- 1Motion and Gait Analysis Lab, Lucile Packard Children’s Hospital, Stanford Medicine Children’s Health, Palo Alto, CA, United States
- 2Department of Mechanical Engineering, Rice University, Houston, TX, United States
- 3Department of Civil and Environmental Engineering, Stanford University, Stanford, CA, United States
- 4Department of Orthopedic Surgery, Stanford University, Stanford, CA, United States
Identification of neuromuscular impairments in cerebral palsy (CP) is essential to providing effective treatment. However, clinical recognition of neuromuscular impairments in CP and their contribution to gait abnormalities is limited, resulting in suboptimal treatment outcomes. While CP is the most common childhood movement disorder, clinical evaluations often do not accurately identify and delineate the primary neuromuscular and secondary musculoskeletal impairments or their specific impact on mobility. Here we discuss the primary neuromuscular impairments of CP that arise from early brain injury and the progressive secondary musculoskeletal impairments, with a focus on spastic CP, the most common form of CP. Spastic CP is characterized by four primary interrelated neuromuscular impairments: 1. muscle weakness, 2. short muscle-tendon units due to slow muscle growth relative to skeletal growth, 3. muscle spasticity characterized by increased sensitivity to stretch, and 4. impaired selective motor control including flexor and extensor muscle synergies. Specific gait events are affected by the four primary neuromuscular impairments of spastic CP and their delineation can improve evaluation to guide targeted treatment, prevent deformities and improve mobility. Emerging information on neural correlates of neuromuscular impairments in CP provides the clinician with a more complete context with which to evaluate and develop effective treatment plans. Specifically, addressing the primary neuromuscular impairments and reducing secondary musculoskeletal impairments are important treatment goals. This perspective on neuromuscular mechanisms underlying gait abnormalities in spastic CP aims to inform clinical evaluation of CP, focus treatment more strategically, and guide research priorities to provide targeted treatments for CP.
Introduction
Cerebral palsy (CP) is the most common movement disorder in children, with prevalence of 2–3/1,000 in general population and higher among preterm children (Oskoui et al., 2013). CP is diagnosed in young children based on abnormal posture or tone, delayed motor milestones, and gait abnormalities (Wu et al., 2004; Zhou et al., 2017). Typically gait stabilizes around age 4 years (Sutherland, 1997), highlighting importance of early identification and treatment. Spastic CP, the most common form of CP (Sellier et al., 2016), is characterized by four interrelated primary neuromuscular impairments: 1. muscle weakness, 2. short muscle-tendon units from slow muscle growth relative to skeletal growth, 3. muscle spasticity, and 4. impaired selective motor control (SMC). Identification neuromuscular impairments and their impact on gait can guide targeted treatment, as recent research indicates current treatment outcomes are modest (Schwartz et al., 2022) and more effective treatments are needed.
While 3D gait analysis is the gold standard for evaluating gait abnormalities in spastic CP (Gage and Novacheck, 2001), too often neuromuscular impairments of CP are not directly addressed. It is vital that clinical evaluation of CP systematically address impacts of primary neuromuscular and secondary musculoskeletal impairments on gait to target treatments. Here we discuss emerging information on neural correlates of neuromuscular impairments in spastic CP and their contribution to gait abnormalities to provide clinicians with more complete context to evaluate patients, develop effective treatment plans, and guide future research priorities.
Brain injury in cerebral palsy
Cerebral palsy describes “a group of permanent disorders affecting the development of movement and posture, causing activity limitation, that are attributed to non-progressive disturbances that occurred in the developing fetal or infant brain” (Rosenbaum et al., 2007). While the initial brain injury is not progressive, the secondary musculoskeletal impairments and the impacts of the neuromuscular impairments on mobility are progressive (Bell et al., 2002; Sanger, 2015). The most common cause of CP is periventricular leukomalacia (PVL) with prematurity, intracerebral hemorrhage, and hydrocephalus (Metz et al., 2022). Other causes of brain injury include infection, metabolic disorders, brain malformation, and bilirubin neurotoxicity (Paneth et al., 2006; Strijbis et al., 2006; Ellenberg and Nelson, 2013; Zhou et al., 2017). Increased risk for CP is associated with multiple-births, such as twins and triplets (Sellier et al., 2021) and adverse neonatal outcomes are more common in males born preterm (Reid S. et al., 2016). Higher prevalence of CP with prematurity is in large part due to selective vulnerability to injury of developing white matter (neuron axons), during early phases of vascularization and white matter myelination (Inder and Volpe, 2000; Hoon et al., 2009). Approximately 30–80% of CP diagnoses with perinatal cause are secondary to genetic risk factors (Costeff, 2004; Moreno-De-Luca et al., 2012; MacLennan et al., 2015; Emrick and DiCarlo, 2020) and genetic testing is increasingly recommended (Pham et al., 2020; Emrick and DiCarlo, 2020; Gonzalez-Mantilla et al., 2023).
Understanding the relevant neuroanatomy can assist with understanding pathophysiology of impairments resulting from the brain injury in CP. The cortical homunculus (Figure 1) illustrates the topographic representation of body regions in the cerebral cortex grey matter (neuron cell bodies) motor and sensory brain regions and descending white matter tracts (Nguyen and Duong, 2023). The corticospinal tract is the primary voluntary motor tract and originates in the cerebral cortex and descends in the pyramidal tract, named for its cross-sectional shape. In the medulla, about 75–90% of the fibers decussate, crossing over to create the lateral corticospinal tract, while the remaining fibers continue to descend making up the anterior corticospinal tract (Figure 1; Natali et al., 2018). The rubrospinal tract is one of several extra-pyramidal motor tracts that mediate involuntary movement; it originates in the red nucleus of the midbrain with decussation at the level of the midbrain (Figure 1; Lee and Muzio, 2020). The basal ganglia also mediate motor control and includes the caudate, putamen, and globus pallidus nuclei. The lateral ventricles are part of a system of ventricles that contain cerebral spinal fluid and provide nutrition, eliminate waste and protect the central nervous system (Scelsi et al., 2020).
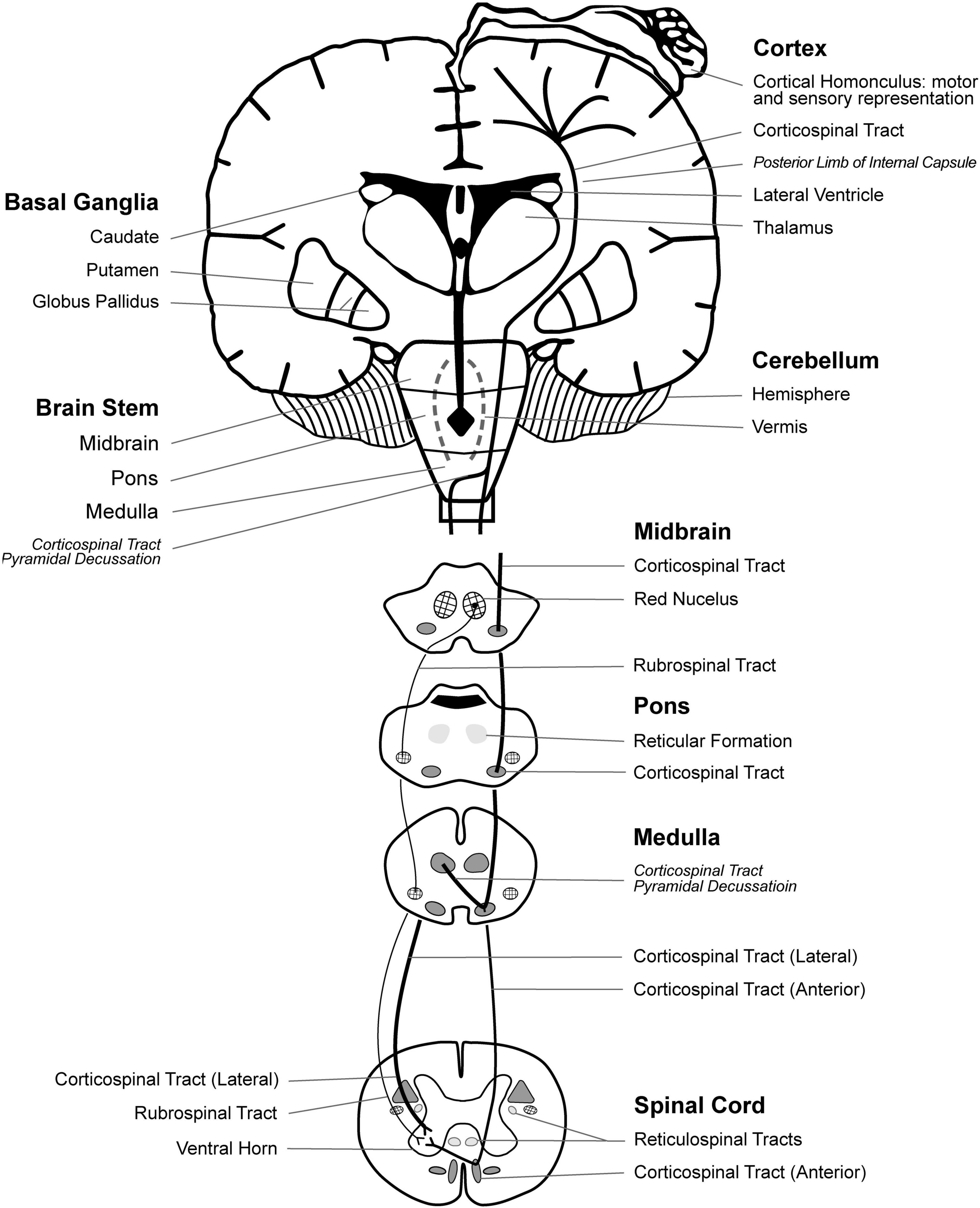
Figure 1. Neuroanatomical representation of brain motor regions, white matter tracts, and the motor and sensory homunculus. Revised with permission from Zhou et al. (2017).
