- 1 Department of Cellular and Molecular Medicine, University of Ottawa, Ottawa, ON, Canada
- 2 Center for Neural Dynamics, University of Ottawa, Ottawa, ON, Canada
- 3 Department of Biology, McGill University, Montreal, QC, Canada
Decision making in invertebrates often relies on simple neural circuits composed of only a few identified neurons. The relative simplicity of these circuits makes it possible to identify the key computation and neural properties underlying decisions. In this review, we summarize recent research on the neural basis of ultrasound avoidance in crickets, a response that allows escape from echolocating bats. The key neural property shaping behavioral output is high-frequency bursting of an identified interneuron, AN2, which carries information about ultrasound stimuli from receptor neurons to the brain. AN2’s spike train consists of clusters of spikes – bursts – that may be interspersed with isolated, non-burst spikes. AN2 firing is necessary and sufficient to trigger avoidance steering but only high-rate firing, such as occurs in bursts, evokes this response. AN2 bursts are therefore at the core of the computation involved in deciding whether or not to steer away from ultrasound. Bursts in AN2 are triggered by synaptic input from nearly synchronous bursts in ultrasound receptors. Thus the population response at the very first stage of sensory processing – the auditory receptor – already differentiates the features of the stimulus that will trigger a behavioral response from those that will not. Adaptation, both intrinsic to AN2 and within ultrasound receptors, scales the burst-generating features according to the stimulus statistics, thus filtering out background noise and ensuring that bursts occur selectively in response to salient peaks in ultrasound intensity. Furthermore AN2’s sensitivity to ultrasound varies adaptively with predation pressure, through both developmental and evolutionary mechanisms. We discuss how this key relationship between bursting and the triggering of avoidance behavior is also observed in other invertebrate systems such as the avoidance of looming visual stimuli in locusts or heat avoidance in beetles.
Bursting as a Neural Code
Behavior, including decision making, is an inherently temporal process so it goes without saying that the underlying neural activity is also temporally patterned. The study of the activity patterns of neurons has revealed clear links between spiking patterns and functions of neurons (Gerstner et al., 1997; Rieke, 1997; Decharms and Zador, 2000). As one of the most easily recognizable spiking patterns, bursting has been suggested to play a crucial role in many systems (Connors and Gutnick, 1990; Gabbiani et al., 1996; Martinez-Conde et al., 2002; Marsat and Pollack, 2006; Schwartz et al., 2007a; Lin and Nicolelis, 2008; Marsat et al., 2009). Bursts are clusters of two or more spikes occurring in quick succession separated from other spikes by longer inter-spike intervals (ISI). Several techniques can be used to determine objectively if a cell is bursting and which spikes are parts of bursts (Cocatre-Zilgien and Delcomyn, 1992; Bastian and Nguyenkim, 2001). Such analyses are particularly important to differentiate cells that are simply firing at high rates or responses with clustered spikes caused by a pulsatile stimulus from truly bursting cells in the narrow sense of the term. The simplest methods, based on ISIs histograms or spike-train autocorrelation functions, identify the range of ISIs typical of bursts (Figure 1). As shown in Figure 1, bursting neurons have specific spiking patterns that reflect the presence of a non-linear transformation between input and output (Chacron et al., 2004). We will not discuss the cellular mechanisms underlying bursting as several reviews are available on the subject (Krahe and Gabbiani, 2004; Izhikevich, 2006, 2007).
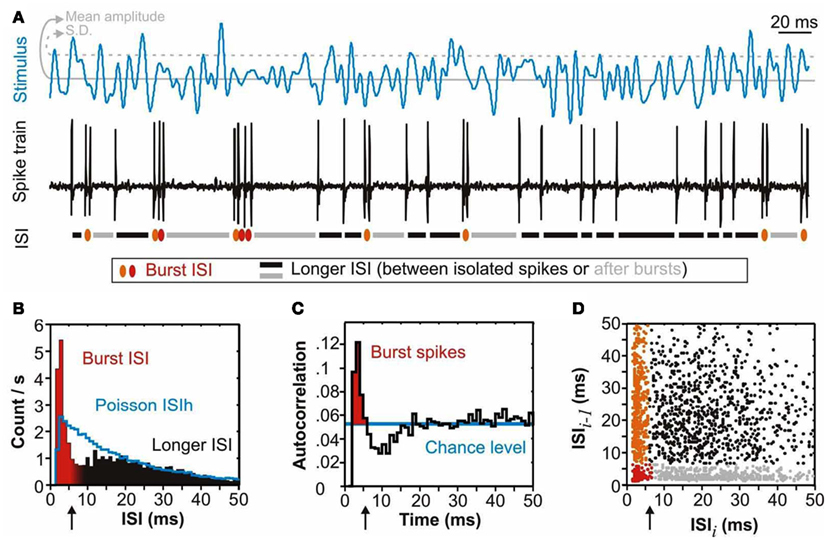
Figure 1. Bursting neural responses. Bursting properties of a neuron can be revealed by stimulating it with a Gaussian input and characterizing its output. We show here an example of a response of the AN2 neuron of crickets which displays a characteristic bursting response to ultrasound stimuli. The lower panels are calculated from 45 s of recording; arrows indicate the threshold of the intra-burst-ISI. (A) Stimulus and response. The stimulus is an ultrasound tone (30 kHz) randomly modulated in amplitude. We display here only the amplitude modulation envelope: a low-pass (<200 Hz) filtered Gaussian with a mean amplitude of 90 dB SPL and an SD of 5 dB. The neuron responds with bursts of spikes to the largest peaks in ultrasound amplitude. The short inter-spike intervals (ISI) of spikes within bursts can clearly be identified (red). The ISI distribution is quantified in (B–D). (B) ISI distribution histogram (ISIh). The ISIhs of bursting neurons have a characteristic bimodal distribution with a peak (shown in red) at short intervals – the intervals of spikes within bursts – and broad tail of longer intervals (black). The bimodal nature of the ISIh – the output – highlights the non-linearity of the input-output transformation since the input – the stimulus – has a Gaussian distribution. A linear transformation would transform a Gaussian input into a Poisson point process. The ISIh of a model Poisson neuron with identical mean firing rate and refractory period is shown in blue. (C) Autocorrelation function. The autocorrelation function gives the probability of having a spike at different time intervals following each spike in the response. A random process would lead to a probability per bin of (Firing rate) × (bin width), in this case 52 Hz × 0.001 s, for all intervals (blue line). The peak at short intervals (red) quantifies the higher-than-chance probability of having spikes separated by short intervals in bursting neurons. (D) ISI return map. The return map plots the ISI that precedes each spike as a function of the ISI that follows it. Several clusters can be observed: a cluster along the y-axis consisting of the first ISIs of each burst (orange), in the bottom left corner the subsequent intra-burst ISIs (red), a cluster along the x-axis for the ISIs that follow bursts (gray) and a more scattered cluster at longer intervals for ISIs between isolated spikes (black). The ISI return map is a way of visually characterizing the spike-train structure and revealing the bursting tendency of the neuron, as non-bursting neurons will not display clusters along the axes at short intervals.
