- 1Mayo Clinic Graduate School of Biomedical Sciences, Mayo Clinic, Rochester, MN, United States
- 2Department of Neurologic Surgery, Mayo Clinic, Rochester, MN, United States
- 3Department of Neurology and Neurosurgery, University of Colorado Anschutz School of Medicine, Aurora, CO, United States
- 4Department of Physiology and Biomedical Engineering, Mayo Clinic, Rochester, MN, United States
Introduction
Invasive neuromodulation is routinely used to effectively treat the symptoms of movement (Dallapiazza et al., 2019; Limousin and Foltynie, 2019) and psychiatric (Visser-Vandewalle et al., 2022) disorders with high success despite a limited understanding of their mechanisms of action. While the distinct neuroanatomical targets that are stimulated vary depending on the condition being treated and any existing comorbidities, the predominant neuromodulation strategy is to apply a fixed-frequency electrical current to the corresponding neural targets for symptom relief. In the case of movement disorders such as Parkinson's disease (PD), symptom reduction manifests within seconds or minutes following stimulation onset and disappears within a similar time course following the cessation of stimulation (Hristova et al., 2000; Temperli et al., 2003; Ducharme et al., 2011; Pugh, 2019). Maladaptive neuroplasticity, defined as plasticity underlying a disruption in normal neural network function, contributes to numerous neurologic and psychiatric conditions such as chronic pain (Kuner and Flor, 2017), mood disorders (Duman, 2002), movement disorders (McPherson et al., 2015; Li, 2017; Seeman et al., 2017; Peng et al., 2018; Versace et al., 2018; Madadi Asl et al., 2022), tinnitus (Engineer et al., 2011), addiction (Kauer and Malenka, 2007; Kalivas and O'Brien, 2008; Famitafreshi and Karimian, 2019), and depression (Duman et al., 2016). While some invasive neuromodulation approaches treat this underlying neuroplasticity (Creed et al., 2015; McPherson et al., 2015; Seeman et al., 2017; Peng et al., 2018; Versace et al., 2018; Asl et al., 2023), most do not. Thus, the neuromodulation community must consider well-characterized biophysical phenomena such as synaptic plasticity as inspiration when developing next-generation neuromodulation therapies rather than re-applying stimulation paradigms designed for movement disorders to improve treatment outcomes in all conditions, such as psychiatric disorders.
Targeted neuroplasticity as a tool to treat neurologic and psychiatric indications
Targeted neuroplasticity encompasses neuromodulation approaches designed to induce and maintain a long-term influence over nervous system function through long-term potentiation (LTP) or long-term depression (LTD) such that symptom improvement persists after stimulation cessation. Examples of non-invasive neuromodulation approaches that maintain targeted neuroplasticity include transcranial magnetic stimulation (TMS) (Horvath et al., 2010; Valero-Cabré et al., 2017) and vibrotactile coordinated reset (CR) (Syrkin-Nikolau et al., 2018; Pfeifer et al., 2021). These approaches contrast with some conventional invasive neuromodulation approaches such as fixed-frequency deep brain stimulation (DBS), in which acute symptoms are managed only during stimulation (Herrington et al., 2015; Ashkan et al., 2017; Pugh, 2019).
Here, we postulate that targeted neuroplasticity through spatiotemporally patterned stimulation may improve clinical outcomes and enhance invasive therapies such as DBS by reversing maladaptive plasticity rather than treating symptoms. To this end, we propose four considerations for incorporating targeted neuroplasticity into invasive neuromodulation therapies (Figure 1).
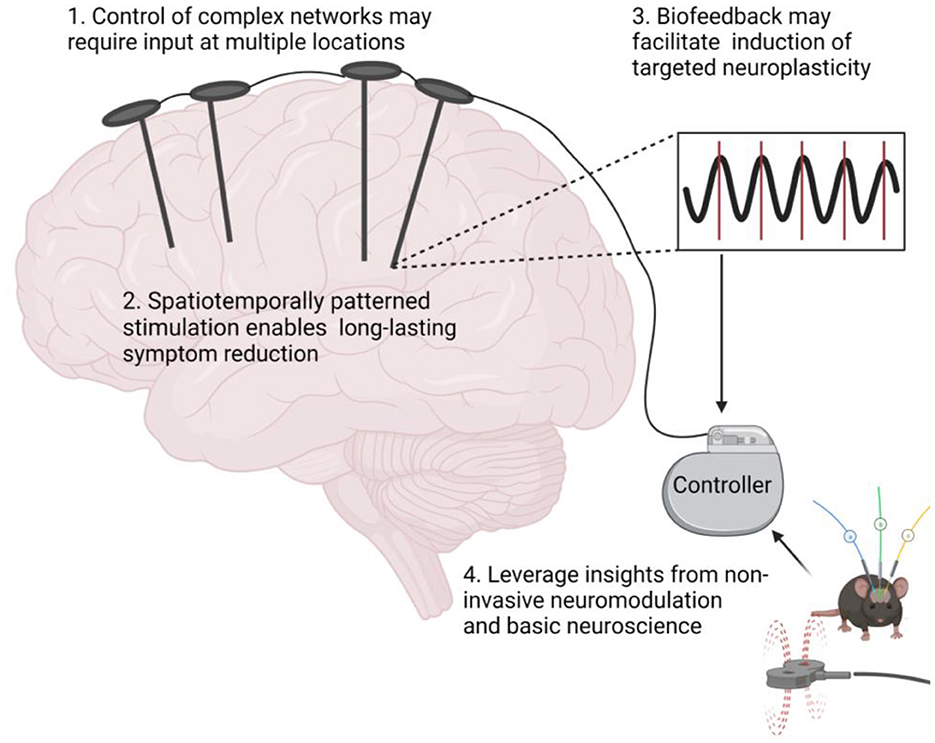
Figure 1. Targeted neuroplasticity approaches for invasive neuromodulation therapies. Figure created with Biorender.com.