Three types of CP, spastic, dyskinetic, and ataxic, arise from different regional brain injury. Spastic CP, the most common, affects approximately 87% of children with CP, while dyskinetic CP affects 7–15%, and ataxic CP affects 4% of children with CP (Sellier et al., 2016; Kitai et al., 2021). The different types of CP can co-exist but have characteristic motor deficits associated with specific regional brain injury.
Spastic CP is linked to damage to the corticospinal tract (CST) periventricular white matter due to prenatal hypoxia-ischemia (Liu et al., 2024). In addition, involvement of sensory-motor regions may further impair proprioception and motor function in spastic CP (Hoon et al., 2009). Spastic CP is further delineated by distribution of affected limbs: unilateral or bilateral.
Spastic CP is characterized by four primary interrelated neuromuscular impairments: 1. muscle weakness 2. short muscle-tendon units due to slow muscle growth relative to skeletal growth 3. muscle spasticity characterized by increased sensitivity to stretch, and 4. impaired selective motor control including flexor and extensor muscle synergies. Muscle weakness and short muscle-tendon unit result from reduced descending neural activation while muscle spasticity and impaired selective motor control (SMC) are thought to result from reduced descending inhibition.
Less severe involvement in spastic CP primarily affects distal joints, whereas more severe involvement affects distal and proximal joints, consistent with extent of brain injury and anatomical representation of cortical homunculus and descending motor tracts (Figure 1; Serdaroglu et al., 2004). Accordingly, Staudt et al. (2000) found correlations between lower limb motor function and extent of lateral lesion in the posterior semi-coronal plane on MRI. Recent evidence indicates motor impairments of spastic CP may be linked to both primary and downstream secondary abnormalities in the somatosensory cortex (Liu et al., 2024)
Neural correlates of gait abnormalities have been investigated in high-risk neonates and children with CP. Currently, structural MRI is standard for identifying neural injury in CP, however brain injuries are not always visible on structural MRI: a review by Reid et al. (2014) revealed 14% of children diagnosed with CP show no signs of brain injury on MRI, particularly in children with ataxic CP (Bax et al., 2006). Diffusion tensor imaging (DTI) provides more precise data on white matter tracts (Reid L. B. et al., 2016). Rose et al. (2007) found that near-term brain microstructure of posterior limb of internal capsule (PLIC) (Figure 1) on DTI in preterm neonates correlated with severity of gait abnormalities at 4 years, assessed with GMFCS and 3D gait kinematics. Rose et al. (2015) also reported that near-term cerebellar macrostructure on MRI, and PLIC and corpus callosum genu microstructure on DTI were predictive of neurodevelopment in toddlers assessed with Bayley Scales of infant Development and by gait temporospatial parameters. Meyns et al. (2016) found that severity of gait abnormality assessed using the Gait Profile Score correlated to total corpus callosum and subpart 1 volumes in children with CP. The Gait Profile Score is a measure based on kinematic parameters which compares the gait pattern of an individual to the mean pattern of normative typically developing group (Christian et al., 2017), similar to the Gait Deviation Index (Schwartz and Rozumalski, 2008). Fiori et al. (2014, 2015) developed a semiquantitative MRI score that correlated with severity of bilateral and unilateral CP as assessed with GMFCS. In a later study of unilateral CP, the semiquantitative MRI score correlated with gross manual dexterity as assessed with Manual Ability Classification Scale and Box and Block Score (Beani et al., 2024). These studies of brain structure-function relationships indicate a potential to identify young children at high risk for motor impairments that could guide early treatments.
Dyskinetic CP, the second most common type of CP, (Sellier et al., 2016; Kitai et al., 2021) is linked to damage of subcortical grey matter, including lower volumes of basal ganglia and thalamus, due to hypoxia-ischemia, as well as hyperbilirubinemia and birth asphyxia (Laporta-Hoyos et al., 2014; Himmelmann and Uvebrant, 2011; Kitai et al., 2021). The definition of dyskinetic CP has evolved to include dystonic and choreoathetoid CP (Aravamuthan and Waugh, 2016). As noted by Sanger (2006), symptoms of dyskinetic CP include both hyperkinetic and dystonic limb movements that impair function. Crichton et al. (2020) found basal ganglia and thalamus lesions on MRI were associated with reduced bimanual performance assessed with the Assisting Hand Assessment. Dyskinetic CP is often associated with GMFCS levels IV or V (Palisano et al., 2008; Himmelmann and Uvebrant, 2011). Milder cases may remain undiagnosed. Research on treatment for dyskinetic CP is needed. Early evidence indicates deep brain stimulation may be beneficial (Fehlings et al., 2024). Dyskinetic CP can co-occur along with spastic CP and delineation is important for appropriate treatment.
Ataxic CP is less common than spastic and dyskinetic CP and is linked with cerebellar vermis injury, cerebellar malformations, and/or genetic mutations (Zhou et al., 2017). Individuals with ataxic CP present with impaired coordination of voluntary movements and impairments to balance, stability, and speech (Hughes and Newton, 1992; Schnekenberg et al., 2015; Graham et al., 2016). The few studies of neurologic correlates of ataxic CP identified cerebral abnormalities and changes in the posterior fossa, involving the vermis of the cerebellum (Miller and Cala, 1989).
Neuromuscular impairments of spastic cerebral palsy
Neuromuscular impairments differ among spastic, dyskinetic, and ataxic CP and are specific to the brain injury. Here we focus on neuromuscular impairments of spastic CP. In spastic CP, neurological injury to corticospinal tract results in four primary neuromuscular impairments: muscle weakness, short muscle-tendon unit, muscle spasticity, and impaired SMC. These primary neuromuscular impairments impact gait in specific ways and are important to delineate for targeted, effective treatment.
Weakness
Muscle weakness in children with CP contributes to abnormal gait and posture (Brown et al., 1991; Damiano et al., 1995; Rose and McGill, 2005; Barber et al., 2012; Noble et al., 2014). Evidence indicates that decreased excitatory descending motor signals in the CST results in reduced neuromuscular activation and muscle volume that is exacerbated by secondary pathological changes in muscle elasticity (Rose and McGill, 2005; Stackhouse et al., 2005; Brown et al., 1991; Wiley and Damiano, 1998; Engsberg et al., 2000). Significant reduction in MVC of quadriceps, plantarflexors, and dorsiflexors occurs in children with CP (Damiano et al., 2002; Elder et al., 2003; Barber et al., 2012). Barber et al. (2012) found that children with CP have 33% lower ankle plantarflexion torque, explained in part by 33% smaller medial gastrocnemius. Rose and McGill (2005) found reduced MVC was associated with reduced neuromuscular activation and motor-unit recruitment in the medial gastrocnemius and tibialis anterior. Weakness in children with spastic CP is often under-recognized and contributes to gait abnormalities and functional limitations.
Short muscle-tendon units
For children with spastic CP, short muscle results from slow muscle growth relative to skeletal growth, secondary muscle and fascia structural changes, and hypo- extensibility. Bi-articulate muscles, such as gastrocnemius, hamstrings, and rectus femoris, are most susceptible to joint contracture from rapid skeletal growth out-pacing muscle growth, which contributes to gait abnormalities. Barber et al. (2016) found reduced normalized muscle growth rate of medial gastrocnemius in CP. Similarly, Herskind et al. (2016) found lower muscle volumes in CP compared to age-matched TD children. Noble et al. (2014) also found children with CP had reduced volumes of lower extremity muscles compared to TD children, even when adjusted for body mass. In unilateral CP, Barrett and Lichtwark (2010) found affected side muscles were smaller than the unaffected side due to a lack of cross-sectional growth, not fascicle length. Thus, evidence indicates reduced muscle growth is a primary impairment in spastic CP.
While slow muscle growth relative to skeletal growth results in short muscle and is a disabling impairment in spastic CP, there is still limited understanding of the role of growth factors in CP. Smith et al. (2009) compared transcriptional profiles of muscle biopsy of six children with spastic CP and two TD children and noted competing upregulation of insulin-like growth factor 1 and myostatin, and aberrant regulation of excitation-contraction coupling genes. Loss of descending neural activation, reduced acetylcholine released at the neuromuscular junction, and altered action potentials within muscle all have potential to reduce muscle and nerve growth factors (Rose and McGill, 2005; Gough and Shortland, 2012), and warrant further investigations.
