008). Early jawless vertebrates and cyclostome origins. Zoolog. Sci. 25, 1045–1056. doi: 10.2108/zsj.25.1045
Jellyman, D. J., Glova, G. J., and Sykes, J. R. E. (2002). Movements and habitats of adult lamprey (Geotria australis) in two New Zealand waterways. N. Z. J. Mar. Freshwatwer. Res. 36, 53–65. doi: 10.1080/00288330.2002.9517070
Jerison, H. J. (1977). The theory of encephalization. Ann. N. Y. Acad. Sci. 299, 146–160. doi: 10.1111/j.1749-6632.1977.tb41903.x
Johnson, N. S., Buchinger, T. J., and Li, W. (2015). “Reproductive ecology of lampreys,” in Lampreys: Biology, Conservation and Control, ed M. F. Docker (New York, NY: Springer), 265–303.
Johnson, N. S., Siefkes, M. J., and Li, W. (2005). Capture of ovulating female sea lampreys in traps baited with spermiating male sea lampreys. North Am. J. Fish. Manage. 25, 67–72. doi: 10.1577/M03-226.1
Johnson, N. S., Yun, S. S., Thompson, H. T., Brant, C. O., and Li, W. (2009). A synthesized pheromone induces upstream movement in female sea lamprey and summons them into traps. Proc. Natl. Acad. Sci. U.S.A. 106, 1021–1026. doi: 10.1073/pnas.0808530106
Johnston, J. B. (1902). The brain of Petromyzon. J. Comp. Neurol. 12, 1–86. doi: 10.1002/cne.910120102
Johnston, J. B. (1912). The telencephalon in cyclostomes. J. Comp. Neurol. 22, 341–404. doi: 10.1002/cne.900220401
Jones, M. R., Grillner, S., and Robertson, B. (2009). Selective projection patterns from subtypes of retinal ganglion cells to tectum and pretectum: distribution and relation to behavior. J. Comp. Neurol. 517, 257–275. doi: 10.1002/cne.22154
Jørgensen, J. (2005). “Morphology of electroreceptive sensory organs,” in Electroreception, eds T. Bullock, C. Hopkins, A. Popper and R. Fay (New York, NY: Springer), 47–67.
Karamian, A. I., Vesselkin, N. P., Belekhova, M. G., and Zagorulko, T. M. (1966). Electrophysiological characteristics of tectal and thalamo-cortical divisions of the visual system in lower vertebrates. J. Comp. Neurol. 127, 559–576. doi: 10.1002/cne.901270408
Karamian, A. I., Vesselkin, N. P., and Agayan, A. L. (1984). “Electrophysiological and behavioral studies of the optic tectum in cyclostomes,” in Comparative Neurology of the Optic Tectum, ed H. Vanegas (New York, NY: Plenum Press), 15–30.
Kardamakis, A. A., Saitoh, K., and Grillner, S. (2015). Tectal microcircuit generating visual selection commands on gaze-controlling neurons. Proc. Natl. Acad. Sci. U.S.A. 112, E1956–E1965. doi: 10.1073/pnas.1504866112
Kaslin, J., Ganz, J., and Brand, M. (2008). Proliferation, neurogenesis and regeneration in the non-mammalian vertebrate brain. Philos. Trans. R. Soc. Lond. B Biol. Sci. 363, 101–122. doi: 10.1098/rstb.2006.2015
Kempermann, G. (2012). New neurons for ‘survival of the fittest.’ Nat. Rev. Neurosci. 13, 727–736. doi: 10.1038/nrn3319
Kennedy, M. C., and Rubinson, K. (1977). Retinal projections in larval, transforming and adult sea lamprey, Petromyzon marinus. J. Comp. Neurol. 171, 465–479. doi: 10.1002/cne.901710404
Khonsari, R. H., Li, B., Vernier, P., Northcutt, R. G., and Janvier, P. (2009). Agnathan brain anatomy and craniate phylogeny. Acta Zool. 90, 52–68. doi: 10.1111/j.1463-6395.2008.00388.x
Kingsford, M. J., Leis, J. M., Shanks, A., Lindeman, K. C., Morgan, S. G., and Pineda, J. (2002). Sensory environments, larval abilities and local self-recruitment. Bull. Mar. Sci. 70, 309–340.