Control of complex networks requires spatiotemporally precise stimulation at multiple network locations to improve clinically significant long-term symptom reduction
Neurologic conditions are often associated with neural network dysfunction (Spencer, 2002; Palop et al., 2006; Rosin et al., 2007), and as such, clinically-effective outcomes require timely interventions at multiple network locations (Tu et al., 2018). While initial studies suggested neural activity could be altered from a single node (Gu et al., 2015), the interconnected topology of neural networks complicates selection of a single control node from which to apply stimulation. Furthermore, multiple studies using functional magnetic resonance imaging (fMRI) and other techniques have demonstrated that stimulation at multiple nodes enhances network control (Capotosto et al., 2014; Fox et al., 2014; Pasqualetti et al., 2014; Tu et al., 2018). More importantly, studies have shown that enhanced multi-node network controllability can be achieved via paired stimulation of multiple connected brain regions such as inter-hemispheric dPM-M1 cortex (Lafleur et al., 2016). A clear example of this concept is the use of dual-site DBS placed in the centromedian-parafascicular complex and ventral capsule/ventral striatum to effectively treat motor and non-motor symptoms of severe, medication-resistant Tourette syndrome (Kakusa et al., 2019). Studies thus suggest that multi-location stimulation may improve control of pathological network function underlying symptoms.
Spatiotemporally patterned stimulation enables long-lasting desynchronization of pathological network activity and sustained symptom reduction
Spatiotemporally patterned stimulation has distinct advantages over traditional high-frequency (>100 Hz) stimulation such as facilitation of long-lasting targeted neuroplasticity and desynchronization of pathological network activity leading to symptom reduction. The relative timing between presynaptic and postsynaptic activation influences synaptic strength through a mechanism known as spike-timing dependent plasticity (STDP), is known to profoundly influence brain network function through changes in the direction and magnitude of synaptic strength (Markram et al., 1997; Bi and Poo, 1998; Dan and Poo, 2004; Caporale and Dan, 2008; Brzosko et al., 2019). STDP mechanisms are leveraged by emerging spatiotemporally patterned neuromodulation approaches such as decoupling time-shifted stimulation (Kromer and Tass, 2020; Asl et al., 2023) periodic multichannel stimulation (Kromer and Tass, 2022), and CR (Pfister et al., 2010). These therapies facilitate long-lasting desynchronization of pathologically coherent network activity underlying conditions like Parkinson's Disease (PD) by applying spatiotemporally patterned electric stimulation across subcortical targets such as the STN (Tass, 2003; Tass and Majtanik, 2006; Pfister et al., 2010; Adamchic et al., 2014; Ebert et al., 2014; Wang et al., 2016; Madadi Asl et al., 2018). From a therapeutic standpoint, a major benefit of spatiotemporally patterned therapies is that discontinuous and lower frequency stimulation may reduce the risk of side effects attributable to chronic continuous stimulation (Ferraye et al., 2008; Xie et al., 2012). Furthermore, therapies such as CR demonstrate sustained symptom reduction after stimulation cessation (Tass et al., 2012; Adamchic et al., 2017; Syrkin-Nikolau et al., 2018; Ho et al., 2021; Pfeifer et al., 2021; Wang et al., 2022). Similarly, paired phase-locked stimulation of the infralimbic cortex and basolateral amygdala alters synaptic strength and theta band coherence in a manner that that persists after stimulation cessation (Lo et al., 2020).
Numerous studies achieve targeted neuroplasticity with spatiotemporally patterned stimulation delivered across multiple stimulation modalities. For example, repeated pairing of low frequency (0.1 Hz) DBS with TMS of M1-cortex alters corticostriatal plasticity in humans (Udupa et al., 2016). Similarly, the application of transcranial direct or alternating current stimulation prior to TMS has been shown to alter the effectiveness of the TMS-based plasticity induction protocol (Cosentino et al., 2012; Guerra et al., 2018; Nakazono et al., 2021). Additionally, pairing DBS of midbrain locomotor regions with epidural stimulation of the lumbar spinal cord improves motor function in a rat model of spinal cord injury (Bonizzato et al., 2021). One clinical case report found improved motor function in a patient with multiple system atrophy and predominant parkinsonism when bilateral subthalamic nucleus (STN) DBS and spinal cord stimulation were combined (Li et al., 2022). Taken together, these examples demonstrate that spatiotemporally patterned simulation may enable long-lasting reductions in symptoms and side effects and expand invasive neuromodulation indications while improving power consumption efficiency.
Biofeedback may facilitate induction of targeted neuroplasticity
Closed-loop neuromodulation approaches leverage biofeedback to guide stimulation parameter selection in a wide range of circuitopathies underlying conditions such as epilepsy (Seitz, 2013), PD (Kühn et al., 2009; Weinberger et al., 2012), essential tremor (Thompson et al., 2014), and dystonia (Barow et al., 2014), in which oscillation frequency abnormalities serve as biomarkers that can inform stimulation parameter selection to improve symptom reduction (Thompson et al., 2014). For example, electrophysiological activity recorded during electrographic seizures can trigger DBS to interrupt seizure progression (Thomas and Jobst, 2015; Razavi et al., 2020). Furthermore, studies indicate that phase-aligned stimulation triggered by local field potentials can alter pathological cortical-striatal-pallidal activity and cortico-amygdalar coherence, reducing symptoms of obsessive-compulsive disorder (OCD) (Olsen et al., 2020) and anxiety (Lo et al., 2020), respectively. Stimulation of the ventrolateral (VL) thalamus aligned to patients' limb tremor reduces tremor severity in essential tremor patients through a mechanism involving STDP (Cagnan et al., 2017). Thus, initial exploration of closed-loop stimulation as a mechanism to achieve targeted neuroplasticity promises to be a versatile tool in the treatment of neurologic disease and injury. As such, an expanded investigation of targeted neuroplasticity that incorporates biofeedback measurements may expand this powerful technique into a readily translatable clinical treatment.
Insights from non-invasive neuromodulation and basic neuroscience may inform novel invasive targeted neuroplasticity approaches
Non-invasive neuromodulation therapies such as TMS or focused ultrasound have embraced the targeted neuroplasticity philosophy out of necessity. The immobile nature of non-invasive systems, frequently due to large size and cost of the necessary hardware (Horvath et al., 2010; Anderson et al., 2012; Santarnecchi et al., 2018; Carmi et al., 2019; Mehta et al., 2019; Sabbagh et al., 2020), has necessitated the development of stimulation protocols designed to induce long-term plastic changes in brain function. Consequently, numerous non-invasive stimulation protocols have been designed to facilitate long-term changes in neuroplasticity (Todd et al., 2010; Bunday and Perez, 2012; Jacobs et al., 2012; Urbin et al., 2017; Aftanas et al., 2018; Kozyrev et al., 2018). Despite being limited to engaging cortical targets at a poor spatial specificity on the order of 1,000 mm2 (van de Ruit and Grey, 2016), TMS has succeeded where more precise invasive approaches such as DBS have failed (e.g., treatment-resistant major depressive disorder). It is thus surprising that few studies are seeking to translate FDA-approved non-invasive plasticity-inducing stimulation protocols to invasive techniques such as DBS, which offer a more selective target engagement and, therefore, fewer side effects (Ni et al., 2019).
