- 1Division of Clinical Cognitive Sciences, Department of Neurology, RWTH Aachen University Hospital, Aachen, Germany
- 2Institute of Neuroscience and Medicine-4, Forschungszentrum Jülich GmbH, Jülich, Germany
- 3Leibniz Research Centre for Working Environment and Human Factors, Department of Psychology and Neurosciences, Dortmund, Germany
- 4JARA-BRAIN-Translational Medicine, Jülich-Aachen-Research-Alliance (JARA), Aachen, Germany
- 5Department of Neurology, RWTH Aachen University Hospital, Aachen, Germany
- 6Institute of Neuroscience and Medicine-11, Forschungszentrum Juelich, Jülich, Germany
Introduction: Anodal transcranial direct current stimulation (tDCS) has been reported to modulate gamma-aminobutyric acid levels and cerebral energy consumption in the brain. This study aims to investigate long-term GABA and cerebral energy modulation following anodal tDCS over the primary motor cortex.
Method: To assess GABA and energy level changes, proton and phosphorus magnetic resonance spectroscopy data were acquired before and after anodal or sham tDCS. In anodal stimulation, a 1 mA current was applied for 20 min, and the duration of ramping the current up/down at the start and end of the intervention was 10 s. In the sham-stimulation condition, the current was first ramped up over a period of 10 s, then immediately ramped down, and the condition was maintained for the next 20 min.
Results: The GABA concentration increased significantly following anodal stimulation in the first and second post-stimulation measurements. Likewise, both ATP/Pi and PCr/Pi ratios increased after anodal stimulation in the first and second post-stimulation measurements.
Conclusion: The approach employed in this study shows the feasibility of measuring long-term modulation of GABA and high-energy phosphates following anodal tDCS targeting the left M1, offering valuable insights into the mechanisms of neuroplasticity and energy metabolism, which may have implications for applications of this intervention in clinical populations.
Introduction
Several studies have demonstrated molecular and neurophysiological evidence linking altered neuronal plasticity to neurological disorders (Di Lazzaro et al., 2010) and altered energy metabolism to metabolic disorders (Soares et al., 2011) in brain areas. It has been suggested that metabolic disorders are associated with neurological disorders (Crabtree and Gogos, 2014; Penninx and Lange, 2018). For instance, abnormal neuroplasticity mediated by altered neurotransmission (Harrison, 1999) has been observed in the prefrontal cortex in schizophrenia patients (Hulshoff Pol and Kahn, 2008). One suggested explanation is that hypofunction of the NMDA-type glutamate receptor cause a decrease in the excitation of GABAergic interneurons, resulting in glutamatergic neurons disinhibition (Kondziella et al., 2007; Moghaddam et al., 1997; Olney et al., 1999; Stone et al., 2009). This disinhibition of glutamatergic neurons may result in excessive glutamate stimulation, which may cause neuronal damage or death via excitotoxicity leading to a hyperdopaminergic state and psychosis. In an EEG study comparing healthy subjects with schizophrenia, variation in the P3a distribution was found which shows differences in the attention system activity (Mugruza-Vassallo and Potter, 2019). Functional imaging studies that assess regional cerebral blood flow (rCBF) can help identify cerebral activity associated with schizophrenia (Tamminga, 1999). For example, Liddle et al. (1992a) and Liddle et al. (1992b) found a negative correlation between the psychomotor poverty symptoms of schizophrenia and rCBF in the lateral prefrontal cortex and overactivity in the striatum.
Pharmacological studies have discovered genes involved in the pathophysiology of schizophrenia (De Jong et al., 2016; Gaspar and Breen, 2017; Rodriguez-Lopez et al., 2020; Ruby et al., 2014), such as GRM3 expression in astrocytic cells, which is required to protect neurons against NMDA induced neurotoxicity (Hikmah et al., 2023). This suggests that diminished GRM3 functionality, which may result in hyper-glutamatergic signalling, may cause more damage to the neurons as a result of the abnormal signaling exhibited in schizophrenia (Saini et al., 2017). In schizoaffective disorder, both schizophrenic and affective occur at the same time (Werner and Covenas, 2016). The mesolimbic system (the hippocampus and prefrontal cortex) plays an important role in the symptoms, which involves alteration in a multi-neurotransmitter system such as dopamine, serotonin, GABA and glutamate (Kulkarni et al., 2022) due to susceptibility genes such as neuregulin-1 dysbindin-1, GAD67 catechol-O-methyl transferase and monoamine oxidase (Haller et al., 2014; Ruby et al., 2014; Werner and Coveñas, 2013).
Several studies using magnetic resonance spectroscopy (MRS) have indicated that major depressive disorder (MDD) individuals have lower GABA concentrations in occipital cortex than healthy controls (Sanacora et al., 2004; Sanacora et al., 1999; Sanacora et al., 2003). MDD, along with GABA, is also related to altered serotonin activity. In many research studies, the binding potential of serotonin receptor, 5-hydroxytryptamine or (5-HT) was found to be lower in patients with MDD than in control subjects (Bhagwagar et al., 2004; Drevets et al., 1999; Hirvonen et al., 2008; Sargent et al., 2000). Selective serotonin reuptake inhibitors (SSRI) are most widely used to treat MDD and have shown significant change in the dorsolateral prefrontal cortex (DLPFC) volume and resting-state functionality (Lee et al., 2023).
Altered neurotransmission as seen in MDD is multifaceted and has been associated with the risk of developing severe medical disorders. i.e. it increases the risk of cardiovascular disorders by 1.5–2 fold (Van der Kooy et al., 2007) for stroke 1.8 fold (Ramasubbu and Patten, 2003) for Alzheimer’s disease by 2.1 fold (Green et al., 2003) and diabetes by 60% (Mezuk et al., 2008). The relationship between metabolic disorder and other psychiatric disorders is evident in the above-mentioned studies. Moreover psychiatric disorders are often accompanied by cognitive impairment in various domains, such as attention, executive functions, memory, and processing speed, as highlighted in studies (Grundman et al., 2004; McIntyre et al., 2015; Millan et al., 2012; Reichenberg, 2010). GABA neurotransmitter plays an important role in cognition and several studies have shown a link between dorsolateral prefrontal cortex GABA to working memory (Duncan et al., 2014; Yoon et al., 2016) and supplementary motor area GABA levels to motor distraction (Duncan et al., 2014; Mullins et al., 2014) and to alterations in cognitive processing with age (Harris et al., 2017; Porges et al., 2017). The interaction between altered neuroplasticity and metabolism has implications on cognitive functions or impairment. Therefore, simultaneously studying the neurochemical mechanisms behind neuronal plasticity and energy metabolism may offer significant breakthroughs for therapeutic interventions. With the advanced assessment of high-energy phosphate and neurotransmitters like gamma amino butyric acid (GABA) we can get a deeper insight into the mechanism of such changes in the brain of patients suffering from psychiatric and metabolic disorders.
A well-established approach for modulating neuronal plasticity and energy in humans is a non-invasive brain stimulation technique known as transcranial direct current stimulation (tDCS). By targeting specific brain regions, tDCS can be used to modulate brain energy metabolism and neuronal plasticity. Human in vivo studies have demonstrated that the application of anodal tDCS in the primary motor cortex can lead to spontaneous firing rates of cortical neurons, inducing neuronal excitation and altering energy consumption (Binkofski et al., 2011; Nitsche and Paulus, 2000). This modulation in excitability and energy is likely mediated by an altered concentration of the inhibitory neurotransmitter GABA (Stagg et al., 2009) and high-energy phosphates, e.g., adenosine triphosphate (ATP) and phosphocreatine (PCr).
Owing to ongoing advancements in magnetic resonance (MR) techniques (Choi et al., 2021; Henning, 2018; Wilson et al., 2019), changes in GABA and high-energy phosphates in M1 following tDCS can be monitored using MR spectroscopy (MRS). Specifically, it has been shown that proton (1H)-MRS (Patel et al., 2019) and phosphorus (31P)-MRS can be used to measure changes in GABA and energy phosphates in the M1, for example, following anodal tDCS as compared to sham tDCS. The time courses of separately measured concentrations of GABA and ATP/PCr in the aforementioned studies indicated that GABAergic activity and bioenergetics inside the M1 might work together to modulate neuronal excitability and energy. Consecutive measurement of both 1H- and 31P-MRS, before and after tDCS, could offer a promising approach to yield information related to plastic adaptation and energy consumption in both healthy and diseased brains.
In this study, we hypothesize that the application of anodal tDCS to the M1 region modulates GABA concentration and brain energy consumption. Notably, this work presents a measurement approach that integrates one pre-tDCS, 1H- and 31P-MRS measurement and two consecutive post-anodal tDCS, 1H- and 31P-MRS measurements to demonstrate the viability of using MRI and MRS to measure the long-term modulation of GABA and high-energy phosphates following anodal tDCS targeting the left M1 for the first time.
Materials and methods
Forty-four healthy subjects (22 anodal and 22 sham) with no history of neurological or psychiatric diseases were recruited for this single-blind, randomized control pilot study. The subjects (Mean age: 28 ± 7; Gender: 26 Female and 18 Male) were all right-handed, as evaluated by the Edinburgh Handedness Inventory (Oldfield, 1971). All examinations were conducted at the Division of Clinical Cognitive Sciences of the RWTH Aachen University, Germany, and subjects provided their written informed consent for participation in this pilot study, which was agreed upon by the local ethics committee.
The non-invasive DC-Stimulator (neuroConn GmbH, Germany) used to stimulate the M1 region was programmed to deliver constant, direct current to the brain via two rubber electrodes (5 × 7 cm) covered by saline-soaked (0.9% NaCl) sponges, which modulate brain activity. One electrode was centered over the left M1 (5 cm lateral to Cz, C3) and the other over the contralateral supraorbital ridge using the conventional EEG 10/20 system. To accomplish the electrical contact between the electrodes and the scalp, 0.9% NaCl solution was used as a conducting medium. In anodal stimulation, a 1 mA current was applied for 20 min, and the duration of ramping the current up/down at the start and end of the intervention was 10 s. In the sham-stimulation condition, the current was first ramped up over a period of 10 s, then immediately ramped down, and the condition was maintained for the next 20 min.