Kolm, N., Gonzalez-Voyer, A., Brelin, D., and Winberg, S. (2009). Evidence for small scale variation in the vertebrate brain: mating strategy and sex affect brain size and structure in wild brown trout (Salmo trutta). J. Evol. Biol. 22, 2524–2531. doi: 10.1111/j.1420-9101.2009.01875.x
Kosareva, A. A. (1980). Retinal projections in lamprey (Lampetra fluviatilis). J. Hirnforsch. 21, 243–256.
Kotrschal, A., Heckel, G., Bonfils, D., and Taborsky, B. (2012). Life-stage specific environments in a cichlid fish: implications for inducible maternal effects. Evol. Ecol. 26, 123–137. doi: 10.1007/s10682-011-9495-5
Kotrschal, K., Adam, H., Brandstätter, R., Junger, H., Zaunreiter, M., and Goldschmid, A. (1990). Larval size constraints determine directional ontogenetic shifts in the visual system of teleosts. J. Zoolog. Syst. Evol. Res. 28, 166–182. doi: 10.1111/j.1439-0469.1990.tb00374.x
Kotrschal, K., and Palzenberger, M. (1992). Neuroecology of cyprinids: comparative, quantitative histology reveals diverse brain patterns. Environ. Biol. Fish. 33, 135–152. doi: 10.1007/BF00002560
Kruska, D. C. T. (1988). The brain of the basking shark (Cetorhinus maximus). Brain Behav. Evol. 32, 353–363. doi: 10.1159/000116562
Kruska, D. C. T. (2005). On the evolutionary significance of encephalization in some eutherian mammals: effects of adaptive radiation, domestication, and feralization. Brain Behav. Evol. 65, 73–108. doi: 10.1159/000082979
Kumar, S., and Hedges, S. B. (1998). A molecular timescale for vertebrate evolution. Nature 392, 917–920. doi: 10.1038/31927
Kuratani, S., Ueki, T., Aizawa, S., and Hirano, S. (1997). Peripheral development of cranial nerves in a cyclostome, Lampetra japonica: morphological distribution of nerve branches and the vertebrate body plan. J. Comp. Neurol. 384, 483–500.
Lecchini, D., Lecellier, G., Lanyon, R. G., Holles, S., Poucet, B., and Duran, E. (2014). Variation in brain organization of coral reef fish larvae according to life history traits. Brain Behav. Evol. 83, 17–30. doi: 10.1159/000356787
Lefebvre, L., Reader, S. M., and Sol, D. (2004). Brains, innovations and evolution in birds and primates. Brain Behav. Evol. 63, 233–246. doi: 10.1159/000076784
Lethbridge, R. C., and Potter, I. C. (1981). The development of teeth and associated feeding structures during the metamorphosis of the lamprey, Geotria australis. Acta Zool. 62, 201–214. doi: 10.1111/j.1463-6395.1981.tb00629.x
Leyhausen, C., Kirschbaum, F., Szabo, T., and Erdelen, M. (1987). Differential growth in the brain of the weakly electric fish, Apteronotus leptorhynchus (Gymnotiformes), during ontogeny. Brain Behav. Evol. 30, 230–248. doi: 10.1159/000118648
Lisney, T. J., Bennett, M. B., and Collin, S. P. (2007). Volumetric analysis of the sensory brain areas indicates ontogenetic shifts in the relative importance of sensory systems in elasmobranchs. Raff. Bull. Zool. 14, 7–15. doi: 10.1111/j.0022-1112.2006.00940.x
Lisney, T. J., and Collin, S. P. (2006). Brain morphology in large pelagic fishes: a comparison between sharks and teleosts. J. Fish Biol. 68, 532–554. doi: 10.1111/j.0022-1112.2006.00940.x
Manzon, R. G., Youson, J. H., and Holmes, J. A. (2015). “Lamprey metamorphosis,” in Lampreys: Biology, Conservation and Control, ed M. F. Docker (New York, NY: Springer), 139–214.
