- 1Department of Anatomy and Neurobiology, Boston University, Boston, MA, USA
- 2Neural Systems Laboratory, Department of Health Sciences, Boston University, Boston, MA, USA
- 3Department of Health Sciences, Boston University, Boston, MA, USA
No other modality is more frequently represented in the prefrontal cortex than the auditory, but the role of auditory information in prefrontal functions is not well understood. Pathways from auditory association cortices reach distinct sites in the lateral, orbital, and medial surfaces of the prefrontal cortex in rhesus monkeys. Among prefrontal areas, frontopolar area 10 has the densest interconnections with auditory association areas, spanning a large antero-posterior extent of the superior temporal gyrus from the temporal pole to auditory parabelt and belt regions. Moreover, auditory pathways make up the largest component of the extrinsic connections of area 10, suggesting a special relationship with the auditory modality. Here we review anatomic evidence showing that frontopolar area 10 is indeed the main frontal “auditory field” as the major recipient of auditory input in the frontal lobe and chief source of output to auditory cortices. Area 10 is thought to be the functional node for the most complex cognitive tasks of multitasking and keeping track of information for future decisions. These patterns suggest that the auditory association links of area 10 are critical for complex cognition. The first part of this review focuses on the organization of prefrontal-auditory pathways at the level of the system and the synapse, with a particular emphasis on area 10. Then we explore ideas on how the elusive role of area 10 in complex cognition may be related to the specialized relationship with auditory association cortices.
Overview
It is quite remarkable that there is not a waking moment that is completely free of sound. Whether it is the buzzing of our surroundings or on-going conversations, our minds are bombarded by endless streams of auditory signals [e.g., (Conway et al., 2001; Denham and Winkler, 2006; Jaaskelainen et al., 2007; Micheyl et al., 2007); reviewed in (Bee and Micheyl, 2008; Winkler et al., 2009)]. Superimposed on the external auditory environment is an inward stream of thoughts akin to the external that contributes to the sea of auditory signals [e.g., (Scott et al., 2013b); reviewed in (Haykin and Chen, 2005; Allen et al., 2008; Perrone-Bertolotti et al., 2014)]. But what is more remarkable is our ability to sort out what is important in this sea of noise. The prefrontal cortex is necessary for the function of selecting relevant information and suppressing irrelevant signals for the task at hand (reviewed in Knight et al., 1999; Miller and Cohen, 2001). The interaction of prefrontal cortices with auditory association cortices provides an excellent demonstration of this prefrontal executive function (Chao and Knight, 1997), which is thought to reach beyond auditory processing per se, and extend to the global process of “using our thoughts” to guide cognitive tasks [e.g., (Frith, 1996; Wenzlaff and Wegner, 2000; Brewin and Smart, 2005); reviewed in (Knight et al., 1999; Allen et al., 2008; Winkler et al., 2009; Perrone-Bertolotti et al., 2014)]. The behavioral exemplars of these prefrontal-auditory interactions are evident in our daily lives—from following a conversation in a crowded room or tackling an inner debate on what to order from a menu—but the neural substrate and mechanisms are unclear.
From a neuroanatomical perspective, the importance of auditory information in prefrontal function is intuitive given that no other sensory modality is more frequently and vastly represented in the prefrontal cortex than the auditory modality (for review see Barbas et al., 2002). Pathways from auditory association cortices reach lateral, medial, and orbital surfaces of the prefrontal cortex. But the densest prefrontal interconnections with auditory association areas are with the frontopolar cortex, area 10, which mediates the most complex and abstract cognitive tasks (Figure 1; reviewed in Barbas et al., 2002; Burgess et al., 2007; Koechlin and Hyafil, 2007; Smith et al., 2007; Badre and D'Esposito, 2009).
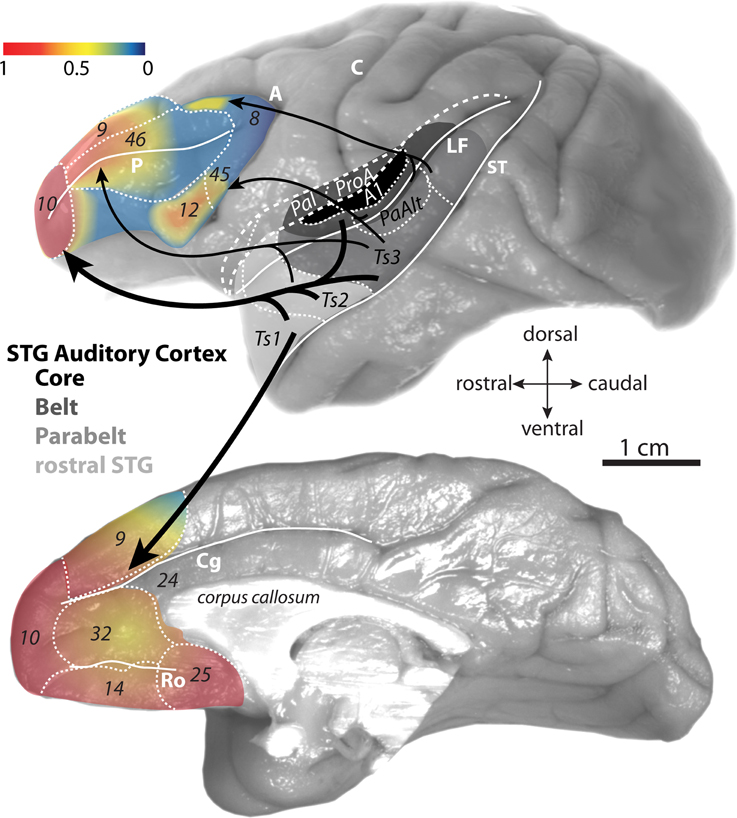
Figure 1. Gradient map of auditory input to the prefrontal cortex. Lateral (top) and medial (bottom) surfaces of the rhesus monkey brain show proportions of afferent pathways (projection neurons) from STG to prefrontal cortex. The proportions (derived from Barbas and Mesulam, 1985 and Barbas et al., 1999) are normalized against the highest number in the set and expressed as a gradient map. The relative proportions are also represented by the weights of the arrows denoting the STG→prefrontal pathways. Architectonic areas of the rhesus monkey auditory cortices in the STG are according to map of Galaburda and Pandya (1983) and functional divisions are according to Hackett et al. (1999). Long dashes demarcate banks of sulci schematically unfolded and short dashes delineate areal boundaries. Abbreviations for sulci: A, arcuate; C, central; Cg, cingulate; LF, lateral fissure; Ro, rostral; ST, superior temporal.
The frontopolar cortex is situated in the most anterior part of the prefrontal cortex, extending from the lateral to the medial and orbital surfaces (Barbas and Pandya, 1989; Petrides, 2005). The function of this region had remained elusive and it was not until the advent of human functional neuroimaging that its role in complex cognition began to emerge (reviewed in Burgess et al., 2007; Koechlin and Hyafil, 2007). Early detailed physiologic work on prefrontal function in non-human primates had focused on visual-related processing, specifically the caudal periarcuate area 46 and area 8, the frontal eye fields (FEF) (Jacobsen, 1936; Robinson and Fuchs, 1969; Fuster, 1973; Niki and Watanabe, 1976). Studies in these periarcuate regions have led to important findings on the role of the prefrontal cortex in the active maintenance of information for a task at hand (working memory) and attention (reviewed in Goldman-Rakic, 1995; Fuster, 2001; Constantinidis and Procyk, 2004; Funahashi, 2006). While these findings are pivotal to our understanding of the prefrontal cortex, it is often not emphasized that visual information in lateral prefrontal cortex is represented in rather restricted areas within the caudal periarcuate region and at the most posterior part of the principal sulcus (Barbas and Mesulam, 1981, 1985; Barbas, 1988; Bullier et al., 1996).
Early anatomic studies showed that visual and visuomotor connections were confined to caudal lateral prefrontal areas (Barbas and Mesulam, 1981, 1985). Moreover, the studies revealed a striking opposite gradient in visual and auditory input along the rostro-caudal extent of the principal sulcus in rhesus monkeys (Barbas and Mesulam, 1985). While visual input was robust caudally, projections from auditory association cortices were sparse in caudal peri-principalis regions and became progressively denser in rostral areas, with the densest auditory pathways directed to the rostral frontopolar cortex in area 10 (Figure 2). While classical and modern anatomic studies have found strong auditory-related connections in the frontal pole (Pandya and Kuypers, 1969; Chavis and Pandya, 1976; Petrides and Pandya, 1988; Barbas et al., 1999, 2005; Hackett et al., 1999; Romanski et al., 1999a,b; Medalla et al., 2007), it is generally understated in the functional literature. This is in striking contrast to the well-known functional involvement of the caudal periarcuate FEF in visual tasks [e.g., (Mishkin, 1966; Robinson and Fuchs, 1969; Mohler et al., 1973); reviewed in (Bruce and Goldberg, 1984; Schiller and Tehovnik, 2001; Schall and Boucher, 2007)]. The extrinsic connections of the frontal pole are nearly exclusively with auditory association areas in rhesus monkeys, which rival in strength the visual-related connections of the FEF [(Barbas and Mesulam, 1985); reviewed in (Barbas et al., 2002; Lynch and Tian, 2005)]. Moreover, while most of the prefrontal cortex receives highly-processed high-order sensory information, both area 10 and the FEF receive projections from relatively “early” unimodal sensory association cortices as well [(e.g., auditory belt and parabelt areas for area 10, and areas V2 and V4 for FEF); (Barbas and Mesulam, 1985); reviewed in (Barbas et al., 2002)]. Early-processing sensory areas receive strong direct input from the primary cortices [e.g., (Pandya et al., 1988; Rauschecker et al., 1997; Kaas and Hackett, 1998)]. In this light, the frontopolar area 10 may be regarded as the frontal “auditory field,” to reflect the emphasis of its rich and varied bidirectional connections with auditory association cortices.
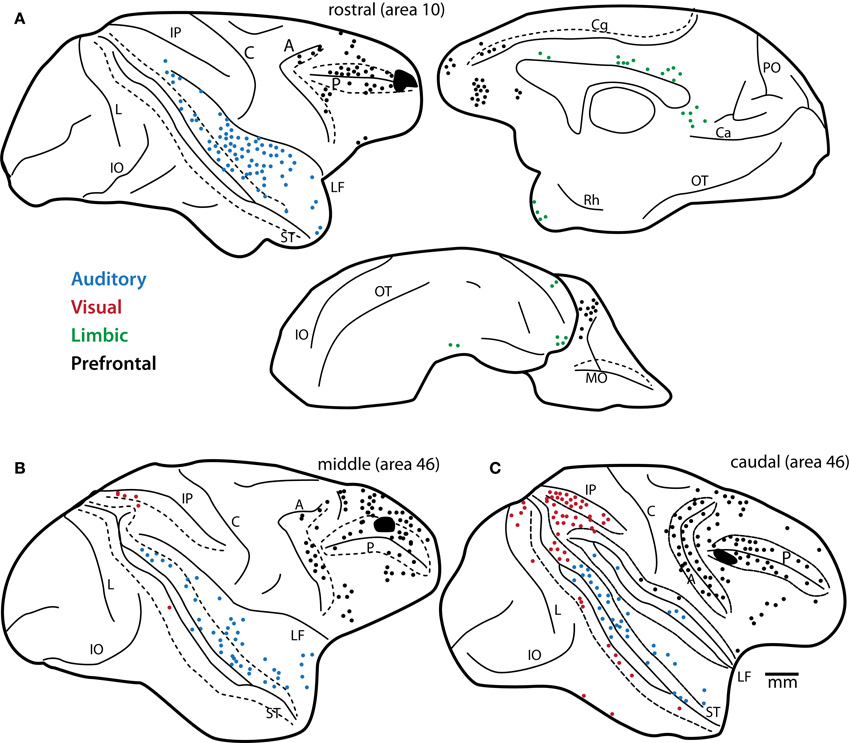
Figure 2. Predominance of auditory projection neurons directed to area 10. Distribution of projection neurons from STG to area 10 in comparison to lateral prefrontal areas. Maps show plots of projection neurons after injection of retrograde neural tracers in (A) rostral (area 10 injection), (B) middle (mid-area 46, dorsal) and (C) caudal (caudal area 46) areas of the lateral prefrontal cortex. Note the decrease in auditory projection neurons (blue) and increase in visual projection neurons (red) in the caudal site (C), shown on the lateral surface. In (A) a complete map of pathways directed to area 10 shows projection neurons in prefrontal (black dots), auditory (blue), and limbic (green) cortices on the lateral, medial and orbital surfaces of the rhesus monkey brain. Note the predominance of auditory projection neurons directed to area 10 from extrinsic sources outside the prefrontal cortex. Long dashes demarcate banks of sulci schematically unfolded. Abbreviations for sulci: A, arcuate; C, central; Ca, calcarine; Cg, cingulate; IO, inferior occipital; IP, intraparietal; L, lunate; LF, lateral fissure; MO, medial orbital; OT, occipitotemporal; PO, parieto-occipital; Rh, rhinal; ST, superior temporal. Adapted from Barbas and Mesulam, 1985.
