- 1 Department of Animals in Science and Society, Division of Behavioural Neuroscience, Faculty of Veterinary Medicine, Utrecht University, Utrecht, Netherlands
- 2 Rudolf Magnus Institute of Neuroscience, Utrecht, Netherlands
- 3 Department of Neuroscience and Pharmacology, Rudolf Magnus Institute of Neuroscience, University Medical Center Utrecht, Utrecht, Netherlands
- 4 Department of Animals in Science and Society, Division of Animal Welfare and Laboratory Animal Science, Faculty of Veterinary Medicine, Utrecht University, Utrecht, Netherlands
In both humans and rats high levels of anxiety impair decision-making in the Iowa gambling task (IGT) in male subjects. Expression of the immediate early gene c-fos as marker of neural activity in rat studies indicated a role of the medial prefrontal cortex (prelimbic and infralimbic region; mPFC) in mediating the relationship between anxiety and decision-making. To delineate this relationship further and assess the underlying neurobiology in more detail, we inactivated in the present study the mPFC in male rats using a mixture of the GABA-receptor agonists muscimol and baclofen. Rats were exposed to the elevated plus maze (EPM) to measure effects on anxiety and to the rodent version of the IGT (r-IGT). Inactivation led to increased levels of anxiety on the EPM, while not affecting general activity. The effect in the r-IGT (trials 61–120) was dependent on levels of performance prior to inactivation (trial 41–60): inactivation of the mPFC hampered task performance in rats, which already showed a preference for the advantageous option, but not in rats which were still choosing in a random manner. These data suggest that the mPFC becomes more strongly involved as rats have learned task-contingencies, i.e., choose for the best long-term option. Furthermore they suggest, along with the data of our earlier study, that both anxiety and decision-making in rats are mediated through a neural circuitry including at least the mPFC. The data are discussed in relation to recent data of rodent studies on the neural circuitry underlying decision-making.
Introduction
Recently, we (De Visser et al., 2010) and others (Miu et al., 2008; conform Haegler et al., 2010) have shown that anxiety affects decision-making. More specifically, both low and high anxious male subjects as well as high anxious female subjects perform poorly in the Iowa gambling task (IGT; De Visser et al., 2010). The IGT measures decision-making processes by simulating real-life decisions involving reward, punishment, and uncertainty of outcomes. While healthy participants learn to prefer long-term advantageous options associated with immediate moderate rewards over long-term disadvantageous options associated with immediate high rewards (Bechara et al., 1994, 1999), high anxious subjects seem to remain exploratory, while low anxious subjects appear to be risk-taking (De Visser et al., 2010; see also Rivalan et al., 2009). However, the neural underpinnings of the relationship between anxiety and decision-making remain elusive.
A number of brain areas have been implicated in both anxiety and IGT-like decision-making in humans, such as the medial prefrontal cortex (mPFC), dorso-lateral prefrontal cortex, anterior cingulate cortex, and amygdala (e.g., Bechara et al., 1999; Grachev and Apkarian, 2000; Ernst et al., 2002; Bishop et al., 2004; Bolla et al., 2004; Etkin et al., 2004; Brand et al., 2006; Lawrence et al., 2009; Li et al., 2010; Salomons et al., 2010). The anterior cingulate cortex and dorso-lateral prefrontal cortex are specifically involved in a negative feedback circuit of cortical control over limbic areas (Ridderinkhof et al., 2004; Bechara, 2005). The function of this top-down control circuit, that likely controls decision-making on the basis of reward and punishment as assessed in the IGT (Quirk et al., 2000; Miller and Cohen, 2001; Rogers et al., 2004; Davis et al., 2010; St Onge and Floresco, 2010), may be impaired in high anxious individuals (Bishop et al., 2004; Roiser et al., 2009), leading to suboptimal decision-making. In rats, the mPFC has been shown to be involved in unconditioned anxiety (Duncan et al., 1996; Jinks and McGregor, 1998; Salomons et al., 2010) and probability-based decision-making in rats (St Onge and Floresco, 2010). The mPFC in rats has been suggested to share an anatomical and functional homology to the anterior cingulate cortex and dorso-lateral prefrontal cortex in humans (Uylings and van Eden, 1990; Brown and Bowman, 2002; Uylings et al., 2003).
To address the underlying neurobiology of anxiety and decision-making we (De Visser et al., 2011) recently conducted a study in male rats combining the elevated plus maze (EPM) to assess levels of anxiety, a rodent analog of the IGT (Van den Bos et al., 2006b; Homberg et al., 2008; De Visser et al., 2011) to determine decision-making performance, and expression of the immediate early gene c-fos as marker of neural activity in areas implicated in anxiety and decision-making. Overall, these data suggested that in high anxious-poor performing male rats among others the mPFC (prelimbic, PrL and infralimbic, IL areas) is poorly recruited during task-progression leading to suboptimal decision-making. To assess this more specifically, we transiently inactivated in this study the mPFC using a mixture of the GABA-receptor agonists muscimol (GABAA receptor) and baclofen (GABAB receptor) before rats were tested on the EPM and the r-IGT. This mixture has been shown to be effective in transiently inactivating the mPFC (e.g., St Onge and Floresco, 2010). As the mPFC is suggested to become active when rats have changed their behavioral strategy toward choosing the long-term advantageous option in the IGT (Van den Bos et al., 2006a, 2007; De Visser et al., 2010, 2011), we inactivated the mPFC in rats that either still showed exploratory behavior or rats that already showed a preference for the long-term advantageous option in the r-IGT. We predicted that inactivation of the mPFC would increase anxiety on the EPM and lead to suboptimal decision-making in the r-IGT in those rats that already showed a preference for the long-term advantageous option.
Materials and Methods
Subjects
Male Wistar rats (n = 30), 10 weeks of age, were purchased from Harlan (Horst, the Netherlands). They were housed individually in Makrolon type IV cages under a reversed 12 h light/dark cycle (lights off at 7 am). A shelter and paper tissues were provided as cage enrichment. Food and water were freely available except during testing (see below). Room temperature was controlled at 21 ± 2°C with a relative humidity of 60 ± 15%. A radio provided background noise. All experiments were approved by the Animal Ethics Committee of Utrecht University and were conducted in agreement with Dutch laws (Wet op de Dierproeven, 1996) and European regulations (Guideline 86/609/EEC).