Interrelation of weak and short muscle
Weak and short muscle are interrelated impairments in CP. Muscle force generation depends on quantity of cross-bridge formations and degree of myofilament overlap; maximal muscle force is produced at intermediate muscle lengths when myofilament overlap is optimal, but not at shortened or lengthened relative lengths (Gordon et al., 1966). Increased muscle sarcomere lengths in CP further contribute to muscle weakness due to inadequate myofilament overlap (Howard et al., 2022; Lieber et al., 2004)
Affected muscles in spastic CP have markedly reduced strength (Damiano et al., 2001); endurance, and neuromuscular activation (Rose and McGill, 2005; Eken et al., 2014) and an inability to recruit motor-units at higher firing rates (Rose and McGill, 2005). Weak, short muscles cause gait abnormalities that often progress as muscle growth fails to keep pace with skeletal growth. Baird et al. (2022) found 23% of participants with CP lost their ability to perform best walking by age 16, due to skeletal deformity, indicating slow muscle growth relative to skeletal growth contributes to gait deterioration.
Corticospinal tract input to the spinal cord begins prenatally and decreased input negatively impacts muscle innervation and activation as early as 17 to 29 weeks after conception (De Beukelaer et al., 2024). Thus, neonatal brain injury likely contributes to poor muscle growth and timing of brain injury during development may impact severity (Thorn et al., 2009; De Beukelaer et al., 2024). De Beukelaer et al. (2024) found children with spastic CP at 12mo of age had lower muscle volume and cross-sectional area of medial gastrocnemius than their TD peers, with lower growth rates found in GMFCS III and IV, compared to GMFCS II and I.
Dayanidhi and Lieber (2014) found 60–70% fewer satellite cells in muscle biopsied from children with CP compared to TD children, indicating satellite cells may play an important role in muscle growth and contracture. Increased stiffness of extracellular matrix and in vivo sarcomere length also contribute to muscle contracture (Smith et al., 2011; Lieber and Theologis, 2021). Increased passive muscle stiffness was proportional to thickened extracellular matrix-based collagen in children with CP (Howard et al., 2022), contributing to hypo-extensibility.
Muscle spasticity
The neural-mediated reflex of muscle spasticity also creates resistance to muscle stretch and limits mobility. Bi-articulate and shortened muscles are more susceptible to influence of spasticity. Spasticity has been defined as “a velocity-dependent increase in muscle tone with exaggerated tendon jerks, resulting from hyper-excitability of the stretch reflex” (Mayer, 1997). Increased sensitivity to stretch in spastic CP is due to loss of inhibition to the muscle stretch reflex (Rose and McGill, 1998).
Despite muscle spasticity being a primary neuromuscular impairment of spastic CP, it is not well quantified (Bar-On et al., 2014a), often over-appreciated, and therefore its impact on gait is not well understood. A study by Choi et al. (2018) assessed hamstring strength and spasticity with the Modified Tardieu Scale and found that the R1 value of fast stretch, is more correlated to knee flexion in gait than R2 (PROM). Understanding how joint rotation impacts passive and active muscle response with precision can help delineate how spasticity impacts gait (Gao et al., 2009; Sloot et al., 2016; Lee et al., 2016). Bar-On et al. (2014b) quantified integrated biomechanical and EMG signals of manually performed passive stretches on medial hamstrings and gastrocnemius and found high measurement reliability, and significantly higher spasticity parameters in children with spastic CP than in TD children. Precise quantification of spasticity clinically and as a feature of musculoskeletal modeling can improve treatment specificity.
Impaired selective motor control
Impaired SMC is defined as “impaired ability to isolate the activation of muscles in a selected pattern in response to demands of a voluntary posture or movement” (Sanger, 2006) including muscle synergies and mirror movements (Rose, 2009; Cahill-Rowley and Rose, 2014; Fahr et al., 2020). Impaired SMC has been assessed using the SCALE, an observation-based measure for children with spastic CP which correlates with GMFCS (Fowler et al., 2009). Impaired SMC assessed with SCALE correlates with knee flexion at IC, short step length, and decreased velocity (Rha et al., 2016). Children with spastic CP demonstrate obligatory co-activation of quadriceps and gastrocnemius on EMG, which distinguishes mild spastic CP from idiopathic toe walking (Rose et al., 1999; Policy et al., 2001). Reduced complexity of neuromuscular control during gait on EMG was found in CP (Steele et al., 2015). Yun et al. (2023) evaluated 36 children with bilateral spastic CP, GMFCS I-II, and found associations between impaired SMC on SCALE and lower scores on GMFM-88, Pediatric Balance Scale, Edinburg Visual Gait Scale,10-Meter Walk Test, and Timed Up and Go Test. Additionally, Noble et al. (2019) studied 11 children with CP and found strong correlations between GMFM- 66 with SCALE, muscle volume on MRI, and spasticity assessed on Modified Ashworth Scale. Recently, Graci et al. (2024) developed indices for coactivation, mirror movement, synergy, and overflow for children with CP using EMG for quantify and classify impaired SMC to guide targeted interventions.
Spared “extrapyramidal” motor tracts, such as the rubrospinal and reticulospinal tracts, may provide imperfect compensation in recovering motor function after corticospinal tract injury (Yeo and Jang, 2010; Rüber et al., 2012). As shown in Figure 1, the corticospinal tract originates in the motor cortex, whereas the rubrospinal tract originates in the mid brain (red nucleus), descends along with the corticospinal tract, and mediates flexion and extension movements (Mtui et al., 2016). Evidence also suggests that there is overlap of the terminating fibers of the corticospinal tract and rubrospinal tracts in the spinal cord grey matter of humans (Olivares-Moreno et al., 2021). The rubrospinal tract is better developed in infants than in children and adults. However, in stroke the rubrospinal tract re-develops, as assessed on DTI, associated with impaired SMC movement patterns post-corticospinal tract injury (Yeo and Jang, 2010; Rüber et al., 2012). Cortical mapping with electroencephalographic and torque metrics in stroke patients suggests that impaired SMC is associated with increased overlap of joint representation in sensorimotor cortices (Yao et al., 2009). Further research can characterize development and function of the rubrospinal and reticulospinal tracts in spastic CP and potential for plasticity to improve SMC.
Neuromuscular impairments and gait in spastic cerebral palsy
Figure 2 shows a gait assessment with the neuromuscular impairments that contribute to specific gait events in spastic CP. This delineation can guide evidence-based, targeted treatment to better improve gait. Figure 2 can also be used clinically as a checklist to help identify the contributions of neuromuscular impairments to gait.
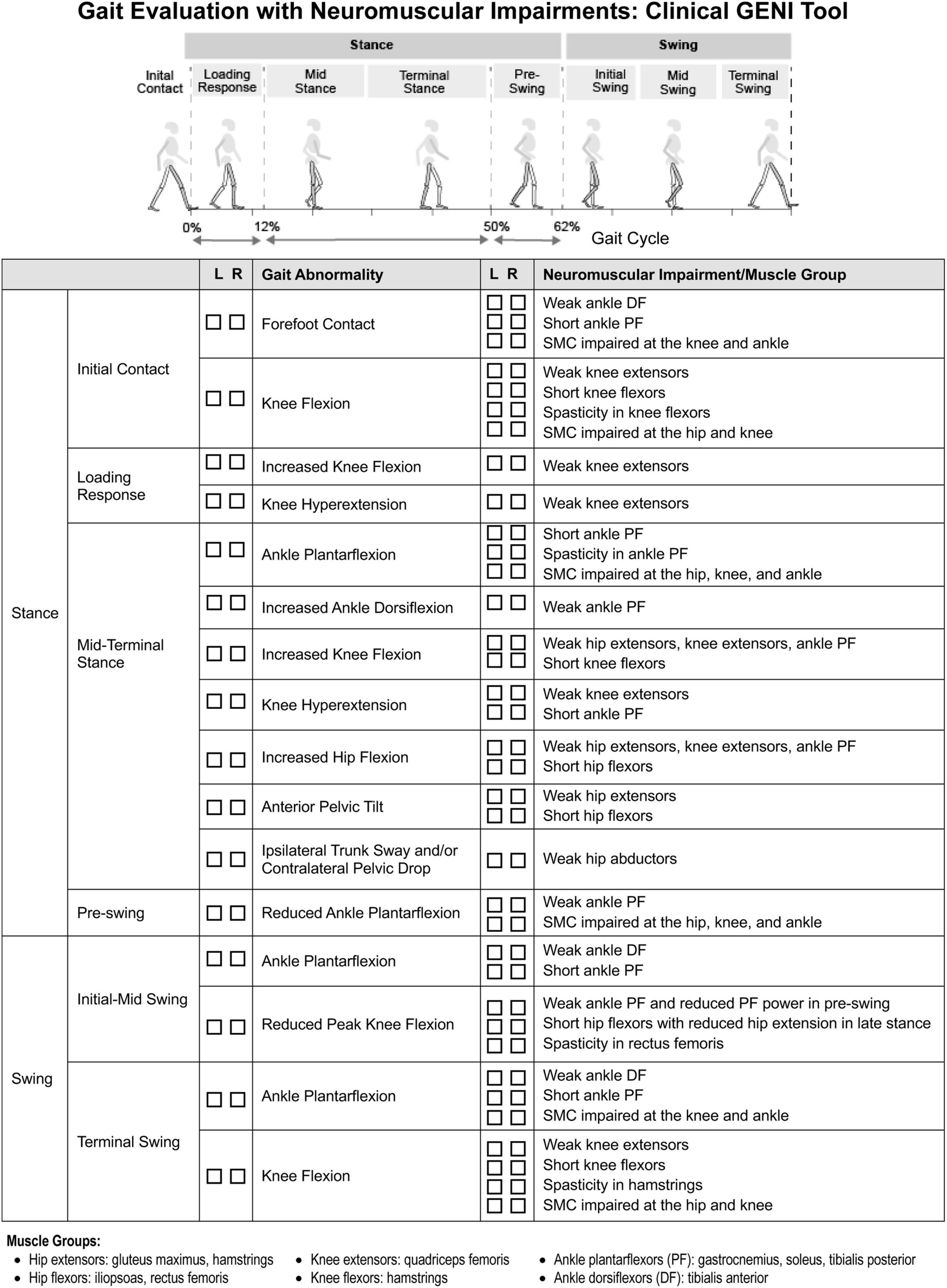
Figure 2. Clinical gait evaluation with consideration of neuromuscular impairments. Revised with permission from Zhou et al. (2017).