Studying spiking patterns became crucial once it was realized that information was contained not only in the mean firing rate of a neuron but also in the detailed temporal structure of its spike train (Gerstner et al., 1997; Rieke, 1997; Eggermont, 1998; Borst and Theunissen, 1999). The instantaneous firing rate of a neuron can sometimes be linearly related to the input signal but the spiking pattern of bursting neurons relates non-linearly to the input signal (Chacron et al., 2004; Marsat and Pollack, 2004; Lesica et al., 2006). Studies in several sensory systems showed that bursting neurons act as feature detectors, where the occurrence of specific patterns of stimulation results in bursts (Gabbiani et al., 1996; Lesica et al., 2006; Marsat and Pollack, 2006). Sensory bursts are often described as units of neuronal information that exhibit several advantages over single spikes (i.e., spikes that are not part of a burst) from the point of view of information coding and neural dynamics (see last section).
Despite numerous studies on bursting in vertebrate and invertebrate sensory systems and the clear understanding of the type of information carried by bursts to higher brain centers, it is only recently that the links between bursts, the information they carry, and behavioral output were firmly established. We review below recent findings about bursting and ultrasound avoidance in crickets that show how the bursting properties of a few sensory neurons determine the behavioral output.
Ultrasound Avoidance in Crickets
Sound perception and production in crickets co-evolved as a communication tool (Stumpner and Von Helversen, 2001). Male crickets produce songs with relatively low sound frequencies (3–6 kHz) to communicate with conspecifics. Crickets are also sensitive to ultrasound, which allows detection of hunting bats. Ultrasound avoidance is widespread among nocturnally flying insects (Hoy et al., 1989; Hoy, 1994). In crickets, the use of hearing for detecting insectivorous bats is thought to have evolved more recently than conspecific communication; the pre-existing hearing range was most probably widened to encompass the ultrasound of echolocating bats (Hoy, 1992; Yager, 1999). Ultrasound stimuli trigger a stereotyped, short-latency (30–40 ms), reflex-like steering response in tethered flying crickets that, in free flight, would direct them away from the sound source (Moiseff et al., 1978; Nolen and Hoy, 1986). Echolocating bats produce sound pulses that vary in duration (1 to >20 ms) and repetition rates (1–200 Hz) depending on the species of bat and the task they perform (for reviews see Schnitzler and Kalko, 2001; Schnitzler et al., 2003). Typically, bats will increase the repetition rate and decrease the sound-pulse duration when they require better temporal resolution in their auditory representation of space. For example, while searching for insects in open spaces, the bats emit pulses at low rates (a few Hertz) but increase the rate as they approach an insect for capture. In the terminal phases of the bat-insect pursuit, pulses are often only 3–5 ms apart. The crickets must therefore be sensitive to ultrasound stimuli with a wide variety of temporal patterns, and experiments with tethered crickets show that this is indeed the case (Pollack and Elfeghaly, 1993).
Bats and insects are engaged in an evolutionary “arms race” of auditory sensitivity, in which each combatant would benefit from long-distance detection of the other. Bat echolocation is a relatively short-range modality; although echolocation calls can be very intense as they leave the bat (∼120 dB SPL; Surlykke and Kalko, 2008), the amplitude of returning echoes is limited by the small reflective surface of an insect’s body, and by frictional loss to the air. As a result, many bats can detect their prey at an average distance of only ∼5–10 m depending on the species of bat and size of the prey (Kick, 1982; Holderied and Von Helversen, 2003; Surlykke and Kalko, 2008). By contrast, ultrasound sensitivity of crickets is such that they should be able to detect a bat at a distance of 10–18 m (Nolen and Hoy, 1986). The best strategy for an insect that detects a distant bat, then, is to steer away from the sound source to escape detection, and indeed that is what both moths and crickets do in response to ultrasound stimuli of low-to-moderate intensity (Hoy, 1994). Once the bat detects its potential prey, however, a chase may ensue. Video recordings show that such “dog fights” between bats and beetles can last for many seconds, during which the beetle continues to exhibit evasive maneuvers (Simmons, 2005). The neural and behavioral responses during close range interactions are not well defined. We know that, in some insects, very loud sound pulses (>95 dB SPL for crickets) will cause a different kind of evasive maneuver that is non-directional, a strategy that may serve to prevent the bat, which can out-fly the insect, from predicting the trajectory of its prey (Roeder, 1967; Nolen and Hoy, 1986). However, considering that bats typically reduce the intensity of their vocalization as they approach their prey (Schnitzler and Kalko, 2001; Fullard et al., 2003; Schnitzler et al., 2003), it is unknown how commonly these evasive maneuvers are triggered. Also, experiments with playbacks of recorded echolocation pulses to crickets and moths (Fullard et al., 2003, 2005) indicate that the temporal pattern and the frequency of the vocalizations of some bats during the final attack phase might not stimulate the neurons very strongly. These experiments however were done in a fixed spatial configuration. The spatial dynamics of a bat-insect interaction can obviously cause large modulations in the intensity of ultrasound as it reaches each ear beyond those present in the pulse train that the bat produces. The consequence of this spatial dynamic on sensory processing remains unexplored and would be an interesting subject for future studies.
Ultrasound Coding in Crickets
The cricket’s ears are located on the tibiae of the fore legs. Auditory information is carried from the ear to the prothoracic ganglion by auditory receptors, and is then relayed to the brain by ascending interneurons (Figure 2). The population of about 60–70 auditory receptors is composed of distinct types of cells based on their anatomy and tuning to sound frequency (Imaizumi and Pollack, 1999, 2001, 2005). About one-fourth of the receptors respond most strongly to high frequencies, including ultrasound. The remaining three-fourth are tuned to the low frequencies used during conspecific communication. The tuning of receptors to either high or low frequencies forms the basis for categorical perception of sound frequency. This was demonstrated by using an habituation-dishabituation paradigm to show that stimuli with various carrier frequencies were categorized either as attractive or repulsive, with a sharp transition between the two at around 16 kHz (Wyttenbach et al., 1996). At this transition frequency, responses depended on the stimulus temporal pattern; non-cricket-like (2 pulses/s) stimuli consistently elicited avoidance steering, but a calling-song model with 15 kHz carrier frequency typically elicited biphasic responses, in which initial steering away from the sound was replaced after a few seconds by steering toward the sound, suggesting inhibitory interactions between song-recognition and predator-avoidance systems (Pollack et al., 1984).