All MR measurements were carried out on a 3 T PRISMA MRI scanner (Siemens Healthineers, Erlangen, Germany) with a dockable patient bed. A quadrature double-tuned head coil (RAPID Biomedical, Wuerzburg, Germany) was used to achieve both 1H and 31P acquisitions. Figure 1 shows a tabular representation of the overall experimental design. Each experimental session started with a localizer followed by the acquisition of whole-brain anatomical images using an MP-RAGE MRI sequence [parameters: voxel size = 1.5 × 1.5 × 1.5 mm3, repetition time (TR) = 2,000 ms, echo time (TE) = 2.05 ms, inversion time (TI) = 900 ms, acquisition time (TA) = 4:32 min], with RF power and global static magnetic field (B0) shimming calibrations. Figure 2 shows the 3D anatomical image used to locate a 30 × 30 × 30 mm3 voxel-of-interest (VOI) within the hand-knob area of the left M1. Prior to the MRS measurements, an additional advanced shimming procedure was employed using a FASTEST map MRS sequence (Gruetter and Tkáč, 2000) to improve the B0 homogeneity in the selected VOI. A full-width at half-maximum (FWHM) of the 1H resonance peak in the VOI was achieved at approximately 15 Hz. A MEGA-PRESS MRS sequence (Mescher et al., 1998; parameters: voxel size = 30 × 30 × 30 mm3, TR = 5,350 ms, TE = 68 ms, TA =17:53 min, averages = 96, vector size = 1,024, flip angle = 90°) and a 3D chemical shift imaging MRS sequence (Wenger et al., 2020; parameters: voxel size = 30 × 30 × 30 mm3, TR = 3,730 ms, TE = 2.3 ms, TA = 14:33 min, averages = 6, vector size = 1,024, flip angle = 90°) were applied to acquire 1H-GABA and 31P spectra, respectively. A standard PRESS MRI sequence with eight averages was also included to record an unedited spectrum for the assessment of the creatine and N-acetylaspartate acid (NAA) linewidths. The nuclear Overhauser effect enhancement technique was employed to improve the quality of the spectra in the 31P acquisition. Reference 1H and 31P spectra as shown in Figure 3 were attained before the application of tDCS (pre-stimulation measurements), which took approximately 35 min. After obtaining the baseline MRS scans, the whole patient table, including the subject and the coil, was undocked from the scanner and moved outside the magnet room for stimulation. The stimulation was delivered for 20 min, during which participants were asked to remain still. Following the stimulation, the patient table was docked back to the MR scanner for the subsequent measurements. Undocking and docking of the table procedure helped to mitigate subject movement between the pre- and post-stimulation MRS measurements. The same MP-RAGE MRI sequence was used post-stimulation to ensure that the location of the VOI was identical to the pre-stimulation position. Two consecutive post-stimulation 1H and 31P MRS measurements were conducted. The 1H MRS measurement was conducted for 17:53 min, and then 31P MRS was conducted for 14:33 min twice, in a constant order post-stimulation. All subjects were informed about the duration of the measurement and were asked to remain awake and not to move during the whole experimental procedure.
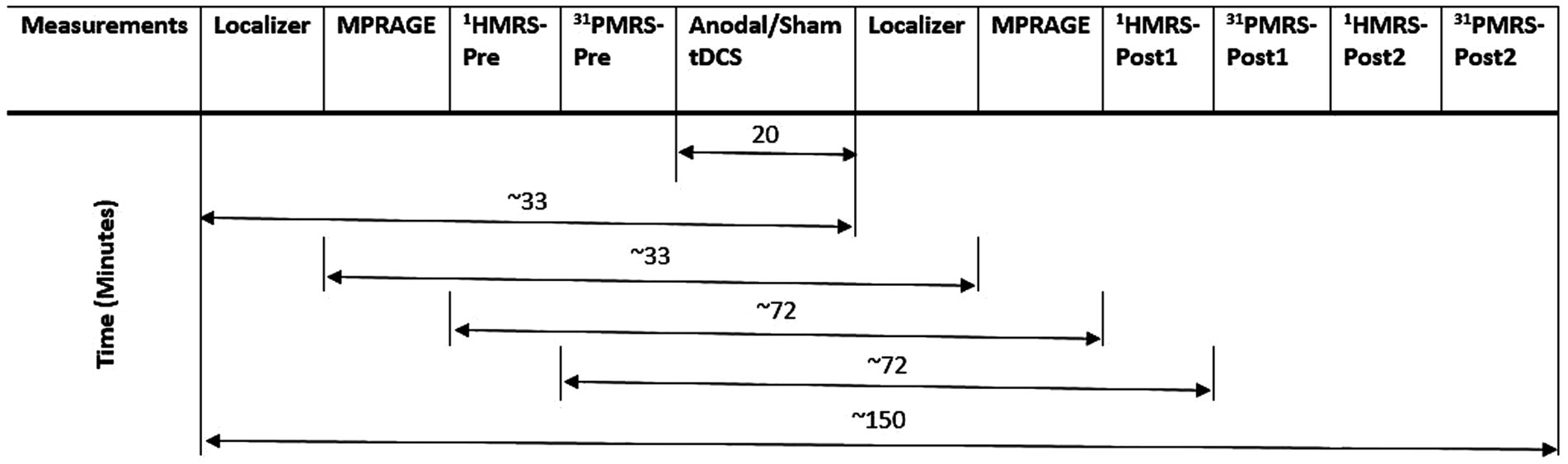
Figure 1. The table shows the 1H GABA, 31P MRS and other MR measurements performed before and after tDCS.
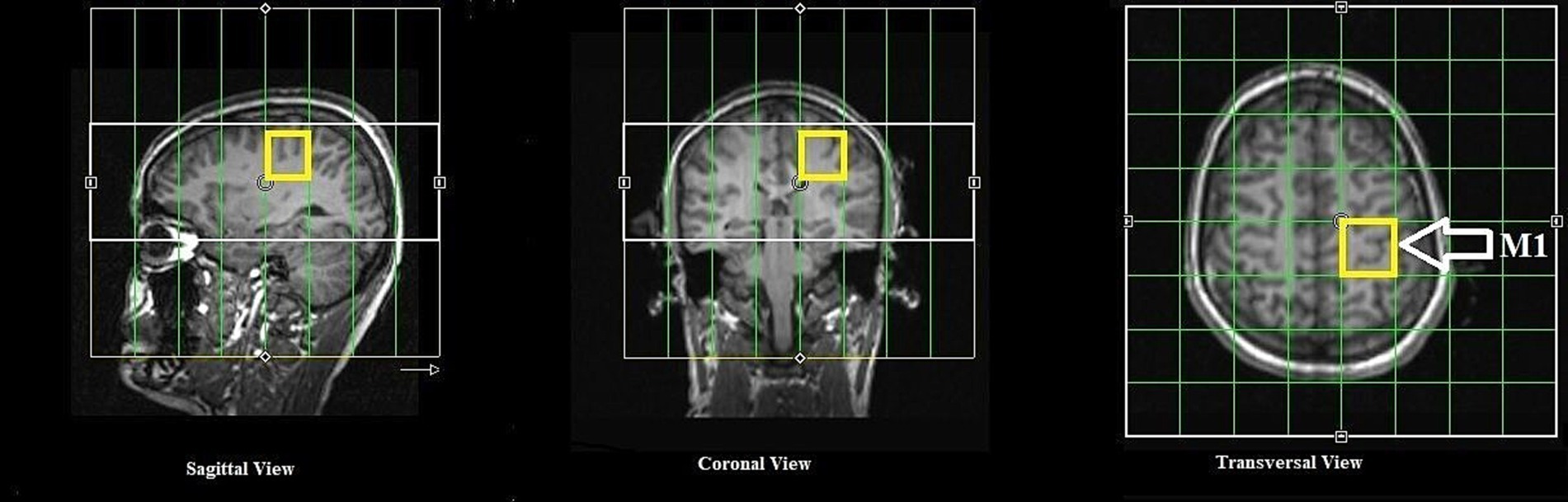
Figure 2. Anatomical MR images in axial, coronal and sagittal slices and the overlaid voxel of interest (in yellow) for 1H and 31P MRS.
All 1H-GABA-MRS data processing was performed using the LCModel (Provencher, 1993), a spectral quantification tool that fits each spectrum as a weighted linear combination of basis spectra from individual brain metabolites. The difference basis set included GABA, Glu, glutamine, glutathione, and NAA. Based on an 8 × 8 CSI grid, the 31P MR spectra from one voxel were analyzed with TARQUIN 4.3.10(Wilson et al., 2011). All data underwent a Fourier transformation, as well as zero and first-order phase correction, and were fitted as linear combinations of the simulated metabolic basis set, including PCr, ATP, Pi, PE, GPC and GPE for 31P-MRS. While PCr, Pi, PE, GPC and GPE are observed as singlet Lorentzian peaks, the signals from the α- and γ-ATP were modelled as doublets. However, the signal from ATP-ß was excluded from the analysis due to phasing instabilities (Novak et al., 2014). The quality of the final spectra was assessed using the Cramér-Rao-Lower-Bounds (Rao, 1947) minimum possible variance on a fit parameter. Only data that had Cramér-Rao-Lower-Bounds values of less than ≤20% were included in the analysis (Kreis, 2016; Öz et al., 2014; Peek et al., 2023; Wilson et al., 2019).
Statistical analysis
In order to investigate the effects of tDCS on the inhibitory neurotransmitter and energy metabolites in the left M1, changes in proton and phosphorus metabolites in pre- and post-stimulation scans were calculated separately to obtain the information about GABA, ATP/Pi and PCr/Pi. Each ratio was normalized (post1-tDCS/pre-tDCS & post2-tDCS/pre-tDCS) with its reference measurement (pre-tDCS). Statistical analysis was conducted with SPSS (version 29.0 Armonk, NY, USA), with p = 0.05 set as the significance threshold. A mixed-design Analysis of variance was performed for GABA concentration, ATP/Pi and PCr/Pi metabolites. Stimulation (sham vs. anodal) was considered as the between-subjects factor, and measurement (three measurements: one pre-stimulation and two post-stimulation measurements [post1 and post2]) was considered as the within-subject factor. Paired sample t-test comparisons (p > 0.05) were performed in the cases of significant ANOVA results.
