- 1Institute of Psychology, University of the Bundeswehr Munich, Neubiberg, Germany
- 2Department of Psychiatry and Psychotherapy, University of Regensburg, Regensburg, Germany
- 3Department of Electrical Engineering, University of the Bundeswehr Munich, Neubiberg, Germany
- 4Institute of Sport Science, University of the Bundeswehr Munich, Neubiberg, Germany
Aims: Motor evoked potentials (MEP) elicited by transcranial magnetic stimulation (TMS) over the primary motor cortex are used as a neurophysiological marker of cortical excitability in clinical and scientific practice. Though, the reliability of this outcome parameter has not been clarified. Using a systematic approach, this work reviews and critically appraises studies on the reliability of MEP outcome parameters derived from hand muscles of healthy subjects and gives a proposal for most reliable TMS practice.
Methods: A systematic literature research was performed in PubMed, according to the PRISMA guidelines. Articles published up to March 2023 that were written in English, conducted repeated measurements from hand muscles of healthy subjects and reliability analysis were included. The risk of publication bias was determined. Two authors conducted the literature search and rated the articles in terms of eligibility and methodological criteria with standardized instruments. Frequencies of the checklist criteria were calculated and inter-rater reliability of the rating procedure was determined. Reliability and stimulation parameters were extracted and summarized in a structured way to conclude best-practice recommendation for reliable measurements.
Results: A total of 28 articles were included in the systematic review. Critical appraisal of the studies revealed methodological heterogeneity and partly contradictory results regarding the reliability of outcome parameters. Inter-rater reliability of the rating procedure was almost perfect nor was there indication of publication bias. Identified studies were grouped based on the parameter investigated: number of applied stimuli, stimulation intensity, reliability of input-output curve parameters, target muscle or hemisphere, inter-trial interval, coil type or navigation and waveform.
Conclusion: The methodology of studies on TMS is still subject to heterogeneity, which could contribute to the partly contradictory results. According to the current knowledge, reliability of the outcome parameters can be increased by adjusting the experimental setup. Reliability of single pulse MEP measurement could be optimized by using (1) at least five stimuli per session, (2) a minimum of 110% resting motor threshold as stimulation intensity, (3) a minimum of 4 s inter-trial interval and increasing the interval up to 20 s, (4) a figure-of-eight coil and (5) a monophasic waveform. MEPs can be reliably operationalized.
1. Introduction
Since the introduction of transcranial magnetic stimulation (TMS) by Barker et al. (1985), the majority of studies use this non-invasive brain stimulation technique to stimulate the primary motor cortex (M1) in order to provoke a quantifiable response of the human motor system. Applied over M1, single TMS pulses elicit motor evoked potentials (MEPs) that can be recorded via electromyography (EMG) from the corresponding contralateral target muscle. MEPs are frequently used as physiological markers of corticospinal excitability (CSE) in scientific research and clinical practice (Rossini et al., 2015). The electrical stimulation of the M1 evokes a complex pattern of early direct pyramidal tract axon activation and later indirect activation of axonal connections (Di Lazzaro and Rothwell, 2014). Activation is triggered due to potential changes along the propagation of the axon, resulting primarily in an activation pattern of axons perpendicular to the induced current flow in the brain (Di Lazzaro and Rothwell, 2014).
Motor evoked potentials are most often derived from the subject’s hand muscles via surface electrodes attached to the target muscle of interest. Commonly used outcome measures of M1 stimulation are the contralateral derived peak-to-peak amplitude, the area under the curve (AUC) which is defined as the integral of the rectified signal and the input-output curve (IO-curve or stimulus-response curve). While the amplitude (MEPamp) is a direct measure of CSE, the IO-curve represents the amplitude as a function of the stimulation intensity (Devanne et al., 1997; Rossini et al., 2015). For the quantification of these outcome measurements, the amplitude signal of EMG responses is necessary. However, it was shown that the amplitude exhibits high intrinsic variability. This variability could be attributed to spontaneous intra-individual changes and fluctuations in CSE (Kiers et al., 1993; Rossini et al., 2015), and is present even at the same level of stimulation intensity (Kiers et al., 1993; Wassermann, 2002). Inter-individual anatomical differences (Pellegrini et al., 2018a) and technical stimulation parameters could contribute to this variability as well. For example, the use of different waveforms, coil-types and the orientation of the coil cause different current flows in the cortex. This in turn leads to varied patterns of focality, neuronal population activation and recruitment (Kiers et al., 1993; Di Lazzaro et al., 2004; Di Lazzaro and Rothwell, 2014; Rossini et al., 2015).
The number of annually published studies using TMS to elicit MEPs is growing steadily. Without sufficient clarification about the reliability of outcome measurements, valid interpretations of available findings are rather limited. Besides validity and objectivity, reliability is one of the main quality criteria requirements for high-quality and standardized research. Reliability is statistically described as the ratio of the variance of the true value to the overall variance. A reliable measurement instrument produces consistent values with low measurement error. As the observed value always consists of the true value and the inseparable measurement error, reliability rather describes an estimation of the error. Or, vice versa, the degree to which the measurement is free from error. Ultimately, the reliable instrument is thus able to distinguish true changes in the target variable from random or systematic errors (Atkinson and Nevill, 1998; Bialocerkowski and Bragge, 2008; Mokkink et al., 2010; Portney and Watkins, 2015). Portney and Watkins (2015) argue that with identifying the factors that affect the observed values, more variance can be predicted and the amount of unaccounted variance attributed to error decreased. To be successfully used in research and clinical practice, e.g., as a diagnostic instrument, the assessment of MEPs must be reliable. The categorization of whether a variable or instrument is reliable or not depends on the one hand on the inherent characteristics of the variable, and on the other hand on the appraisal of the reliability coefficient by the experimenter. The experimenter must decide which reliability coefficient value is suitable on the basis of the knowledge about the target variable (Portney and Watkins, 2015).
However, at this stage no review has systematically addressed the reliability of single pulse MEP-measurements in healthy subjects. Thus, this systematic review aims to identify studies reporting on the reliability of MEPs evoked via single TMS-pulses and derived from relaxed hand muscles of healthy individuals. The main objective of the present review is to not only give an overview about the available studies addressing the reliability of MEPs, but also to reach a conclusion about the reliability of MEP-measurements as well as to identify stimulation parameters that potentially produce most reliable MEP measurements. These will be combined into a best-practice recommendation for the reliable detection of single-pulse MEPs. For this purpose, the MEP amplitude, AUC and IO-curves as stimulation outcome measures together with the corresponding statistical reliability parameters are extracted per study for reliability evaluation. In order to be able to assess the quality of the individual studies with regard to the experimental procedure and to increase transparency, a critical assessment of the quality and methodology of the articles is carried out by two authors using a standardized evaluation scale, namely, Chipchase et al.’s (2012) Checklist.
2. Methods
2.1. Literature research
A systematic literature search was conducted in March 2023 according to the Preferred Reporting System for Reviews and Meta-Analysis (PRISMA) guidelines (Page et al., 2021). All articles published up to that time were considered for further assessment. The publication date of the earliest included study was 2001, and that of the most recent was 2022. The literature search was performed using the keywords and the Medical Subjects Headings thesaurus (MeSH-terms) of the National Library of Medicine indexing PubMed articles “transcranial magnetic stimulation” and “motor evoked potential” or “MEPs” or “cortical excitability” and “reliability” or “repeatability” or “reproducibility” in PubMed. In addition, the reference sections of the resulting single studies were screened for further applicable papers. Two independent authors (MO and CK) conducted the literature search separately as well as rated the found articles with respect to eligibility: In a first step, the titles and abstracts of the entries were screened whether they were addressing the corresponding topic. In a second step, the articles thus classified as suitable were examined in full-text form with respect to the inclusion and exclusion criteria as outlined in the following section.
2.2. Inclusion- and exclusion criteria
Studies were classified as eligible if they met the following inclusion criteria: (1) TMS application in healthy adult subjects; (2) derivation of MEPs from hand muscles; (3) written in English; (4) conducted repeated measures, respectively proper reliability analysis (test-retest, intra- or inter-rater reliability); (5) report of at least one statistical reliability parameter.