Adapting classical neuroplasticity induction protocols rooted in basic neuroscience may form the foundation for novel therapies for treatment-resistant clinical indications. An example where DBS has produced less-than-satisfactory results is in the treatment of Alzheimer's disease. A randomized, sham-controlled, double-blinded clinical trial of patients with Alzheimer's disease found continuous high frequency (130 Hz) DBS of the fornix, a brain region implicated in learning and memory (Douet and Chang, 2015), does not improve cognitive function (Lozano et al., 2016). Theta burst microstimulation (5 pulses separated by 200 ms, 100 Hz) is a well-described plasticity induction protocol established ex vivo to cause LTP in neural circuits (Abrahamsson et al., 2016). Theta burst stimulation of the right entorhinal cortex significantly increased performance on pattern separation and memory recall, suggesting utility for the treatment of Alzheimer's disease (Titiz et al., 2017). Moreover, intermittent theta-burst stimulation results in safe and reliable changes in dorsolateral prefrontal cortex electrophysiology (Bentley et al., 2020) and may improve treatment of neurological conditions with historically poor success rates. Emerging optogenetics-inspired DBS protocols consisting of 1 Hz electrical stimulation of the Nucleus Accumbens paired with a D1-Dopamine receptor antagonist reverse behavioral adaptations in a rodent model of addiction (Creed et al., 2015). Similarly, brief bursts of electrical stimulation in the external Globus Pallidus enables control of distinct neuronal subpopulations and produces long-lasting therapeutic benefits in dopamine depleted mice (Spix et al., 2021). Taken together, targeted neuroplasticity induction protocols should be considered as an alternative to high-frequency stimulation to treat neurological conditions in which disease symptomology is predicated on maladaptive neuroplasticity.
Discussion
A strong feature of traditional DBS is its reversibility, which led it to become a favorable alternative to lesioning procedures for treatment of neurologic and psychiatric disorders (Pugh, 2019). While targeted plasticity can be viewed as a shift away from a reversible surgical procedure, it must be noted that traditional DBS, such as STN DBS also causes changes in plasticity (Herrington et al., 2015; Melon et al., 2015; Chassain et al., 2016). However, high-frequency STN DBS does not create long-lasting neuroplastic changes that may support symptom reduction after cessation of stimulation, supporting the reversibility of DBS therapies (Pugh, 2019).
Interventions that provide long-term changes in targeted neuroplasticity through spatiotemporally patterned stimulation offer distinct advantages over traditional high-frequency invasive neuromodulation, chiefly the ability to manipulate underlying disease pathophysiology, persistent symptom improvement after stimulation cessation, reduced power consumption from lower stimulation frequencies, amplitudes, and duty cycles, and improved circuit specificity that minimizes off-target effects. Thus, targeted neuroplasticity approaches may enable expanded avenues for treatment of disorders associated with maladaptive plasticity, such as Tourette's syndrome (Nespoli et al., 2018), OCD (Kreitzer and Malenka, 2008; Maia et al., 2008), Schizophrenia (McCutcheon et al., 2019), PD (Shen et al., 2008; Kravitz et al., 2010; Parker et al., 2018), and Manic Depression (Lee et al., 2018).
Despite the advantages of leveraging targeted neuroplasticity in spatiotemporally patterned invasive neuromodulation therapies, there remain numerous barriers to clinical implementation. When considering the need for multi-nodal circuit control, it is paramount to consider that additional hardware may incur additional surgical risks (Chiong et al., 2018). However, multi-lead DBS procedures are safe and routinely performed (Dallapiazza et al., 2019). Non-invasive options such as TMS can be paired with invasive stimulation to decrease surgical risk of additional implants while enabling additional therapeutic approaches.
There remains a real risk that preclinical findings do not translate between species, particularly to humans (de Oliveira et al., 2021). Consequently, caution must be taken when applying plasticity induction protocols clinically. While application of any novel stimulation paradigm comes with risk, a reasonable starting point for translating a novel neuroplasticity induction protocol to humans is to test plasticity induction protocols in individuals with existing implanted pulse generators, particularly if the system is capable of electrophysiological monitoring. An example of this strategy is evident in the previously mentioned multi-modal approach, where TMS pulses were paired with electrical stimulation of previously indwelling STN DBS electrodes (Udupa et al., 2016). Testing plasticity protocols in such a manner enables feasibility testing in humans without risks inherent in de novo surgical procedures.
Considering the advantages of invasive over non-invasive neuromodulation approaches, we must ask the question, “Why is it that targeted neuroplasticity-inducing protocols such as those used by non-invasive therapies are not widely used invasive neuromodulation therapies?” Perhaps the immediately effective therapeutic benefits of invasive neuromodulation approaches unnecessarily constrain parameter selection. Rather than treat stimulation-induced synaptic plasticity as an obstacle that interferes with long-term efficacy of traditional high-frequency stimulation, stimulation-induced neuroplasticity should be considered as a therapeutic mechanism. This mechanism may be sensitive to numerous parameters, including the type of underlying synaptic plasticity, synaptic transmission delays, the spatiotemporal stimulation pattern, the stimuli shape, and stimulation context. Borrowing inspiration from the protocols of non-invasive neuromodulation like TMS, vibrotactile CR, and basic neuroscience may help improve the clinical outcomes of DBS by creating lasting symptom benefit while broadening the clinical indications that can be treated with invasive therapies.
Clinical invasive neuromodulation approaches have remained largely unchanged since their inception. For example, high-frequency DBS is still the gold standard for treating medically refractory movement disorders. However, neuromodulation is limited in its ability to relieve disease symptoms after stimulation cessation. Re-designing stimulation protocols to address the underlying pathophysiology of disease circuitopathies may improve the current treatment of disorders and expand clinical applications. Integrating this approach into stimulation protocols may require control of complex networks through input at multiple nodes, long-lasting desynchronization of pathologically coherent network activity for long-lasting symptom reduction, and insight from non-invasive neuromodulation and basic neuroscience. Thus, targeted neuroplasticity may pave new paths in neuromodulation, expanding indications and improving disease pathophysiology.