Experimental Procedure
After arrival, rats were allowed to habituate to the housing conditions in the animal facility for 2–3 weeks. Cages were cleaned once a week. Rats were handled two to three times a week to familiarize them with the experimenters. After this habituation period, surgery followed. All rats were allowed to recover for at least 10 days before behavioral testing started. During this recovery period, animals were handled daily and habituated to the infusion procedure. Rats were then tested on the EPM. One week later, rats were tested in the rodent IGT (r-IGT) for 2 weeks under mild food restriction. All experiments were carried out during the dark phase of the day–night cycle, between 8.30 am and 5 pm.
Surgery
Rats (380–420 g) were anesthetized using a mixture of fentanyl (0.25 mg/kg, i.p., Fentanyl Bipharma, Hameln Pharmaceuticals GmbH, Hameln, Germany, 0.05 mg/mL fentanyl citrate) and dexmedetomidine (0.15 mg/kg, i.p., Dexdomitor®, Pfizer Animal Health BV, Capelle a/d IJssel, the Netherlands, 1 mg/mL medetomidine hydrochloride). Further induction of anesthesia was carried out when necessary by administration via mask inhalation of isoflurane (IsoFlo®, AST Farma BV, Oudewater, the Netherlands) vaporized in oxygen at concentrations of up to 5%.
Rats were implanted bilaterally with stainless steel guide cannulas (length: 5 mm; 22 ga; Plastics One type C313GRL, Plastics One Inc., Roanoke, VA, USA) using an in-house built stereotaxic model (Bayer Elberfeld Appnr. 159406; Tropon Inv. Nr. 36774). The cannulas were aimed at the prelimbic cortex under a lateral angle of 20° using the following coordinates adapted from the atlas of Paxinos and Watson (2005) to our rats: anteroposterior (AP): 10.46 mm (1.46 from bregma); mediolateral (ML): ±1.7 mm (from midline); dorsoventral (DV): −3.6 (flat skull). The AP coordinates were adjusted when necessary, i.e., when the distance between the interaural line and bregma deviated from the value of the atlas (9 mm). Stylets were inserted into the cannulas and remained in place until the infusions were made.
Elevated Plus Maze
The EPM was made of gray PVC and elevated 75 cm above the floor. The four arms (50 cm × 10 cm) formed a cross with the central platform. A wall (height: 30 cm) of non-transparent material enclosed two arms, located opposite to each other. Each rat was placed on the central platform facing one of the enclosed arms and allowed to freely explore the maze for 5 min. In between trials, the maze was cleaned with warm water and dried thoroughly using clean towels. Behavior was recorded on DVD and scored afterward using Observer 5.0 (Noldus Information Technology, Wageningen, the Netherlands).
Rodent Iowa gambling task
The same apparatus and procedure was used as previously described (Van den Bos et al., 2006b; Homberg et al., 2008; De Visser et al., 2011) with minor modifications, such as the number of trials per day (see below). The r-IGT apparatus was made of wood and consisted of a start box, choice area, and four arms. Before the start of testing, rats were habituated to the apparatus in a 10-min free exploration trial. Two days later, they were mildly food restricted (approximately 95% of free feeding body weight) and tested for a period of 9 days, i.e., a 5-day period and a 4-day period, interspersed by a two test free days (weekend days). Food was freely available on weekend days. A trial started by lifting the slide door of the start box. The rat could freely enter the choice area of the apparatus and choose one of the four arms. The chosen arm was only closed when the rat had entered a choice arm with its full body, including its tail. At the end of the arm, rats could obtain sucrose pellets or quinine-treated sucrose pellets (baited arms; see below) or no pellets at all (empty arms). Each trial had a maximum duration of 6 min. The inter-trial interval was 30 s. The rats received a total of 120 trials: 6 days of 10 trials, and 3 days of 20 trials. Intra-cerebral injections were given on the three sessions of 20 trials (see below). Rewards were 45 mg sucrose pellets (BioServe Inc., Frenchtown, NJ, USA) and punishments were quinine-treated sucrose pellets that were unpalatable but not uneatable. Most rats consumed the quinine-treated pellets once, but left them uneaten on subsequent encounters. Rats that consistently ate the quinine-treated sucrose pellets were excluded from the analysis. Of the four arms in the maze, two were baited and two were empty. The two empty arms were included to measure non-reward related exploration (Van den Bos et al., 2006b; Homberg et al., 2008; De Visser et al., 2011). The two baited arms consisted of a “bad” arm and a “good” arm. In the “bad” arm, the rats received occasional big rewards (three sucrose pellets in 1 out of 10 trials) among frequent punishments (three quinine-treated sucrose pellets in 9 out of 10 trials). In the “good” arm, the rats received frequent small rewards (one sucrose pellet in 8 out of 10 trials) and infrequent punishments (one quinine-treated sucrose pellet in 2 out of 10 trials). This provided the same principle as in the human IGT: an option with a chance of a big reward (three sucrose pellets), but with little long-term success (three sucrose pellets per 10 trials; cf. decks A and B; Bechara et al., 1994) and an option with a chance of a small reward (one sucrose pellet), but with bigger long-term success (eight sucrose pellets per 10 trials; cf. decks C and D). The location of the baited and empty arms, as well as “good” and “bad” arms was counterbalanced across subjects.
