- Department of Cognitive Science, Institute of Philosophy, Jagiellonian University, Kraków, Poland
Introduction: Recent studies have suggested that transcranial alternating current stimulation (tACS), and especially the theta-frequency tACS, can improve human performance on working memory tasks. However, evidence to date is mixed. Moreover, the two WM tasks applied most frequently, namely the n-back and change-detection tasks, might not constitute canonical measures of WM capacity.
Method: In a relatively large sample of young healthy participants (N = 62), we administered a more canonical WM task that required stimuli recall, as well as we applied two WM tasks tapping into other key WM functions: attention control (the antisaccade task) and relational integration (the graph mapping task). The participants performed these three tasks three times: during the left frontal 5.5-Hz and the left parietal 5.5-Hz tACS session as well as during the sham session, with a random order of sessions. Attentional vigilance and subjective experience were monitored.
Results: For each task administered, we observed significant gains in accuracy neither for the frontal tACS session nor for the parietal tACS session, as compared to the sham session. By contrast, the scores on each task positively inter-correlated across the three sessions.
Discussion: The results suggest that canonical measures of WM capacity are strongly stable in time and hardly affected by theta-frequency tACS. Either the tACS effects observed in the n-back and change detection tasks do not generalize onto other WM tasks, or the tACS method has limited effectiveness with regard to WM, and might require further methodological advancements.
Introduction
A key cognitive function is working memory (WM) – the brain network responsible for the active maintenance, transformation, and recall of information for the task at hand (Cowan, 2022). WM capacity positively predicts performance on multiple cognitive tasks such as stimulus discrimination (Troche et al., 2014), learning (Kaufman et al., 2009), language comprehension (Daneman and Merikle, 1996), reasoning (Chuderski, 2013), and problem solving (Wittmann and Süß, 1999). The recent decade has erupted with studies using various non-invasive interventions in order to improve WM, including neurofeedback (for review see Jiang et al., 2022) and transcranial magnetic stimulation (TMS; for review see Sloan et al., 2021). Another non-invasive method, growing in popularity, is transcranial electrical stimulation (tES). Evidence (see Thut et al., 2011; Abd Hamid et al., 2015; Santarnecchi et al., 2015; Herrmann et al., 2016; Galli et al., 2019; Lee et al., 2021; Grover et al., 2023; Wischnewski et al., 2023) suggested that it might be possible to improve WM using transcranial direct current stimulation (tDCS) and alternating current stimulation (tACS). Importantly, injected currents are so weak (typically 1000 mA−2000 mA) that they are barely detected and quickly habituated by participants, so placebo-controlled experiments (including so-called sham condition pretending real stimulation) can be easily implemented to test the effectiveness of various stimulation protocols, with great potential for both basic cognitive neuroscience research and clinical interventions.
As WM mechanisms are frequently associated with patterns of neural oscillations (Axmacher et al., 2010; Palva et al., 2010; Lisman and Jensen, 2013; Roux and Uhlhaas, 2014; Sauseng et al., 2019), the tACS technique has been especially popular in the ongoing attempts to enhance WM. A number of tACS studies (e.g., Hoy et al., 2015; Alekseichuk et al., 2016; Feurra et al., 2016; Santarnecchi et al., 2016; Tseng et al., 2016; Möller et al., 2017; Borghini et al., 2018; Pahor and Jaušovec, 2018; Misselhorn et al., 2020; Thompson et al., 2021; Kim et al., 2022; Palm et al., 2022; Park et al., 2022; Zeng et al., 2022; Grover et al., 2023; Kvašnák et al., 2023) targeted the fast rhythms, such as the gamma (>30 Hz) and, more rarely, the beta band (14 Hz−30 Hz), following the fact that coordinated fast oscillations were linked with active maintenance of particular objects in WM (e.g., Lisman and Jensen, 2013; Leszczyński et al., 2015). Several such studies reported positive effects of stimulation on WM (e.g., Hoy et al., 2015; Alekseichuk et al., 2016). The alpha band activity (8–13 Hz) was associated with distractor suppression in WM in MEG (Bonnefond and Jensen, 2012), EEG (Zhou et al., 2023) and TMS studies (Hamidi et al., 2009; Sauseng et al., 2009), but it was also related with coordination and controlled access (Klimesch, 2012), especially in visuo-spatial WM (Roux and Uhlhaas, 2014). The alpha band was targeted by tACS less frequently, and such studies reported its negligible effects on WM performance (e.g., Feurra et al., 2016; Borghini et al., 2018; Soutschek et al., 2022; Chen et al., 2023).
The largest number of tACS studies (e.g., Jaušovec and Jaušovec, 2014; Jaušovec et al., 2014; Meiron and Lavidor, 2014; Vosskuhl et al., 2015; Alekseichuk et al., 2016, 2017; Feurra et al., 2016; Santarnecchi et al., 2016; Kleinert et al., 2017; Borghini et al., 2018; Pahor and Jaušovec, 2018; Röhner et al., 2018; Tseng et al., 2018; Wolinski et al., 2018; Bender et al., 2019; Jones et al., 2019; Reinhart and Nguyen, 2019; Abellaneda-Pérez et al., 2020; Guo et al., 2021; Hosseinian et al., 2021; Sahu and Tseng, 2021; Biel et al., 2022; Draaisma et al., 2022; Hu et al., 2022; Soutschek et al., 2022; Zeng et al., 2022; Zhang et al., 2022; Grover et al., 2023; Rauh et al., 2023; Yang et al., 2023) targeted the theta band (4 Hz−7 Hz), which was proposed as the brain rhythm most strongly involved in encoding and maintaining the item sequences in WM (e.g., Lisman and Idiart, 1995; Jacob et al., 2018; Sauseng et al., 2019).
In one of the first attempts to stimulate the theta band frequency, Jaušovec et al. (2014) induced the current for 15 min at the individually adapted theta frequency, and then applied to his participants the forward and backward recall tasks as well as the 3-back task. The two recall tasks required repeating the sequence of items in either the same or the reversed order, respectively. The 3-back task required memorizing the last three items of a continuous stream of items, and responding each time the current item matched the item shown three items back. Depending on the stimulation location (left frontal, left parietal, right parietal), an improvement in each task was observed, as compared to the sham condition. A corresponding improvement for the forward and backward recall tasks was replicated by Vosskuhl et al. (2015) as a result of stimulating at individual theta frequency minus 1 Hz (i.e., presumably slowing down a key theta rhythm). Zhang et al. (2022) observed no increase for the visuospatial forward recall task when slowing the theta down, but noted an increase when tuning stimulation precisely to individual theta frequency (at around 6 Hz). Null effects were reported also by Feurra et al. (2016) for 5-Hz tACS. We are aware of no more replications of the theta stimulation effects on the working memory recall tasks in healthy adults. For the n-back task, enhancement effects of the theta frequency stimulation were suggested by Alekseichuk et al. (2016), Abellaneda-Pérez et al. (2020), and Biel et al. (2022) for 6 Hz, and Zeng et al. (2022) for 8 Hz.
Jaušovec and Jaušovec (2014; see also Reinhart and Nguyen, 2019) replicated the theta stimulation effect targeting individual theta frequency also for another WM task – the change detection task, in which a pattern of several items was masked and then replaced either by the same pattern or the pattern with a single item changed, and the participants had to detect the change. Resembling the Vosskuhl et al. (2015) findings, but in contrast with Zhang et al. (2022), Wolinski et al. (2018) found that stimulating at 4 Hz increased change detection for stimuli contralateral to the stimulation, while the 7-Hz protocol deteriorated change detection. Bender et al. (2019) reported a similar effect for 4 Hz, and Sahu and Tseng (2021) found it for 5 Hz. Analogous effects were observed for 5-Hz repetitive TMS in the visual (Riddle et al., 2020) and auditory matching task (Albouy et al., 2017).