Author contributions
AA and JL conceived of the idea. YC and AA wrote the manuscript. AA, YC, and SH prepared figures. All authors discussed the results and contributed to the final manuscript.
Acknowledgments
We would like to thank Megan L. Gill, P.T., D.P.T. for reviewing and providing feedback on the manuscript.
Conflict of interest
The authors declare that the research was conducted in the absence of any commercial or financial relationships that could be construed as a potential conflict of interest.
Publisher's note
All claims expressed in this article are solely those of the authors and do not necessarily represent those of their affiliated organizations, or those of the publisher, the editors and the reviewers. Any product that may be evaluated in this article, or claim that may be made by its manufacturer, is not guaranteed or endorsed by the publisher.
References
Abrahamsson, T., Lalanne, T., Watt, A. J., and Sjöström, P. J. (2016). Long-term potentiation by theta-burst stimulation using extracellular field potential recordings in acute hippocampal slices. Cold Spring Harb. Protoc. 2016, pdb.prot091298. doi: 10.1101/pdb.prot091298
Adamchic, I., Hauptmann, C., Barnikol, U. B., Pawelczyk, N., Popovych, O., Barnikol, T. T., et al. (2014). Coordinated reset neuromodulation for Parkinson's disease: proof-of-concept study. Mov. Disord. Off. J. Mov. Disord. Soc. 29, 1679–1684. doi: 10.1002/mds.25923
Adamchic, I., Toth, T., Hauptmann, C., Walger, M., Langguth, B., Klingmann, I., et al. (2017). Acute effects and after-effects of acoustic coordinated reset neuromodulation in patients with chronic subjective tinnitus. NeuroImage Clin. 15, 541–558. doi: 10.1016/j.nicl.2017.05.017
Aftanas, L. I., Gevorgyan, M. M., Zhanaeva, S. Y., Dzemidovich, S. S., Kulikova, K. I., Al'perina, E. L., et al. (2018). Therapeutic effects of repetitive transcranial magnetic stimulation (rTMS) on neuroinflammation and neuroplasticity in patients with parkinson's disease: a placebo-controlled study. Bull. Exp. Biol. Med. 165, 195–199. doi: 10.1007/s10517-018-4128-4
Anderson, R. J., Frye, M. A., Abulseoud, O. A., Lee, K. H., McGillivray, J. A., Berk, M., et al. (2012). Deep brain stimulation for treatment-resistant depression: efficacy, safety and mechanisms of action. Neurosci. Biobehav. Rev. 36, 1920–1933. doi: 10.1016/j.neubiorev.2012.06.001
Ashkan, K., Rogers, P., Bergman, H., and Ughratdar, I. (2017). Insights into the mechanisms of deep brain stimulation. Nat. Rev. Neurol. 13, 548–554. doi: 10.1038/nrneurol.2017.105
Asl, M. M., Valizadeh, A., and Tass, P. A. (2023). Decoupling of interacting neuronal populations by time-shifted stimulation through spike-timing-dependent plasticity. PLoS Comput. Biol. 19, e1010853. doi: 10.1371/journal.pcbi.1010853
Barow, E., Neumann, W.-. J, Brücke, C., Huebl, J., Horn, A., et al. (2014). Deep brain stimulation suppresses pallidal low frequency activity in patients with phasic dystonic movements. Brain J. Neurol. 137, 3012–3024. doi: 10.1093/brain/awu258
Bentley, J. N., Irwin, Z. T., Black, S. D., Roach, M. L., Vaden, R. J., Gonzalez, C. L., et al. (2020). Subcortical intermittent theta-burst stimulation (iTBS) increases theta-power in dorsolateral prefrontal cortex (DLPFC). Front. Neurosci. 14, 1–10. doi: 10.3389/fnins.2020.00041
Bi, G., and Poo, M. (1998). Synaptic modifications in cultured hippocampal neurons: dependence on spike timing, synaptic strength, and postsynaptic cell type. J. Neurosci. 18, 10464–10472. doi: 10.1523/JNEUROSCI.18-24-10464.1998
Bonizzato, M., James, N. D., Pidpruzhnykova, G., Pavlova, N., Shkorbatova, P., Baud, L., et al. (2021). Multi-pronged neuromodulation intervention engages the residual motor circuitry to facilitate walking in a rat model of spinal cord injury. Nat. Commun. 12, 1925. doi: 10.1038/s41467-021-22137-9
Brzosko, Z., Mierau, S. B., and Paulsen, O. (2019). Neuromodulation of spike-timing-dependent plasticity: past, present, and future. Neuron. 103, 563–581. doi: 10.1016/j.neuron.2019.05.041
Bunday, K. L., and Perez, M. A. (2012). Motor recovery after spinal cord injury enhanced by strengthening corticospinal synaptic transmission. Curr. Biol. 22, 2355–2361. doi: 10.1016/j.cub.2012.10.046
Cagnan, H., Pedrosa, D., Little, S., Pogosyan, A., Cheeran, B., Aziz, T., et al. (2017). Stimulating at the right time: phase-specific deep brain stimulation. Brain. 140, 132–145. doi: 10.1093/brain/aww286
Caporale, N., and Dan, Y. (2008). Spike timing-dependent plasticity: a Hebbian learning rule. Annu. Rev. Neurosci. 31, 25–46. doi: 10.1146/annurev.neuro.31.060407.125639
Capotosto, P., Babiloni, C., Romani, G. L., and Corbetta, M. (2014). Resting-state modulation of α rhythms by interference with angular gyrus activity. J. Cogn. Neurosci. 26, 107–119. doi: 10.1162/jocn_a_00460
Carmi, L., Tendler, A., Bystritsky, A., Hollander, E., Blumberger, D. M., Daskalakis, J., et al. (2019). Efficacy and safety of deep transcranial magnetic stimulation for obsessive-compulsive disorder: a prospective multicenter randomized double-blind placebo-controlled trial. Am. J. Psychiatry. 176, 931–938. doi: 10.1176/appi.ajp.2019.18101180
Chassain, C., Melon, C., Salin, P., Vitale, F., Couraud, S., Durif, F., et al. (2016). Metabolic, synaptic and behavioral impact of 5-week chronic deep brain stimulation in hemiparkinsonian rats. J. Neurochem. 136, 1004–1016. doi: 10.1111/jnc.13438