Microinfusion procedure
The mPFC was bilaterally inactivated by infusion of a drug mixture containing the GABAA agonist muscimol (MSM; 0.1 nmol, Sigma-Aldrich, St. Louis, MO, USA) and the GABAB agonist baclofen (BAC; 1.0 nmol, Sigma-Aldrich) dissolved in saline and injected in a volume of 1.0 μL per side using a 26-ga injection needle protruding 0.5 mm past the end of the cannulas (Martin and Ghez, 1999; McFarland and Kalivas, 2001). Infusions were done using a device consisting of a 10-μL Hamilton syringe attached to tubing and the injection needle. Either the muscimol/baclofen mixture (MSM/BAC) or saline was injected by hand at a rate of approximately 0.5 μL/min. Rats were injected in a Makrolon type II cage, using a swivel allowing the animals to move freely during the procedure. The needle was left in place for an additional 1 min to allow for diffusion. Hereafter rats were returned to their home cage. After 15–20 min behavioral testing (EPM, r-IGT) started.
Rats were randomly allocated to the experimental groups: control rats received saline, while MSM/BAC rats received the mixture of GABA-agonists. Each rat received a single infusion prior to testing on the EPM. For the r-IGT, all rats were first trained for a total of 60 trials before receiving three daily infusions of either saline or MSM/BAC. After each infusion they received 20 trials, reaching a total of 120 trials by the end of the experiment.
Histology
After completion of behavioral testing rats were decapitated and the brains were quickly removed and frozen in liquid (−80°C) 2-methylbutane which was cooled with dry ice and stored at −80°C. Coronal sections (20 μm) were cut on a cryostat and mounted on Menzel SuperFrost Plus slides (Menzel GmbH & Co, Braunschweig, Germany) and stained with cresyl violet. Cannula placements were verified with reference to the neuro-anatomical atlas of Paxinos and Watson (2005).
Behavioral Measures
EPM measures
Behavior on the EPM was analyzed as in our previous study (De Visser et al., 2011). Based on the data analysis of that study, the following parameters were taken: time spent on the open arm, as a measure of anxiety, and the number of closed arm entries, as a measure of general activity. An arm entry was scored when the animals had at least three paws on the arm.
r-IGT measures
To determine the choice behavior of the rats, the number of visits to the “bad” or disadvantageous arm was calculated as a fraction of the total visits to the two baited arms. To measure choices for unrewarded arms, the number of visits to the empty arms was calculated as a fraction of the total number of trials per block. From trial block 41–60 onward clear differences begin to emerge between “poor performers” and “good performers” (see Figure 2, panel A: De Visser et al., 2011). Therefore, we used a split-median approach to differentiate “good performers” from “poor performers”: subjects below the median were designated as “good performers,” subjects above the median “poor performers.” The performance in trial blocks 61–80, 81–100, and 101–120 was measured as per cent change from the respective base-line values at trial block 41–60. This split-median approach was done separately for empty arms and baited arms.
Responses to encounters with quinine-treated sucrose pellets or sucrose pellets in the advantageous arm were measured as win-stay/lose-shift behavior (see De Visser et al., 2011). As the number of visits to this arm may be low in animals treated with MSM/BAC in the mPFC the data were analyzed in one single trial block, i.e., trial block 61–120. Thus, when rats encountered a sucrose reward, its subsequent choice was scored as a win-stay when it revisited the advantageous arm. When rats encountered a quinine punishment, its subsequent choice was scored as a loose-shift when the rat switched to another arm. Win-stay and lose-shift was calculated as a fraction of the number of encounters with either sucrose pellets (win) or quinine-treated sucrose pellets (loss). Furthermore, the total number of switches between different arms was calculated as a measure of exploratory behavior (De Visser et al., 2011).
Statistical Analysis
All statistical analyses were carried out using SPSS 16.0 for Windows. For the EPM Student t-tests were performed to determine differences between the control and the MSM/BAC group on the time spent on the open arms and the number of closed arm entries. For the r-IGT a two-way analysis of variance (ANOVA) was run, with one factor encompassing treatment (saline versus MSM/BAC) and one factor as repeated measure (trial blocks 61–80, 81–100, and 101–120). This was done for the choices of both empty and baited arms. One sample t-tests were used to determine whether rats improved from base-line (trial block 41–60 = 100%). Student t-tests were used to assess significant differences between treatments (saline versus MSM/BAC) for the number of switches, win-stays, and lose-shifts.
Statistical significance was set at p ≤ 0.05 (two-tailed); p-values ≤ 0.10 (two-tailed) were considered trends (t), NS: non-significant [p > 0.10 (two-tailed)].
Results
General
Five rats (n = 2 MSM/BAC group, n = 3 saline group) were excluded from analysis due to incorrect placement of the cannulas or to not completing the IGT because of problems with cannulas. No rats were excluded for eating quinine pellets. This left n = 25 rats for data analysis.
Injection Sites
Figure 1 shows the location of tip of the cannulas. Injections were directed at the PrL of the mPFC.
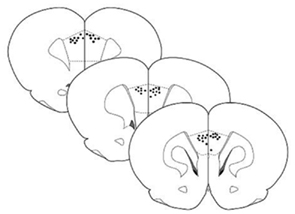
Figure 1. Schematic drawing of coronal sections of rat brain showing the location of cannula tips used for micro infusions into the mPFC. Sections correspond to the atlas of Paxinos and Watson (2005).
Elevated Plus Maze
Due to a technical problem with the injection device (leakage), we lost one batch of rats (n = 7 animals), leaving 18 rats for further testing. Rats in the MSM/BAC group (n = 10) spent less time on the open arms of the EPM (t = 3.508, df = 16, p = 0.003) than rats in the saline group (n = 8; Figure 2). No difference existed regarding the number of closed arm entries (t = −0.712, df = 16, p = 0.487, NS). Thus, inactivation of the mPFC resulted in an increase in anxiety-related behavior without changes in general activity.