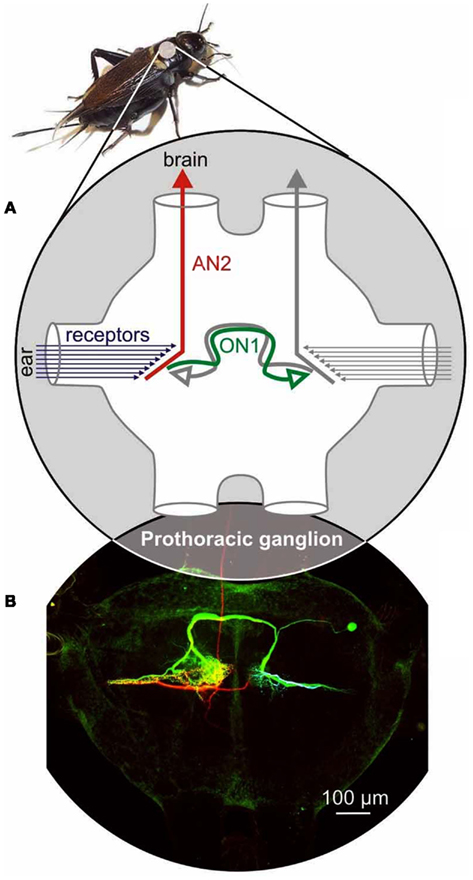
Figure 2. Auditory neurons of the prothorax of crickets. (A) Auditory receptors project from the ears located on the tibiae of the forelegs to the prothoracic ganglion. A population of approximately 15 ultrasound receptors on each side provides inputs to the Ascending Neuron 2 (AN2) which carries the information to the brain, and to the Omega Neuron 1 (ON1), which inhibits the contralateral interneurons (both AN2 and ON1). (B) Morphologies of AN2 (red), ON1 (green), and an ultrasound receptor (blue) filled with fluorescent dyes. Note that while the AN2 and ON1 neurons were imaged together, the receptor has been superimposed post hoc.
Auditory information is processed initially by bilaterally paired, identified interneurons that receive monaural excitatory inputs from receptors (Hennig, 1988; Hardt and Watson, 1994). Two well studied and particularly important bilaterally paired neurons for the processing of ultrasound are Ascending Neuron 2 (AN2) and Omega Neuron 1 (ON1; Figure 2). AN2 is the main conduit by which information about ultrasound reaches the brain, and can thus be seen as a bottleneck in the ultrasound processing pathway. ON1 is a local interneuron; it receives input from the receptors of one ear and provides contralateral inhibition to several interneurons that receive input from the other ear, including AN2 and the contralateral ON1 (Selverston et al., 1985), thereby enhancing bilateral differences used to localize sound.
Several high-frequency-sensitive brain neurons have been described, some of which, based on their anatomical and physiological characteristics, are candidates for receiving input directly from AN2 (Boyan, 1980, 1981; Schildberger, 1984; Brodfuehrer and Hoy, 1990); however paired simultaneous recordings from AN2 and its potential targets have not yet been performed. Such experiments are necessary not only to confirm putative connectivity, but also to study the effects of AN2 bursts on its targets. One type of local brain neuron, which arborizes on both sides of the brain, is excited by ipsilateral sound pulses and inhibited by contralateral pulses and thus could, in principle, act as a bilateral comparator. Such a bilateral comparison could be involved in processing the spatial information of the sound necessary to direct behavior away from the source but nothing is known about this process.
Another group of brain neurons, which are probably at least two synapses removed from AN2, carry the output of brain circuits to lower ganglia where motor responses are generated (Boyan and Williams, 1981; Brodfuhrer and Hoy, 1989; Staudacher, 2001). The most interesting of these, with respect to avoidance responses, is a subset the activity of which is modulated by flight (Brodfuhrer and Hoy, 1989). Negative phonotaxis to ultrasound occurs only when crickets are actively flying (or, much more weakly, while walking: Pollack et al., 1984); quiescent animals do not respond to ultrasound, no matter how intense the stimulus. Thus, information about the sound stimulus and about behavioral state must be integrated somewhere along the processing chain. Flight has no effect on responses of AN2, ruling out early processing as the locus of this integration, but responses of several descending brain neurons are both stronger and more rapid during flight than while animals are quiescent, pointing to the brain as the site where auditory and behavioral-state information are combined. Although responses are enhanced during flight, the neurons do respond even while the animal is quiescent. Indeed, response magnitude of quiescent animals to intense stimuli can be larger than that of flying animals to stimuli that, though less intense, are nevertheless above typical threshold for eliciting negative phonotaxis. Thus, the role of these descending neurons in triggering or controlling flight-steering responses remains unclear.
Nevertheless, key elements of the network generating the ultrasound avoidance behavior are understood. AN2 in particular has a deterministic role in triggering avoidance steering even though it is on the sensory side of the pathway. The causal relationship between AN2’s activity and avoidance steering was first demonstrated by Nolen and Hoy(1984, 1986). They performed intracellular recording of AN2 in a tethered cricket while also monitoring the activity of muscles used for steering during flight (see Figure 3). They showed that hyperpolarizing AN2 abolished the motor response to ultrasound, and that depolarizing AN2 evoked a motor response even though no sound was presented, thereby demonstrating AN2’s necessity and sufficiency. Thus, an interneuron directly post-synaptic to sensory receptors is the decisive trigger for ultrasound avoidance. Most interestingly, one property of AN2 underlies the decision to trigger avoidance steering or not: bursting.
