- 1Department of Gastroenterology, University Hospitals of North Midlands NHS Trust, Stoke on Trent, United Kingdom
- 2Department of Neurology, Epilepsy Center Frankfurt Rhine-Main, Goethe-University Frankfurt, Frankfurt am Main, Germany
- 3Scientific Institute, IRCCS E. Medea, Pasian di Prato, Italy
- 4Department of Neuroscience, Physiology and Pharmacology, Centre for Cardiovascular and Metabolic Neuroscience, University College London, London, United Kingdom
- 5Institute for Neuromodulation and Neurotechnology, University Hospital and University of Tuebingen, Tuebingen, Germany
- 6Department of Psychiatry, Psychotherapy and Psychosomatics, Medical Faculty, University of Augsburg, Augsburg, Germany
- 7Department of Psychiatry and Psychotherapy, University Hospital, LMU Munich, Munich, Germany
- 8Laboratory for Biological Psychology, Faculty of Psychology and Educational Sciences, University of Leuven, Leuven, Belgium
- 9Leibniz Institute for Neurobiology, Magdeburg, Germany
- 10Department of Neurology, Institute for Neuroscience, 4Brain, Ghent University Hospital, Gent, Belgium
- 11Sheffield Institute for Translational Neuroscience (SITraN), University of Sheffield, Sheffield, United Kingdom
- 12Clinical Psychology and the Leiden Institute of Brain and Cognition, Leiden University, Leiden, Netherlands
- 13Department of Psychiatry, Medical University of South Carolina, Charleston, SC, United States
- 14Department of Biological Psychology and Affective Science, Faculty of Human Sciences, University of Potsdam, Potsdam, Germany
- 15Migraine and Headache Clinic Koenigstein, Königstein im Taunus, Germany
- 16Cognitive Neurophysiology, Department of Child and Adolescent Psychiatry, Faculty of Medicine, TU Dresden, Dresden, Germany
- 17Utah State University, Logan, UT, United States
- 18NORMENT, Division of Mental Health and Addiction, University of Oslo and Oslo University Hospital, Oslo, Norway
- 19Department of Psychology, University of Oslo, Oslo, Norway
- 20KG Jebsen Centre for Neurodevelopmental Disorders, University of Oslo, Oslo, Norway
- 21Medical Faculty, Institute of Cognitive Neurology and Dementia Research, Otto-von-Guericke University, Magdeburg, Germany
- 22Institute of Cognitive Neuroscience, University College London, London, United Kingdom
- 23Center for Behavioral Brain Sciences Magdeburg (CBBS), Otto-von-Guericke University, Magdeburg, Germany
- 24Department of Pediatric Neuroscience, Fondazione IRCCS Istituto Neurologico Carlo Besta, Milan, Italy
- 25Pain and Integrative Neuroscience Branch, National Center for Complementary and Integrative Health, NIH, Bethesda, MD, United States
- 26Department of Internal Medicine, Fondazione IRCCS Ca' Granda, Ospedale Maggiore Policlinico, Milan, Italy
- 27Department of Clinical Sciences and Community Health, University of Milan, Milan, Italy
- 28Institute of Electrodynamics, Microwave and Circuit Engineering, TU Wien, Vienna, Austria
- 29SzeleSTIM GmbH, Vienna, Austria
- 30Unit of Neurology, Neurophysiology, Neurobiology, Department of Medicine, Università Campus Bio-Medico di Roma, Rome, Italy
- 31Faculty of Biology and Faculty of Optics, Complutense University of Madrid and Institute for Health Research, San Carlos Clinical Hospital (IdISSC), Madrid, Spain
- 32Translational Medicine and Therapeutics, Barts and The London School of Medicine and Dentistry, William Harvey Research Institute, Queen Mary University of London, London, United Kingdom
- 33Department of Pharmaceutical Sciences, School of Biosciences and Biotechnology, Babasaheb Bhimrao Ambedkar University (A Central University), Lucknow, India
- 34Department of Biological Psychology, Clinical Psychology and Psychotherapy, University of Würzburg, Würzburg, Germany