Chiong, W., Leonard, M. K., and Chang, E. F. (2018). Neurosurgical patients as human research subjects: ethical considerations in intracranial electrophysiology research. Neurosurgery. 83, 29–37. doi: 10.1093/neuros/nyx361
Cosentino, G., Fierro, B., Paladino, P., Talamanca, S., Vigneri, S., Palermo, A., et al. (2012). Transcranial direct current stimulation preconditioning modulates the effect of high-frequency repetitive transcranial magnetic stimulation in the human motor cortex. Eur. J. Neurosci. 35, 119–124. doi: 10.1111/j.1460-9568.2011.07939.x
Creed, M., Pascoli, V. J., and Lüscher, C. (2015). Refining deep brain stimulation to emulate optogenetic treatment of synaptic pathology. Science. 347, 659–664. doi: 10.1126/science.1260776
Dallapiazza, R. F., Lee, D. J., De Vloo, P., Fomenko, A., Hamani, C., Hodaie, M., et al. (2019). Outcomes from stereotactic surgery for essential tremor. J. Neurol. Neurosurg. Psychiatry. 90, 474–482. doi: 10.1136/jnnp-2018-318240
Dan, Y., and Poo, M. (2004). Spike timing-dependent plasticity of neural circuits. Neuron. 44, 23–30. doi: 10.1016/j.neuron.2004.09.007
de Oliveira, A. R., Reimer, A. E., Simandl, G. J., Nagrale, S. S., and Widge, A. S. (2021). Lost in translation: no effect of repeated optogenetic cortico-striatal stimulation on compulsivity in rats. Transl. Psychiatry. 11, 315. doi: 10.1038/s41398-021-01448-x
Douet, V., and Chang, L. (2015). Fornix as an imaging marker for episodic memory deficits in healthy aging and in various neurological disorders. Front. Aging Neurosci. 6, 1–19. doi: 10.3389/fnagi.2014.00343
Ducharme, S., Flaherty, A. W., Seiner, S. J., Dougherty, D. D., and Morales, O. G. (2011). Temporary interruption of deep brain stimulation for parkinson's disease during outpatient electroconvulsive therapy for major depression: a novel treatment strategy. J. Neuropsychiatry Clin. Neurosci. 23, 194–197. doi: 10.1176/jnp.23.2.jnp194
Duman, R. S. (2002). Synaptic plasticity and mood disorders. Mol. Psychiatry. 7, S29–S34. doi: 10.1038/sj.mp.4001016
Duman, R. S., Aghajanian, G. K., Sanacora, G., and Krystal, J. H. (2016). Synaptic plasticity and depression: new insights from stress and rapid-acting antidepressants. Nat. Med. 22, 238–249. doi: 10.1038/nm.4050
Ebert, M., Hauptmann, C., and Tass, P. A. (2014). Coordinated reset stimulation in a large-scale model of the STN-GPe circuit. Front. Comput. Neurosci. 8, 154. doi: 10.3389/fncom.2014.00154
Engineer, N. D., Riley, J. R., Seale, J. D., Vrana, W. A., Shetake, J. A., Sudanagunta, S. P., et al. (2011). Reversing pathological neural activity using targeted plasticity. Nature. 470, 101–104. doi: 10.1038/nature09656
Famitafreshi, H., and Karimian, M. (2019). Neuroplasticity-targeted therapy alleviates severe addiction. 9, 2436–2443. Available online at: https://www.jneuropsychiatry.org/peer-review/neuroplasticitytargeted-therapy-alleviates-severe-addiction-13112.html
Ferraye, M. U., Debû, B., Fraix, V., Xie-Brustolin, J., Chabardès, S., Krack, P., et al. (2008). Effects of subthalamic nucleus stimulation and levodopa on freezing of gait in Parkinson disease. Neurology. 70, 1431–1437. doi: 10.1212/01.wnl.0000310416.90757.85
Fox, M. D., Buckner, R. L., Liu, H., Chakravarty, M. M., Lozano, A. M., Pascual-Leone, A., et al. (2014). Resting-state networks link invasive and noninvasive brain stimulation across diverse psychiatric and neurological diseases. Proc. Natl. Acad. Sci. U S A. 111, E4367–4375. doi: 10.1073/pnas.1405003111
Gu, S., Pasqualetti, F., Cieslak, M., Telesford, Q. K., Yu, A. B., Kahn, A. E., et al. (2015). Controllability of structural brain networks. Nat. Commun. 6, 8414. doi: 10.1038/ncomms9414
Guerra, A., Suppa, A., Bologna, M., D'Onofrio, V., Bianchini, E., Brown, P., et al. (2018). Boosting the LTP-like plasticity effect of intermittent theta-burst stimulation using gamma transcranial alternating current stimulation. Brain Stimulat. 11, 734–742. doi: 10.1016/j.brs.2018.03.015
Herrington, T. M., Cheng, J. J., and Eskandar, E. N. (2015). Mechanisms of deep brain stimulation. J. Neurophysiol. 115, 19–38. doi: 10.1152/jn.00281.2015
Ho, A. L., Feng, A. Y., Barbosa, D. A., Wu, H., Smith, M. L., Malenka, R. C., et al. (2021). Accumbens coordinated reset stimulation in mice exhibits ameliorating aftereffects on binge alcohol drinking. Brain Stimulat. 14, 330–334. doi: 10.1016/j.brs.2021.01.015
Horvath, J., Mathews, J., Demitrack, M., and Pascual-Leone, A. (2010). The neurostar TMS device: conducting the FDA approved protocol for treatment of depression. J. Vis. Exp. JoVE. 54, e2354. doi: 10.3791/2345
Hristova, A., Lyons, K., Tröster, A. I., Pahwa, R., Wilkinson, S. B., Koller, W. C., et al. (2000). Effect and time course of deep brain stimulation of the globus pallidus and subthalamus on motor features of parkinson's disease. Clin. Neuropharmacol. 23, 208–211. doi: 10.1097/00002826-200007000-00007
Jacobs, M., Premji, A., and Nelson, A. J. (2012). Plasticity-inducing TMS protocols to investigate somatosensory control of hand function. Neural Plast. 2012, 350574. doi: 10.1155/2012/350574