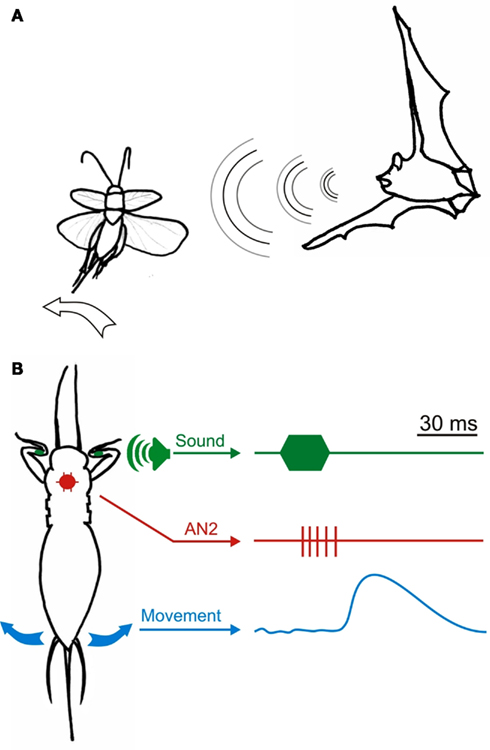
Figure 3. Studying the neural basis of ultrasound avoidance. (A) Ultrasound pulses such as the ones produced by foraging insectivorous bats cause avoidance steering in flying crickets directed away from the sound source. The steering movements consist of changes in the wingbeat pattern but also of movements of the abdomen, hindlegs and antennae in the direction of the intended turn. (B) Tethered crickets continue to produce the motor pattern of flight and can thus be stimulated with ultrasound while the neural and/or motor output is recorded (Nolen and Hoy, 1984, 1986; Marsat and Pollack, 2006, 2010).
The temporal coding properties of AN2 were investigated by Marsat and Pollack(2005, 2006, 2007, 2010) using ultrasound stimuli with random amplitude modulations (Figure 1A), rather than using more naturalistic pulsed stimuli. The use of a “white noise” amplitude envelope is convenient because it allows one to quickly characterize the temporal tuning properties of the cell, and also reveals some of the coding strategies. Two key findings came from comparing the input – the pattern of amplitude modulation to be encoded – to the output spike train. First, AN2 is able to encode a wide range of amplitude modulation rates that encompass those typical of bat echolocation signals (Marsat and Pollack, 2005). Second, the neuron encodes the signal in a non-linear manner. Indeed, the input stimulus has a Gaussian amplitude distribution but the output spike train has a bimodal distribution of ISI; the short ISIs of spikes within bursts form the first peak in the distribution, and the longer ISIs between isolated spikes, or between bursts, form the second, broader, peak (Figure 1). Because ISI is the inverse of instantaneous firing rate, the distribution of the latter is also bimodal. No linear transformation can turn a Gaussian distribution signal into a bimodal distribution, and information-theoretic quantification of AN2’s coding of stimuli with white noise amplitude envelopes showed that the majority of the information is encoded non-linearly (Marsat and Pollack, 2005). Similar to bursting neurons in other sensory systems, AN2 bursts act as feature detectors (Marsat and Pollack, 2006), signaling the occurrence of specific patterns of amplitude modulation. Specifically, AN2 bursts are triggered by peaks in amplitude larger than the standard deviation of the stimulus, in other words, salient peaks in amplitude (Figure 1A). Bursts are very reliable in their timing and thus carry accurate information about the occurrence of these features. These response characteristics presumably allow differentiating background noise from those peaks in ultrasound amplitude that are most likely to originate from a hunting bat.
The bursting characteristics of AN2 have a direct impact on behavior. Nolen and Hoy(1984, 1986) showed that AN2 evokes avoidance steering only if it fires at 180 spikes/s or more. This threshold corresponds to the longest ISIs that occur in AN2’s bursts (∼6 ms; Figure 1; Marsat and Pollack, 2005, 2006). Marsat and Pollack examined explicitly the relationships between bursts or isolated spikes and behavior (see Figure 3). They showed that avoidance steering was triggered only by bursts whereas spikes that were not part of a burst had no detectable influence on avoidance behavior (Marsat and Pollack, 2006).
Although bursts are often considered as all-or-none units, they may vary in the number of spikes they contain or in the intra-burst firing rate. The role of burst structure in temporal coding has been discussed in several systems (Kepecs et al., 2002; Oswald et al., 2007; Butts et al., 2010), because this can carry fine-scale information about the feature that the bursts encode. For example, the intra-burst firing rate of AN2 varies with the duration of the peak in ultrasound amplitude, and number of spikes per burst is correlated with the intensity of the ultrasound peak. Marsat and Pollack (2010) examined the influence of these parameters on behavior. They found that the amplitude of steering responses varied with the number of spikes per burst, but that the intra-burst firing rate had no effect on behavior. These results make the point that even though information about a stimulus can be retrieved by the experimenter by analyzing a specific aspect of the spike train, this does not necessarily mean that the nervous system uses that information.
Together these results show that the bursting properties of AN2 have a key impact on ultrasound avoidance behavior. A stimulus that does not trigger spiking in AN2, or that triggers only non-burst spikes, will not cause ultrasound avoidance. Therefore the bursting mechanism is a key component of the decision mechanism. Although it is not yet known whether AN2’s intrinsic properties contribute to bursting, Sabourin and Pollack (2009) showed that ultrasound receptors also burst and that these bursts have a high probability of causing a burst in AN2.
Neural Dynamic Underlying Ultrasound Avoidance
When neurons are part of a population, as is the case for the 15 or so ultrasound-sensitive receptors, the relationship between the spike trains of the different neurons shapes the population response. Ultrasound receptors tend to burst synchronously, causing a large input to AN2 (Sabourin and Pollack, 2009). Redundancy in response patterns across a population has the well known consequence of limiting the amount of information carried by the population, since different neurons do not carry entirely different information (Sompolinsky et al., 2001; Puchalla et al., 2005; Sabourin and Pollack, 2010). A useful comparison can be established with the population of low-frequency receptors, the spike trains of which show much less redundancy than those of ultrasound receptors. Consequently the accuracy of stimulus coding is greatly improved by pooling the information from many of these low-frequency receptors. It is tempting to relate these two coding strategies to behavior: low-frequency communication sounds need to be encoded with much detail to permit, for example, the fine discrimination of male quality during mate choice, whereas bat-like ultrasound need only be detected to trigger a stereotyped avoidance response.
Interestingly, it is not sufficient that the population response of ultrasound receptors be composed of many synchronous spikes to elicit bursting in AN2; rather, individual receptors must burst (Sabourin and Pollack, 2009). A “distributed burst” of eight spikes (eight single spikes coming in close temporal proximity from eight different receptors) has much less chance of triggering a burst in AN2 than eight spikes produced by four cells, each producing a two-spike burst. These results suggest that synaptic properties contribute to processing the signal, specifically, that facilitation might enhance the response to true bursts, acting as a filter to prevent responses to coincident non-burst spikes in different receptors. This mechanism helps to maintain the separation between burst-encoded information and that encoded by isolated spikes.