Organization of Auditory-Related Prefrontal Areas
While auditory connections predominate for area 10 among prefrontal areas, auditory input impinges on several prefrontal auditory “hotspots” on the lateral and medial surfaces (Figure 1; Pandya and Kuypers, 1969; Chavis and Pandya, 1976; Barbas and Mesulam, 1981, 1985; Petrides and Pandya, 1988, 2002; Morel et al., 1993; Kaas and Hackett, 1998; Barbas et al., 1999, 2005; Hackett et al., 1999; Romanski et al., 1999a,b; Medalla et al., 2007). Auditory pathways also reach areas within the largely multimodal orbitofrontal cortex, but this is discussed elsewhere (reviewed in Barbas et al., 2011).
Auditory Association Cortices that are Linked with Prefrontal Cortices
The auditory cortices that are most strongly connected with prefrontal areas lie within the superior temporal gyrus (STG), extending from the inferior bank of the lateral fissure to the upper (medial) bank of the superior temporal sulcus (Figure 1, top). This temporal region is subdivided into distinct areas, according to the maps of Galaburda and Pandya (1983) and Hackett et al. (1999). These areas fall within four main subdivisions of the functional map of the auditory cortex: the core area, which includes the primary auditory cortex; the adjacent belt and parabelt region, and the anterior temporal polar region (reviewed in Romanski and Averbeck, 2009; Figure 1, top).
The prefrontal cortex is interconnected roughly with the anterior two thirds of STG, which extends from temporal polar cortex through anterior parabelt and belt areas (Figure 1, top). These parts of STG consist mostly of high-order auditory association areas that respond to complex auditory stimuli [(Plakke et al., 2013; Ng et al., 2014); reviewed in (Romanski and Averbeck, 2009)]. A small subset of prefrontal interconnections include caudal auditory belt areas (Figure 1, top; Hackett et al., 1999; Romanski et al., 1999a,b). Physiologic and metabolic mapping studies show activation of these temporal and interconnected prefrontal areas in response to auditory stimuli (Rauschecker et al., 1997; Poremba et al., 2004; Plakke et al., 2013; Ng et al., 2014). Details of the organization of the auditory cortex can be found elsewhere (reviewed in Romanski and Averbeck, 2009). This review focuses specifically on the anatomic organization of prefrontal-temporal pathways that may shed light on the mechanism of communication and information transfer within a network for high-order cognition.
Topography of Prefrontal “Hotspots” for Auditory Inputs and Outputs
In the lateral prefrontal cortex, there is a graded increase in the density of auditory connections along the caudal to rostral axis (Figure 2; Barbas and Mesulam, 1985). Within the caudal lateral prefrontal cortex, auditory input is relatively restricted to specific domains of rostral dorsal area 8 (Barbas and Mesulam, 1981) and areas 45 and 12 in the ventrolateral prefrontal cortex (Figure 1, top; Hackett et al., 1999; Romanski et al., 1999a,b). These areas receive pathways from auditory association cortices from a restricted and more caudal part of STG, within the parabelt and belt areas (Figure 1, top; Barbas and Mesulam, 1981, 1985; Hackett et al., 1999; Romanski et al., 1999a,b). These areas also receive significant projections from visual association cortices and are thought to be sites of visual-auditory convergence in the prefrontal cortex [(Barbas, 1988); reviewed in (Romanski, 2007)]. Electrical stimulation of rostral dorsal area 8 (area 8a) in the upper bank of the arcuate sulcus, elicits large amplitude saccades (Robinson and Fuchs, 1969; Mohler et al., 1973; Bruce and Goldberg, 1985) and has been discussed as a region that may help direct attention to peripheral visual and auditory stimuli [e.g., (Barbas and Mesulam, 1981)]. Ventrolateral areas 12 and 45 have been the most well studied in terms of single-unit recordings during auditory tasks in awake behaving monkeys. In the ventrolateral prefrontal cortex, neurons are responsive to complex acoustic stimuli, including species-specific vocalizations that involve a complex interplay of visual and auditory information (reviewed in Romanski and Averbeck, 2009). In the more anterior lateral prefrontal areas, mid-dorsolateral areas 46 and 9, pathways from auditory cortices are stronger as visual input wanes (Figures 2B,C). This mid-dorsolateral prefrontal cortex is thought to play a prominent role in classic working memory-temporary active maintenance of information needed to perform the task at hand (reviewed in Levy and Goldman-Rakic, 2000; Petrides, 2005).
Unlike the restricted patches in lateral prefrontal cortices that have connections with auditory association cortices, the representation of the auditory modality is widespread in the medial prefrontal cortex (Figure 1). Dorsolateral area 9 extends to the medial surface, where there is graded increase in connections with auditory cortices (Barbas et al., 1999). Dense auditory pathways along the medial wall reach all the way from rostral medial prefrontal area 10 to more caudally situated areas 14, 32, and 25 (Figure 1, bottom; Barbas et al., 1999). In particular, auditory connections in areas 32 and 25 of the anterior cingulate cortex (ACC) rival in density auditory connections with area 10. However, unlike area 10, which is privy to information from both early and high-order auditory association cortices, the ACC has access only to highly-processed auditory information through dense interconnections with the rostral STG, especially with areas near the temporal pole [(Barbas et al., 1999); reviewed in (Barbas et al., 2002)]. Nonetheless, with its robust anatomic links with the rostral auditory association cortices, the ACC can be regarded as the medial frontal auditory “hotspot.” In fact, the ACC has a demonstrated robust and functional interaction with auditory areas. Electrical stimulation of the ACC can evoke species-specific vocalizations in monkeys, a pathway thought to mediate emotional communication [(Muller-Preuss et al., 1980; Muller-Preuss and Ploog, 1981); reviewed in (Vogt and Barbas, 1988)]. Activity in the ACC has also been correlated with auditory processing of actual and internal speech in humans (Frith et al., 1995; McGuire et al., 1995).
In contrast to the lateral and medial prefrontal cortices described above, the role of auditory connections in area 10 processing is largely unknown. It was not until recently that the first electrophysiologic recordings from individual neurons in area 10 of non-human primates were conducted, ironically using visual stimuli (Tsujimoto et al., 2010). In that study, a subset of neurons in area 10 showed decision-selective activity but only during the feedback period of a visual working memory task. In humans, highly complex cognitive tasks that engage area 10 also entail high-order verbal processing [e.g., (Brewin and Smart, 2005; Bunge et al., 2005; Christoff et al., 2011); reviewed in (Wenzlaff and Wegner, 2000; Burgess et al., 2007; Koechlin and Hyafil, 2007; Badre and D'Esposito, 2009)]. Nonetheless, the functionally unexplored frontopolar-auditory network is anatomically robust and in recent years we have used high-resolution tract-tracing and imaging techniques to elucidate the organization of these pathways at the level of the system and the synapse.
Structural Organization of Frontopolar Area 10
Frontopolar area 10 in rhesus monkeys is a granular cortical area with a well-defined layer IV; it encompasses about the anterior quarter of the prefrontal cortex (Figure 3A; Barbas and Pandya, 1989). Area 10 has expanded significantly in humans, especially on the lateral surface, concomitant with the expansion of other lateral prefrontal areas (Semendeferi et al., 2011). Recent mapping studies that compare functional coupling of activation across cortical areas in humans and monkeys have shown that the entire macaque area 10 corresponds only to the medial part of the human frontal pole (Sallet et al., 2013; Neubert et al., 2014).
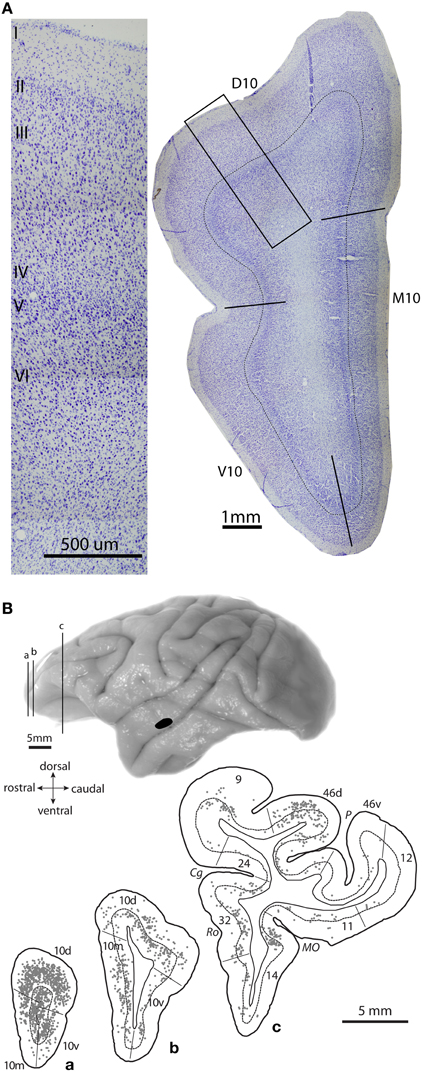
Figure 3. Architecture of area 10 and prefrontal projection neurons to auditory cortex. (A) Photomicrograph of a coronal Nissl-stained section shows the cytoarchitecture of area 10 in a rhesus monkey brain, with delineated dorsal, medial and ventral subregions (right). Thin line marks the top of layer IV. Inset shows location of a higher magnification photomicrograph of dorsal area 10 (left). (B) Lateral view of a rhesus monkey brain shows injection site of a retrograde tracer in STG area Ts2 (black); (a–c), Coronal sections show plots of projection neurons in prefrontal cortices directed to the STG site in area Ts2. Adapted from Medalla et al., 2007.
In the rhesus monkey, area 10 has dorsal, medial and ventral (basal) subdivisions, all of which are interconnected with auditory areas of the STG (Figures 3A, right, 3B; Pandya and Kuypers, 1969; Chavis and Pandya, 1976; Petrides and Pandya, 1988). In addition to auditory connections, area 10 is heavily interconnected with other parts of the prefrontal cortex, especially dorsolateral prefrontal areas 9/46 and ACC area 32 [Figure 2A, black; (Barbas and Pandya, 1989; Barbas et al., 1999; Medalla and Barbas, 2010); reviewed in (Barbas et al., 2002)]. With regard to the extrinsic connections of area 10 outside the prefrontal cortex, most are with auditory association areas (Figure 2A, blue), and the rest are with the cortical limbic system in the cingulate, retrosplenial, rhinal, and anterior temporal polar cortices [Figure 2A, green; (Barbas and Mesulam, 1985; Barbas et al., 1999); reviewed in (Barbas et al., 2002)]. Area 10 receives massive input from a wide spectrum of STG areas, spanning from rostral sectors (temporal pole, Ts1-2 or STGr) to the more caudally situated anterior parabelt (area Ts3 or areas RP/RTL/AL) and belt (PaI/RTM and ProA/RM) auditory association cortices (Figures 1A, 2A; Barbas and Mesulam, 1981, 1985; Hackett et al., 1999; Romanski et al., 1999a,b). The reciprocal connections from area 10 to STG are also robust, encompassing a similarly widespread rostro-caudal extent along the STG (Figures 3B, 4A; Barbas et al., 2005; Germuska et al., 2006; Medalla et al., 2007). In recent years, we have investigated the fine structural features of these pathways from area 10 to STG to shed light on the potential influence of frontopolar area 10 on auditory processing within the STG.