- 35Division of Nuclear Medicine and Molecular Imaging, Department of Radiology, Massachusetts General Hospital and Harvard Medical School, Boston, MA, United States
- 36Faculty of Health, Medicine and Life Sciences, School for Mental Health and Neuroscience, Alzheimer Centre Limburg, Maastricht University, Maastricht, Netherlands
- 37Research Group Health Psychology, Faculty of Psychology and Educational Sciences, University of Leuven, Leuven, Belgium
- 38Headache Research Unit, Department of Neurology-Citadelle Hospital, University of Liège, Liège, Belgium
- 39Functional Imaging Lab, Department of Radiology, Guang An Men Hospital, China Academy of Chinese Medical Sciences, Beijing, China
- 40School of Biomedical Science, Faculty of Biological Science, University of Leeds, Leeds, United Kingdom
- 41Division for Vascular Surgery, Department of Surgery, Medical University of Vienna, Vienna, Austria
- 42Department of Psychological Science, University of California, Irvine, Irvine, CA, United States
- 43Institute for Cognitive Neurology and Dementia Research, Otto-von-Guericke-University Magdeburg, Magdeburg, Germany
- 44Neuromodulatory Networks, Leibniz Institute for Neurobiology, Magdeburg, Germany
- 45Clinical and Cognitive Psychology and the Leiden Institute of Brain and Cognition, Leiden University, Leiden, Netherlands
- 46NeuroV̇ASQ̇ - Integrative Physiology Laboratory, Faculty of Physical Education, University of Brasilia, Brasilia, Brazil
- 47Department of Anatomy, Faculty of Medicine, Mersin University, Mersin, Turkey
- 48Mental Health and Wellbeing Research Group, Vrije Universiteit Brussel, Brussels, Belgium
- 49Faculty of Health Care, University College Odisee, Aalst, Belgium
- 50Division of Epileptology, Fondazione IRCCS Istituto Neurologico C. Besta, Milan, Italy
- 51Department of Neurosurgery, University of Tübingen, Tübingen, Germany
- 52Department of Biomedical Engineering, City College of New York, New York, NY, United States
- 53Department of Psychology, Education and Child Studies, Erasmus University Rotterdam, Rotterdam, Netherlands
- 54Faculty of Health Sciences Brandenburg, University of Potsdam, Potsdam, Germany
- 55Deutsches Zentrum für Neurodegenerative Erkrankungen (DZNE), Magdeburg, Germany
- 56Center for Behavioral Brain Sciences, Otto-von-Guericke University, Magdeburg, Germany
- 57University Hospital of Child and Adolescent Psychiatry and Psychotherapy, University of Bern, Bern, Switzerland
- 58Section for Translational Psychobiology in Child and Adolescent Psychiatry, Department of Child and Adolescent Psychiatry, Centre for Psychosocial Medicine, University of Heidelberg, Heidelberg, Germany
- 59Division of Endocrinology and Diabetology, Medical Faculty, Heinrich-Heine University Düsseldorf, Düsseldorf, Germany
- 60Institute for Clinical Diabetology, German Diabetes Center, Leibniz Center for Diabetes Research at Heinrich Heine University, Düsseldorf, Germany
- 61German Center for Diabetes Research, Munich, Germany
- 62Department of Psychology, College of Liberal Arts, University of Minnesota, Minneapolis, MN, United States
- 63Department of Anesthesiology, Center for Pain Medicine, University of California, San Diego Health System, La Jolla, CA, United States
- 64Department of Psychiatry and Psychotherapy, University of Göttingen, Göttingen, Germany
- 65Laboratory of Systems Neuroscience and Imaging in Psychiatry (SNIPLab), University of Göttingen, Göttingen, Germany
- 66Department of Psychiatry and Psychotherapy, University of Tübingen, Tübingen, Germany
- 67Institute of Acupuncture and Moxibustion, China Academy of Chinese Medical Sciences, Beijing, China
- 68Department of Psychology, University of Greifswald, Greifswald, Germany