McMenamin, S. K., and Parichy, D. M. (2013). Metamorphosis in teleosts. Curr. Top. Dev. Biol. 103, 127–165. doi: 10.1016/b978-0-12-385979-2.00005-8
Melendez-Ferro, M., Perez-Costas, E., Villar-Cheda, B., Rodriguez-Munoz, R., Anadon, R., and Rodicio, M. C. (2003). Ontogeny of gamma-aminobutyric acid-immunoreactive neurons in the rhombencephalon and spinal cord of the sea lamprey. J. Comp. Neurol. 464, 17–35. doi: 10.1002/cne.10773
Melendez-Ferro, M., Villar-Cheda, B., Abalo, X. M., Perez-Costas, E., Rodriguez-Munoz, R., Degrip, W. J., et al. (2002). Early development of the retina and pineal complex in the sea lamprey: comparative immunocytochemical study. J. Comp. Neurol. 442, 250–265. doi: 10.1002/cne.10090
Meyer-Rochow, V. B., and Stewart, D. (1996). Review of larval and postlarval eye ultrastructure in the lamprey (Cyclostomata) with special emphasis on Geotria australis (Gray). Microsc. Res. Tech. 35, 431–444.
Montgomery, J. C., Bodznick, D., and Yopak, K. E. (2012). The cerebellum and cerebellum-like structures of cartilaginous fishes. Brain Behav. Evol. 80, 152–165. doi: 10.1159/000339868
Montgomery, J. C., and Sutherland, K. B. W. (1997). Sensory development of the antarctic silverfish Pleuragramma antarcticum: a test for the ontogenetic shift hypothesis. Polar Biol. 18, 112–115. doi: 10.1007/s003000050165
Moore, J. W., and Mallat, J. M. (1980). Feeding of larval lamprey. Can. J. Fish. Aquat. Sci. 37, 1658–1664. doi: 10.1139/f80-213
Neira, F. J. (1984). Biomorfología de las lampreas parasitarias chilenas Geotria australis (Gray, 1851) y Mordacia lapicida (Gray, 1851)(Petromyzontiformes). Gayana Zool. 48, 3–40.
Ngwenya, A., Patzke, N., Spocter, M. A., Kruger, J.-L., Dell, L.-A., Chawana, R., et al. (2013). The continuously growing central nervous system of the Nile crocodile (Crocodylus niloticus). Anat. Rec. 296, 1489–1500. doi: 10.1002/ar.22752
Nieuwenhuys, R. (1977). The brain of the lamprey in a comparative perspective. Ann. N. Y. Acad. Sci. 299, 97–145. doi: 10.1111/j.1749-6632.1977.tb41902.x
Nieuwenhuys, R., and Nicholson, C. (1998). “Lampreys, Petromyzontoidae,” in The Central Nervous System of Vertebrates, eds R. Nieuwenhuys, H. J. T. Donkelaar, and C. Nicholson (Berlin: Springer), 397–495. doi: 10.1007/978-3-642-18262-4_10
Northcutt, R. G. (2002). Understanding vertebrate brain evolution. Integr. Comp. Biol. 42, 743–756. doi: 10.1093/icb/42.4.743
Northcutt, R. G., and Puzdrowski, R. L. (1988). Projections of the olfactory bulb and nervus terminalis in the silver lamprey. Brain Behav. Evol. 32, 96–107. doi: 10.1159/000116537
Northcutt, R. G., and Wicht, H. (1997). Afferent and efferent connections of the lateral and medial pallia of the silver lamprey. Brain Behav. Evol. 49, 1–19. doi: 10.1159/000112978
Ocaña, F. M., Suryanarayana, S. M., Saitoh, K., Kardamakis, A. A., Capantini, L., Robertson, B., et al. (2015). The lamprey pallium provides a blueprint of the mammalian motor projections from cortex. Curr. Biol. 25, 413–423. doi: 10.1016/j.cub.2014.12.013
Ota, K. G., and Kuratani, S. (2007). Cyclostome embryology and early evolutionary history of vertebrates. Integr. Comp. Biol. 47, 329–337. doi: 10.1093/icb/icm022
Paton, K. R., Cake, M. H., and Potter, I. C. (2011). Metabolic responses to exhaustive exercise change markedly during the protracted non-trophic spawning migration of the lamprey Geotria australis. J. Comp. Physiol. B 181, 751–763. doi: 10.1007/s00360-011-0570-6
Pena, E. A., and Slate, E. H. (2014). gvlma: Global Validation of Linear Models Assumptions. R Package Version 1.0.0.2. Available online at: http://CRAN.R-project.org/package=gvlma
Piavis, G. W. (1971). “Embriology,” in The Biology of Lampreys, eds M. W. Hardisty and I. C. Potter (London: Academic Press), 361–400.