Not included were (1) other reviews, single case or single trial studies, study protocols or comments, studies that investigated (2) animal models or (3) participants under the age of 18 years or (4) lower limb or arm muscles and (5) papers not written in English. Figure 1 shows the literature search process according to the PRISMA guidelines (Page et al., 2021). A total number of 2,501 entries could be identified using the above-mentioned search string in PubMed. Three additional studies were found, screened and included based on the references of the PubMed articles. Of the 2,501 records, 585 were removed by automatic search filters, i.e., human subjects, ≥18 years. During the screening of titles and abstracts, a further 1,861 articles were sorted out individually by both raters. The remaining 52 entries were reviewed in detail for meeting or not meeting the inclusion and exclusion criteria. At the end of the process, a total number of 28 studies were identified as eligible and included in the present review.
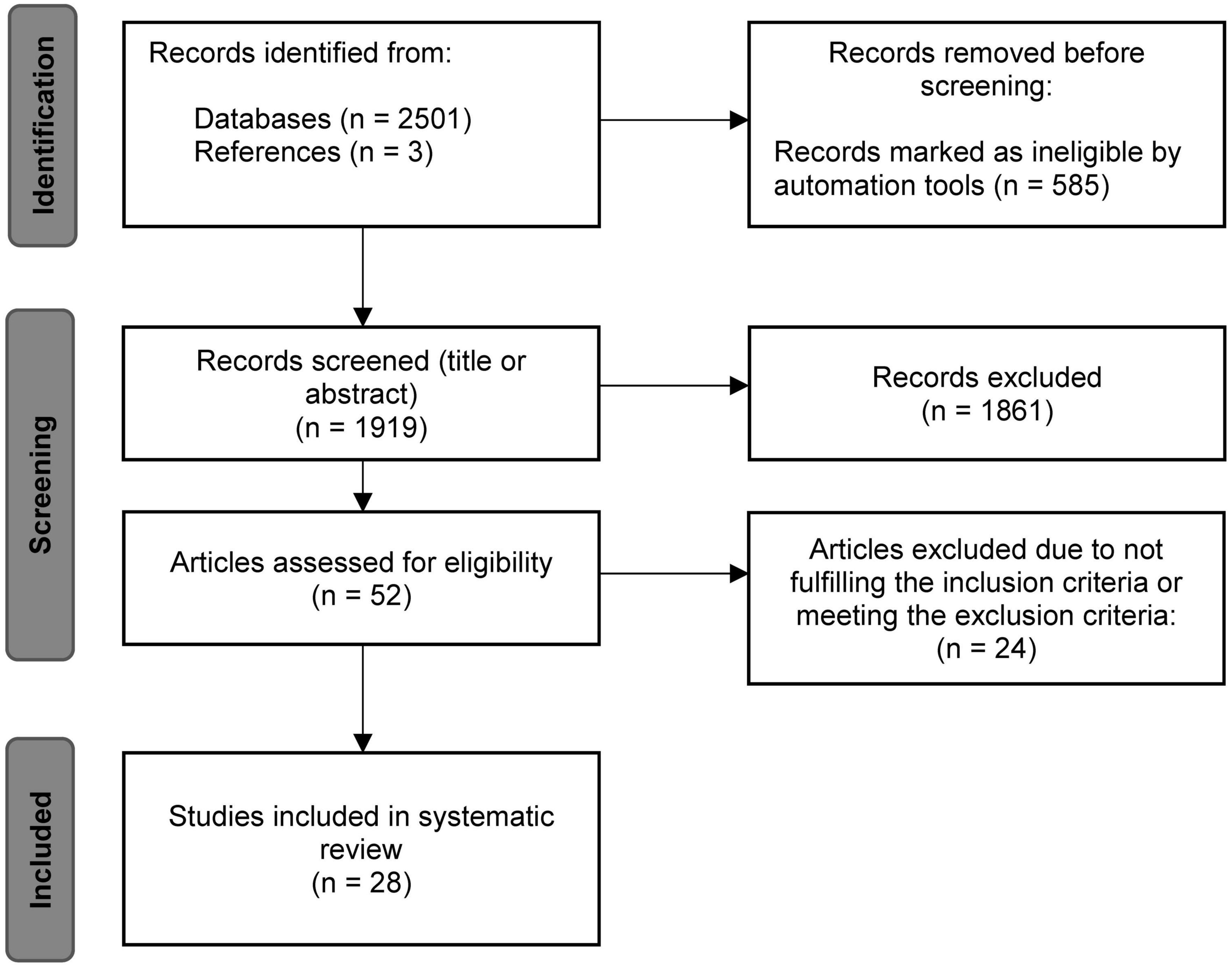
Figure 1. Flowchart of the systematic literature search based on the PRISMA statement (Page et al., 2021).
2.3. Study and reliability assessment
After article eligibility evaluation, the data on the (1) subject characteristics, (2) stimulators and coils used, (3) stimulation intensity, (4) target muscle, (5) waveform as well as the (6) number of sessions, (7) time interval between measurements, (8) applied stimuli, (9) TMS outcome parameters, (10) their reliability parameters, and (11) intervals between measurements were extracted from the final 28 articles and summarized by the first author. Next, the two raters (MO and CK) assessed the studies independently regarding the fulfilment of items in the checklist of Chipchase et al. (2012) which is described in detail in the next section. The inter-rater reliability of the checklist rating was determined via calculation of Cohen’s kappa (Cohen, 1960). Absolute and relative frequencies of the criteria fulfilment were determined study- and item-wise. A total score was calculated by adding the number of fulfilled criteria and dividing by the total number of applicable criteria per study. The method of checklist application and inter-rater reliability calculation was conducted following Beaulieu et al. (2017).
In order to further check for possible publication bias, a funnel plot was used (Light and Pillemer, 1984) and tested for asymmetry with linear regression after the method of Egger et al. (1997). As described, a rigid classification of reliability coefficients is not recommended and existing limits are arbitrary (Portney and Watkins, 2015). In order to give an orientation about the existing values, reliability coefficients below 0.50 are described as poor, between 0.50 and 0.75 as moderate and above 0.75 as good (Portney and Watkins, 2015), while the value 1.00 would indicate perfect reliability.
2.4. Chipchase et al.’s Checklist
Given the growing number of TMS studies of the human motor system and the variability in outcome measures, Chipchase et al. (2012) designed a checklist to assess the methodological quality of studies with the goal of increasing data quality in this research field. The checklist consists of 30 items which allow a critical evaluation of the reported methodology (Chipchase et al., 2012). As we were not interested in paired-pulse paradigms, the items concerning these and the unconditioned MEP size, were excluded from the rating and analysis. The items assessing the use of medication (i.e., use of CNS active drugs and prescribed medication) were combined. The checklist was completed under the following assumptions:
In the scope of the checklist, the gender is not a variable that would necessarily be important to control and extent of relaxation of muscle other than those being tested is not a reportable factor. In the present review, these items and other items were assessed as controllable, i.e., when the sample was gender-balanced and reportable, i.e., if the activation level of other muscles was monitored. The sole statement that a procedure (e.g., determination of resting motor threshold, RMT) was carried out was not sufficient to evaluate the item as reported - this only applied if the used method was mentioned. If variables were balanced (e.g., gender balance), controlled via e.g., a questionnaire or included as a factor in the statistical analysis, they were rated as controlled. An item that is considered as controlled will also be rated as reported. Since the term gender was used in the checklist, this term refers to the sex of the studied subjects and is retained to avoid further complexity. Results regarding the checklist and inter-rater reliability can be found in the Supplementary material.
2.5. Association of checklist criteria or reliability values with publication date
Based on the hypothesis that scientific and technological progress in the scientific field increases with time, Pearson correlation was calculated in SPSS (V29.0, IBM Corp., USA) to test whether there is an association between the number of fulfilled checklist criteria or reliability values with ongoing publication year.