Kakusa, B., Saluja, S., Tate, W. J., Espil, F. M., Halpern, C. H., Williams, N. R., et al. (2019). Robust clinical benefit of multi-target deep brain stimulation for treatment of Gilles de la Tourette syndrome and its comorbidities. Brain Stimul. Basic Transl. Clin. Res. Neuromodulation. 12, 816–818. doi: 10.1016/j.brs.2019.02.026
Kalivas, P. W., and O'Brien, C. (2008). Drug addiction as a pathology of staged neuroplasticity. Neuropsychopharmacology. 33, 166–180. doi: 10.1038/sj.npp.1301564
Kauer, J. A., and Malenka, R. C. (2007). Synaptic plasticity and addiction. Nat. Rev. Neurosci. 8, 844–858. doi: 10.1038/nrn2234
Kozyrev, V., Staadt, R., Eysel, U. T., and Jancke, D. (2018). TMS-induced neuronal plasticity enables targeted remodeling of visual cortical maps. Proc. Natl. Acad. Sci. U S A. 115, 6476–6481. doi: 10.1073/pnas.1802798115
Kravitz, A. V., Freeze, B. S., Parker, P. R. L., Kay, K., Thwin, M. T., Deisseroth, K., et al. (2010). Regulation of parkinsonian motor behaviours by optogenetic control of basal ganglia circuitry. Nature. 466, 622–626. doi: 10.1038/nature09159
Kreitzer, A. C., and Malenka, R. C. (2008). Striatal plasticity and basal ganglia circuit function. Neuron. 60, 543–554. doi: 10.1016/j.neuron.2008.11.005
Kromer, J. A., and Tass, P. A. (2020). Long-lasting desynchronization by decoupling stimulation. Phys. Rev. Res. 2, 033101. doi: 10.1103/PhysRevResearch.2.033101
Kromer, J. A., and Tass, P. A. (2022). Synaptic reshaping of plastic neuronal networks by periodic multichannel stimulation with single-pulse and burst stimuli. PLoS Comput. Biol. 18, e1010568. doi: 10.1371/journal.pcbi.1010568
Kühn, A. A., Tsui, A., Aziz, T., Ray, N., Brücke, C., Kupsch, A., et al. (2009). Pathological synchronisation in the subthalamic nucleus of patients with Parkinson's disease relates to both bradykinesia and rigidity. Exp. Neurol. 215, 380–387. doi: 10.1016/j.expneurol.2008.11.008
Kuner, R., and Flor, H. (2017). Structural plasticity and reorganisation in chronic pain. Nat. Rev. Neurosci. 18, 20–30. doi: 10.1038/nrn.2016.162
Lafleur, L-. P, Tremblay, S., Whittingstall, K., and Lepage, J-.F. (2016). Assessment of effective connectivity and plasticity with dual-coil transcranial magnetic stimulation. Brain Stimulat. 9, 347–355. doi: 10.1016/j.brs.2016.02.010
Lee, Y., Zhang, Y., Kim, S., and Han, K. (2018). Excitatory and inhibitory synaptic dysfunction in mania: an emerging hypothesis from animal model studies. Exp. Mol. Med. 50, 1–11. doi: 10.1038/s12276-018-0187-x
Li, J., Mei, S., Zhang, X., Wang, Y., Jia, X., Liu, J., et al. (2022). Case report: combined therapy of bilateral subthalamic nucleus deep brain stimulation and spinal cord stimulation significantly improves motor function in a patient with multiple system atrophy with predominant parkinsonism. Front. Neurosci. 16, 929273. doi: 10.3389/fnins.2022.929273
Li, S. (2017). Spasticity motor recovery, and neural plasticity after stroke. Front. Neurol. 8, 120. doi: 10.3389/fneur.2017.00120
Limousin, P., and Foltynie, T. (2019). Long-term outcomes of deep brain stimulation in Parkinson disease. Nat. Rev. Neurol. 15, 234–242. doi: 10.1038/s41582-019-0145-9
Lo, M-.C , Younk, R., and Widge, A. S. (2020). Paired electrical pulse trains for controlling connectivity in emotion-related brain circuitry. IEEE Trans. Neural. Syst. Rehabil. Eng. 28, 2721–2730. doi: 10.1109/TNSRE.2020.3030714
Lozano, A. M., Fosdick, L., Chakravarty, M. M., Leoutsakos, J. M., Munro, C., Oh, E., et al. (2016). A phase II study of fornix deep brain stimulation in mild Alzheimer's disease. J. Alzheimers Dis. 54, 777–787. doi: 10.3233/JAD-160017
Madadi Asl, M., Vahabie, A-. H, Valizadeh, A., and Tass, P. A. (2022). Spike-timing-dependent plasticity mediated by dopamine and its role in parkinson's disease pathophysiology. Front. Netw. Physiol. 2, 1–18. doi: 10.3389/fnetp.2022.817524
Madadi Asl, M., Valizadeh, A., and Tass, P. A. (2018). Dendritic and axonal propagation delays may shape neuronal networks with plastic synapses. Front. Physiol. 9, 1849. doi: 10.3389/fphys.2018.01849
Maia, T. V., Cooney, R. E., and Peterson, B. S. (2008). The neural bases of obsessive-compulsive disorder in children and adults. Dev. Psychopathol. 20, 1251–1283. doi: 10.1017/S0954579408000606
Markram, H., Lübke, J., Frotscher, M., and Sakmann, B. (1997). Regulation of synaptic efficacy by coincidence of postsynaptic APs and EPSPs. Science. 275, 213–215. doi: 10.1126/science.275.5297.213
McCutcheon, R. A., Abi-Dargham, A., and Howes, O. D. (2019). Schizophrenia, dopamine and the striatum: from biology to symptoms. Trends Neurosci. 42, 205–220. doi: 10.1016/j.tins.2018.12.004
McPherson, J. G., Miller, R. R., and Perlmutter, S. I. (2015). Targeted, activity-dependent spinal stimulation produces long-lasting motor recovery in chronic cervical spinal cord injury. Proc. Natl. Acad. Sci. U S A. 112, 12193–12198. doi: 10.1073/pnas.1505383112
Mehta, U. M., Thanki, M. V., Padmanabhan, J., Pascual-Leone, A., and Keshavan, M. S. (2019). Motor cortical plasticity in schizophrenia: a meta-analysis of transcranial magnetic stimulation-electromyography studies. Schizophr. Res. 207, 37–47. doi: 10.1016/j.schres.2018.10.027
Melon, C., Chassain, C., Bielicki, G., Renou, J.-. P, Goff, L., et al. (2015). Progressive brain metabolic changes under deep brain stimulation of subthalamic nucleus in parkinsonian rats. J. Neurochem. 132, 703–712. doi: 10.1111/jnc.13015
