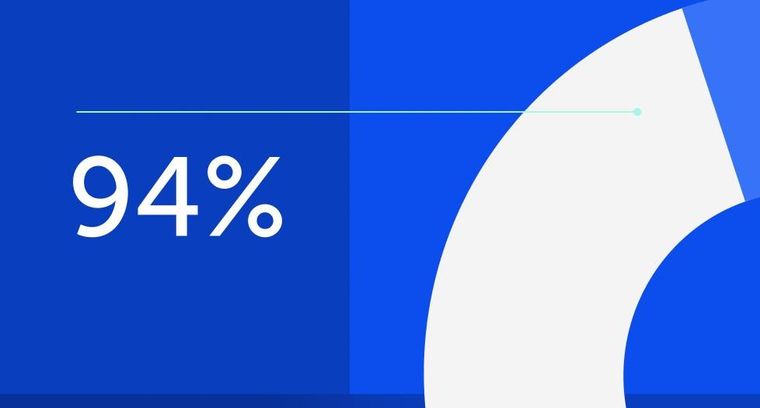
94% of researchers rate our articles as excellent or good
Learn more about the work of our research integrity team to safeguard the quality of each article we publish.
Find out more
MINI REVIEW article
Front. Hum. Neurosci., 02 December 2021
Sec. Brain Imaging and Stimulation
Volume 15 - 2021 | https://doi.org/10.3389/fnhum.2021.802244
This article is part of the Research TopicInterindividual Differences in Efficacy of Non-Invasive Brain StimulationView all 4 articles
Transcranial magnetic stimulation (TMS) can cause measurable effects on neural activity and behavioral performance in healthy volunteers. In addition, TMS is increasingly used in clinical practice for treating various neuropsychiatric disorders. Unfortunately, TMS-induced effects show large intra- and inter-subject variability, hindering its reliability, and efficacy. One possible source of this variability may be the spontaneous fluctuations of neuronal oscillations. We present recent studies using multimodal TMS including TMS-EMG (electromyography), TMS-tACS (transcranial alternating current stimulation), and concurrent TMS-EEG-fMRI (electroencephalography, functional magnetic resonance imaging), to evaluate how individual oscillatory brain state affects TMS signal propagation within targeted networks. We demonstrate how the spontaneous oscillatory state at the time of TMS influences both immediate and longer-lasting TMS effects. These findings indicate that at least part of the variability in TMS efficacy may be attributable to the current practice of ignoring (spontaneous) oscillatory fluctuations during TMS. Ignoring this state-dependent spread of activity may cause great individual variability which so far is poorly understood and has proven impossible to control. We therefore also compare two technical solutions to directly account for oscillatory state during TMS, namely, to use (a) tACS to externally control these oscillatory states and then apply TMS at the optimal (controlled) brain state, or (b) oscillatory state-triggered TMS (closed-loop TMS). The described multimodal TMS approaches are paramount for establishing more robust TMS effects, and to allow enhanced control over the individual outcome of TMS interventions aimed at modulating information flow in the brain to achieve desirable changes in cognition, mood, and behavior.
Barker et al. (1985) were the first to show that the human brain could be stimulated non-invasively using rapidly changing magnetic fields. This transcranial magnetic stimulation (TMS) method was virtually painless, required minimal preparation, and offered a flexible stimulation coil which could be rapidly and easily moved between scalp locations (brain areas). When the TMS coil was placed on the scalp above the motor cortex, movements could be induced in contralateral body parts, and the muscles’ responses could be measured using electromyography (EMG) (Rothwell et al., 1999; Hallett, 2000, 2007). These so-called “motor-evoked potentials” (MEPs) are caused by the excitation of corticospinal neurons (Berardelli et al., 1990; Burke et al., 1993; Di Lazzaro et al., 1998), and MEPs are still used in contemporary research as a measure of motor cortex excitability (Boroojerdi et al., 2002; Rossini et al., 2015).
Given its ability to directly influence brain processing (Romero et al., 2019), TMS can serve several purposes. It can be used to investigate whether and when a brain area is causally relevant for a cognitive function (Sack, 2006; Sack et al., 2006; de Graaf et al., 2009, 2015; Schuhmann et al., 2009; Jacobs et al., 2012b), and to map the brain’s functional connectivity profile (Pascual-Leone et al., 2000; Sack and Linden, 2003; Sack et al., 2007; Bäumer et al., 2009; de Graaf et al., 2009, 2012; Reithler et al., 2011; Arai et al., 2012). Since its development, TMS has therefore been widely used in cognitive neuroscience research (Walsh and Cowey, 2000; O’Shea and Walsh, 2007), not only to map the motor cortex (Gunduz et al., 2020), but also to study domains such as visual perception (Amassian et al., 1989; Kammer, 2007; Jacobs et al., 2012a, 2014; de Graaf et al., 2014; de Graaf and Sack, 2014; Janssens et al., 2020b), attention (Ashbridge et al., 1997; Sack et al., 2002, 2007; Rushworth and Taylor, 2006; Ronconi et al., 2014; Duecker and Sack, 2015), imagery (Sack et al., 2002, 2005; Cattaneo et al., 2012), language (Pascual-Leone et al., 1991; Schuhmann et al., 2012; Acheson and Hagoort, 2013; Tarapore et al., 2013), learning (de Weerd et al., 2012; Platz et al., 2012a,b), and memory (Osaka et al., 2007; van de Ven et al., 2012; van de Ven and Sack, 2013; Bonnì et al., 2015; Rademaker et al., 2017; Ferrari et al., 2018). In addition, TMS is increasingly used in clinical practice for treating various neuropsychiatric disorders (Lefaucheur et al., 2014, 2020; de Graaf et al., 2021a,b). TMS is used during stroke rehabilitation (Hummel and Cohen, 2006; Di Pino et al., 2014; Wessel et al., 2015), and as treatment for depression (Loo and Mitchell, 2005; Perera et al., 2016; Donse et al., 2018; Baeken et al., 2019; Sonmez et al., 2019) and schizophrenia (Cole et al., 2015).
Given the widespread use of TMS in research and clinical settings, one might assume that TMS generally leads to positive and consistent findings. Yet, the effects of TMS are not always robust and reliable. Inconsistent TMS effects between experiments/clinical trials could partially be due to methodological factors, such as differences in the coil placement method (Beam et al., 2009; Rusjan et al., 2010; Gomez et al., 2021). But even if methodological factors are kept constant, TMS effects can show substantial variability. There are two types of variability in the effects of TMS: different individuals may respond differently to TMS (inter-subject variability), and the effect of TMS may differ within the same individual over time (intra-subject variability). We should furthermore distinguish between two types of TMS effects: the immediate effects of single-pulse TMS, and the aftereffects of repetitive TMS (“rTMS”). Below, we present evidence that suggests that both the immediate and aftereffects of TMS show substantial inter- and intra-individual variability.
The immediate effects of single-pulse TMS to the primary motor cortex are often measured with MEPs, which provide a measure of the momentary TMS reactivity (Rossini et al., 2015). Within the same individual, TMS-MEP amplitudes vary over trials (Kiers et al., 1993; Burke et al., 1995; Wassermann, 2002; Rösler et al., 2008; Goetz et al., 2014; Goldsworthy et al., 2016a). Interestingly, optimization of TMS target localization does not necessarily improve the variability and reproducibility of TMS-induced MEPs (Jung et al., 2010). This finding already suggests that factors beyond the TMS parameters may contribute to immediate TMS reactivity.
Such variability in immediate TMS effects is not limited to the motor network. When stimulating early visual cortex, some individuals can perceive “phosphenes” (an illusory percept). The “phosphene threshold” (the minimal TMS intensity required to perceive a phosphene in half of the cases) is often used as a measure of visual cortex excitability (Boroojerdi et al., 2002; Bestmann et al., 2007; de Graaf et al., 2017). The probability of inducing phosphenes within the same participant can vary over time (Gerwig et al., 2003; Romei et al., 2008a, b; Dugué et al., 2011).
Variability in TMS aftereffects can be illustrated by evaluating individual responses to rTMS protocols that were designed to modulate synaptic plasticity beyond the duration of stimulation (Pascual-Leone et al., 1998; Ridding and Ziemann, 2010). Low (<1 Hz) and high (>1 Hz) frequency rTMS were originally reported to decrease and increase the excitability of the human motor cortex, respectively (Wassermann et al., 1998). This may indeed be the case on average, but when inspecting individual responses, not all participants showed these effects (Maeda et al., 2000). Similarly, intermittent and continuous theta burst stimulation (iTBS and cTBS, two forms of patterned rTMS) were reported to enhance and suppress motor cortex excitability for ∼30 min after stimulation, respectively (Huang et al., 2005). These findings have not always been replicated in another subject sample (Goldsworthy et al., 2012; Hordacre et al., 2017), and even if they are present at the group level, not all individuals show these effects (Cheeran et al., 2008; Nettekoven et al., 2015; Schilberg et al., 2017). In fact, one study reported that only 1 in 4 participants showed the expected pattern of results (Hamada et al., 2013). Another TMS procedure aimed at modulating neuroplasticity is called “paired associative stimulation” (PAS). Originally, PAS involved peripheral nerve stimulation that was paired with single-pulse TMS to primary motor cortex in order to enhance corticomotor excitability (Stefan et al., 2000), but PAS has also been employed to facilitate communication between the motor cortex and interconnected cortical areas (Veniero et al., 2013). As for the other plasticity-inducing TMS protocols, there is high inter-subject variability in the effects of PAS (Sale et al., 2007; Florian et al., 2008; López-Alonso et al., 2014), with a recent study reporting that only 61% of participants responded to PAS (Minkova et al., 2019).