Weakness: gait abnormalities and treatment considerations
Muscle weakness impacts both stance and swing phases of gait (Figure 2). At initial contact (IC), weak ankle dorsiflexors (DF) contribute to ankle plantarflexion and foot-slap. In stance, weak ankle plantarflexors (PF), knee extensors and hip extensors contribute to flexed hip, knee, and ankle gait pattern. When the foot is planted on the ground, both the hip and ankle extensors act to extend the knee, through their actions on the femur and tibia respectively, along with the knee extensors. Quadriceps weakness can also contribute to knee hyperextension in stance, compensatory to reduce demand on quadriceps. In pre-swing as the ankle transitions from DF to PF, weak ankle PF decreases ankle PF power which reduces peak knee flexion in swing. Weak ankle DF contributes to ankle PF and poor foot clearance in swing. In terminal swing, weak knee extensors limit knee extension, causing knee flexion in terminal swing and IC. Steele et al. (2012) found children with flexed gait had less passive skeletal support of body weight and utilized higher active muscle forces to walk than TD children.
Proximal stability is critical for independent gait: weak trunk and pelvic musculature can contribute to ipsilateral trunk sway and contralateral pelvic drop in stance, trunk and pelvic rotation, and increased anterior pelvic tilt with lumbar lordosis.
Interventions targeting weak muscles are effective at improving strength and mobility without adverse effects in CP (Dodd et al., 2002; Scholtes et al., 2012). While evidence is accumulating on ideal dosing of strength training programs, additional research is needed. Cho and Lee (2020) conducted a randomized control trial with 25 children with spastic CP performing functional progressive resistance exercise for 30 minutes, 3x/wk, for 6 weeks and found improved strength assessed with hand-held dynamometer, increased rectus femoris cross-sectional area and quadriceps thickness on ultrasound imaging, and improved functional ability on GMFM-88. Verschuren et al. (2016) reviewed dosing recommendations for exercise and physical activity for CP. A scoping review by Mooney and Rose (2019) found weakness and spasticity were improved with single-channel neuromuscular electrical stimulation (NMES) during gait for children with CP. More research is needed on strengthening and multi-channel NMES to develop effective treatment that translate to improved gait.
Short muscle and hypo-extensibility: gait abnormalities and treatment considerations
Short ankle PF contribute to forefoot IC and ankle PF in stance and swing (Figure 2). Short tibialis posterior contributes to plantarflexed-varus posture. Over time, short PF increase midfoot breakdown, reduce pre-swing PF power, and reduce foot-floor clearance in swing. Rha et al. (2016) reported short medial gastrocnemius correlated with knee flexion angle at IC. Additionally, short hamstrings limit terminal swing knee extension causing knee flexion at IC. Arnold et al. (2006) reported two-thirds of flexed-knee gait cases were related to short semitendinosus at IC and single leg stance based on musculoskeletal modeling. Short rectus femoris can limit knee flexion in swing. Normally, passive structures store elastic energy and act as a spring, such as hip flexors in pre-swing, as highlighted in a systematic review by Koussou et al. (2021). Consequently, short hip flexors limit hip extension in terminal stance, dampen the spring, and reduce knee flexion in swing. Additionally, short adductors contribute to increased hip adduction, narrow step width, and scissoring gait.
The challenge is, how to address short muscle while also preserving strength? Given the pennate fiber angle of gastrocnemius, strengthening which increases muscle fiber diameter, can increase muscle length across joint axes (Shortland et al., 2001; Gough and Shortland, 2012). Kalkman et al. (2019) conducted a randomized controlled trial which found resistance training with passive stretching improved tendon stiffness and muscle fascicle length in CP. Additionally, eccentric strengthening improves ROM and strength (O’Sullivan et al., 2012).
Research indicates that soft-tissue surgery, such as tendo-Achilles lengthening and gastrocnemius recession, may reduce muscle strength (Wren et al., 2010). This may in part may explain modest outcomes of surgery for children with spastic CP (Schwartz et al., 2022). Howard et al. (2022) reported with gastrocnemius-soleus recession, there was decreased fascicle length, increased pennation angle with no change in muscle volume; however, a reduction in muscle volume was found after surgery to semitendinosus. Additionally, serial casting contributes to muscle weakness due to prolonged immobilization. Further research is needed to improve clinical monitoring of muscle growth relative to skeletal growth, such as with ultrasound, to better target and evaluate interventions. In the absence of ultrasound, for example, using a tape measure for upper and lower leg length measurement in relation to muscle girth at a percent distance from boney landmark may offer valuable clinical monitoring of muscle growth.
Spasticity: gait abnormalities and treatment considerations
Spasticity most prominently impacts bi-articulate muscles, which experience greater stretch excursion. Short muscles, which experience stretch more frequently. However, the effect of spasticity on gait is often overestimated, whereas short muscle and structural hypo-extensibility may be under appreciated. Similar to short muscle, spasticity of gastrocnemius can contribute to forefoot IC and ankle PF in stance, with rapid stretch during the ankle rocker (Figure 2). Hamstring spasticity can contribute to limited knee extension in terminal swing and knee flexion at IC and stance (Steele et al., 2012). Additionally, spasticity of rectus femoris can contribute to reduced knee flexion in early swing, decreased peak knee flexion, limiting foot-floor clearance. Hip adductor spasticity can contribute to adducted, scissoring gait pattern.
BoNT-A injections, baclofen, and selective dorsal rhizotomy are common treatments for spasticity, but have risks, including muscle weakness (Walhain et al., 2021). There is some evidence that non-pharmacologic and non-surgical interventions can be effective at reducing spasticity while also preserving strength. Williams et al. (2013) found BoNT-A combined with strength training reduced spasticity, and improved strength when compared to BoNT-A alone in 15 children with spastic diplegic CP aged 5–12 years. A study conducted by Mahmood et al. (2024) found spasticity was reduced after six and 12 weeks of high dosed massage home program. Further research is needed to assess the efficacy of treatments that promote muscle strength and length, reduce spasticity, and improve gait.
Impaired selective motor control: gait abnormalities and treatment considerations
Impaired SMC arises from in obligatory synergistic co-activation and impacts the gait cycle when the hip, knee, and ankle are required to flex or extend independently. For example, impaired SMC contributes to ankle PF in terminal swing and IC as the knee extends and also contributes to ankle PF in stance as the hip and knee extend (Figure 2). Impaired SMC can also reduce pre-swing push-off mechanics by reducing ankle PF as the hip and knee begin to flex, slowing the transition into hip and knee flexion in swing. Additionally, impaired SMC can contribute to knee flexion in terminal swing due to hip flexion. A study conducted by Rha et al. (2016) found that impaired SMC, assessed with SCALE, was a stronger correlate of knee flexion at IC than estimated length of semitendinosus assessed with musculoskeletal modeling.
Research investigating impaired SMC is limited, however exercises promoting movement patterns outside of synergy may prove beneficial and warrant further study. A systematic review by Fahr et al. (2020) found existing interventions had variable efficacy in improving SMC. There is indication that younger children with CP may have greater sensitivity to interventions addressing muscle synergies due to higher brain plasticity (Yang et al., 2013; Bekius et al., 2020). Research is needed to determine if treatment can improve SMC and translate to improved gait.