Recent studies have also revealed that adaptation in AN2 can lead to filtering out weaker signals (Wimmer et al., 2008; Hildebrandt et al., 2011). Based on experiments combining current injection and pharmacological manipulation Hildebrandt et al. (2011) suggested that both cell-intrinsic currents and pre-synaptic inhibition contribute to adaptation of AN2. The former mechanism has a subtractive effect on the input-output relationship whereas the latter has a divisive effect. Both mechanisms influence the response to ultrasound and presumably help to scale AN2’s response so that only peaks in ultrasound amplitude larger than the recent average amplitude elicit bursts. Another factor contributing to adaptation of AN2 is the very pronounced adaptation of ultrasound-tuned receptors (Sabourin and Pollack, 2009).
Bursts appear as a distinct medium of information transmission and the computations performed by network interactions can operate selectively on the burst-component of the response. This is apparent, as described above, when only bursts in receptors can cause bursts in AN2. It is also apparent in the synaptic interaction between ON1 and AN2. ON1 provides contralateral inhibition to AN2, and its response pattern to ultrasound – including bursting – is very similar to that of AN2. Interestingly, ON1 does not produce bursts when stimulated with low, cricket-like sound frequencies (Marsat and Pollack, 2004, 2005). By using dichotic stimulation and judicious choice of stimuli, it was shown that ON1’s coding properties – in particular its bursting tendency – play an important role in shaping the influence of ON1 on AN2. Specifically, bursts in AN2 are inhibited most efficiently by coincident bursts in ON1 (Marsat and Pollack, 2005, 2007). The role of ON1 is to enhance the bilateral contrast that serves as a basis for sound localization. As a consequence, stimuli that trigger bursts in AN2 and ON1 will be represented with larger bilateral contrast, and thus sound localization will be selectively enhanced for these stimuli. These observations support the idea that neural codes and the architecture of neural computations are mutually determined.
Modulation of Neural Properties
Crickets are most at risk of predation by bats while flying. Although some bats are able to glean prey from the tops of vegetation, this is unlikely to be a problem for crickets, which spend their time on the ground in burrows, under the leaf litter, etc. Not all crickets can fly. In many species, individuals may develop either with stunted wings and weak flight muscles, or with full-length wings and strong muscles; the former cannot fly, and the latter can. As crickets age their flight muscles undergo histolysis, so that even though the wings remain long, the flight muscles can no longer generate sufficient force for flight. Thus, there are three flight-classes in the same species; individuals that could never fly, those that can, and those that could at one time but can no longer. Interestingly, sensitivity to ultrasound varies with flight capability: the minimum sound level required to elicit avoidance steering is lower in flight-capable than in flight-incapable individuals; and this is paralleled by a lower threshold of AN2 for ultrasound stimuli (Pollack and Martins, 2007) and by an increased rate of bursting in AN2 compared with that of flight-incapable crickets (Pollack, unpublished observations). These flight-related changes in sensitivity are specific to ultrasound; neither behavioral threshold – in this case for attraction to a model of the species’ communication signal – nor threshold sound level to stimulate AN2, differs between flight-classes for low-frequency, cricket-like sounds (Pollack and Martins, 2007).
The “decision” to develop with short or long wings may be mediated in part by the titer of Juvenile Hormone (JH) during late larval stages (Zera and Tiebel, 1988), with high JH-level leading to short-winged phenotype. An increase in JH-level during adulthood also triggers wing-muscle histolysis (Shiga et al., 1991). Experiments suggest that JH may also be involved in setting sensitivity to ultrasound. Treatment with a JH analog of late-stage larvae of a species in which all individuals are normally long-winged and flight-capable, results in adults with poor sensitivity to ultrasound. As in wing-dimorphic species, the loss of sensitivity is specific to high sound frequencies (Narbonne and Pollack, 2008). The possible role of JH in the loss of ultrasound sensitivity that accompanies wing-muscle histolysis remains to be tested.
Another factor affecting the risk of predation by bats is the presence of bats themselves. Bat-free islands in French Polynesia have been invaded by a cricket species that also occurs in bat-rich areas, including Australia. Behavioral threshold for ultrasound avoidance, and AN2 threshold for ultrasound stimulation, are both higher for crickets in the bat-free environment (Fullard et al., 2010), suggesting that sensitivity to ultrasound has considerable evolutionary, as well as developmental, plasticity. The fact that this plasticity influences the sensitivity of sensory neurons and is reflected in the behavioral output argues for the importance of these neurons’ properties in the decision process.
Bursts, a Code for Rapid Sensory Processing of Important Signals?
The preceding paragraphs describe the relationship between the bursting properties of the cricket auditory neurons and ultrasound processing. The results summarized in these paragraphs also show that beyond determining the information available to the nervous system, the response properties of the sensory neurons relate to the motor output. Specifically, the encoding of different parts of the ultrasound stimulus is multiplexed onto two channels within the neuron’s response: some features of the stimulus are encoded by bursts and others by isolated spike; only the “burst channel” influences avoidance behavior. Consequently, the neuronal properties that generate bursts in response to specific stimulus features also determine when avoidance steering is triggered and can therefore be considered part of the decision mechanism. Although some aspects of ultrasound avoidance, such as its reliability and short-latency, are reflex-like in nature, the fact that responses occur only following specific patterns of sensory input is indicative that a decision has been made. More specifically, the neural network generating the response must decide whether an ultrasound stimulus is sufficiently intense, relative to recent experience, to represent a potential threat and, if so, in which direction to steer.
The influence of a sensory neuron’s coding properties on behavioral decisions has been demonstrated in many systems and is particularly clear in reflex-like behaviors such as the escape behavior of fish triggered by the activity of Mauthner cells (Korn and Faber, 2005), the crayfish escape response (Edwards et al., 1999), or the avoidance of looming stimuli in locust (Fotowat and Gabbiani, 2011). The influence of sensory neuron properties on decisions has also been examined in much more complex behaviors. For example, the decisions of a monkey asked to perform a discrimination task seem to be correlated with the response patterns of populations of sensory neurons, although the causal nature of the relationship is difficult to establish (Nienborg and Cumming, 2010).
Indeed, a clear, causal link between the bursting properties of sensory neurons and behavior has not yet been demonstrated in other systems. Nevertheless, the central role of burst codes becomes obvious in a growing number of cases. In locusts, the presence of a looming stimulus is detected through sophisticated computation carried out at the level of the sensory neuron LGMD (Fotowat and Gabbiani, 2011) which triggers an escape response (Fotowat et al., 2011). LGMD exhibits intrinsic bursting properties and strong spike-frequency adaptation. LGMD is pre-synaptic to the descending neuron DCMD, which provides the motor command. DCMD mirrors LGMD’s spiking pattern; thus LGMD’s bursting properties are likely to have a crucial influence on behavior. Heat sensitive neurons of the beetle provide another example of a probable link between sensory bursts and behavior in invertebrates (Must et al., 2010). In this case burst timing does not relate to the temporal features of the stimulus; rather, burst rate indicates the presence of dangerously high temperatures that the beetle tries to avoid.