However, other studies yielded null or at best mixed results regarding the theta-frequency tACS for the n-back (Pahor and Jaušovec, 2018; Röhner et al., 2018; Jones et al., 2019; Soutschek et al., 2022; Zeng et al., 2022; Yang et al., 2023) and change-detection task (Santarnecchi et al., 2016; Alekseichuk et al., 2017; Kleinert et al., 2017; Pahor and Jaušovec, 2018; Tseng et al., 2018; Zhang et al., 2022).
The above summary suggests that the majority of WM measures in the theta tACS studies so far comprised the n-back and change-detection tasks. However, even though the n-back task is frequently used in cognitive neuroscience (probably due to its perceptual and response simplicity), it is not a canonical WM task, because beyond WM maintenance it also requires rapid decision making (which relies on individual decisional criteria) and memory updating (which involves specific cognitive functions such as memory removal; see Ecker et al., 2014), and thus this task not always fully overlaps with more typical WM recall tasks (Kane et al., 2007; Schmiedek et al., 2009). Relatedly, the change detection task is mainly interpreted as a measure of the visual short-term memory buffer capacity (Cowan, 2022), and it can be easily turned into an iconic memory measure (Sligte et al., 2008), so the construct it taps might not be equivalent to general WM capacity (see Jastrzebski et al., 2021). By contrast, more canonical WM tasks require encoding, storage, and serial or free recall of a number of distinctive memory items (see Unsworth and Engle, 2007). So, more stimulation studies using typical recall tasks are needed to generalize the theta-frequency tACS effects onto a broader WM construct.
Moreover, WM capacity has frequently been associated with another two key functions – attention control and relational integration – which have never been targeted by the theta-frequency tACS thus far. Attention control is the ability for goal-directed processing that blocks goal-unrelated distraction (Kane and Engle, 2002). Capacious WM may reflect strong attention on items to be memorized, while ignoring the irrelevant stimuli. In this perspective, WM is viewed as a part of executive functions architecture underlying flexible, controlled cognition (McCabe et al., 2010; Diamond, 2013). Previous work suggested a key role of the theta rhythm for neural control and communication (Colgin, 2013), and several recent studies linked frontal midline theta to control within and over WM (e.g., Cavanagh and Frank, 2014; Berger et al., 2019; Ratcliffe et al., 2022). Thus, it was crucial to examine if processing on a task that taps into control over distraction can be modulated by stimulation at the theta frequency.
Relational integration reflects the ability to construct meaningful structures (sequences, relations) from single items held in WM (Oberauer and Lewandowsky, 2008). Tasks capturing relation integration strongly predict reasoning and problem solving and explain more or less the same variance that is measured by more typical WM tasks (Jastrzebski et al., 2020). Processing relations in the brain was also frequently linked to the theta rhythms (e.g., Brzezicka et al., 2011; Knowlton et al., 2012; Zhang et al., 2015), so theta band seemed the most promising target for relational integration stimulation.
Our general objective was to validate theta-frequency tACS modulation of WM, which is the most popular line of tACS-WM research. More specifically, our objective was to extend the existing tACS literature with regard to the three above discussed WM functions: storage and recall, attention control, and relational integration. To this aim we applied the theta-frequency stimulation to a relatively large sample of participants (N = 62), as compared to the sample sizes of tACS studies in the WM domain examined so far (Ns ≈ 30). Using a within-subjects design, we applied the frontal and the parietal stimulation, and compared their effects to the sham condition. We chose a fixed theta frequency of 5.5 Hz, because the mean frequency applied in 47 separate experiments reported thus far equaled 5.47 Hz, which is close to the medium point of a typical theta band range (i.e., 4 Hz−7 Hz).
Method
Participants
Healthy adult participants were recruited via internet advertisements from a general population in Krakow, Poland. The age range of 38 women and 24 men was 18–29 years (M = 22.5, SD = 2.6). The participants had normal or corrected-to-normal vision and no history of neurological problems. During recruitment to the study and before each its session, each participant underwent a safety survey to make sure that there were no health issues that would prevent them from participating. The participants were informed that their data would be anonymous and they could interrupt the data collection and exit the laboratory at any moment. They signed a written consent form to participate and were paid the equivalent of 30 euros in local currency. Each participant was tested in a soundproof, dimly lit cabin, under the supervision of an experimenter. The study was approved by the local ethics board, and fully conformed with the Declaration of Helsinki.
WM tasks
One task per each of three investigated WM functions was applied. In order to avoid that any differences in the potential stimulation effects between the tasks could depend on their visual characteristics, we made these characteristics similar across the tasks, with each task including colored geometric shapes. The tasks are described in the order they were attempted by participants.
Attention control – the antisaccade task: The antisaccade task is likely the most common and reliable test of attention control (Draheim et al., 2021). We adapted the task variant previously used in our laboratory (Jastrzebski et al., 2021), only substituting original arrows with colored squares. The task consisted of 100 trials. Each trial consisted of three events (see Figure 1A). First, a fixation point was presented at the center of the screen for between 3 s and 5 s (randomly, to avoid automatizing the task performance). Next, a rapidly flashing white circle (3 cm in size) was shown either on the left or right side of the screen for 0.25 s. Finally, 0.1 s after the circle onset, a 2-cm blue, green, or brown square was presented at the location opposite to the circle for 0.15 s and was then replaced by a mask. The visual angle from both the circle and the square to the fixation point was around 8 angular degrees. The task was to look away from the flashing square, to detect the color of the square, and to press a key associated with this color, as indicated by the color sticks attached. Participants were given 1.75 s to respond, otherwise the trial elapsed. The score in the task consisted of the proportion of correct responses out of 100.
Storage and recall – the color recall task: The recall task was designed specifically for the purposes of the current study. It also consisted of 100 trials. Each trial (see Figure 1B) started with the fixation point shown for 1 s to 3 s. Next, the rapidly flashing white circle was shown at the center of the screen for 0.25 s. With the circle offset, squares in yellow, green, and brown colors were shown centrally at random positions (in an area spanning 6 angular degrees). There were always from one to four squares per color, totaling to seven squares. After 0.5 s, the squares were replaced by a mask. Exactly 0.5 s after the mask onset, the white rim was shown at the location of one of the previously shown colored squares, and the task was to press, within 1.75 s, the key marked with the color stick matching its color. The task score was the proportion of correct responses out of 100.
Relational integration – the graph mapping task: We used the graph mapping task originally developed by Jastrzebski et al. (2023), which is a highly valid measure of relational integration. Each trial started with the fixation point shown for 0 to 2 s followed with main relational integration task. In this task two perceptually-different but relationally-isomorphic directed graphs were presented on the left- and right-hand side of the screen, with each graph consisting of black dots representing vertices and directed arrows representing edges (see Figure 1C). Each graph occupied area spanning around 6 angular degrees horizontally and vertically. Participants were required to match two vertices across the two graphs. Some vertices could be identified by the unique number of incoming and outgoing edges (its degree). Encoding the degree required representing a relation of the specific vertex with the number of its incoming and outgoing edges. Such a relation might be binary (e.g., “the vertex has one incoming edge”) or ternary (e.g., “one incoming and two outgoing edges”). Some other vertices had to be identified by representing the unique connections with the other vertices, such as “the vertex with one outgoing edge connects to the vertex with another two incoming vertices.” Therefore, the task required to integrate the relations between vertices and edges into a more complex graph structure. The two vertices to be mapped were highlighted in the left-hand graph with the blue and yellow color, respectively. The task was to click with the left mouse button on a vertex of the right-hand graph which mapped relationally onto the blue vertex in the left-hand graph, and to click with the right mouse button on a vertex of the right-hand graph which mapped onto the yellow vertex of the left-hand graph (the order of clicks was irrelevant). The participants were allowed (9 + 1.5*k)s for responding before the trial elapsed (where k = 1 to 5 represents level of difficulty of task). There were 36 trials. The task score was the proportion of correct responses, defined as the correct mapping of both the blue and the yellow vertex in a trial, out of 36 trials.