Of note, Figure 2 addresses gait abnormalities that occur during specific phases of gait and are directly related to neuromuscular impairments of spastic CP. Figure 2 does not address gait abnormalities directly related to balance impairment, such as wide-base gait, or those directly related to pain, such as short stance phase of antalgic gait and ipsilateral trunk sway, or those directly related to skeletal deformities and leg length discrepancies which also can contribute to gait abnormalities, including abnormal foot progression angle, in spastic CP. It also does not address gait abnormalities that are compensatory, such as increased hip and knee flexion of steppage gait which compensates for ankle plantarflexion in swing. These impairments and compensations need to be delineated in order to guide effective treatment.
Conclusion
This perspective reviewed links between brain injury, neuromuscular impairments and resulting gait abnormalities in spastic CP. By providing this context, we hope to encourage recognition of the neuromuscular and musculoskeletal mechanisms underlying gait abnormalities in CP and focus research to better identify and quantify these impairments, delineate their contribution to gait abnormalities, and develop targeted treatments. For example, research as well as regular clinical assessment of muscle growth relative to skeletal growth would better quantify this consequential impairment and help develop standardized outcome measures. Additionally, future research should focus on how muscle growth, especially in bi-articulate muscles, affects the impact of spasticity on gait in order to better focus treatments. Expanding the features of musculoskeletal modeling and artificial intelligence applications to include neuromuscular impairments, such as impaired SMC. and discover targeted treatment opportunities that can address muscle growth and SMC to substantially improve functional outcomes for individuals with CP.
Data availability statement
The original contributions presented in the study are included in the article/supplementary material, further inquiries can be directed to the corresponding author.
Author contributions
KC: Conceptualization, Data curation, Formal analysis, Investigation, Resources, Writing – original draft, Writing – review and editing. CH: Conceptualization, Data curation, Formal analysis, Investigation, Resources, Writing – original draft, Writing – review and editing. YD: Conceptualization, Data curation, Formal analysis, Investigation, Resources, Writing – original draft, Writing – review and editing. MM: Conceptualization, Data curation, Formal analysis, Investigation, Resources, Writing – original draft, Writing – review and editing. EL: Conceptualization, Data curation, Formal analysis, Investigation, Resources, Writing – original draft, Writing – review and editing. JR: Conceptualization, Data curation, Formal analysis, Funding acquisition, Investigation, Project administration, Resources, Writing – original draft, Writing – review and editing.
Funding
The author(s) declare financial support was received for the research, authorship, and/or publication of this article. This work was supported in part by the Mary Baracchi Fund, Stanford Medicine Children’s Health, LPCH Motion and Gait Lab Stanford University.
Acknowledgments
We wish to thank Katelyn Cahill-Rowley, Joanne Zhou, Kornél Schadl and MaryAnn Wijtman for helping to create and revise (Figures 1, 2).
Conflict of interest
The authors declare that the research was conducted in the absence of any commercial or financial relationships that could be construed as a potential conflict of interest.
Publisher’s note
All claims expressed in this article are solely those of the authors and do not necessarily represent those of their affiliated organizations, or those of the publisher, the editors and the reviewers. Any product that may be evaluated in this article, or claim that may be made by its manufacturer, is not guaranteed or endorsed by the publisher.
Abbreviations
BoNT-A, botulinum toxin-A; CP, cerebral palsy; CST, corticospinal tract; DTI, diffusion tensor imaging; EMG, electromyography; GM, gray matter; GMFCS, Gross Motor Function Classification Scale; MRI, magnetic resonance imaging; PVL, periventricular leukomalacia; SCALE, Selective Control Assessment of the Lower Extremity; SMC, selective motor control; TD, typically developing; VLBW, very low birthweight; WM, white matter; MVC, maximum voluntary contraction; PROM, passive range of motion; RCT, randomized control trial.
References
Aravamuthan, B. R., and Waugh, J. L. (2016). Localization of basal ganglia and thalamic damage in dyskinetic cerebral palsy. Pediatr. Neurol. 54, 11–21. doi: 10.1016/j.pediatrneurol.2015.10.005
Arnold, A. S., Liu, M. Q., Schwartz, M. H., Ounpuu, S., and Delp, S. L. (2006). The role of estimating muscle-tendon lengths and velocities of the hamstrings in the evaluation and treatment of crouch gait. Gait Post. 23, 273–281. doi: 10.1016/j.gaitpost.2005.03.003
Baird, G., Chandler, S., Shortland, A., Will, E., Simonoff, E., Scrutton, D., et al. (2022). Acquisition and loss of best walking skills in children and young people with bilateral cerebral palsy. Dev. Med. Child Neurol. 64, 235–242.
Barber, L. A., Read, F., Lovatt Stern, J., Lichtwark, G., and Boyd, R. N. (2016). Medial gastrocnemius muscle volume in ambulant children with unilateral and bilateral cerebral palsy aged 2 to 9 years. Dev. Med. Child Neurol. 58, 1146–1152. doi: 10.1111/dmcn.13132
Barber, L., Barrett, R., and Lichtwark, G. (2012). Medial gastrocnemius muscle fascicle active torque-length and Achilles tendon properties in young adults with spastic cerebral palsy. J. Biomech. 45, 2526–2530. doi: 10.1016/j.jbiomech.2012.07.018
Bar-On, L., Aertbeliën, E., Molenaers, G., Dan, B., and Desloovere, K. (2014a). Manually controlled instrumented spasticity assessments: A systematic review of psychometric properties. Dev. Med. Child Neurol. 56, 932–950. doi: 10.1111/dmcn.12419
Bar-On, L., Desloovere, K., Molenaers, G., Harlaar, J., Kindt, T., and Aertbeliën, E. (2014b). Identification of the neural component of torque during manually applied spasticity assessments in children with cerebral palsy. Gait Post. 40, 346–351. doi: 10.1016/j.gaitpost.2014.04.207
Barrett, R. S., and Lichtwark, G. A. (2010). Gross muscle morphology and structure in spastic cerebral palsy: A systematic review. Dev. Med. Child Neurol. 52, 794–804. doi: 10.1111/j.1469-8749.2010.03686.x
Bax, M., Tydeman, C., and Flodmark, O. (2006). Clinical and MRI correlates of cerebral palsy: The European cerebral palsy study. JAMA 296, 1602–1608. doi: 10.1001/jama.296.13.1602
Beani, E., Barzacchi, V., Scaffei, E., Ceragioli, B., Festante, F., Filogna, S., et al. (2024). Neuroanatomical correlates of gross manual dexterity in children with unilateral spastic cerebral palsy. Front. Hum. Neurosci. 18, 1–10.
Bekius, A., Bach, M., Krogt, M., Vries, R., Buizer, A., and Dominici, N. (2020). Muscle synergies during walking in children with cerebral palsy: A systematic review. Front. Physiol. 11:632. doi: 10.3389/fphys.2020.00632
Bell, K. J., Ounpuu, S., DeLuca, P. A., and Romness, M. J. (2002). Natural progression of gait in children with cerebral palsy. J. Pediatr. Orthop. 22, 677–682. doi: 10.1097/01241398-200209000-00020
Brown, J. K., Rodda, J., Walsh, E. G., and Wright, G. W. (1991). Neurophysiology of lower-limb function in hemiplegic children. Dev. Med. Child Neurol. 33, 1037–1047. doi: 10.1111/j.1469-8749.1991.tb14825.x
Cahill-Rowley, K., and Rose, J. (2014). Etiology of impaired selective motor control: Emerging evidence and its implications for research and treatment in cerebral palsy. Dev. Med. Child Neurol. 56, 522–528. doi: 10.1111/dmcn.12355
Cho, H., and Lee, B. (2020). Effect of functional progressive resistance exercise on lower extremity structure, muscle tone, dynamic balance and functional ability in children with spastic cerebral palsy. Children 7:85.
Choi, J., Park, E., Park, D., and Rha, D. (2018). Dynamic spasticity determines hamstring length and knee flexion angle during gait in children with spastic cerebral palsy. Gait Post. 64, 255–259.
Christian, J., Kröll, J., and Schwameder, H. (2017). Comparison of the classifier oriented gait score and the gait profile score based on imitated gait impairments. Gait Post. 55, 49–54.
Costeff, H. (2004). Estimated frequency of genetic and nongenetic causes of congenital idiopathic cerebral palsy in west Sweden. Ann. Hum. Genet. 68, 515–520. doi: 10.1046/j.1529-8817.2004.00105.x
Crichton, A., Ditchfield, M., Gwini, S., Wallen, M., Thorley, M., Bracken, J., et al. (2020). Brain magnetic resonance imaging is a predictor of bimanual performance and executive function in children with unilateral cerebral palsy. Dev. Med. Child Neurol. 62, 615–624.