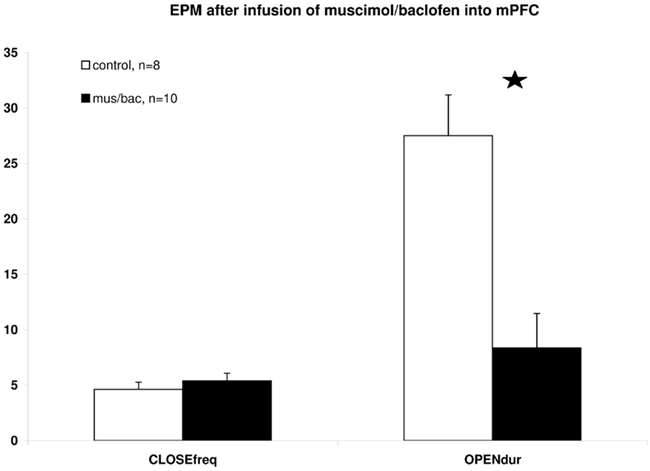
Figure 2. Differences in EPM behavior between mPFC saline injected and MSM/BAC injected rats. CLOSEfreq = the number of closed arm entries, OPENdur = the time spent on one of the open arms as a percentage of total observation time. Shown are means ± SEMs, *p ≤ 0.01 between groups.
r-IGT performance
Good performing rats showed a lower fraction of visits to the disadvantageous arm (mean ± SEM: 0.25 ± 0.03; n = 13) than poor performing rats (0.52 ± 0.03; n = 12) at trial block 41–60. As can be seen in Figure 3A, saline-treated good performing rats improved in choosing the long-term advantageous arm from base-line in trial blocks 61–80, 81–100, and 101–120, while MSM/BAC-treated rats remained nearly at the same level of performance. Statistical analysis revealed a significant treatment effect in trial blocks 61–80, 81–100, and 101–120 [F(1,11) = 5.665, p = 0.04] but no interaction term [trial block * treatment F(2,22) = 0.162, NS]. In contrast, as can be seen in Figure 3B, both saline-treated and MSM/BAC-treated poor performing rats improved in choosing the long-term advantageous arm from base-line in trial blocks 61–80, 81–100, and 101–120. Statistical analysis revealed no significant differences between saline-treated and MSM/BAC-treated rats [trial block * treatment F(2,20) = 0.460, NS; treatment F(1,11) = 0.101, NS].
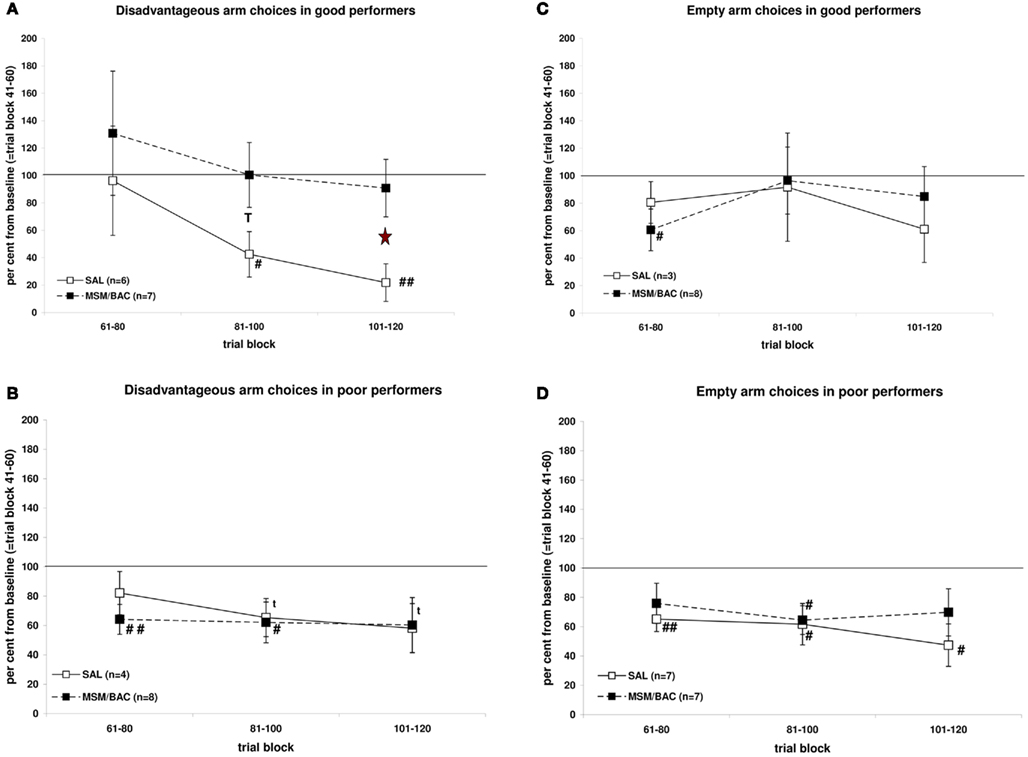
Figure 3. Effects of injections of MSM/BAC or saline into the mPFC on r-IGT performance for the disadvantageous choices (A,B) and empty arm choices (C,D). Shown are means ± SEMs of per cent change from base-line (=trial block 41–60). T: p ≤ 0.10 MSM/BAC versus saline; *: p ≤ 0.05 MSM/BAC versus saline; t: p ≤ 0.10 relative to 100%; #: p ≤ 0.05 relative to 100%; ##: p ≤ 0.01 relative to 100%.
Good performing rats showed a lower fraction of visits to the empty arms (mean ± SEM: 0.24 ± 0.02; n = 11) than poor performing rats (0.46 ± 0.02; n = 14) at trial block 41–60. As can be seen in Figure 3C, neither saline-treated nor MSM/BAC-treated good performing rats improved in choosing baited over empty arms from base-line in trial blocks 61–80, 81–100, and 101–120. Statistical analysis revealed no significant differences between saline-treated or MSM/BAC-treated rats [trial block * treatment F(2,18) = 0.559, NS; treatment F(1,9) = 0.008, NS]. As can be seen in Figure 3D, both saline-treated and MSM/BAC-treated poor performing rats improved in choosing baited arms from base-line in trial blocks 61–80, 81–100, and 101–120. Statistical analysis revealed no significant differences between saline-treated or MSM/BAC -treated rats [trial block * treatment F(2,24) = 0.458, NS; treatment F(1,12) = 0.715, NS].
Tables 1 and 2 show that neither in good performing nor in poor performing rats differences occurred between saline-treated and MSM/BAC-treated rats regarding the number of switches, win-stay behavior or lose-shift behavior.