Results
Effect of tDCS on GABA
For GABA, the main effect of the stimulation was found to be significant [F(1, 42) = 4.742, p = 0.035]; however, the main effect of the measurement was not significant [F(2, 84) = 2.855, p = 0.063]. That being said, the stimulation × measurement interaction was significant [F(2,84) = 3.387, p = 0.038]. Paired sample t-test comparisons show that concentration did not change significantly from pre-tDCS measurement to post1- and post2-tDCS measurements [ts(21) ≤ 0.277 ps > 0.05] for the sham group. Conversely, relative to the pre-tDCS, GABA concentration in the anodal tDCS group significantly increased after 14 min at the post1-tDCS measurement [ts(21) = 3.380 p = 0.001], and also after 48 min at the post2-tDCS measurement [ts(21) = 1.893 p = 0.036]. Thus, in the sham group, GABA concentration stayed at the pre-tDCS baseline level across all post-tDCS measurements. In contrast, data from the anodal group suggest an increase in GABA concentration across the two time points in the post-tDCS phase. Figure 4A shows the changes in normalized GABA concentration levels induced by tDCS at pre-tDCS, post1-tDCS and post2-tDCS spectroscopy measurements.
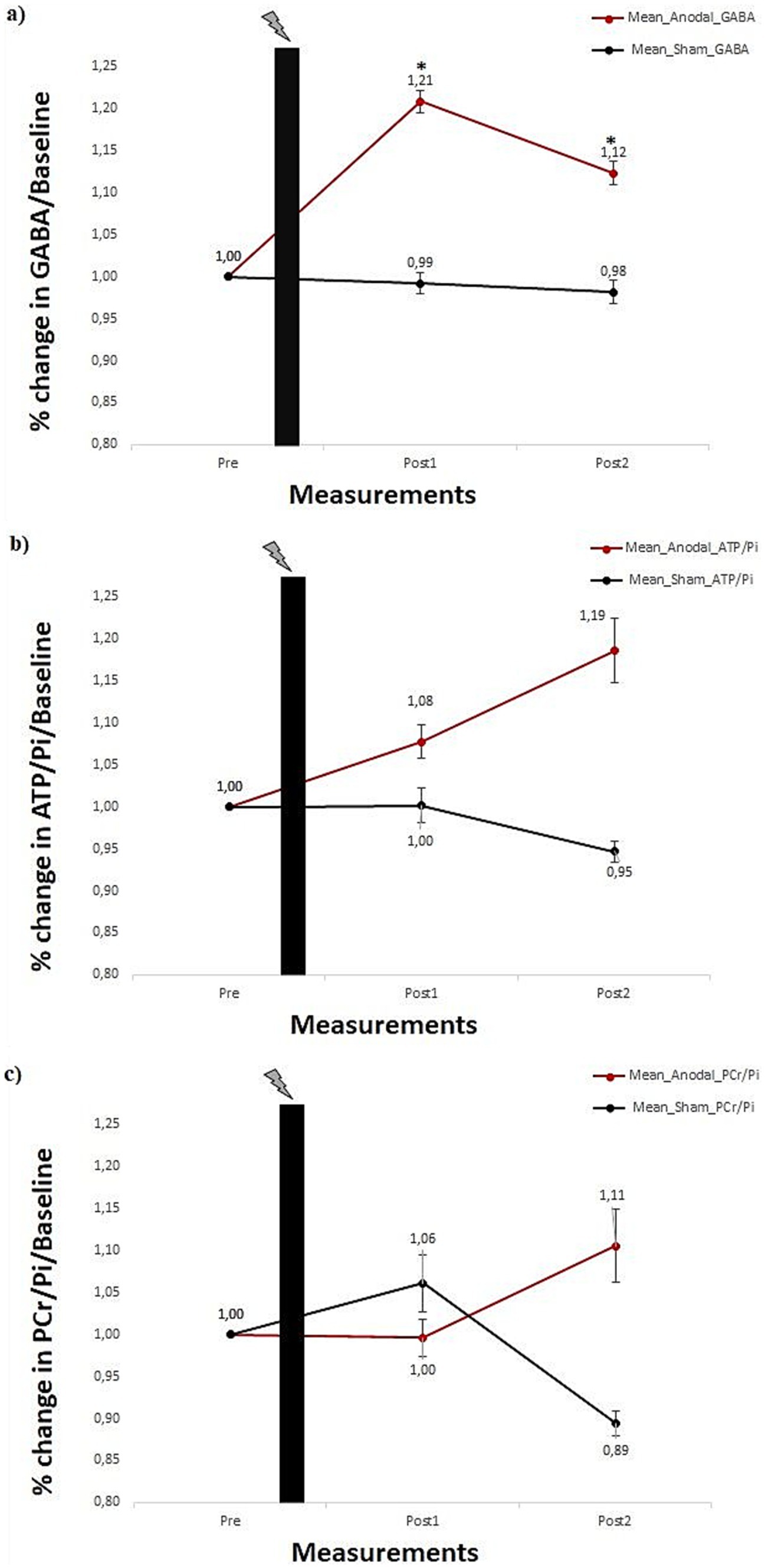
Figure 4. (A) Percentage change in mean GABA concentration. (B) ATP/Pi ratio, and (C) PCr/Pi ratio for anodal and sham stimulation groups, each comprising 22 subjects. Error bars reflect the standard error of the mean. Black plot: anodal/sham stimulation. *Show significances (p < 0.05) for a paired sample t-test.
Effect of tDCS on energy phosphates (ATP/pi & PCr/pi)
For ATP/Pi ratios, neither the main effect of stimulation [F(1,42) = 1.573, p = 0.217] nor the main effect of the measurement [F(2,84) = 0.259, p = 0.772] was significant. Furthermore, the interaction stimulation × measurement was also not significant [F(2,84) = 0.887, p = 0.416]. Figure 4B shows changes in normalized ATP/Pi ratios for pre-tDCS, post1-tDCS and post2-tDCS spectroscopy measurements. For PCr/Pi ratios, neither the main effect of stimulation [F(1,42) = 0.218, p = 0.643] nor the main effect of the measurement [F(2,84) = 0.045, p = 0.956] was significant. Additionally, the interaction stimulation × measurement was also not shown to be significant [F(2,84) = 852, p = 0.430]. Figure 4C shows tDCS-induced effects on normalized PCr/Pi ratios for pre-tDCS, post1-tDCS and post2-tDCS spectroscopy measurements.
Discussion
The human brain functions as a dynamic system, continually adjusting its metabolic interactions to accommodate the activation status and energy requirements of the entire organism. Therefore, static MRS measurements obtained in single sessions necessarily deliver an incomplete picture of the state of the brain. To address this limitation, our feasibility study demonstrates the acquisition of combined measurements using 1H-GABA-MRS, 31P-MRS and anodal stimulation to provide unique information relating to adaptive plasticity and energy consumption in the human brain during extended time periods after plasticity-inducing brain stimulation.
Our results indicate a notable increase in GABA levels measured by 1H-MRS following 20 min of anodal stimulation over the M1 at both the initial post-tDCS measurement and the second post-tDCS measurement, which contradicts previous reports showing a reduction in GABA levels at these time points (Agboada et al., 2019; Patel et al., 2019). Studies have shown that changes in cortical excitability due to tDCS are calcium-dependent (Grundey et al., 2018; Nitsche et al., 2003) and that calcium concentration within a specific range is required for LTP induction (Lisman, 2001). Therefore, it could be argued that previous studies (Patel et al., 2019; Stagg et al., 2009) utilizing relatively low tDCS intensities and/or short durations might have been operating at the lower limit of the calcium concentrations required for inducing the aforementioned neuroplastic changes. Within the range given in Lisman (2001), increasing calcium concentration should, theoretically, increase the efficacy of LTP induction. Hence, prolonging the duration of stimulation beyond a critical time point may lead to a saturation of the after-effects, possibly caused by calcium overflow (Monte-Silva et al., 2013). Consequently, higher calcium concentration might activate counteracting homeostatic mechanisms, such as the activation of potassium channels or the saturation of NMDA receptors, thereby limiting the amount of plasticity (An et al., 2000; Lau and Zukin, 2007; Misonou et al., 2004). These potential mechanisms require further exploration. To the best of our knowledge, there are very few studies investigating the mechanism underlying these non-linear effects (Batsikadze et al., 2013; Jamil et al., 2017; Monte-Silva et al., 2013). In line with this notion, studies using different non-invasive brain stimulation techniques have also reported non-linear cortical excitability effects post-tDCS (Batsikadze et al., 2013; Kuo et al., 2013) with respect to varying intensity (Moliadze et al., 2012) and duration (Choi et al., 2021; Heimrath et al., 2020; Monte-Silva et al., 2013).
In an apparent contradiction to the results obtained in our study, a study from Agboada et al. (2019) reported that, having applied anodal tDCS with 1 mA current for 20 min, cortical excitation could be subsequently monitored for two hours by measuring motor evoked potentials (MEPs) using TMS. However, the reason for these opposing results might be due to a difference in the study population. Furthermore, the amount of extracellular GABA measured by MRS might not correlate with TMS-induced activity in the GABAergic synapses. As neurotransmitters are active in intracellular and extracellular space (Belelli et al., 2009; Martin and Rimvall, 1993), and MRS is not sensitive enough to differentiate between these sources, it is only possible to speculate about mechanisms that are known to change GABA concentration. Moreover, animal studies conducted by Purpura and McMurtry (1965) have shown that direct stimulation in the deep cortical layers leads to the activation of neurons through cathodal stimulation and deactivation through anodal stimulation. Hence, the longer tDCS protocol used in the present study might have more significant effects on GABAergic neurons, which are less affected under the weaker electric field induced by a shorter stimulation duration. It is also possible that longer tDCS protocols may affect the target neurons as well as neighbouring non-target neurons, which might change the direction of plasticity in the target regions.