Pirlot, P., and Bernier, R. (1991). Brain growth and differentiation in two fetal bats: qualitative and quantitative aspects. Am. J. Anat. 190, 167–181. doi: 10.1002/aja.1001900206
Platel, R., and Delfini, C. (1981). L'Encéphalisation chez la myxine (Myxine glutinosa L.). Analyse quantifiée des principales subdivisions encéphaliques. Cah. Biol. Mar. 22, 407–430.
Platel, R., and Delfini, C. (1986). L'Encéphalisation chez la lamproie marine, Petromyzon marinus (L.). Analyse quantifiée des principales subdivisions encéphaliques. J. Hirnforsch. 3, 279–293.
Platel, R., and Vesselkin, N. P. (1988). Analysis of brain-body allometries in the lamprey Lampetra fluviatilis L. J. Evol. Biochem. Phys. 24, 138–145.
Platel, R., and Vesselkin, N. P. (1989). Comparative study of the encephalization in 3 species of Petromyzonidae (agnatha) - Petromyzon marinus, Lampetra fluviatilis and Lampetra planeri. J. Hirnforsch. 30, 23–32.
Polenova, O. A., and Vesselkin, N. P. (1993). Olfactory and nonolfactory projections in the river lamprey (Lampetra fluviatilis) telencephalon. J. Hirnforsch. 34, 261–279.
Pollen, A. A., Dobberfuhl, A. P., Scace, J., Igulu, M. M., Renn, S. C. P., Shumway, C. A., et al. (2007). Environmental complexity and social organization sculpt the brain in Lake Tanganyikan cichlid fish. Brain. Behav. Evol. 70, 21–39. doi: 10.1159/000101067
Pombal, M. A., Alvarez-Otero, R., Perez-Fernandez, J., Solveira, C., and Megias, M. (2011). Development and organization of the lamprey telencephalon with special reference to the GABAergic system. Front. Neuroanat. 5:20. doi: 10.3389/fnana.2011.00020
Pombal, M. A., Marin, O., and Gonzalez, A. (2001). Distribution of choline acetyltransferase-immunoreactive structures in the lamprey brain. J. Comp. Neurol. 431, 105–126. doi: 10.1002/1096-9861(20010226)431:13.0.CO;2-P
Pombal, M. A., Megias, M., Bardet, S. M., and Puelles, L. (2009). New and old thoughts on the segmental organization of the forebrain in lampreys. Brain Behav. Evol. 74, 7–19. doi: 10.1159/000229009
Pombal, M. A., and Puelles, L. (1999). Prosomeric map of the lamprey forebrain based on calretinin immunocytochemistry, Nissl stain, and ancillary markers. J. Comp. Neurol. 414, 391–422.
Pombal, M. A., Rodicio, M. C., and Anadon, R. (1994). Development and organization of the ocular motor nuclei in the larval sea lamprey, Petromyzon marinus L.: an HRP study. J. Comp. Neurol. 341, 393–406. doi: 10.1002/cne.903410309
Pombal, M. A., Yanez, J., Marin, O., Gonzalez, A., and Anadon, R. (1999). Cholinergic and GABAergic neuronal elements in the pineal organ of lampreys, and tract-tracing observations of differential connections of pinealofugal neurons. Cell Tissue Res. 295, 215–223. doi: 10.1007/s004410051227
Potter, I. C. (1980). Ecology of larval and metamorphosing lampreys. Can. J. Fish. Aquat. Sci. 37, 1641–1657. doi: 10.1139/f80-212
Potter, I. C., Gill, H. S., Renaud, C. B., and Haoucher, D. (2015). “The taxonomy, phylogeny, and distribution of lampreys,” in Lampreys: Biology, Conservation and Control, ed M. F. Docker (New York, NY: Springer), 35–73.
