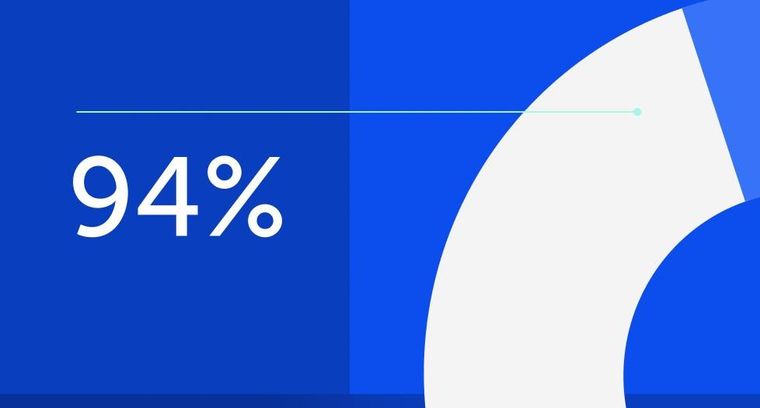
94% of researchers rate our articles as excellent or good
Learn more about the work of our research integrity team to safeguard the quality of each article we publish.
Find out more
REVIEW article
Front. Hum. Neurosci., 28 September 2021
Sec. Brain Imaging and Stimulation
Volume 15 - 2021 | https://doi.org/10.3389/fnhum.2021.749162
This article is part of the Research TopicNeural Bases of Neurological and Psychiatric Disorders and Their Neuromodulation TreatmentsView all 36 articles
Non-invasive neuromodulation technology is important for the treatment of brain diseases. The effects of focused ultrasound on neuronal activity have been investigated since the 1920s. Low intensity transcranial focused ultrasound (tFUS) can exert non-destructive mechanical pressure effects on cellular membranes and ion channels and has been shown to modulate the activity of peripheral nerves, spinal reflexes, the cortex, and even deep brain nuclei, such as the thalamus. It has obvious advantages in terms of security and spatial selectivity. This technology is considered to have broad application prospects in the treatment of neurodegenerative disorders and neuropsychiatric disorders. This review synthesizes animal and human research outcomes and offers an integrated description of the excitatory and inhibitory effects of tFUS in varying experimental and disease conditions.
Brain stimulation techniques have shown efficacy for ameliorating neurological and psychiatric disorders (Hoy and Fitzgerald, 2010). Invasive electrical brain stimulation modalities, such as vagus nerve stimulation (VNS) or deep brain stimulation (DBS), require the surgical placement of electrodes in the brain (Rahimpour et al., 2021; Wang Y. et al., 2021). The modulation effects of non-invasive strategies, such as transcranial direct current stimulation (tDCS) and repetitive transcranial magnetic stimulation (rTMS) are limited to the cortical surface (Hoy and Fitzgerald, 2010; Romanella et al., 2020). Transcranial focused ultrasound (tFUS) transmits low-intensity ultrasound into the brain non-invasively and focuses on deep brain regions. It is an emerging neuromodulation technology with the advantages of better spatial resolution and safety (Mehic et al., 2014).
The frequency of the ultrasound mechanical wave is higher than the range of human hearing (> 20 kHz). Ultrasound can be focused across solid structures and transmitted long distances with minimal power loss in soft biological tissues (Bystritsky et al., 2011). It has been the most widely used biomedical imaging modality for a long history. Ultrasound display different biological effect based on a different intensity. High-intensity (> 200 W/cm2) focused ultrasound induces permanent lesions through coagulation of cellular proteins and thermal ablation. It has been approved by the FDA for the treatment of tremors associated with Parkinson’s disease and essential tremors. Medium intensity (100–200 W/cm2) ultrasound has been used to break through the blood-brain barrier for drug delivery. Low-intensity (< 100 W/cm2) focused ultrasound is considered the main form of non-invasive neuromodulation (Kubanek, 2018).
Research on the potential clinical value of focused ultrasound as a neuromodulation method started half a century ago, with interest increasing dramatically over the past decade (Fini and Tyler, 2017). Studies using various parameters have shown that tFUS can modulate neural activity in the brains of animals and humans. It has the potential to modulate brain activities indicated by sensory or motor behaviors, functional magnetic resonance imaging (fMRI), electroencephalography (EEG), and near-infrared spectroscopy (NIRS) (Krishna et al., 2018; Legon et al., 2018b). The findings of in vitro and in vivo experiments have promoted the understanding of ultrasound neuromodulation. However, the high variability in experimental conditions, different ultrasound parameters, as well as partially conflicting results, led to contradictory interpretations. To achieve clinical application, it is important to clarify the excitatory and inhibitory effects of tFUS. In this review, we summarize the current findings of the brain modulatory effects of tFUS in both animal and human studies. We discussed findings of the excitatory or inhibitory effect of tFUS in light of varying experimental and disease conditions. Finally, the proposed mechanism for ultrasound neuromodulation was discussed.
We searched the literature in the databases of the PubMed/MEDLINE/EMBASE with the following research string, in March 2021: (“Low-intensity focused ultrasound[Title/Abstract]” OR “Transcranial focused ultrasound[Title/Abstract]”) AND (“Neuromodulation[Title/Abstract]” OR “Brain Stimulation [Title/Abstract]”). Non-English language studies and duplicates were excluded. Studies were also excluded according to the following criteria: (1) Study investigating the diagnostic aspect of transcranial ultrasound rather than neuromodulation effects; (2) Study investigating the application of transcranial ultrasound in ablation neurosurgery or drug delivery; (3) Study focusing on the development of new ultrasound device; (4) Study published as the conference abstract without a full text, as dissertation or those published in books or only providing surrogate biomarkers. The resulting original articles that reported the use of tFUS in animals and human subjects to modulate brain activity were included (Tables 1, 2). Ultimately 41 studies were selected for discussion in this review, including 23 animals and 18 human studies.