Besides inter-subject variability, TMS aftereffects also show significant intra-subject variability. Some reports indicated that the aftereffects of iTBS and cTBS were relatively stable within the same individuals (Hinder et al., 2014; Vernet et al., 2014), but a recent study showed the opposite (Schilberg et al., 2017). Schilberg et al. (2017) further investigated the within-subject reliability of iTBS effects over the course of 60 min, and across two experimental sessions that were scheduled ∼8 days apart. They found that the effect of iTBS on corticospinal excitability (as measured with MEP amplitude) differed between sessions. The average increase in MEP amplitude was approximately 23% in the first session, but only approximately 6% during the second visit.
From these examples, it becomes clear that TMS effects show considerable inter- and intra-subject variability, for both the immediate effects of single-pulse TMS (MEP amplitudes, phosphene induction) and the longer-lasting plasticity effects as induced by rTMS, TBS, or PAS. The limited consistency of TMS effects can have negative consequences in research and clinical settings, because TMS effects are not always predictable or optimized. If TMS effects are not sufficiently reliable, they thus have limited use as a biomarker for individual changes in neuroplasticity and concomitant desirable changes in cognition and behavior (Schambra et al., 2015). It is therefore important to identify the factors that contribute to the variability of TMS effects (Corp et al., 2020, 2021), such that the consistency and efficacy of TMS can be improved. We here discuss one possible source of this variance, namely, spontaneous fluctuations in neuronal oscillations (Buzsáki and Draguhn, 2004; Pasley et al., 2009; Iscan et al., 2016; Bergmann, 2018). Below, we explain how spontaneous fluctuations in oscillatory brain state contribute to variability both in the immediate effects of TMS and in TMS-induced plasticity effects.
To investigate the link between TMS effect variability and ongoing neuronal oscillations, TMS can be combined with magneto- or electroencephalography (M/EEG). Specific characteristics of neuronal oscillations (i.e., their frequency, power, or phase; Palva and Palva, 2007) might be correlated with the immediate responsivity to single-pulse TMS. Indeed, the probability of inducing phosphenes when applying TMS to early visual cortex was negatively correlated with EEG alpha power prior to TMS (Romei et al., 2008a,b). The probability of perceiving TMS-induced phosphenes was also associated with the phase of ongoing EEG alpha oscillations (Dugué et al., 2011). Results have been less clear for the motor system. Some studies reported a negative association between pre-TMS EEG alpha power and TMS-induced MEP amplitude (Sauseng et al., 2009; Zarkowski et al., 2016). Others reported a negative association between TMS-MEP amplitude and oscillatory beta power (Lepage et al., 2008; Mäki and Ilmoniemi, 2010; Schulz et al., 2014), or no relation with oscillatory power in any frequency band (Mitchell et al., 2007; Berger et al., 2014). Spontaneous fluctuations in the phase of ongoing beta (Keil et al., 2014) and alpha (Schaworonkow et al., 2018, 2019; Bergmann et al., 2019) oscillations may also play a role in TMS-MEP variability. Note that inconsistencies across studies may in part be explained by methodological differences, such as differences in TMS intensity (Pellegrini et al., 2018).
Schilberg et al. (2021) recently assessed the relation between the power and phase of ongoing EEG alpha and beta oscillations with motor cortex TMS reactivity. They found that TMS-MEP amplitude correlated positively with pre-TMS oscillatory power in the alpha and beta bands. The authors also reported a significant effect of alpha phase on TMS-MEP amplitude, but there was no consistent alpha phase that led to high TMS-MEP amplitudes across participants. The latter is in contrast with previous reports showing that higher TMS-induced MEP amplitudes are mostly induced during alpha troughs instead of peaks (Schaworonkow et al., 2018, 2019; Zrenner et al., 2018). Interestingly, a standard FFT analysis did not reveal a significant correlation between pre-TMS beta phase and TMS-MEP amplitude, while a Hilbert transform did show an effect (Schilberg et al., 2021). This discrepancy between analyses may be partially explained by the variability in individual beta frequency (IBF), which is larger than the variability in individual alpha frequency (IAF) (Haegens et al., 2014). The Hilbert transform is less affected by frequency variations compared to the FFT approach, since the former can be used for non-stationary time series (Schilberg et al., 2021). Another contributing factor might be that participants were not involved in any active motor task. Ongoing beta power was therefore naturally low, making it more difficult to reliably estimate beta phase. When TMS is applied at high beta power, the relation between beta phase and TMS-MEP amplitude indeed becomes evident (Torrecillos et al., 2020). In any case, most of the evidence presented above is of correlational nature, because oscillations were measured rather than experimentally manipulated.
Transcranial alternating current stimulation (tACS) can be used to establish the causal relevance of neuronal oscillations (Herrmann et al., 2016). TACS is a form of non-invasive brain stimulation (NIBS) that involves electrical stimulation with a sinusoidal waveform (Antal and Paulus, 2013). It can be used to enhance the power of oscillations of a certain frequency within the stimulated brain area (Herrmann et al., 2013; Vossen et al., 2015; Vieira et al., 2020), potentially through mechanisms of entrainment (Thut et al., 2011; Huang et al., 2021) or spike-timing dependent plasticity (Herrmann et al., 2013; Vossen et al., 2015). The causal relevance of oscillatory phase can then be established by presenting stimuli at certain phases of the tACS waveform (de Graaf et al., 2020). It was previously shown that it is possible to apply TMS at certain tACS phases with high temporal precision (ten Oever et al., 2016), and that it is feasible to use simultaneous tACS-TMS to investigate the causal relation between oscillatory tACS phase and TMS-MEP amplitudes (Raco et al., 2016). The same logic was applied by Schilberg et al. (2018), who administered TMS pulses at eight equidistant phases of a tACS waveform, using IBF-, IAF-, or sham tACS to primary motor cortex. The authors found that tACS modulated TMS-MEP amplitude only for the IBF-tACS condition, and this effect seemed to be specific to individuals with lower IBF frequencies. These findings suggest that beta-tACS phase at the time of TMS influences the immediate effects of TMS (intra-subject variability), and that this effect interacts with the individual dominant beta frequency (between-subject variability) (Haegens et al., 2014).
Simultaneously combining TMS with M/EEG or tACS is an excellent approach to investigate the link between ongoing neuronal oscillations and the variability of TMS effects. However, this approach does not allow an accurate (high-resolution) visualization of the immediate effects of TMS at the level of the brain. Functional magnetic resonance imaging (fMRI) can be used to visualize TMS signal propagation, given its potential to measure whole-brain activation with good spatial resolution (Walsh and Cowey, 2000; Sack and Linden, 2003; Sack, 2006; Bestmann et al., 2008; Reithler et al., 2011). Simultaneous TMS-fMRI studies have shown that the effects of TMS pulses can extend beyond the targeted brain area, since signals can spread toward interconnected brain areas (Ruff et al., 2006; Sack et al., 2007; Blankenburg et al., 2010). Though the local effects of TMS pulses do not reach deeper than the superficial cortex, remote effects can even be observed in subcortical areas (Bergmann et al., 2021). Nonetheless, to achieve a full understanding of how TMS pulses propagate through functionally connected networks, it is important to investigate whether and how TMS-evoked fMRI responses vary as a function of ongoing neuronal oscillations on a trial-by-trial level. This was made possible with a unique setup, which simultaneously combines TMS, EEG, and fMRI.
This technically challenging experimental triad approach was introduced by our lab in 2013 (Peters et al., 2013). We demonstrated that concurrent TMS-EEG-fMRI is feasible and safe in both phantom and human measurements, and we showed that the EEG and fMRI data were of sufficient quality. Yet, the full potential of this approach only became apparent in a recent publication from our lab, in which we mapped whole-brain TMS signal propagation as a function of the pre-TMS oscillatory state as indexed by simultaneous EEG (Peters et al., 2020). In four healthy individuals, we applied triple-pulse (15-Hz) TMS to the right dorsal premotor area (PMd), while continuously measuring EEG. Triple-pulse TMS was used to probe the motor network with a sufficiently strong stimulus, rather than to modulate neuroplasticity as with typical rTMS protocols (the findings described here thus relate to immediate TMS effects).
TMS to PMd evoked both local and remote fMRI activation in a cortico-subcortical motor network, resembling the activations as seen for voluntary movements. It again became evident that different individuals may respond differently to TMS (inter-subject variability): two individuals showed less/more confined activations in response to TMS compared to the other two individuals. These individuals also showed less engagement of the motor network irrespective of TMS (“low activators,” the others were called “high activators”). It should be noted that the difference in TMS-evoked responses may in part be due to differences in TMS intensity between the “low activators” and “high activators.” In any case, to evaluate immediate TMS-evoked responses within the cortico-subcortical motor network as a function of oscillatory state, it was crucial that participants showed reliable engagement of the motor network. The EEG-informed analyses were therefore performed only for the two “high activators.”
The main question of interest was whether TMS signal propagation within a cortico-subcortical motor network varies with pre-TMS parietal alpha power. Pre-TMS alpha power was negatively correlated with TMS-evoked fMRI responses in both local and remote (including subcortical) areas of the motor network. This negative association is in line with the supposed inhibitory role of alpha oscillations (Klimesch et al., 2007). From these findings, we can conclude that, within the same individual, TMS pulses may propagate differently throughout the motor network depending on pre-TMS oscillatory state (intra-subject variability). Our group has recently also established the feasibility of using simultaneous TMS-EEG-fMRI for non-motor areas (Janssens et al., 2020a). This comes with additional technical challenges, including the determination of the TMS site and intensity, because most non-motor areas are so-called “silent” areas that do not show any overt response to TMS.