Apparatus
The three tasks were controlled and displayed using a high-performance PC computer. The electrical stimulation was applied using the StarStim device (Neuroelectrics, Barcelona, Spain). To deliver the tACS, two 5 sq cm circular electrodes were used. Two stimulation layouts were applied (see Figure 2). For the frontal layout, the F3 was the stimulating electrode and the Fp1 was the return electrode (i.e., stimulation area including left dorsolateral prefrontal cortex, DLPFC). For the parietal layout, the respective electrodes were the P3 and the O1 (stimulation area including associative visual cortex and inferior parietal lobule, IPL). The F3 and/or P3 electrode were targeted by 18 studies (Jaušovec and Jaušovec, 2014; Jaušovec et al., 2014; Meiron and Lavidor, 2014; Feurra et al., 2016; Santarnecchi et al., 2016; Alekseichuk et al., 2017; Borghini et al., 2018; Pahor and Jaušovec, 2018; Röhner et al., 2018; Tseng et al., 2018; Jones et al., 2019; Reinhart and Nguyen, 2019; Abellaneda-Pérez et al., 2020; Hosseinian et al., 2021; Thompson et al., 2021; Biel et al., 2022; Hu et al., 2022; Rauh et al., 2023), which comprise the majority of theta-tACS studies on WM administered so far. Some of the above and 7 other studies targeted also the F4 and/or P4 electrodes, that is, right DLPFC and right IPL (Kleinert et al., 2017; Wolinski et al., 2018; Bender et al., 2019; Guo et al., 2021; Sahu and Tseng, 2021; Draaisma et al., 2022; Zhang et al., 2022). Because meta-analyses suggested that primarily the left DLPCF and IPL structures are associated with WM functions (Hill et al., 2014) and processing relations (Wertheim and Ragni, 2018), and the F3 and P3 electrodes comprised the most frequent choice among existing theta-tACS studies, we decided to target the F3 and P3 electrodes also in the current study.
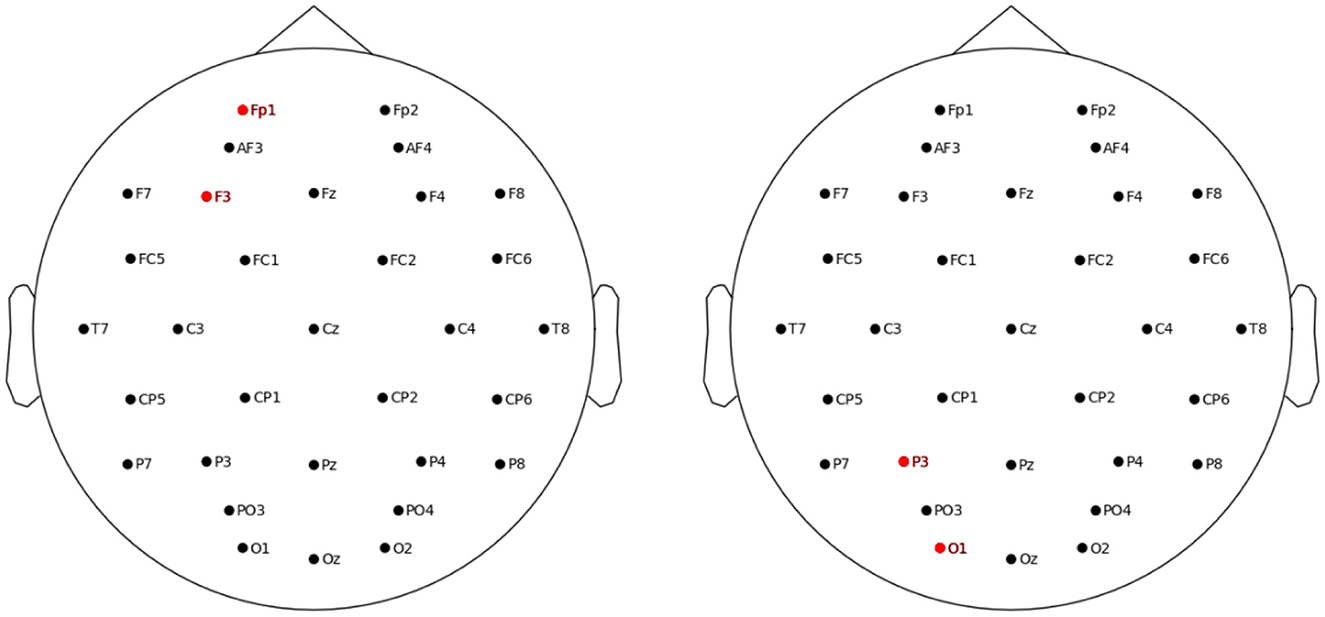
Figure 2. Location of the left frontal (left panel) and left parietal (right panel) stimulation electrodes.
Procedure
Each participant visited the sound-proof, dimly-lit laboratory for three sessions, each lasting ~1 h. Two consecutive sessions were separated by at least 24 h, for any previous session effects to fade out. Each session started with mounting the electrode cap and the short training for the three tasks. Then, the three tasks were administered. Together with the antisaccade task onset, either a stimulation or the sham condition started. In the verum stimulation, the 2000-mA current was injected at 5.5 Hz frequency (i.e., the anode and cathode switched eleven times per second) for 20 min (i.e., below maximum 30 min stimulation time recommended for our tACS device), starting with the 30-s ramping up interval, and ending with 30-s fading out interval, with 19 min of full power stimulation in-between. The stimulation end co-occurred with the late stage of the recall task, so the antisaccade and the recall task were performed during stimulation, while the graph mapping task – just after the stimulation (as a recent meta-analysis suggests, offline tACS aftereffects are comparable to active stimulation; Grover et al., 2023). In the sham condition, there was only 30 s of ramping up after the antisaccade task onset, followed immediately by 30 s of fading out (no actual stimulation), in order to yield a similar initial subjective experience as in the verum stimulation. Half of participants received sham in the frontal layout, half – in the parietal layout. As a result of the procedure, three respective task scores were obtained in each of the three sessions, administered to each participant in the random order.
Before and after each session, the participants performed a vigilance check by observing a clock on the computer screen for 5 min. Five times per minute, the second hand “jumped” by 2 s instead of 1 second. The participants were required to focus attention on the second hand and press the key each time it jumped. The goal of introducing this task was to monitor the participants' level of attention during the session, as a drop in that level might affect potential stimulation gains. After each session, the participants (with one participant failing at this stage) reported qualitatively any of their negative subjective experience related to stimulation as well as they evaluated qualitatively its strength (from 0 = none via 5 = moderate up to 10 = very strong).
Results
Table 1 presents the descriptive statistics for the three tasks and the three sessions. All the scores yielded normal distribution, with absolute values of both skew and kurtosis falling below 0.8. Figure 3 presents box-plots for sham-corrected performance in the frontal and parietal stimulation sessions.

Table 1. Mean scores (M), their standard deviations (SD) and ranges for the antisaccade, recall, and graph mapping task for the frontal and parietal verum stimulation sessions and the sham session.
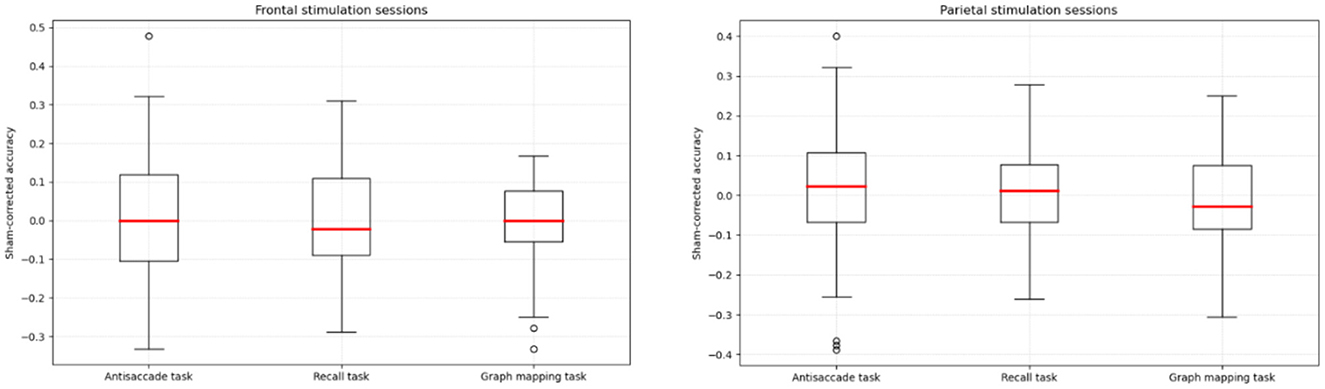
Figure 3. Sham-corrected accuracy (stimulation condition minus sham condition) in the antisaccade, recall, and graph mapping task, respectively, for the frontal and parietal verum stimulation sessions.