The neuromodulation effect of ultrasound depends on the stimulation parameters used. Pulsed ultrasound is the main form of neuromodulation application to minimize the probability of tissue heating or damage. Fundamental frequency, pulse repetition frequency (PRF), duty cycle (DC), sonication duration (SD), and intensity are the five most important elements that define a sonication protocol. The fundamental frequency refers to the number of oscillatory cycles per unit time and is inversely proportional to wavelength. Fundamental frequency significantly affects the spatial targeting of brain regions. A higher fundamental frequency induces stimulation with a tighter focus (when the frequency is > 1 MHz, the diameter can be as narrow as few millimeters). But ultrasound with higher fundamental frequency has more transcranial attenuation and scattering. 200–650 kHz have been used in most human and animal studies. PRF refers to the rate of the pulses delivered. DC is the proportion of each pulse filled with ultrasound cycles, which is an important parameter that determines the direction of the neuromodulation effect. SD refers to the total time from the onset of the first pulse to the termination of the last pulse, which may determine the total intensity and tissue heating. The combination of PRF, DC, and SD may affect the inhibition or excitation of cortical neurons. The intensity of acoustic exposure is commonly characterized by two parameters: the spatial-peak temporal average (Ispta) and the spatial-peak pulse average (Isppa). Ispta measures the average intensity during the entire sonication therapy and is proportional to SD. Isppa measures the average intensity of a single pulse and provides estimates of short-term mechanical biological effects. The mechanical index (MI) is an indicator of the risk of potentially destructive biomechanical effects on tissues, such as inertial cavitation. It is equal to the peak negative pressure divided by the square of the fundamental frequency. Considering that thermal and mechanical effects may cause brain damage, FDA guidelines for cephalic ultrasound suggest a maximum safety value range of Ispta < 94 mW/cm2, Isppa < 190 W/cm2, and MI < 1.9 to avoid cavitation and heating in humans (United States Food and Drug Administration. Marketing clearance of diagnostic ultrasound systems and transducers. Draft guidance for industry and food and drug administration staff. 2019).
To use ultrasound for neuromodulation therapy, an important issue is to clarify its excitatory or inhibitory effects on the central nervous system. We reviewed the reports of the neuromodulatory effects of ultrasound in animals. Studies have been conducted in mice, rats, ovines, swine, sheep, and macaques with different ultrasound parameters, and brain targets. The reported brain targets included the motor cortex, midbrain, somatosensory cortex, hypothalamus, right anterior cortex, hippocampus, thalamus, and visual cortex. The excitatory and inhibitory effects vary with the stimulation targets and sonication parameters (Table 1).
Several studies have reported excitatory effects of tFUS targeting the cortex and deep brain areas. For targeting at the motor cortex, unfocused ultrasound with a frequency of 500 kHz evokes motor responses, and the responses are not related to the stimulation of the peripheral auditory system, which emphasizes the direct action of motor neurons in the brain (Mohammadjavadi et al., 2019). Another study used low-intensity tFUS (500 kHz) to stimulate mouse motor cortical regions and demonstrated that tFUS induced tail movement, neural activity, and hemodynamic responses (cortical blood flow, CBF) immediately and returned to baseline at ∼5.5 s. The relationships between CBF and key ultrasound parameters showed that the CBF responses increasing with increasing intensities (Isppa = 0.2–1.1 W/cm2, SD = 400 ms, DC = 40%) and SD (SD = 50–400 ms, Isppa = 0.8 W/cm2, DC = 40%), while had a weak dependence on DC (DC = 10–40%, SD = 50–400 ms, Isppa = 0.8 W/cm2) (Yuan et al., 2020). Kim et al. (2014) examined the stimulation effects of tFUS with a range of sonication parameters administrated to the somatomotor cortex of rats in vivo. Based on comparison experiments, the authors found that 50% of DC outperforms 30 and 70%. Operating at 50% DC, the use of TBDs in the range of 1–5 ms, serving as an effective pulsing scheme, 350 kHz fundamental frequency outperforms 650 kHz, pulsed tFUS outperforms equivalent continuous sonication stimulate the target cortex most effectively. Further, for the parameter of SD, they found that 400 ms required higher Isppa for eliciting motor activity than 300 ms, which may indicate that the longer SD might have recruited inhibitory neural circuits or neural cells. Overall, this study found non-linear neural responses to tFUS that might include the activation of motor neurons and inhibitory interneurons (Kim et al., 2014).
For other cortex regions, Yu et al. (2016) demonstrated that low intensity (Ispta < 1 Mw/cm2) 500 kHz pulsed tFUS targeting the right anterior cortex of rats evoked time-locked activation of the brain, as correlated to intensity and SD. They also suggested electrophysiological source imaging as a useful tool to quantify tFUS effects, guide its use for neuromodulation (Yu et al., 2016). The study of Moore et al. (2015) recorded local field potential fluctuations in the motor cortex in response to ultrasound stimulation of the mouse somatosensory barrel cortex. Ultrasound stimulation at 350 kHz and 42.8% DC induced depolarization of cerebral cortical pyramidal neurons, which indicates an excitatory effect of nerve transmission (Moore et al., 2015). Fisher and Gumenchuk (2018) demonstrated that tFUS with a frequency of 510 kHz reduced the latency and concentrates the spatial patterns of neural activity in the primary somatosensory cortex. The findings suggest the using of tFUS to target and alter spatial aspects of sensory receptive fields on the cerebral cortex (Fisher and Gumenchuk, 2018). As for deep brain targets, the research of Yoo et al. (2011) revealed that pulsed FUS targeting at the thalamus significantly decreased the time to emergence from ketamine/xylazine anesthesia in rats. This study provided early evidence for the excitatory neuromodulatory potential of tFUS targeting the deep brain area (Yoo et al., 2011). The study of Baek et al. (2018) provides the first evidence in the disease model showing that tFUS-induced cerebellar modulation improved impaired sensorimotor function in stroke mice and could be a potential strategy for post stroke recovery. They hypothesized that the effectiveness could be attributable to long-term potentiation of hypoactive neural connections between the motor cortex and deep cerebellar nucleus induced by tFUS. Longer follow-up studies are necessary to fully confirm the effects of tFUS on post stroke recovery (Baek et al., 2018).
Three studies targeting the thalamus showed inhibitory effects of tFUS. The study of Darrow et al. (2019) revealed that 3.2 MHz tFUS targeting the rat thalamus reversibly suppressed somatosensory evoked potentials (SEPs) in a spatially and intensity-dependent manner. The effect was independent of the parameters of DC, peak pressure, or modulation frequency. The ultrasound frequency used in this study is higher than most other studies, which may cause an obvious thermal effect and lead to the suppression effect (Darrow et al., 2019). The study of Dallapiazza et al. (2018) demonstrated that low-intensity focused ultrasound with frequencies of 1.1 MHz, 220 kHz, and 650 kHz inhibited sensory evoked potentials with a spatial resolution of ∼2 mm in swine, supporting its potential use in non-invasive brain mapping. Longer ultrasound pulses delivered over prolonged periods tend to result in a more substantial and sustained decrease in neural function. The physiological effects lasted for a period of several minutes without inducing tissue heating or histological damage (Dallapiazza et al., 2018). Kim et al. (2015) reported that the application of pulsed 350 kHz tFUS using a 5% DC and Isppa intensity of 3 W/cm2 to the visual cortex area suppressed the magnitude of visual evoked potentials (VEPs) in rats. Under the same conditions, higher intensity (5 W/cm2) or DC (8.3%) induced slight elevation in VEPs (Kim et al., 2015). Yoon et al. (2019) investigated the bimodal effects of tFUS in the modulation of the sensorimotor cortex and thalamus in an ovine model by evaluating the rate and magnitude of electrophysiological responses to a wide range of sonication parameters. The study suggests that a shorter SD (≤ ∼500 ms) at a higher DC (30%) favored excitation, and a longer SD (∼1 min) at a lower DC (≤ 10%) resulted in suppression. For excitation effects, the use of 15.8 W/cm2 Isppa generated a higher response rate than the use of 18.2 W/cm2. This study provided important evidence for the effects of varying sonication parameters on neuromodulation response (Yoon et al., 2019).