Damiano, D. L., Dodd, K., and Taylor, N. F. (2002). Should we be testing and training muscle strength in cerebral palsy? Dev. Med. Child Neurol. 44, 68–72. doi: 10.1017/S0012162201001682
Damiano, D. L., Quinlivan, J., Owen, B. F., Shaffrey, M., and Abel, M. F. (2001). Spasticity versus strength in cerebral palsy: Relationships among involuntary resistance, voluntary torque, and motor function. Eur. J. Neurol. 8, 40–49. doi: 10.1046/j.1468-1331.2001.00037.x
Damiano, D. L., Vaughan, C. L., and Abel, M. E. (1995). Muscle response to heavy resistance exercise in children with spastic cerebral palsy. Dev. Med. Child Neurol. 37, 731–739. doi: 10.1111/j.1469-8749.1995.tb15019.x
Dayanidhi, S., and Lieber, R. L. (2014). Skeletal muscle satellite cells: Mediators of muscle growth during development and implications for developmental disorders. Muscle Nerve 50, 723–732. doi: 10.1002/mus.24441
De Beukelaer, N., Vandekerckhove, I., Molenberghs, G., Naulaers, G., Thewissen, L., Costamagna, D., et al. (2024). Longitudinal trajectory of medial gastrocnemius muscle growth in the first years of life. Dev. Med. Child Neurol. 66, 531–540.
Dodd, K. J., Taylor, N. F., and Damiano, D. L. (2002). A systematic review of the effectiveness of strength-training programs for people with cerebral palsy. Arch. Phys. Med. Rehabil. 83, 1157–1164. doi: 10.1053/apmr.2002.34286
Eken, M. M., Dallmeijer, A. J., Doorenbosch, C. A., Dekkers, H., Becher, J. G., and Houdijk, H. (2014). Assessment of muscle endurance of the knee extensor muscles in adolescents with spastic cerebral palsy using a submaximal repetitions-to-fatigue protocol. Arch. Phys. Med. Rehabil. 95, 1888–1894. doi: 10.1016/j.apmr.2014.05.010
Elder, G. C., Kirk, J., Stewart, G., Cook, K., Weir, D., Marshall, A., et al. (2003). Contributing factors to muscle weakness in children with cerebral palsy. Dev. Med. Child Neurol. 45, 542–550. doi: 10.1111/j.1469-8749.2003.tb00954.x
Ellenberg, J. H., and Nelson, K. B. (2013). The association of cerebral palsy with birth asphyxia: A definitional quagmire. Dev. Med. Child Neurol. 55, 210–216. doi: 10.1111/dmcn.12016
Emrick, L., and DiCarlo, S. (2020). The expanding role of genetics in cerebral palsy. Phys. Med. Rehabil. Clin. N. Am. 31, 15–24.
Engsberg, J. R., Ross, S. A., Olree, K. S., and Park, T. S. (2000). Ankle spasticity and strength in children with spastic diplegic cerebral palsy. Dev. Med. Child Neurol. 42, 42–47.
Fahr, A., Keller, J., and Hedel, H. (2020). A systematic review of training methods that may improve selective voluntary motor control in children with spastic cerebral palsy. Front. Neurol. 11:572038. doi: 10.3389/fneur.2020.572038
Fehlings, D., Agnew, B., Gimeno, H., Harvey, A., Himmelmann, K., Lin, J., et al. (2024). Pharmacological and neurosurgical management of cerebral palsy and dystonia: Clinical practice guideline update. Dev. Med. Child Neurol. 66, 1133–1147.
Fiori, S., Cioni, G., Klingels, K., Ortibus, E., Van Gestel, L., Rose, S., et al. (2014). Reliability of a novel, semi-quantitative scale for classification of structural brain magnetic resonance imaging in children with cerebral palsy. Dev. Med. Child Neurol. 56, 839–845.
Fiori, S., Guzzetta, A., Pannek, K., Ware, R. S., Rossi, G., Klingels, K., et al. (2015). Validity of semi-quantitative scale for brain MRI in unilateral cerebral palsy due to periventricular white matter lesions: Relationship with hand sensorimotor function and structural connectivity. Neuroimage 8, 104–109. doi: 10.1016/j.nicl.2015.04.005
Fowler, E. G., Staudt, L. A., Greenberg, M. B., and Oppenheim, W. L. (2009). Selective control assessment of the lower extremity (SCALE): Development, validation, and interrater reliability of a clinical tool for patients with cerebral palsy. Dev. Med. Child Neurol. 51, 607–614. doi: 10.1111/j.1469-8749.2008.03186.x
Gage, J. R., and Novacheck, T. F. (2001). An update on the treatment of gait problems in cerebral palsy. J. Pediatr. Orthop. B 10, 265–274.
Gao, F., Grant, T. H., Roth, E. J., and Zhang, L. Q. (2009). Changes in passive mechanical properties of the gastrocnemius muscle at the muscle fascicle and joint levels in stroke survivors. Arch. Phys. Med. Rehabil. 90, 819–826.
Gonzalez-Mantilla, P., Hu, Y., Myers, S., Finucane, B., Ledbetter, D., Martin, C., et al. (2023). Diagnostic yield of exome sequencing in cerebral palsy and implications for genetic testing guidelines: A systematic review and meta-analysis. JAMA Pediatr. 177, 472–478.
Gordon, A. M., Huxley, A. F., and Julian, F. J. (1966). The variation in isometric tension with sarcomere length in vertebrate muscle fibres. J. Physiol. 184:170. doi: 10.1113/jphysiol.1966.sp007909
Gough, M., and Shortland, A. P. (2012). Could muscle deformity in children with spastic cerebral palsy be related to an impairment of muscle growth and altered adaptation? Dev. Med. Child Neurol. 54, 495–499. doi: 10.1111/j.1469-8749.2012.04229.x
Graci, V., O’Neill, M., Bloss, M., Akkem, R., Paremski, A. C., Sanders, O., et al. (2024). A new methodological approach to characterize selective motor control in children with cerebral palsy. Front. Hum. Neurosci. 18:1330315. doi: 10.3389/fnhum.2024.1330315
Graham, H. K., Rosenbaum, P., Paneth, N., Dan, B., Lin, J.-P., Damiano, D. L., et al. (2016). Cerebral palsy. Nat. Rev. Dis. Prim. 2:15082. doi: 10.1038/nrdp.2015.82
Herskind, A., Ritterband-Rosenbaum, A., Willerslev-Olsen, M., Lorentzen, J., Hanson, L., Lichtwark, G., et al. (2016). Muscle growth is reduced in 15-month-old children with cerebral palsy. Dev. Med. Child Neurol. 58, 485–491.
Himmelmann, K., and Uvebrant, P. (2011). Function and neuroimaging in cerebral palsy: A population-based study. Dev. Med. Child Neurol. 53, 516–521. doi: 10.1111/j.1469-8749.2011.03932.x
Hoon, A. H., Stashinko, E. E., Nagae, L. M., Lin, D. D. M., Keller, J., Bastian, A., et al. (2009). Sensory and motor deficits in children with cerebral palsy born preterm correlate with diffusion tensor imaging abnormalities in thalamocortical pathways. Dev. Med. Child Neurol. 51, 697–704. doi: 10.1111/j.1469-8749.2009.03306.x
Howard, J., Graham, K., and Shortland, A. (2022). Understanding skeletal muscle in cerebral palsy: A path to personalized medicine? Dev. Med. Child Neurol. 64, 289–295.
Hughes, I., and Newton, R. (1992). Genetic aspects of cerebral palsy. Dev. Med. Child Neurol. 34, 80–86. doi: 10.1111/j.1469-8749.1992.tb08568.x
Inder, T. E., and Volpe, J. J. (2000). Mechanisms of perinatal brain injury. Semin. Neonatol. 5, 3–16. doi: 10.1053/siny.1999.0112
Kalkman, B., Holmes, G., Bar-On, L., Maganaris, C., Barton, G., Bass, A., et al. (2019). Resistance training combined with stretching increases tendon stiffness and is more effective than stretching alone in children with cerebral palsy: A randomized controlled trial. Front. Pediatr. 7:333. doi: 10.3389/fped.2019.00333
Kitai, Y., Hirai, S., Okuyama, N., Hirotsune, M., Nishimoto, S., Hirano, S., et al. (2021). Functional outcomes of children with dyskinetic cerebral palsy depend on etiology and gestational age. Eur. J. Paediatr. Neurol. 30, 108–112.
Koussou, A., Desailly, E., and Dumas, R. (2021). Contribution of passive moments to inter-segmental moments during gait: A systematic review. J. Biomech. 122:110450.
Laporta-Hoyos, O., Ballester-Plané, J., Vázquez, E., Delgado, I., Narberhaus, A., Póo, P., et al. (2014). PS-247 association of motor function with basal ganglia and thalamus volumes in dyskinetic cerebral palsy. Arch. Dis. Child. 99, A202–A202. doi: 10.1136/archdischild-2014-307384.546
Lee, J., and Muzio, M. R. (2020). Neuroanatomy, extrapyramidal system: StatPearls [Internet]. Treasure Island, FL: StatPearls Publishing.
Lee, S. S., Gaebler-Spira, D., Zhang, L. Q., Rymer, W. Z., and Steele, K. M. (2016). Use of shear wave ultrasound elastography to quantify muscle properties in cerebral palsy. Clin. Biomech. 31, 20–28. doi: 10.1016/j.clinbiomech.2015.10.006
Lieber, R. L., Steinman, S., Barash, I. A., and Chambers, H. (2004). Structural and functional changes in spastic skeletal muscle. Muscle Nerve 29, 615–627. doi: 10.1002/mus.20059
Lieber, R., and Theologis, T. (2021). Muscle-tendon unit in children with cerebral palsy. Dev. Med. Child Neurol. 63, 908–913.