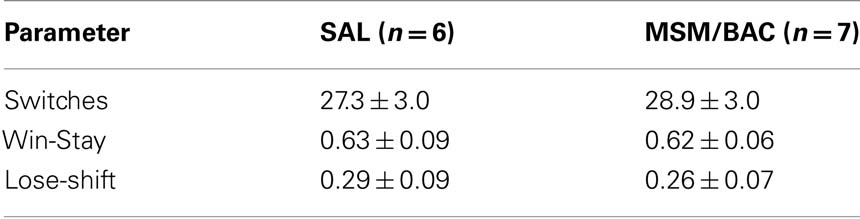
Table 1. Mean (±SEM) values of behavior related parameters for saline-treated and MSM/BAC-treated rats in trial block 61–120 in good performing animals (trial block 41–60).
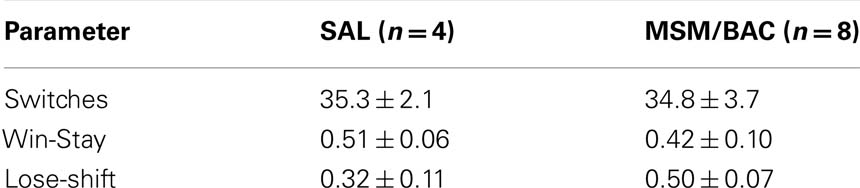
Table 2. Mean (±SEM) values of behavior related parameters for saline-treated and MSM/BAC-treated rats in trial block 61–120 in poor performing animals (trial block 41–60).
Discussion
The present study yielded two main findings. Transient inactivation of the mPFC by injecting the GABA-agonists muscimol and baclofen (1) enhanced anxiety on the EPM, and (2) disrupted improvement of choosing the long-term advantageous option in the second part of the r-IGT in rats that already showed a good performance, but not in rats that showed a poor performance.
Our injection sites were mainly within the prelimbic area of the mPFC. However as we used an injection volume of 1.0 μL we probably also inactivated the underlying infralimbic area. However both structures are implicated in the relationship between anxiety and decision-making as exemplified from our earlier study (De Visser et al., 2011). Accordingly, we will refer to the mPFC in the remainder of the text.
Inactivation of the mPFC decreased the percentage of time spent on the open arms of the EPM, but did not affect the number of closed arm entries. Thus, levels of anxiety were increased after inactivation of the mPFC, but not levels of general activity. This finding is in line with the data of some studies which indicated that inactivation of the mPFC (PrL and/or IL) increased anxiety on the EPM (Silva et al., 1986; Jinks and McGregor, 1998), but not with those of others (Sullivan and Gratton, 2002; Shah and Treit, 2003; Davis et al., 2010; Stern et al., 2010). Although various reasons may underlie these differences between studies including the present study, such as different procedures used (permanent lesions versus transient inactivation, EPM protocols used, handling of animals), we show here that inactivation of the mPFC also hampered task-progression for choosing the best long-term option in the r-IGT in good performing rats as predicted from our earlier study (De Visser et al., 2011). Recent studies using the EPM, r-IGT, and c-fos expression confirmed the relationship between mPFC c-fos activity, r-IGT performance, and levels of anxiety in another strain of rats (Long Evans rats; Van Hasselt et al., in preparation). Overall, these data suggest that at least in our hands mPFC inactivation is associated with increased levels of anxiety.
The fact that we observed a selective effect – no effect on empty arm choices and only an effect within the baited arms in good performing rats – indicates that inactivation of the mPFC did no lead to general effects on working memory or attention, in which the mPFC has been implicated (Seamans et al., 1998; Vertes, 2006; Maddux and Holland, 2011; conform Enemoto et al., 2011). The mPFC was inactivated during the second part of the r-IGT. Analogous to the human IGT (Bechara et al., 1994), the rat task consists of two phases: initially, subjects gradually learn the contingencies of the advantageous and disadvantageous options by exploration, while during the later stages of the task they establish and express a preference for the advantageous option, and show a clear increase in the number of choices for that option, i.e., express task-learning. Indeed, as was suggested in earlier studies using this version of the IGT (Van den Bos et al., 2006b; Homberg et al., 2008; De Visser et al., 2011) the transition from exploration to establishing a long-term advantageous choice occurs during the second part of the task, i.e., after trial block 41–60. We have argued earlier that the mPFC becomes more involved as subjects express their preference for the best long-term option, while cortical structures such as the ventromedial prefrontal cortex/orbitofrontal cortex are more involved during the exploratory phase as subjects learn the overall reward value of the different options (Van den Bos et al., 2006a, 2007; Lawrence et al., 2009; De Visser et al., 2010, 2011). In line with this, the effect of mPFC inactivation on r-IGT performance was dependent on the level of base-line performance as we only observed effects in good performing rats, i.e., rats which showed a clear preference for the advantageous arm. The poor performing rats at trial block 41–60 improve their task performance, reflected by a decrease in the number of disadvantageous choices, irrespective of treatment. The good performing saline-treated rats still show an increased performance, while the MSM/BAC-treated rats did not show this improvement. These findings indicate that the activity of the mPFC may be critical in a window during decision-making where subjects have changed their behavioral strategy toward choosing the long-term advantageous option, i.e., shifting their behavioral strategy from exploration to exploitation.
The present data are therefore in line with a growing body of literature that the mPFC is critically involved in strategy shifting, behavioral flexibility, and goal-directed learning behavior by encoding task-rules (Dias et al., 1996; Ragozzino et al., 1999; Birrell and Brown, 2000; Floresco et al., 2008; Tran-Tu-Yen et al., 2009; Young and Shapiro, 2009; Balleine and O’Doherty, 2010; Sul et al., 2010). The functional integrity of the mPFC may allow for the coupling of the history of the choices of an animal and rewards (computation) as well as behavioral flexibility to generate and implement an optimal decision-making strategy under conditions of uncertainty. The present findings echo those of a recent study in which the mPFC was shown to be involved in proper performance in a probabilistic discounting task, which shares characteristics with the r-IGT (St Onge and Floresco, 2010). Interestingly, involvement of the mPFC in the human IGT has been especially associated with punishment processing (Lin et al., 2008) and risk anticipation (Fukui et al., 2005). In this scenario, the mPFC contributes to cognitive control over emotional influences on behavior, allowing the subject to maintain a long-term perspective and withhold responding to immediate rewards or losses (McClure et al., 2004; Tanaka et al., 2004).