The above studies that reported excitatory and inhibitory regulation effects demonstrated the feasibility of tFUS to the clinical application of neural modulation. However, the ultrasound parameters used by different research groups are different, no two studies used consistent parameters. The rules of the neuromodulation effect corresponding to parameter changes are still unclear. Change of any one of the main ultrasound parameters may produce a change of efficacy of excitatory or inhibitory effects, with the correlation relationship is considered to be non-linear. Nevertheless, the influence of any parameters on the modulatory direction is inconclusive, and the combination of multiple parameters seems more difficult. Further research should be conducted to explore how to precisely control parameters to achieve a specific adjustment purpose.
Other evidence of inhibitory neuromodulation effects comes from research in animal models of epilepsy. An early study by Min et al. (2011) reported that low-intensity, pulsed FUS (690 kHz) sonication suppressed the number of epileptic signal bursts and severe epileptic behavior using an acute epilepsy model in animals. Yang et al. (2020) delivered pulsed closed-loop transcranial ultrasound stimulation with a frequency of 500 kHz to the hippocampus to modulate neural oscillation and effectively inhibited the seizure of a temporal lobe epilepsy (TLE) mouse. The study of Chen et al. (2020) reported that pulsed tFUS (500 kHz) effectively suppressed epileptic spikes in an acute epilepsy animal model and found that ultrasound pulsation interferes with neuronal activity. Zhengrong Lin et al. (2020) demonstrated that low-intensity pulsed FUS could improve the electrophysiological activities and behavioral outcomes in non-human primate models of epilepsy. The study also demonstrated that ultrasound suppressed abnormal epileptiform activities of neurons from human epileptic slices (Lin et al., 2020). The suppression of epileptic neuro-electric activity and the behavior of tFUS may mainly indicate inhibitory effects.
Moreover, most current animal experiments are performed using anesthetized animal models to prevent animal motion during ultrasound administration. A recently published study by Wang X. et al. (2021) demonstrated that the behavioral states, especially anesthesia, modulate the ultrasound stimulation-induced neural activity. The effect of pharmacological sedation on tFUS induced effects is not clear and may obfuscate the interpretation of the tFUS neuromodulation response (Jerusalem et al., 2019). Studies in awake and freely moving animals are needed. Lee et al. (2018) conducted the first study that using awake rats to evaluate the neuromodulatory efficacy of tFUS. They developed a miniature tFUS headgear with a frequency of 600 kHz, and the stimulation of motor cortical areas by their configuration elicited body movements from various areas in freely moving rats. Compared with the anesthetic rats, the stimulation in awake rats induced an increased response rate with reduced variability and shorter latency. However, the lack of measurement of electrophysiological signals in this study limited its information (Lee et al., 2018). Li et al. (2019) designed a miniature and lightweight head-mounted ultrasound stimulator that can be used in freely moving mice. When target at the primary somatosensory cortex barrel field, the device evoked head-turning behaviors and action potentials recorded in situ (Li et al., 2019). The application of stimulation in awake animals is valuable for the research on the neuromodulation effects and mechanisms of tFUS, especially for investigations that are not possible with anesthesia, such as social behavioral studies and disease models that are influenced by anesthesia (e.g., epilepsy).
The excitatory effects of tFUS were identified in healthy human subjects in 9 studies as indicated by fMRI and EEG. Six studies showed the inhibitory effects of TUS indicated by SEPs, motor induced potentials (MEPs), and intracortical facilitation (ICF). The modulated brain targets included the motor cortex, somatosensory cortex, thalamus, caudate nuclei, and visual cortex. Several studies have reported the effect of FUS stimulation on patients with chronic pain, posttraumatic disorder of consciousness, and Alzheimer’s disease (AD) (Table 2).
Studies provide evidence that FUS stimulation can activate the brain to produce sensory and motor nerve responses without any external stimulation. Three studies conducted by Lee et al. (2015) showed excitatory effects of tFUS on the human primary and secondary somatosensory cortex and visual cortex. tFUS targeting the human hand somatosensory cortex (S1) elicited somatosensory sensations with anatomical specificity up to a finger and evoked EEG potentials similar to the classical SEPs generated by median nerve stimulation (Lee et al., 2015). In another study, they reported that FUS targeting to the human visual cortex (V1) induced the perception of phosphine and activated a network of regions involved in visual and higher-order cognitive processes (Lee et al., 2016b). They also reported that tFUS application to the human bilateral primary somatosensory cortex (S1) and secondary somatosensory cortex (S2) elicited various tactile sensations in the targeted hand area. This study also showed the possibility of simultaneous stimulation of the S1 and S2 (proximal to each other) with ultrasound, which has not been feasible with conventional non-invasive brain stimulation approaches such as TMS or tDCS. The ability to selectively stimulate multiple human brain areas in a spatially restricted manner may offer an unprecedented opportunity to study the causal relationships between brain activity and subsequent efferent behaviors (Lee et al., 2016a). Ai et al. (2018) combined tFUS with high-field 7T fMRI in humans and evaluated its neuromodulation effect by the BOLD response. tFUS stimulation targeted individual finger representations within M1 increased the activation volumes of the M1 thumb representation in a spatially restricted manner. These results provide a more detailed perspective on the spatial resolution of tFUS for neuromodulation of individual finger representations within a single gyrus reflected by the BOLD response (Leo et al., 2016; Ai et al., 2018).
FUS stimulation modulates the neural response induced by behavioral tasks and TMS-induced MEPs. Liu et al. (2021) evaluated the interference of tFUS stimulation at S1 on the performances in a mechanical vibration frequency discrimination task in a group of healthy human participants. The behavioral results indicated that low-intensity tFUS stimulation improved vibration frequency discrimination capability. EEG and electrophysiological source imaging (ESI) results revealed that tFUS improved sensory discrimination capability through exciting the targeted sensory cortex (Liu et al., 2021). The study by Gibson et al. (2018) used a diagnostic imaging ultrasound system to stimulate the motor cortex. Increased TMS-induced MEPs amplitude (34%) was recorded up to 6 min after stimulation but disappeared 11 min later (Gibson et al., 2018). This result contrasts with the research of Legon et al., who reported tFUS inhibited MEPs induced by TMS (Legon et al., 2018b). As discussed by the authors, the different findings may be caused by stimulation parameters and other methodological factors. Yu et al. (2020) demonstrated the neuromodulatory effects of low-intensity tFUS on human voluntary movement-related cortical potential (MRCP). Through ESI, the results showed that tFUS modulates MRCP source dynamics with high spatiotemporal resolutions and significantly increases the MRCP source profile amplitude (MSPA), and further, a high PRF enhances the MSPA outperforms a low UPRF does. This study provides the first evidence that tFUS enhances human endogenous motor cortical activities through excitatory modulation (Yu et al., 2020).