Potter, I. C., and Hilliard, R. W. (1986). Growth and the average duration of larval life in the southern hemisphere lamprey, Geotria australis Gray. Experientia 42, 1170–1173. doi: 10.1007/BF01941297
Potter, I. C., Hilliard, R. W., and Bird, D. J. (1980). Metamorphosis in the southern hemisphere lamprey, Geotria australis. J. Zool. 190, 405–430. doi: 10.1111/j.1469-7998.1980.tb01435.x
Potter, I. C., Hilliard, R. W., Bird, D. J., and Macey, D. J. (1983). Quantitative data on morphology and organ weights during the protracted spawning−run period of the Southern Hemisphere lamprey Geotria australis. J. Zool. 200, 1–20. doi: 10.1111/j.1469-7998.1983.tb06106.x
Potter, I. C., Prince, P. A., and Croxall, J. P. (1979). Data on the adult marine and migratory phases in the life cycle of the southern hemisphere lamprey, Geotria australis Gray. Env. Biol. Fishes 4, 65–69. doi: 10.1007/BF00005929
Puzdrowski, R. L., and Northcutt, R. G. (1989). Central projections of the pineal complex in the silver lamprey Ichthyomyzon unicuspis. Cell Tissue Res. 255, 269–274. doi: 10.1007/BF00224108
Ralph, C. L. (1975). The pineal gland and geographical distribution of animals. Int. J. Biometeorol. 19, 289–303. doi: 10.1007/BF01451040
Rasband, W. S. (1997). ImageJ. Bethesda, MD: U. S. National Institutes of Health. 1997–2014. Available online at: http://imagej.nih.gov/ij/
R Core Team. (2013). R: A Language and Environment for Statistical Computing. Vienna: R Foundation for Statistical Computing.
Reep, R. L., Finlay, B. L., and Darlington, R. B. (2007). The limbic system in mammalian brain evolution. Brain Behav. Evol. 70, 57–70. doi: 10.1159/000101491
Reis-Santos, P., McCormick, S. D., and Wilson, J. M. (2008). Ionoregulatory changes during metamorphosis and salinity exposure of juvenile sea lamprey (Petromyzon marinus L.). J. Exp. Biol. 211, 978–988. doi: 10.1242/jeb.014423
Ren, X., Chang, S., Laframboise, A., Green, W., Dubuc, R., and Zielinski, B. (2009). Projections from the accessory olfactory organ into the medial region of the olfactory bulb in the sea lamprey (Petromyzon marinus): a novel vertebrate sensory structure? J. Comp. Neurol. 516, 105–116. doi: 10.1002/cne.22100
Renaud, C. B. (2011). Lampreys of the World - An Annotated and Illustrated Catalogue of Lamprey Species Known to Date. Rome: Food and Agriculture Organization of the United Nations (FAO).
Renaud, C. B., Gill, H. S., and Potter, I. C. (2009). Relationships between the diets and characteristics of the dentition, buccal glands and velar tentacles of the adults of the parasitic species of lamprey. J. Zool. 278, 231–242. doi: 10.1111/j.1469-7998.2009.00571.x
Richardson, M. K., Admiraal, J., and Wright, G. M. (2010). Developmental anatomy of lampreys. Biol. Rev. 85, 1–33. doi: 10.1111/j.1469-185X.2009.00092.x
Robertson, B., Saitoh, K., Menard, A., and Grillner, S. (2006). Afferents of the lamprey optic tectum with special reference to the GABA input: combined tracing and immunohistochemical study. J. Comp. Neurol. 499, 106–119. doi: 10.1002/cne.21078
Ronan, M. (1988). Anatomical and physiological evidence for electroreception in larval lampreys. Brain Res. 448, 173–177. doi: 10.1016/0006-8993(88)91115-8
Ronan, M., and Bodznick, D. (1991). Behavioral and neurophysiological demonstration of a lateralis skin photosensitivity in larval sea lampreys. J. Exp. Biol. 161, 97–117.