Examples of the relevance of sensory bursts for behavior also abound in vertebrate model systems. Synchronized bursts in a subset of cells in a sensory processing area signal the occurrence of aggressive communication signals in electric fish, whereas in a different subset of cells, bursts code for prey (Gabbiani et al., 1996; Oswald et al., 2004; Marsat et al., 2009; Marsat and Maler, 2010, 2012). In the retina and the lateral geniculate nucleus of mammals, bursts also reliably signal the occurrence of specific behaviorally relevant features of visual inputs such as a sharp contrast or motion reversal (Lesica and Stanley, 2004; Schwartz et al., 2007b). Thus in many model systems and modalities, sensory bursts seem to serve similar purposes: extracting from the sensory environment features that are of particular relevance to the organism and signaling their occurrence reliably to post-synaptic networks.
Burst coding is well suited to this task. Bursts can be seen as self-contained units of information because they capture, with high reliability, the timing and the identity of specific stimulus features, making this information readily available to decoding circuits. This property is particularly advantageous when a signal must trigger a response very quickly, as in escape behaviors. Also, the fact that bursts consist of several closely spaced spikes suggests that they will have strong effects on post-synaptic cells, where a simple threshold mechanism could separate relevant signals encoded by bursts from background noise. Moreover, frequency-dependent mechanisms, such as facilitation, depression, or resonance, can allow post-synaptic cells to respond differently to bursts and to non-burst spikes (Izhikevich et al., 2003). It is known for example that burst-induced facilitation can lead to an increase in the reliability of synaptic transmission (Lisman, 1997; see also: Harvey-Girard et al., 2010; Bol et al., 2011). Therefore bursts can carry reliable information quickly and efficiently to the next stage of processing. On the other hand bursts might be limited in their capacity to carry information in their internal structure about fine-scale variations in the feature that they represent. In particular we note that because intra-burst ISIs are small, a decoder would have to be sensitive to minute differences to detect variations and might thus be more sensitive to noise (Avila-Akerberg and Chacron, 2011). Furthermore, the internal structure of bursts is often determined by neuron-intrinsic burst-generating mechanisms rather than by subtle stimulus features, thus limiting their coding capacity.
In conclusion we suggest that, because of the properties outlined above, bursts are frequently observed in networks involved in behaviors requiring quick stereotyped responses. In crickets, the use of bursts as an indicator of impending danger allows the decision to take evasive action to be made very early in the sensory system, promoting reliable and rapid responses. We argue that the same strategy may be used by other systems as a way to reduce the need for temporally costly decision networks.
Conflict of Interest Statement
The authors declare that the research was conducted in the absence of any commercial or financial relationships that could be construed as a potential conflict of interest.
References
Avila-Akerberg, O., and Chacron, M. (2011). In vivo conditions influence the coding of stimulus features by bursts of action potentials. J. Comput. Neurosci. 31, 369–383.
Bastian, J., and Nguyenkim, J. (2001). Dendritic modulation of burst-like firing in sensory neurons. J. Neurophysiol. 85, 10–22.
Bol, K., Marsat, G., Harvey-Girard, E., Longtin, A., and Maler, L. (2011). Frequency-tuned cerebellar channels and burst-induced ltd lead to the cancellation of redundant sensory inputs. J. Neurosci. 31, 11028–11038.
Borst, A., and Theunissen, F. E. (1999). Information theory and neural coding. Nat. Neurosci. 2, 947–957.
Boyan, G. S. (1980). Auditory neurones in the brain of the cricket Gryllus bimaculatus (De Geer). J. Comp. Physiol. A 140, 81–93.
Boyan, G. S. (1981). Two-tone suppression of an identified auditory neurone in the brain of the cricket Gryllus bimaculatus (De Geer). J. Comp. Physiol. A 144, 117–125.
Boyan, G. S., and Williams, J. L. D. (1981). Descending interneurones in the brain of the cricket. Naturwissenschaft 68, 486–487.
Brodfuehrer, P. D., and Hoy, R. R. (1990). Ultrasound sensitive neurons in the cricket brain. J. Comp. Physiol. A 166, 651–662.
Brodfuhrer, P. D., and Hoy, R. R. (1989). Integration of ultrasound and flight inputs on descending neurons in the cricket brain. J. Exp. Biol. 145, 157–171.
Butts, D. A., Desbordes, G., Weng, C., Jin, J. Z., Alonso, J. M., and Stanley, G. B. (2010). The episodic nature of spike trains in the early visual pathway. J. Neurophysiol. 104, 3371–3387.
Chacron, M. J., Longtin, A., and Maler, L. (2004). To burst or not to burst? J. Comput. Neurosci. 17, 127–136.
Cocatre-Zilgien, J. H., and Delcomyn, F. (1992). Identification of bursts in spike trains. J. Neurosci. Methods 41, 19–30.
Connors, B. W., and Gutnick, M. J. (1990). Intrinsic firing patterns of diverse neocortical neurons. Trends Neurosci. 13, 99–104.
Decharms, R. C., and Zador, A. (2000). Neural representation and the cortical code. Annu. Rev. Neurosci. 23, 613–647.
Edwards, D. H., Heitler, W. J., and Krasne, F. B. (1999). Fifty years of a command neuron: the neurobiology of escape behavior in the crayfish. Trends Neurosci. 22, 153–161.
Fotowat, H., and Gabbiani, F. (2011). Collision detection as a model for sensory-motor integration. Annu. Rev. Neurosci. 34, 1–19.
Fotowat, H., Harrison, R. R., and Gabbiani, F. (2011). Multiplexing of motor information in the discharge of a collision detecting neuron during escape behaviors. Neuron 69, 147–158.
Fullard, J. H., Dawson, J. W., and Jacobs, D. S. (2003). Auditory encoding during the last moment of a moth’s life. J. Exp. Biol. 206, 281–294.
Fullard, J. H., Ratcliffe, J. M., and Guignion, C. (2005). Sensory ecology of predator-prey interactions: responses of the AN2 interneuron in the field cricket, Teleogryllus oceanicus to the echolocation calls of sympatric bats. J. Comp. Physiol. A 191, 605–618.