Thus far, we focused on within- and between-subject variability in the immediate effects of TMS, and how such variability can be linked to ongoing neuronal oscillations. There is reason to believe that changes in oscillatory state also contribute to variations in TMS-induced neuroplasticity (TMS aftereffects). Goldsworthy et al. (2016b) applied cTBS to the primary motor cortex, while phase-aligning the TMS pulses to either the peak or the trough of concurrent alpha-tACS. They investigated whether the response to cTBS, as measured with TMS-induced MEP amplitudes, depended on the alpha-tACS phase. The excitability of the motor cortex was suppressed (TMS-MEP amplitudes were reduced) when cTBS was aligned with alpha-tACS troughs. Crucially, cTBS did not modulate motor cortex plasticity when cTBS was aligned with alpha-tACS peaks. Furthermore, the effect of tACS-trough-aligned cTBS was greater for individuals with higher IAFs (Goldsworthy et al., 2016b). Thus, TMS-induced neuroplasticity may vary both as a function of the controlled momentary oscillatory state and the intrinsic dominant oscillatory frequency.
Besides oscillatory phase, the power of ongoing neuronal oscillations might be relevant for TMS-induced neuroplasticity as well. Guerra et al. (2018) showed that concurrent gamma tACS enhanced and prolonged iTBS-induced increases in TMS-MEP amplitude, in contrast to beta-tACS and sham-tACS (Guerra et al., 2018). This positive effect of simultaneous gamma tACS on iTBS efficacy was later replicated, but it seems that simultaneous gamma tACS reduced the efficacy of cTBS (Guerra et al., 2020a). These findings are especially relevant in a clinical context, where the goal is to employ rTMS to modulate neuroplasticity for longer periods of time. It would be beneficial to optimize plasticity-inducing TMS protocols based on oscillatory brain state, such that treatment efficacy can be improved.
Thus far, we have outlined that immediate and prolonged TMS effects vary considerably within- and between-individuals. We also showed that spontaneous fluctuations in neuronal oscillations can explain at least part of the variability in TMS effects, as can more stable oscillatory characteristics (individual peak frequencies). The question then becomes: how can we incorporate such oscillatory information into our TMS protocols?
The first step is to form a clear hypothesis regarding the to-be-targeted oscillatory frequency, since different frequency bands are associated with different functions (Başar et al., 1999; Ward, 2003; Clayton et al., 2018). Even within the same (e.g., alpha) frequency band, there might be different functionally relevant oscillation generators in the brain, which are not easily disentangled in the M/EEG signal (Bollimunta et al., 2011; Haegens et al., 2015; Sokoliuk et al., 2019). More advanced techniques might be needed to extract the relevant oscillatory frequency from the M/EEG signal (Schaworonkow et al., 2018). Once the relevant oscillatory frequency has been determined, there are two potential technical solutions that can directly account for oscillatory brain state during TMS: simultaneous tACS-TMS, and M/EEG-based “closed-loop” TMS (Huang et al., 2017).
As discussed previously, TMS can be applied at the (controlled) optimal tACS phase (Raco et al., 2016; ten Oever et al., 2016; Fehér et al., 2017). Crucially, individuals differ in terms of their oscillatory brain rhythms. For instance, peak alpha frequencies (IAFs) can range between 7 and 14 Hz across individuals (Haegens et al., 2014). To ensure optimal tACS efficacy, it is therefore important to individually calibrate the tACS frequency, for instance based on a resting state M/EEG measurement (Janssens et al., 2021) or through functional identification (Gundlach et al., 2017; Schilberg et al., 2018). Besides personalizing the tACS frequency, it might also be necessary to individually determine the optimal tACS phase to deliver TMS, given the recent finding that no consistent alpha phase was correlated to high TMS-MEP amplitudes (i.e., high TMS responsivity) across participants (Schilberg et al., 2021). Simultaneous tACS-TMS has already been used to link tACS beta phase to motor cortex TMS reactivity (Guerra et al., 2016; Schilberg et al., 2018). It has furthermore been shown that single TMS pulses applied to dorsolateral prefrontal cortex propagate differently through a cortical network depending on the phase of concurrent theta-tACS (Fehér et al., 2017). Thus, by applying single-pulse TMS at the optimal (controlled) tACS phase, TMS signal propagation may be modulated. Besides its relevance for immediate TMS effects, tACS can also be used to enhance and prolong TMS aftereffects, as described above (Goldsworthy et al., 2016b; Guerra et al., 2018, 2020a).
Simultaneous tACS-TMS is useful, but not perfect. Individual peak frequencies show good within-subject test-retest reliability (Grandy et al., 2013; Haegens et al., 2014; Janssens et al., 2021), but peak frequencies can still fluctuate, and the extent to which this happens differs across individuals. For example, IAF decreased over the course of 1 h during visual task performance, with some participants showing reductions of up to 2 Hz (Benwell et al., 2019). If tACS were to be applied at the originally determined peak frequency, tACS efficacy may be compromised, since the matching between the endogenous dominant frequency and the driving (tACS) frequency would not always be optimal (Romei et al., 2016). The best approach might thus be to continuously track the instantaneous dominant frequency, and to adjust the tACS frequency accordingly. However, it is difficult to recover EEG signals during tACS due to the sizeable tACS artifacts (Kasten and Herrmann, 2019). Another complication of the simultaneous tACS-TMS approach is that if the effect of tACS on oscillatory activity is not verified through means of concurrent M/EEG measurements, we cannot be certain that the applied tACS phase corresponds to the phase of ongoing neuronal oscillations. Finally, it could be the case that there is an “optimal” amount of oscillatory power, in the sense that if tACS enhances oscillatory power above a certain threshold, it might reduce the reactivity of a brain area to TMS.
In contrast to simultaneous tACS-TMS, the second technical solution to account for oscillatory brain state during TMS does measure ongoing neuronal oscillations. In this so-called “closed-loop” TMS approach, the M/EEG signal is continuously measured, and the timing of TMS pulses is adjusted to the optimal power and/or phase of the ongoing oscillations (Bergmann et al., 2016; Zrenner et al., 2016; Thut et al., 2017; Guerra et al., 2020b). This method can only be successful if the instantaneous phase can be reliably estimated (that is, if the power of the ongoing oscillations is sufficiently high). This has two important implications if the aim is to target specific oscillatory phases. Firstly, it might be necessary to control participants’ cognitive state (i.e., task engagement vs. rest) to ensure high oscillatory power. Secondly, the closed-loop TMS approach might fail in individuals that show naturally/pathologically low oscillatory power.
Irrespective of these technical challenges, EEG-based closed-loop TMS has already been applied successfully. It was shown that MEP amplitudes were higher during the rising phase of ongoing slow (<1 Hz) oscillations compared to the falling phase, when TMS was applied to primary motor cortex (Bergmann et al., 2012). Interestingly, these findings were consistent across two cognitive states (wakefulness and sleep). In another study, rTMS applied to primary motor cortex at the troughs of the ongoing alpha rhythm enhanced MEP amplitudes, while rTMS applied at alpha peaks did not (Zrenner et al., 2018). These findings clearly show that temporally targeting TMS pulses to the optimal oscillatory state improves its efficacy both in terms of signal propagation (immediate effects) and the induction of neuroplasticity (aftereffects).
TMS is widely used in both research and clinical settings. Still, its immediate and prolonged effects are not robust and reliable, as is evident from both intra- and inter-subject variability. One potential source of this variability may be the spontaneous fluctuations of neuronal oscillations. We showed this for both immediate TMS effects (TMS-MEP amplitudes, TMS phosphene induction, TMS-fMRI signal propagation), and for TMS aftereffects (of rTMS, TBS, or PAS). The oscillatory brain state can be accounted for during TMS by using either simultaneous tACS-TMS or closed-loop M/EEG-TMS. This may reduce both inter- and intra-individual variability in TMS effects. The described multimodal TMS approaches allow enhanced control over the individual outcome of TMS protocols aimed at modulating information flow and/or neuronal plasticity in the healthy and diseased brain. They therefore pave the way to stronger and more consistent TMS-induced improvements in cognition, mood, and behavior.
SJ: conceptualization, writing—original draft, writing—review and editing, and funding acquisition. AS: conceptualization, writing—review and editing, funding acquisition, and supervision. Both authors contributed to the article and approved the submitted version.
This research was supported by the Netherlands Organization for Scientific Research (AS: VICI grant 453-15-008; SJ: Research Talent grant 406-17- 540).
The authors declare that the research was conducted in the absence of any commercial or financial relationships that could be construed as a potential conflict of interest.
All claims expressed in this article are solely those of the authors and do not necessarily represent those of their affiliated organizations, or those of the publisher, the editors and the reviewers. Any product that may be evaluated in this article, or claim that may be made by its manufacturer, is not guaranteed or endorsed by the publisher.
Acheson, D. J., and Hagoort, P. (2013). Stimulating the brain’s language network: syntactic ambiguity resolution after TMS to the inferior frontal gyrus and middle temporal gyrus. J. Cogn. Neurosci. 25, 1664–1677. doi: 10.1162/JOCN_A_00430
Amassian, V. E., Cracco, R. Q., Maccabee, P. J., Cracco, J. B., Rudell, A., and Eberle, L. (1989). Suppression of visual perception by magnetic coil stimulation of human occipital cortex. Electroencephalogr. Clin. Neurophysiol. Evoked Potentials 74, 458–462. doi: 10.1016/0168-5597(89)90036-1
Antal, A., and Paulus, W. (2013). Transcranial alternating current stimulation (tACS). Front. Hum. Neurosci. 7:317. doi: 10.3389/fnhum.2013.00317
Arai, N., Lu, M.-K., Ugawa, Y., and Ziemann, U. (2012). Effective connectivity between human supplementary motor area and primary motor cortex: a paired-coil TMS study. Exp. Brain Res. 220, 79–87. doi: 10.1007/s00221-012-3117-5
Ashbridge, E., Walsh, V., and Cowey, A. (1997). Temporal aspects of visual search studied by transcranial magnetic stimulation. Neuropsychologia 35, 1121–1131.