- 69Department of Psychiatry, University of Pittsburgh School of Medicine, UPMC Western Psychiatric Hospital, Pittsburgh, PA, United States
- 70Department of Radiology, Athinoula A. Martinos Center for Biomedical Imaging, Massachusetts General Hospital, Charlestown, MA, United States
- 71Department of Radiology, Logan University, Chesterfield, MO, United States
- 72Cognitive Psychology Unit, Institute of Psychology, Leiden University, Leiden, Netherlands
- 73Leiden Institute for Brain and Cognition, Leiden, Netherlands
- 74Department of Developmental Psychology and Socialisation, University of Padova, Padova, Italy
- 75Athinoula A. Martinos Center for Biomedical Imaging, Department of Radiology, Massachusetts General Hospital, Harvard Medical School, Charlestown, MA, United States
- 76Department of Psychiatry, Massachusetts General Hospital, Harvard Medical School, Boston, MA, United States
- 77Heart Rhythm Institute, University of Oklahoma Health Sciences Center, Oklahoma City, OK, United States
- 78Faculty of Biological Science, School of Biomedical Science, University of Leeds, Leeds, United Kingdom
- 79Department of Surgery, University Hospital Bonn, Bonn, Germany
- 80Department of Performance Psychology, Institute of Psychology, Deutsche Sporthochschule, Köln, Germany
- 81Department of Anesthesiology, University Medicine Greifswald, Greifswald, Germany
- 82Department of Anesthesia, McMaster University, Hamilton, ON, Canada
- 83Laboratory of Functional Neurovascular Diagnostics, AG Early Diagnosis of Dementia, Department of Psychiatry, Psychosomatics and Psychotherapy, University Clinic Würzburg, Würzburg, Germany
- 84Department of Neurology, Otto-von-Guericke University, Magdeburg, Germany
- 85Department of Social and Health Psychology, Institute of Psychology, Deutsche Sporthochschule, Köln, Germany
- 86Department of Epidemiology and Public Health, Faculty of Medicine, University of Ostrava, Ostrava, Czechia
- 87Department of Human Movement Studies, Faculty of Education, University of Ostrava, Ostrava, Czechia
- 88Section for Experimental Child and Adolescent Psychiatry, Department of Child and Adolescent Psychiatry, Centre for Psychosocial Medicine, University of Heidelberg, Heidelberg, Germany
Given its non-invasive nature, there is increasing interest in the use of transcutaneous vagus nerve stimulation (tVNS) across basic, translational and clinical research. Contemporaneously, tVNS can be achieved by stimulating either the auricular branch or the cervical bundle of the vagus nerve, referred to as transcutaneous auricular vagus nerve stimulation(VNS) and transcutaneous cervical VNS, respectively. In order to advance the field in a systematic manner, studies using these technologies need to adequately report sufficient methodological detail to enable comparison of results between studies, replication of studies, as well as enhancing study participant safety. We systematically reviewed the existing tVNS literature to evaluate current reporting practices. Based on this review, and consensus among participating authors, we propose a set of minimal reporting items to guide future tVNS studies. The suggested items address specific technical aspects of the device and stimulation parameters. We also cover general recommendations including inclusion and exclusion criteria for participants, outcome parameters and the detailed reporting of side effects. Furthermore, we review strategies used to identify the optimal stimulation parameters for a given research setting and summarize ongoing developments in animal research with potential implications for the application of tVNS in humans. Finally, we discuss the potential of tVNS in future research as well as the associated challenges across several disciplines in research and clinical practice.