3. Results
The included studies as well as subject characteristics, stimulators and coils used, stimulation intensity, target muscle and waveform are shown in Table 1. In summary, 588 subjects with an average age of 32 ± 6 years were examined in the studies, of which 247 (42%) were female participants. Regarding the different muscles of the hand, the majority of the studies examined the first dorsal interosseus muscle (FDI) (n = 23), followed by the abductor pollicis brevis (APB) (n = 5) and abductor digiti minimi (ADM) (n = 1). In total, 19 studies used a device from Magstim®, six from MagVenture® and two studies each used a device from Cadwell® or NexStim®. Most frequently, a figure-of-eight (Fof8) coil was used for stimulation (n = 22, of which one was angulated), five studies used a circular coil and in three cases the coil type was not defined. The range of stimulation intensities used in the studies ranged from 90 to 170% RMT, respectively 5–100% of the maximum stimulator output (MSO). Two studies used a stimulation intensity that elicits a MEPamp of approximately 1 mV (SI1mV). The majority of the experiments was conducted with a monophasic (n = 17) or a biphasic (n = 5) waveform, whilst two studies applied both waveforms. In the remaining four studies, the used pulse shape could not be clearly identified. For 13 studies the waveform is derived e.g., from the description of the current flow in the respective studies marked with asterisks in Table 1. Note that some studies used multiple stimulators, different coil types and waveforms or compared more than one muscle.
3.1. TMS and reliability measurement within the studies
Table 2 shows the TMS outcome parameters, the number of sessions, time interval between measurements and applied stimuli and the statistical reliability indices of the individual studies. These were grouped according to the variable for which reliability was determined: Number of applied stimuli, stimulation intensity (SI), target muscle or target hemisphere, reliability of IO-curve parameters, inter-trial interval (ITI), current direction, coil type and use of neuronavigation systems, used for precise positioning of the coil relative to the brain. The listing of studies in multiple categories is possible.
Nine of the identified studies investigated the effect of the number of applied stimuli on the reliability of the MEPamp (Christie et al., 2007; Bastani and Jaberzadeh, 2012; Goldsworthy et al., 2016; Hashemirad et al., 2017; Biabani et al., 2018) or the probability of inclusion of the running average of MEPamp - the average calculated on consecutive trials - in the 95% confidence interval of all trials (CIn-method) (Cuypers et al., 2014; Chang et al., 2016; Goldsworthy et al., 2016; Bashir et al., 2017; Biabani et al., 2018). One study used a principal component regression approach to determine the number of trials and the corresponding amount of variance that is accounted for by them (Nguyen et al., 2019).
Seven studies investigated the effects of stimulation intensity on reliability (Kamen, 2004; Christie et al., 2007; Ngomo et al., 2012; Cueva et al., 2016; Brown et al., 2017; Pellegrini et al., 2018b) as well as the inter-rater reliability (Cohen’s κ) and internal consistency (Cronbach’s α) of the ratings (Cueva et al., 2016) and the influence of intensity in the scope of the CI method (Cuypers et al., 2014).
The majority of the identified studies chose the FDI as the target muscle for MEP derivation, two studies performed a direct comparison with other hand or forearm muscles in an experiment (McDonnell et al., 2004; Malcolm et al., 2006).
Reliability of the different IO-curve parameters (slope, peak-slope and maximum/plateau) were also investigated by five studies (Carroll et al., 2001; Kukke et al., 2014; Liu and Au-Yeung, 2014; Dyke et al., 2018; Therrien-Blanchet et al., 2022) and compared between the contra- and ipsilateral sides within one study (Schambra et al., 2015).
The influence of the length of the ITI was investigated by two studies (Vaseghi et al., 2015; Hassanzahraee et al., 2019), the influence of current direction by one study (Davila-Pérez et al., 2018) and the influence of the used coil (Fof8 coil vs. circular coil) was directly compared within one study (Fleming et al., 2012). Two direct comparisons between the measurement with and without the use of navigation were made based on coefficient of variation (CV) values (Julkunen et al., 2009; Jung et al., 2010).
3.2. Identifying best practice
Comparing the results of the identified studies, recommendations regarding the reliable estimation, i.e., a high ratio of true variance to overall variance, of CSE parameters (MEPamp, IO-curve, AUC) with single-pulse TMS can be derived.
With a minimum of 19 and a maximum of 31 pulses an estimation with 100% chance of inclusion in the running average 95% CI of the intra- (Cuypers et al., 2014; Chang et al., 2016; Goldsworthy et al., 2016; Biabani et al., 2018) and inter-session (Bashir et al., 2017) amplitude is possible. One study used the CI-method to compare the inclusion probability between 110 and 120% RMT stimulation intensity and reported an attainment of 100% inclusion in the CI after 26 pulses at the lower stimulation intensity and after 30 pulses at the higher intensity (Cuypers et al., 2014).
The reliability values for the minimum number of trials required within- and between a session are heterogeneous. While three studies report “poor” values for five or less trials (Christie et al., 2007; Goldsworthy et al., 2016; Biabani et al., 2018) within a session, one study reported moderate reliability for four (Brown et al., 2017) and two studies good values for five trials (Christie et al., 2007; Bastani and Jaberzadeh, 2012).
For between-session comparisons, values were also “poor” [<0.40 (Goldsworthy et al., 2016);0.16 (Biabani et al., 2018)] for five or less trials in two studies, but also classified as good (0.88) within one study (Bastani and Jaberzadeh, 2012).
Applying six to 15 trials within a session resulted in “fair” (0.40–0.58 after Cicchetti and Sparrow, 1981) reliability in one study (Goldsworthy et al., 2016), while almost perfect values of ICC = 0.98 were reached after 10 trials within one study. This value did not increase further when increasing the number from 10 to 15 trials (Bastani and Jaberzadeh, 2012).
In between-session comparisons, one study reported “poor” (<0.40) values for six to 10 trials (Goldsworthy et al., 2016). Almost good [>0.851 (Hashemirad et al., 2017)] to perfect values [ = 0.93, (Bastani and Jaberzadeh, 2012)] were reported applying 10 to 15 trials by two studies, while one reported “fair” [0.40–0.58 (Goldsworthy et al., 2016)] values for 11 to 15 trials.
Increasing the number of applied stimuli within a session further was done within one study, resulting in “good” (0.59–0.75 after Cicchetti and Sparrow, 1981) reliability for 16 to 20 trials, while after the 21st pulse up to 35 pulses “excellent” (>0.75) values were reported (Goldsworthy et al., 2016).
Between-sessions, “fair” [0.40–0.58 (Goldsworthy et al., 2016)] values were reported for 16 to 25 trials by one study, with increasing values up to “good” (0.59–0.75) reliability after 26 to 35 trials (Goldsworthy et al., 2016); while in another study good reliability values for amplitudes were reached after applying 15 and up to 35 trials (Biabani et al., 2018). For SI1mV, between-session reliability values linearly increased from the moderate values within the first 10 and 15 applied stimuli to good values applying 20 stimuli (Hashemirad et al., 2017).
This heterogeneous pattern of results regarding the optimal number of applied stimuli within and between sessions does not allow an unambiguous statement. However, the values suggest that a minimum number of five stimuli within and between sessions should be applied per intensity for reliable measurement. Furthermore, at least a trend of increasing ICC values with the number of stimuli seems reasonable, as shown for SI1mV (Hashemirad et al., 2017).
The stimulation intensity shows a heterogeneous pattern as well. One study reports a decrease in reliability with increasing stimulation intensity (Kamen, 2004). However, in addition to a finding with high reliability at lowest and highest intensity and a decrease at medium intensity (u-shape) (Christie et al., 2007), four studies show higher reliability with increasing stimulation intensity within- and between-sessions (Ngomo et al., 2012; Cueva et al., 2016; Brown et al., 2017; Pellegrini et al., 2018b). On a descriptive basis, the use of a stimulation intensity ≥ 110% RMT could produce more reliable results.
Reliability of the parameters of the IO-curve (MEPmax, slope and s50 - the amplitude that evokes a MEP halfway between baseline and MEPmax) were estimated within seven studies (Carroll et al., 2001; Malcolm et al., 2006; Kukke et al., 2014; Liu and Au-Yeung, 2014; Schambra et al., 2015; Dyke et al., 2018; Therrien-Blanchet et al., 2022). ICC values for the slope were classified as good in five studies (Carroll et al., 2001; McDonnell et al., 2004; Malcolm et al., 2006; Liu and Au-Yeung, 2014; Therrien-Blanchet et al., 2022) and moderate in one (Kukke et al., 2014). Peak slope ICC values and maxima were classified as good in three (Carroll et al., 2001; Kukke et al., 2014; Schambra et al., 2015) respective four studies (Carroll et al., 2001; Kukke et al., 2014; Liu and Au-Yeung, 2014; Schambra et al., 2015) and moderate in one each (Carroll et al., 2001). Only in one study investigating older adults, the reliability of the slope was classified as poor (Schambra et al., 2015). In that study, a comparison of IO-curve parameters collected in both hemispheres from primarily right-handed elderly subjects showed good ICC values for s50 and plateau in both hemispheres, whereas the values for the slope were poor (ICC < 0.07). Therefore, reliable derivation of s50 and plateau is possible in both the right and left hemisphere, i.e., bilateral (Schambra et al., 2015).