In our earlier study we observed that the good performing – low anxious rats were characterized by a decrease in the overall number of switches, and a strong increase in win-stay behavior, and strong decrease in lose-shift behavior in the advantageous arm, while poor performing – high anxious rats were characterized by an overall high number of switches, and a weak increase in win-stay behavior, and weak decrease in lose-shift behavior in the advantageous arm (De Visser et al., 2011). Accordingly, these data suggested that poor performing – high anxious rats remained exploratory and responsive to for instance immediate losses in contrast to good performing – low anxious rats. Here we show that the effects of mPFC inactivation did not completely mirror our earlier findings: we did not observe an effect on switches, win-stay, and lose-shift strategies. However it should also be noted that differences between good performing – low anxious rats and poor performing – high anxious rats were not solely related to differences in c-fos expression in the mPFC (PrL and IL) but also to differences in the core and shell of the nucleus accumbens (De Visser et al., 2011). More specifically, poor performers showed an increased level of c-fos expression in the nucleus accumbens shell compared to good performers, while increased levels of neural activity in the nucleus accumbens core were found in good performers compared to poor performers. This implies also a crucial role for the nucleus accumbens in regulating both decision-making and anxiety (conform Lopes et al., 2007; da Cunha et al., 2008). To what extent therefore differences in switches, win-stay, and lose-shift behavior, that underlie or are related to differences in overall IGT performance between individuals are specifically associated with differences in the interaction of cortical (mPFC) and subcortical (nucleus accumbens; Yin et al., 2008; see also De Visser et al., 2011) structures remains to be determined.
It should finally be noted that given the small number of rats the results are still preliminary. However, the present data contribute to understanding the role of prefrontal areas in performing the r-IGT and complement recent lesion-studies on the role of prefrontal areas in the r-IGT (Rivalan et al., 2011; Zeeb and Winstanley, 2011). In these studies the mPFC (PrL) was also implicated and suggested to play a role in detecting action–outcome contingency variations, i.e., conditions of uncertainty, leading to an inability to change behavior when lesioned, i.e., perseverative responding (Rivalan et al., 2011).
Conclusion
The data of this study suggest that impaired function of the mPFC may be one factor leading to both high anxiety and poor decision-making.
Conflict of Interest Statement
The authors declare that the research was conducted in the absence of any commercial or financial relationships that could be construed as a potential conflict of interest.
Acknowledgments
We would like to thank Bart Houx for assistance with data analysis, Marla Lavrijsen for support with histology, and Jules Limpens for support with the surgeries. Furthermore, we wish to thank three anonymous reviewers for constructive comments which helped to improve the quality of the manuscript. The research was supported by Utrecht University.
References
Balleine, B. W., and O’Doherty, J. P. (2010). Human and rodent homologies in action control: corticostriatal determinants of goal-directed and habitual action. Neuropsychopharmacology 35, 48–69.
Bechara, A. (2005). Decision making, impulse control and loss of willpower to resist drugs: a neurocognitive perspective. Nat. Neurosci. 8, 1458–1463.
Bechara, A., Damasio, A. R., Damasio, H., and Anderson, S. W. (1994). Insensitivity to future consequences following damage to human prefrontal cortex. Cognition 50, 7–15.
Bechara, A., Damasio, H., Damasio, A. R., and Lee, G. P. (1999). Different contributions of the human amygdala and ventromedial prefrontal cortex to decision-making. J. Neurosci. 19, 5473–5481.
Birrell, J. M., and Brown, V. J. (2000). Medial frontal cortex mediates perceptual attentional set shifting in the rat. J. Neurosci. 20, 4320–4324.
Bishop, S., Duncan, J., Brett, M., and Lawrence, A. D. (2004). Prefrontal cortical function and anxiety: controlling attention to threat-related stimuli. Nat. Neurosci. 7, 184–188.
Bolla, K. I., Eldreth, D. A., Matochik, J. A., and Cadet, J. L. (2004). Sex-related differences in a gambling task and its neurological correlates. Cereb. Cortex 14, 1226–1232.
Brand, M., Labudda, K., and Markowitsch, H. J. (2006). Neuropsychological correlates of decision-making in ambiguous and risky situations. Neural Netw. 6, 1266–1276.
Brown, V. J., and Bowman, E. M. (2002). Rodent models of prefrontal cortical function. Trends Neurosci. 25, 340–343.
da Cunha, I. C., Lopes, A. P., Steffens, S. M., Ferraz, A., Vargas, J. C., de Lima, T. C., Marino Neto, J., Paschoalini, M. A., and Faria, M. S. (2008) , The microinjection of AMPA receptor antagonist into the accumbens shell, but not into the accumbens core, induces anxiolysis in an animal model of anxiety. Behav. Brain Res. 188, 91–99.
Davis, J. F., Loos, M., Di Sebastiano, A. R., Brown, J. L., Lehman, M. N., and Coolen, L. M. (2010). Lesions of the medial prefrontal cortex cause maladaptive sexual behavior in male rats. Biol. Psychiatry 67, 1199–1204.
De Visser, L., Baars, A. M., Lavrijsen, M., Van der Weerd, C. M. M., and van den Bos, R. (2011). Decision-making performance is related to levels of anxiety and differential recruitment of frontostriatal areas in male rats. Neuroscience 184, 97–106.
De Visser, L., van der Knaap, L. J., van de Loo, A. J. A. E., van der Weerd, C. M. M., Ohl, F., and Bos, van den, R. (2010). Trait anxiety affects decision-making differently in healthy men and women: towards gender-specific endophenotypes of anxiety. Neuropsychologia 48, 1598–1606.