The levels of MRS GABA have been linked to behavioural measures in M1, where higher GABA levels were associated with greater inhibition and slower reaction times (Stagg et al., 2011). Changes in GABA levels in the dorsolateral prefrontal cortex (DLPFC) have also been observed in studies on impulsivity, a personality trait associated with various psychiatric disorders in the DSM. Studies have indicated that higher GABA levels in the DLPFC are linked to lower urgency scores, suggesting a role in self-control for the GABA-mediated inhibitory mechanism (Boy et al., 2011). Additionally, research by Kühn et al. (2016) and Yoon et al. (2016) demonstrated that higher GABA levels in the DLPFC and anterior cingulate cortex (Rossini et al., 1994) are associated with less performance degradation under higher working memory load compared to subjects with lower GABA levels. Furthermore, elevated GABA levels in the visual cortex have been associated with improved cognitive and perceptual performance in visual discrimination tasks (Cook et al., 2016; Edden et al., 2009; Porges et al., 2017; Sumner et al., 2010). van Vugt et al. (2020) investigated the influence of GABA modulation on audio perception discrimination and mapping motor responses with perceived sounds. Elevated GABA levels in the left sensorimotor cortex (SM1) during training were associated with enhanced behavioural learning, highlighting the role of GABA modulation in forming unique audio-motor learning tasks. The elevated GABA levels in the ACC during situations involving conflict or uncertainty (Bezalel et al., 2019) contribute to decision-making by maintaining inhibition and preventing excessive excitement. A further study investigated the relationship between GABA levels within the sensorimotor cortex and measures of sensory discrimination and demonstrated that healthy subjects who had higher GABA levels showed greater frequency discrimination (Puts et al., 2011). Higher GABA levels were associated with improved performance in orientation discrimination task as seen in studies of the primary visual cortex (Edden et al., 2009). Similar relationships between a variety of task-specific behaviours and levels of GABAergic inhibition have also been demonstrated in a number of brain regions outside the sensorimotor cortex (Boy et al., 2010; Boy et al., 2011; Jocham et al., 2012; Sumner et al., 2010). This implies that MRS-derived measurements of GABA are behaviorally relevant in a variety of cortical areas, and not limited to M1.GABA modulation is not exclusive to learning and has been observed in memory consolidation as well. For an increase in GABA levels in the occipital lobes following a visual learning task helps in fast memory consolidation and protects against interference (Shibata et al., 2017). The use of Zolpidem, a GABAA agonist, during sleep has been shown to enhance episodic memories, indicating the role of GABA in memory consolidation. The Increased GABA activity during sleep supports memory consolidation (Mednick et al., 2013; Zhang et al., 2020).
Coxon and colleagues discovered that engaging in high-intensity interval exercise resulted in elevated GABA levels in SM1 (Coxon et al., 2018). In a study by Maddock, it was demonstrated that vigorous cycling increased GABA/Cr levels in the primary visual cortex post-exercise (Maddock et al., 2016). This suggests that physical exercise influences regional GABA levels beyond SM1, but the regional specificity of GABA changes due to exercise require further investigation.
It has been well reported that GABA and Glu interact along the same biochemical pathway, as Glu is the primary precursor for GABA synthesis (Petroff, 2002; Rae et al., 2003). Given that GABA is synthesized from the alpha decarboxylation of Glu by glutamic acid decarboxylase (GAD-67), the activity-driven expression of GAD-67 controls GABA synthesis and determines the concentration of GABA in interneurons (Lau and Murthy, 2012). It is possible that anodal tDCS may subtly interfere with GAD-67 activity and consequently affect this pathway, resulting in a change in the GABA concentration. However, we were only able to access the Glx information at 3 T, which combined glutamate and glutamine signals due to their close chemical shift range. A study, for example, using 7 T MRI, might help to investigate long-term glutamate modulation and allow further exploration of the mechanism of excitatory glutamatergic neurons in long-term plasticity.
The observed increase in ATP/Pi and PCr/Pi ratios shown in our study can be partially compared to findings from Binkofski et al. (2011), who reported their first non-significant post-tDCS measurement between the end of tDCS and before around 65th minute of MRS measurement, thus also showing an initial rise in energy followed by a subsequent decline after the 65th minute. The increase in ATP/Pi ratio and PCr/Pi ratio in our study could be attributed to a higher rate of energy phosphate synthesis compared to its consumption as a consequence of the longer tDCS duration. ATP concentration has been observed as a potential modulator of neurotransmission (Miller et al., 1980; Miller et al., 1977), and the neurotransmitter glutamate is a known precursor of GABA synthesis and uses glutamic acid decarboxylase (GAD) to synthesize GABA. At least 50% of GAD is present in the brain as apoenzyme (apoGAD), thereby providing a reservoir of inactive GAD that can be drawn on when additional GABA synthesis is required (Itoh and Uchimura, 1981; Miller et al., 1980; Miller et al., 1977). It seems that GAD plays an important role in regulating the transmitter pool of GABA. Studies indicate that the increase in the ATP/Pi ratio accelerates the formation of apoGAD (Meeley and Martin, 1983; Wu and Martin, 1984), inhibits its activation (Porter and Martin, 1988; Sze et al., 1983) and stabilizes apoGAD against thermal denaturation (Meeley and Martin, 1983; Porter and Martin, 1988). It appears that the activation of GAD is regulated by energy metabolism, and increased neural activity leads to a higher turnover of energy metabolites. Moreover, it is plausible that these energy metabolites play a vital role in linking GAD activity with neuronal activity. Thus, it is plausible that elevated levels of ATP might stimulate the activation of apoGAD, thereby promoting GABA synthesis.
GABA appears to have two major functions in nervous tissue, acting both as a neurotransmitter and as an intermediate in the energy metabolism of GABAergic neurons (Shelp et al., 2012). While much attention is given to the role of GABA as a neurotransmitter, its role as a metabolic intermediate is also important and may help to explain the link between neuronal plasticity and energy metabolism. This metabolic function of GABA arises from its relationship with the tricarboxylic acid (TCA) cycle (Baxter, 1970). The TCA cycle is a series of processes that produce high-energy phosphate, such as ATP, following glycolysis. Three enzymes of GABA pathways, GAD, GABA-a-oxoglutarate transaminase and succinic semialdehyde dehydrogenase, facilitate a bypass known as the GABA shunt. This bypass allows for the circumnavigation of two steps of the TCA cycle, leading to the production of glutamate for GABA synthesis. In this way, GABA is metabolized via the GABA shunt within the TCA cycle, thus emphasizing the role of GABA synthesis in maintaining adequate GABA levels. Based on this neurochemical mechanism, it might be possible that the longer stimulation protocol demands higher energy phosphate synthesis and also higher GABA synthesis, which may work together to regulate the higher demand for energy phosphate and the corresponding GABA synthesis in the brain. Our study demonstrates that anodal tDCS leads to an increase in ATP resynthesis to meet the energy demand, which is similar to the energy regulation observed after physical exercise (Hargreaves and Spriet, 2020). Modulation of energy following physical activity has shown to improve memory, reasoning, planning, motor skill (Nanda et al., 2013; Statton et al., 2015) and promote cognitive functioning in both healthy as well as clinical populations such as stroke, multiple sclerosis and depression (Moriarty et al., 2019; Moriya et al., 2016; Sandroff et al., 2015; Sibley et al., 2006; Vasques et al., 2011; Wang et al., 2016). Physical activity boosts brain-derived neurotrophic factor (BDNF), promoting neuroplasticity (Loprinzi and Frith, 2019), and consequently enhancing memory and learning (Piepmeier and Etnier, 2015), while also offering protection against Alzheimer’s and depression (Bjornebekk et al., 2005; Erickson et al., 2011; Liu and Nusslock, 2018). Elevated BDNF levels are associated with the survival and growth of neurons (McAllister et al., 1999). Lower BDNF levels are linked to ageing and neurodegenerative diseases (Holsinger et al., 2000). These findings reveal the usefulness of physical exercise or alteration of energy regulation as shown in our study can be employed as an auxiliary tool for rehabilitation because it appears to affect the neurological system for learning and skill acquisition.
The results of this study suggest that the new combined approach introduced here has strong implications for the measurement of energy and neural plasticity in both healthy subjects and neuropsychiatric patients (Gobel et al., 2013; Jauch-Chara et al., 2015; Stagg et al., 2011; Stagg and Johansen-Berg, 2013). For instance, psychiatric and metabolic diseases have been found to be interconnected, often acting as a precursor to each other (Estrov et al., 2000; Zuccoli et al., 2017). In this context, combined 1H- and 31P-MRS together with anodal tDCS may represent a two-pronged approach to better understand impaired glucose metabolism and psychiatric disorders without the need for pharmacological intervention. Furthermore, this approach can be employed in numerous additional experiments aiming to optimize the described experiment from a clinical perspective. For instance, improvements in terms of shortening acquisition time and increasing the signal-to-noise ratio can be achieved at ultra-high field strengths (Pradhan et al., 2015), particularly with the use of double-tuned, multi-channel array coils (Rowland et al., 2020). Additionally, further investigation of the intensity and duration of the stimulation in order to understand the neurochemical mechanisms involved could also extend the impact of the tDCS. Apart from tDCS, future research may explore other transcranial electrical stimulation options, including transcranial alternating current stimulation (tACS), transcranial pulsed current stimulation (tPCS) or transcranial random noise stimulation (tRNS), to investigate neurochemical pathways in the brain. This feasibility study serves as a potential tool for expanding our understanding of the neurochemistry underlying energy metabolism and neuronal plasticity. Moreover, our experimental approach represents an encouraging nonpharmacological alternative to investigate altered neuronal plasticity and energy metabolism in neurological and metabolic disorders.
Limitations of the study
The current study is limited by the relatively short duration of the post-tDCS measurements, i.e., 67 min following tDCS. This narrow timeframe could be one possible reason for the absence of late significant recovery of energy phosphates. To address this shortcoming and to capture the late energy effects following tDCS, as shown in the study by Binkofski et al. (2011), future studies should aim to measure energy metabolites over a longer time. The follow-up period may not be sufficient to assess the long-term effects of tDCS. Longer-term studies are necessary to determine the durability of the observed changes and their potential implications for clinical applications. Moreover, as this feasibility study is only based on the motor cortex, it cannot be generalized to other brain regions. More studies are needed to explore other brain areas with similar quantitative and statistical investigations.