Prolonging the time-interval from 5, 10, 15 to 20 s between single pulse applications further increases good intra- and inter-session reliability values in one experiment (Hassanzahraee et al., 2019). In contrast, another study directly comparing ITIs of 4 s and 10 s did not show an increasing reliability with increasing ITI. In this case, intra- and inter-session reliability was good for 4 s as well as 10 s intervals (Vaseghi et al., 2015). A reliable estimation of MEP amplitude with a minimum ITI of 4 s therefore is possible and an increase of up to 20 s could further increase reliability.
In addition, the use of a Fof8 coil was superior in terms of between-session reliability to the use of a circular coil, also under the benefit of navigation (Fleming et al., 2012). Regarding the comparison between the applied pulse shape, the use of a monophasic waveform was more beneficial in reliably estimating amplitude than a biphasic waveform (Davila-Pérez et al., 2018).
The contradictory nature of the results on the influence of navigation on the CV does not yet allow a statement to be made at this point in time (Julkunen et al., 2009; Jung et al., 2010).
3.3. Publication bias
A funnel plot (Figure 2) was created in R (R Core Team, Austria, V. 4.0.5; Schwarzer et al., 2015) to check for publication bias within studies reporting ICC-values (Light and Pillemer, 1984). The linear regression approach to test for asymmetry after Egger et al. (1997) revealed no significant asymmetric distribution (t = 2.12, df = 8, p = 0.067), indicating that included studies are not subject to publication bias. As standard error decreases with increasing sample size it would theoretically reach zero with infinite sample size. It can be seen from the distribution of the single study values at the bottom of the plot, that all studies deploy a small sample size as indicated by relatively higher standard errors, as typical for non-invasive brain stimulation studies.
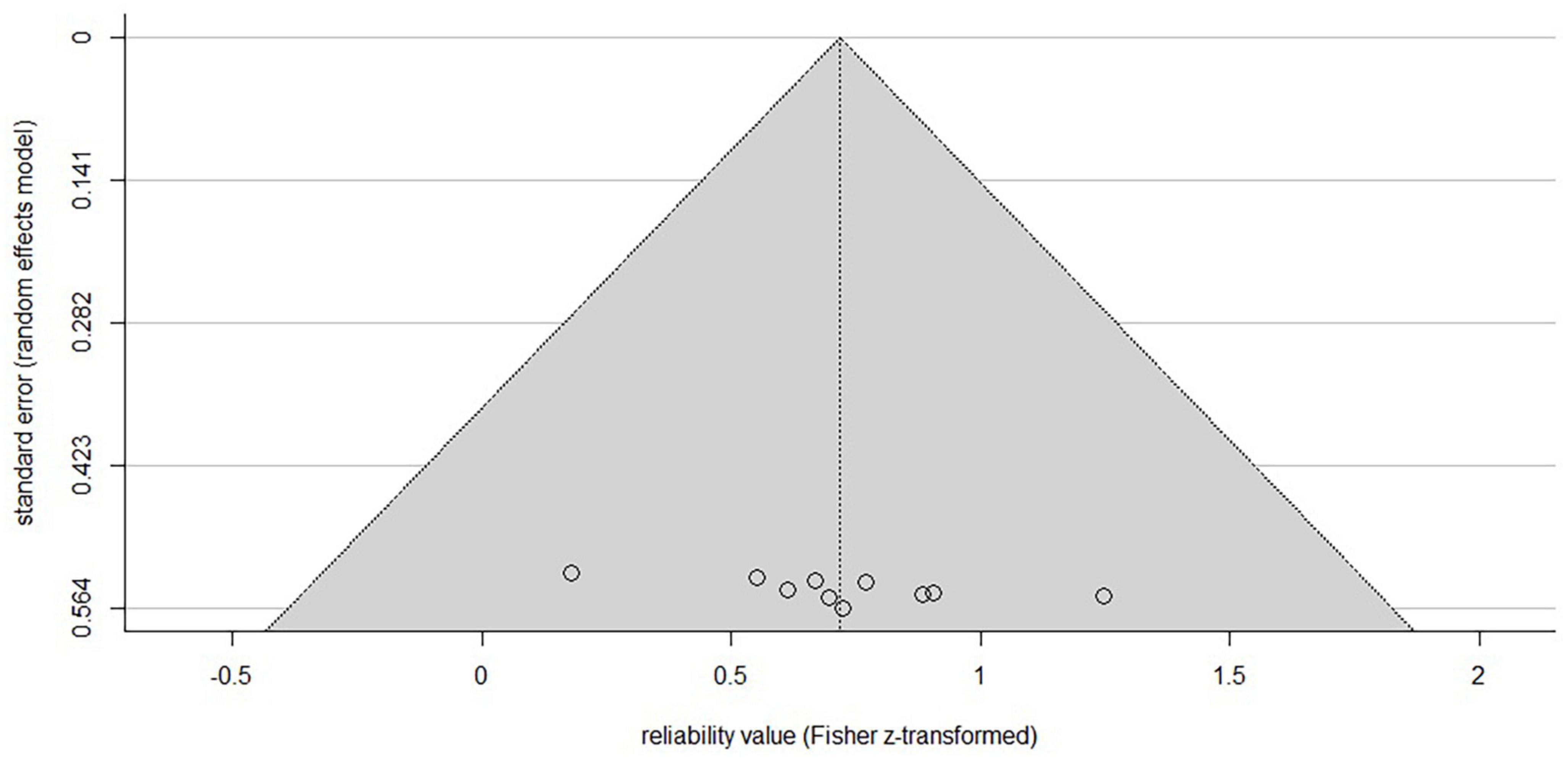
Figure 2. Funnel plot of the included studies reporting ICC-values. X-axis is showing the z-transformed mean ICC-values, the y-axis the standard error. The horizontal line indicates the population effect size, skewed lines the 95% CI. Although the typical inverted funnel shape is not evident, a significant symmetrical distribution centered at the bottom indicates the typical small sample sizes (higher standard error) in non-invasive brain studies with low risk of publication bias. Note that studies reporting other parameters than ICC are not included.
4. Discussion
The present review work identified studies on the reliability of MEPs evoked via single TMS-pulses and derived from relaxed hand muscles of healthy individuals. It aims to give an overview of the available studies addressing the reliability of MEPs and to identify technical TMS parameters that produce most reliable MEP measurements. For this purpose, a systematic literature search up to March 2023 was conducted, according to the PRISMA guidelines (Page et al., 2021). A total of 28 articles addressing the research topic were identified and most relevant parameters were descriptively summarized. The identified studies were assigned to seven different categories and the results are discussed in detail: number of applied stimuli (n = 9 studies); stimulation intensity (n = 7); target muscle or hemisphere (n = 3); IO-curve (n = 6); ITI (n = 2); waveform and current direction (n = 1); coil type and navigation (n = 3).