Nakazono, H., Ogata, K., Takeda, A., Yamada, E., Oka, S., Tobimatsu, S., et al. (2021). A specific phase of transcranial alternating current stimulation at the β frequency boosts repetitive paired-pulse TMS-induced plasticity. Sci. Rep. 11, 13179. doi: 10.1038/s41598-021-92768-x
Nespoli, E., Rizzo, F., Boeckers, T., Schulze, U., and Hengerer, B. (2018). Altered dopaminergic regulation of the dorsal striatum is able to induce tic-like movements in juvenile rats. PLoS ONE. 13, e0196515. doi: 10.1371/journal.pone.0196515
Ni, Z., Udupa, K., Hallett, M., and Chen, R. (2019). Effects of deep brain stimulation on the primary motor cortex: insights from transcranial magnetic stimulation studies. Clin. Neurophysiol. Off. J. Int Fed. Clin. Neurophysiol. 130, 558–567. doi: 10.1016/j.clinph.2018.10.020
Olsen, S. T., Basu, I., Bilge, M. T., Kanabar, A., Boggess, M. J., Rockhill, A. P., et al. (2020). Case report of dual-site neurostimulation and chronic recording of cortico-striatal circuitry in a patient with treatment refractory obsessive compulsive disorder. Front. Hum. Neurosci. 14, 1–22. doi: 10.3389/fnhum.2020.569973
Palop, J., Chin, J., and Mucke, L. A. (2006). Network dysfunction perspective on neurodegenerative diseases. Nature. 443, 768–773. doi: 10.1038/nature05289
Parker, J. G., Marshall, J. D., Ahanonu, B., Wu, Y.-. W, Kim, T. H., et al. (2018). Diametric neural ensemble dynamics in parkinsonian and dyskinetic states. Nature. 557, 177–182. doi: 10.1038/s41586-018-0090-6
Pasqualetti, F., Zampieri, S., and Bullo, F. (2014). “Controllability metrics, limitations and algorithms for complex networks.” In: 2014 American Control Conference, 3287–3292. doi: 10.1109/ACC.2014.6858621
Peng, X., Hickman, J. L., Bowles, S. G., Donegan, D. C., and Welle, C. G. (2018). Innovations in electrical stimulation harness neural plasticity to restore motor function. Bioelectron. Med. 1, 251–263. doi: 10.2217/bem-2019-0002
Pfeifer, K. J., Kromer, J. A., Cook, A. J., Hornbeck, T., Lim, E. A., Mortimer, B. J. P., et al. (2021). Coordinated reset vibrotactile stimulation induces sustained cumulative benefits in parkinson's disease. Front. Physiol. 12, 624317. doi: 10.3389/fphys.2021.624317
Pfister, J.-. P, and Tass, P. A. (2010). STDP in oscillatory recurrent networks: theoretical conditions for desynchronization and applications to deep brain stimulation. Front. Comput. Neurosci. 4, 1–10. doi: 10.3389/fncom.2010.00022
Pugh, J. (2019). No going back? reversibility and why it matters for deep brain stimulation. J. Med. Ethics. 45, 225. doi: 10.1136/medethics-2018-105139
Razavi, B., Rao, V. R., Lin, C., Bujarski, K. A., Patra, S. E., Burdette, D. E., et al. (2020). Real-world experience with direct brain-responsive neurostimulation for focal onset seizures. Epilepsia. 61, 1749–1757. doi: 10.1111/epi.16593
Rosin, B., Nevet, A., Elias, S., Rivlin-Etzion, M., Israel, Z., Bergman, H., et al. (2007). Physiology and pathophysiology of the basal ganglia-thalamo-cortical networks. Parkinsonism Relat. Disord. 13(Suppl 3), S437–439. doi: 10.1016/S1353-8020(08)70045-2
Sabbagh, M., Sadowsky, C., Tousi, B., Agronin, M. E., Alva, G., Armon, C., et al. (2020). Effects of a combined transcranial magnetic stimulation (TMS) and cognitive training intervention in patients with Alzheimer's disease. Alzheimers Dement. 16, 641–650. doi: 10.1016/j.jalz.2019.08.197
Santarnecchi, E., Momi, D., Sprugnoli, G., Neri, F., Pascual-Leone, A., Rossi, A., et al. (2018). Modulation of network-to-network connectivity via spike-timing-dependent noninvasive brain stimulation. Hum. Brain. Mapp. 39, 4870–4883. doi: 10.1002/hbm.24329
Seeman, S. C., Mogen, B. J., Fetz, E. E., and Perlmutter, S. I. (2017). Paired stimulation for spike-timing-dependent plasticity in primate sensorimotor cortex. J. Neurosci. 37, 1935–1949. doi: 10.1523/JNEUROSCI.2046-16.2017
Seitz, A. R. (2013). Cognitive neuroscience: targeting neuroplasticity with neural decoding and biofeedback. Curr. Biol. 23, R210–R212. doi: 10.1016/j.cub.2013.01.015
Shen, W., Flajolet, M., Greengard, P., and Surmeier, D. J. (2008). Dichotomous dopaminergic control of striatal synaptic plasticity. Science. 321, 848–851. doi: 10.1126/science.1160575
Spencer, S. S. (2002). Neural networks in human epilepsy: evidence of and implications for treatment. Epilepsia. 43, 219–227. doi: 10.1046/j.1528-1157.2002.26901.x
Spix, T. A., Nanivadekar, S., Toong, N., Kaplow, I. M., Isett, B. R., Goksen, Y., et al. (2021). Population-specific neuromodulation prolongs therapeutic benefits of deep brain stimulation. Science. 374, 201–206. doi: 10.1126/science.abi7852
Syrkin-Nikolau, J., Neuville, R., O'Day, J., Anidi, C., Koop, M. M., Martin, T., et al. (2018). Coordinated reset vibrotactile stimulation shows prolonged improvement in Parkinson's disease. Mov. Disord. Off. J. Mov. Disord. Soc. 33, 179–180. doi: 10.1002/mds.27223
Tass, P. A. (2003). A model of desynchronizing deep brain stimulation with a demand-controlled coordinated reset of neural subpopulations. Biol. Cybern. 89, 81–88. doi: 10.1007/s00422-003-0425-7
Tass, P. A., and Majtanik, M. (2006). Long-term anti-kindling effects of desynchronizing brain stimulation: a theoretical study. Biol. Cybern. 94, 58–66. doi: 10.1007/s00422-005-0028-6