Introduction
Brief History of Transcutaneous Vagus Nerve Stimulation
The vagus nerve (VN) is the Xth cranial nerve and the longest nerve, which courses from the brainstem to the distal third of the colon. It is the main neural substrate of the parasympathetic nervous system and is composed of afferent and efferent pathways, although the former predominate (80%) (Butt et al., 2020). As part of a complex network of neural structures that serves to maintain psychophysiological balance in the organism, its importance cannot be underestimated. The vagus “nerve” is actually two nerves, a left vagus and a right vagus, with slightly different neural origins and targets. It is composed of different types of fibers that vary in myelination, size, and conduction speed (e.g., for an excellent review on vagus nerve physiology see Yuan and Silberstein, 2016a,b). Three types of fibers have been identified, each with distinct physiological properties. In general, the larger the fiber, the faster the conduction speed. Myelinated A-fibers are composed of small and large fibers. The small fibers are visceral afferent fibers and the large are both afferent and efferent somatic fibers. Afferent and efferent preganglionic fibers are called B-fibers. Finally, ~70% of all vagal fibers are unmyelinated C-fibers and convey visceral information from the vast array of visceral organs. Acetylcholine is the primary neurotransmitter of the vagus nerve. It activates cholinergic receptors that are subdivided into nicotinic and muscarinic receptors. However, there is evidence of cross-talk between the vagus and sympathetic nerve fibers as evidenced by tyrosine hydroxylase in the thoracic and cervical trunks of the vagus. There are four vagal nuclei in the medulla, each with distinct but often overlapping targets. The nucleus ambiguus is the source of most cardiovagal motor neurons. The dorsal motor nucleus also contains some cardiovagal motor neurons but primarily innervates the subdiaphragmatic visceral organs. The nucleus of the solitary tract (NTS) is the major hub for afferent information. Finally, the spinal nucleus of the trigeminal nerve, via the superior jugular ganglion, transmits afferent and efferent impulses primarily from the head and vocal structures and has several branches including the auditory branch (Yuan and Silberstein, 2016a). Furthermore, the vagus nerve has projections to higher brain centers including the prefrontal cortex primarily via synaptic connections in the NTS (Thayer and Lane, 2009). In addition, there may be variation among species in the anatomy and physiology of the vagus requiring comparisons of studies across species to be done mindfully. An understanding of the complex anatomy and physiology of the vagus nerve is essential to an understanding of vagus nerve stimulation.
According to the reports of historians and archaeologists, clinical applications of auricular stimulation (broadly defined) were used across many ancient cultures. For instance, tactile stimulation of the auditory meatus was mentioned in some of the earliest known texts on Chinese medicine and acupuncture (Hou et al., 2015). Interestingly, therapeutic auricular stimulation was not confined to China, and was prevalent across many cultures. Thousands of years ago, the practice of cauterizing a portion of the auricle was common amongst certain tribes in Arabia, while in ancient Egypt, women pricked or cauterized the external auricle for contraceptive purposes and physicians in ancient Persia treated sciatic pain and sexually-related diseases by auricular cauterization (Hou et al., 2015). The Italian anatomist and surgeon Antonio Valsalva published his famous Tractatus de Aure Humana, where he described the treatment of toothache by scarification of the antitragus (Valsalva, 1704). In the last half of the twentieth century, the auricular acupuncture (i.e., needling of specific areas of external auricle) became popular in clinical medicine (Nogier, 1957). Based on Nogier's work in the German Journal of Acupuncture 1957, the Nanjing Army Ear Acupuncture Research Group from China further evaluated auricular somatotopy (Huang, 1974), and auricular acupuncture developed as a unique “microsystem” for acupuncture therapy. Currently, auricular acupuncture, which can mimic transcutaneous vagus nerve stimulation (tVNS), is reported in numerous systematic reviews to be effective in treatment of insomnia and relief of acute and chronic pain (Vieira et al., 2018). Ultimately, there is a great deal of overlap between acupuncture, particularly electroacupuncture, and neuromodulation therapies such as tVNS (Usichenko et al., 2017b), and the rich evidence base supporting auricular acupuncture should be better integrated to help inform further development of tVNS therapy (Napadow, 2019).