As studies using identical tDCS protocols have demonstrated different effects in healthy young adults (Alexandersen et al., 2022; Boayue et al., 2020; Willmot et al., 2024), the results obtained from this study are not one-to-one transferable to different age populations or patient groups. However, investigating inter-individual variability was not the aim of the study, and hence, subtle differences between individuals may have been concealed due to the small sample size. For future studies that aim to study inter-individual variability, a larger sample size would be useful for detecting subtle differences. One-to-one comparison with other studies is not possible due to differences in the method, material, acquisition protocols, etc. Further research is needed to investigate the relevance of calcium dynamics at deeper cortical layers and neighbouring brain regions to evaluate the effects of prolonged stimulation protocols. While we have proposed some reasonable explanations and possible molecular mechanisms underlying the aftereffects of prolonged tDCS duration, the precise neurobiological framework regarding neuroplasticity and energy metabolism remains unclear and requires further study.
Conclusion
We have demonstrated that it is possible to induce long lasting effects by anodal tDCS and measure the corresponding GABA and energy phosphate change in left primary motor cortex using proton and phosphorus magnetic resonance spectroscopy. Hence, this new approach may help to better understand the neurochemical mechanism underlying neurological and metabolic disorders.
Data availability statement
The raw data supporting the conclusions of this article will be made available by the authors, without undue reservation.
Ethics statement
The studies involving humans were approved by the Ethical committee of the Faculty of medicine, RWTH Aachen University. The studies were conducted in accordance with the local legislation and institutional requirements. The participants provided written informed consent to participate in this study.
Author contributions
HP: Writing – original draft, Writing – review & editing, Conceptualization, Data curation, Formal analysis, Investigation, Methodology, Project administration, Software. L-SS: Data curation, Writing – review & editing. C-HC: Data curation, Resources, Writing – review & editing. MN: Writing – review & editing. NS: Resources, Supervision, Writing – review & editing. FB: Conceptualization, Methodology, Resources, Supervision, Writing – review & editing.
Funding
The author(s) declare that no financial support was received for the research, authorship, and/or publication of this article.
Acknowledgments
The authors would like to thank Edward J. Auerbach and Małgorzata Marjańska from University of Minnesota for providing the CMRR (Center for Magnetic Resonance Research) spectroscopy package to run MEGA-PRESS and FASTMAP sequence. We also thank Ms. Claire Rick for English proofreading and Ms. Elene Iordanishvili for assisting in data collection.
Conflict of interest
Michael A. Nitsche is Scientific Advisory Boards of Neuroelectrics and Precisis.
The remaining authors declare that the research was conducted in the absence of any commercial or financial relationships that could be construed as a potential conflict of interest.
The author(s) declared that they were an editorial board member of Frontiers, at the time of submission. This had no impact on the peer review process and the final decision.
Publisher’s note
All claims expressed in this article are solely those of the authors and do not necessarily represent those of their affiliated organizations, or those of the publisher, the editors and the reviewers. Any product that may be evaluated in this article, or claim that may be made by its manufacturer, is not guaranteed or endorsed by the publisher.
References
Agboada, D., Mosayebi Samani, M., Jamil, A., Kuo, M. F., and Nitsche, M. A. (2019). Expanding the parameter space of anodal transcranial direct current stimulation of the primary motor cortex. Sci. Rep. 9:18185. doi: 10.1038/s41598-019-54621-0
Alexandersen, A., Csifcsák, G., Groot, J., and Mittner, M. (2022). The effect of transcranial direct current stimulation on the interplay between executive control, behavioral variability and mind wandering: a registered report. Neuroimage Rep. 2:100109. doi: 10.1016/j.ynirp.2022.100109
An, W. F., Bowlby, M. R., Betty, M., Cao, J., Ling, H. P., Mendoza, G., et al. (2000). Modulation of A-type potassium channels by a family of calcium sensors. Nature 403, 553–556. doi: 10.1038/35000592
Batsikadze, G., Moliadze, V., Paulus, W., Kuo, M. F., and Nitsche, M. A. (2013). Partially non-linear stimulation intensity-dependent effects of direct current stimulation on motor cortex excitability in humans. J. Physiol. 591, 1987–2000. doi: 10.1113/jphysiol.2012.249730
Baxter, C. F. (1970). “The nature of γ-aminobutyric acid” in Metabolic reactions in the nervous system. ed. A. Lajtha (Boston, MA: Springer US), 289–353.
Belelli, D., Harrison, N. L., Maguire, J., Macdonald, R. L., Walker, M. C., and Cope, D. W. (2009). Extrasynaptic GABAA receptors: form, pharmacology, and function. J. Neurosci. 29, 12757–12763. doi: 10.1523/JNEUROSCI.3340-09.2009
Bezalel, V., Paz, R., and Tal, A. (2019). Inhibitory and excitatory mechanisms in the human cingulate-cortex support reinforcement learning: a functional proton magnetic resonance spectroscopy study. NeuroImage 184, 25–35. doi: 10.1016/j.neuroimage.2018.09.016
Bhagwagar, Z., Rabiner, E. A., Sargent, P. A., Grasby, P. M., and Cowen, P. J. (2004). Persistent reduction in brain serotonin1A receptor binding in recovered depressed men measured by positron emission tomography with [11C]WAY-100635. Mol. Psychiatry 9, 386–392. doi: 10.1038/sj.mp.4001401
Binkofski, F., Loebig, M., Jauch-Chara, K., Bergmann, S., Melchert, U. H., Scholand-Engler, H. G., et al. (2011). Brain energy consumption induced by electrical stimulation promotes systemic glucose uptake. Biol. Psychiatry 70, 690–695. doi: 10.1016/j.biopsych.2011.05.009
Bjornebekk, A., Mathe, A. A., and Brene, S. (2005). The antidepressant effect of running is associated with increased hippocampal cell proliferation. Int. J. Neuropsychopharmacol. 8, 357–368. doi: 10.1017/S1461145705005122
Boayue, N. M., Csifcsak, G., Aslaksen, P., Turi, Z., Antal, A., Groot, J., et al. (2020). Increasing propensity to mind-wander by transcranial direct current stimulation? A registered report. Eur. J. Neurosci. 51, 755–780. doi: 10.1111/ejn.14347
Boy, E., Evans, C. J., Edden, R. A. E., Lawrence, A. D., Singh, K. D., Husain, M., et al. (2011). Dorsolateral prefrontal gamma-aminobutyric acid in men predicts individual differences in rash impulsivity. Biol. Psychiatry 70, 866–872. doi: 10.1016/j.biopsych.2011.05.030
Boy, E., Evans, C. J., Edden, R. A. E., Singh, K. D., Husain, M., and Sumner, P. (2010). Individual differences in subconscious motor control predicted by GABA concentration in SMA. Curr. Biol. 20, 1779–1785. doi: 10.1016/j.cub.2010.09.003
Choi, C. H., Iordanishvili, E., Shah, N. J., and Binkofski, F. (2021). Magnetic resonance spectroscopy with transcranial direct current stimulation to explore the underlying biochemical and physiological mechanism of the human brain: a systematic review. Hum. Brain Mapp. 42, 2642–2671. doi: 10.1002/hbm.25388
Cook, E., Hammett, S. T., and Larsson, J. (2016). GABA predicts visual intelligence. Neurosci. Lett. 632, 50–54. doi: 10.1016/j.neulet.2016.07.053
Coxon, J. P., Cash, R. F. H., Hendrikse, J. J., Rogasch, N. C., Stavrinos, E., Suo, C., et al. (2018). GABA concentration in sensorimotor cortex following high-intensity exercise and relationship to lactate levels. J. Physiol. 596, 691–702. doi: 10.1113/JP274660
Crabtree, G. W., and Gogos, J. A. (2014). Synaptic plasticity, neural circuits, and the emerging role of altered short-term information processing in schizophrenia. Front. Synaptic Neurosci. 6:28. doi: 10.3389/fnsyn.2014.00028
De Jong, S., Vidler, L. R., Mokrab, Y., Collier, D. A., and Breen, G. (2016). Gene-set analysis based on the pharmacological profiles of drugs to identify repurposing opportunities in schizophrenia. J. Psychopharmacol. 30, 826–830. doi: 10.1177/0269881116653109
Di Lazzaro, V., Profice, P., Pilato, F., Capone, F., Ranieri, F., Pasqualetti, P., et al. (2010). Motor cortex plasticity predicts recovery in acute stroke. Cereb. Cortex 20, 1523–1528. doi: 10.1093/cercor/bhp216
Drevets, W. C., Frank, E., Price, J. C., Kupfer, D. J., Holt, D., Greer, P. J., et al. (1999). PET imaging of serotonin 1A receptor binding in depression. Biol. Psychiatry 46, 1375–1387. doi: 10.1016/S0006-3223(99)00189-4
Duncan, N. W., Wiebking, C., and Northoff, G. (2014). Associations of regional GABA and glutamate with intrinsic and extrinsic neural activity in humans-a review of multimodal imaging studies. Neurosci. Biobehav. Rev. 47, 36–52. doi: 10.1016/j.neubiorev.2014.07.016
Edden, R. A., Muthukumaraswamy, S. D., Freeman, T. C., and Singh, K. D. (2009). Orientation discrimination performance is predicted by GABA concentration and gamma oscillation frequency in human primary visual cortex. J. Neurosci. 29, 15721–15726. doi: 10.1523/JNEUROSCI.4426-09.2009
Erickson, K. I., Voss, M. W., Prakash, R. S., Basak, C., Szabo, A., Chaddock, L., et al. (2011). Exercise training increases size of hippocampus and improves memory. Proc. Natl. Acad. Sci. USA 108, 3017–3022. doi: 10.1073/pnas.1015950108
Estrov, Y., Scaglia, F., and Bodamer, O. A. (2000). Psychiatric symptoms of inherited metabolic disease. J. Inherit. Metab. Dis. 23, 2–6. doi: 10.1023/A:1005685010766
Gaspar, H., and Breen, G. (2017). Drug enrichment and discovery from schizophrenia genome-wide association results: an analysis and visualisation approach. Sci. Rep. 7:12460. doi: 10.1038/s41598-017-12325-3
Gobel, B., Oltmanns, K. M., and Chung, M. (2013). Linking neuronal brain activity to the glucose metabolism. Theor. Biol. Med. Model. 10:50. doi: 10.1186/1742-4682-10-50
Green, R. C., Cupples, L. A., Kurz, A., Auerbach, S., Go, R., Sadovnick, D., et al. (2003). Depression as a risk factor for Alzheimer disease - the MIRAGE study. Arch. Neurol. 60, 753–759. doi: 10.1001/archneur.60.5.753
Gruetter, R., and Tkáč, I. (2000). Field mapping without reference scan using asymmetric echo-planar techniques. Mag. Resonan. Med. 43, 319–323. doi: 10.1002/(SICI)1522-2594(200002)43:2<319::AID-MRM22>3.0.CO;2-1
Grundey, J., Barlay, J., Batsikadze, G., Kuo, M. F., Paulus, W., and Nitsche, M. (2018). Nicotine modulates human brain plasticity via calcium-dependent mechanisms. J. Physiol. 596, 5429–5441. doi: 10.1113/JP276502
Grundman, M., Petersen, R. C., Ferris, S. H., Thomas, R. G., Aisen, P. S., Bennett, D. A., et al. (2004). Mild cognitive impairment can be distinguished from Alzheimer disease and normal aging for clinical trials. Arch. Neurol. 61, 59–66. doi: 10.1001/archneur.61.1.59
Haller, C. S., Padmanabhan, J. L., Lizano, P., Torous, J., and Keshavan, M. (2014). Recent advances in understanding schizophrenia. F1000prime Rep. 6:57. doi: 10.12703/P6-57
Hargreaves, M., and Spriet, L. L. (2020). Skeletal muscle energy metabolism during exercise. Nat. Metab. 2, 817–828. doi: 10.1038/s42255-020-0251-4
Harris, A. D., Saleh, M. G., and Edden, R. A. E. (2017). Edited H magnetic resonance spectroscopy in vivo: methods and metabolites. Magn. Reson. Med. 77, 1377–1389. doi: 10.1002/mrm.26619
Harrison, P. J. (1999). The neuropathology of schizophrenia - a critical review of the data and their interpretation. Brain 122, 593–624. doi: 10.1093/brain/122.4.593
Heimrath, K., Brechmann, A., Blobel-Luer, R., Stadler, J., Budinger, E., and Zaehle, T. (2020). Transcranial direct current stimulation (tDCS) over the auditory cortex modulates GABA and glutamate: a 7 T MR-spectroscopy study. Sci. Rep. 10:20111. doi: 10.1038/s41598-020-77111-0
Henning, A. (2018). Proton and multinuclear magnetic resonance spectroscopy in the human brain at ultra-high field strength: a review. NeuroImage 168, 181–198. doi: 10.1016/j.neuroimage.2017.07.017
Hikmah, D. N., Syarifuddin, A., Santoso, S. B., Wijayatri, R., and Hidayat, I. W. (2023). “The role of genetic mutation on schizophrenia: a basic review prior to pharmacogenomics.” in Paper presented at the 4th Borobudur International Symposium on Humanities and Social Science 2022 (BIS-HSS 2022).