4.1. Number of applied stimuli
According to the CI method, the 100% probability of inclusion in the 95% of the respective studies was achieved for 19–31 stimuli (Cuypers et al., 2014; Chang et al., 2016; Goldsworthy et al., 2016; Bashir et al., 2017; Biabani et al., 2018). For reliable detection of amplitude within and between sessions, at least five stimuli should be applied, whereby higher ICC values are also reported with an increasing number of stimuli (Christie et al., 2007; Bastani and Jaberzadeh, 2012; Goldsworthy et al., 2016; Hashemirad et al., 2017; Biabani et al., 2018). In their calculations, Nguyen et al. (2019) described that 20 stimuli within a session held circa 90% of the total variance of the dataset. Based on reliability theory, the true MEP amplitude cannot be measured, as all measured values contain inseparable systematic or random measurement errors. Thus, to judge what degree of reliability is sufficient for the measured variable is strongly based on the nature of the variable itself and evaluation of the experimenter. Therefore, following reliability theory and considering amplitude variability, the best approximate estimate of true MEPamp can be achieved by averaging single trials (Portney and Watkins, 2015; Rossini et al., 2015). As systematic errors are constant and make up a smaller proportion of the total error than random error, they rather impact validity than reliability. Therefore, with averaging trials, the random errors arising from e.g., unknown technical interfering in the laboratory, could cancel each other out (Portney and Watkins, 2015). These assumptions lead to the question of how many individual stimuli should be applied during a session, which is always a trade-off between time and accuracy. Ammann et al. (2020) addressed the question of the optimal number of stimuli per session in their theoretical and experimental framework. Their results support the assumption that an exact optimal number of pulses is not generalizable for such a highly intrinsic-variable outcome parameter as the MEPamp. Rather, the assumption of reliability theory must also be considered here as to what extent of error variance is assumed to be reasonable for the experiment and variable. Furthermore, their analytical results suggest that the optimal number of stimuli needed for reliable MEP amplitude estimation is dependent on the total number of applied stimuli, and the more stimuli are applied in total the more are needed for a suitable estimation (Ammann et al., 2020). Thus, the analytical results of Ammann et al. (2020) are a limiting factor in the generalizability of the studies investigating the optimal number of stimuli. However, they provide support for the observation in the included studies of our review that at a certain number of stimuli, a plateau effect occurs (which seems to occur between 19 and 31 stimuli in the studies examined here) at which reliability does not appear to increase further.
4.2. Stimulation intensity
While in one case lower reliability values are described with increasing stimulation intensity and in another case a u-shaped course is reported, four studies show a linear increase (Kamen, 2004; Christie et al., 2007; Ngomo et al., 2012; Cueva et al., 2016; Brown et al., 2017; Pellegrini et al., 2018b). The majority of results regarding higher stimulation intensities and increasing reliability values are to be expected due to the underlying corticospinal processes: with an increasing stimulation intensity, the MEPamp increases due to a faster and uniform recruitment of the underlying neural connections and corticospinal fibers (Rossini et al., 2015), which could reflect in the positive linear relationship of increased reliability and lowered variability at higher stimulator output. As the stimulation intensity and MEP amplitude increase, a plateau is reached from which the CSE does not increase further, partially based on the rising phase cancellation of the underlying motor unit action potentials (Rossini et al., 2015). This is also partly observable for the reliability values at higher intensities. For example, between two sessions, the reliability continues to rise with an increase from 150 to 165% RMT and remains in the upper category within a session from 135% RMT on to 165% (Pellegrini et al., 2018b). However, this does not explain the results of decreasing reliability values with increasing stimulation intensity, which were reported in two studies (Kamen, 2004; Christie et al., 2007). One possible explanation is the heterogeneity of the technical experimental parameters used (e.g., other stimulator and coil type), which are described further below.
4.3. Maximum stimulator output
One problem of comparability between different TMS studies is the parameter Maximum Stimulator Output to which the used stimulation intensity is mostly relativized to. This indicates the stimulator-specific generated output and is not transferable to other stimulators due to different manufacturers and models, which makes results comparability more difficult. In order to still be able to achieve a replicability of the stimulation dose, Peterchev et al. (2012) recommend reporting all parameters that have an influence on the induced electromagnetic field (i.e., stimulation device, settings, coil type and waveform parameters e.g., pulse width, ITI).
4.4. Inter-trial and inter-session interval
With increasing the ITI up to 20 s, variability could be reduced; amplitude - and in one case reliability - could be increased (Kamen, 2004; Hassanzahraee et al., 2019). The underlying mechanisms are not yet fully understood, but the authors attribute them to suprathreshold post-stimulus change of hemodynamic processes that take a certain amount of time to return to baseline levels. For example, after suprathreshold stimulation of the prefrontal cortex, the level of oxy-hemoglobin decreases, reaching a minimum at circa 8 s post-stimulus (Kamen, 2004; Thomson et al., 2011, 2012; Hassanzahraee et al., 2019). As the studies did not compare ITI shorter than 4 s, assumption about shorter time-intervals can not be made. Results regarding the underlying hemodynamic processes would suggest that a further reduction of ITI might not be beneficial.
The inter-session interval within the studies was grouped in short-term (≤24 h) and long term (≥24 h) intervals. Descriptively, no trend of higher reliability with short- or long intervals can be derived.
4.5. Current direction
One study showed that applying pulses with a monophasic waveform resulted in higher reliability than compared to a biphasic waveform, regardless of the direction of induced current flow in the cortex (Davila-Pérez et al., 2018). Both the waveform of a pulse applied with a controllable pulse TMS (cTMS) and the induced current flow in the motor cortex affect the motor threshold, MEP latency and steepness of the IO-curve in the FDI at rest (Sommer et al., 2018). In the cTMS study is described that a symmetrical biphasic pulse can be viewed as two monophasic pulses with opposite directions, which result in the activation of distinct directional specific neuronal populations (Sommer et al., 2018). At this point, our literature search identified one study (Davila-Pérez et al., 2018) dedicated to the reliability of CSE parameters with different pulse shapes and current directions. The authors suggest that the successive components of the biphasic pulse lead to a cancellation of activation due to simultaneous activation of inhibitory and excitatory neuronal circuits (Davila-Pérez et al., 2018). Thus, the inconsistent activation pattern at the investigated stimulation intensity could have led to the low reliability for the biphasic in comparison to the monophasic waveform.
At this point it is important to highlight the general differences between the stimulator manufacturers, complicating the comparison of the output of the devices already described. As Schoisswohl et al. (2023) highlighted in their comparison of current directions in the repetitive TMS treatment of tinnitus disorder, the default current direction of TMS-devices varies between fabricators. The manufacturer differences also relate to the winding of the coils and nomenclature of the current direction in the coil. Also surprising in this context is that the majority of the included studies used a monophasic waveform for stimulation, as primarily biphasic pulses are used for repetitive TMS-treatment (Rossini et al., 2015).
4.6. Coil-type
Using a Fof8 coil was superior in terms of between-session reliability to the use of a circular coil, regardless of whether neuronavigation was used. The Fof8 coil with its two interfering electric and magnetic fields induces higher currents directly under the coil than in the periphery, whilst the circular coil induces a steady circular current flow under the coil (Di Lazzaro and Rothwell, 2014). Stimulation with circular coils tends to be less focal than stimulation with Fof8 coils and results in higher descending output when applied above motor threshold intensity. This higher output of non-focal stimulation in the form of spinal volleys could be due to the more widespread activation that can also occur on the non-targeted hemisphere. Further, it is possible that the direction of the induced current under the round coil in the brain tissue is more inhomogeneous than the current flow generated by a Fof8 coil (Di Lazzaro et al., 2004). It is therefore obvious that despite the use of the same stimulation parameters, different corticospinal excitation patterns are produced when using the two types of coils (Di Lazzaro et al., 2004) and comparability of studies using distinct coil models is further limited. Future studies should explicitly investigate the reliability of different coil types and also include new coil designs.
4.7. Use of navigation
Surprisingly, the results of the direct comparisons of the influence of navigation on the CV are contrary. The CV as a measure of outcome parameter stability decreased significantly when navigation was used for MEPamp measurement in one study (Julkunen et al., 2009), but remained unchanged in another study measuring IO-curves (Jung et al., 2010). These results are counterintuitive, as coil positioning stability is increased when neuronavigation is used (Cincotta et al., 2010). Jung et al. (2010) state that they controlled other sources of MEP variability e.g., coil orientation, coil type, electrode placement and level of target muscle relaxation. Therefore, the authors propose the result of comparable CV values measured with and without the use of navigation in their findings to be caused by the spontaneous fluctuations of CSE, as described earlier. In contrast, Julkunen et al. (2009) interpret the observed higher and more stable MEP amplitudes as a result of higher stimulation precision, leading to a more efficient recruitment of neurons. As a result, intra-individual variation decreased (Julkunen et al., 2009).