Liu, C., Peng, Y., Yang, Y., Li, P., Chen, D., Nie, D., et al. (2024). Structure of brain grey and white matter in infants with spastic cerebral palsy and periventricular white matter injury. Dev. Med. Child Neurol. 66, 514–522.
MacLennan, A. H., Thompson, S. C., and Gecz, J. (2015). Cerebral palsy: Causes, pathways, and the role of genetic variants. Am. J. Obstet. Gynecol. 213, 779–788. doi: 10.1016/j.ajog.2015.05.034
Mahmood, Q., Habibullah, S., and Aurakzai, H. (2024). Effectiveness of simple and basic home-based exercise programs including pediatric massage executed by caregivers at their homes in the management of children with spastic cerebral palsy: A randomized controlled trial. J. Pediatr. Rehabil. Med. 17, 97–106.
Mayer, N. H. (1997). Clinicophysiologic concepts of spasticity and motor dysfunction in adults with an upper motoneuron lesion. Muscle Nerve 20, 1–14. doi: 10.1002/(SICI)1097-4598(1997)6+<1::AID-MUS2=3.0.CO;2-D
Metz, C., Jaster, M., Walch, E., Sarpong-Bengelsdorf, A., Kaindl, A., and Schneider, J. (2022). Clinical phenotype of cerebral palsy depends on the cause: Is it really cerebral palsy? A retrospective study. J. Child Neurol. 37, 112–118.
Meyns, P., Van Gestel, L., Leunissen, I., De Cock, P., Sunaert, S., Feys, H., et al. (2016). Macrostructural and microstructural brain lesions relate to gait pathology in children with cerebral palsy. Neurorehabil. Neural Repair 30, 817–833. doi: 10.1177/1545968315624782
Miller, G., and Cala, L. A. (1989). Ataxic cerebral palsy-clinico-radiologic correlations. Neuropediatrics 20, 84–89. doi: 10.1055/s-2008-1071271
Mooney, J., and Rose, J. (2019). A scoping review of neuromuscular electrical stimulation to improve gait in cerebral palsy: The arc of progress and future strategies. Front. Neurol. 10:887. doi: 10.3389/fneur.2019.00887
Moreno-De-Luca, A., Ledbetter, D. H., and Martin, C. L. (2012). Genetic insights into the causes and classification of the cerebral palsies. Lancet Neurol. 11, 283–292. doi: 10.1016/S1474-4422(11)70287-3
Mtui, E., Gruener, G., and Dockery, P. (2016). FitzGerald’s clinical neuroanatomy and neuroscience, 7th Edn. Philadelphia, PA: Elsevier Health Sciences.
Natali, A. L., Reddy, V., and Bordoni, B. (2018). Neuroanatomy, corticospinal cord tract: StatPearls [Internet]. Treasure Island, FL: StatPearls Publishing.
Nguyen, J. D., and Duong, H. (2023). Neurosurgery, sensory homunculus: Statpearls [internet]. Treasure Island, FL: StatPearls Publishing.
Noble, J. J., Fry, N. R., Lewis, A. P., Keevil, S. F., Gough, M., and Shortland, A. P. (2014). Lower limb muscle volumes in bilateral spastic cerebral palsy. Brain Dev. 36, 294–300. doi: 10.1016/j.braindev.2013.05.008
Noble, J. J., Gough, M., and Shortland, A. P. (2019). Selective motor control and gross motor function in bilateral spastic cerebral palsy. Dev. Med. Child Neurol. 61, 57–61.
Olivares-Moreno, R., Rodriguez-Moreno, P., Lopez-Virgen, V., Macías, M., Altamira-Camacho, M., and Rojas-Piloni, G. (2021). Corticospinal vs Rubrospinal revisited: An evolutionary perspective for sensorimotor integration. Front. Neurosci. 15:686481. doi: 10.3389/fnins.2021.686481
Oskoui, M., Coutinho, F., Dykeman, J., Jetté, N., and Pringsheim, T. (2013). An update on the prevalence of cerebral palsy: A systematic review and metaanalysis. Dev. Med. Child Neurol. 55, 509–519. doi: 10.1111/dmcn.12080
O’Sullivan, K., McAuliffe, S., and DeBurca, N. (2012). The effects of eccentric training on lower limb flexibility: A systematic review. Br. J. Sports Med. 46, 838–845.
Palisano, R. J., Rosenbaum, P., Bartlett, D., and Livingston, M. H. (2008). Content validity of the expanded and revised gross motor function classification system. Dev. Med. Child Neurol. 50, 744–750. doi: 10.1111/j.1469-8749.2008.03089.x
Paneth, N., Hong, T., and Korzeniewski, S. (2006). The descriptive epidemiology of cerebral palsy. Clin. Perinatol. 33, 251–267. doi: 10.1016/j.clp.2006.03.011
Pham, R., Mol, B., Gecz, J., MacLennan, A., MacLennan, S., Corbett, M., et al. (2020). Definition and diagnosis of cerebral palsy in genetic studies: A systematic review. Dev. Med. Child Neurol. 62, 1024–1030.
Policy, J. F., Torburn, L., Rinsky, L. A., and Rose, J. (2001). Electromyographic test to differentiate mild diplegic cerebral palsy and idiopathic toe-walking. J. Pediatr. Orthop. 21, 784–789. doi: 10.1097/01241398-200111000-00016
Reid, L. B., Cunnington, R., Boyd, R. N., and Rose, S. E. (2016). Surfacebased fMRI-driven diffusion tractography in the presence of significant brain pathology: A study linking structure and function in cerebral palsy. PLoS One 11:e0159540. doi: 10.1371/journal.pone.0159540
Reid, S. M., Dagia, C. D., Ditchfield, M. R., Carlin, J. B., and Reddihough, D. S. (2014). Population-based studies of brain imaging patterns in cerebral palsy. Dev. Med. Child Neurol. 56, 222–232. doi: 10.1186/1471-2377-13-57
Reid, S., Meehan, E., Gibson, C., Scott, H., and Delacy, M. (2016). Biological sex and the risk of cerebral palsy in Victoria, Australia. Dev. Med. Child Neurol. 58, 43–49.
Rha, D. W., Cahill-Rowley, K., Young, J., Torburn, L., Stephenson, K., and Rose, J. (2016). Biomechanical and clinical correlates of stance-phase knee flexion in persons with spastic cerebral palsy. PM R 8, 11–18. doi: 10.1016/j.pmrj.2015.06.003
Rose, J. (2009). Selective motor control in spastic cerebral palsy. Dev. Med. Child Neurol. 51, 578–579. doi: 10.1111/j.1469-8749.2009.03401.x
Rose, J., Martin, J. G., Torburn, L., Rinsky, L. A., and Gamble, J. G. (1999). Electromyographic differentiation of diplegic cerebral palsy from idiopathic toe walking: Involuntary coactivation of the quadriceps and gastrocnemius. J. Pediatr. Orthop. 19:677. doi: 10.1097/01241398-199909000-00025
Rose, J., and McGill, K. C. (1998). The motor unit in cerebral palsy. Dev. Med. Child Neurol. 40, 270–277. doi: 10.1111/j.1469-8749.1998.tb15461.x
Rose, J., and McGill, K. C. (2005). Neuromuscular activation and motor-unit firing characteristics in cerebral palsy. Dev. Med. Child Neurol. 47, 329–336. doi: 10.1017/S0012162205000629
Rose, J., Cahill-Rowley, K., Vassar, R., Yeom, K. W., Stecher, X., Stevenson, D. K., et al. (2015). Neonatal brain microstructure correlates of neurodevelopment and gait in preterm children 18-22 mo of age: An MRI and DTI study. Pediatr. Res. 78, 700–708. doi: 10.1038/pr.2015.157
Rose, J., Mirmiran, M., Butler, E. E., Lin, C. Y., Barnes, P. D., Kermoian, R., et al. (2007). Neonatal microstructural development of the internal capsule on diffusion tensor imaging correlates with severity of gait and motor deficits. Dev. Med. Child Neurol. 49, 745–750. doi: 10.1111/j.1469-8749.2007.00745.x
Rosenbaum, P., Paneth, N., Leviton, A., Goldstein, M., Damiano, D., Dan, B., et al. (2007). A report?: The definition and classification of cerebral palsy April 2006. Dev. Med. Child Neurol. 49, 8–14. doi: 10.1111/j.1469-8749.2007.tb12610.x
Rüber, T., Schlaug, G., and Lindenberg, R. (2012). Compensatory role of the cortico-rubro-spinal tract in motor recovery after stroke. Neurology 79, 515–522.