Baeken, C., Brem, A.-K., Arns, M., Brunoni, A. R., Filipčić, I., Ganho-Ávila, A., et al. (2019). Repetitive transcranial magnetic stimulation treatment for depressive disorders: current knowledge and future directions. Curr. Opin. Psychiatry 32:409. doi: 10.1097/YCO.0000000000000533
Barker, A. T., Jalinous, R., and Freeston, I. L. (1985). Non-invasive magnetic stimulation of human motor cortex. Lancet 325, 1106–1107. doi: 10.1016/S0140-6736(85)92413-4
Başar, E., Başar-Eroglu, C., Karakaş, S., and Schürmann, M. (1999). Are cognitive processes manifested in event-related gamma, alpha, theta and delta oscillations in the EEG? Neurosci. Lett. 259, 165–168. doi: 10.1016/S0304-3940(98)00934-3
Bäumer, T., Schippling, S., Kroeger, J., Zittel, S., Koch, G., Thomalla, G., et al. (2009). Inhibitory and facilitatory connectivity from ventral premotor to primary motor cortex in healthy humans at rest – a bifocal TMS study. Clin. Neurophysiol. 120, 1724–1731. doi: 10.1016/J.CLINPH.2009.07.035
Beam, W., Borckardt, J. J., Reeves, S. T., and George, M. S. (2009). An efficient and accurate new method for locating the F3 position for prefrontal TMS applications. Brain Stimul. 2, 50–54. doi: 10.1016/J.BRS.2008.09.006
Benwell, C. S. Y., London, R. E., Tagliabue, C. F., Veniero, D., Gross, J., Keitel, C., et al. (2019). Frequency and power of human alpha oscillations drift systematically with time-on-task. Neuroimage 192, 101–114. doi: 10.1016/j.neuroimage.2019.02.067
Berardelli, A., Inghilleri, M., Cruccu, G., and Manfredi, M. (1990). Descending volley after electrical and magnetic transcranial stimulation in man. Neurosci. Lett. 112, 54–58. doi: 10.1016/0304-3940(90)90321-Y
Berger, B., Minarik, T., Liuzzi, G., Hummel, F. C., and Sauseng, P. (2014). EEG oscillatory phase-dependent markers of corticospinal excitability in the resting brain. Biomed. Res. Int. 2014:936096. doi: 10.1155/2014/936096
Bergmann, T. O. (2018). Brain state-dependent brain stimulation. Front. Psychol. 9:2108. doi: 10.3389/fpsyg.2018.02108
Bergmann, T. O., Karabanov, A., Hartwigsen, G., Thielscher, A., and Siebner, H. R. (2016). Combining non-invasive transcranial brain stimulation with neuroimaging and electrophysiology: current approaches and future perspectives. Neuroimage 140, 4–19. doi: 10.1016/J.NEUROIMAGE.2016.02.012
Bergmann, T. O., Lieb, A., Zrenner, C., and Ziemann, U. (2019). Pulsed facilitation of corticospinal excitability by the sensorimotor μ-Alpha rhythm. J. Neurosci. 39, 10034–10043. doi: 10.1523/JNEUROSCI.1730-19.2019
Bergmann, T. O., Mölle, M., Schmidt, M. A., Lindner, C., Marshall, L., Born, J., et al. (2012). EEG-guided transcranial magnetic stimulation reveals rapid shifts in motor cortical excitability during the human sleep slow oscillation. J. Neurosci. 32, 243–253. doi: 10.1523/JNEUROSCI.4792-11.2012
Bergmann, T. O., Varatheeswaran, R., Hanlon, C. A., Madsen, K. H., Thielscher, A., and Siebner, H. R. (2021). Concurrent TMS-fMRI for causal network perturbation and proof of target engagement. Neuroimage 237:118093. doi: 10.1016/J.NEUROIMAGE.2021.118093
Bestmann, S., Ruff, C. C., Blakemore, C., Driver, J., and Thilo, K. V. (2007). Spatial attention changes excitability of human visual cortex to direct stimulation. Curr. Biol. 17, 134–139. doi: 10.1016/j.cub.2006.11.063
Bestmann, S., Ruff, C. C., Blankenburg, F., Weiskopf, N., Driver, J., and Rothwell, J. C. (2008). Mapping causal interregional influences with concurrent TMS-fMRI. Exp. Brain Res. 191, 383–402. doi: 10.1007/s00221-008-1601-8
Blankenburg, F., Ruff, C. C., Bestmann, S., Bjoertomt, O., Josephs, O., Deichmann, R., et al. (2010). Studying the role of human parietal cortex in visuospatial attention with concurrent TMS-fMRI. Cereb. Cortex 20, 2702–2711. doi: 10.1093/cercor/bhq015
Bollimunta, A., Mo, J., Schroeder, C. E., and Ding, M. (2011). Neuronal mechanisms and attentional modulation of corticothalamic alpha oscillations. J. Neurosci. 31, 4935–4943. doi: 10.1523/JNEUROSCI.5580-10.2011
Bonnì, S., Veniero, D., Mastropasqua, C., Ponzo, V., Caltagirone, C., Bozzali, M., et al. (2015). TMS evidence for a selective role of the precuneus in source memory retrieval. Behav. Brain Res. 282, 70–75. doi: 10.1016/J.BBR.2014.12.032
Boroojerdi, B., Meister, I. G., Foltys, H., Sparing, R., Cohen, L. G., and Töpper, R. (2002). Visual and motor cortex excitability: a transcranial magnetic stimulation study. Clin. Neurophysiol. 113, 1501–1504. doi: 10.1016/S1388-2457(02)00198-0
Burke, D., Hicks, R., Gandevia, S. C., Stephen, J., Woodforth, I., and Crawford, M. (1993). Direct comparison of corticospinal volleys in human subjects to transcranial magnetic and electrical stimulation. J. Physiol. 470, 383–393. doi: 10.1113/JPHYSIOL.1993.SP019864
Burke, D., Hicks, R., Stephen, J., Woodforth, I., and Crawford, M. (1995). Trial-to-trial variability of corticospinal volleys in human subjects. Electroencephalogr. Clin. Neurophysiol. 97, 231–237. doi: 10.1016/0924-980X(95)00005-4
Buzsáki, G., and Draguhn, A. (2004). Neuronal olscillations in cortical networks. Science 304, 1926–1929. doi: 10.1126/SCIENCE.1099745
Cattaneo, Z., Bona, S., and Silvanto, J. (2012). Cross-adaptation combined with TMS reveals a functional overlap between vision and imagery in the early visual cortex. Neuroimage 59, 3015–3020. doi: 10.1016/j.neuroimage.2011.10.022
Cheeran, B., Talelli, P., Mori, F., Koch, G., Suppa, A., Edwards, M., et al. (2008). A common polymorphism in the brain-derived neurotrophic factor gene (BDNF) modulates human cortical plasticity and the response to rTMS. J. Physiol. 586, 5717–5725. doi: 10.1113/JPHYSIOL.2008.159905
Clayton, M. S., Yeung, N., and Cohen Kadosh, R. (2018). The many characters of visual alpha oscillations. Eur. J. Neurosci. 48, 2498–2508. doi: 10.1111/ejn.13747
Cole, J. C., Bernacki, C. G., Helmer, A., Pinninti, N., and O’reardon, J. P. (2015). Efficacy of transcranial magnetic stimulation (TMS) in the treatment of schizophrenia: a review of the literature to date. Innov. Clin. Neurosci. 12:12.