Tass, P. A., Qin, L., Hauptmann, C., Dovero, S., Bezard, E., Boraud, T., et al. (2012). Coordinated reset has sustained aftereffects in Parkinsonian monkeys. Ann. Neurol. 72, 816–820. doi: 10.1002/ana.23663
Temperli, P., Ghika, J., Villemure, J-. G, Burkhard, P. R., Bogousslavsky, J., et al. (2003). How do parkinsonian signs return after discontinuation of subthalamic DBS? Neurology. 60, 78–81. doi: 10.1212/WNL.60.1.78
Thomas, G. P., and Jobst, B. C. (2015). Critical review of the responsive neurostimulator system for epilepsy. Med. Devices Auckl. NZ. 8, 405–411. doi: 10.2147/MDER.S62853
Thompson, J. A., Lanctin, D., Ince, N. F., and Abosch, A. (2014). Clinical implications of local field potentials for understanding and treating movement disorders. Stereotact. Funct. Neurosurg. 92, 251–263. doi: 10.1159/000364913
Titiz, A. S., Hill, M. R. H., Mankin, E. A., Aghajan, Z. M., Tchemodanov, N., Maoz, U., et al. (2017). Theta-burst microstimulation in the human entorhinal area improves memory specificity. eLife. 6, 1–18. doi: 10.7554/eLife.29515
Todd, G., Kimber, T. E., Ridding, M. C., and Semmler, J. G. (2010). Reduced motor cortex plasticity following inhibitory rTMS in older adults. Clin. Neurophysiol. Off. J. Int. Fed. Clin. Neurophysiol. 121, 441–447. doi: 10.1016/j.clinph.2009.11.089
Tu, C., Rocha, R. P., Corbetta, M., Zampieri, S., Zorzi, M., Suweis, S., et al. (2018). Warnings and caveats in brain controllability. NeuroImage. 176, 83–91. doi: 10.1016/j.neuroimage.2018.04.010
Udupa, K., Bahl, N., Ni, Z., Gunraj, C., Mazzella, F., Moro, E., et al. (2016). Cortical plasticity induction by pairing subthalamic nucleus deep-brain stimulation and primary motor cortical transcranial magnetic stimulation in parkinson's disease. J. Neurosci. 36, 396–404. doi: 10.1523/JNEUROSCI.2499-15.2016
Urbin, M. A., Ozdemir, R. A., Tazoe, T., and Perez, M. A. (2017). Spike-timing-dependent plasticity in lower-limb motoneurons after human spinal cord injury. J. Neurophysiol. 118, 2171–2180. doi: 10.1152/jn.00111.2017
Valero-Cabré, A., Amengual, J. L., Stengel, C., Pascual-Leone, A., and Coubard, O. A. (2017). Transcranial magnetic stimulation in basic and clinical neuroscience: a comprehensive review of fundamental principles and novel insights. Neurosci. Biobehav. Rev. 83, 381–404. doi: 10.1016/j.neubiorev.2017.10.006
van de Ruit, M., and Grey, M. J. (2016). The TMS map scales with increased stimulation intensity and muscle activation. Brain Topogr. 29, 56–66. doi: 10.1007/s10548-015-0447-1
Versace, V., Langthaler, P. B., Höller, Y., Frey, V. N., Brigo, F., Sebastianelli, L., et al. (2018). Abnormal cortical neuroplasticity induced by paired associative stimulation after traumatic spinal cord injury: a preliminary study. Neurosci. Lett. 664, 167–171. doi: 10.1016/j.neulet.2017.11.003
Visser-Vandewalle, V., Andrade, P., Mosley, P. E., Greenberg, B. D., Schuurman, R., McLaughlin, N. C., et al. (2022). Deep brain stimulation for obsessive–compulsive disorder: a crisis of access. Nat. Med. 28, 1529–1532. doi: 10.1038/s41591-022-01879-z
Wang, J., Fergus, S. P., Johnson, L. A., Nebeck, S. D., Zhang, J., and Kulkarni, S. Shuffling improves the acute carryover effect of subthalamic coordinated reset deep brain stimulation. Front. Neurol. (2022) 13, 716046. doi: 10.3389/fneur.2022.716046.
Wang, J., Nebeck, S., Muralidharan, A., Johnson, M. D., Vitek, J. L., Baker, K. B., et al. (2016). Coordinated reset deep brain stimulation of subthalamic nucleus produces long-lasting, dose-dependent motor improvements in the 1-Methyl-4-phenyl-1,2,3,6-tetrahydropyridine non-human primate model of parkinsonism. Brain Stimulat. 9, 609–617. doi: 10.1016/j.brs.2016.03.014
Weinberger, M., Hutchison, W. D., Alavi, M., Hodaie, M., Lozano, A. M., Moro, E., et al. (2012). Oscillatory activity in the globus pallidus internus: comparison between Parkinson's disease and dystonia. Clin. Neurophysiol. 123, 358–368. doi: 10.1016/j.clinph.2011.07.029
Keywords: neuroplasticity, spike timing dependant plasticity, deep brain stimulation (DBS), biofeedback, network control, transcranial magnetic stimulation (TMS), neuromodulation, neurosurgery
Citation: Asp AJ, Chintaluru Y, Hillan S and Lujan JL (2023) Targeted neuroplasticity in spatiotemporally patterned invasive neuromodulation therapies for improving clinical outcomes. Front. Neuroinform. 17:1150157. doi: 10.3389/fninf.2023.1150157
Received: 23 January 2023; Accepted: 06 March 2023;
Published: 24 March 2023.
Edited by:
Peter A. Tass, Stanford University, United StatesReviewed by:
Mojtaba Madadi Asl, Institute for Research in Fundamental Sciences (IPM), IranJustus Alfred Kromer, Stanford University, United States
Copyright © 2023 Asp, Chintaluru, Hillan and Lujan. This is an open-access article distributed under the terms of the Creative Commons Attribution License (CC BY). The use, distribution or reproduction in other forums is permitted, provided the original author(s) and the copyright owner(s) are credited and that the original publication in this journal is cited, in accordance with accepted academic practice. No use, distribution or reproduction is permitted which does not comply with these terms.
*Correspondence: J. Luis Lujan, bHVqYW4ubHVpcyYjeDAwMDQwO21heW8uZWR1