To test whether the particular task scores differed significantly between the sessions, each score was submitted to repeated measures ANOVA with a single factor of session (frontal, parietal, sham). No effect of session was statistically significant: F(2,122) = 0.097, p = 0.907, η2 = 0.002 for the antisaccade task, F(2,122) = 0.145, p = 0.865, η2 = 0.002 for the recall task, and F(2,122) = 0.672, p = 0.513, η2 = 0.010 for the graph mapping task. In order to confirm that these results can support the null hypothesis, we calculated the Bayes factor in favor of the null hypothesis, which yielded values of BF01 = 16.40, BF01 = 16.41, BF01 = 10.18, respectively, that is, each value provided strong support for the hypothesis that our tACS stimulation yielded no effect on any of task scores, as compared to the sham condition. Null stimulation effects were also found, each p > 0.50, after seven participants identified in Figure 2 as outliers (an absolute difference larger than M = 0.30 for a given task score observed between any stimulation session and the sham session) were excluded from the analysis.
A factor that needs to be accounted for in any test-retest design is the size of regression to the mean effect, that is, the tendency for people why scored externally low (high) in one session to score less low (less high) in another session (see Smoleń et al., 2018). In order to qualify the regression to the mean effect, we computed correlations between the given task's score in the sham session and the gain (a difference in that score between either the frontal or the parietal stimulation session and the sham session). The consecutive correlations equaled r = −0.555 (antisaccade, frontal), r = −0.546 (antisaccade, parietal), r = −0.332 (recall, frontal), r = −0.265 (recall, parietal), r = −0.473 (graph mapping, frontal), r = −0.693 (graph mapping, parietal), each p < 0.001, except for p = 0.037 for the parietal stimulation of recall. In consequence, we observed moderate to strong regression to the mean. Moreover, there was an analogous trend for the parietal stimulation gain to be large (small), if the frontal stimulation gain was large (small), and positive (negative) when the latter was positive (negative), with substantial correlations of r = 0.425 for the antisaccade, r = 0.353 for the recall, and r = 0.616 for the graph mapping task (see Figure 4).
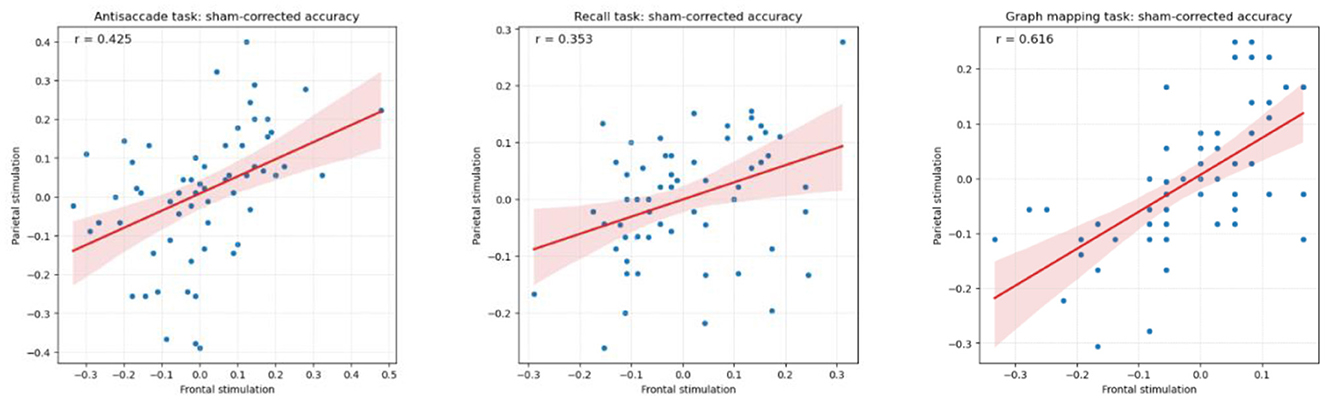
Figure 4. Pearson correlation between sham-corrected accuracy in the frontal and the parietal stimulation condition (respective stimulation condition minus sham condition) for the antisaccade, recall, and graph mapping task, showing substantial stability of performance in these two conditions.
There was a marginal drop in the clock task accuracy between pre-test (M = 0.991) and post-test (M = 0.989), F(1,61) = 4.15, p = 0.046, η2 = 0.064, but accuracy was very high anyway and was not affected by the session factor, F(2,122) = 1.50, p = 0.222. No effect pertained to time of reaction to the hand jumps, each p > 0.20. Individual accuracy never dropped below 0.90, and typically fell in the 0.95–1.0 range. Therefore, any potential gains from stimulation could not be counteracted by attention lapses.
Finally, out of 183 cases of reported subjective experience, in 47 (25.7%) cases the participants reported no negative experience, in 82 (44.8%) cases they reported weak experience (scores 1–3), in 37 (20.2%) cases – moderate experience (scores 4–6), and in the remaining 17 (9.3%) cases – strong experience (scores 7–10). Most of descriptions of experience referred to feelings of (in the order of appearance): tingling, smarting, heat, tickling, pain, lassitude, stinging, and current flow. In seven cases, some visual effects (flashes, dots, eye watering) were reported. There were significant and strong differences in subjective experience strength across the sessions, F(2,120) = 17.99, p < 0.001, η2 = 0.230, with the frontal stimulation (mean score = 3.5) yielding a stronger experience than the sham (mean score = 2.4), F(1,60) = 12.88, p < 0.001, and the sham yielding a stronger experience than the parietal stimulation (mean score = 1.7), F(1,60) = 5.65, p = 0.021. The latter difference, however, was driven by the frontal sham (mean score = 2.9), while the parietal sham yielded comparable experience (mean score = 1.9) to the parietal stimulation, F < 1. The frontal sham and the frontal stimulation experience did not differ significantly, either, F < 1. The three estimates of experience strength (i.e., frontal, parietal, and sham) moderately correlated, with mean r = 0.432, each p < 0.001, but neither performance nor gains in any of the three tasks were significantly related with any of these subjective estimates or their differences. So, the subjective experience related with the procedure was primarily determined by the individual proneness to report such experience as well as by the area of the skull involved (stronger experience reported for the frontal than the parietal area). By contrast, the presence or absence of actual stimulation did not matter for the strength of subjective experience, and the latter was unrelated to the indices of performance on the WM tasks.
Discussion
This study aimed to add to the unequivocal literature pertaining to effects of the theta-frequency alternating current stimulation on the WM performance, extending the range of examined tasks from primarily the n-back and change-detection tasks (neither being a canonical WM task) to three tasks that tap into central functions of working memory: attention control, serial recall, and relational integration. We controlled for the level of attentional vigilance during the sessions as well as obtained self-reports on subjective experience related to either verum or sham stimulation. Neither the frontal nor the parietal left hemisphere stimulation yielded any significant effect for the three WM tasks, despite the fact that for the present design our relatively large sample allowed for detecting effects larger than Cohen's f = 0.20 with 95% power, and effects larger than f = 0.15 with 80% power. Thus, we should have observed even medium effects if they existed. In consequence, this study provided evidence in line with studies that failed to observe clear effects of the theta frequency stimulation on working memory performance (e.g., Kleinert et al., 2017; Pahor and Jaušovec, 2018; Röhner et al., 2018; Jones et al., 2019; Soutschek et al., 2022). Our findings match also outcomes of a recent meta-analysis, suggesting no significant TMS effects on WM (Patel et al., 2020).