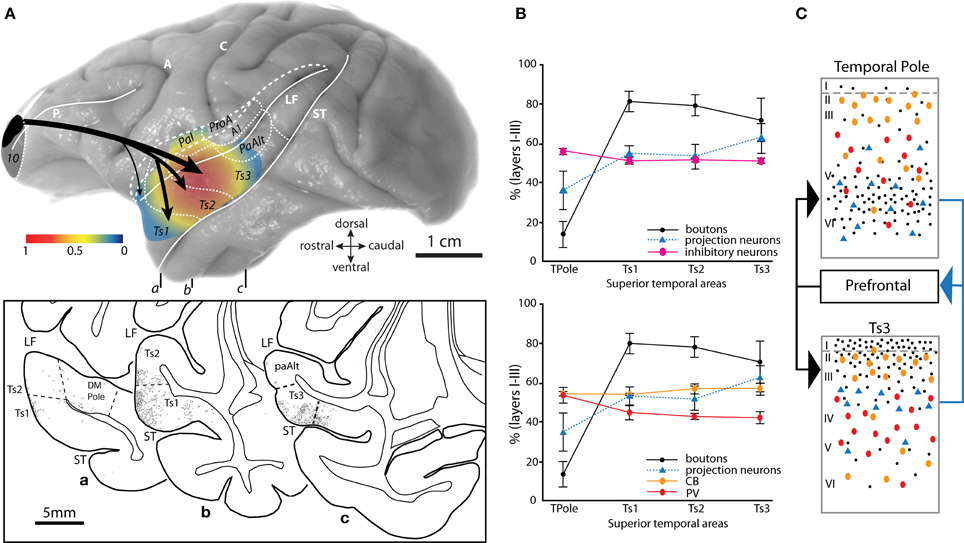
Figure 4. Topography and laminar terminals of pathways from area 10 in distinct auditory cortices. (A) Gradient map shows the relative density of area 10 pathway terminations in distinct STG areas. Axon terminals were labeled after injection of anterograde tracers in dorsal area 10. Density is normalized to the highest in the set. Long dashes demarcate banks of sulci schematically unfolded; short dashes delineate areal boundaries. Bottom inset shows coronal sections (a–c) through STG with plots of labeled terminations from area 10. Rostro-caudal level of each section is indicated on the whole brain (top). (B) The laminar distribution (expressed as percent in the upper layers I–III) of prefrontal interconnections relative to the laminar distribution of inhibitory neurons labeled with PV and CB in distinct STG areas. Top panel shows the combined proportion of CB and PV, bottom panel shows CB and PV proportions depicted separately. (C) Schematic summarizes the predominant pattern of connections (boutons, black dots; projection neurons, blue triangles) of prefrontal cortices with the agranular and dysgranular (limbic) parts of the temporal pole (top) and with a caudal eulaminate area of the superior temporal cortex (bottom), and their relationship to PV+ (red ovals) and CB+ (orange ovals) inhibitory interneurons. Black arrows (left) show the predominant laminar termination of prefrontal axons in superior temporal areas; blue arrow (right) shows the predominant laminar origin of projection neurons in superior temporal areas directed to prefrontal cortex. Abbreviations as in Figures 1, 2. Adapted from Barbas et al., 2005.
Frontopolar Area 10 Pathways to Auditory Association Cortices
Graded Laminar Terminations from Area 10 to Distinct Auditory Association Areas
Pathways from area 10 terminate densely in rostral parts of STG and extend caudally to auditory parabelt and belt areas (Figure 4A; Barbas et al., 2005; Germuska et al., 2006; Medalla et al., 2007). The highest densities of axon terminals from area 10 reach areas Ts1-3, especially anterior parabelt area Ts3 (Figure 4A). There is a graded pattern of terminations in the cortical layers targeted by area 10 in STG (Figures 4B,C). Interestingly, this graded pattern of laminar termination coincides with graded changes in cortical structure of the targeted cortices in STG, characterized by an increase in neuronal density and granularity (appearance of layer IV) from temporal polar areas to more posterior STG cortices (Barbas and Rempel-Clower, 1997; Rempel-Clower and Barbas, 2000; Barbas et al., 2005). A large part of the temporal polar cortex is limbic type of cortex, characterized by an absent layer IV (agranular) or a poorly delineated layer IV (dysgranular). Posterior association areas are granular, with well-delineated cortical layers (Galaburda and Pandya, 1983). Axon terminals from area 10 are densest in the deep cortical layers (V–VI) of the agranular/dysgranular STG cortices in the temporal pole (Figures 4B, black dots; 4C top), but are densest in the upper layers (I–IIIa) of the granular posterior auditory association areas Ts1-3 (Figures 4B, black dots, 4C bottom; Barbas et al., 2005). This pattern is consistent with our structural model for cortico-cortical connections, which relates the laminar pattern of connections to the structural difference between interconnected areas (Barbas and Rempel-Clower, 1997; Rempel-Clower and Barbas, 2000; Barbas et al., 2005; Medalla and Barbas, 2006). Pathways that terminate in the upper layers emanate from areas that have a simpler laminar structure than the area of termination (e.g., a pathway from an agranular to a granular cortex). Pathways that terminate in the middle-deep layers link areas with the opposite structural relationship.
The significance of laminar termination patterns is that cortical layers are also distinct in terms of the excitatory and inhibitory neuronal microenvironment in different auditory association cortices [Figures 4B,C; (Barbas et al., 2005); reviewed in (Barbas et al., 2002)]. In the temporal pole, fibers from area 10 are concentrated in the middle-deep layers where they overlap with the dense population of excitatory layer V–VI pyramidal neurons that project back to area 10, but largely avoid the large population of inhibitory neurons found in the upper layers (Figures 4B,C, top). In contrast, axon fibers from area 10 reach primarily the upper layers of auditory association areas Ts1-3, where they co-mingle with the population of excitatory projection neurons in layers II–III that project to area 10, as well as the population of calbindin and calretinin inhibitory neurons, which are dense in the upper layers (Figures 4B,C, bottom). Thus, while there is a laminar “match” with regard to the prevalence of excitatory connections and inhibitory neurons in the auditory association areas Ts1-3, there is a laminar “mismatch” in the temporal pole where excitatory connections predominate in the deep layers but most inhibitory neurons are found in the upper layers in this region (Barbas et al., 2005).
Laminar Specific Synaptic Features of Area 10 Terminations in Auditory Association Cortex
Our recent work has focused on the pathways within the prefrontal-auditory network at the synaptic level, including features of axon terminals from area 10 to distinct cortical layers of STG areas Ts1-2 (Figures 5A,B, pathways a, b, c). We found a progressive increase in the size (volume) of area 10 axon terminals (boutons) in STG from layer I (Figures 5A,B, a), to layers II–IIIa (Figures 5A,B, b), and to the middle layer IV (Figures 5A,B, c; Germuska et al., 2006; Medalla et al., 2007). Thus, boutons from area 10 (Figure 5B, blue dots) in layer IV are larger than terminals in layer I of STG. The middle cortical layers are recipient of cortico-cortical and cortico-thalamic “feedforward” driving input, while layer I receives “feedback” modulatory pathways [e.g., (Hashikawa et al., 1995); reviewed in (Felleman and Van Essen, 1991; Jones, 1998; Abbott and Chance, 2005; Lee and Sherman, 2010)]. Large boutons that terminate in layer IV contain more synaptic vesicles and have a larger mitochondrial content than small boutons in layer I (Germuska et al., 2006), suggesting higher synaptic efficacy. The size of presynaptic terminals is correlated with the number of synaptic vesicles (Germuska et al., 2006; Zikopoulos and Barbas, 2007) and the probability of neurotransmitter release (Tong and Jahr, 1994; Murthy et al., 1997, 2001). One of the most efficient “drivers” of cortical neurons, especially in sensory areas, is the thalamic pathway that terminates in layer IV [(Rose and Metherate, 2005; Lee and Sherman, 2008; Cruikshank et al., 2010); reviewed in (Castro-Alamancos and Connors, 1997; Sherman and Guillery, 1998, 2002; Guillery and Sherman, 2002; Jones, 2002; Abbott and Chance, 2005; Silberberg et al., 2005; Lee and Sherman, 2010)]. Thus, as we have suggested previously (Germuska et al., 2006; Medalla et al., 2007) the laminar differences in size of axonal boutons from area 10 that terminate in auditory association cortex suggest differences in the strength of synaptic influence across cortical layers. This evidence suggests that area 10 can exercise diverse excitatory effects on STG association areas depending on the predominance of terminations in the upper or middle layers. In areas Ts1-2, the smaller upper layer terminals from area 10 suggest a predominant modulatory role of this specific pathway. Based on these synaptic relationships and findings of changes in the relative density of upper vs. deep layer terminals from area 10 to distinct STG areas, we have suggested that area 10 may drive activity in the temporal polar areas of STG through dense terminations in the middle-deep layers (Barbas et al., 2005).
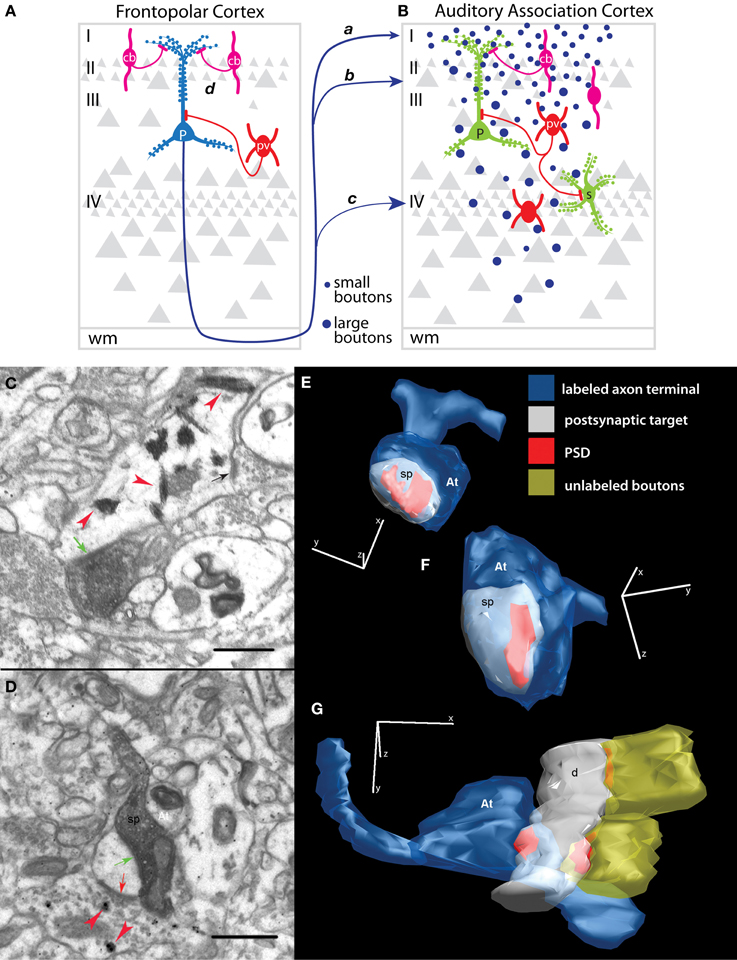
Figure 5. Synapses of prefrontal axons in auditory association cortex. (A,B) Schematic summarizes the predominant synaptic connections of the pathway from frontopolar area 10 to auditory association areas Ts1-3. The pathway from area 10, which terminates predominantly in the upper layers, shows a progressive increase in the volume of axon terminals (B, blue dots) from smallest in layer I (pathway a), through layers II–IIIa (b) and largest in layer IV (c). Boutons that terminate in different layers interact with specific excitatory (green) and inhibitory (red and magenta) dendritic domains and possibly with distinct populations of inhibitory neurons. Area 10 innervates mostly spines of pyramidal neurons (P) in layers I–IIIa, but may interact with other excitatory neuronal types, such as the spiny stellate neurons (s) in layer IV. Among the minority of area 10 axons that innervate inhibitory neurons, synapses are formed on both PV+ (red) and CB+ (magenta) inhibitory neurons that inhibit specific dendritic domains of pyramidal neurons. Inhibitory control may also occur at the site of origin of the pathway (A, pathway d) through inhibition of STG-directed projection neurons in area 10 (P, blue). STG-directed projection neurons are dense in the upper layers of area 10, with apical dendrites overlapping extensively with CB+ inhibitory neurons. (C) Example of an electron micrograph shows tracer-labeled bouton from prefrontal cortex forming an asymmetric (excitatory) synapse (green arrow) on a PV+ dendrite (red arrowheads) in STG. Note the nearby unlabeled synapse (black arrow) on the PV+ dendritic shaft. (D) An electron micrograph shows a labeled prefrontal bouton forming a synapse with a spine in STG (green arrow). Note that the spine receives a symmetric (inhibitory) synapse (red arrow) from a CB+ inhibitory terminal (red arrowheads). (E–G) Examples of three-dimensional reconstructions from serial sections through labeled presynaptic axon terminals (At, blue) from prefrontal pathways and their corresponding postsynaptic densities (PSD, red) and postsynaptic targets in STG photographed in the electron microscope. (E) A small and (F) a large prefrontal bouton (At) each form a synapse (PSD) on a spine (sp, white). (G) A prefrontal bouton forms a synapse with a smooth/aspiny dendrite from a presumed inhibitory neuron in STG. Note the lack of spines and presence of nearby synapses on the shaft from unlabeled boutons, characteristics of smooth dendrites of inhibitory neurons. Adapted from Medalla et al., 2007.