Hirvonen, J., Karlsson, H., Kajander, J., Lepola, A., Markkula, J., Rasi-Hakala, H., et al. (2008). Decreased brain serotonin 5-HT1A receptor availability in medication-naive patients with major depressive disorder: an in-vivo imaging study using PET and [carbonyl-11C]WAY-100635. Int. J. Neuropsychopharmacol. 11, 465–476. doi: 10.1017/S1461145707008140
Holsinger, R. M., Schnarr, J., Henry, P., Castelo, V. T., and Fahnestock, M. (2000). Quantitation of BDNF mRNA in human parietal cortex by competitive reverse transcription-polymerase chain reaction: decreased levels in Alzheimer's disease. Brain Res. Mol. Brain Res. 76, 347–354. doi: 10.1016/S0169-328X(00)00023-1
Hulshoff Pol, H. E., and Kahn, R. S. (2008). What happens after the first episode? A review of progressive brain changes in chronically ill patients with schizophrenia. Schizophr. Bull. 34, 354–366. doi: 10.1093/schbul/sbm168
Itoh, M., and Uchimura, H. (1981). Regional differences in cofactor saturation of glutamate decarboxylase (GAD) in discrete brain nuclei of the rat. Effect of repeated administration of haloperidol on GAD activity in the substantia nigra. Neurochem. Res. 6, 1283–1289. doi: 10.1007/BF00964349
Jamil, A., Batsikadze, G., Kuo, H. I., Labruna, L., Hasan, A., Paulus, W., et al. (2017). Systematic evaluation of the impact of stimulation intensity on neuroplastic after-effects induced by transcranial direct current stimulation. J. Physiol. 595, 1273–1288. doi: 10.1113/JP272738
Jauch-Chara, K., Binkofski, F., Loebig, M., Reetz, K., Jahn, G., Melchert, U. H., et al. (2015). Blunted brain energy consumption relates to insula atrophy and impaired glucose tolerance in obesity. Diabetes 64, 2082–2091. doi: 10.2337/db14-0421
Jocham, G., Hunt, L. T., Near, J., and Behrens, T. E. (2012). A mechanism for value-guided choice based on the excitation-inhibition balance in prefrontal cortex. Nat. Neurosci. 15, 960–961. doi: 10.1038/nn.3140
Kondziella, D., Brenner, E., Eyjolfsson, E. M., and Sonnewald, U. (2007). How do glial–neuronal interactions fit into current neurotransmitter hypotheses of schizophrenia? Neurochem. Int. 50, 291–301. doi: 10.1016/j.neuint.2006.09.006
Kreis, R. (2016). The trouble with quality filtering based on relative Cramér-Rao lower bounds. Magn. Reson. Med. 75, 15–18. doi: 10.1002/mrm.25568
Kühn, S., Schubert, F., Mekle, R., Wenger, E., Ittermann, B., Lindenberger, U., et al. (2016). Neurotransmitter changes during interference task in anterior cingulate cortex: evidence from fMRI-guided functional MRS at 3 T. Brain Struct. Funct. 221, 2541–2551. doi: 10.1007/s00429-015-1057-0
Kulkarni, G., Murala, S., and Bollu, P. C. (2022). History of serotonin. Neurochem. Clin. Pract. 25, 25–43. doi: 10.1007/978-3-031-07897-2_2
Kuo, H. I., Bikson, M., Datta, A., Minhas, P., Paulus, W., Kuo, M. F., et al. (2013). Comparing cortical plasticity induced by conventional and high-definition 4 x 1 ring tDCS: a neurophysiological study. Brain Stimul. 6, 644–648. doi: 10.1016/j.brs.2012.09.010
Lau, C. G., and Murthy, V. N. (2012). Activity-dependent regulation of inhibition via GAD67. J. Neurosci. 32, 8521–8531. doi: 10.1523/JNEUROSCI.1245-12.2012
Lau, C. G., and Zukin, R. S. (2007). NMDA receptor trafficking in synaptic plasticity and neuropsychiatric disorders. Nat. Rev. Neurosci. 8, 413–426. doi: 10.1038/nrn2153
Lee, K. H., Shin, J., Lee, J., Yoo, J. H., Kim, J.-W., and Brent, D. A. (2023). Measures of connectivity and dorsolateral prefrontal cortex volumes and depressive symptoms following treatment with selective serotonin reuptake inhibitors in adolescents. JAMA Netw. Open 6:e2327331. doi: 10.1001/jamanetworkopen.2023.27331
Liddle, P., Friston, K., Frith, C., and Frackowiak, R. (1992a). Cerebral blood flow and mental processes in schizophrenia. J. R. Soc. Med. 85, 224–227. doi: 10.1177/014107689208500415
Liddle, P., Friston, K., Frith, C., Hirsch, S., Jones, T., and Frackowiak, R. (1992b). Patterns of cerebral blood flow in schizophrenia. Br. J. Psychiatry 160, 179–186. doi: 10.1192/bjp.160.2.179
Lisman, J. E. (2001). Three Ca2+ levels affect plasticity differently: the LTP zone, the LTD zone and no man's land. J. Physiol. London 532:285. doi: 10.1111/j.1469-7793.2001.0285f.x
Liu, P. Z., and Nusslock, R. (2018). Exercise-mediated neurogenesis in the Hippocampus via BDNF. Front. Neurosci. 12:52. doi: 10.3389/fnins.2018.00052
Loprinzi, P. D., and Frith, E. (2019). A brief primer on the mediational role of BDNF in the exercise-memory link. Clin. Physiol. Funct. Imaging 39, 9–14. doi: 10.1111/cpf.12522
Maddock, R. J., Casazza, G. A., Fernandez, D. H., and Maddock, M. I. (2016). Acute modulation of cortical glutamate and GABA content by physical activity. J. Neurosci. 36, 2449–2457. doi: 10.1523/JNEUROSCI.3455-15.2016
Martin, D. L., and Rimvall, K. (1993). Regulation of gamma-aminobutyric acid synthesis in the brain. J. Neurochem. 60, 395–407. doi: 10.1111/j.1471-4159.1993.tb03165.x
McAllister, A. K., Katz, L. C., and Lo, D. C. (1999). Neurotrophins and synaptic plasticity. Annu. Rev. Neurosci. 22, 295–318. doi: 10.1146/annurev.neuro.22.1.295
McIntyre, R. S., Xiao, H. X., Syeda, K., Vinberg, M., Carvalho, A. F., Mansur, R. B., et al. (2015). The prevalence, measurement, and treatment of the cognitive dimension/domain in major depressive disorder. CNS Drugs 29, 577–589. doi: 10.1007/s40263-015-0263-x
Mednick, S. C., McDevitt, E. A., Walsh, J. K., Wamsley, E., Paulus, M., Kanady, J. C., et al. (2013). The critical role of sleep spindles in hippocampal-dependent memory: a pharmacology study. J. Neurosci. 33, 4494–4504. doi: 10.1523/JNEUROSCI.3127-12.2013
Meeley, M. P., and Martin, D. L. (1983). Inactivation of brain glutamate decarboxylase and the effects of adenosine 5′-triphosphate and inorganic phosphate. Cell. Mol. Neurobiol. 3, 39–54. doi: 10.1007/BF00734997
Mescher, M., Merkle, H., Kirsch, J., Garwood, M., and Gruetter, R. (1998). Simultaneous in vivo spectral editing and water suppression. NMR Biomed. 11, 266–272. doi: 10.1002/(SICI)1099-1492(199810)11:6<266::AID-NBM530>3.0.CO;2-J
Mezuk, B., Eaton, W. W., Albrecht, S., and Golden, S. H. (2008). Depression and type 2 diabetes over the lifespan: a meta-analysis. Diabetes Care 31, 2383–2390. doi: 10.2337/dc08-0985
Millan, M. J., Agid, Y., Brune, M., Bullmore, E. T., Carter, C. S., Clayton, N. S., et al. (2012). Cognitive dysfunction in psychiatric disorders: characteristics, causes and the quest for improved therapy. Nat. Rev. Drug Discov. 11, 141–168. doi: 10.1038/nrd3628
Miller, L. P., Walters, J. R., Eng, N., and Martin, D. L. (1980). Glutamate holodecarboxylase levels and the regulation of GABA synthesis. Brain Res. Bull. 5, 89–94. doi: 10.1016/0361-9230(80)90014-3
Miller, L. P., Walters, J. R., and Martin, D. L. (1977). Post-mortem changes implicate adenine nucleotides and pyridoxal-5′ -phosphate in regulation of brain glutamate decarboxylase. Nature 266, 847–848. doi: 10.1038/266847a0
Misonou, H., Mohapatra, D. P., Park, E. W., Leung, V., Zhen, D., Misonou, K., et al. (2004). Regulation of ion channel localization and phosphorylation by neuronal activity. Nat. Neurosci. 7, 711–718. doi: 10.1038/nn1260
Moghaddam, B., Adams, B., Verma, A., and Daly, D. (1997). Activation of glutamatergic neurotransmission by ketamine: a novel step in the pathway from NMDA receptor blockade to dopaminergic and cognitive disruptions associated with the prefrontal cortex. J. Neurosci. 17, 2921–2927. doi: 10.1523/JNEUROSCI.17-08-02921.1997
Moliadze, V., Atalay, D., Antal, A., and Paulus, W. (2012). Close to threshold transcranial electrical stimulation preferentially activates inhibitory networks before switching to excitation with higher intensities. Brain Stimul. 5, 505–511. doi: 10.1016/j.brs.2011.11.004
Monte-Silva, K., Kuo, M. F., Hessenthaler, S., Fresnoza, S., Liebetanz, D., Paulus, W., et al. (2013). Induction of late LTP-like plasticity in the human motor cortex by repeated non-invasive brain stimulation. Brain Stimul. 6, 424–432. doi: 10.1016/j.brs.2012.04.011
Moriarty, T., Bourbeau, K., Bellovary, B., and Zuhl, M. N. (2019). Exercise intensity influences prefrontal cortex oxygenation during cognitive testing. Behav. Sci. 9:83. doi: 10.3390/bs9080083
Moriya, M., Aoki, C., and Sakatani, K. (2016). “Effects of physical exercise on working memory and prefrontal cortex function in post-stroke patients.” in Paper presented at the Oxygen Transport to Tissue XXXVIII.