Dias, R., Robbins, T. W., and Roberts, A. C. (1996). Dissociation in prefrontal cortex of affective and attentional shifts. Nature 380, 69–72.
Duncan, G. E., Knapp, D. J., and Breese, G. R. (1996). Neuroanatomical characterization of Fos induction in rat behavioral models of anxiety. Brain Res. 713, 79–91.
Enemoto, T., Tse, M. T., and Floresco, S. B. (2011). Reducing prefrontal gamma-aminobutyric acid activity induces cognitive, behavioral, and dopaminergic abnormalities that resemble schizophrenia. Biol. Psychiatry 69, 432–441.
Ernst, M., Bolla, K., Mouratidis, M., Contoreggi, C., Matochik, J. A., Kurian, V., Cadet, J.-L., Kimes, A. S., and London, E. D. (2002). Decision-making in a risk-taking task: a PET study. Neuropsychopharmacology 26, 682–691.
Etkin, A., Klemenhagen, K. C., Dudman, J. T., Rogan, M. T., Hen, R., Kandel, E. R., and Hirsch, J. (2004). Individual differences in trait anxiety predict the response of the basolateral amygdala to unconsciously processed fearful faces. Neuron 44, 1043–1055.
Floresco, S. B., Block, A. E., and Tse, M. T. L. (2008). Inactivation of the medial prefrontal cortex of the rat impairs strategy set-shifting, but not reversal learning, using a novel, automated procedure. Behav. Brain Res. 190, 85–96.
Fukui, H., Murai, T., Fukuyama, H., Hayashi, T., and Hanakawa, T. (2005). Functional activity related to risk anticipation during performance of the Iowa gambling task. Neuroimage 24, 253–259.
Grachev, I. D., and Apkarian, A. V. (2000). Anxiety in healthy humans is associated with orbital frontal chemistry. Mol. Psychiatry 5, 482–488.
Haegler, K., Zernecke, R., Kleemann, A. M., Albrecht, J., Pollatos, O., Brückmann, H., and Wiesmann, M. (2010). No fear no risk! Human risk behavior is affected by chemosensory anxiety signals. Neuropsychologia 48, 3901–3908.
Homberg, J. R., Van den Bos, R., Den Heijer, E., Suer, R., and Cuppen, E. (2008). Serotonin transporter dosages modulates long-term decision-making in rat and human. Neuropharmacology 55, 80–84.
Jinks, A. L., and McGregor, I. S. (1998). Modulation of anxiety-related behaviours following lesions of the prelimbic or infralimbic cortex in the rat. Brain Res. 772, 181–190.
Lawrence, N. S., Jollant, F., O’Daly, O., Zelaya, F., and Phillips, M. L. (2009). Distinct roles of prefrontal cortical subregions in the Iowa gambling task. Cereb. Cortex 19, 1134–1143.
Li, X., Lu, Z. L., D’Argembeau, A., Ng, M., and Bechara, A. (2010). The Iowa gambling task in fMRI images. Hum. Brain Mapp. 31, 410–423.
Lin, C. H., Chiu, Y. C., Cheng, C. M., and Hsieh, J. C. (2008). Brain maps of Iowa gambling task. BMC Neurosci. 9, 72.
Lopes, A. P., da Cunha, I. C., Steffens, S. M., Ferraz, A., Vargas, J. C., de Lima, T. C., Neto, J. M., Faria, M. S., and Paschoalini, M. A. (2007). GABAA and GABAB agonist microinjections into medial accumbens shell increase feeding and induce anxiolysis in an animal model of anxiety. Behav. Brain Res. 184, 142–149.
Maddux, J.-M., and Holland, P. C. (2011). Effects of dorsal or ventral medial prefrontal cortical lesions on five-choice serial reaction time performance in rats. Behav. Brain Res. 221, 63–74.
Martin, J. H., and Ghez, C. (1999). Pharmacological inactivation in the analysis of the central control of movement. J. Neurosci. Methods 86, 145–159.
McClure, S. M., Laibson, D. I., Loewenstein, G., and Cohen, J. D. (2004). Separate neural systems value immediate and delayed monetary rewards. Science 306, 503–507.
McFarland, K., and Kalivas, P. W. (2001). The circuitry mediating cocaine-induced reinstatement of drug-seeking behavior. J. Neurosci. 21, 8655–8663.
Miller, E. K., and Cohen, J. D. (2001). An integrative theory of prefrontal cortex function. Annu. Rev. Neurosci. 24, 167–202.
Miu, A. C., Heilman, R. M., and Houser, D. (2008). Anxiety impairs decision-making: psychophysiological evidence from an Iowa gambling task. Biol. Psychol. 77, 353–358.
Paxinos, G., and Watson, C. (2005). The Rat Brain in Stereotaxic Coordinates, 5th Edn. Amsterdam: Elsevier Academic Press.
Quirk, G., Russo, G. K., Barron, J., and Lebron, K. (2000). The role of ventromedial prefrontal cortex in the recovery of extinguished fear. J. Neurosci. 16, 6225–6231.
Ragozzino, M. E., Detrick, S., and Kesner, R. P. (1999). Involvement of the prelimbic-infralimbic areas of the rodent prefrontal cortex in behavioral flexibility for place and response learning. J. Neurosci. 19, 4585–4594.
Ridderinkhof, K. R., Ulsperger, M., Crone, E. A., and Nieuwenhuis, S. (2004). The role of the medial frontal cortex in cognitive control. Science 306, 443–447.
Rivalan, M., Ahmed, S. H., and Dellu-Hagedorn, F. (2009). Risk-prone individuals prefer the wrong options on a rat version of the Iowa gambling task. Biol. Psychiatry 66, 743–749.