Five studies by one research group reported the inhibitory modulation effect of tFUS targeting the primary somatosensory cortex (CP3), unilateral sensory nuclei of the thalamus, and primary motor cortex. They used similar ultrasound parameters with the 500 kHz frequency, 36% DC, 500 ms SD, and 1 kHz PRF. The first study reported that tFUS targeting CP3 significantly attenuated the amplitudes of SEPs and modulated the spectral content of brain oscillations elicited by median nerve stimulation, while enhanced the somatosensory discrimination abilities of participants. Importantly, this research also showed that the influence of tFUS on brain activity can be spatially restricted within 1 cm (Legon et al., 2014). The following study by Mueller et al. (2014) found that tFUS preferentially affected the phase distribution of the beta band and modulated the phase rate of both beta and gamma frequencies. The third study reported that tFUS targeting the thalamus attenuated the SEPs generated in the ventral-lateral nucleus and serially connected cortical regions. The study provided initial evidence that tFUS can non-invasively modulate subcortical areas of the human brain with good spatial precision and resolution (Legon et al., 2018a). The fourth study showed that tFUS inhibits the amplitude of single-pulse MEPs and attenuates intracortical facilitation, which confirms previous results that ultrasound results in effective neuronal inhibition. tFUS did not affect short interval intracortical inhibition (SICI) or reduce reaction time in a simple stimulus response task in this study (Legon et al., 2018b). The studies of Fomenko et al. (2020) reported that tFUS targeting at the motor cortex displayed inhibitory effects on cortical excitability, but the effects on SICI were inconsistent. Furthermore, other studies have reported heterogeneous effects of FUS targeting on the motor cortex, demonstrating increased cortical excitability or the amplitude of MEPs after prolonged sonication (Gibson et al., 2018; Yu et al., 2020).
Like animal studies, human studies have inconsistent conclusions about whether ultrasound modulation is exciting or inhibiting. In addition to the influence of sonication parameters, differences in detection methods also may introduce inherent variability. For example, the study of Leo et al. (2016) and Legon et al. (2018a) used similar FUS protocols (500 kHz frequency, 36% DC, and 1 kHz RPF) targeting the M1 cortex demonstrated excitatory or inhibitory effect, respectively. Leo et al. (2016) reported BOLD fMRI signals were induced by tFUS, while Legon et al. (2018a) detected reduced amplitude of MEPs and intracortical facilitation. Further prospective studies are needed to elucidate region- and neuron-specific sensitivity to focused ultrasound with a wider range of parameters.
Preliminary research has explored the role of this technology in the treatment of human brain functional diseases. In healthy subjects, Sanguinetti et al. (2020) reported two experiments that demonstrated that tFUS targeting the right inferior frontal gyrus (rIFG) enhances mood and changed the functional connectivity in networks related to emotional regulation. These results suggest that tFUS may be useful in modulating mood and emotional regulation networks (Sanguinetti et al., 2020). Badran BW conducted a controlled, double-blind study to investigate whether sonication targeting deep brain structures produces quantifiable antinociceptive effects in healthy adults. The study reveals that two 10-min sessions of tFUS delivered to the right anterior thalamus produced significant antinociceptive effects in thermal pain threshold ratings, suggesting that tFUS appears to be able to focally target deep brain structures and modulate pain perceptions (Badran et al., 2020).
Four studies have provided evidence for its potential in disease treatment in patients with chronic pain, a minimally conscious state, AD and epilepsy. Hameroff et al. (2013) reported a double-blind, sham-controlled crossover study applying 8 MHz unfocused transcranial ultrasound stimulation targeted to the posterior frontal cortex in 31 patients with chronic pain. They found improvement in subjective mood 10 and 40 min after stimulation, suggesting that ultrasound treatment can beneficially affect mental state. However, the study was limited in clinic time and unable to perform extensive psychological testing (Hameroff et al., 2013). Monti et al. (2016) published a clinical case report showed that thalamic tFUS stimulation improved the minimally conscious state in a patient after acute brain injury. An important study by Beisteiner et al. (2020) introduces a clinical sonication technique based on single ultrashort ultrasound pulses (3 μs) repeated every 200–300 ms transcranial pulse stimulation (TPS), which markedly differs from others. The TPS was applied to brain regions of patients with probable AD. The researchers found that the treatment significantly improved neuropsychological scores and upregulated corresponding memory network. This study provided comprehensive preclinical and clinical feasibility, safety, and efficacy data for TPS in the treatment of AD. Thus, widespread neuroscientific application and translation of the method to clinical therapy is encouraged (Beisteiner et al., 2020). An early research by Brinker et al. (2020) developed a laboratory-built experimental device platform and successfully delivered repetitive low-intensity tFUS across the hippocampus in seizure onset zones of patients with drug-resistant TLE. Further study is still ongoing for investigating the effects of tFUS therapeutic neuromodulation in the patients with TLE (Brinker et al., 2020). Overall, to move tFUS forward as a potential therapy for brain diseases, more researches in patients are needed to explore targeting, dosing, and parameter optimization modes.
The precise mechanisms of neuromodulation with ultrasound are unclear. Studies have explored the mechanism for the neuromodulation effect of tFUS from the perspective of brain networks, neural cells, and molecules (Table 1). The effect of ultrasound neuromodulation on brain network connections has been reported in researches using macaques and rats. Verhagen et al. (2019) reported that 40 s of ultrasound stimulation in macaques modulated the brain activation for more than 1 h and the ultrasound displays offline and sustained impact on the connectional fingerprint of stimulated brain regions. The study of Folloni et al. (2019) demonstrated that a tFUS protocol impacts activity in the subcortical brain structure of the amygdala and deep cortical region of the anterior cingulate cortex in macaques. The stimulation suppressed the connectivity of the targeted brain area to its network (Folloni et al., 2019). The study of Fouragnan et al. (2019) investigated how representations of counterfactual choices are held in memory and guide behavior in macaque monkeys. tFUS was used to focally alter the neural activity to examine the causal importance of the anterior cingulate cortex (ACC) for behavior. They found that tFUS significantly impaired translation of counterfactual choice values into actual behavioral change and changed the activity and connectivity maps of the ACC (Fouragnan et al., 2019). The study of Zhang et al. (2021) reported that FUS reduced the network connections of epilepsy circuits and change the structure of the brain network at the whole-brain level. The adjustment effect of tFUS on neural network connection may be the mechanism of its repeated application to improve brain diseases.