Corp, D. T., Bereznicki, H. G. K., Clark, G. M., Youssef, G. J., Fried, P. J., Jannati, A., et al. (2020). Large-scale analysis of interindividual variability in theta-burst stimulation data: results from the ‘big TMS data collaboration.’. Brain Stimul. 13, 1476–1488. doi: 10.1016/J.BRS.2020.07.018
Corp, D. T., Bereznicki, H. G. K., Clark, G. M., Youssef, G. J., Fried, P. J., Jannati, A., et al. (2021). Large-scale analysis of interindividual variability in single and paired-pulse TMS data. Clin. Neurophysiol. 132, 2639–2653. doi: 10.1016/J.CLINPH.2021.06.014
de Graaf, T. A., Duecker, F., Fernholz, M. H. P., and Sack, A. T. (2015). Spatially specific vs. unspecific disruption of visual orientation perception using chronometric pre-stimulus TMS. Front. Behav. Neurosci 9:5. doi: 10.3389/fnbeh.2015.00005
de Graaf, T. A., Duecker, F., Stankevich, Y., ten Oever, S., and Sack, A. T. (2017). Seeing in the dark: phosphene thresholds with eyes open versus closed in the absence of visual inputs. Brain Stimul. 10, 828–835. doi: 10.1016/j.brs.2017.04.127
de Graaf, T. A., Goebel, R., and Sack, A. T. (2012). Feedforward and quick recurrent processes in early visual cortex revealed by TMS? Neuroimage 61, 651–659. doi: 10.1016/J.NEUROIMAGE.2011.10.020
de Graaf, T. A., Jacobs, C., Roebroeck, A., and Sack, A. T. (2009). FMRI effective connectivity and TMS chronometry: complementary accounts of causality in the visuospatial judgment network. PLoS One 4:e8307. doi: 10.1371/journal.pone.0008307
de Graaf, T. A., Janssens, S. E. W., and Sack, A. T. (2021a). “Is non-invasive brain stimulation the low-hanging fruit?,” in Modern CNS Drug Discovery, ed. R. Schreiber (Cham: Springer), 115–128. doi: 10.1007/978-3-030-62351-7_8
de Graaf, T. A., Koivisto, M., Jacobs, C., and Sack, A. T. (2014). The chronometry of visual perception: review of occipital TMS masking studies. Neurosci. Biobehav. Rev. 45, 295–304. doi: 10.1016/j.neubiorev.2014.06.017
de Graaf, T. A., and Sack, A. T. (2014). Using brain stimulation to disentangle neural correlates of conscious vision. Front. Psychol. 5:1019. doi: 10.3389/FPSYG.2014.01019
de Graaf, T. A., Thomson, A., Duecker, F., and Sack, A. T. (2021b). “The various forms of non-invasive brain stimulation and their clinical relevance,” in Modern CNS Drug Discovery, ed. R. Schreiber (Cham: Springer), 103–113. doi: 10.1007/978-3-030-62351-7_7
de Graaf, T. A., Thomson, A., Janssens, S. E. W., van Bree, S., ten Oever, S., and Sack, A. T. (2020). Does alpha phase modulate visual target detection? three experiments with tACS-phase-based stimulus presentation. Eur. J. Neurosci. 51, 2299–2313. doi: 10.1111/ejn.14677
de Weerd, P., Reithler, J., van de Ven, V., Been, M., Jacobs, C., and Sack, A. T. (2012). Posttraining transcranial magnetic stimulation of striate cortex disrupts consolidation early in visual skill learning. J. Neurosci. 32, 1981–1988. doi: 10.1523/JNEUROSCI.3712-11.2011
Di Lazzaro, V., Restuccia, D., Oliviero, A., Profice, P., Ferrara, L., Insola, A., et al. (1998). Effects of voluntary contraction on descending volleys evoked by transcranial stimulation in conscious humans. J. Physiol. 508, 625–633. doi: 10.1111/J.1469-7793.1998.625BQ.X
Di Pino, G., Pellegrino, G., Assenza, G., Capone, F., Ferreri, F., Formica, D., et al. (2014). Modulation of brain plasticity in stroke: a novel model for neurorehabilitation. Nat. Rev. Neurol. 10, 597–608. doi: 10.1038/nrneurol.2014.162
Donse, L., Padberg, F., Sack, A. T., Rush, A. J., and Arns, M. (2018). Simultaneous rTMS and psychotherapy in major depressive disorder: clinical outcomes and predictors from a large naturalistic study. Brain Stimul. 11, 337–345. doi: 10.1016/J.BRS.2017.11.004
Duecker, F., and Sack, A. T. (2015). The hybrid model of attentional control: new insights into hemispheric asymmetries inferred from TMS research. Neuropsychologia 74, 21–29. doi: 10.1016/j.neuropsychologia.2014.11.023
Dugué, L., Marque, P., and VanRullen, R. (2011). The phase of ongoing oscillations mediates the causal relation between brain excitation and visual perception. J. Neurosci. 31, 11889–11893. doi: 10.1523/JNEUROSCI.1161-11.2011
Fehér, K. D., Nakataki, M., and Morishima, Y. (2017). Phase-dependent modulation of signal transmission in cortical networks through tACS-induced neural oscillations. Front. Hum. Neurosci. 11:471. doi: 10.3389/FNHUM.2017.00471
Ferrari, C., Cattaneo, Z., Oldrati, V., Casiraghi, L., Castelli, F., D’Angelo, E., et al. (2018). TMS over the cerebellum interferes with short-term memory of visual sequences. Sci. Rep. 8, 1–8. doi: 10.1038/s41598-018-25151-y
Florian, J., Müller-Dahlhaus, M., Orekhov, Y., Liu, Y., and Ziemann, U. (2008). Interindividual variability and age-dependency of motor cortical plasticity induced by paired associative stimulation. Exp. Brain Res. 187, 467–475. doi: 10.1007/s00221-008-1319-7
Gerwig, M., Kastrup, O., Meyer, B. U., and Niehaus, L. (2003). Evaluation of cortical excitability by motor and phosphene thresholds in transcranial magnetic stimulation. J. Neurol. Sci. 215, 75–78. doi: 10.1016/S0022-510X(03)00228-4
Goetz, S. M., Luber, B., Lisanby, S. H., and Peterchev, A. V. (2014). A novel model incorporating two variability sources for describing motor evoked potentials. Brain Stimul. 7, 541–552. doi: 10.1016/J.BRS.2014.03.002
Goldsworthy, M. R., Hordacre, B., and Ridding, M. C. (2016a). Minimum number of trials required for within- and between-session reliability of TMS measures of corticospinal excitability. Neuroscience 320, 205–209. doi: 10.1016/J.NEUROSCIENCE.2016.02.012
Goldsworthy, M. R., Pitcher, J. B., and Ridding, M. C. (2012). The application of spaced theta burst protocols induces long-lasting neuroplastic changes in the human motor cortex. Eur. J. Neurosci. 35, 125–134. doi: 10.1111/J.1460-9568.2011.07924.X
Goldsworthy, M. R., Vallence, A.-M., Yang, R., Pitcher, J. B., and Ridding, M. C. (2016b). Combined transcranial alternating current stimulation and continuous theta burst stimulation: a novel approach for neuroplasticity induction. Eur. J. Neurosci. 43, 572–579. doi: 10.1111/EJN.13142
Gomez, L. J., Dannhauer, M., and Peterchev, A. V. (2021). Fast computational optimization of TMS coil placement for individualized electric field targeting. Neuroimage 228:117696. doi: 10.1016/J.NEUROIMAGE.2020.117696
Grandy, T. H., Werkle-Bergner, M., Chicherio, C., Schmiedek, F., Lövdén, M., and Lindenberger, U. (2013). Peak individual alpha frequency qualifies as a stable neurophysiological trait marker in healthy younger and older adults. Psychophysiology 50, 570–582. doi: 10.1111/psyp.12043
Guerra, A., Asci, F., Zampogna, A., D’Onofrio, V., Petrucci, S., Ginevrino, M., et al. (2020a). Gamma-transcranial alternating current stimulation and theta-burst stimulation: inter-subject variability and the role of BDNF. Clin. Neurophysiol. 131, 2691–2699. doi: 10.1016/J.CLINPH.2020.08.017
Guerra, A., López-Alonso, V., Cheeran, B., and Suppa, A. (2020b). Solutions for managing variability in non-invasive brain stimulation studies. Neurosci. Lett. 719:133332. doi: 10.1016/J.NEULET.2017.12.060
Guerra, A., Pogosyan, A., Nowak, M., Tan, H., Ferreri, F., Di Lazzaro, V., et al. (2016). Phase dependency of the human primary motor cortex and cholinergic inhibition cancelation during beta tACS. Cereb. Cortex 26, 3977–3990. doi: 10.1093/CERCOR/BHW245
Guerra, A., Suppa, A., Bologna, M., D’Onofrio, V., Bianchini, E., Brown, P., et al. (2018). Boosting the LTP-like plasticity effect of intermittent theta-burst stimulation using gamma transcranial alternating current stimulation. Brain Stimul. 11, 734–742. doi: 10.1016/J.BRS.2018.03.015
Gundlach, C., Müller, M. M., Nierhaus, T., Villringer, A., and Sehm, B. (2017). Modulation of somatosensory alpha rhythm by transcranial alternating current stimulation at Mu-frequency. Front. Hum. Neurosci. 11:432. doi: 10.3389/fnhum.2017.00432
Gunduz, M. E., Pinto, C. B., Saleh Velez, F. G., Duarte, D., Pacheco-Barrios, K., Lopes, F., et al. (2020). Motor cortex reorganization in limb amputation: a systematic review of TMS motor mapping studies. Front. Neurosci. 14:314. doi: 10.3389/FNINS.2020.00314
Haegens, S., Barczak, A., Musacchia, G., Lipton, M. L., Mehta, A. D., Lakatos, P., et al. (2015). Laminar profile and physiology of the α rhythm in primary visual, auditory, and somatosensory regions of neocortex. J. Neurosci. 35, 14341–14352. doi: 10.1523/JNEUROSCI.0600-15.2015
Haegens, S., Cousijn, H., Wallis, G., Harrison, P. J., and Nobre, A. C. (2014). Inter- and intra-individual variability in alpha peak frequency. Neuroimage 92, 46–55. doi: 10.1016/j.neuroimage.2014.01.049
Hallett, M. (2000). Transcranial magnetic stimulation and the human brain. Nature 406, 147–150. doi: 10.1038/35018000