Sanger, T. D. (2006). Arm trajectories in dyskinetic cerebral palsy have increased random variability. J. Child Neurol. 21, 551–557. doi: 10.2310/7010.2006.00113
Scelsi, C. L., Rahim, T. A., Morris, J. A., Kramer, G. J., Gilbert, B. C., and Forseen, S. E. (2020). The lateral ventricles: A detailed review of anatomy, development, and anatomic variations. Am. J. Neuroradiol. 41, 566–572.
Schnekenberg, R. P., Perkins, E. M., Miller, J. W., Davies, W. I., D’Adamo, M. C., Pessia, M., et al. (2015). De novo point mutations in patients diagnosed with ataxic cerebral palsy. Brain 138, 1817–1832. doi: 10.1093/brain/awv117
Scholtes, V. A., Becher, J. G., Janssen-Potten, Y. J., Dekkers, H., Smallenbroek, L., and Dallmeijer, A. J. (2012). Effectiveness of functional progressive resistance exercise training on walking ability in children with cerebral palsy: A randomized controlled trial. Res. Dev. Disabil. 33, 181–188. doi: 10.1016/j.ridd.2011.08.026
Schwartz, M. A., and Rozumalski, A. (2008). The gait deviation index: A new comprehensive index of gait pathology. Gait Post. 28, 351–357.
Schwartz, M. H., Ries, A. J., and Georgiadis, A. G. (2022). Short-term causal effects of common treatments in ambulatory children and young adults with cerebral palsy: Three machine learning estimates. Sci. Rep. 12:7818.
Sellier, E., Goldsmith, S., McIntyre, S., Perra, O., Rackauskaite, G., Badawi, N., et al. (2021). Cerebral palsy in twins and higher multiple births: A Europe-Australia population-based study. Dev. Med. Child Neurol. 63, 712–720.
Sellier, E., Platt, M. J., Andersen, G. L., Krägeloh-Mann, I., De La Cruz, J., and Cans, C. (2016). Decreasing prevalence in cerebral palsy: A multi-site European population-based study, 1980 to 2003. Dev. Med. Child Neurol. 58, 85–92. doi: 10.1111/dmcn.12865
Serdaroglu, G., Tekgul, H., Kitis, O., Serdaroglu, E., and Gökben, S. (2004). Correlative value of magnetic resonance imaging for neurodevelopmental outcome in periventricular leukomalacia. Dev. Med. Child Neurol. 46, 733–739. doi: 10.1111/j.1469-8749.2004.tb00992.x
Shortland, A. P., Harris, C. A., Gough, M., and Robinson, R. O. (2001). Architecture of the medial gastrocnemius in children with spastic diplegia. Dev. Med. Child Neurol. 43, 796–801. doi: 10.1017/S001216220100144X
Sloot, L., Bar-On, L., van der Krogt, M., Aertbeliën, E., Buizer, A., Desloovere, K., et al. (2016). Motor-driven versus manual instrumented spasticity assessment in children with cerebral palsy. Dev. Med. Child Neurol. 59, 145–151. doi: 10.1111/dmcn.13194
Smith, L. R., Lee, K. S., Ward, S. R., Chambers, H. G., and Lieber, R. L. (2011). Hamstring contractures in children with spastic cerebral palsy result from a stiffer extracellular matrix and increased in vivo sarcomere length. J. Physiol. 589, 2625–2639. doi: 10.1113/jphysiol.2010.203364
Smith, L. R., Pontén, E., Hedström, Y., Ward, S. R., Chambers, H. G., Subramaniam, S., et al. (2009). Novel transcriptional profile in wrist muscles from cerebral palsy patients. BMC Med. Genom. 2:44. doi: 10.1186/1755-8794-2-44
Stackhouse, S. K., Binder-Macleod, S. A., and Lee, S. C. (2005). Voluntary muscle activation, contractile properties, and fatigability in children with and without cerebral palsy. Muscle Nerve 31, 594–601. doi: 10.1002/mus.20302
Staudt, M., Niemann, G., Grodd, W., and Krägeloh-Mann, I. (2000). The pyramidal tract in congenital hemiparesis: Relationship between morphology and function in periventricular lesions. Neuropediatrics 31, 257–264. doi: 10.1055/s-2000-9239
Steele, K. M., DeMers, M. S., Schwartz, M. H., and Delp, S. L. (2012). Compressive tibiofemoral force during crouch gait. Gait Post. 35, 556–560. doi: 10.1016/j.gaitpost.2011.11.023
Steele, K. M., Rozumalski, A., and Schwartz, M. H. (2015). Muscle synergies and complexity of neuromuscular control during gait in cerebral palsy. Dev. Med. Child Neurol. 57, 1176–1182. doi: 10.1111/dmcn.12826
Strijbis, E. M., Oudman, I., van Essen, P., and MacLennan, A. H. (2006). Cerebral palsy and the application of the international criteria for acute intrapartum hypoxia. Obstet. Gynecol. 107, 1357–1365. doi: 10.1097/01.AOG.0000220544.21316.80
Thorn, S. R., Regnault, T. R., Brown, L. D., Rozance, P. J., Keng, J., Roper, M., et al. (2009). Intrauterine growth restriction increases fetal hepatic gluconeogenic capacity and reduces messenger ribonucleic acid translation initiation and nutrient sensing in fetal liver and skeletal muscle. Endocrinology 150, 3021–3030. doi: 10.1210/en.2008-1789
Verschuren, O., Peterson, M. D., Balemans, A. C., and Hurvitz, E. A. (2016). Exercise and physical activity recommendations for people with cerebral palsy. Dev. Med. Child Neurol. 58, 798–808.
Walhain, F., Desloovere, K., Declerck, M., Van Campenhout, A., and Bar-On, L. (2021). Interventions and lower-limb macroscopic muscle morphology in children with spastic cerebral palsy: A scoping review. Dev. Med. Child Neurol. 63, 274–286.
Wiley, M. E., and Damiano, D. L. (1998). Lower-Extremity strength profiles in spastic cerebral palsy. Dev. Med. Child Neurol. 40, 100–107. doi: 10.1111/j.1469-8749.1998.tb15369.x
Williams, S. A., Elliott, C., Valentine, J., Gubbay, A., Shipman, P., and Reid, S. (2013). Combining strength training and botulinum neurotoxin intervention in children with cerebral palsy: The impact on muscle morphology and strength. Disabil. Rehabil. 35, 596–605. doi: 10.3109/09638288.2012.711898
Wren, T. A., Cheatwood, A. P., Rethlefsen, S. A., Hara, R., Perez, F. J., and Kay, R. M. (2010). Achilles tendon length and medial gastrocnemius architecture in children with cerebral palsy and equinus gait. J Ped Orthop. 30, 479–484. doi: 10.1097/BPO.0b013e3181e00c80
Wu, Y. W., Day, S. M., Strauss, D. J., and Shavelle, R. M. (2004). Prognosis for ambulation in cerebral palsy: A population-based study. Pediatrics 114, 1264–1271. doi: 10.1542/peds.2004-0114
Yang, J., Livingstone, D., Brunton, K., Kim, D., Lopetinsky, B., Roy, F., et al. (2013). Training to enhance walking in children with cerebral palsy: Are we missing the window of opportunity? Semin. Pediatr. Neurol. 20, 106–115.
Yao, J., Chen, A., Carmona, C., and Dewald, J. P. (2009). Cortical overlap of joint representations contributes to the loss of independent joint control following stroke. Neuroimage 45, 490–499. doi: 10.1016/j.neuroimage.2008.12.002
Yeo, S. S., and Jang, S. H. (2010). Changes in red nucleus after pyramidal tract injury in patients with cerebral infarct. NeuroRehabilitation 27, 373–377. doi: 10.3233/NRE-2010-0622
Yun, G., Huang, M., Cao, J., and Hu, X. (2023). Selective motor control correlates with gross motor ability, functional balance and gait performance in ambulant children with bilateral spastic cerebral palsy. Gait Post. 99, 9–13.
Keywords: cerebral palsy, brain injury, neuromuscular impairments, musculoskeletal impairments, gait abnormalities
Citation: Clewes K, Hammond C, Dong Y, Meyer M, Lowe E and Rose J (2024) Neuromuscular impairments of cerebral palsy: contributions to gait abnormalities and implications for treatment. Front. Hum. Neurosci. 18:1445793. doi: 10.3389/fnhum.2024.1445793
Received: 08 June 2024; Accepted: 19 August 2024;
Published: 18 September 2024.
Edited by:
Nadia Dominici, Vrije Universiteit Amsterdam, NetherlandsReviewed by:
Ahad Behboodi, University of Nebraska Omaha, United StatesCopyright © 2024 Clewes, Hammond, Dong, Meyer, Lowe and Rose. This is an open-access article distributed under the terms of the Creative Commons Attribution License (CC BY). The use, distribution or reproduction in other forums is permitted, provided the original author(s) and the copyright owner(s) are credited and that the original publication in this journal is cited, in accordance with accepted academic practice. No use, distribution or reproduction is permitted which does not comply with these terms.
*Correspondence: Jessica Rose, amVzc2ljYS5yb3NlQHN0YW5mb3JkLmVkdQ==