At the cellular level of neurons, two studies reported bimodal neural modulation effects of tFUS in macaques. The study of Wattiez et al. (2017) assessed the neuromodulatory effects of tFUS in awake behaving monkeys by recording discharge activity from a brain region (supplementary eye field) reciprocally connected with the stimulated region (frontal eye field). They demonstrated that stimulation in the visual cortex significantly changed the activity of 40% of neurons in the recorded region. Half of the neurons showed transiently increased activity, and the other half showed decreased activity. In this study, the ultrasonic effect was quantified based on the direct measurement of the intensity of the modulation induced on a single neuron in a freely performing animal. In particular, the study suggests that different neurons respond differently to tFUS stimuli and indicates further parametric studies should pay attention to the regulation of neural activity at the cell level (Wattiez et al., 2017). Yang et al. (2021) reported that medium amplitude tFUS (425 kPa free-field at 250 kHz) in the macaque brain modulated the activity of neurons at the target in dual directions (excitation and suppression). This simultaneous excitatory and suppressive neuromodulation may be mediated by activation of large excitatory pyramidal and small inhibitory interneurons, respectively. This study first examined the neuromodulation effects of FUS at the whole-brain level and the ability of concurrent FUS and MRI to evaluate causal interactions between functional circuits and neuron-class selectivity (Yang et al., 2021).
Several studies provided evidence for the mechanism of the neuromodulation effect at the molecular level. Recent investigations on the interactions between sound pressure waves and brain tissue suggest that ultrasound primarily exerts its modulatory effects through mechanical action on cell membranes, notably affecting ion channel gating (Kubanek et al., 2016). Liang et al. (2020) explored the efficacy and mechanisms of tFUS in treating pain caused by soft tissue injury. Low-intensity focused ultrasound relieved pain, and the mechanism could be attributed to decreasing the release of analgesic substances from the nerve and reducing local inflammatory factors (Liang et al., 2020). Na Pang et al. (2021) demonstrated that transcranial ultrasound stimulation was effective in modulating the learning behaviors of mice and the expression of apoptosis, oxidative stress, and inflammation. Xu et al. (2020) demonstrated that low-intensity ultrasound is able to stimulate DA release and helps to regenerate dopaminergic neurons in a mouse model of Parkinson’s disease (PD). Huang et al. (2019) reported that non-invasive low-intensity pulsed ultrasound stimulation improved c-fos expression and reduced the incidence rate (p < 0.05) and length of primary cilia (p < 0.01) of neurons in the rat CA1 hippocampus. Bobola et al. (2020) reported that acutely applied tFUS activated microglia to colocalize with Aβ plaques in a mouse model of AD, and 5 days of tFUS cleared almost 50% of the Aβ plaque. The study of Chen et al. (2020) suggested that pulsed tFUS exposure effectively suppresses epileptic spikes in an acute epilepsy animal model and revealed that ultrasound pulsation affects the PI3K-Akt-mTOR pathway, which might be the molecular mechanism. These studies only provide preliminary clues, and more studies are needed in the future.
Currently, ultrasound for neuromodulation in humans generally follows the guidelines of the FDA for adult cephalic applications. Legon et al. (2020) qualitatively evaluated minor adverse events in seven independent experiments using different ultrasound protocols for human neuromodulation. The intensity (Isppa) used in these studies ranged from 11.56 to 17.12 W/cm2 that is considerably lower than FDA thresholds for ultrasound diagnostics. The parameter of Ispta, which is defined as the Isppa multiplied by the duty factor, in these studies was above FDA thresholds for diagnostics. They found that none of the participants experienced serious adverse effects, and 7/64 reported mild to moderate symptoms probably related to ultrasound treatment. The symptoms include neck pain, attention problems, muscle twitches, and anxiety. They concluded that the symptom rate and type induced by tFUS are similar to other forms of human non-invasive neuromodulation, such as TMS and tDCS, these two have been used as safe forms of human neuromodulation. Despite the causation role of ultrasound has not been defined, the findings suggest limiting the intensity used in future ultrasound experiments for neuromodulation (Legon et al., 2020). Gaur et al. (2020) examined a range of experimental parameters, including the number of focal spot locations, the number of FUS bursts applied to each spot, the timing between FUS sessions, and applied acoustic intensity, to investigate the safety of FUS neuromodulation. The in situ intensities were 9.5 W/cm2 in macaques and 6.7 W/cm2 in sheep, similar to and slightly higher than previously reported Ispta values of up to 4.4 W/cm2 in humans. Repeated FUS neuromodulation at various intensity levels for multiple days did not cause histologic damage. The study suggesting that the neuromodulation protocols evaluated do not cause tissue damage and provide important information for the safety profiles of FUS neuromodulation (Gaur et al., 2020).
Overall, tFUS is a promising non-invasive brain stimulation technology. The current findings in both animal and human studies reported both the excitatory and inhibitory modulatory effects of tFUS on brain activity. These findings confirmed the application prospects of this technology in the treatment of brain functional diseases. However, the current research does not clarify the correlation among the roles of the stimulus parameters in excitatory or inhibitory modulatory effects. The excitatory and inhibitory effects shown in current research are mostly on a macro level, and it is not clear whether these effects are derived from inhibitory or excitatory neuronal activity. Inhibitory effects may come from the excitement of inhibitory neurons or the inhibition of excitatory neurons, and the opposite is also possible. The physiological state (active or resting) of neurons in the stimulated area and its connected areas and how these neurons interact during the modulation process could also influence the results. Like clinical trials, animal experiments have been conducted in normal animals and disease model animals, the targets include the sensory cortex, motor cortex, and deep nuclei such as the thalamus. The range of ultrasonic parameters used in animal studies were similar to that in clinical trials. Due to the differences in skull size and geometry of the brain, the parameters from tFUS used in animals are less likely to be translated to humans. Further animal studies should focus on the change law of excitation or inhibition effect with the fine change of parameters, and to further reveal the mechanism of regulation effect at the nerve conduction, cellular and molecular levels. The safety profile, effectiveness, and finer device parameters in humans in either healthy or diseased conditions need further research.
TZ drafted this report. SH, CL, NP, and YW revised and expanded. All authors contributed to the article and approved the submitted version.
We acknowledge the support by the National Natural Science Foundation of China (No. 82071483) and the Natural Science Foundation of Beijing (No. 7212048) within the funding program Open Access Publishing.