Laminar terminations encounter specific microenvironments with regard to populations and dendritic segments of excitatory and inhibitory postsynaptic targets (reviewed in Peters, 1987; White, 1989; Callaway, 2002; Douglas and Martin, 2004). We found that most (about 80%) of the synapses in the pathway from area 10 to STG (areas Ts1-2) target spines (Germuska et al., 2006; Medalla et al., 2007), which are enriched on the dendrites of cortical excitatory neurons (Figures 5B, green; 5C,E,F from Medalla et al., 2007). The laminar specificity of these spine-targeting boutons can influence which dendritic domains or population of neurons are innervated (reviewed in Silberberg et al., 2005; Spruston, 2008). Layer I is populated with the distal apical dendrites of neurons from the layers below, while the middle-deep layers consist mostly of proximal and basal dendrites of pyramidal neurons (Figure 5B, green P; Larkman and Mason, 1990; Larkman, 1991). Layer IV consists mostly of spiny stellate excitatory neurons (Figure 5B, s) that receive direct thalamic input in sensory cortices [(Peters et al., 1994); reviewed in (White, 1989)]. Thus, area 10 innervates mostly spines from apical dendrites of pyramidal neurons in layer I, but may interact with other dendritic segments and excitatory neuronal types in the deep layers.
Synaptic Interaction of Area 10 with Inhibitory Neurons in STG
Inhibitory neurons in the primate cortex can be reliably identified and grouped by the expression of one of three calcium-binding proteins. One group of neurons expresses parvalbumin (PV, Figures 5A,B, red), a second group expresses calbindin (CB, Figures 5A,B, magenta), and a third group expresses calretinin (not shown). In primates, these neurochemical classes of inhibitory neurons represent distinct non-overlapping populations that differ in distribution, morphology, physiology and synaptic interactions with neighboring neurons (reviewed in Defelipe, 1997). Parvalbumin labels inhibitory neurons that innervate neighboring pyramidal neurons at their proximal dendrites or somata (basket cells) or the axon initial segments (chandelier cells) (Figures 5A,B, red; Defelipe et al., 1989b; Kawaguchi and Kubota, 1997; Thomson and Deuchars, 1997). Parvalbumin neurons have distinct fast-spiking firing properties and are the most reliably identified by physiologic methods [(Kawaguchi and Kubota, 1997; Krimer et al., 2005); reviewed in (Markram et al., 2004)]. The proximal innervation pattern and fast-firing properties of PV neurons suggest strong inhibition with rapid temporal dynamics for controlling the timing of spike output of pyramidal neurons (Rao et al., 1999; Constantinidis and Goldman-Rakic, 2002; Trevelyan and Watkinson, 2005). On the other hand, calbindin labels inhibitory neurons that innervate the distal dendrites and spines of excitatory neurons (Figures 5A,B, magenta; Defelipe et al., 1989a; Kawaguchi and Kubota, 1997; Peters and Sethares, 1997). CB inhibitory neurons are physiologically diverse but they are non-fast spiking and generally have slower firing dynamics than PV neurons (Kawaguchi and Kubota, 1997; Krimer et al., 2005; Zaitsev et al., 2005). It has been suggested that CB neurons engage a modulatory type of dendritic inhibition to selectively enhance the signal-to-noise ratio of relevant inputs within a cortical column (Wang et al., 2004). Interestingly, PV and CB inhibitory neurons in the primate cortex, including area 10 and the STG, have complementary laminar distributions (Hendry et al., 1989; Conde et al., 1994; Kondo et al., 1994; Gabbott and Bacon, 1996; Dombrowski et al., 2001; Medalla and Barbas, 2006). While PV neurons predominate in the middle-deep layers (IIIb–VI), CB neurons are densest in the upper layers (II–IIIa).
In addition to synapses on spines of presumed excitatory neurons, a smaller subset (~20% or less) of synapses from area 10 innervates dendrites of presumed inhibitory neurons in areas Ts1-2 (Figures 5D,G; Germuska et al., 2006; Medalla et al., 2007). By morphology, cortical inhibitory neurons have smooth or sparsely spiny dendrites [(Feldman and Peters, 1978; Kawaguchi et al., 2006); reviewed in (Peters et al., 1991; Fiala and Harris, 1999)], which are features that can readily be assessed at high-resolution, using three-dimensional electron microscopic methods (Figure 5G; Germuska et al., 2006; Medalla et al., 2007; Medalla and Barbas, 2009, 2010, 2012). We have found that area 10 innervates inhibitory neurons in layers I, II–IIIa, and IV of STG, with a trend for a slightly higher frequency in progressively deeper layers (II–IIIa and IV) compared to layer I (Germuska et al., 2006; Medalla et al., 2007). The middle-deep layers of STG are more densely populated by PV inhibitory neurons (Barbas et al., 2005). These layers are also innervated by excitatory “feedforward” cortico-cortical and cortico-thalamic fibers in the auditory cortex (Rose and Metherate, 2005; Lee and Sherman, 2008) and other cortical areas [(Melchitzky et al., 1999; Zhu and Connors, 1999; Beierlein et al., 2003; Gonchar and Burkhalter, 2003; Negyessy and Goldman-Rakic, 2005; Zikopoulos and Barbas, 2007; Cruikshank et al., 2010); reviewed in (White, 1989; Peters et al., 1994)]. We found that terminals from area 10 in layers II–IIIa of STG (areas Ts1-2) innervate CB neurons, as well as PV neurons (Figure 5B; Medalla et al., 2007). Thus, area 10 has the potential to engage two distinct modes of inhibition in STG: modulatory CB-mediated as well as rapid and strong PV-mediated inhibition.
In addition to inhibition at the site of termination in STG, inhibitory control via the area 10 pathway may also occur locally within area 10, by engaging inhibitory neurons that innervate projection neurons directed to STG (Figure 5A, pathway d). We have shown that projection neurons directed to STG areas arise mostly from the upper layers (II–III) of area 10 (Medalla et al., 2007). Pyramidal neurons in layers II–III extend their apical dendrites and arborize profusely in layer I [e.g., (Larkman and Mason, 1990; Larkman, 1991); reviewed in (Silberberg et al., 2005)]. Thus, the proximal and extensive distal apical domains of STG-directed projection neurons in area 10 are sites of potential synaptic innervation by the distinct classes of inhibitory neurons. In particular, the robust laminar overlap of STG projection neurons and CB neurons in layers II–IIIa suggests CB-mediated inhibition of auditory-directed projection neurons in area 10 (Figure 5A, magenta; Medalla et al., 2007).
In summary, pathway terminations from area 10 are diverse in distribution and synaptic features, which depend on the specific STG area and cortical layer of termination. These varied innervation patterns suggest that area 10 may have diverse influences on excitatory and inhibitory microcircuits in STG areas, allowing flexibility in prefrontal-auditory functional interactions for complex cognition.
Functional Implications of Prefrontal-Auditory Pathways in High-Order Cognition
Auditory Connections of Area 10 for Complex Cognition
The robust and diverse synaptic pathways from area 10 to the STG suggest a tight link between area 10 function and auditory processing. The evidence reviewed points to a specialized relationship of area 10 with the auditory association cortex as a key frontal “auditory field.” Area 10 receives information from almost all levels of processing in the STG—from the very detailed and early sensory processing in belt and parabelt areas to the complex high-order processing of acoustic stimuli for con-specific communication in temporal polar areas [e.g., (Poremba et al., 2004; Kusmierek and Rauschecker, 2009; Kikuchi et al., 2010); reviewed in (Romanski and Averbeck, 2009)]. The question thus arises as to how these connections are used in high-order cognitive functions mediated by area 10.
The frontal pole is thought to be part of the working memory network together with dorsolateral prefrontal areas 9/46, engaged for active maintenance of information for the task at hand (reviewed in Petrides, 2000; Barbas et al., 2002). Baddeley (1996) proposed two important components for working memory in humans—a visuospatial scratchpad and an articulatory loop. This is not surprising from an anatomical perspective, given that the working memory system in the lateral prefrontal cortex is indeed predominated posteriorly by visual-related periarcuate areas (caudal area 46 and FEF) and anteriorly by auditory-related areas (area 10 and mid-dorsolateral areas 46/9). Interestingly, this auditory-visual gradient along the rostro-caudal axis of lateral prefrontal areas is thought to coincide with a functional hierarchy by complexity of processing (reviewed in Petrides, 2000; Barbas et al., 2002; Burgess et al., 2007; Koechlin and Hyafil, 2007; Smith et al., 2007; Badre and D'Esposito, 2009). Area 10 is thought to be at the top of this hierarchy, which mediates the most complex and abstract cognitive tasks. This idea is exemplified in human functional neuroimaging studies that have shown specific recruitment of area 10 during complex multi-tasking, when one task must be temporarily suspended to attend to another task [(Koechlin et al., 1999; Burgess et al., 2000; Braver et al., 2003; Dreher et al., 2008; Tsujimoto et al., 2010); reviewed in (Burgess et al., 2007; Koechlin and Hyafil, 2007; Smith et al., 2007; Badre and D'Esposito, 2009)]. This can be illustrated for instance when a person interrupts cooking a meal to answer the phone and subsequently resumes cooking from where one left off. Moreover, the functional imaging studies have shown that multi-task functions and other related complex cognitive tasks mediated by area 10 rely on phonologic processing of “inner thoughts” for mental tracking of multiple information streams [e.g., (Bunge et al., 2005; Christoff et al., 2011); reviewed in (Burgess et al., 2007)]. Thus, area 10 may engage its strong auditory links for abstract representation of information in organized thought during complex cognitive tasks.
It is also interesting that the evolved complexity of cognition from monkeys to humans seems to parallel the cortical expansion of both the auditory system and the frontal pole. In particular, in humans the language cortices have evolved as specialized systems for verbal articulation as the frontal pole has also expanded laterally (Semendeferi et al., 2011; Sallet et al., 2013; Neubert et al., 2014). This evolutionary trend is also evident in the connections of area 10 in different species of non-human primates. Neural tracing in marmoset monkeys has shown a smaller representation of auditory connections in area 10 compared to macaque monkeys (Barbas et al., 1999; Burman et al., 2011). The above evidence is consistent with the idea that as the auditory system evolved in humans, area 10 kept pace with more extensive auditory connections. This trend is also reflected in behavior: as cognitive tasks in humans rely more on verbal information, more complex tasks can be tackled [e.g., (Frith, 1996; Wenzlaff and Wegner, 2000; Brewin and Smart, 2005; Bunge et al., 2005; Christoff et al., 2011); reviewed in (Knight et al., 1999; Allen et al., 2008; Winkler et al., 2009; Perrone-Bertolotti et al., 2014)]. By comparison with humans, monkeys have relatively poor capacity for working memory in the auditory domain (Ng et al., 2009; Scott et al., 2012, 2013a). Based on the functional and anatomical evidence, it is likely that the auditory interactions in a highly evolved area 10 are crucial to a role in high-order cognition in humans.