The origins of VN stimulation (VNS) date back in excess of 100 years. In the late eighteenth and early nineteenth century, it was believed that epilepsy was caused by excessive blood flow to the brain, termed venous hyperaemia, with patients frequently being treated by manual compression of the carotid arteries in the neck to suppress blood flow. In the late nineteenth century, American neurologist James L. Corning developed a “carotid fork”—a device to facilitate carotid compression, which was later augmented by stimulation electrodes. Corning intended to stimulate cervical branches of the VN, which course in close proximity to the carotid artery, in order to decrease heart rate (HR) and, subsequently, blood flow to the brain. Even though Corning reported treatment success, the method was not widely accepted at the time due to safety concerns and a lack of reproducibility of therapeutic response (Lanska, 2002).
Implantable VNS (iVNS) was developed by Jake Zabara in the 1980s as it was found to have promising antiepileptic effects in canine models (Zabara, 1985, 1992) and proceeded to become one of the earliest forms of neuromodulation in humans (Yuan and Silberstein, 2016a). Globally, by 2014 over 100,000 patients have had iVNS implanted (Johnson and Wilson, 2018; also see Chakravarthy et al., 2015). The first controlled clinical trials of iVNS as a treatment for refractory epilepsy were conducted in the early 1990s (Penry and Dean, 1990; Uthman et al., 1993) and reported substantial reductions in seizure frequency, even though a significant proportion of patients did not display a symptomatic improvement. Following a number of further clinical trials, iVNS, applied to the left cervical VN, was approved by the US Food and Drug Administration (FDA) for management of pharmacoresistant epilepsy in 1997 (Morris et al., 2013). In subsequent observational studies of patients with epilepsy, it was reported that patients' mood improved following iVNS treatment (Harden et al., 2000). These results spawned a series of studies in patients with depression which led, in 2005, to FDA approval of iVNS for the treatment of pharmacoresistant depression (Cristancho et al., 2011; Desbeaumes Jodoin et al., 2018). More recently, iVNS has been evaluated as treatment for a diverse array of disorders including heart failure (Ferrari et al., 2011; Wang et al., 2015a), rheumatoid arthritis (Koopman et al., 2016), inflammatory bowel disease (Levine et al., 2014), sepsis (Wang et al., 2016; Yang et al., 2017), and chronic pain (Lange et al., 2011).
iVNS necessitates a costly, invasive surgical procedure involving the implantation of a bipolar helical electrode to the left cervical VN which is subsequently attached to a pulse generator, most frequently positioned in a left infraclavicular subcutaneous pocket. Non-invasive tVNS approaches have been developed as a less expensive, patient friendly and rapidly deployable alternative. Transcutaneous cervical VNS (tcVNS) is conceptually similar to Corning's initial approach of transcutaneously stimulating the VN in the neck, adjacent to the carotid artery. tcVNS is FDA-approved for the treatment of migraine and cluster headache management and has been subjected to intensive research effort (Goadsby et al., 2014; Nesbitt et al., 2015).
The most widely commercially available tcVNS device (gammaCore®, electroCore, Inc.) is hand-held and delivers sinusoidal alternating current with a broadband amplitude-modulated frequency spectrum (Nesbitt et al., 2015). In the USA, the gammaCore® device received FDA approval for the treatment of acute cluster headache treatment in 2017, and for acute migraine treatment and adjunctive cluster headache prevention in 2018. Transcutaneous auricular VNS (taVNS) is under investigation for a wide range of clinical applications, however, is not FDA-cleared for the treatment of any disorder. The most widely used commercially available taVNS device (NEMOS®, tVNS technologies) delivers current in rhythmic square pulses (Yuan and Silberstein, 2016b). The NEMOS® device received European certification (CE certification, which indicates legal conformity and safety, but not necessarily clinical efficacy) as a treatment for epilepsy and depression in 2010, for chronic pain in 2012 and for anxiety in 2019. Importantly, given their non-invasiveness, tcVNS and taVNS are widely used not only for clinical purposes, but also in healthy populations for basic research in cognitive neuroscience and related fields (Yuan and Silberstein, 2016b). The increased availability of these devices, coupled with their user friendliness, has resulted in an increase in research publications on tVNS, see Figure 1.