The authors declare that the research was conducted in the absence of any commercial or financial relationships that could be construed as a potential conflict of interest.
All claims expressed in this article are solely those of the authors and do not necessarily represent those of their affiliated organizations, or those of the publisher, the editors and the reviewers. Any product that may be evaluated in this article, or claim that may be made by its manufacturer, is not guaranteed or endorsed by the publisher.
Ai, L., Bansal, P., Mueller, J. K., and Legon, W. (2018). Effects of transcranial focused ultrasound on human primary motor cortex using 7T fMRI: a pilot study. BMC Neurosci. 19:56. doi: 10.1186/s12868-018-0456-6
Badran, B. W., Caulfield, K. A., Stomberg-Firestein, S., Summers, P. M., Dowdle, L. T., Savoca, M., et al. (2020). Sonication of the anterior thalamus with MRI-Guided transcranial focused ultrasound (tFUS) alters pain thresholds in healthy adults: a double-blind, sham-controlled study. Brain Stimul. 13, 1805–1812. doi: 10.1016/j.brs.2020.10.007
Baek, H., Pahk, K. J., Kim, M. J., Youn, I., and Kim, H. (2018). Modulation of cerebellar cortical plasticity using low-intensity focused ultrasound for poststroke sensorimotor function recovery. Neurorehabil. Neural Repair 32, 777–787. doi: 10.1177/1545968318790022
Beisteiner, R., Matt, E., Fan, C., Baldysiak, H., Schonfeld, M., Philippi Novak, T., et al. (2020). Transcranial pulse stimulation with ultrasound in Alzheimer’s disease-a new navigated focal brain therapy. Adv. Sci. 7:1902583. doi: 10.1002/advs.201902583
Bobola, M. S., Chen, L., Ezeokeke, C. K., Olmstead, T. A., Nguyen, C., Sahota, A., et al. (2020). Transcranial focused ultrasound, pulsed at 40 Hz, activates microglia acutely and reduces Abeta load chronically, as demonstrated in vivo. Brain Stimul. 13, 1014–1023. doi: 10.1016/j.brs.2020.03.016
Brinker, S. T., Preiswerk, F., White, P. J., Mariano, T. Y., McDannold, N. J., and Bubrick, E. J. (2020). Focused ultrasound platform for investigating therapeutic neuromodulation across the human hippocampus. Ultrasound Med. Biol. 46, 1270–1274. doi: 10.1016/j.ultrasmedbio.2020.01.007
Bystritsky, A., Korb, A. S., Douglas, P. K., Cohen, M. S., Melega, W. P., Mulgaonkar, A. P., et al. (2011). A review of low-intensity focused ultrasound pulsation. Brain Stimul. 4, 125–136. doi: 10.1016/j.brs.2011.03.007
Chen, S. G., Tsai, C. H., Lin, C. J., Lee, C. C., Yu, H. Y., Hsieh, T. H., et al. (2020). Transcranial focused ultrasound pulsation suppresses pentylenetetrazol induced epilepsy in vivo. Brain Stimul. 13, 35–46. doi: 10.1016/j.brs.2019.09.011
Dallapiazza, R. F., Timbie, K. F., Holmberg, S., Gatesman, J., Lopes, M. B., Price, R. J., et al. (2018). Noninvasive neuromodulation and thalamic mapping with low-intensity focused ultrasound. J. Neurosurg. 128, 875–884. doi: 10.3171/2016.11.jns16976
Darrow, D. P., O’Brien, P., Richner, T. J., Netoff, T. I., and Ebbini, E. S. (2019). Reversible neuroinhibition by focused ultrasound is mediated by a thermal mechanism. Brain Stimul. 12, 1439–1447. doi: 10.1016/j.brs.2019.07.015
Fini, M., and Tyler, W. J. (2017). Transcranial focused ultrasound: a new tool for non-invasive neuromodulation. Int. Rev. Psychiatry 29, 168–177. doi: 10.1080/09540261.2017.1302924
Fisher, J. A. N., and Gumenchuk, I. (2018). Low-intensity focused ultrasound alters the latency and spatial patterns of sensory-evoked cortical responses in vivo. J. Neural Eng. 15:e035004. doi: 10.1088/1741-2552/aaaee1
Folloni, D., Verhagen, L., Mars, R. B., Fouragnan, E., Constans, C., Aubry, J. F., et al. (2019). Manipulation of subcortical and deep cortical activity in the primate brain using transcranial focused ultrasound stimulation. Neuron 101, 1109.e5–1116.e5. doi: 10.1016/j.neuron.2019.01.019
Fomenko, A., Chen, K. S., Nankoo, J. F., Saravanamuttu, J., Wang, Y., El-Baba, M., et al. (2020). Systematic examination of low-intensity ultrasound parameters on human motor cortex excitability and behavior. eLife 9:e54497. doi: 10.7554/eLife.54497
Fouragnan, E. F., Chau, B. K. H., Folloni, D., Kolling, N., Verhagen, L., Klein-Flugge, M., et al. (2019). The macaque anterior cingulate cortex translates counterfactual choice value into actual behavioral change. Nat. Neurosci. 22, 797–808. doi: 10.1038/s41593-019-0375-6
Gaur, P., Casey, K. M., Kubanek, J., Li, N., Mohammadjavadi, M., Saenz, Y., et al. (2020). Histologic safety of transcranial focused ultrasound neuromodulation and magnetic resonance acoustic radiation force imaging in rhesus macaques and sheep. Brain Stimul. 13, 804–814. doi: 10.1016/j.brs.2020.02.017
Gibson, B. C., Sanguinetti, J. L., Badran, B. W., Yu, A. B., Klein, E. P., Abbott, C. C., et al. (2018). Increased excitability induced in the primary motor cortex by transcranial ultrasound stimulation. Front. Neurol. 9:1007. doi: 10.3389/fneur.2018.01007
Hameroff, S., Trakas, M., Duffield, C., Annabi, E., Gerace, M. B., Boyle, P., et al. (2013). Transcranial ultrasound (TUS) effects on mental states: a pilot study. Brain Stimul. 6, 409–415. doi: 10.1016/j.brs.2012.05.002
Hoy, K. E., and Fitzgerald, P. B. (2010). Brain stimulation in psychiatry and its effects on cognition. Nat. Rev. Neurol. 6, 267–275. doi: 10.1038/nrneurol.2010.30
Huang, X., Lin, Z., Meng, L., Wang, K., Liu, X., Zhou, W., et al. (2019). Non-invasive low-intensity pulsed ultrasound modulates primary cilia of rat hippocampal neurons. Ultrasound Med. Biol. 45, 1274–1283. doi: 10.1016/j.ultrasmedbio.2018.12.012