Another possibility may be that even though the n-back task and the change-detection task performance can indeed be sensitive to the theta-frequency stimulation (e.g., Alekseichuk et al., 2016; Tseng et al., 2018; Wolinski et al., 2018; Bender et al., 2019; Abellaneda-Pérez et al., 2020; Riddle et al., 2020; Sahu and Tseng, 2021; Zeng et al., 2022), this effect does not easily generalize onto the recall tasks (contrasting Jaušovec et al., 2014; Vosskuhl et al., 2015), as well as to attention control and relation integration tasks (no data available thus far).
One plausible explanation of the null results of our stimulation procedure is suggested by the strong effect of the regression to the mean. The fact that the performance in the sham session strongly but negatively predicted performance in the stimulation sessions, together with fact that after subtracting the sham performance the resulting difference in the frontal and parietal stimulation sessions correlated strongly positively, indicate that actually the individual performance across sessions was exceptionally stable. As the recall (Unsworth and Engle, 2007), the antisaccade (Draheim et al., 2021), and the graph mapping task (Jastrzebski et al., 2020) are known as highly reliable and valid markers of individual differences in WM capacity, scores on these tasks may reflect individual effectiveness of neurocognitive mechanisms underlying such capacity, which might be difficult to change with a relatively simple tACS intervention (see analogous failures to increase WM capacity and fluid intelligence using cognitive training protocols; Sala et al., 2019; Ripp et al., 2022). At the same time, the n-back and the change detection tasks, as less reliable and less indicative of individual differences in WM capacity (see Schmiedek et al., 2009; Jastrzebski et al., 2021), may be more sensitive to subtle improvements to the testing conditions resulting from tACS (e.g., improved attention focusing). These two tasks impose also simpler processing requirements (recognizing whether the current stimulus is the same as, or different from, 2–4 other simple stimuli), as compared to the recall tasks (reproduction of a list of stimuli in the correct order), the antisaccade task (going through a sequence of rapid events on the screen), and the graph mapping task (representation and transformation of a complex mental structure). With a lower complexity of a WM task, it might be more likely that the tACS interventions tap into the processes that translate into the final task score, while in more complex tasks only improving the coordination of an entire network of processes (and not a single mechanism) can affect the final score. Definitely, future studies of tACS effects on WM should take into account the requirement of various types of tasks applied to measure this construct.
This study had certain limitations. First, like most of other studies, we applied only a single stimulation session to our participants (i.e., did not successively repeat stimulation of the same skull area). Perhaps, like in some clinical areas of tACS intervention (e.g., Sprugnoli et al., 2021), multiple sessions are necessary to observe significant stimulation effects. However, at this stage of tACS research, combining large samples and sophisticated cognitive tasks with multiple sessions would be unfeasible practically. Second, perhaps cognitive performance of young healthy adults is close to ceiling, and thus difficult to further improve, so it might be easier to observe significant tACS effects in non-adult samples (children, older people) or in clinical populations. However, we were primarily interested in studying general effects of tACS. Third, we stimulated at the fixed frequency of 5.5 Hz, while using an individually adapted theta frequency might have yielded a different outcome. Future tACS studies of the recall, antisaccade, and relation integration tasks could address these limitations.
Concluding, this study examined a relatively large (as for a tACS study) sample of young healthy participants, drawn from a general population (not entirely students), tested with three different but canonical WM tasks, and used two different stimulation protocols (left frontal and left parietal), in a within-subjects design, controlling also for attentional vigilance and subjective experience. Nevertheless, we observed no reliable effect of any tACS protocol on WM performance. As the recent replication crisis in psychology and neuroscience have shown that null findings (given they were obtained with a sufficient power) may be comparably important to science as are significant effects, this study adds a substantial piece of data to our existing (still scarce) knowledge on the mechanisms of WM and ways to improve them. Even though a recent meta-analysis of transcranial electrical stimulation studies (see Grover et al., 2023) suggested a moderate optimism with regard to improving cognitive performance, much more data from various populations, tasks, stimulation protocols is needed to create a more complete picture of opportunities and limitations related with the tACS technology, and the current study may help in creating such a more complete picture.
Data availability statement
The original contributions presented in the study are included in the article/Supplementary material, further inquiries can be directed to the corresponding authors.
Ethics statement
The studies involving humans were approved by the Faculty Research Ethics Committee, Philosophical Faculty, Jagiellonian University Kraków. The studies were conducted in accordance with the local legislation and institutional requirements. The participants provided their written informed consent to participate in this study.
Author contributions
MO: Writing – original draft, Conceptualization, Data curation, Investigation, Methodology, Software. SC: Investigation, Writing – review & editing. PB: Writing – review & editing, Visualization. AC: Writing – original draft, Conceptualization, Supervision, Formal analysis, Funding acquisition, Project administration, Validation.
Funding
The author(s) declare financial support was received for the research, authorship, and/or publication of this article. This study was supported by the National Science Centre Poland, grant #2019/33/B/HS6/00321.
Conflict of interest
The authors declare that the research was conducted in the absence of any commercial or financial relationships that could be construed as a potential conflict of interest.
Publisher's note
All claims expressed in this article are solely those of the authors and do not necessarily represent those of their affiliated organizations, or those of the publisher, the editors and the reviewers. Any product that may be evaluated in this article, or claim that may be made by its manufacturer, is not guaranteed or endorsed by the publisher.
Supplementary material
The Supplementary Material for this article can be found online at: https://www.frontiersin.org/articles/10.3389/fnhum.2024.1354671/full#supplementary-material
References
Abd Hamid, A. I., Gall, C., Speck, O., Antal, A., and Sabel, B. A. (2015). Effects of alternating current stimulation on the healthy and diseased brain. Front. Neurosci. 9, 391. doi: 10.3389/fnins.2015.00391