Fullard, J. H., Ter Hofstede, H. M., Ratcliffe, J. M., Pollack, G. S., Brigidi, G. S., Tinghitella, R. M., and Zuk, M. (2010). Release from bats: genetic distance and sensoribehavioural regression in the Pacific field cricket, Teleogryllus oceanicus. Naturwissenschaften 97, 53–61.
Gabbiani, F., Metzner, W., Wessel, R., and Koch, C. (1996). From stimulus encoding to feature extraction in weakly electric fish. Nature 384, 564–567.
Gerstner, W., Kreiter, A. K., Markram, H., and Herz, A. V. (1997). Neural codes: firing rates and beyond. Proc. Natl. Acad. Sci. U.S.A. 94, 12740–12741.
Hardt, M., and Watson, A. H. D. (1994). Distribution of synapses on two ascending interneurones carrying frequency-specific information in the auditory system of the cricket: evidence for GABAergic inputs. J. Comp. Neurol. 345, 481–495.
Harvey-Girard, E., Lewis, J., and Maler, L. (2010). Burst-induced anti-hebbian depression acts through short-term synaptic dynamics to cancel redundant sensory signals. J. Neurosci. 30, 6152–6169.
Hennig, R. M. (1988). Ascending auditory interneurons in the cricket Teleogryllus commmodus (Walker) – comparative physiology and direct connections with afferents. J. Comp. Physiol. A 163, 135–143.
Hildebrandt, K. J., Benda, J., and Hennig, R. M. (2011). Multiple arithmetic operations in a single neuron: the recruitment of adaptation processes in the cricket auditory pathway depends on sensory context. J. Neurosci. 31, 14142–14150.
Holderied, M. W., and Von Helversen, O. (2003). Echolocation range and wingbeat period match in aerial-hawking bats. Proc. Biol. Sci. 270, 2293–2299.
Hoy, R., Nolen, T., and Brodfuehrer, P. (1989). The neuroethology of acoustic startle and escape in flying insects. J. Exp. Biol. 146, 287–306.
Hoy, R. R. (1992). “The evolution of hearing in insects as an adaptation to predation from bats,” in The Evolutionary Biology of Hearing, eds D. B. Webster, R. R. Fay and A. N. Popper (New York: Springer-Verlag), 115–129.
Hoy, R. R. (1994). “Ultrasound acoustic startle in flying insects: some neuroethological and comparative aspects,” in Neural Basis of Behavioral Adaptations, eds K. Schildberger and N. Elsner (Stuttgart: Gustav Fischer), 227–241.
Imaizumi, K., and Pollack, G. S. (1999). Neural coding of sound frequency by cricket auditory receptors. J. Neurosci. 19, 1508–1516.
Imaizumi, K., and Pollack, G. S. (2001). Neural representation of sound amplitude by functionally different auditory receptors in crickets. J. Acoust. Soc. Am. 109, 1247–1260.
Imaizumi, K., and Pollack, G. S. (2005). Central projections of auditory receptor neurons of crickets. J. Comp. Neurol. 493, 439–447.
Izhikevich, E. M. (2007). Dynamical Systems in Neuroscience: The Geometry of Excitability and Bursting. Cambridge, MA: MIT Press.
Izhikevich, E. M., Desai, N. S., Walcott, E. C., and Hoppensteadt, F. C. (2003). Bursts as a unit of neural information: selective communication via resonance. Trends Neurosci. 26, 161–167.
Kepecs, A., Wang, X. J., and Lisman, J. (2002). Bursting neurons signal input slope. J. Neurosci. 22, 9053–9062.
Kick, S. A. (1982). Target-detection by the echolocating bat. Eptesicus fuscus. J. Comp. Physiol. 145, 431–435.
Korn, H., and Faber, D. S. (2005). The Mauthner cell half a century later: a neurobiological model for decision-making? Neuron 47, 13–28.
Lesica, N. A., and Stanley, G. B. (2004). Encoding of natural scene movies by tonic and burst spikes in the lateral geniculate nucleus. J. Neurosci. 24, 10731–10740.
Lesica, N. A., Weng, C., Jin, J., Yeh, C. I., Alonso, J. M., and Stanley, G. B. (2006). Dynamic encoding of natural luminance sequences by LGN bursts. PLoS Biol. 4, e209. doi:10.1371/journal.pbio.0040209
Lin, S. C., and Nicolelis, M. A. (2008). Neuronal ensemble bursting in the basal forebrain encodes salience irrespective of valence. Neuron 59, 138–149.
Lisman, J. E. (1997). Bursts as a unit of neural information: making unreliable synapses reliable. Trends Neurosci. 20, 38–43.
Marsat, G., and Maler, L. (2010). Neural heterogeneity and efficient population codes for communication signals. J. Neurophysiol. 104, 2543–2555.
Marsat, G., and Maler, L. (2012). Preparing for the unpredictable: adaptive feedback enhances the response to unexpected communication signals. J. Neurophysiol. 107, 1241–1246.
Marsat, G., and Pollack, G. S. (2004). Differential temporal coding of rhythmically diverse acoustic signals by a single interneuron. J. Neurophysiol. 92, 939–948.
Marsat, G., and Pollack, G. S. (2005). Effect of the temporal pattern of contralateral inhibition on sound localization cues. J. Neurosci. 25, 6137–6144.
Marsat, G., and Pollack, G. S. (2006). A behavioral role for feature detection by sensory bursts. J. Neurosci. 26, 10542–10547.
Marsat, G., and Pollack, G. S. (2007). Efficient inhibition of bursts by bursts in the auditory system of crickets. J. Comp. Physiol. A 193, 625–633.
Marsat, G., and Pollack, G. S. (2010). The structure and size of sensory bursts encode stimulus information but only size affects behavior. J. Comp. Physiol. A 196, 315–320.
Marsat, G., Proville, R. D., and Maler, L. (2009). Transient signals trigger synchronous bursts in an identified population of neurons. J. Neurophysiol. 102, 714–723.
Martinez-Conde, S., Macknik, S. L., and Hubel, D. H. (2002). The function of bursts of spikes during visual fixation in the awake primate lateral geniculate nucleus and primary visual cortex. Proc. Natl. Acad. Sci. U.S.A. 99, 13920–13925.
Moiseff, A., Pollack, G. S., and Hoy, R. R. (1978). Steering responses of flying crickets to sound and ultrasound: Mate attraction and predator avoidance. Proc. Natl. Acad. Sci. U.S.A. 75, 4052–4056.