Jerusalem, A., Al-Rekabi, Z., Chen, H., Ercole, A., Malboubi, M., Tamayo-Elizalde, M., et al. (2019). Electrophysiological-mechanical coupling in the neuronal membrane and its role in ultrasound neuromodulation and general anaesthesia. Acta Biomater. 97, 116–140. doi: 10.1016/j.actbio.2019.07.041
Kim, H., Chiu, A., Lee, S. D., Fischer, K., and Yoo, S. S. (2014). Focused ultrasound-mediated non-invasive brain stimulation: examination of sonication parameters. Brain Stimul. 7, 748–756. doi: 10.1016/j.brs.2014.06.011
Kim, H., Park, M. Y., Lee, S. D., Lee, W., Chiu, A., and Yoo, S. S. (2015). Suppression of EEG visual-evoked potentials in rats through neuromodulatory focused ultrasound. Neuroreport 26, 211–215. doi: 10.1097/wnr.0000000000000330
Krishna, V., Sammartino, F., and Rezai, A. (2018). A review of the current therapies, challenges, and future directions of transcranial focused ultrasound technology: advances in diagnosis and treatment. JAMA Neurol. 75, 246–254. doi: 10.1001/jamaneurol.2017.3129
Kubanek, J. (2018). Neuromodulation with transcranial focused ultrasound. Neurosurg. Focus 44:E14. doi: 10.3171/2017.11.focus17621
Kubanek, J., Shi, J., Marsh, J., Chen, D., Deng, C., and Cui, J. (2016). Ultrasound modulates ion channel currents. Sci. Rep. 6:24170. doi: 10.1038/srep24170
Lee, W., Kim, H. C., Jung, Y., Chung, Y. A., Song, I. U., Lee, J. H., et al. (2016b). Transcranial focused ultrasound stimulation of human primary visual cortex. Sci. Rep. 6:34026. doi: 10.1038/srep34026
Lee, W., Chung, Y. A., Jung, Y., Song, I. U., and Yoo, S. S. (2016a). Simultaneous stimulation of the human primary and secondary somatosensory cortices using transcranial focused ultrasound. J. Ther. Ultras. 4:31. doi: 10.1186/s40349-016-0076-5
Lee, W., Croce, P., Margolin, R. W., Cammalleri, A., Yoon, K., and Yoo, S. S. (2018). Transcranial focused ultrasound stimulation of motor cortical areas in freely-moving awake rats. BMC Neurosci. 19:57. doi: 10.1186/s12868-018-0459-3
Lee, W., Kim, H., Jung, Y., Song, I. U., Chung, Y. A., and Yoo, S. S. (2015). Image-guided transcranial focused ultrasound stimulates human primary somatosensory cortex. Sci. Rep. 5:8743. doi: 10.1038/srep08743
Legon, W., Adams, S., Bansal, P., Patel, P. D., Hobbs, L., Ai, L., et al. (2020). A retrospective qualitative report of symptoms and safety from transcranial focused ultrasound for neuromodulation in humans. Sci. Rep. 10:5573. doi: 10.1038/s41598-020-62265-8
Legon, W., Bansal, P., Tyshynsky, R., Ai, L., and Mueller, J. K. (2018b). Transcranial focused ultrasound neuromodulation of the human primary motor cortex. Sci. Rep. 8:10007. doi: 10.1038/s41598-018-28320-1
Legon, W., Ai, L., Bansal, P., and Mueller, J. K. (2018a). Neuromodulation with single-element transcranial focused ultrasound in human thalamus. Hum. Brain Mapp. 39, 1995–2006. doi: 10.1002/hbm.23981
Legon, W., Sato, T. F., Opitz, A., Mueller, J., Barbour, A., Williams, A., et al. (2014). Transcranial focused ultrasound modulates the activity of primary somatosensory cortex in humans. Nat. Neurosci. 17, 322–329. doi: 10.1038/nn.3620
Leo, A., Mueller, J. K., Grant, A., Eryaman, Y., and Wynn, L. (2016). Transcranial focused ultrasound for BOLD fMRI signal modulation in humans. IEEE Eng. Med. Biol. Soc. 2016, 1758–1761. doi: 10.1109/EMBC.2016.7591057
Li, G., Qiu, W., Zhang, Z., Jiang, Q., Su, M., Cai, R., et al. (2019). Noninvasive ultrasonic neuromodulation in freely moving mice. IEEE Trans. Biomed. Eng. 66, 217–224. doi: 10.1109/TBME.2018.2821201
Liang, D., Chen, J., Zhou, W., Chen, J., Chen, W., and Wang, Y. (2020). Alleviation effects and mechanisms of low-intensity focused ultrasound on pain triggered by soft tissue injury. J. Ultrasound Med. 39, 997–1005. doi: 10.1002/jum.15185
Lin, Z., Meng, L., Zou, J., Zhou, W., Huang, X., Xue, S., et al. (2020). Non-invasive ultrasonic neuromodulation of neuronal excitability for treatment of epilepsy. Theranostics 10, 5514–5526. doi: 10.7150/thno.40520
Liu, C., Yu, K., Niu, X., and He, B. (2021). Transcranial focused ultrasound enhances sensory discrimination capability through somatosensory cortical excitation. Ultras. Med. Biol. 47, 1356–1366. doi: 10.1016/j.ultrasmedbio.2021.01.025
Mehic, E., Xu, J. M., Caler, C. J., Coulson, N. K., Moritz, C. T., and Mourad, P. D. (2014). Increased anatomical specificity of neuromodulation via modulated focused ultrasound. PLoS One 9:e86939. doi: 10.1371/journal.pone.0086939
Min, B. K., Bystritsky, A., Jung, K. I., Fischer, K., Zhang, Y., Maeng, L. S., et al. (2011). Focused ultrasound-mediated suppression of chemically-induced acute epileptic EEG activity. BMC Neurosci. 12:23. doi: 10.1186/1471-2202-12-23
Mohammadjavadi, M., Ye, P. P., Xia, A., Brown, J., Popelka, G., and Pauly, K. B. (2019). Elimination of peripheral auditory pathway activation does not affect motor responses from ultrasound neuromodulation. Brain Stimul. 12, 901–910. doi: 10.1016/j.brs.2019.03.005
Monti, M. M., Schnakers, C., Korb, A. S., Bystritsky, A., and Vespa, P. M. (2016). Non-invasive ultrasonic thalamic stimulation in disorders of consciousness after severe brain injury: a first-in-man report. Brain Stimul. 9, 940–941. doi: 10.1016/j.brs.2016.07.008
Moore, M. E., Loft, J. M., Clegern, W. C., and Wisor, J. P. (2015). Manipulating neuronal activity in the mouse brain with ultrasound: a comparison with optogenetic activation of the cerebral cortex. Neurosci. Lett. 604, 183–187. doi: 10.1016/j.neulet.2015.07.024
Mueller, J., Legon, W., Opitz, A., Sato, T. F., and Tyler, W. J. (2014). Transcranial focused ultrasound modulates intrinsic and evoked EEG dynamics. Brain Stimul. 7, 900–908. doi: 10.1016/j.brs.2014.08.008