Abellaneda-Pérez, K., Vaqué-Alcázar, L., Perellón-Alfonso, R., Bargalló, N., Kuo, M. F., Pascual-Leone, A., et al. (2020). Differential tDCS and tACS effects on working memory-related neural activity and resting-state connectivity. Front. Neurosci. 13, 14440. doi: 10.3389/fnins.2019.01440
Albouy, P., Weiss, A., Baillet, S., and Zatorre, R. J. (2017). Selective entrainment of theta oscillations in the dorsal stream causally enhances auditory working memory performance. Neuron 94, 193–206. doi: 10.1016/j.neuron.2017.03.015
Alekseichuk, I., Pabel, S. C., Antal, A., and Paulus, W. (2017). Intrahemispheric theta rhythm desynchronization impairs working memory. Restor. Neurol. Neurosci. 35, 147–158. doi: 10.3233/RNN-160714
Alekseichuk, I., Turi, Z., De Lara, A., Antal, G. A., and Paulus, W. (2016). Spatial working memory in humans depends on theta and high gamma synchronization in the prefrontal cortex. Curr. Biol. 26, 1513–1521. doi: 10.1016/j.cub.2016.04.035
Axmacher, N., Henseler, M. M., Jensen, O., Weinreich, I., Elger, C. E., Fell, J., et al. (2010). Cross-frequency coupling supports multi-item working memory in the human hippocampus. Proc. Nat. Acad. Sci. 107, 3228–3233. doi: 10.1073/pnas.0911531107
Bender, M., Romei, V., and Sauseng, P. (2019). Slow theta tACS of the right parietal cortex enhances contralateral visual working memory capacity. Brain Topography 32, 477–481. doi: 10.1007/s10548-019-00702-2
Berger, B., Griesmayr, B., Minarik, T., Biel, A. L., Pinal, D., Sterr, A., et al. (2019). Dynamic regulation of interregional cortical communication by slow brain oscillations during working memory. Nat. Commun. 10, 1. doi: 10.1038/s41467-019-12057-0
Biel, A. L., Sterner, E., Röll, L., and Sauseng, P. (2022). Modulating verbal working memory with fronto-parietal transcranial electric stimulation at theta frequency: Does it work? Eur. J. Neurosci. 55, 405–425. doi: 10.1111/ejn.15563
Bonnefond, M., and Jensen, O. (2012). Alpha oscillations serve to protect working memory maintenance against anticipated distracters. Curr. Biol. 22, 1969–1974. doi: 10.1016/j.cub.2012.08.029
Borghini, G., Candini, M., Filannino, C., Hussain, M., Walsh, V., Romei, V., et al. (2018). Alpha oscillations are causally linked to inhibitory abilities in ageing. J. Neurosci. 38, 4418–4429. doi: 10.1523/JNEUROSCI.1285-17.2018
Brzezicka, A., Kamiński, M., Kamiński, J., and Blinowska, K. (2011). Information transfer during a transitive reasoning task. Brain Topograph. 24, 1–8. doi: 10.1007/s10548-010-0158-6
Cavanagh, J. F., and Frank, M. J. (2014). Frontal theta as a mechanism for cognitive control. Trends Cognit. Sci. 18, 414–421. doi: 10.1016/j.tics.2014.04.012
Chen, X., Ma, R., Zhang, W., Zeng, G. Q., Wu, Q., Yimiti, A., et al. (2023). Alpha oscillatory activity is causally linked to working memory retention. PLoS Biol. 21, e3001999. doi: 10.1371/journal.pbio.3001999
Chuderski, A. (2013). When are fluid intelligence and working memory isomorphic and when are they not?. Intelligence 41, 244–262. doi: 10.1016/j.intell.2013.04.003
Colgin, L. L. (2013). Mechanisms and functions of theta rhythms. Ann. Rev. Neurosci. 36, 295–312. doi: 10.1146/annurev-neuro-062012-170330
Cowan, N. (2022). Working memory development: a 50-year assessment of research and underlying theories. Cognition 224, 105075. doi: 10.1016/j.cognition.2022.105075
Daneman, M., and Merikle, P. M. (1996). Working memory and language comprehension: a meta-analysis. Psychonomic Bullet. Rev. 3, 422–433. doi: 10.3758/BF03214546
Diamond, A. (2013). Executive functions. Ann. Rev. Psychol. 64, 135–168. doi: 10.1146/annurev-psych-113011-143750
Draaisma, L. R., Wessel, M. J., Moyne, M., Morishita, T., and Hummel, F. C. (2022). Targeting the frontoparietal network using bifocal transcranial alternating current stimulation during a motor sequence learning task in healthy older adults. Brain Stim. 15, 968–979. doi: 10.1016/j.brs.2022.06.012
Draheim, C., Tsukahara, J. S., Martin, J. D., Mashburn, C. A., and Engle, R. W. (2021). A toolbox approach to improving the measurement of attention control. J. Exp. Psychol. Gen. 150, 242–275. doi: 10.1037/xge0000783
Ecker, U. K., Oberauer, K., and Lewandowsky, S. (2014). Working memory updating involves item-specific removal. J. Memory Lang. 74, 1–15. doi: 10.1016/j.jml.2014.03.006
Feurra, M., Galli, G., Pavone, E. F., Rossi, A., and Rossi, S. (2016). Frequency-specific insight into short-term memory capacity. J. Neurophysiol. 116, 153–158. doi: 10.1152/jn.01080.2015
Galli, G., Vadillo, M. A., Sirota, M., Feurra, M., and Medvedeva, A. (2019). A systematic review and meta-analysis of the effects of transcranial direct current stimulation (tDCS) on episodic memory. Brain Stim. 12, 231–241. doi: 10.1016/j.brs.2018.11.008
Grover, S., Fayzullina, R., Bullard, B. M., Levina, V., and Reinhart, R. M. (2023). A meta-analysis suggests that tACS improves cognition in healthy, aging, and psychiatric populations. Sci. Transl. Med. 15, eabo2044. doi: 10.1126/scitranslmed.abo2044
Guo, X., Li, Z., Zhang, L., and Liu, Q. (2021). Modulation of visual working memory performance via different theta frequency stimulations. Brain Sci. 11, 1358. doi: 10.3390/brainsci11101358
Hamidi, M., Tononi, G., and Postle, B. R. (2009). Evaluating the role of prefrontal and parietal cortices in memory-guided response with repetitive transcranial magnetic stimulation. Neuropsychologia 47, 295–302. doi: 10.1016/j.neuropsychologia.2008.08.026
Herrmann, C. S., Strüber, D., Helfrich, R. F., and Engel, A. K. (2016). EEG oscillations: from correlation to causality. Int. J. Psychophysiol. 103, 12–21. doi: 10.1016/j.ijpsycho.2015.02.003
Hill, A. C., Laird, A. R., and Robinson, J. L. (2014). Gender differences in working memory networks: a BrainMap meta-analysis. Biol. Psychol. 102, 18–29. doi: 10.1016/j.biopsycho.2014.06.008
Hosseinian, T., Yavari, F., Kuo, M. F., Nitsche, M. A., and Jamil, A. (2021). Phase synchronized 6 Hz transcranial electric and magnetic stimulation boosts frontal theta activity and enhances working memory. Neuroimage 245, 118772. doi: 10.1016/j.neuroimage.2021.118772
Hoy, K. E., Bailey, N., Arnold, S., Windsor, K., John, J., Daskalakis, Z. J., et al. (2015). The effect of γ-tACS on working memory performance in healthy controls. Brain Cognit. 101, 51–56. doi: 10.1016/j.bandc.2015.11.002
Hu, Z., Samuel, I. B. H., Meyyappan, S., Bo, K., Rana, C., Ding, M., et al. (2022). Aftereffects of frontoparietal theta tACS on verbal working memory: Behavioral and neurophysiological analysis. IBRO Neurosci. Rep. 13, 469–477. doi: 10.1016/j.ibneur.2022.10.013
Jacob, S. N., Hähnke, D., and Nieder, A. (2018). Structuring of abstract working memory content by fronto-parietal synchrony in primate cortex. Neuron 99, 588–597. doi: 10.1016/j.neuron.2018.07.025
Jastrzebski, J., Kroczek, B., and Chuderski, A. (2021). Galton and Spearman revisited: Can single general discrimination ability drive performance on diverse sensorimotor tasks and explain intelligence?. J. Exp. Psychol. Gen. 150, 1279. doi: 10.1037/xge0001005
Jastrzebski, J., Ociepka, M., and Chuderski, A. (2020). Fluid reasoning is equivalent to relation processing. Intelligence 82, 101489. doi: 10.1016/j.intell.2020.101489
Jastrzebski, J., Ociepka, M., and Chuderski, A. (2023). Graph Mapping: a novel and simple test to validly assess fluid reasoning. Behav. Res. Methods 55, 448–460. doi: 10.3758/s13428-022-01846-z
Jaušovec, N., and Jaušovec, K. (2014). Increasing working memory capacity with theta transcranial alternating current stimulation (tACS). Biol. Psychol. 96, 42–47. doi: 10.1016/j.biopsycho.2013.11.006