Hallett, M. (2007). Transcranial magnetic stimulation: a primer. Neuron 55, 187–199. doi: 10.1016/J.NEURON.2007.06.026
Hamada, M., Murase, N., Hasan, A., Balaratnam, M., and Rothwell, J. C. (2013). The role of interneuron networks in driving human motor cortical plasticity. Cereb. Cortex 23, 1593–1605. doi: 10.1093/CERCOR/BHS147
Herrmann, C. S., Rach, S., Neuling, T., and Strüber, D. (2013). Transcranial alternating current stimulation: a review of the underlying mechanisms and modulation of cognitive processes. Front. Hum. Neurosci. 7:279. doi: 10.3389/fnhum.2013.00279
Herrmann, C. S., Strüber, D., Helfrich, R. F., and Engel, A. K. (2016). EEG oscillations: from correlation to causality. Int. J. Psychophysiol. 103, 12–21. doi: 10.1016/j.ijpsycho.2015.02.003
Hinder, M. R., Goss, E. L., Fujiyama, H., Canty, A. J., Garry, M. I., Rodger, J., et al. (2014). Inter- and intra-individual variability following intermittent theta burst stimulation: implications for rehabilitation and recovery. Brain Stimul. 7, 365–371. doi: 10.1016/J.BRS.2014.01.004
Hordacre, B., Goldsworthy, M. R., Vallence, A. M., Darvishi, S., Moezzi, B., Hamada, M., et al. (2017). Variability in neural excitability and plasticity induction in the human cortex: a brain stimulation study. Brain Stimul. 10, 588–595. doi: 10.1016/J.BRS.2016.12.001
Huang, W. A., Stitt, I. M., Negahbani, E., Passey, D. J., Ahn, S., Davey, M., et al. (2021). Transcranial alternating current stimulation entrains alpha oscillations by preferential phase synchronization of fast-spiking cortical neurons to stimulation waveform. Nat. Commun. 12:3151. doi: 10.1038/s41467-021-23021-2
Huang, Y. Z., Edwards, M. J., Rounis, E., Bhatia, K. P., and Rothwell, J. C. (2005). Theta burst stimulation of the human motor cortex. Neuron 45, 201–206. doi: 10.1016/j.neuron.2004.12.033
Huang, Y. Z., Lu, M. K., Antal, A., Classen, J., Nitsche, M., Ziemann, U., et al. (2017). Plasticity induced by non-invasive transcranial brain stimulation: a position paper. Clin. Neurophysiol. 128, 2318–2329. doi: 10.1016/J.CLINPH.2017.09.007
Hummel, F. C., and Cohen, L. G. (2006). Non-invasive brain stimulation: a new strategy to improve neurorehabilitation after stroke? Lancet Neurol. 5, 708–712. doi: 10.1016/S1474-4422(06)70525-7
Iscan, Z., Nazarova, M., Fedele, T., Blagovechtchenski, E., and Nikulin, V. V. (2016). Pre-stimulus alpha oscillations and inter-subject variability of motor evoked potentials in single- and paired-pulse TMS paradigms. Front. Hum. Neurosci. 10:504. doi: 10.3389/FNHUM.2016.00504
Jacobs, C., de Graaf, T. A., Goebel, R., and Sack, A. T. (2012a). The temporal dynamics of early visual cortex involvement in behavioral priming. PLoS One 7:e48808. doi: 10.1371/journal.pone.0048808
Jacobs, C., de Graaf, T. A., and Sack, A. T. (2014). Two distinct neural mechanisms in early visual cortex determine subsequent visual processing. Cortex 59, 1–11. doi: 10.1016/j.cortex.2014.06.017
Jacobs, C., Goebel, R., and Sack, A. T. (2012b). Visual awareness suppression by pre-stimulus brain stimulation; a neural effect. Neuroimage 59, 616–624. doi: 10.1016/J.NEUROIMAGE.2011.07.090
Janssens, S. E. W., Sack, A. T., Duecker, F., Schuhmann, T., and de Graaf, T. A. (2020a). “Assessing brain-wide TMS-evoked responses depending on ocular and oscillatory state: a simultaneous TMS-EEG-fMRI project,” in Poster at the Cognitive Neuroscience Society Annual Meeting (Maastricht: Maastricht University), doi: 10.13140/RG.2.2.35677.74
Janssens, S. E. W., Sack, A. T., Jessen, S., and de Graaf, T. A. (2020b). Can processing of face trustworthiness bypass early visual cortex? A transcranial magnetic stimulation masking study. Neuropsychologia 137:107304. doi: 10.1016/j.neuropsychologia.2019.107304
Janssens, S. E. W., Sack, A. T., Ten Oever, S., and de Graaf, T. A. (2021). Calibrating rhythmic stimulation parameters to individual EEG markers: the consistency of individual alpha frequency in practical lab settings. Eur. J. Neurosci. doi: 10.1111/EJN.15418
Jung, N. H., Delvendahl, I., Kuhnke, N. G., Hauschke, D., Stolle, S., and Mall, V. (2010). Navigated transcranial magnetic stimulation does not decrease the variability of motor-evoked potentials. Brain Stimul. 3, 87–94. doi: 10.1016/J.BRS.2009.10.003
Kammer, T. (2007). Masking visual stimuli by transcranial magnetic stimulation. Psychol. Res. 71, 659–666. doi: 10.1007/s00426-006-0063-5
Kasten, F. H., and Herrmann, C. S. (2019). Recovering brain dynamics during concurrent tACS-M/EEG: an overview of analysis approaches and their methodological and interpretational pitfalls. Brain Topogr. 32, 1013–1019. doi: 10.1007/S10548-019-00727-7
Keil, J., Timm, J., SanMiguel, I., Schulz, H., Obleser, J., and Schönwiesner, M. (2014). Cortical brain states and corticospinal synchronization influence TMS-evoked motor potentials. J. Neurophysiol. 111, 513–519. doi: 10.1152/JN.00387.2013
Kiers, L., Cros, D., Chiappa, K. H., and Fang, J. (1993). Variability of motor potentials evoked by transcranial magnetic stimulation. Electroencephalogr. Clin. Neurophysiol. 89, 415–423. doi: 10.1016/0168-5597(93)90115-6
Klimesch, W., Sauseng, P., and Hanslmayr, S. (2007). EEG alpha oscillations: the inhibition-timing hypothesis. Brain Res. Rev. 53, 63–88. doi: 10.1016/j.brainresrev.2006.06.003
Lefaucheur, J. P., Aleman, A., Baeken, C., Benninger, D. H., Brunelin, J., Di Lazzaro, V., et al. (2020). Evidence-based guidelines on the therapeutic use of repetitive transcranial magnetic stimulation (rTMS): an update (2014–2018). Clin. Neurophysiol. 131, 474–528. doi: 10.1016/j.clinph.2019.11.002
Lefaucheur, J. P., André-Obadia, N., Antal, A., Ayache, S. S., Baeken, C., Benninger, D. H., et al. (2014). Evidence-based guidelines on the therapeutic use of repetitive transcranial magnetic stimulation (rTMS). Clin. Neurophysiol. 125, 2150–2206. doi: 10.1016/j.clinph.2014.05.021
Lepage, J. F., Saint-Amour, D., and Théoret, H. (2008). EEG and neuronavigated single-pulse TMS in the study of the observation/execution matching system: are both techniques measuring the same process? J. Neurosci. Methods 175, 17–24. doi: 10.1016/J.JNEUMETH.2008.07.021
Loo, C. K., and Mitchell, P. B. (2005). A review of the efficacy of transcranial magnetic stimulation (TMS) treatment for depression, and current and future strategies to optimize efficacy. J. Affect. Disord. 88, 255–267. doi: 10.1016/J.JAD.2005.08.001
López-Alonso, V., Cheeran, B., Río-Rodríguez, D., and Fernández-Del-Olmo, M. (2014). Inter-individual variability in response to non-invasive brain stimulation paradigms. Brain Stimul. 7, 372–380. doi: 10.1016/J.BRS.2014.02.004
Maeda, F., Keenan, J. P., Tormos, J. M., Topka, H., and Pascual-Leone, A. (2000). Interindividual variability of the modulatory effects of repetitive transcranial magnetic stimulation on cortical excitability. Exp. Brain Res. 133, 425–430. doi: 10.1007/S002210000432
Mäki, H., and Ilmoniemi, R. J. (2010). EEG oscillations and magnetically evoked motor potentials reflect motor system excitability in overlapping neuronal populations. Clin. Neurophysiol. 121, 492–501. doi: 10.1016/J.CLINPH.2009.11.078
Minkova, L., Peter, J., Abdulkadir, A., Schumacher, L. V., Kaller, C. P., Nissen, C., et al. (2019). Determinants of inter-individual variability in corticomotor excitability induced by paired associative stimulation. Front. Neurosci. 13:841. doi: 10.3389/FNINS.2019.00841
Mitchell, W. K., Baker, M. R., and Baker, S. N. (2007). Muscle responses to transcranial stimulation in man depend on background oscillatory activity. J. Physiol. 583, 567–579. doi: 10.1113/JPHYSIOL.2007.134031
Nettekoven, C., Volz, L. J., Leimbach, M., Pool, E. M., Rehme, A. K., Eickhoff, S. B., et al. (2015). Inter-individual variability in cortical excitability and motor network connectivity following multiple blocks of rTMS. Neuroimage 118, 209–218. doi: 10.1016/J.NEUROIMAGE.2015.06.004
Osaka, N., Otsuka, Y., Hirose, N., Ikeda, T., Mima, T., Fukuyama, H., et al. (2007). Transcranial magnetic stimulation (TMS) applied to left dorsolateral prefrontal cortex disrupts verbal working memory performance in humans. Neurosci. Lett. 418, 232–235. doi: 10.1016/J.NEULET.2007.01.087
O’Shea, J., and Walsh, V. (2007). Transcranial magnetic stimulation. Curr. Biol. 17, R196–R199. doi: 10.1016/j.cub.2007.01.030
Palva, S., and Palva, J. M. (2007). New vistas for α-frequency band oscillations. Trends Neurosci. 30, 150–158. doi: 10.1016/j.tins.2007.02.001
Pascual-Leone, A., Tormos, J. M., Keenan, J., Tarazona, F., Canete, C., and Catala, D. (1998). Study and modulation of human cortical excitability with transcranial magnetic stimulation. J. Clin. Neurophysiol. 15, 333–343.