Mugruza-Vassallo, C., and Potter, D. (2019). Context dependence signature, stimulus properties and stimulus probability as predictors of ERP amplitude variability. Front. Hum. Neurosci. 13:39. doi: 10.3389/fnhum.2019.00039
Mullins, P. G., McGonigle, D. J., O'Gorman, R. L., Puts, N. A., Vidyasagar, R., Evans, C. J., et al. (2014). Current practice in the use of MEGA-PRESS spectroscopy for the detection of GABA. NeuroImage 86, 43–52. doi: 10.1016/j.neuroimage.2012.12.004
Nanda, B., Balde, J., and Manjunatha, S. (2013). The acute effects of a single bout of moderate-intensity aerobic exercise on cognitive functions in healthy adult males. J. Clin. Diagn. Res. 7, 1883–1885. doi: 10.7860/JCDR/2013/5855.3341
Nitsche, M. A., Fricke, K., Henschke, U., Schlitterlau, A., Liebetanz, D., Lang, N., et al. (2003). Pharmacological modulation of cortical excitability shifts induced by transcranial direct current stimulation in humans. J. Physiol. 553, 293–301. doi: 10.1113/jphysiol.2003.049916
Nitsche, M. A., and Paulus, W. (2000). Excitability changes induced in the human motor cortex by weak transcranial direct current stimulation. J. Physiol. 527, 633–639. doi: 10.1111/j.1469-7793.2000.t01-1-00633.x
Novak, J., Wilson, M., MacPherson, L., Arvanitis, T. N., Davies, N. P., and Peet, A. C. (2014). Clinical protocols for 31P MRS of the brain and their use in evaluating optic pathway gliomas in children. Eur. J. Radiol. 83, e106–e112. doi: 10.1016/j.ejrad.2013.11.009
Oldfield, R. C. (1971). The assessment and analysis of handedness: the Edinburgh inventory. Neuropsychologia, 9, 97–113.
Olney, J. W., Newcomer, J. W., and Farber, N. B. (1999). NMDA receptor hypofunction model of schizophrenia. J. Psychiatr. Res. 33, 523–533. doi: 10.1016/S0022-3956(99)00029-1
Öz, G., Alger, J. R., Barker, P. B., Bartha, R., Bizzi, A., Boesch, C., et al. (2014). Clinical proton MR spectroscopy in central nervous system disorders. Radiology 270, 658–679. doi: 10.1148/radiol.13130531
Patel, H. J., Romanzetti, S., Pellicano, A., Nitsche, M. A., Reetz, K., and Binkofski, F. (2019). Proton magnetic resonance spectroscopy of the motor cortex reveals long term GABA change following anodal transcranial direct current stimulation. Sci. Rep. 9:2807. doi: 10.1038/s41598-019-39262-7
Peek, A. L., Rebbeck, T. J., Leaver, A. M., Foster, S. L., Refshauge, K. M., Puts, N. A., et al. (2023). A comprehensive guide to MEGA-PRESS for GABA measurement. Anal. Biochem. 669:115113. doi: 10.1016/j.ab.2023.115113
Penninx, B. W., and Lange, S. M. (2018). Metabolic syndrome in psychiatric patients: overview, mechanisms, and implications. Dialogues Clin. Neurosci. 20, 63–73. doi: 10.31887/DCNS.2018.20.1/bpenninx
Petroff, O. A. (2002). Book review: GABA and glutamate in the human brain. Neuroscientist 8, 562–573. doi: 10.1177/1073858402238515
Piepmeier, A. T., and Etnier, J. L. (2015). Brain-derived neurotrophic factor (BDNF) as a potential mechanism of the effects of acute exercise on cognitive performance. J. Sport Health Sci. 4, 14–23. doi: 10.1016/j.jshs.2014.11.001
Porges, E. C., Woods, A. J., Edden, R. A., Puts, N. A., Harris, A. D., Chen, H., et al. (2017). Frontal gamma-aminobutyric acid concentrations are associated with cognitive performance in older adults. Biol. Psychiatry 2, 38–44. doi: 10.1016/j.bpsc.2016.06.004
Porter, T. G., and Martin, D. L. (1988). Stability and activation of glutamate apodecarboxylase from pig brain. J. Neurochem. 51, 1886–1891. doi: 10.1111/j.1471-4159.1988.tb01173.x
Pradhan, S., Bonekamp, S., Gillen, J. S., Rowland, L. M., Wijtenburg, S. A., Edden, R. A., et al. (2015). Comparison of single voxel brain MRS AT 3 T and 7 T using 32-channel head coils. Magn. Reson. Imaging 33, 1013–1018. doi: 10.1016/j.mri.2015.06.003
Provencher, S. W. (1993). Estimation of metabolite concentrations from localized in vivo proton NMR spectra. Magn. Reson. Med. 30, 672–679. doi: 10.1002/mrm.1910300604
Purpura, D. P., and McMurtry, J. G. (1965). Intracellular activities and evoked potential changes during polarization of motor cortex. J. Neurophysiol. 28, 166–185. doi: 10.1152/jn.1965.28.1.166
Puts, N. A., Edden, R. A., Evans, C. J., McGlone, F., and McGonigle, D. J. (2011). Regionally specific human GABA concentration correlates with tactile discrimination thresholds. J. Neurosci. 31, 16556–16560. doi: 10.1523/JNEUROSCI.4489-11.2011
Rae, C., Hare, N., Bubb, W. A., McEwan, S. R., Bröer, A., McQuillan, J. A., et al. (2003). Inhibition of glutamine transport depletes glutamate and GABA neurotransmitter pools:: further evidence for metabolic compartmentation. J. Neurochem. 85, 503–514. doi: 10.1046/j.1471-4159.2003.01713.x
Ramasubbu, R., and Patten, S. B. (2003). Effect of depression on stroke morbidity and mortality. Can. J. Psychiatr. 48, 250–257. doi: 10.1177/070674370304800409
Rao, C. R. (1947). “Minimum variance and the estimation of several parameters.” in Paper presented at the Mathematical Proceedings of the Cambridge Philosophical Society. 43, 280, 283.