Interaction of Frontopolar and Medial Prefrontal “Auditory Fields” for Cognitive Control
In addition to area 10, auditory signals impinge on a wide spectrum of prefrontal areas (Figure 1). Particularly strong auditory connections are seen for medial prefrontal areas 32 and 25 in the ACC. Importantly, these prefrontal auditory “hotspots” are also robustly interconnected with each other through intrinsic prefrontal pathways (Barbas and Mesulam, 1985; Barbas and Pandya, 1989; Barbas et al., 1999; Medalla and Barbas, 2010). Thus, we have previously suggested that the prefrontal cortex may use auditory information either through direct connections with STG, or indirectly through local interconnections between auditory-related prefrontal areas (Barbas et al., 2005; Medalla et al., 2007; Medalla and Barbas, 2010).
Frontopolar area 10 and anterior cingulate areas, the rostral and medial frontal “auditory fields” that are most strongly interconnected with the STG, are also robustly linked with each other. In particular, a pathway from ACC area 32 innervates spines of excitatory neurons in area 10 through large and synaptically-effective boutons in layers II–III [(Medalla and Barbas, 2010); reviewed in (Barbas et al., 2013)]. These ACC boutons are larger than in the pathways linking area 10 with other dorsolateral prefrontal areas (46 or 9), and they are comparable in size to the “feedforward/driving” pathway terminations in layer IV of sensory cortices (Melchitzky et al., 2001; Anderson and Martin, 2002, 2005, 2006; Medalla et al., 2007), including the terminations from area 10 to layer IV of STG (Germuska et al., 2006). We have previously suggested that the large ACC terminations may drive and redirect activity in area 10 to help select relevant signals (presumably from auditory areas), and suppress noise for complex multi-task functions (Medalla and Barbas, 2010). This idea is consistent with the prominent role of the ACC in allocating attention and in task-switching, especially when cognitive demand is high (reviewed in Barbas and Zikopoulos, 2007; Botvinick, 2007; Lee et al., 2007; Schall and Boucher, 2007). Interestingly, the ACC has a demonstrated influence in several high-order auditory-related functions by affecting activity in auditory cortices. Microstimulation of ACC evokes species-specific vocalization in monkeys and affects activity in auditory cortices [(Muller-Preuss et al., 1980; Muller-Preuss and Ploog, 1981); reviewed in (Vogt and Barbas, 1988)]. In humans, correlated gamma-band activity in ACC and auditory areas suggests functional coupling between these cortices during demanding cognitive tasks (Mulert et al., 2007).
The ACC-frontopolar-auditory network may mediate high-order filtering of auditory processing to allow communication in a noisy environment. Such filtering has been discussed for the auditory modality, in general (reviewed in Conway et al., 2001; Denham and Winkler, 2006; Jaaskelainen et al., 2007; Micheyl et al., 2007). The pathways that link area 10 with the auditory areas may help keep track of internal thoughts, which is important for working memory and problem solving [e.g., (Brewin and Smart, 2005); reviewed in (Wenzlaff and Wegner, 2000)]. This hypothesis is consistent with findings that the ACC and area 10 are activated during mental tracking of multiple tasks (reviewed in Burgess et al., 2007). The anatomical evidence supports this hypothesis, but behavioral and functional studies that employ auditory-related tasks are needed to investigate the role of auditory input, and the specialized projections from ACC, for cognitive processing in area 10.
Prefrontal-Auditory Pathway Disruption in Disease
Pathology in the prefrontal-auditory network has been implicated in schizophrenia, a disease characterized by high distractibility, disordered thought patterns and auditory hallucinations (reviewed in Cohen et al., 1996; Honey and Fletcher, 2006; Allen et al., 2008). Post-mortem studies in brains of schizophrenic patients show that specific markers for populations of inhibitory and excitatory neurons are diminished in auditory-related ACC and mid-dorsolateral prefrontal areas, disrupting the excitatory and inhibitory balance necessary for normal cognitive functions (reviewed in Benes, 2000; Beasley et al., 2002; Volk and Lewis, 2002; Vogels and Abbott, 2007; Fornito et al., 2009; Eisenberg and Berman, 2010). For instance, the ACC shows a decrease in pyramidal neuron density in the deep layers (Benes et al., 2001) and reduced overall activity in schizophrenia (Fletcher et al., 1999; Kerns et al., 2005; Snitz et al., 2005; Allen et al., 2007; Leicht et al., 2010). The deep layers of ACC give rise to projections to lateral prefrontal cortices in monkeys, in a pattern expected to hold for humans based on the predictions of the structural model for connections (Barbas and Rempel-Clower, 1997). Based on available data on the synaptic circuits within the prefrontal-auditory network, we have speculated that pathologic hypofunction in ACC may weaken its output to inhibitory neurons in other auditory-related prefrontal cortices such as frontopolar area 10 and mid-dorsolateral areas 9/46 (Medalla and Barbas, 2009, 2010). The strong influence of the ACC on CB inhibitory neurons, in particular, suggests a mechanism to suppress noise (Wang et al., 2004). By the same principle, hypofunction especially in the deep layers of ACC may reduce excitation to frontopolar area 10, which is engaged when keeping track of internal thoughts to perform multiple tasks in humans (reviewed in Burgess et al., 2007; Koechlin and Hyafil, 2007; Smith et al., 2007; Badre and D'Esposito, 2009). Weakening of these interactions may account for the high distractibility and disordered thought patterns in schizophrenia (reviewed in Cohen et al., 1996; Honey and Fletcher, 2006; Allen et al., 2008). Finally, the relative activation of ACC and auditory cortices appears to help distinguish actual from inner speech in humans, in functions that are disrupted in schizophrenic patients who experience auditory hallucinations (Frith et al., 1995; McGuire et al., 1995). The strong and specific anatomic pathways interlinking prefrontal and auditory cortices reviewed here thus suggest a key role of these interactions in high-order cognition, and may help explain the impairments in processing of “inner thoughts” that account for the distractibility, disordered thought process and auditory hallucinations in schizophrenia.
Conflict of Interest Statement
The authors declare that the research was conducted in the absence of any commercial or financial relationships that could be construed as a potential conflict of interest.
Acknowledgments
This work is supported by grants from NIH (NIMH R01MH057414 and NINDS R01NS024760, Barbas; NIMH K99MH101234, Medalla) and CELEST, an NSF Science of Learning Center (NSF OMA-0835976).
References
Abbott, L. F., and Chance, F. S. (2005). Drivers and modulators from push-pull and balanced synaptic input. Prog. Brain Res. 149, 147–155. doi: 10.1016/S0079-6123(05)49011-1
Allen, P., Amaro, E., Fu, C. H., Williams, S. C., Brammer, M. J., Johns, L. C., et al. (2007). Neural correlates of the misattribution of speech in schizophrenia. Br. J. Psychiatry 190, 162–169. doi: 10.1192/bjp.bp.106.025700
Allen, P., Laroi, F., McGuire, P. K., and Aleman, A. (2008). The hallucinating brain: a review of structural and functional neuroimaging studies of hallucinations. Neurosci. Biobehav. Rev. 32, 175–191. doi: 10.1016/j.neubiorev.2007.07.012
Anderson, J. C., and Martin, K. A. (2002). Connection from cortical area V2 to MT in macaque monkey. J. Comp. Neurol. 443, 56–70. doi: 10.1002/cne.10100
Anderson, J. C., and Martin, K. A. (2005). Connection from cortical area V2 to V3 A in macaque monkey. J. Comp. Neurol. 488, 320–330. doi: 10.1002/cne.20580
Anderson, J. C., and Martin, K. A. (2006). Synaptic connection from cortical area V4 to V2 in macaque monkey. J. Comp. Neurol. 495, 709–721. doi: 10.1002/cne.20914
Baddeley, A. (1996). The fractionation of working memory. Proc. Natl. Acad. Sci. U.S.A. 93, 13468–13472. doi: 10.1073/pnas.93.24.13468
Badre, D., and D'Esposito, M. (2009). Is the rostro-caudal axis of the frontal lobe hierarchical? Nat. Rev. Neurosci. 10, 659–669. doi: 10.1038/nrn2667
Barbas, H. (1988). Anatomic organization of basoventral and mediodorsal visual recipient prefrontal regions in the rhesus monkey. J. Comp. Neurol. 276, 313–342. doi: 10.1002/cne.902760302
Barbas, H., Bunce, J. G., and Medalla, M. (2013). “Prefrontal pathways that control attention,” in Principles of Frontal Lobe Functions, 2nd Edn, eds D. T. Stuss and R. Knight (New York, NY: Oxford University Press), 31–48.
Barbas, H., Ghashghaei, H., Dombrowski, S. M., and Rempel-Clower, N. L. (1999). Medial prefrontal cortices are unified by common connections with superior temporal cortices and distinguished by input from memory-related areas in the rhesus monkey. J. Comp. Neurol. 410, 343–367. doi: 10.1002/(SICI)1096-9861(19990802)410:3%3C343::AID-CNE1%3E3.0.CO;2-1
Barbas, H., Ghashghaei, H., Rempel-Clower, N., and Xiao, D. (2002). “Anatomic basis of functional specialization in prefrontal cortices in primates,” in Handbook of Neuropsychology, Vol. 7: The Frontal Lobes, ed J. Grafman (Amsterdam: Elsevier Science B. V.), 1–27.
Barbas, H., Medalla, M., Alade, O., Suski, J., Zikopoulos, B., and Lera, P. (2005). Relationship of prefrontal connections to inhibitory systems in superior temporal areas in the rhesus monkey. Cereb. Cortex 15, 1356–1370. doi: 10.1093/cercor/bhi018
Barbas, H., and Mesulam, M. M. (1981). Organization of afferent input to subdivisions of area 8 in the rhesus monkey. J. Comp. Neurol. 200, 407–431. doi: 10.1002/cne.902000309
Barbas, H., and Mesulam, M. M. (1985). Cortical afferent input to the principalis region of the rhesus monkey. Neuroscience 15, 619–637. doi: 10.1016/0306-4522(85)90064-8
Barbas, H., and Pandya, D. N. (1989). Architecture and intrinsic connections of the prefrontal cortex in the rhesus monkey. J. Comp. Neurol. 286, 353–375. doi: 10.1002/cne.902860306
Barbas, H., and Rempel-Clower, N. (1997). Cortical structure predicts the pattern of corticocortical connections. Cereb. Cortex 7, 635–646. doi: 10.1093/cercor/7.7.635
Barbas, H., and Zikopoulos, B. (2007). The prefrontal cortex and flexible behavior. Neuroscientist 13, 532–545. doi: 10.1177/1073858407301369
Barbas, H., Zikopoulos, B., and Timbie, C. (2011). Sensory pathways and emotional context for action in primate prefrontal cortex. Biol. Psychiatry 69, 1133–1139. doi: 10.1016/j.biopsych.2010.08.008
Beasley, C. L., Zhang, Z. J., Patten, I., and Reynolds, G. P. (2002). Selective deficits in prefrontal cortical GABAergic neurons in schizophrenia defined by the presence of calcium-binding proteins. Biol. Psychiatry 52, 708–715. doi: 10.1016/S0006-3223(02)01360-4
Bee, M. A., and Micheyl, C. (2008). The cocktail party problem: what is it? How can it be solved? And why should animal behaviorists study it? J. Comp. Psychol. 122, 235–251. doi: 10.1037/0735-7036.122.3.235
Beierlein, M., Gibson, J. R., and Connors, B. W. (2003). Two dynamically distinct inhibitory networks in layer 4 of the neocortex. J. Neurophysiol. 90, 2987–3000. doi: 10.1152/jn.00283.2003
Benes, F. M. (2000). Emerging principles of altered neural circuitry in schizophrenia. Brain Res. Brain Res. Rev. 31, 251–269. doi: 10.1016/S0165-0173(99)00041-7
Benes, F. M., Vincent, S. L., and Todtenkopf, M. (2001). The density of pyramidal and nonpyramidal neurons in anterior cingulate cortex of schizophrenic and bipolar subjects. Biol. Psychiatry 50, 395–406. doi: 10.1016/S0006-3223(01)01084-8
Botvinick, M. M. (2007). Conflict monitoring and decision making: reconciling two perspectives on anterior cingulate function. Cogn. Affect. Behav. Neurosci. 7, 356–366. doi: 10.3758/CABN.7.4.356
Braver, T. S., Reynolds, J. R., and Donaldson, D. I. (2003). Neural mechanisms of transient and sustained cognitive control during task switching. Neuron 39, 713–726. doi: 10.1016/S0896-6273(03)00466-5
Brewin, C. R., and Smart, L. (2005). Working memory capacity and suppression of intrusive thoughts. J. Behav. Ther. Exp. Psychiatry 36, 61–68. doi: 10.1016/j.jbtep.2004.11.006
Bruce, C. J., and Goldberg, M. E. (1984). Physiology of the frontal eye fields. Trends Neurosci. 7, 436–441. doi: 10.1016/S0166-2236(84)80149-6
Bruce, C. J., and Goldberg, M. E. (1985). Primate frontal eye fields. I. Single neurons discharging before saccades. J. Neurophysiol. 53, 603–635.