Ronan, M., and Northcutt, R. G. (1990). Projections ascending from the spinal cord to the brain in petromyzontid and myxinoid agnathans. J. Comp. Neurol. 291, 491–508. doi: 10.1002/cne.902910402
Rovainen, C. M. (1996). Feeding and breathing in lampreys. Brain Behav. Evol. 48, 297–305. doi: 10.1159/000113208
Rubinson, K. (1990). The developing visual system and metamorphosis in the lamprey. J. Neurobiol. 21, 1123–1135. doi: 10.1002/neu.480210715
Saitoh, K., Menard, A., and Grillner, S. (2007). Tectal control of locomotion, steering, and eye movements in lamprey. J. Neurophysiol. 97, 3093–3108. doi: 10.1152/jn.00639.2006
Sala, R., Santamaría, C. A., and Crespo, S. (2005). Growth of organ systems of Dentex dentex (L) and Psetta maxima (L) during larval development. J. Fish Biol. 66, 315–326. doi: 10.1111/j.0022-1112.2005.00580.x
Scott, W. B. (1887). Notes on the development of Petromyzon. J. Morphol. 1, 253–310. doi: 10.1002/jmor.1050010203
Siefkes, M. J., Scott, A. P., Zielinski, B., Yun, S. S., and Li, W. (2003). Male sea lampreys, Petromyzon marinus L., excrete a sex pheromone from gill epithelia. Biol. Reprod. 69, 125–132. doi: 10.1095/biolreprod.102.014472
Smith, J. J., Kuraku, S., Holt, C., Sauka-Spengler, T., Jiang, N., Campbell, M. S., et al. (2013). Sequencing of the sea lamprey (Petromyzon marinus) genome provides insights into vertebrate evolution. Nat. Genet. 45, 415–421. doi: 10.1038/ng.2568
Sorensen, P. W., Fine, J. M., Dvornikovs, V., Jeffrey, C. S., Shao, F., Wang, J., et al. (2005). Mixture of new sulfated steroids functions as a migratory pheromone in the sea lamprey. Nat. Chem. Biol. 1, 324–328. doi: 10.1038/nchembio739
Sousa, R., Araújo, M. J., and Antunes, C. (2012). Habitat modifications by sea lampreys (Petromyzon marinus) during the spawning season: effects on sediments. J. Appl. Ichthyol. 28, 766–771. doi: 10.1111/j.1439-0426.2012.02025.x
Stephan, H. (1960). Methodische studien über den quantitativen vergleich architektonischer struktureinheiten des gehirns. Z. Wiss. Zool. 64, 143–172.
Suárez, R., Garcia-Gonzalez, D., and De Castro, F. (2012). Mutual influences between the main olfactory and vomeronasal systems in development and evolution. Front. Neuroanat. 6:50. doi: 10.3389/fnana.2012.00050
Suárez, R., Gobius, I., and Richards, L. J. (2014). Evolution and development of interhemispheric connections in the vertebrate forebrain. Front. Hum. Neurosci. 8:497. doi: 10.3389/fnhum.2014.00497
Tamotsu, S., and Morita, Y. (1986). Photoreception in pineal organs of larval and adult lampreys, Lampetra japonica. J. Comp. Physiol. 159, 1–5. doi: 10.1007/BF00612489
Tomoda, H., and Uematsu, K. (1996). Morphogenesis of the brain in larval and juvenile Japanese eels, Anguilla japonica. Brain Behav. Evol. 47, 33–41. doi: 10.1159/000113227
Ullen, F., Deliagina, T. G., Orlovsky, G. N., and Grillner, S. (1995). Spatial orientation in the lamprey. 2. Visual influence on orientation during locomotion and in the attached state. J. Exp. Biol. 198, 675–681.