Jaušovec, N., Jaušovec, K., and Pahor, A. (2014). The influence of theta transcranial alternating current stimulation (tACS) on working memory storage and processing functions. Acta Psychol. 146, 1–6. doi: 10.1016/j.actpsy.2013.11.011
Jiang, Y., Jessee, W., Hoyng, S., Borhani, S., Liu, Z., Zhao, X., et al. (2022). Sharpening working memory with real-time electrophysiological brain signals: which neurofeedback paradigms work? Front. Aging Neurosci. 14, 780817. doi: 10.3389/fnagi.2022.780817
Jones, K. T., Arciniega, H., and Berryhill, M. E. (2019). Replacing tDCS with theta tACS provides selective, but not general WM benefits. Brain Res. 1720, 146324. doi: 10.1016/j.brainres.2019.146324
Kane, M. J., Conway, A. R., Miura, T. K., and Colflesh, G. J. (2007). Working memory, attention control, and the N-back task: a question of construct validity. J. Exp. Psychol. Learn. Memory Cognit. 33, 615–622. doi: 10.1037/0278-7393.33.3.615
Kane, M. J., and Engle, R. W. (2002). The role of prefrontal cortex in working-memory capacity, executive attention, and general fluid intelligence: an individual-differences perspective. Psychon. Bullet. Rev. 9, 637–671. doi: 10.3758/BF03196323
Kaufman, S. B., DeYoung, C. G., Gray, J. R., Brown, J., and Mackintosh, N. (2009). Associative learning predicts intelligence above and beyond working memory and processing speed. Intelligence 37, 374–382. doi: 10.1016/j.intell.2009.03.004
Kim, S. E., Kim, H. S., Kwak, Y., Ahn, M. H., Choi, K. M., Min, B. K., et al. (2022). Neurodynamic correlates for the cross-frequency coupled transcranial alternating current stimulation during working memory performance. Front. Neurosci. 16, 1013691. doi: 10.3389/fnins.2022.1013691
Kleinert, M. L., Szymanski, C., and Müller, V. (2017). Frequency-unspecific effects of θ-tACS related to a visuospatial working memory task. Front. Hum. Neurosci. 11, 367. doi: 10.3389/fnhum.2017.00367
Klimesch, W. (2012). Alpha-band oscillations, attention, and controlled access to stored information. Trends Cognit. Sci. 16, 606–617. doi: 10.1016/j.tics.2012.10.007
Knowlton, B. J., Morrison, R. G., Hummel, J. E., and Holyoak, K. J. (2012). A neurocomputational system for relational reasoning. Trends Cognit. Sci. 16, 373–381. doi: 10.1016/j.tics.2012.06.002
Kvašnák, E., Magyarová, E., Domankuš, M., Tesar, M., Kymplová, J., Fetissov, V., et al. (2023). 10 minutes frontal 40 Hz tACS—effects on working memory tested by luck-vogel task. Behav. Sci. 13, 39. doi: 10.3390/bs13010039
Lee, J. S. A., Bestmann, S., and Evans, C. (2021). A future of current flow modelling for transcranial electrical stimulation? Curr. Behav. Neurosci. Rep. 8, 150–159. doi: 10.1007/s40473-021-00238-5
Leszczyński, M., Fell, J., and Axmacher, N. (2015). Rhythmic working memory activation in the human hippocampus. Cell Rep. 13, 1272–1282. doi: 10.1016/j.celrep.2015.09.081
Lisman, J. E., and Idiart, M. A. (1995). Storage of 7±2 short-term memories in oscillatory subcycles. Science 267, 1512–1515. doi: 10.1126/science.7878473
Lisman, J. E., and Jensen, O. (2013). The theta-gamma neural code. Neuron 77, 1002–1016. doi: 10.1016/j.neuron.2013.03.007
McCabe, D. P., Roediger, I. I. I., McDaniel, H. L., and Balota, M. A. D. A., and Hambrick, D. Z. (2010). The relationship between working memory capacity and executive functioning: Evidence for a common executive attention construct. Neuropsychology 24, 222–243. doi: 10.1037/a0017619
Meiron, O., and Lavidor, M. (2014). Prefrontal oscillatory stimulation modulates access to cognitive control references in retrospective metacognitive commentary. Clin. Neurophysiol. 125, 77–82. doi: 10.1016/j.clinph.2013.06.013
Misselhorn, J., Göschl, F., Higgen, F. L., and Hummel, F. C., Gerloff, C.h., and Engel, A. K. (2020). Sensory capability and information integration independently explain the cognitive status of healthy older adults. Sci. Rep. 10, 22437. doi: 10.1038/s41598-020-80069-8
Möller, A., Nemmi, F., Karlsson, K., and Klingberg, T. (2017). Transcranial electric stimulation can impair gains during working memory training and affects the resting state connectivity. Front. Hum. Neurosci. 11, 364. doi: 10.3389/fnhum.2017.00364
Oberauer, K., and Lewandowsky, S. (2008). Forgetting in immediate serial recall: decay, temporal distinctiveness, or interference? Psychol. Rev. 115, 544–576. doi: 10.1037/0033-295X.115.3.544
Pahor, A., and Jaušovec, N. (2018). The effects of theta and gamma tACS on working memory and electrophysiology. Front. Hum. Neurosci. 11, 651. doi: 10.3389/fnhum.2017.00651
Palm, U., Baumgartner, C., Hoffmann, L., Padberg, F., Hasan, A., Strube, W., et al. (2022). Single session gamma transcranial alternating stimulation does not modulate working memory in depressed patients and healthy controls. Neurophysiol. Clin. 52, 128–136. doi: 10.1016/j.neucli.2022.03.002
Palva, J. M., Monto, S., Kulashekhar, S., and Palva, S. (2010). Neuronal synchrony reveals working memory networks and predicts individual memory capacity. Proc. Nat. Acad. Sci. 107, 7580–7585. doi: 10.1073/pnas.0913113107
Park, J., Lee, C., Lee, S., and Im, C. H. (2022). 80 Hz but not 40 Hz, transcranial alternating current stimulation of 80 Hz over right intraparietal sulcus increases visuospatial working memory capacity. Sci. Rep. 12, 1–8. doi: 10.1038/s41598-022-17965-8
Patel, R., Silla, F., Pierce, S., Theule, J., and Giard, T. A. (2020). Cognitive functioning before and after repetitive transcranial magnetic stimulation (rTMS): a quantitative meta-analysis in healthy adults. Neuropsychologia 141, 107395.
Ratcliffe, O., Shapiro, K., and Staresina, B. P. (2022). Fronto-medial theta coordinates posterior maintenance of working memory content. Curr. Biol. 32, 2121–2129. doi: 10.1016/j.cub.2022.03.045
Rauh, J., Theresa, H., Moritz, H., Christoph, M., and Gregor, L. (2023). Changes of WM network activity following HD-tACS revealed by simultaneous measurement of fMRI. Brain Stim. Basic Transl. Clin. Res. Neuromod. 16, 330. doi: 10.1016/j.brs.2023.01.620
Reinhart, R. M. G., and Nguyen, J. A. (2019). Working memory revived in older adults by synchronizing rhythmic brain circuits. Nat. Neurosci. 22, 5. doi: 10.1038/s41593-019-0371-x
Riddle, J., Scimeca, J. M., Cellier, D., Dhanani, S., and D'Esposito, M. (2020). Causal evidence for a role of theta and alpha oscillations in the control of working memory. Curr. Biol. 30, 1748–1754. doi: 10.1016/j.cub.2020.02.065
Ripp, I., Wu, Q., Wallenwein, L., Emch, M., Yakushev, I., Koch, K., et al. (2022). Neuronal efficiency following n-back training task is accompanied by a higher cerebral glucose metabolism. NeuroImage 253, 119095. doi: 10.1016/j.neuroimage.2022.119095
Röhner, F., Breitling, C., Rufener, K. S., Heinze, H. J., Hinrichs, H., Krauel, K., et al. (2018). Modulation of working memory using transcranial electrical stimulation: a direct comparison between TACS and TDCS. Front. Neurosci. 12, 761. doi: 10.3389/fnins.2018.00761
Roux, F., and Uhlhaas, P. J. (2014). Working memory and neural oscillations: alpha-gamma versus theta-gamma codes for distinct WM information?. Trends Cognit. Sci. 18, 16–25. doi: 10.1016/j.tics.2013.10.010
Sahu, P. P., and Tseng, P. (2021). Frontoparietal theta tACS nonselectively enhances encoding, maintenance, and retrieval stages in visuospatial working memory. Neurosci. Res. 172, 41–50. doi: 10.1016/j.neures.2021.05.005