Pang, N., Huang, X., Zhou, H., Xia, X., Liu, X., Wang, Y., et al. (2021). Transcranial ultrasound stimulation of hypothalamus in aging mice. IEEE Trans. Ultrason. Ferroelectr. Freq. Control 68, 29–37. doi: 10.1109/TUFFC.2020.2968479
Rahimpour, S., Kiyani, M., Hodges, S. E., and Turner, D. A. (2021). Deep brain stimulation and electromagnetic interference. Clin. Neurol. Neurosurg. 203:106577. doi: 10.1016/j.clineuro.2021.106577
Romanella, S. M., Sprugnoli, G., Ruffini, G., Seyedmadani, K., Rossi, S., and Santarnecchi, E. (2020). Noninvasive brain stimulation & space exploration: opportunities and challenges. Neurosci. Biobehav. Rev. 119, 294–319. doi: 10.1016/j.neubiorev.2020.09.005
Sanguinetti, J. L., Hameroff, S., Smith, E. E., Sato, T., Daft, C. M. W., Tyler, W. J., et al. (2020). Transcranial focused ultrasound to the right prefrontal cortex improves mood and alters functional connectivity in humans. Front. Hum. Neurosci. 14:52. doi: 10.3389/fnhum.2020.00052
Verhagen, L., Gallea, C., Folloni, D., Constans, C., Jensen, D. E., Ahnine, H., et al. (2019). Offline impact of transcranial focused ultrasound on cortical activation in primates. eLife 8:e40541. doi: 10.7554/eLife.40541
Wang, X., Zhang, Y., Zhang, K., and Yuan, Y. (2021). Influence of behavioral state on the neuromodulatory effect of low-intensity transcranial ultrasound stimulation on hippocampal CA1 in mouse. Neuroimage 241:118441. doi: 10.1016/j.neuroimage.2021.118441
Wang, Y., Zhan, G., Cai, Z., Jiao, B., Zhao, Y., Li, S., et al. (2021). Vagus nerve stimulation in brain diseases: therapeutic applications and biological mechanisms. Neurosci. Biobehav. Rev. 127, 37–53. doi: 10.1016/j.neubiorev.2021.04.018
Wattiez, N., Constans, C., Deffieux, T., Daye, P. M., Tanter, M., Aubry, J. F., et al. (2017). Transcranial ultrasonic stimulation modulates single-neuron discharge in macaques performing an antisaccade task. Brain Stimul. 10, 1024–1031. doi: 10.1016/j.brs.2017.07.007
Xu, T., Lu, X., Peng, D., Wang, G., Chen, C., Liu, W., et al. (2020). Ultrasonic stimulation of the brain to enhance the release of dopamine - A potential novel treatment for Parkinson’s disease. Ultrason. Sonochem. 63:104955. doi: 10.1016/j.ultsonch.2019.104955
Yang, H., Yuan, Y., Wang, X., and Li, X. (2020). Closed-loop transcranial ultrasound stimulation for real-time non-invasive neuromodulation in vivo. Front. Neurosci. 14:445. doi: 10.3389/fnins.2020.00445
Yang, P. F., Phipps, M. A., Jonathan, S., Newton, A. T., Byun, N., Gore, J. C., et al. (2021). Bidirectional and state-dependent modulation of brain activity by transcranial focused ultrasound in non-human primates. Brain Stimul. 14, 261–272. doi: 10.1016/j.brs.2021.01.006
Yoo, S. S., Kim, H., Min, B. K., Franck, E., and Park, S. (2011). Transcranial focused ultrasound to the thalamus alters anesthesia time in rats. Neuroreport 22, 783–787. doi: 10.1097/WNR.0b013e32834b2957
Yoon, K., Lee, W., Lee, J. E., Xu, L., Croce, P., Foley, L., et al. (2019). Effects of sonication parameters on transcranial focused ultrasound brain stimulation in an ovine model. PLoS One 14:e0224311. doi: 10.1371/journal.pone.0224311
Yu, K., Liu, C., Niu, X., and He, B. (2020). Transcranial focused ultrasound neuromodulation of voluntary movement-related cortical activity in humans. IEEE Trans. BioMed. Eng. 68, 1923–1931. doi: 10.1109/TBME.2020.3030892
Yu, K., Sohrabpour, A., and He, B. (2016). Electrophysiological source imaging of brain networks perturbed by low-intensity transcranial focused ultrasound. IEEE Trans. Biomed. Eng. 63, 1787–1794. doi: 10.1109/tbme.2016.2591924
Yuan, Y., Wang, Z., Liu, M., and Shoham, S. (2020). Cortical hemodynamic responses induced by low-intensity transcranial ultrasound stimulation of mouse cortex. Neuroimage 211:116597. doi: 10.1016/j.neuroimage.2020.116597
Keywords: transcranial focused ultrasound, neuromodulation, non-invasive brain stimulation, human, animal
Citation: Zhang T, Pan N, Wang Y, Liu C and Hu S (2021) Transcranial Focused Ultrasound Neuromodulation: A Review of the Excitatory and Inhibitory Effects on Brain Activity in Human and Animals. Front. Hum. Neurosci. 15:749162. doi: 10.3389/fnhum.2021.749162
Received: 29 July 2021; Accepted: 06 September 2021;
Published: 28 September 2021.
Edited by:
Jiaojian Wang, University of Electronic Science and Technology of China, ChinaReviewed by:
Xiaojing Long, Shenzhen Institutes of Advanced Technology, Chinese Academy of Sciences (CAS), ChinaCopyright © 2021 Zhang, Pan, Wang, Liu and Hu. This is an open-access article distributed under the terms of the Creative Commons Attribution License (CC BY). The use, distribution or reproduction in other forums is permitted, provided the original author(s) and the copyright owner(s) are credited and that the original publication in this journal is cited, in accordance with accepted academic practice. No use, distribution or reproduction is permitted which does not comply with these terms.
*Correspondence: Shimin Hu, NTgzNTM0MDM1QHFxLmNvbQ==; Chunyan Liu, bGN5X2VfbWFpbEAxNjMuY29t
Disclaimer: All claims expressed in this article are solely those of the authors and do not necessarily represent those of their affiliated organizations, or those of the publisher, the editors and the reviewers. Any product that may be evaluated in this article or claim that may be made by its manufacturer is not guaranteed or endorsed by the publisher.
Research integrity at Frontiers
Learn more about the work of our research integrity team to safeguard the quality of each article we publish.