4.8. Target muscle or hemisphere
Studies in which the result parameters were directly compared explicitly in different hand muscles are still rare, but one study showed comparable good reliability values in the two hand muscles (Malcolm et al., 2006), while the slope of the IO-curve in FDI scored slightly higher ICC-values than the APB (ICC 0.82 > 0.78). Comparison with upper extremity muscles within the study showed comparable or lower ICC values for muscles of the forearm. However, in a direct comparison of the FDI with forearm muscle Flexor carpi ulnaris, ICC values of amplitude were classified as poor to moderate but higher than in the forearm (McDonnell et al., 2004). Future studies should aim for a reliability comparison of MEP measures across single target muscles.
4.9. IO-curve
The IO-curve represents the amplitude as a function of the stimulation intensity, which follows a sigmoidal shape that can be described by the slope, the intensity that evokes a response half the size of the maximum amplitude and the maximal amplitude respective plateau (Rossini et al., 2015). Except for one study describing poor reliability for the slope parameter, the other five studies show moderate to good slope reliability. Overall, the IO-curve can be used to reliably measure CSE in healthy humans.
4.10. Reliability coefficients and statistical parameters
The most common outcome parameter for determining reliability within the studies examined was the ICC, which ranged from −0.16 for biphasic pulses between sessions (Davila-Pérez et al., 2018) to 0.98 for 15 trials applied with monophasic pulses within a session (Bastani and Jaberzadeh, 2012). As the coefficient is calculated on the basis of intra-subject and sample variances, which certainly differ between samples, the comparison between studies is generally difficult and results can only be extrapolated to resembling samples. The second most frequently reported measure of outcome parameter stability was CV, which is a relative, unit-free measure that allows for comparability between studies (Portney and Watkins, 2015). In terms of ICC, reporting the exact model that was used for calculation (Koo and Li, 2016) and all relevant experimental parameters is an approach to increase transparency and comparability in the research field. Like the MEPamp itself is highly variable, so are the studies examining it. The problem of inconsistent results does not only concern studies on single pulses, but has also been described for widely used repetitive neuromodulatory TMS-protocols and repetitive heuristics (Prei et al., 2023). One approach to increase transparency and decrease inconsistency of results can be the use of standardized checklists.
4.11. Chipchase et al.’s Checklist and inter-rater agreement
To increase transparency, the studies were evaluated by two raters with regard to their methodological criteria using the standardized checklist by Chipchase et al. (2012). Inter-rater reliability of the checklist rating was calculated. Furthermore, the hypothesis that the number of fulfilled checklist criteria also increases with progressing publication year, due to advances in technology and research, was tested via Pearson correlation. Contrary to expectations, no association was found between publication date and checklist score. On average, the relative sum of reported and controlled items per study reached 46.8% and 17 of the 28 rated studies reached a total score of ≥ 50%. Mean inter-rater reliability, expressed by Cohen’s Kappa, was 0.87. This value describes an almost perfect agreement (Landis and Koch, 1977) between the two authors who rated the studies independently. Detailed results can be found in the Supplementary material. Future studies should use the checklist for orientation and report the listed parameters in order to increase the interpretability of individual studies and transparency within the research field. The development of a standardized scale for categorizing the results could increase comparability.
4.12. Identifying best practice
For reliable measurement it can be beneficial to use: (1) at least five stimuli per session, (2) a minimum of 110% RMT as stimulation intensity, (3) a minimum of 4 s ITI and increasing the ITI up to 20 s, (4) a figure-of-eight coil, and (5) a monophasic waveform.
4.13. Limitations
This systematic review is limited by several factors e.g., the specific focus on those studies that have investigated the reliability of single parameters, thus, generalizable statements about possible interactions are not applicable. Furthermore, despite a careful literature search and selection of criteria, it cannot be disproven that relevant articles were not included. Since a meta-analytic summary was not appropriate due to the number and structure of the available data, the results are on a descriptive basis and as outlined in the discussion, interpretation should be done with caution. Further studies should target the topic of reliability in comprehensive designs. e.g., targeting the interaction of stimulation intensity, number of pulses and pulse shape. Lastly, this study only investigated studies of hand muscles of healthy individuals, and it is therefore unclear whether these results can be extrapolated to other muscle groups or clinical populations. Computational models and simulations are necessary to include multiple parameters and evaluate their interactional impact in the future.
5. Conclusion
This systematic review aimed to give an overview about studies reporting on the reliability of MEPs evoked via single TMS-pulses in relaxed hand muscles of healthy adults. It gives a summary statement of the reliability scores as well as identified technical parameters and their influence on these reliability values. Parameters that could contribute to more reliable outcome measures can be descriptively identified. For reliable measurement it can be beneficial to use: (1) at least five stimuli per session, (2) a minimum of 110% RMT as stimulation intensity, (3) a minimum of 4 s ITI and increasing the ITI up to 20 s, (4) a figure-of-eight coil, and (5) a monophasic waveform. MEPs can be reliably derived and expressed with MEPamp, AUC and IO-curve from the hand muscles of healthy subjects. Future studies are needed to investigate reliability in clinical populations and in experimental designs examining factor interactions.
Data availability statement
The original contributions presented in this study are included in the article/Supplementary material, further inquiries can be directed to the corresponding author.
Author contributions
MO did the conceptualization of methodology, literature search, rating, visualization, analysis, and wrote the original draft. CK did literature search, rating, visualization, analysis, reviewed, and edited the manuscript. FS, KL, WS, WM, and MS reviewed and edited the manuscript. SS did the supervision, conceptualization of methodology and writing, and reviewed and edited the manuscript. All authors read and approved the final manuscript.
Funding
This research manuscript was funded by dtec.bw–Digitalization and Technology Research Centre of the Bundeswehr [project MEXT]. dtec.bw is funded by the European Union–NextGenerationEU.
Conflict of interest
The authors declare that the research was conducted in the absence of any commercial or financial relationships that could be construed as a potential conflict of interest.
Publisher’s note
All claims expressed in this article are solely those of the authors and do not necessarily represent those of their affiliated organizations, or those of the publisher, the editors and the reviewers. Any product that may be evaluated in this article, or claim that may be made by its manufacturer, is not guaranteed or endorsed by the publisher.
Supplementary material
The Supplementary Material for this article can be found online at: https://www.frontiersin.org/articles/10.3389/fnhum.2023.1237712/full#supplementary-material
Abbreviations
ADM, abductor digiti minimi; APB, abductor pollicis brevis; AUC, area under the curve; CI, confidence interval; CNS, central nervous system; CSE, corticospinal excitability; cTMS, controllable pulse TMS; CV, coefficient of variation; FCU, flexor carpi ulnaris; FDI, first dorsal interosseus; Fof8, figure-of-eight, EMG, electromyography; IC, internal consistency, Cronbach’s alpha; ICC, intraclass correlation coefficient; IO-curve, input-output curve; ITI, inter-trial-interval/inter-stimulus-interval; κ, Cohen’s Kappa; M1, primary motor cortex; MEP, motor evoked potential; MEPamp, motor evoked potential amplitude; MEPmax, plateau of the IO-curve; MEPrunning average amp, running average of the MEPamp; MeSH-terms, medical subjects headings thesaurus the national library of medicine; MSO, maximum stimulator output; n.a., not applicable; PRISMA, preferred reporting system for reviews and meta-analysis; RMT, resting motor threshold; s50, SI that evokes a MEP size halfway between the baseline and plateau; SI, stimulus intensity; SI1mV, stimulus intensity that evokes a MEP of approximately 1 mV; TMS, transcranial magnetic stimulation.
References
Ammann, C., Guida, P., Caballero-Insaurriaga, J., Pineda-Pardo, J. A., Oliviero, A., and Foffani, G. (2020). A framework to assess the impact of number of trials on the amplitude of motor evoked potentials. Sci. Rep. 10:21422. doi: 10.1038/s41598-020-77383-6
Atkinson, G., and Nevill, A. M. (1998). Statistical methods for assessing measurement error (reliability) in variables relevant to sports medicine. Sports Med. 26, 217–238. doi: 10.2165/00007256-199826040-00002
Barker, A. T., Jalinous, R., and Freeston, I. L. (1985). Non-invasive magnetic stimulation of human motor cortex. Lancet 1, 1106–1107.