Must, A., Merivee, E., Luik, A., Williams, I., Ploomi, A., and Heidemaa, M. (2010). Spike bursts generated by the thermosensitive (cold) neuron from the antennal campaniform sensilla of the ground beetle Platynus assimilis. J. Insect Physiol. 56, 412–421.
Narbonne, R., and Pollack, G. S. (2008). Developmental control of ultrasound sensitivity by a juvenile hormone analog in crickets (Teleogryllus oceanicus). J. Insect Physiol. 54, 1552–1556.
Nienborg, H., and Cumming, B. (2010). Correlations between the activity of sensory neurons and behavior: how much do they tell us about a neuron’s causality? Curr. Opin. Neurobiol. 20, 376–381.
Nolen, T. G., and Hoy, R. R. (1984). Initiation of behavior by single neurons: the role of behavioral context. Science 226, 992–994.
Nolen, T. G., and Hoy, R. R. (1986). Phonotaxis in flying crickets. I. Attraction to the calling song and avoidance of bat-like ultrasound are discrete behaviors. J. Comp. Physiol. A 159, 423–439.
Oswald, A. M., Chacron, M. J., Doiron, B., Bastian, J., and Maler, L. (2004). Parallel processing of sensory input by bursts and isolated spikes. J. Neurosci. 24, 4351–4362.
Oswald, A. M. M., Doiron, B., and Maler, L. (2007). Interval coding. I. Burst interspike intervals as indicators of stimulus intensity. J. Neurophysiol. 97, 2731–2743.
Pollack, G. S., and Elfeghaly, E. (1993). Calling song recognition in the cricket Teleogryllus oceanicus: comparison of the effects of stimulus intensity and sound spectrum on selectivity for temporal pattern. J. Comp. Physiol. A 171, 759–765.
Pollack, G. S., Huber, F., and Weber, T. (1984). Frequency and temporal-pattern dependent phonotaxis of crickets (Teleogryllus oceanicus) during tethered flight and compensated walking. J. Comp. Physiol. A 154, 13–26.
Pollack, G. S., and Martins, R. (2007). Flight and hearing: ultrasound sensitivity differs between flight-capable and flight-incapable morphs of a wing-dimorphic cricket species. J. Exp. Biol. 210, 3160–3164.
Puchalla, J. L., Schneidman, E., Harris, R. A., and Berry, M. J. (2005). Redundancy in the population code of the retina. Neuron 46, 493–504.
Roeder, K. D. (1967). Turning tendency of moths exposed to ultrasound while in stationary flight. J. Insect Physiol. 13, 873–888.
Sabourin, P., and Pollack, G. S. (2009). Behaviorally relevant burst coding in primary sensory neurons. J. Neurophysiol. 102, 1086–1091.
Sabourin, P., and Pollack, G. S. (2010). Temporal coding by populations of auditory receptor neurons. J. Neurophysiol. 103, 1614–1621.
Schildberger, K. (1984). Temporal selectivity of identified auditory neurons in the cricket brain. J. Comp. Physiol. A 155, 171–185.
Schnitzler, H. U., and Kalko, E. K. V. (2001). Echolocation by insect-eating bats. Bioscience 51, 557–569.
Schnitzler, H. U., Moss, C. F., and Denzinger, A. (2003). From spatial orientation to food acquisition in echolocating bats. Trends Ecol. Evol. 18, 386–394.
Schwartz, G., Harris, R., Shrom, D., and Berry, M. J. (2007a). Detection and prediction of periodic patterns by the retina. Nat. Neurosci. 10, 552–554.
Schwartz, G., Taylor, S., Fisher, C., Harris, R., and Ii, M. J. B. (2007b). Synchronized firing among retinal ganglion cells signals motion reversal. Neuron 55, 958–969.
Selverston, A. I., Kleindienst, H. U., and Huber, F. (1985). Synaptic connectivity between cricket auditory interneurons as studied by selective photoinactivation. J. Neurosci. 5, 1283–1292.
Shiga, S., Kogawauchi, S., Yasuyama, K., and Yamaguchi, T. (1991). Flight behavior and selective degeneration of flight muscles in the adult cricket (Gryllus bimaculatus). J. Exp. Biol. 155, 661–668.
Simmons, J. A. (2005). Big brown bats and June beetles: multiple pursuit strategies in a seasonal acoustic predator-prey system. Acoust. Res. Lett. Online 6, 238–242.
Sompolinsky, H., Yoon, H., Kang, K., and Shamir, M. (2001). Population coding in neuronal systems with correlated noise. Phys. Rev. E 64, 051904.
Staudacher, E. M. (2001). Sensory responses of descending brain neurons in the walking cricket, Gryllus bimaculatus. J. Comp. Physiol. A 187, 1–17.
Stumpner, A., and Von Helversen, D. (2001). Evolution and function of auditory systems in insects. Naturwissenschaften 88, 159–170.
Surlykke, A., and Kalko, E. K. (2008). Echolocating bats cry out loud to detect their prey. PLoS ONE 3, e2036. doi:10.1371/journal.pone.0002036
Wimmer, K., Hildebrandt, K. J., Hennig, R. M., and Obermayer, K. (2008). Adaptation and selective information transmission in the cricket auditory neuron AN2. PLoS Comput. Biol. 4, e1000182. doi:10.1371/journal.pcbi.1000182
Wyttenbach, R. A., May, M. L., and Hoy, R. R. (1996). Categorical perception of sound frequency by crickets. Science 273, 1542–1544.
Yager, D. D. (1999). Structure, development, and evolution of insect auditory systems. Microsc. Res. Tech. 47, 380–400.
Keywords: burst, feature extraction, insect flight, phonotaxis, predator-avoidance, sensory coding
Citation: Marsat G and Pollack GS (2012) Bursting neurons and ultrasound avoidance in crickets. Front. Neurosci. 6:95. doi: 10.3389/fnins.2012.00095
Received: 19 January 2012; Paper pending published: 14 May 2012;
Accepted: 11 June 2012; Published online: 02 July 2012.
Edited by:
Björn Brembs, Freie Universität Berlin, GermanyReviewed by:
Philippe N. Tobler, University of Zurich, SwitzerlandShunsuke Kobayashi, Fukushima Medical University, Japan
Copyright: © 2012 Marsat and Pollack. This is an open-access article distributed under the terms of the Creative Commons Attribution Non Commercial License, which permits non-commercial use, distribution, and reproduction in other forums, provided the original authors and source are credited.
*Correspondence: Gary Marsat, Department of Cellular and Molecular Medicine, University of Ottawa, 451 Smyth Road, Ottawa, Canada, K1H8M5. e-mail: gmarsat@gmail.com