Ullen, F., Deliagina, T. G., Orlovsky, G. N., and Grillner, S. (1997). Visual pathways for postural control and negative phototaxis in lamprey. J. Neurophysiol. 78, 960–976.
Vandenbossche, J., Seelye, J. G., and Zielinski, B. S. (1995). The morphology of the olfactory epithelium in larval, juvenile and upstream migrant stages of the sea lamprey, Petromyzon marinus. Brain Behav. Evol. 45, 19–24. doi: 10.1159/000113382
Vernadakis, A. J., Bemis, W. E., and Bittman, E. L. (1998). Localization and partial characterization of melatonin receptors in amphioxus, hagfish, lamprey, and skate. Gen. Comp. Endocr. 110, 67–78. doi: 10.1006/gcen.1997.7042
Vidal Pizarro, I., Swain, G. P., and Selzer, M. E. (2004). Cell proliferation in the lamprey central nervous system. J. Comp. Neurol. 469, 298–310. doi: 10.1002/cne.11013
Vigh, B., Manzano, M. J., Zadori, A., Frank, C. L., Lukats, A., Rohlich, P., et al. (2002). Nonvisual photoreceptors of the deep brain, pineal organs and retina. Histol. Histopathol. 17, 555–590.
Villar-Cheda, B., Abalo, X. M., Villar-Cervino, V., Barreiro-Iglesias, A., Anadon, R., and Rodicio, M. C. (2008). Late proliferation and photoreceptor differentiation in the transforming lamprey retina. Brain Res. 1201, 60–67. doi: 10.1016/j.brainres.2008.01.077
Villar-Cheda, B., Perez-Costas, E., Melendez-Ferro, M., Abalo, X. M., Rodriguez-Munoz, R., Anadon, R., et al. (2006). Cell proliferation in the forebrain and midbrain of the sea lamprey. J. Comp. Neurol. 494, 986–1006. doi: 10.1002/cne.20851
Vrieze, L. A., Bergstedt, R. A., and Sorensen, P. W. (2011). Olfactory-mediated stream-finding behavior of migratory adult sea lamprey (Petromyzon marinus). Can. J. Fish. Aquat. Sci. 68, 523–533. doi: 10.1139/F10-169
Vrieze, L. A., Bjerselius, R., and Sorensen, P. W. (2010). Importance of the olfactory sense to migratory sea lampreys Petromyzon marinus seeking riverine spawning habitat. J. Fish Biol. 76, 949–964. doi: 10.1111/j.1095-8649.2010.02548.x
Vrieze, L. A., and Sorensen, P. W. (2001). Laboratory assessment of the role of a larval pheromone and natural stream odor in spawning stream localization by migratory sea lamprey (Petromyzon marinus). Can. J. Fish. Aquat. Sci. 58, 2374–2385. doi: 10.1139/f01-179
Wagner, C. M., Twohey, M. B., and Fine, J. M. (2009). Conspecific cueing in the sea lamprey: do reproductive migrations consistently follow the most intense larval odour? Anim. Behav. 78, 593–599. doi: 10.1016/j.anbehav.2009.04.027
Wagner, H. J. (2001). Sensory brain areas in mesopelagic fishes. Brain Behav. Evol. 57, 117–133. doi: 10.1159/000047231
Wagner, H. J. (2003). Volumetric analysis of brain areas indicates a shift in sensory orientation during development in the deep-sea grenadier Coryphaenoides armatus. Mar. Biol. 142, 791–797. doi: 10.1007/s00227-002-0990-7
Wagner, H. J., and Mattheus, U. (2002). Pineal organs in deep demersal fish. Cell Tissue Res. 307, 115–127. doi: 10.1007/s00441-001-0482-y
Weigle, C., and Northcutt, R. G. (1998). To the phylogenetic origin of the cerebellum: tracing studies on the silver lamprey Ichthyomyzon unicuspis. Eur. J. Neurosci. 10, 196–196.