Reichenberg, A. (2010). The assessment of neuropsychological functioning in schizophrenia. Dialogues Clin. Neurosci. 12, 383–392. doi: 10.31887/DCNS.2010.12.3/areichenberg
Rodriguez-Lopez, J., Arrojo, M., Paz, E., Paramo, M., and Costas, J. (2020). Identification of relevant hub genes for early intervention at gene coexpression modules with altered predicted expression in schizophrenia. Prog. Neuro-Psychopharmacol. Biol. Psychiatry 98:109815. doi: 10.1016/j.pnpbp.2019.109815
Rossini, P. M., Barker, A., Berardelli, A., Caramia, M., Caruso, G., Cracco, R., et al. (1994). Non-invasive electrical and magnetic stimulation of the brain, spinal cord and roots: basic principles and procedures for routine clinical application. Report of an IFCN committee. Electroencephalogr. Clin. Neurophysiol. 91, 79–92. doi: 10.1016/0013-4694(94)90029-9
Rowland, B. C., Driver, I. D., Tachrount, M., Klomp, D. W., Rivera, D., Forner, R., et al. (2020). Whole brain 31P MRSI at 7T with a dual-tuned receive array. Magn. Reson. Med. 83, 765–775. doi: 10.1002/mrm.27953
Ruby, E., Polito, S., McMahon, K., Gorovitz, M., Corcoran, C., and Malaspina, D. (2014). Pathways associating childhood trauma to the neurobiology of schizophrenia. Front. Psychol. Behav. Sci. 3, 1–17. Available at: https://pubmed.ncbi.nlm.nih.gov/25419548/
Saini, S., Mancuso, S., Mostaid, M. S., Liu, C., Pantelis, C., Everall, I., et al. (2017). Meta-analysis supports GWAS-implicated link between GRM3 and schizophrenia risk. Transl. Psychiatry 7:e1196. doi: 10.1038/tp.2017.172
Sanacora, G., Gueorguieva, R., Epperson, C. N., Wu, Y.-T., Appel, M., Rothman, D. L., et al. (2004). Subtype-specific alterations of γ-aminobutyric acid and glutamatein patients with major depression. Arch. Gen. Psychiatry 61, 705–713. doi: 10.1001/archpsyc.61.7.705
Sanacora, M., Mason, G. F., Rothman, D. L., Behar, K. L., Hyder, F., Petroff, O. A. C., et al. (1999). Reduced cortical gamma-aminobutyric acid levels in depressed patients determined by proton magnetic resonance spectroscopy. Arch. Gen. Psychiatry 56, 1043–1047. doi: 10.1001/archpsyc.56.11.1043
Sanacora, G., Rothman, D. L., Mason, G., and Krystal, J. H. (2003). Clinical studies implementing glutamate neurotransmission in mood disorders. Ann. N. Y. Acad. Sci. 1003, 292–308. doi: 10.1196/annals.1300.018
Sandroff, B. M., Hillman, C. H., Benedict, R. H., and Motl, R. W. (2015). Acute effects of walking, cycling, and yoga exercise on cognition in persons with relapsing-remitting multiple sclerosis without impaired cognitive processing speed. J. Clin. Exp. Neuropsychol. 37, 209–219. doi: 10.1080/13803395.2014.1001723
Sargent, P. A., Kjaer, K. H., Bench, C. J., Rabiner, E. A., Messa, C., Meyer, J., et al. (2000). Brain serotonin1A receptor binding measured by positron emission tomography with [11C]WAY-100635: effects of depression and antidepressant treatment. Arch. Gen. Psychiatry 57, 174–180. doi: 10.1001/archpsyc.57.2.174
Shelp, B. J., Mullen, R. T., and Waller, J. C. (2012). Compartmentation of GABA metabolism raises intriguing questions. Trends Plant Sci. 17, 57–59. doi: 10.1016/j.tplants.2011.12.006
Shibata, K., Sasaki, Y., Bang, J. W., Walsh, E. G., Machizawa, M. G., Tamaki, M., et al. (2017). Overlearning hyperstabilizes a skill by rapidly making neurochemical processing inhibitory-dominant. Nat. Neurosci. 20, 470–475. doi: 10.1038/nn.4490
Sibley, B. A., Etnier, J. L., and Le Masurier, G. C. (2006). Effects of an acute bout of exercise on cognitive aspects of Stroop performance. J. Sport Exerc. Psychol. 28, 285–299. doi: 10.1123/jsep.28.3.285
Soares, M. J., Cummings, N. K., and Ping-Delfos, W. L. C. S. (2011). Energy metabolism and the metabolic syndrome: does a lower basal metabolic rate signal recovery following weight loss? Diabetes Metab. Syndr. Clin. Res. Rev. 5, 98–101. doi: 10.1016/j.dsx.2012.03.003
Stagg, B., Bachtiar, V., and Johansen-Berg, H. (2011). The role of GABA in human motor learning. Curr. Biol. 21, 480–484. doi: 10.1016/j.cub.2011.01.069
Stagg, B., Best, J. G., Stephenson, M. C., O'Shea, J., Wylezinska, M., Kincses, Z. T., et al. (2009). Polarity-sensitive modulation of cortical neurotransmitters by transcranial stimulation. J. Neurosci. 29, 5202–5206. doi: 10.1523/JNEUROSCI.4432-08.2009
Stagg, C. J., and Johansen-Berg, H. (2013). Studying the effects of transcranial direct-current stimulation in stroke recovery using magnetic resonance imaging. Front. Hum. Neurosci. 7:857. doi: 10.3389/fnhum.2013.00857
Statton, M. A., Encarnacion, M., Celnik, P., and Bastian, A. J. (2015). A single bout of moderate aerobic exercise improves motor skill acquisition. PLoS One 10:e0141393. doi: 10.1371/journal.pone.0141393
Stone, J. M., Day, F., Tsagaraki, H., Valli, I., McLean, M. A., Lythgoe, D. J., et al. (2009). Glutamate dysfunction in people with prodromal symptoms of psychosis: relationship to gray matter volume. Biol. Psychiatry 66, 533–539. doi: 10.1016/j.biopsych.2009.05.006
Sumner, P., Edden, R. A., Bompas, A., Evans, C. J., and Singh, K. D. (2010). More GABA, less distraction: a neurochemical predictor of motor decision speed. Nat. Neurosci. 13, 825–827. doi: 10.1038/nn.2559
Sze, P. Y., Sullivan, P., Alderson, R. F., and Towle, A. C. (1983). ATP binding to brain l-glutamate decarboxylase: a study by affinity chromatography. Neurochem. Int. 5, 51–56. doi: 10.1016/0197-0186(83)90008-6
Tamminga, C. A. (1999). Schizophrenia in a molecular age. Washington, DC, USA: American Psychiatric Press, Inc.
Van der Kooy, K., van Hout, H., Marwijk, H., Marten, H., Stehouwer, C., and Beekman, A. (2007). Depression and the risk for cardiovascular diseases: systematic review and meta analysis. Int. J. Geriatr. Psychiatry 22, 613–626. doi: 10.1002/gps.1723
van Vugt, F. T., Near, J., Hennessy, T., Doyon, J., and Ostry, D. J. (2020). Early stages of sensorimotor map acquisition: neurochemical signature in primary motor cortex and its relation to functional connectivity. J. Neurophysiol. 124, 1615–1624. doi: 10.1152/jn.00285.2020
Vasques, P. E., Moraes, H., Silveira, H., Deslandes, A. C., and Laks, J. (2011). Acute exercise improves cognition in the depressed elderly: the effect of dual-tasks. Clinics 66, 1553–1557. doi: 10.1590/S1807-59322011000900008
Wang, J., D’Amato, A., Bambrough, J., Swartz, A. M., and Miller, N. E. (2016). A positive association between active lifestyle and hemispheric lateralization for motor control and learning in older adults. Behav. Brain Res. 314, 38–44. doi: 10.1016/j.bbr.2016.07.048
Wenger, K. J., Wagner, M., Harter, P. N., Franz, K., Bojunga, J., Fokas, E., et al. (2020). Maintenance of energy homeostasis during calorically restricted ketogenic diet and fasting-MR-spectroscopic insights from the ERGO2 trial. Cancer 12:3549. doi: 10.3390/cancers12123549
Werner, F.-M., and Coveñas, R. (2013). Classical neurotransmitters and neuropeptides involved in schizophrenia: how to choose the appropriate antipsychotic drug? Curr. Drug Therapy 8, 132–143. doi: 10.2174/15748855113089990003
Werner, F.-M., and Covenas, R. (2016). Classical neurotransmitters and neuropeptides involved in schizoaffective disorder: Focus on prophylactic medication. Saif Zone Sharjah, U.A.E: Bentham Science Publishers.
Willmot, N., Leow, L. A., Filmer, H. L., and Dux, P. E. (2024). Exploring the intra-individual reliability of tDCS: a registered report. Cortex 173, 61–79. doi: 10.1016/j.cortex.2023.12.015
Wilson, M., Andronesi, O., Barker, P. B., Bartha, R., Bizzi, A., Bolan, P. J., et al. (2019). Methodological consensus on clinical proton MRS of the brain: Review and recommendations. Magn. Reson. Med. 82, 527–550. doi: 10.1002/mrm.27742
Wilson, M., Reynolds, G., Kauppinen, R. A., Arvanitis, T. N., and Peet, A. C. (2011). A constrained least-squares approach to the automated quantitation of in vivo 1H magnetic resonance spectroscopy data. Magn. Reson. Med. 65, 1–12. doi: 10.1002/mrm.22579
Wu, S. J., and Martin, D. L. (1984). Binding of Atp to brain glutamate-decarboxylase as studied by affinity-chromatography. J. Neurochem. 42, 1607–1612. doi: 10.1111/j.1471-4159.1984.tb12749.x
Yoon, J. H., Grandelis, A., and Maddock, R. J. (2016). Dorsolateral prefrontal cortex GABA concentration in humans predicts working memory load processing capacity. J. Neurosci. 36, 11788–11794. doi: 10.1523/JNEUROSCI.1970-16.2016
Zhang, J., Yetton, B., Whitehurst, L. N., Naji, M., and Mednick, S. C. (2020). The effect of zolpidem on memory consolidation over a night of sleep. Sleep 43:zsaa084. doi: 10.1093/sleep/zsaa084
Zuccoli, G. S., Saia-Cereda, V. M., Nascimento, J. M., and Martins-de-Souza, D. (2017). The energy metabolism dysfunction in psychiatric disorders postmortem brains: focus on proteomic evidence. Front. Neurosci. 11:493. doi: 10.3389/fnins.2017.00493
Glossary
Keywords: tDCS, GABA, 31PMRS, 1HMRS, primary motor cortex, neuroplasticity, energy metabolism
Citation: Patel HJ, Stollberg L-S, Choi C-H, Nitsche MA, Shah NJ and Binkofski F (2024) A study of long-term GABA and high-energy phosphate alterations in the primary motor cortex using anodal tDCS and 1H/31P MR spectroscopy. Front. Hum. Neurosci. 18:1461417. doi: 10.3389/fnhum.2024.1461417
Edited by:
Samar S. Ayache, Hôpitaux Universitaires Henri Mondor, FranceReviewed by:
Yuhei Takado, National Institutes for Quantum and Radiological Science and Technology (Japan), JapanCarlos Andrés Mugruza Vassallo, San Juan Bautista Private University, Peru
Copyright © 2024 Patel, Stollberg, Choi, Nitsche, Shah and Binkofski. This is an open-access article distributed under the terms of the Creative Commons Attribution License (CC BY). The use, distribution or reproduction in other forums is permitted, provided the original author(s) and the copyright owner(s) are credited and that the original publication in this journal is cited, in accordance with accepted academic practice. No use, distribution or reproduction is permitted which does not comply with these terms.
*Correspondence: Ferdinand Binkofski, ZmJpbmtvZnNraUB1a2FhY2hlbi5kZQ==