Pascual-Leone, A., Walsh, V., and Rothwell, J. (2000). Transcranial magnetic stimulation in cognitive neuroscience – virtual lesion, chronometry, and functional connectivity. Curr. Opin. Neurobiol. 10, 232–237. doi: 10.1016/S0959-4388(00)00081-7
Pascual-Leone, A., Gates, J. R., and Dhuna, A. (1991). Induction of speech arrest and counting errors with rapid-rate transcranial magnetic stimulation. Neurology 41, 697–702. doi: 10.1212/WNL.41.5.697
Pasley, B. N., Allen, E. A., and Freeman, R. D. (2009). State-dependent variability of neuronal responses to transcranial magnetic stimulation of the visual cortex. Neuron 62, 291–303. doi: 10.1016/J.NEURON.2009.03.012
Pellegrini, M., Zoghi, M., and Jaberzadeh, S. (2018). The effect of transcranial magnetic stimulation test intensity on the amplitude, variability and reliability of motor evoked potentials. Brain Res. 1700, 190–198. doi: 10.1016/J.BRAINRES.2018.09.002
Perera, T., George, M. S., Grammer, G., Janicak, P. G., Pascual-Leone, A., and Wirecki, T. S. (2016). The clinical TMS society consensus review and treatment recommendations for TMS therapy for major depressive disorder. Brain Stimul. 9, 336–346. doi: 10.1016/J.BRS.2016.03.010
Peters, J. C., Reithler, J., de Graaf, T. A., Schuhmann, T., Goebel, R., and Sack, A. T. (2020). Concurrent human TMS-EEG-fMRI enables monitoring of oscillatory brain state-dependent gating of cortico-subcortical network activity. Commun. Biol. 3:40. doi: 10.1038/s42003-020-0764-0
Peters, J. C., Reithler, J., Schuhmann, T., de Graaf, T., Uludağ, K., Goebel, R., et al. (2013). On the feasibility of concurrent human TMS-EEG-fMRI measurements. J. Neurophysiol. 109, 1214–1227.
Platz, T., Roschka, S., Christel, M. I., Duecker, F., Rothwell, J. C., and Sack, A. T. (2012a). Early stages of motor skill learning and the specific relevance of the cortical motor system – a combined behavioural training and theta burst TMS study. Restor. Neurol. Neurosci. 30, 199–211. doi: 10.3233/RNN-2012-110204
Platz, T., Roschka, S., Doppl, K., Roth, C., Lotze, M., Sack, A. T., et al. (2012b). Prolonged motor skill learning – a combined behavioural training and theta burst TMS study. Restor. Neurol. Neurosci. 30, 213–224. doi: 10.3233/RNN-2012-110205
Raco, V., Bauer, R., Tharsan, S., and Gharabaghi, A. (2016). Combining TMS and tACS for closed-loop phase-dependent modulation of corticospinal excitability: a feasibility study. Front. Cell. Neurosci. 10:143. doi: 10.3389/FNCEL.2016.00143
Rademaker, R. L., van de Ven, V. G., Tong, F., and Sack, A. T. (2017). The impact of early visual cortex transcranial magnetic stimulation on visual working memory precision and guess rate. PLoS One 12:e0175230. doi: 10.1371/JOURNAL.PONE.0175230
Reithler, J., Peters, J. C., and Sack, A. T. (2011). Multimodal transcranial magnetic stimulation: using concurrent neuroimaging to reveal the neural network dynamics of noninvasive brain stimulation. Prog. Neurobiol. 94, 149–165. doi: 10.1016/j.pneurobio.2011.04.004
Ridding, M. C., and Ziemann, U. (2010). Determinants of the induction of cortical plasticity by non-invasive brain stimulation in healthy subjects. J. Physiol. 588, 2291–2304. doi: 10.1113/JPHYSIOL.2010.190314
Romei, V., Brodbeck, V., Michel, C., Amedi, A., Pascual-Leone, A., and Thut, G. (2008a). Spontaneous fluctuations in posterior α-Band EEG activity reflect variability in excitability of human visual areas. Cereb. Cortex 18, 2010–2018. doi: 10.1093/cercor/bhm229
Romei, V., Rihs, T., Brodbeck, V., and Thut, G. (2008b). Resting electroencephalogram alpha-power over posterior sites indexes baseline visual cortex excitability. Neuroreport 19, 203–208. doi: 10.1097/WNR.0B013E3282F454C4
Romei, V., Thut, G., and Silvanto, J. (2016). Information-based approaches of noninvasive transcranial brain stimulation. Trends Neurosci. 39, 782–795. doi: 10.1016/j.tins.2016.09.001
Romero, M. C., Davare, M., Armendariz, M., and Janssen, P. (2019). Neural effects of transcranial magnetic stimulation at the single-cell level. Nat. Commun. 10:2642. doi: 10.1038/s41467-019-10638-7
Ronconi, L., Basso, D., Gori, S., and Facoetti, A. (2014). TMS on right frontal eye fields induces an inflexible focus of attention. Cereb. Cortex 24, 396–402. doi: 10.1093/CERCOR/BHS319
Rösler, K. M., Roth, D. M., and Magistris, M. R. (2008). Trial-to-trial size variability of motor-evoked potentials. A study using the triple stimulation technique. Exp. Brain Res. 187, 51–59. doi: 10.1007/S00221-008-1278-Z
Rossini, P. M., Burke, D., Chen, R., Cohen, L. G., Daskalakis, Z., Di Iorio, R., et al. (2015). Non-invasive electrical and magnetic stimulation of the brain, spinal cord, roots and peripheral nerves: basic principles and procedures for routine clinical and research application. An updated report from an I.F.C.N. committee. Clin. Neurophysiol. 126, 1071–1107. doi: 10.1016/J.CLINPH.2015.02.001
Rothwell, J. C., Hallett, M., Berardelli, A., Eisen, A., Rossini, P., and Paulus, W. (1999). Magnetic stimulation: motor evoked potentials. Electroencephalogr. Clin. Neurophysiol. 52, 97–103.
Ruff, C. C., Blankenburg, F., Bjoertomt, O., Bestmann, S., Freeman, E., Haynes, J. D., et al. (2006). Concurrent TMS-fMRI and psychophysics reveal frontal influences on human retinotopic visual cortex. Curr. Biol. 16, 1479–1488. doi: 10.1016/j.cub.2006.06.057
Rushworth, M. F. S., and Taylor, P. C. J. (2006). TMS in the parietal cortex: updating representations for attention and action. Neuropsychologia 44, 2700–2716. doi: 10.1016/J.NEUROPSYCHOLOGIA.2005.12.007
Rusjan, P. M., Barr, M. S., Farzan, F., Arenovich, T., Maller, J. J., Fitzgerald, P. B., et al. (2010). Optimal transcranial magnetic stimulation coil placement for targeting the dorsolateral prefrontal cortex using novel magnetic resonance image-guided neuronavigation. Hum. Brain Mapp. 31, 1643–1652. doi: 10.1002/HBM.20964
Sack, A. T. (2006). Transcranial magnetic stimulation, causal structure–function mapping and networks of functional relevance. Curr. Opin. Neurobiol. 16, 593–599. doi: 10.1016/j.conb.2006.06.016
Sack, A. T., Camprodon, J. A., Pascual-Leone, A., and Goebel, R. (2005). The dynamics of interhemispheric compensatory processes in mental imagery. Science 308, 702–704. doi: 10.1126/SCIENCE.1107784
Sack, A. T., Kohler, A., Bestmann, S., Linden, D. E. J., Dechent, P., Goebel, R., et al. (2007). Imaging the brain activity changes underlying impaired visuospatial judgments: simultaneous fMRI, TMS, and behavioral studies. Cereb. Cortex 17, 2841–2852. doi: 10.1093/cercor/bhm013
Sack, A. T., Kohler, A., Linden, D. E. J., Goebel, R., and Muckli, L. (2006). The temporal characteristics of motion processing in hMT/V5+: combining fMRI and neuronavigated TMS. Neuroimage 29, 1326–1335. doi: 10.1016/J.NEUROIMAGE.2005.08.027
Sack, A. T., and Linden, D. E. J. (2003). Combining transcranial magnetic stimulation and functional imaging in cognitive brain research: possibilities and limitations. Brain Res. Rev. 43, 41–56. doi: 10.1016/S0165-0173(03)00191-7
Sack, A. T., Sperling, J. M., Prvulovic, D., Formisano, E., Goebel, R., Di Salle, F., et al. (2002). Tracking the mind’s image in the brain II: transcranial magnetic stimulation reveals parietal asymmetry in visuospatial imagery. Neuron 35, 195–204. doi: 10.1016/S0896-6273(02)00745-6
Sale, M. V., Ridding, M. C., and Nordstrom, M. A. (2007). Factors influencing the magnitude and reproducibility of corticomotor excitability changes induced by paired associative stimulation. Exp. Brain Res. 18, 615–626. doi: 10.1007/S00221-007-0960-X
Sauseng, P., Klimesch, W., Gerloff, C., and Hummel, F. C. (2009). Spontaneous locally restricted EEG alpha activity determines cortical excitability in the motor cortex. Neuropsychologia 47, 284–288. doi: 10.1016/J.NEUROPSYCHOLOGIA.2008.07.021
Schambra, H. M., Ogden, R. T., Martínez-Hernández, I., Lin, X., Chang, Y. B., Rahman, A., et al. (2015). The reliability of repeated TMS measures in older adults and in patients with subacute and chronic stroke. Front. Cell. Neurosci. 9:335. doi: 10.3389/FNCEL.2015.00335
Schaworonkow, N., Caldana Gordon, P., Belardinelli, P., Ziemann, U., Bergmann, T. O., and Zrenner, C. (2018). μ-rhythm extracted with personalized EEG filters correlates with corticospinal excitability in real-time phase-triggered EEG-TMS. Front. Neurosci. 12:954. doi: 10.3389/FNINS.2018.00954/BIBTEX