Bashir, S., Yoo, W.-K., Kim, H. S., Lim, H. S., Rotenberg, A., and Abu Jamea, A. (2017). The number of pulses needed to measure corticospinal excitability by navigated transcranial magnetic stimulation: Eyes open vs. close condition. Front. Hum. Neurosci. 11:121. doi: 10.3389/fnhum.2017.00121
Bastani, A., and Jaberzadeh, S. (2012). A higher number of TMS-elicited MEP from a combined hotspot improves intra- and inter-session reliability of the upper limb muscles in healthy individuals. PLoS One 7:e47582. doi: 10.1371/journal.pone.0047582
Beaulieu, L.-D., Flamand, V. H., Massé-Alarie, H., and Schneider, C. (2017). Reliability and minimal detectable change of transcranial magnetic stimulation outcomes in healthy adults: A systematic review. Brain Stimul. 10, 196–213. doi: 10.1016/j.brs.2016.12.008
Biabani, M., Farrell, M., Zoghi, M., Egan, G., and Jaberzadeh, S. (2018). The minimal number of TMS trials required for the reliable assessment of corticospinal excitability, short interval intracortical inhibition, and intracortical facilitation. Neurosci. Lett. 674, 94–100. doi: 10.1016/j.neulet.2018.03.026
Bialocerkowski, A. E., and Bragge, P. (2008). Measurement error and reliability testing: Application to rehabilitation. Int. J. Ther. Rehabil. 15, 422–427. doi: 10.12968/ijtr.2008.15.10.31210
Brown, K. E., Lohse, K. R., Mayer, I. M. S., Strigaro, G., Desikan, M., Casula, E. P., et al. (2017). The reliability of commonly used electrophysiology measures. Brain Stimul. 10, 1102–1111. doi: 10.1016/j.brs.2017.07.011
Carroll, T. J., Riek, S., and Carson, R. G. (2001). Reliability of the input–output properties of the cortico-spinal pathway obtained from transcranial magnetic and electrical stimulation. J. Neurosci. Methods 112, 193–202. doi: 10.1016/S0165-0270(01)00468-X
Chang, W. H., Fried, P. J., Saxena, S., Jannati, A., Gomes-Osman, J., Kim, Y.-H., et al. (2016). Optimal number of pulses as outcome measures of neuronavigated transcranial magnetic stimulation. Clin. Neurophysiol. 127, 2892–2897. doi: 10.1016/j.clinph.2016.04.001
Chipchase, L., Schabrun, S., Cohen, L., Hodges, P., Ridding, M., Rothwell, J., et al. (2012). A checklist for assessing the methodological quality of studies using transcranial magnetic stimulation to study the motor system: An international consensus study. Clin. Neurophysiol. 123, 1698–1704. doi: 10.1016/j.clinph.2012.05.003
Christie, A., Fling, B., Crews, R. T., Mulwitz, L. A., and Kamen, G. (2007). Reliability of motor-evoked potentials in the ADM muscle of older adults. J. Neurosci. Methods 164, 320–324. doi: 10.1016/j.jneumeth.2007.05.011
Cicchetti, D. V., and Sparrow, S. A. (1981). Developing criteria for establishing interrater reliability of specific items: Applications to assessment of adaptive behavior. Am. J. Ment. Defic. 86, 127–137.
Cincotta, M., Giovannelli, F., Borgheresi, A., Balestrieri, F., Toscani, L., Zaccara, G., et al. (2010). Optically tracked neuronavigation increases the stability of hand-held focal coil positioning: Evidence from “transcranial” magnetic stimulation-induced electrical field measurements. Brain Stimul. 3, 119–123. doi: 10.1016/j.brs.2010.01.001
Cueva, A. S., Galhardoni, R., Cury, R. G., Parravano, D. C., Correa, G., Araujo, H., et al. (2016). Normative data of cortical excitability measurements obtained by transcranial magnetic stimulation in healthy subjects. Neurophysiol. Clin. 46, 43–51. doi: 10.1016/j.neucli.2015.12.003
Cuypers, K., Thijs, H., and Meesen, R. L. J. (2014). Optimization of the transcranial magnetic stimulation protocol by defining a reliable estimate for corticospinal excitability. PLoS One 9:e86380. doi: 10.1371/journal.pone.0086380
Davila-Pérez, P., Jannati, A., Fried, P. J., Cudeiro Mazaira, J., and Pascual-Leone, A. (2018). The effects of waveform and current direction on the efficacy and test-retest reliability of transcranial magnetic stimulation. Neuroscience 393, 97–109. doi: 10.1016/j.neuroscience.2018.09.044
Devanne, H., Lavoie, B. A., and Capaday, C. (1997). Input-output properties and gain changes in the human corticospinal pathway. Exp. Brain. Res. 114, 329–338. doi: 10.1007/pl00005641
Di Lazzaro, V., and Rothwell, J. C. (2014). Corticospinal activity evoked and modulated by non-invasive stimulation of the intact human motor cortex. J. Physiol. 592, 4115–4128. doi: 10.1113/jphysiol.2014.274316
Di Lazzaro, V., Oliviero, A., Pilato, F., Saturno, E., Dileone, M., Mazzone, P., et al. (2004). The physiological basis of transcranial motor cortex stimulation in conscious humans. Clin. Neurophysiol. 115, 255–266. doi: 10.1016/j.clinph.2003.10.009
Dyke, K., Kim, S., Jackson, G. M., and Jackson, S. R. (2018). Reliability of single and paired pulse transcranial magnetic stimulation parameters across eight testing sessions. Brain Stimul. 11, 1393–1394. doi: 10.1016/j.brs.2018.08.008
Egger, M., Davey Smith, G., Schneider, M., and Minder, C. (1997). Bias in meta-analysis detected by a simple, graphical test. BMJ 315, 629–634. doi: 10.1136/bmj.315.7109.629
Fleming, M. K., Sorinola, I. O., Di Newham, J., Roberts-Lewis, S. F., and Bergmann, J. H. M. (2012). The effect of coil type and navigation on the reliability of transcranial magnetic stimulation. IEEE Trans. Neural Syst. Rehabil. Eng. 20, 617–625. doi: 10.1109/TNSRE.2012.2202692
Goldsworthy, M. R., Hordacre, B., and Ridding, M. C. (2016). Minimum number of trials required for within- and between-session reliability of TMS measures of corticospinal excitability. Neuroscience 320, 205–209. doi: 10.1016/j.neuroscience.2016.02.012
Hashemirad, F., Zoghi, M., Fitzgerald, P. B., and Jaberzadeh, S. (2017). Reliability of motor evoked potentials induced by transcranial magnetic stimulation: The effects of initial motor evoked potentials removal. Basic Clin. Neurosci. 8, 43–50. doi: 10.15412/J.BCN.03080106
Hassanzahraee, M., Zoghi, M., and Jaberzadeh, S. (2019). Longer transcranial magnetic stimulation intertrial interval increases size, reduces variability, and improves the reliability of motor evoked potentials. Brain Connect. 9, 770–776. doi: 10.1089/brain.2019.0714
Julkunen, P., Säisänen, L., Danner, N., Niskanen, E., Hukkanen, T., Mervaala, E., et al. (2009). Comparison of navigated and non-navigated transcranial magnetic stimulation for motor cortex mapping, motor threshold and motor evoked potentials. Neuroimage 44, 790–795. doi: 10.1016/j.neuroimage.2008.09.040
Jung, N. H., Delvendahl, I., Kuhnke, N. G., Hauschke, D., Stolle, S., and Mall, V. (2010). Navigated transcranial magnetic stimulation does not decrease the variability of motor-evoked potentials. Brain Stimul. 3, 87–94. doi: 10.1016/j.brs.2009.10.003
Kamen, G. (2004). Reliability of motor-evoked potentials during resting and active contraction conditions. Med. Sci. Sports Exerc. 36, 1574–1579. doi: 10.1249/01.mss.0000139804.02576.6a
Kiers, L., Cros, D., Chiappa, K. H., and Fang, J. (1993). Variability of motor potentials evoked by transcranial magnetic stimulation. Electroencephalogr. Clin. Neurophysiol. 89, 415–423. doi: 10.1016/0168-5597(93)90115-6
Koo, T. K., and Li, M. Y. (2016). A guideline of selecting and reporting intraclass correlation coefficients for reliability research. J. Chiropr. Med. 15, 155–163. doi: 10.1016/j.jcm.2016.02.012
Kukke, S. N., Paine, R. W., Chao, C.-C., de Campos, A. C., and Hallett, M. (2014). Efficient and reliable characterization of the corticospinal system using transcranial magnetic stimulation. J. Clin. Neurophysiol. 31, 246–252. doi: 10.1097/WNP.0000000000000057
Landis, J. R., and Koch, G. G. (1977). The measurement of observer agreement for categorical data. Biometrics 33, 159–174.