Rivalan, M., Coutureau, E., Fitoussi, A., and Dellu-Hagendorn, F. (2011). Inter-individual decision-making differences in the effects of cingulate, orbitofrontal and prelimbic cortex lesions in a rat gambling task. Front. Behav. Neurosci. 5:22
Rogers, R. D., Ramanani, N., Mackay, C., Wilson, J. L., Jezzard, P., Carter, C. S., and Smith, S. M. (2004). Distinct portions of anterior cingulate cortex and medial prefrontal cortex are activated by reward processing in separable phases of decision-making cognition. Biol. Psychiatry 55, 594–602.
Roiser, J. P., de Martino, B., Tan, G. C., Kumaran, D., Seymour, B., Wood, N. W., and Dolan, R. J. (2009). A genetically mediated bias in decision making driven by failure of amygdala control. J. Neurosci. 29, 5985–5991.
Salomons, A. R., van Luijk, J. A., Reinders, N. R., Kirchhoff, S., Arndt, S. S., and Ohl, F. (2010). Identifying emotional adaptation: behavioural habituation to novelty and immediate early gene expression in two inbred mouse strains. Genes Brain Behav. 9, 1–10.
Seamans, J. K., Floresco, S. B., and Phillips, A. G. (1998). D1 receptor modulation of hippocampal-prefrontal cortical circuits integrating spatial memory with executive functions in the rat. J. Neurosci. 18, 1613–1621.
Shah, A. A., and Treit, D. (2003). Excitotoxic lesions of the medial prefrontal cortex attenuate fear responses in the elevated-plus maze, social interaction and shock probe burying tests. Brain Res. 969, 183–194.
Silva, M. G., Boyle, M. A., Finger, S., Numan, B., Bouzrara, A. A., and Almli, C. R. (1986). Behavioral effects of large and small lesions of the rat medial frontal cortex. Exp. Brain Res. 65, 176–181.
St Onge, J. R., and Floresco, S. B. (2010). Prefrontal cortical contribution to risk-based decision making. Cereb. Cortex 20, 1816–1828.
Stern, C. A. J., Do Monte, F. H. M., Gazarini, L., Carobrez, A. P., and Betroglio, L. J. (2010). Activity in prelimbic cortex is required for adjusting the anxiety response level during the elevated plus maze retest. Neuroscience 170, 214–222.
Sul, J. H., Kim, H., Huh, N., Lee, D., and Jung, M. W. (2010). Distinct roles of rodent orbitofrontal and medial prefrontal cortex in decision-making. Neuron 66, 449–460.
Sullivan, R. M., and Gratton, A. (2002). Behavioral effects of excitotoxic lesions of ventral medial prefrontal cortex in the rat are hemisphere-dependent. Brain Res. 927, 69–79.
Tanaka, S. C., Doya, K., Okada, G., Ueda, K., Okamoto, Y., and Yamawaki, S. (2004). Prediction of immediate and future rewards differentially recruits cortico-basal ganglia loops. Nat. Neurosci. 7, 887–893.
Tran-Tu-Yen, D. A., Marchand, A. R., Di Scala, G., and Coutureau, E. (2009). Transient role of the rat prelimbic cortex in goal-directed behaviour. Eur. J. Neurosci. 30, 464–471.
Uylings, H. B., Groenewegen, H. J., and Kolb, B. (2003). Do rats have a prefrontal cortex? Behav. Brain Res. 146, 3–17.
Uylings, H. B., and van Eden, C. G. (1990). Qualitative and quantitative comparison of the prefrontal cortex in rat and in primates, including humans. Prog. Brain Res. 85, 31–62.
Van den Bos, R., Den Heijer, E., Vlaar, S., and Houx, B. B. (2007). “Exploring gender-differences in decision-making using the Iowa gambling task,” in Psychology of Decision Making in Education, Behavior and High Risk Situations, ed. J. E. (Ellsworth: Nova Science Publications), 207–226.
Van den Bos, R., Houx, B. B., and Spruijt, B. M. (2006a). The effect of reward magnitude differences on choosing disadvantageous decks in the Iowa gambling task. Biol. Psychol. 71, 155–161.
Van den Bos, R., Lasthuis, W., den Heijer, E., van der Harst, J., and Spruijt, B. M. (2006b). Toward a rodent model of the Iowa gambling task. Behav. Res. Methods 38, 470–478.
Vertes, R. P. (2006). Interactions among the medial prefrontal cortex, hippocampus and midline thalamus in emotional and cognitive processing in the rat. Neuroscience 142, 1–20.
Yin, H. H., Ostlund, S. B., and Balleine, B. W. (2008). Reward-guided learning beyond dopamine in the nucleus accumbens: the integrative functions of cortico-basal ganglia networks. Eur. J. Neurosci. 28, 1437–1448.
Young, J. J., and Shapiro, M. L. (2009). Double dissociation and hierarchical organization of strategy switches and reversals in the rat PFC. Behav. Neurosci. 123, 1028–1035.
Keywords: anxiety, decision-making, rats, medial prefrontal cortex
Citation: de Visser L, Baars AM, van ’t Klooster J and van den Bos R (2011) Transient inactivation of the medial prefrontal cortex affects both anxiety and decision-making in male Wistar rats. Front. Neurosci. 5:102. doi: 10.3389/fnins.2011.00102
Received: 09 June 2011; Accepted: 18 August 2011;
Published online: 09 September 2011.
Edited by:
Tobias Kalenscher, Heinrich-Heine University Duesseldorf, GermanyReviewed by:
Toshiya Matsushima, Hokkaido University, JapanEtienne Coutureau, CNRS/Université de Bordeaux, France
Matthijs Feenstra, Netherlands Institute for Neuroscience, Netherlands
Copyright: © 2011 de Visser, Baars, van ’t Klooster and van den Bos. This is an open-access article subject to a non-exclusive license between the authors and Frontiers Media SA, which permits use, distribution and reproduction in other forums, provided the original authors and source are credited and other Frontiers conditions are complied with.
*Correspondence: Ruud van den Bos, Department of Animals in Science and Society, Division of Behavioural Neuroscience, Faculty of Veterinary Medicine, Utrecht University, Yalelaan 2, 3584 CM Utrecht, Netherlands. e-mail: r.vandenbos@uu.nl