Bullier, J., Schall, J. D., and Morel, A. (1996). Functional streams in occipito-frontal connections in the monkey. Behav. Brain Res. 76, 89–97. doi: 10.1016/0166-4328(95)00182-4
Bunge, S. A., Wendelken, C., Badre, D., and Wagner, A. D. (2005). Analogical reasoning and prefrontal cortex: evidence for separable retrieval and integration mechanisms. Cereb. Cortex 15, 239–249. doi: 10.1093/cercor/bhh126
Burgess, P. W., Gilbert, S. J., and Dumontheil, I. (2007). Function and localization within rostral prefrontal cortex (area 10). Philos. Trans. R. Soc. Lond. B Biol. Sci. 362, 887–899. doi: 10.1098/rstb.2007.2095
Burgess, P. W., Veitch, E., De Lacy, C. A., and Shallice, T. (2000). The cognitive and neuroanatomical correlates of multitasking. Neuropsychologia 38, 848–863. doi: 10.1016/S0028-3932(99)00134-7
Burman, K. J., Reser, D. H., Yu, H. H., and Rosa, M. G. P. (2011). Cortical input to the frontal pole of the marmoset monkey. Cereb. Cortex 21, 1712–1737. doi: 10.1093/cercor/bhq239
Callaway, E. M. (2002). Cell type specificity of local cortical connections. J. Neurocytol. 31, 231–237. doi: 10.1023/A:1024165824469
Castro-Alamancos, M. A., and Connors, B. W. (1997). Thalamocortical synapses. Prog. Neurobiol. 51, 581–606. doi: 10.1016/S0301-0082(97)00002-6
Chao, L. L., and Knight, R. T. (1997). Age-related prefrontal alterations during auditory memory. Neurobiol. Aging 18, 87–95. doi: 10.1016/S0197-4580(96)00161-3
Chavis, D. A., and Pandya, D. N. (1976). Further observations on corticofrontal connections in the rhesus monkey. Brain Res. 117, 369–386. doi: 10.1016/0006-8993(76)90089-5
Christoff, K., Prabhakaran, V., Dorfman, J., Zhao, Z., Kroger, J. K., Holyoak, K. J., et al. (2011). Rostrolateral prefrontal cortex involvement in relational integration during reasoning. Neuroimage 14, 1136–1149. doi: 10.1006/nimg.2001.0922
Cohen, J. D., Braver, T. S., and O'Reilly, R. C. (1996). A computational approach to prefrontal cortex, cognitive control and schizophrenia: recent developments and current challenges. Philos. Trans. R. Soc. Lond. B Biol. Sci. 351, 1515–1527. doi: 10.1098/rstb.1996.0138
Conde, F., Lund, J. S., Jacobowitz, D. M., Baimbridge, K. G., and Lewis, D. A. (1994). Local circuit neurons immunoreactive for calretinin, calbindin D- 28k or parvalbumin in monkey prefrontal cortex: distribution and morphology. J. Comp. Neurol. 341, 95–116. doi: 10.1002/cne.903410109
Constantinidis, C., and Goldman-Rakic, P. S. (2002). Correlated discharges among putative pyramidal neurons and interneurons in the primate prefrontal cortex. J. Neurophysiol. 88, 3487–3497. doi: 10.1152/jn.00188.2002
Constantinidis, C., and Procyk, E. (2004). The primate working memory networks. Cogn. Affect. Behav. Neurosci. 4, 444–465. doi: 10.3758/CABN.4.4.444
Conway, A. R., Cowan, N., and Bunting, M. F. (2001). The cocktail party phenomenon revisited: the importance of working memory capacity. Psychon. Bull. Rev. 8, 331–335. doi: 10.3758/BF03196169
Cruikshank, S. J., Urabe, H., Nurmikko, A. V., and Connors, B. W. (2010). Pathway-specific feedforward circuits between thalamus and neocortex revealed by selective optical stimulation of axons. Neuron 65, 230–245. doi: 10.1016/j.neuron.2009.12.025
Defelipe, J. (1997). Types of neurons, synaptic connections and chemical characteristics of cells immunoreactive for calbindin-D28K, parvalbumin and calretinin in the neocortex. J. Chem. Neuroanat. 14, 1–19. doi: 10.1016/S0891-0618(97)10013-8
Defelipe, J., Hendry, S. H., and Jones, E. G. (1989a). Synapses of double bouquet cells in monkey cerebral cortex visualized by calbindin immunoreactivity. Brain Res. 503, 49–54. doi: 10.1016/0006-8993(89)91702-2
Defelipe, J., Hendry, S. H., and Jones, E. G. (1989b). Visualization of chandelier cell axons by parvalbumin immunoreactivity in monkey cerebral cortex. Proc. Natl. Acad. Sci. U.S.A. 86, 2093–2097. doi: 10.1073/pnas.86.6.2093
Denham, S. L., and Winkler, I. (2006). The role of predictive models in the formation of auditory streams. J. Physiol. Paris 100, 154–170. doi: 10.1016/j.jphysparis.2006.09.012
Dombrowski, S. M., Hilgetag, C. C., and Barbas, H. (2001). Quantitative architecture distinguishes prefrontal cortical systems in the rhesus monkey. Cereb. Cortex 11, 975–988. doi: 10.1093/cercor/11.10.975
Douglas, R. J., and Martin, K. A. (2004). Neuronal circuits of the neocortex. Annu. Rev. Neurosci. 27, 419–451. doi: 10.1146/annurev.neuro.27.070203.144152
Dreher, J. C., Koechlin, E., Tierney, M., and Grafman, J. (2008). Damage to the fronto-polar cortex is associated with impaired multitasking. PLoS ONE 3:e3227. doi: 10.1371/journal.pone.0003227
Eisenberg, D. P., and Berman, K. F. (2010). Executive function, neural circuitry, and genetic mechanisms in schizophrenia. Neuropsychopharmacology 35, 258–277. doi: 10.1038/npp.2009.111
Feldman, M. L., and Peters, A. (1978). The forms of non-pyramidal neurons in the visual cortex of the rat. J. Comp. Neurol. 179, 761–793. doi: 10.1002/cne.901790406
Felleman, D. J., and Van Essen, D. C. (1991). Distributed hierarchical processing in the primate cerebral cortex. Cereb. Cortex 1, 1–47. doi: 10.1093/cercor/1.1.1
Fiala, J. C., and Harris, K. M. (1999). “Dendrite structure,” in Dendrites, eds G. Stuart, N. Spruston, and M. Husser (Oxford: Oxford University Press), 1–34.
Fletcher, P., McKenna, P. J., Friston, K. J., Frith, C. D., and Dolan, R. J. (1999). Abnormal cingulate modulation of fronto-temporal connectivity in schizophrenia. Neuroimage 9, 337–342. doi: 10.1006/nimg.1998.0411
Fornito, A., Yucel, M., Dean, B., Wood, S. J., and Pantelis, C. (2009). Anatomical abnormalities of the anterior cingulate cortex in schizophrenia: bridging the gap between neuroimaging and neuropathology. Schizophr. Bull. 35, 973–993. doi: 10.1093/schbul/sbn025
Frith, C. (1996). The role of the prefrontal cortex in self-consciousness: the case of auditory hallucinations. Philos. Trans. R Soc. Lond. B Biol. Sci. 351, 1505–1512. doi: 10.1098/rstb.1996.0136
Frith, C. D., Friston, K. J., Herold, S., Silbersweig, D., Fletcher, P., Cahill, C., et al. (1995). Regional brain activity in chronic schizophrenic patients during the performance of a verbal fluency task. Br. J. Psychiatry 167, 343–349. doi: 10.1192/bjp.167.3.343
Funahashi, S. (2006). Prefrontal cortex and working memory processes. Neuroscience 139, 251–261. doi: 10.1016/j.neuroscience.2005.07.003
Fuster, J. M. (1973). Unit activity in prefrontal cortex during delayed-response performance: neuronal correlates of transient memory. J. Neurophysiol. 36, 61–78.
Fuster, J. M. (2001). The prefrontal cortex–an update: time is of the essence. Neuron 30, 319–333. doi: 10.1016/S0896-6273(01)00285-9
Gabbott, P. L., and Bacon, S. J. (1996). Local circuit neurons in the medial prefrontal cortex (areas 24a,b,c, 25 and 32) in the monkey: II. Quantitative areal and laminar distributions. J. Comp. Neurol. 364, 609–636. doi: 10.1002/(SICI)1096-9861(19960122)364:4%3C609::AID-CNE2%3E3.3.CO;2-E
Galaburda, A. M., and Pandya, D. N. (1983). The intrinsic architectonic and connectional organization of the superior temporal region of the rhesus monkey. J. Comp. Neurol. 221, 169–184. doi: 10.1002/cne.902210206
Germuska, M., Saha, S., Fiala, J. C., and Barbas, H. (2006). Synaptic distinction of laminar specific prefrontal-temporal pathways in primates. Cereb. Cortex 16, 865–875. doi: 10.1093/cercor/bhj030
Goldman-Rakic, P. S. (1995). Cellular basis of working memory. Neuron 14, 477–485. doi: 10.1016/0896-6273(95)90304-6
Gonchar, Y., and Burkhalter, A. (2003). Distinct GABAergic targets of feedforward and feedback connections between lower and higher areas of rat visual cortex. J. Neurosci. 23, 10904–10912.
Guillery, R. W., and Sherman, S. M. (2002). Thalamic relay functions and their role in corticocortical communication: generalizations from the visual system. Neuron 33, 163–175. doi: 10.1016/S0896-6273(01)00582-7
Hackett, T. A., Stepniewska, I., and Kaas, J. H. (1999). Prefrontal connections of the parabelt auditory cortex in macaque monkeys. Brain Res. 817, 45–58. doi: 10.1016/S0006-8993(98)01182-2
Hashikawa, T., Molinari, M., Rausell, E., and Jones, E. G. (1995). Patchy and laminar terminations of medial geniculate axons in monkey auditory cortex. J. Comp. Neurol. 362, 195–208. doi: 10.1002/cne.903620204
Haykin, S., and Chen, Z. (2005). The cocktail party problem. Neural Comput. 17, 1875–1902. doi: 10.1162/0899766054322964
Hendry, S. H. C., Jones, E. G., Emson, P. C., Lawson, D. E. M., Heizmann, C. W., and Streit, P. (1989). Two classes of cortical GABA neurons defined by differential calcium binding protein immunoreactivities. Exp. Brain Res. 76, 467–472. doi: 10.1007/BF00247904
Honey, G. D., and Fletcher, P. C. (2006). Investigating principles of human brain function underlying working memory: what insights from schizophrenia? Neuroscience 139, 59–71. doi: 10.1016/j.neuroscience.2005.05.036
Jaaskelainen, I. P., Ahveninen, J., Belliveau, J. W., Raij, T., and Sams, M. (2007). Short-term plasticity in auditory cognition. Trends Neurosci. 30, 653–661. doi: 10.1016/j.tins.2007.09.003
Jacobsen, C. F. (1936). Studies of cerebral function in primates: I. The functions of the frontal association area in monkeys. Comp. Psychol. Monogr. 13, 3–60.