Weigle, C., and Northcutt, R. G. (1999). The chemoarchitecture of the forebrain of lampreys: evolutionary implications by comparisons with gnathostomes. Eur. J. Morphol. 37, 122–125. doi: 10.1076/ejom.37.2-3.0122
Wicht, H. (1996). The brains of lampreys and hagfishes: characteristics, characters, and comparisons. Brain Behav. Evol. 48, 248–261. doi: 10.1159/000113204
Willemet, R. (2012). Understanding the evolution of mammalian brain structures; the need for a (new) cerebrotype approach. Brain Sci. 2, 203–224. doi: 10.3390/brainsci2020203
Willemet, R. (2013). Reconsidering the evolution of brain, cognition, and behavior in birds and mammals. Front. Psychol. 4:396. doi: 10.3389/fpsyg.2013.00396
Yáñez, J., Pombal, M. A., and Anadón, R. (1999). Afferent and efferent connections of the parapineal organ in lampreys: a tract tracing and immunocytochemical study. J. Comp. Neurol. 403, 171–189.
Yopak, K. E. (2012). Neuroecology of cartilaginous fishes: the functional implications of brain scaling. J. Fish Biol. 80, 1968–2023. doi: 10.1111/j.1095-8649.2012.03254.x
Yopak, K. E., and Frank, L. R. (2009). Brain size and brain organization of the whale shark, Rhincodon typus, using magnetic resonance imaging. Brain Behav. Evol. 74, 121–142. doi: 10.1159/000235962
Yopak, K. E., and Lisney, T. J. (2012). Allometric scaling of the optic tectum in cartilaginous fishes. Brain Behav. Evol. 80, 108–126. doi: 10.1159/000339875
Yopak, K. E., Lisney, T. J., Darlington, R. B., Collin, S. P., Montgomery, J. C., and Finlay, B. L. (2010). A conserved pattern of brain scaling from sharks to primates. Proc. Natl. Acad. Sci. U.S.A. 107, 12946–12951. doi: 10.1073/pnas.1002195107
Yopak, K. E., and Montgomery, J. C. (2008). Brain organization and specialization in deep-sea chondrichthyans. Brain Behav. Evol. 71, 287–304. doi: 10.1159/000127048
Yopak, K. E., Lisney, T. J., and Collin, S. P. (2015). Not all sharks are “swimming noses”: variation in olfactory bulb size in cartilaginous fishes. Brain Struct. Funct. 220, 1127–1143. doi: 10.1007/s00429-014-0705-0
Youson, J. H., Wright, G. M., and Ooi, E. C. (1977). The timing of changes in several internal organs during metamorphosis of anadromous larval lamprey Petromyzon marinus. Can. J. Zool. 55, 469–473. doi: 10.1139/z77-063
Keywords: growth, agnathan, lifestyle, filter feeder, heterochrony, jawless vertebrate, metamorphosis, parasite
Citation: Salas CA, Yopak KE, Warrington RE, Hart NS, Potter IC and Collin SP (2015) Ontogenetic shifts in brain scaling reflect behavioral changes in the life cycle of the pouched lamprey Geotria australis. Front. Neurosci. 9:251. doi: 10.3389/fnins.2015.00251
Received: 17 April 2015; Accepted: 03 July 2015;
Published: 28 July 2015.
Edited by:
Jorge Mpodozis, Universidad de Chile, ChileReviewed by:
Andrew Iwaniuk, University of Lethbridge, CanadaThomas Lisney, University of Western Australia, Australia
Copyright © 2015 Salas, Yopak, Warrington, Hart, Potter and Collin. This is an open-access article distributed under the terms of the Creative Commons Attribution License (CC BY). The use, distribution or reproduction in other forums is permitted, provided the original author(s) or licensor are credited and that the original publication in this journal is cited, in accordance with accepted academic practice. No use, distribution or reproduction is permitted which does not comply with these terms.
*Correspondence: Carlos A. Salas, Neuroecology Group, School of Animal Biology, The University of Western Australia, 35 Stirling Highway, Crawley, WA 6009, Australia,Y2FybG9zLnNhbGFzLnV3YUBnbWFpbC5jb20=