Sala, G., Aksayli, N. D., Tatlidil, K. S., Gondo, Y., and Gobet, F. (2019). Working memory training does not enhance older adults' cognitive skills: a comprehensive meta-analysis. Intelligence 77, 101386. doi: 10.1016/j.intell.2019.101386
Santarnecchi, E., Brem, A. K., Levenbaum, E., Thompson, T., Kadosh, R. C., Pascual-Leone, A., et al. (2015). Enhancing cognition using transcranial electrical stimulation. Curr. Opin. Behav. Sci. 4, 171–178. doi: 10.1016/j.cobeha.2015.06.003
Santarnecchi, E., Muller, T., Rossi, S., Sarkar, A., Polizzotto, N. R., Rossi, A., et al. (2016). Individual differences and specificity of prefrontal gamma frequency-tACS on fluid intelligence capabilities. Cortex 75, 33–43. doi: 10.1016/j.cortex.2015.11.003
Sauseng, P., Klimesch, W., Heise, K. F., Gruber, W. R., Holz, E., Karim, A. A., et al. (2009). Brain oscillatory substrates of visual short-term memory capacity. Curr. Biol. 19, 1846–1852. doi: 10.1016/j.cub.2009.08.062
Sauseng, P., Peylo, C., Biel, A. L., and Friedrich, E. V., and Romberg-Taylor, C. (2019). Does cross-frequency phase coupling of oscillatory brain activity contribute to a better understanding of visual working memory? Br. J. Psychol. 110, 245–255. doi: 10.1111/bjop.12340
Schmiedek, F., Hildebrandt, A., Lövdén, M., Wilhelm, O., and Lindenberger, U. (2009). Complex span versus updating tasks of working memory: the gap is not that deep. J. Exp. Psychol. Learn. Memory Cognit. 35, 1089. doi: 10.1037/a0015730
Sligte, I. G., Scholte, H. S., and Lamme, V. A. (2008). Are there multiple visual short-term memory stores?. PLoS ONE 3, e1699. doi: 10.1371/journal.pone.0001699
Sloan, N. P., Byrne, L. K., Enticott, P. G., and Lum, J. A. G. (2021). Non-invasive brain stimulation does not improve working memory in schizophrenia: a meta-analysis of randomised controlled trials. Neuropsychol. Rev. 31, 115–138. doi: 10.1007/s11065-020-09454-4
Smoleń, T., Jastrzebski, J., Estrada, E., and Chuderski, A. (2018). Most evidence for the compensation account of cognitive training is unreliable. Mem. Cognit. 46, 1315–1330. doi: 10.3758/s13421-018-0839-z
Soutschek, A., Nadporozhskaia, L., and Christian, P. (2022). Brain stimulation over dorsomedial prefrontal cortex modulates effort-based decision making. Cognit. Aff. Behav. Neurosci. 22, 1264–1274. doi: 10.3758/s13415-022-01021-z
Sprugnoli, G., Munsch, F., Cappon, D., Paciorek, R., Macone, J., Connor, A., et al. (2021). Impact of multisession 40Hz tACS on hippocampal perfusion in patients with Alzheimer's disease. Alzheimer's Res. Ther. 13, 203. doi: 10.1186/s13195-021-00922-4
Thompson, L., Khuc, J., Saccani, M. S., Zokaei, N., and Cappelletti, M. (2021). Gamma oscillations modulate working memory recall precision. Exp. Brain Res. 239, 2711–2724. doi: 10.1007/s00221-021-06051-6
Thut, G., Schyns, P. G., and Gross, J. (2011). Entrainment of perceptually relevant brain oscillations by non-invasive rhythmic stimulation of the human brain. Front. Psychol. 2, 170. doi: 10.3389/fpsyg.2011.00170
Troche, S. J., Wagner, F. L., Voelke, A. E., Roebers, C. M., and Rammsayer, T. H. (2014). Individual differences in working memory capacity explain the relationship between general discrimination ability and psychometric intelligence. Intelligence 44, 40–50. doi: 10.1016/j.intell.2014.02.009
Tseng, P., Chang, Y. T., Chang, C. F., Liang, W. K., and Juan, C. H. (2016). The critical role of phase difference in gamma oscillation within the temporoparietal network for binding visual working memory. Sci. Rep. 6, 32138. doi: 10.1038/srep32138
Tseng, P., Iu, K. C., and Juan, C. H. (2018). The critical role of phase difference in theta oscillation between bilateral parietal cortices for visuospatial working memory. Sci. Rep. 8, 349. doi: 10.1038/s41598-017-18449-w
Unsworth, N., and Engle, R. W. (2007). The nature of individual differences in working memory capacity: active maintenance in primary memory and controlled search from secondary memory. Psychol. Rev. 114, 104–132. doi: 10.1037/0033-295X.114.1.104
Vosskuhl, J., Huster, R. J., and Herrmann, C. S. (2015). Increase in short-term memory capacity induced by down-regulating individual theta frequency via transcranial alternating current stimulation. Front. Hum. Neurosci. 9, 257. doi: 10.3389/fnhum.2015.00257
Wertheim, J., and Ragni, M. (2018). The neural correlates of relational reasoning: a meta-analysis of 47 functional magnetic resonance studies. J. Cognit. Neurosci. 30, 1734–1748. doi: 10.1162/jocn_a_01311
Wischnewski, M., Alekseichuk, I., and Opitz, A. (2023). Neurocognitive, physiological, and biophysical effects of transcranial alternating current stimulation. Trends Cognit. Sci. 27, 189–205. doi: 10.1016/j.tics.2022.11.013
Wittmann, W. W., and Süß, H. M. (1999). “Investigating the paths between working memory, intelligence, knowledge, and complex problem-solving performances via Brunswik symmetry,” in Learning and Individual Differences: Process, Trait, and Content Determinants, eds P. L. Ackerman, P. C. Kyllonen, and R. D. Roberts (New York, NY: American Psychological Association), 77–108.
Wolinski, N., Cooper, N. R., Sauseng, P., and Romei, V. (2018). The speed of parietal theta frequency drives visuospatial working memory capacity. PLoS Biol. 16, e2005348. doi: 10.1371/journal.pbio.2005348
Yang, D., Ghafoor, U., Eggebrecht, A. T., and Hong, K. S. (2023). Effectiveness assessment of repetitive transcranial alternating current stimulation with concurrent EEG and fNIRS measurement. Health Inf. Sci. Syst. 11, 35. doi: 10.1007/s13755-023-00233-y
Zeng, L., Guo, M., Wu, R., Luo, Y., and Wei, P. (2022). The effects of electroencephalogram feature-based transcranial alternating current stimulation on working memory and electrophysiology. Front. Aging Neurosci. 14, 828377. doi: 10.3389/fnagi.2022.828377
Zhang, D. W., Moraidis, A., and Klingberg, T. (2022). Individually tuned theta HD-tACS improves spatial performance. Brain Stim. 15, 1439–1447. doi: 10.1016/j.brs.2022.10.009
Zhang, L., Gan, J. Q., and Wang, H. (2015). Mathematically gifted adolescents mobilize enhanced workspace configuration of theta cortical network during deductive reasoning. Neuroscience 289, 334–348. doi: 10.1016/j.neuroscience.2014.12.072
Keywords: working memory, stimulation, transcranial alternate current stimulation (tACS), theta, theta frequency band
Citation: Ociepka M, Chinta SR, Basoń P and Chuderski A (2024) No effects of the theta-frequency transcranial electrical stimulation for recall, attention control, and relation integration in working memory. Front. Hum. Neurosci. 18:1354671. doi: 10.3389/fnhum.2024.1354671
Received: 12 December 2023; Accepted: 05 February 2024;
Published: 19 February 2024.
Edited by:
Gregor Thut, University of Glasgow, United KingdomReviewed by:
Nicolas Zink, University of California, Los Angeles, United StatesEugen Kvasnak, Charles University, Czechia
Copyright © 2024 Ociepka, Chinta, Basoń and Chuderski. This is an open-access article distributed under the terms of the Creative Commons Attribution License (CC BY). The use, distribution or reproduction in other forums is permitted, provided the original author(s) and the copyright owner(s) are credited and that the original publication in this journal is cited, in accordance with accepted academic practice. No use, distribution or reproduction is permitted which does not comply with these terms.
*Correspondence: Michał Ociepka, bWljaGFsLm9jaWVwa2FAdWouZWR1LnBs; Paweł Basoń, cGF3ZWwuYmFzb25Ac3R1ZGVudC51ai5lZHUucGw=