Jones, E. G. (1998). A new view of specific and nonspecific thalamocortical connections. Adv. Neurol. 77, 49–71.
Jones, E. G. (2002). Thalamic circuitry and thalamocortical synchrony. Philos. Trans. R. Soc. Lond. B Biol. Sci. 357, 1659–1673. doi: 10.1098/rstb.2002.1168
Kaas, J. H., and Hackett, T. A. (1998). Subdivisions of auditory cortex and levels of processing in primates. Audiol. Neuro-otol. 3, 73–85. doi: 10.1159/000013783
Kawaguchi, Y., Karube, F., and Kubota, Y. (2006). Dendritic branch typing and spine expression patterns in cortical nonpyramidal cells. Cereb. Cortex 16, 696–711. doi: 10.1093/cercor/bhj015
Kawaguchi, Y., and Kubota, Y. (1997). GABAergic cell subtypes and their synaptic connections in rat frontal cortex. Cereb. Cortex 7, 476–486. doi: 10.1093/cercor/7.6.476
Kerns, J. G., Cohen, J. D., Macdonald, A. W. 3rd., Johnson, M. K., Stenger, V. A., and Carter, C. S. (2005). Decreased conflict- and error-related activity in the anterior cingulate cortex in subjects with schizophrenia. Am. J. Psychiatry 162, 1833–1839. doi: 10.1176/appi.ajp.162.10.1833
Kikuchi, Y., Horwitz, B., and Mishkin, M. (2010). Hierarchical auditory processing directed rostrally along the monkey's supratemporal plane. J. Neurosci. 30, 13021–13030. doi: 10.1523/JNEUROSCI.2267-10.2010
Knight, R. T., Staines, W. R., Swick, D., and Chao, L. L. (1999). Prefrontal cortex regulates inhibition and excitation in distributed neural networks. Acta Psychol. (Amst) 101, 159–178. doi: 10.1016/S0001-6918(99)00004-9
Koechlin, E., Basso, G., Pietrini, P., Panzer, S., and Grafman, J. (1999). The role of the anterior prefrontal cortex in human cognition. Nature 399, 148–151. doi: 10.1038/20178
Koechlin, E., and Hyafil, A. (2007). Anterior prefrontal function and the limits of human decision-making. Science 318, 594–598. doi: 10.1126/science.1142995
Kondo, H., Hashikawa, T., Tanaka, K., and Jones, E. G. (1994). Neurochemical gradient along the monkey occipito-temporal cortical pathway. Neuroreport 5, 613–616. doi: 10.1097/00001756-199401000-00020
Krimer, L. S., Zaitsev, A. V., Czanner, G., Kroner, S., Gonzalez-Burgos, G., Povysheva, N. V., et al. (2005). Cluster analysis-based physiological classification and morphological properties of inhibitory neurons in layers 2-3 of monkey dorsolateral prefrontal cortex. J. Neurophysiol. 94, 3009–3022. doi: 10.1152/jn.00156.2005
Kusmierek, P., and Rauschecker, J. P. (2009). Functional specialization of medial auditory belt cortex in the alert rhesus monkey. J. Neurophysiol. 102, 1606–1622. doi: 10.1152/jn.00167.2009
Larkman, A., and Mason, A. (1990). Correlations between morphology and electrophysiology of pyramidal neurons in slices of rat visual cortex. I. Establishment of cell classes. J. Neurosci. 10, 1407–1414.
Larkman, A. U. (1991). Dendritic morphology of pyramidal neurones of the visual cortex of the rat: I. Branching patterns. J. Comp. Neurol. 306, 307–319. doi: 10.1002/cne.903060207
Lee, C. C., and Sherman, S. M. (2008). Synaptic properties of thalamic and intracortical inputs to layer 4 of the first- and higher-order cortical areas in the auditory and somatosensory systems. J. Neurophysiol. 100, 317–326. doi: 10.1152/jn.90391.2008
Lee, C. C., and Sherman, S. M. (2010). Drivers and modulators in the central auditory pathways. Front. Neurosci. 4:79. doi: 10.3389/neuro.01.014.2010
Lee, D., Rushworth, M. F., Walton, M. E., Watanabe, M., and Sakagami, M. (2007). Functional specialization of the primate frontal cortex during decision making. J. Neurosci. 27, 8170–8173. doi: 10.1523/JNEUROSCI.1561-07.2007
Leicht, G., Kirsch, V., Giegling, I., Karch, S., Hantschk, I., Moller, H. J., et al. (2010). Reduced early auditory evoked gamma-band response in patients with schizophrenia. Biol. Psychiatry 67, 224–231. doi: 10.1016/j.biopsych.2009.07.033
Levy, R., and Goldman-Rakic, P. S. (2000). Segregation of working memory functions within the dorsolateral prefrontal cortex. Exp. Brain Res. 133, 23–32. doi: 10.1007/s002210000397
Lynch, J. C., and Tian, J. R. (2005). Cortico-cortical networks and cortico-subcortical loops for the higher control of eye movements. Prog. Brain Res. 151, 461–501. doi: 10.1016/S0079-6123(05)51015-X
Markram, H., Toledo-Rodriguez, M., Wang, Y., Gupta, A., Silberberg, G., and Wu, C. (2004). Interneurons of the neocortical inhibitory system. Nat. Rev. Neurosci. 5, 793–807. doi: 10.1038/nrn1519
McGuire, P. K., Silbersweig, D. A., Wright, I., Murray, R. M., David, A. S., Frackowiak, R. S. J., et al. (1995). Abnormal monitoring of inner speech: a physiological basis for auditory hallucinations. Lancet 346, 596–600. doi: 10.1016/S0140-6736(95)91435-8
Medalla, M., and Barbas, H. (2006). Diversity of laminar connections linking periarcuate and lateral intraparietal areas depends on cortical structure. Eur. J. Neurosci. 23, 161–179. doi: 10.1111/j.1460-9568.2005.04522.x
Medalla, M., and Barbas, H. (2009). Synapses with inhibitory neurons differentiate anterior cingulate from dorsolateral prefrontal pathways associated with cognitive control. Neuron 61, 609–620. doi: 10.1016/j.neuron.2009.01.006
Medalla, M., and Barbas, H. (2010). Anterior cingulate synapses in prefrontal areas 10 and 46 suggest differential influence in cognitive control. J. Neurosci. 30, 16068–16081. doi: 10.1523/JNEUROSCI.1773-10.2010
Medalla, M., and Barbas, H. (2012). The anterior cingulate cortex may enhance inhibition of lateral prefrontal cortex via m2 cholinergic receptors at dual synaptic sites. J. Neurosci. 32, 15611–15625. doi: 10.1523/JNEUROSCI.2339-12.2012
Medalla, M., Lera, P., Feinberg, M., and Barbas, H. (2007). Specificity in inhibitory systems associated with prefrontal pathways to temporal cortex in primates. Cereb. Cortex 17(Suppl. 1), i136–i150. doi: 10.1093/cercor/bhm068
Melchitzky, D. S., Gonzalez-Burgos, G., Barrionuevo, G., and Lewis, D. A. (2001). Synaptic targets of the intrinsic axon collaterals of supragranular pyramidal neurons in monkey prefrontal cortex. J. Comp. Neurol. 430, 209–221. doi: 10.1002/1096-9861(20010205)430:2%3C209::AID-CNE1026%3E3.0.CO;2-%23
Melchitzky, D. S., Sesack, S. R., and Lewis, D. A. (1999). Parvalbumin-immunoreactive axon terminals in macaque monkey and human prefrontal cortex: laminar, regional, and target specificity of type I and type II synapses. J. Comp. Neurol. 408, 11–22. doi: 10.1002/(SICI)1096-9861(19990524)408:1%3C11::AID-CNE2%3E3.3.CO;2-K
Micheyl, C., Carlyon, R. P., Gutschalk, A., Melcher, J. R., Oxenham, A. J., Rauschecker, J. P., et al. (2007). The role of auditory cortex in the formation of auditory streams. Hear. Res. 229, 116–131. doi: 10.1016/j.heares.2007.01.007
Miller, E. K., and Cohen, J. D. (2001). An integrative theory of prefrontal cortex function. Annu. Rev. Neurosci. 24, 167–202. doi: 10.1146/annurev.neuro.24.1.167
Mishkin, M. (1966). “Visual mechanisms beyond the striate cortex,” in Frontiers of Physiological Psychology, ed R. Russel (New York, NY: Academic Press), 93–117.
Mohler, C. W., Goldberg, M. E., and Wurtz, R. H. (1973). Visual receptive fields of frontal eye field neurons. Brain Res. 61, 385–389. doi: 10.1016/0006-8993(73)90543-X
Morel, A., Garraghty, P. E., and Kaas, J. H. (1993). Tonotopic organization, architectonic fields, and connections of auditory cortex in macaque monkeys. J. Comp. Neurol. 335, 437–459. doi: 10.1002/cne.903350312
Mulert, C., Leicht, G., Pogarell, O., Mergl, R., Karch, S., Juckel, G., et al. (2007). Auditory cortex and anterior cingulate cortex sources of the early evoked gamma-band response: relationship to task difficulty and mental effort. Neuropsychologia 45, 2294–2306. doi: 10.1016/j.neuropsychologia.2007.02.020
Muller-Preuss, P., Newman, J. D., and Jurgens, U. (1980). Anatomical and physiological evidence for a relationship between the cingular vocalization area and the auditory cortex in the squirrel monkey. Brain Res. 202, 307–315. doi: 10.1016/0006-8993(80)90143-2
Muller-Preuss, P., and Ploog, D. (1981). Inhibition of auditory cortical neurons during phonation. Brain Res. 215, 61–76. doi: 10.1016/0006-8993(81)90491-1
Murthy, V. N., Schikorski, T., Stevens, C. F., and Zhu, Y. (2001). Inactivity produces increases in neurotransmitter release and synapse size. Neuron 32, 673–682. doi: 10.1016/S0896-6273(01)00500-1
Murthy, V. N., Sejnowski, T. J., and Stevens, C. F. (1997). Heterogeneous release properties of visualized individual hippocampal synapses. Neuron 18, 599–612. doi: 10.1016/S0896-6273(00)80301-3
Negyessy, L., and Goldman-Rakic, P. S. (2005). Morphometric characterization of synapses in the primate prefrontal cortex formed by afferents from the mediodorsal thalamic nucleus. Exp. Brain Res. 164, 148–154. doi: 10.1007/s00221-005-2237-6
Neubert, F. X., Mars, R. B., Thomas, A. G., Sallet, J., and Rushworth, M. F. (2014). Comparison of human ventral frontal cortex areas for cognitive control and language with areas in monkey frontal cortex. Neuron 81, 700–713. doi: 10.1016/j.neuron.2013.11.012
Ng, C. W., Plakke, B., and Poremba, A. (2009). Primate auditory recognition memory performance varies with sound type. Hear. Res. 256, 64–74. doi: 10.1016/j.heares.2009.06.014
Ng, C. W., Plakke, B., and Poremba, A. (2014). Neural correlates of auditory recognition memory in the primate dorsal temporal pole. J. Neurophysiol. 111, 455–469. doi: 10.1152/jn.00401.2012
Niki, H., and Watanabe, M. (1976). Prefrontal unit activity and delayed response: relation to cue location versus direction of response. Brain Res. 105, 79–89. doi: 10.1016/0006-8993(76)90924-0
Pandya, D. N., and Kuypers, H. G. J. M. (1969). Cortico-cortical connections in the rhesus monkey. Brain Res. 13, 13–36. doi: 10.1016/0006-8993(69)90141-3
Pandya, D. N., Seltzer, B., and Barbas, H. (1988). “Input-output organization of the primate cerebral cortex,” in Comparative Primate Biology, Vol. 4: Neurosciences. Eds H. D. Steklis and J. Erwin, (New York, NY: Alan R. Liss), 39–80.
Perrone-Bertolotti, M., Rapin, L., Lachaux, J. P., Baciu, M., and Loevenbruck, H. (2014). What is that little voice inside my head? Inner speech phenomenology, its role in cognitive performance, and its relation to self-monitoring. Behav. Brain Res. 261, 220–239. doi: 10.1016/j.bbr.2013.12.034
Peters, A. (1987). “Number of neurons and synapses in primary visual cortex,” in Cerebral Cortex: Further Aspects of Cortical Function, Including Hippocampus, eds E. G. Jones and A. Peters (New York, NY: Plenum Press), 267–294.