Light, R. J., and Pillemer, D. B. (1984). Summing up: The science of reviewing research. Cambridge, MA: Harvard University Press.
Liu, H., and Au-Yeung, S. S. Y. (2014). Reliability of transcranial magnetic stimulation induced corticomotor excitability measurements for a hand muscle in healthy and chronic stroke subjects. J. Neurol. Sci. 341, 105–109. doi: 10.1016/j.jns.2014.04.012
Malcolm, M. P., Triggs, W. J., Light, K. E., Shechtman, O., Khandekar, G., and Gonzalez Rothi, L. J. (2006). Reliability of motor cortex transcranial magnetic stimulation in four muscle representations. Clin. Neurophysiol. 117, 1037–1046. doi: 10.1016/j.clinph.2006.02.005
McDonnell, M. N., Ridding, M. C., and Miles, T. S. (2004). Do alternate methods of analysing motor evoked potentials give comparable results? J. Neurosci. Methods 136, 63–67. doi: 10.1016/j.jneumeth.2003.12.020
Mokkink, L. B., Terwee, C. B., Patrick, D. L., Alonso, J., Stratford, P. W., Knol, D. L., et al. (2010). The COSMIN study reached international consensus on taxonomy, terminology, and definitions of measurement properties for health-related patient-reported outcomes. J. Clin. Epidemiol. 63, 737–745. doi: 10.1016/j.jclinepi.2010.02.006
Ngomo, S., Leonard, G., Moffet, H., and Mercier, C. (2012). Comparison of transcranial magnetic stimulation measures obtained at rest and under active conditions and their reliability. J. Neurosci. Methods 205, 65–71. doi: 10.1016/j.jneumeth.2011.12.012
Nguyen, D. T. A., Rissanen, S. M., Julkunen, P., Kallioniemi, E., and Karjalainen, P. A. (2019). Principal component regression on motor evoked potential in single-pulse transcranial magnetic stimulation. IEEE Trans. Neural. Syst. Rehabil. Eng. 27, 1521–1528. doi: 10.1109/TNSRE.2019.2923724
Page, M. J., McKenzie, J. E., Bossuyt, P. M., Boutron, I., Hoffmann, T. C., Mulrow, C. D., et al. (2021). The PRISMA 2020 statement: An updated guideline for reporting systematic reviews. BMJ 372:n71. doi: 10.1136/bmj.n71
Pellegrini, M., Zoghi, M., and Jaberzadeh, S. (2018a). Biological and anatomical factors influencing interindividual variability to noninvasive brain stimulation of the primary motor cortex: A systematic review and meta-analysis. Rev. Neurosci. 29, 199–222. doi: 10.1515/revneuro-2017-0048
Pellegrini, M., Zoghi, M., and Jaberzadeh, S. (2018b). The effect of transcranial magnetic stimulation test intensity on the amplitude, variability and reliability of motor evoked potentials. Brain Res. 1700, 190–198. doi: 10.1016/j.brainres.2018.09.002
Peterchev, A. V., Wagner, T. A., Miranda, P. C., Nitsche, M. A., Paulus, W., Lisanby, S. H., et al. (2012). Fundamentals of transcranial electric and magnetic stimulation dose: Definition, selection, and reporting practices. Brain Stimul. 5, 435–453. doi: 10.1016/j.brs.2011.10.001
Portney, L. G., and Watkins, M. P. (2015). Foundations of clinical research: Applications to practice. Philadelphia, PA: F.A. Davis.
Prei, K., Kanig, C., Osnabruegge, M., Langguth, B., Mack, W., Abdelnaim, M., et al. (2023). Limited evidence for validity and reliability of non-navigated low and high frequency rTMS over the motor cortex. medRxiv [Preprint] doi: 10.1101/2023.01.24.23284951
Rossini, P. M., Burke, D., Chen, R., Cohen, L. G., Daskalakis, Z., Di Iorio, R., et al. (2015). Non-invasive electrical and magnetic stimulation of the brain, spinal cord, roots and peripheral nerves: Basic principles and procedures for routine clinical and research application. An updated report from an I.F.C.N. Committee. Clin. Neurophysiol. 126, 1071–1107. doi: 10.1016/j.clinph.2015.02.001
Schambra, H. M., Ogden, R. T., Martínez-Hernández, I. E., Lin, X., Chang, Y. B., Rahman, A., et al. (2015). The reliability of repeated TMS measures in older adults and in patients with subacute and chronic stroke. Front. Cell. Neurosci. 9:335. doi: 10.3389/fncel.2015.00335
Schoisswohl, S., Langguth, B., Weber, F. C., Abdelnaim, M. A., Hebel, T., Mack, W., et al. (2023). One way or another: Treatment effects of 1 Hz rTMS using different current directions in a small sample of tinnitus patients. Neurosci. Lett. 797:137026. doi: 10.1016/j.neulet.2022.137026
Sommer, M., Ciocca, M., Chieffo, R., Hammond, P., Neef, A., Paulus, W., et al. (2018). TMS of primary motor cortex with a biphasic pulse activates two independent sets of excitable neurones. Brain Stimul. 11, 558–565. doi: 10.1016/j.brs.2018.01.001
Therrien-Blanchet, J.-M., Ferland, M. C., Rousseau, M.-A., Badri, M., Boucher, E., Merabtine, A., et al. (2022). Stability and test-retest reliability of neuronavigated TMS measures of corticospinal and intracortical excitability. Brain Res. 1794:148057. doi: 10.1016/j.brainres.2022.148057
Thomson, R. H., Maller, J. J., Daskalakis, Z. J., and Fitzgerald, P. B. (2011). Blood oxygenation changes resulting from suprathreshold transcranial magnetic stimulation. Brain Stimul. 4, 165–168. doi: 10.1016/j.brs.2010.10.003
Thomson, R. H., Maller, J. J., Daskalakis, Z. J., and Fitzgerald, P. B. (2012). Blood oxygenation changes resulting from trains of low frequency transcranial magnetic stimulation. Cortex 48, 487–491. doi: 10.1016/j.cortex.2011.04.028
Vaseghi, B., Zoghi, M., and Jaberzadeh, S. (2015). Inter-pulse Interval affects the size of single-pulse TMS-induced motor evoked potentials: A reliability study. Basic Clin. Neurosci. 6, 44–51.
Keywords: transcranial magnetic stimulation, motor evoked potentials, reliability, primary motor cortex, hand muscles, healthy humans, systematic review
Citation: Osnabruegge M, Kanig C, Schwitzgebel F, Litschel K, Seiberl W, Mack W, Schecklmann M and Schoisswohl S (2023) On the reliability of motor evoked potentials in hand muscles of healthy adults: a systematic review. Front. Hum. Neurosci. 17:1237712. doi: 10.3389/fnhum.2023.1237712
Received: 09 June 2023; Accepted: 08 August 2023;
Published: 31 August 2023.
Edited by:
Chris Baeken, Ghent University, BelgiumReviewed by:
Hela Zouari, University of Sfax, TunisiaSimranjit Kaur Sidhu, The University of Adelaide, Australia
Laura Säisänen, Kuopio University Hospital, Finland
Copyright © 2023 Osnabruegge, Kanig, Schwitzgebel, Litschel, Seiberl, Mack, Schecklmann and Schoisswohl. This is an open-access article distributed under the terms of the Creative Commons Attribution License (CC BY). The use, distribution or reproduction in other forums is permitted, provided the original author(s) and the copyright owner(s) are credited and that the original publication in this journal is cited, in accordance with accepted academic practice. No use, distribution or reproduction is permitted which does not comply with these terms.
*Correspondence: Mirja Osnabruegge, bWlyamEub3NuYWJydWVnZ2VAdW5pYncuZGU=