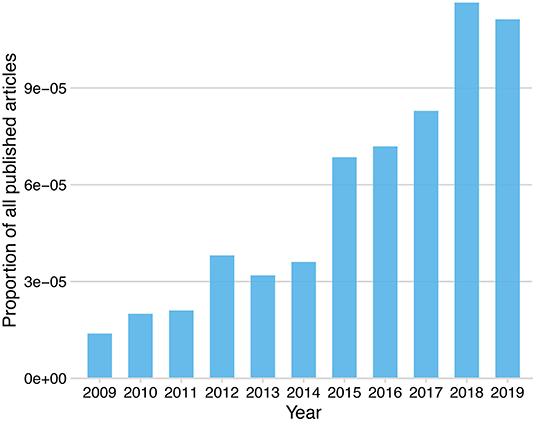
Figure 1. Proportion of published research articles including the keyword “transcutaneous vagus nerve stimulation” listed on PubMed by year.
An array of stimulation parameters needs to be considered when it comes to using tVNS in both research and clinical settings. Stimulation parameters of tVNS can vary in terms of its current intensity (mA), pulse width (μs), frequency (Hz), duty cycle (s), and session duration (min) (Badran et al., 2019). Furthermore, side effects of stimulation, type of sham or control stimulation, location of the stimulation and sham electrode placement may influence the outcomes of tVNS. The impact of each of these stimulation parameters on psychophysiology and on clinical outcomes is incompletely understood. Despite the increasing number of studies, there is no clear consensus regarding the optimal parameters that need to be adopted for tVNS research. Moreover, there is no clear consensus regarding the minimal standard reporting items within the tVNS literature. Recently, calls for full disclosure of tVNS stimulation parameters have been made (Redgrave et al., 2018; Burger et al., 2020a). Herein, we aim to provide multidisciplinary recommendations regarding standard reporting items for future tVNS research. These recommendations are based on a systematic review of existing tVNS studies, evaluation of current reporting practices and finally on a broad consensus among research groups studying tVNS.
VNS Nomenclature: Techniques and Targets
The following section reviews four currently accepted VNS modalities—(1) cervically implanted VNS (iVNS), (2) transcutaneous cervical VNS (tcVNS), (3) transcutaneous auricular VNS (taVNS) (4) percutaneous auricular VNS (paVNS). For simplicity, hereinafter, we will refer to both transcutaneous forms of VNS (taVNS and tcVNS) as tVNS.
Cervically Implanted VNS (iVNS)
Classically, the VN is stimulated via implanted electrodes targeting (mostly) the left cervical branch of the VN (Mertens et al., 2018; Kaniusas et al., 2019a). iVNS commonly uses a bipolar cuff electrode (e.g., VNS Therapy, LivaNova). Despite being well-established, this method remains expensive and is associated with peri and post implantation risks. Furthermore, the electrode implant is irreversible. Moreover, stimulation is not restricted to afferent fibers of the cervical VN—as usually targeted by the therapy—but extends to (visceral) efferent fibers of the VN as well (Howland, 2014). Consequently, several adverse effects such as cough, voice alteration, swallowing difficulties, or bradycardia have been reported (Liporace et al., 2001).