Schaworonkow, N., Triesch, J., Ziemann, U., and Zrenner, C. (2019). EEG-triggered TMS reveals stronger brain state-dependent modulation of motor evoked potentials at weaker stimulation intensities. Brain Stimul. 12, 110–118. doi: 10.1016/J.BRS.2018.09.009
Schilberg, L., Engelen, T., ten Oever, S., Schuhmann, T., de Gelder, B., de Graaf, T. A., et al. (2018). Phase of beta-frequency tACS over primary motor cortex modulates corticospinal excitability. Cortex 103, 142–152. doi: 10.1016/j.cortex.2018.03.001
Schilberg, L., Schuhmann, T., and Sack, A. T. (2017). Interindividual variability and intraindividual reliability of intermittent theta burst stimulation-induced neuroplasticity mechanisms in the healthy brain. J. Cogn. Neurosci. 29, 1022–1032. doi: 10.1162/JOCN_A_01100
Schilberg, L., ten Oever, S., Schuhmann, T., and Sack, A. T. (2021). Phase and power modulations on the amplitude of TMS-induced motor evoked potentials. PLoS One 16:e0255815. doi: 10.1371/JOURNAL.PONE.0255815
Schuhmann, T., Schiller, N. O., Goebel, R., and Sack, A. T. (2009). The temporal characteristics of functional activation in Broca’s area during overt picture naming. Cortex 45, 1111–1116. doi: 10.1016/J.CORTEX.2008.10.013
Schuhmann, T., Schiller, N. O., Goebel, R., and Sack, A. T. (2012). Speaking of which: dissecting the neurocognitive network of language production in picture naming. Cereb. Cortex 22, 701–709. doi: 10.1093/CERCOR/BHR155
Schulz, H., Übelacker, T., Keil, J., Müller, N., and Weisz, N. (2014). Now I am ready—now I am not: the influence of pre-TMS oscillations and corticomuscular coherence on motor-evoked potentials. Cereb. Cortex 24, 1708–1719. doi: 10.1093/CERCOR/BHT024
Sokoliuk, R., Mayhew, S. D., Aquino, K. M., Wilson, R., Brookes, M. J., Francis, S. T., et al. (2019). Two spatially distinct posterior alpha sources fulfill different functional roles in attention. J. Neurosci. 39, 7183–7194. doi: 10.1523/JNEUROSCI.1993-18.2019
Sonmez, A. I., Camsari, D. D., Nandakumar, A. L., Voort, J. L. V., Kung, S., Lewis, C. P., et al. (2019). Accelerated TMS for depression: a systematic review and meta-analysis. Psychiatry Res. 273, 770–781. doi: 10.1016/J.PSYCHRES.2018.12.041
Stefan, K., Kunesch, E., Cohen, L. G., Benecke, R., and Classen, J. (2000). Induction of plasticity in the human motor cortex by paired associative stimulation. Brain 123, 572–584. doi: 10.1093/BRAIN/123.3.572
Tarapore, P. E., Findlay, A. M., Honma, S. M., Mizuiri, D., Houde, J. F., Berger, M. S., et al. (2013). Language mapping with navigated repetitive TMS: proof of technique and validation. Neuroimage 82, 260–272. doi: 10.1016/J.NEUROIMAGE.2013.05.018
ten Oever, S., de Graaf, T. A., Bonnemayer, C., Ronner, J., Sack, A. T., and Riecke, L. (2016). Stimulus presentation at specific neuronal oscillatory phases experimentally controlled with tACS: implementation and applications. Front. Cell. Neurosci 10:240. doi: 10.3389/fncel.2016.00240
Thut, G., Bergmann, T. O., Fröhlich, F., Soekadar, S. R., Brittain, J. S., Valero-Cabré, A., et al. (2017). Guiding transcranial brain stimulation by EEG/MEG to interact with ongoing brain activity and associated functions: a position paper. Clin. Neurophysiol. 128, 843–857. doi: 10.1016/J.CLINPH.2017.01.003
Thut, G., Veniero, D., Romei, V., Miniussi, C., Schyns, P., and Gross, J. (2011). Rhythmic TMS causes local entrainment of natural oscillatory signatures. Curr. Biol. 21, 1176–1185. doi: 10.1016/j.cub.2011.05.049
Torrecillos, F., Falato, E., Pogosyan, A., West, T., Di Lazzaro, V., and Brown, P. (2020). Motor cortex inputs at the optimum phase of beta cortical oscillations undergo more rapid and less variable corticospinal propagation. J. Neurosci. 40, 369–381. doi: 10.1523/JNEUROSCI.1953-19.2019
van de Ven, V., Jacobs, C., and Sack, A. T. (2012). Topographic contribution of early visual cortex to short-term memory consolidation: a transcranial magnetic stimulation study. J. Neurosci. 32, 4–11. doi: 10.1523/JNEUROSCI.3261-11.2012
van de Ven, V., and Sack, A. T. (2013). Transcranial magnetic stimulation of visual cortex in memory: cortical state, interference and reactivation of visual content in memory. Behav. Brain Res. 236, 67–77. doi: 10.1016/J.BBR.2012.08.001
Veniero, D., Ponzo, V., and Koch, G. (2013). Paired associative stimulation enforces the communication between interconnected areas. J. Neurosci. 33, 13773–13783. doi: 10.1523/JNEUROSCI.1777-13.2013
Vernet, M., Bashir, S., Yoo, W. K., Oberman, L., Mizrahi, I., Ifert-Miller, F., et al. (2014). Reproducibility of the effects of theta burst stimulation on motor cortical plasticity in healthy participants. Clin. Neurophysiol. 125, 320–326. doi: 10.1016/J.CLINPH.2013.07.004
Vieira, P. G., Krause, M. R., and Pack, C. C. (2020). tACS entrains neural activity while somatosensory input is blocked. PLoS Biol. 18:e3000834. doi: 10.1371/journal.pbio.3000834
Vossen, A., Gross, J., and Thut, G. (2015). Alpha power increase after transcranial alternating current stimulation at alpha frequency (a-tACS) reflects plastic changes rather than entrainment. Brain Stimul. 8, 499–508. doi: 10.1016/j.brs.2014.12.004
Walsh, V., and Cowey, A. (2000). Transcranial magnetic stimulation and cognitive neuroscience. Nat. Rev. Neurosci. 1, 73–80. doi: 10.1038/35036239
Ward, L. M. (2003). Synchronous neural oscillations and cognitive processes. Trends Cogn. Sci. 7, 553–559. doi: 10.1016/J.TICS.2003.10.012
Wassermann, E. M. (2002). Variation in the response to transcranial magnetic brain stimulation in the general population. Clin. Neurophysiol. 113, 1165–1171. doi: 10.1016/S1388-2457(02)00144-X
Wassermann, E. M., Wedegaertner, F. R., Ziemann, U., George, M. S., and Chen, R. (1998). Crossed reduction of human motor cortex excitability by 1-Hz transcranial magnetic stimulation. Neurosci. Lett. 250, 141–144. doi: 10.1016/S0304-3940(98)00437-6
Wessel, M. J., Zimerman, M., and Hummel, F. C. (2015). Non-invasive brain stimulation: an interventional tool for enhancing behavioral training after stroke. Front. Hum. Neurosci. 9:265. doi: 10.3389/FNHUM.2015.00265
Zarkowski, P., Shin, C. J., Dang, T., Russo, J., and Avery, D. (2016). EEG and the variance of motor evoked potential amplitude. Clin. EEG Neurosci. 37, 247–251. doi: 10.1177/155005940603700316
Zrenner, C., Belardinelli, P., Müller-Dahlhaus, F., and Ziemann, U. (2016). Closed-loop neuroscience and non-invasive brain stimulation: a tale of two loops. Front. Cell. Neurosci. 10:92. doi: 10.3389/FNCEL.2016.00092
Keywords: transcranial magnetic stimulation (TMS), inter-and intra-subject variability, neuronal oscillations, multimodal TMS, closed-loop TMS
Citation: Janssens SEW and Sack AT (2021) Spontaneous Fluctuations in Oscillatory Brain State Cause Differences in Transcranial Magnetic Stimulation Effects Within and Between Individuals. Front. Hum. Neurosci. 15:802244. doi: 10.3389/fnhum.2021.802244
Received: 26 October 2021; Accepted: 16 November 2021;
Published: 02 December 2021.
Edited by:
Philipp Ruhnau, University of Central Lancashire, United KingdomReviewed by:
Christopher Gundlach, Leipzig University, GermanyCopyright © 2021 Janssens and Sack. This is an open-access article distributed under the terms of the Creative Commons Attribution License (CC BY). The use, distribution or reproduction in other forums is permitted, provided the original author(s) and the copyright owner(s) are credited and that the original publication in this journal is cited, in accordance with accepted academic practice. No use, distribution or reproduction is permitted which does not comply with these terms.
*Correspondence: Shanice E. W. Janssens, U2hhbmljZS5qYW5zc2Vuc0BtYWFzdHJpY2h0dW5pdmVyc2l0eS5ubA==
Disclaimer: All claims expressed in this article are solely those of the authors and do not necessarily represent those of their affiliated organizations, or those of the publisher, the editors and the reviewers. Any product that may be evaluated in this article or claim that may be made by its manufacturer is not guaranteed or endorsed by the publisher.
Research integrity at Frontiers
Learn more about the work of our research integrity team to safeguard the quality of each article we publish.