Peters, A., Palay, S. L., and Webster, H. D. (1991). The Fine Structure of The Nervous System. Neurons and Their Supporting Cells. New York, NY: Oxford University Press.
Peters, A., Payne, B. R., and Budd, J. (1994). A numerical analysis of the geniculocortical input to striate cortex in the monkey. Cereb. Cortex 4, 215–229. doi: 10.1093/cercor/4.3.215
Peters, A., and Sethares, C. (1997). The organization of double bouquet cells in monkey striate cortex. J. Neurocytol. 26, 779–797. doi: 10.1023/A:1018518515982
Petrides, M. (2000). The role of the mid-dorsolateral prefrontal cortex in working memory. Exp. Brain Res. 133, 44–54. doi: 10.1007/s002210000399
Petrides, M. (2005). Lateral prefrontal cortex: architectonic and functional organization. Philos. Trans. R. Soc. Lond. B Biol. Sci. 360, 781–795. doi: 10.1098/rstb.2005.1631
Petrides, M., and Pandya, D. N. (1988). Association fiber pathways to the frontal cortex from the superior temporal region in the rhesus monkey. J. Comp. Neurol. 273, 52–66. doi: 10.1002/cne.902730106
Petrides, M., and Pandya, D. N. (2002). Comparative cytoarchitectonic analysis of the human and the macaque ventrolateral prefrontal cortex and corticocortical connection patterns in the monkey. Eur. J. Neurosci. 16, 291–310. doi: 10.1046/j.1460-9568.2001.02090.x
Plakke, B., Ng, C. W., and Poremba, A. (2013). Neural correlates of auditory recognition memory in primate lateral prefrontal cortex. Neuroscience 244, 62–76. doi: 10.1016/j.neuroscience.2013.04.002
Poremba, A., Malloy, M., Saunders, R. C., Carson, R. E., Herscovitch, P., and Mishkin, M. (2004). Species-specific calls evoke asymmetric activity in the monkey's temporal poles. Nature 427, 448–451. doi: 10.1038/nature02268
Rao, S. G., Williams, G. V., and Goldman-Rakic, P. S. (1999). Isodirectional tuning of adjacent interneurons and pyramidal cells during working memory: evidence for microcolumnar organization in PFC. J. Neurophysiol. 81, 1903–1916.
Rauschecker, J. P., Tian, B., Pons, T., and Mishkin, M. (1997). Serial and parallel processing in rhesus monkey auditory cortex. J. Comp. Neurol. 382, 89–103. Doi: 10.1002/(SICI)1096-9861(19970526)382:1%3C89::AID-CNE6%3E3.3.CO;2-Y
Rempel-Clower, N. L., and Barbas, H. (2000). The laminar pattern of connections between prefrontal and anterior temporal cortices in the rhesus monkey is related to cortical structure and function. Cereb. Cortex 10, 851–865. doi: 10.1093/cercor/10.9.851
Robinson, D. A., and Fuchs, A. F. (1969). Eye movements evoked by stimulation of the frontal eye fields. J. Neurophysiol. 32, 637–648.
Romanski, L. M. (2007). Representation and integration of auditory and visual stimuli in the primate ventral lateral prefrontal cortex. Cereb. Cortex 17(Suppl. 1), i61–i69. doi: 10.1093/cercor/bhm099
Romanski, L. M., and Averbeck, B. B. (2009). The primate cortical auditory system and neural representation of conspecific vocalizations. Annu. Rev. Neurosci. 32, 315–346. doi: 10.1146/annurev.neuro.051508.135431
Romanski, L. M., Bates, J. F., and Goldman-Rakic, P. S. (1999a). Auditory belt and parabelt projections to the prefrontal cortex in the rhesus monkey. J. Comp. Neurol. 403, 141–157. doi: 10.1002/(SICI)1096-9861(19990111)403:2%3C141::AID-CNE1%3E3.0.CO;2-V
Romanski, L. M., Tian, B., Fritz, J., Mishkin, M., Goldman-Rakic, P. S., and Rauschecker, J. P. (1999b). Dual streams of auditory afferents target multiple domains in the primate prefrontal cortex. Nat. Neurosci. 2, 1131–1136. doi: 10.1038/16056
Rose, H. J., and Metherate, R. (2005). Auditory thalamocortical transmission is reliable and temporally precise. J. Neurophysiol. 94, 2019–2030. doi: 10.1152/jn.00860.2004
Sallet, J., Mars, R. B., Noonan, M. P., Neubert, F. X., Jbabdi, S., O'Reilly, J. X., et al. (2013). The organization of dorsal frontal cortex in humans and macaques. J. Neurosci. 33, 12255–12274. doi: 10.1523/JNEUROSCI.5108-12.2013
Schall, J. D., and Boucher, L. (2007). Executive control of gaze by the frontal lobes. Cogn. Affect. Behav. Neurosci. 7, 396–412. doi: 10.3758/CABN.7.4.396
Schiller, P. H., and Tehovnik, E. J. (2001). Look and see: how the brain moves your eyes about. Prog. Brain Res. 134, 127–142. doi: 10.1016/S0079-6123(01)34010-4
Scott, B. H., Mishkin, M., and Yin, P. (2012). Monkeys have a limited form of short-term memory in audition. Proc. Natl. Acad. Sci. U.S.A. 109, 12237–12241. doi: 10.1073/pnas.1209685109
Scott, B. H., Mishkin, M., and Yin, P. (2013a). Effect of acoustic similarity on short-term auditory memory in the monkey. Hear. Res. 298, 36–48. doi: 10.1016/j.heares.2013.01.011
Scott, M., Yeung, H. H., Gick, B., and Werker, J. F. (2013b). Inner speech captures the perception of external speech. J. Acoust. Soc. Am. 133, EL286–EL292. doi: 10.1121/1.4794932
Semendeferi, K., Teffer, K., Buxhoeveden, D. P., Park, M. S., Bludau, S., Amunts, K., et al. (2011). Spatial organization of neurons in the frontal pole sets humans apart from great apes. Cereb. Cortex 21, 1485–1497. doi: 10.1093/cercor/bhq191
Sherman, S. M., and Guillery, R. W. (1998). On the actions that one nerve cell can have on another: distinguishing drivers from modulators. Proc. Natl. Acad. Sci. U.S. A. 95, 7121–7126. doi: 10.1073/pnas.95.12.7121
Sherman, S. M., and Guillery, R. W. (2002). The role of the thalamus in the flow of information to the cortex. Philos. Trans. R. Soc. Lond. B Biol. Sci. 357, 1695–1708. doi: 10.1098/rstb.2002.1161
Silberberg, G., Grillner, S., Lebeau, F. E., Maex, R., and Markram, H. (2005). Synaptic pathways in neural microcircuits. Trends Neurosci. 28, 541–551. doi: 10.1016/j.tins.2005.08.004
Smith, R., Keramatian, K., and Christoff, K. (2007). Localizing the rostrolateral prefrontal cortex at the individual level. Neuroimage 36, 1387–1396. doi: 10.1016/j.neuroimage.2007.04.032
Snitz, B. E., Macdonald, A. 3rd., Cohen, J. D., Cho, R. Y., Becker, T., and Carter, C. S. (2005). Lateral and medial hypofrontality in first-episode schizophrenia: functional activity in a medication-naive state and effects of short-term atypical antipsychotic treatment. Am. J. Psychiatry 162, 2322–2329. doi: 10.1176/appi.ajp.162.12.2322
Spruston, N. (2008). Pyramidal neurons: dendritic structure and synaptic integration. Nat. Rev. Neurosci. 9, 206–221. doi: 10.1038/nrn2286
Thomson, A. M., and Deuchars, J. (1997). Synaptic interactions in neocortical local circuits: dual intracellular recordings in vitro. Cereb. Cortex 7, 510–522. doi: 10.1093/cercor/7.6.510
Tong, G., and Jahr, C. E. (1994). Multivesicular release from excitatory synapses of cultured hippocampal neurons. Neuron 12, 51–59. doi: 10.1016/0896-6273(94)90151-1
Trevelyan, A. J., and Watkinson, O. (2005). Does inhibition balance excitation in neocortex? Prog. Biophys. Mol. Biol. 87, 109–143. doi: 10.1016/j.pbiomolbio.2004.06.008
Tsujimoto, S., Genovesio, A., and Wise, S. P. (2010). Evaluating self-generated decisions in frontal pole cortex of monkeys. Nat. Neurosci. 13, 120–126. doi: 10.1038/nn.2453
Vogels, T. P., and Abbott, L. F. (2007). Gating deficits in model networks: a path to schizophrenia? Pharmacopsychiatry 40(Suppl. 1), S73–S77. doi: 10.1055/s-2007-992130
Vogt, B. A., and Barbas, H. (1988). “Structure and connections of the cingulate vocalization region in the rhesus monkey,” in The Physiological Control of Mammalian Vocalization, ed J. D. Newman (New York, NY: Plenum Publ. Corp.), 203–225.
Volk, D. W., and Lewis, D. A. (2002). Impaired prefrontal inhibition in schizophrenia: relevance for cognitive dysfunction. Physiol. Behav. 77, 501–505. doi: 10.1016/S0031-9384(02)00936-8
Wang, X. J., Tegner, J., Constantinidis, C., and Goldman-Rakic, P. S. (2004). Division of labor among distinct subtypes of inhibitory neurons in a cortical microcircuit of working memory. Proc. Natl. Acad. Sci. U.S.A. 101, 1368–1373. doi: 10.1073/pnas.0305337101
Wenzlaff, R. M., and Wegner, D. M. (2000). Thought suppression. Annu. Rev. Psychol. 51, 59–91. doi: 10.1146/annurev.psych.51.1.59
White, E. L. (1989). Cortical Circuits. Synaptic Organization of the Cerebral Cortex. Structure, Function and Theory. Boston, MA: Birkhäuser. doi: 10.1007/978-1-4684-8721-3
Winkler, I., Denham, S. L., and Nelken, I. (2009). Modeling the auditory scene: predictive regularity representations and perceptual objects. Trends Cogn. Sci. 13, 532–540. doi: 10.1016/j.tics.2009.09.003
Zaitsev, A. V., Gonzalez-Burgos, G., Povysheva, N. V., Kroner, S., Lewis, D. A., and Krimer, L. S. (2005). Localization of calcium-binding proteins in physiologically and morphologically characterized interneurons of monkey dorsolateral prefrontal cortex. Cereb. Cortex 15, 1178–1186. doi: 10.1093/cercor/bhh218
Zhu, J. J., and Connors, B. W. (1999). Intrinsic firing patterns and whisker-evoked synaptic responses of neurons in the rat barrel cortex. J. Neurophysiol. 81, 1171–1183.
Keywords: frontopolar cortex, frontal pole, area 10, anterior cingulate cortex, synaptic pathways, inhibitory neurons, laminar connections
Citation: Medalla M and Barbas H (2014) Specialized prefrontal “auditory fields”: organization of primate prefrontal-temporal pathways. Front. Neurosci. 8:77. doi: 10.3389/fnins.2014.00077
Received: 02 February 2014; Paper pending published: 14 February 2014;
Accepted: 27 March 2014; Published online: 16 April 2014.
Edited by:
Monica Munoz-Lopez, University of Castilla-La Mancha, SpainReviewed by:
Ricardo Insausti, University of Castilla-La Mancha, SpainBrian H. Scott, National Institute of Mental Health, USA
Copyright © 2014 Medalla and Barbas. This is an open-access article distributed under the terms of the Creative Commons Attribution License (CC BY). The use, distribution or reproduction in other forums is permitted, provided the original author(s) or licensor are credited and that the original publication in this journal is cited, in accordance with accepted academic practice. No use, distribution or reproduction is permitted which does not comply with these terms.
*Correspondence: Helen Barbas, Neural Systems Laboratory, Boston University, 635 Commonwealth Ave., Room 431, Boston, MA 02215, USA e-mail:YmFyYmFzQGJ1LmVkdQ==