Transcutaneous Cervical VNS (tcVNS)
The cervical VN can also be stimulated transcutaneously by using two skin electrodes, e.g., by a hand-held device (e.g., GammaCore, electroCore, Inc.), which are applied at the neck (Barbanti et al., 2015; Gaul et al., 2016; Silberstein et al., 2016a; Frangos and Komisaruk, 2017). This form of transcutaneous stimulation is now FDA approved for the acute treatment of migraine and for the acute treatment and prevention of episodic cluster headache. However, despite its relative convenience, this method is not devoid of adverse effects. Given that tVNS requires the stimulation to pass through the skin barrier, relatively strong currents are needed. The resulting stimulation fields in the neck are diffuse, so that cervical non-vagal nerves can be co-stimulated, as well as efferent cervical fibers. Commonly observed adverse effects for the cervical tVNS approach include prickling at the stimulation site, neck pain, dizziness, headache, nasopharyngitis, oropharyngeal pain and sensitivity to the conducting gel (Gaul et al., 2016; Redgrave et al., 2018). An MRI-derived Finite Element Method model was developed to analyze the cellular components activated with tcVNS. Due to the different types of tissue between the surface electrodes on the skin and the VN, both macroscopic (skin, muscle, fat) and mesoscopic (nerve sheath, cerebrospinal fluid) components were used to predict activation thresholds and electric field changes. It was demonstrated that the overall current requirement to achieve adequate stimulation is influenced by deeper tissues and that tissue conductivity has a direct effect on axon membrane polarization. This model predicts that tcVNS will activate A and B axon fibers, but not C fibers (Mourdoukoutas et al., 2018).
Transcutaneous Auricular VNS (taVNS)
The auricular branch of the VN is primarily an afferent fiber which innervates the ear and joins the main bundle of the VN projecting to the nucleus tractus solitarius (NTS). taVNS is achieved via surface skin electrodes applied in the vagally-innervated ear regions (Ellrich, 2011) on the outer ear (Ellrich, 2011; Frangos et al., 2015; Straube et al., 2015; Badran et al., 2018a,b). Typically, taVNS uses two surface electrodes (e.g., NEMOS, tVNS Technologies GmbH). This method is CE marked (but not FDA approved) for epilepsy, depression, anxiety, pain, and migraine. A relatively large surface of electrodes yields diffuse stimulation fields, so that not only vagal but also non-vagal auricular nerves can be recruited, the implications of which remain controversial (Kaniusas et al., 2019a). The stimulation is considered safe (Badran et al., 2018c). As in the case of the percutaneous tcVNS, the expected side effects are mostly minor and may include headache, pain and skin irritation at the stimulation site, and dizziness (Mertens et al., 2018). Researchers are still working to determine optimal ear targeting approaches as there is paucity of data comprehensively describing the innervation of the ear. An anatomical dissection of the human auricle describes how the auricular branch of the VN diffusely innervates the ear (Peuker and Filler, 2002), with the cymba concha region being exclusively innervated by the auricular branch of the VN, along with other areas such as the posterior and inferior walls of the ear canal. Many of these targets are hypothesized to be regions for engagement of vagal afferents (Badran et al., 2018a; Burger and Verkuil, 2018). Various ear targets, practical procedures and electrode placement techniques for taVNS in the laboratory or clinical setting have been outlined along with stimulation parameter considerations (Badran et al., 2019; Sclocco et al., 2019, 2020).
Percutaneous Auricular VNS (paVNS)
A minimally invasive form of paVNS (Kampusch et al., 2013) can be performed with miniature needle electrodes penetrating the skin in the targeted outer ear regions innervated mainly by the auricular branch of the VN (Sator-Katzenschlager et al., 2004; Kaniusas et al., 2019b). This so-called paVNS typically uses 2–3 needle electrodes (e.g., AuriStim, DyAnsys). The small size of needle electrodes and the resulting spatially focused stimulating fields favors precise and specific stimulation of the local afferent auricular branch VN endings. Minor side effects of paVNS are local skin irritation (dermatitis), local bleeding, pain at the stimulation site, and dizziness.
Thus, the term tVNS is a broadly encompassing term and is not location-specific, i.e., neck or ear, as both tcVNS and taVNS may have similar biological effects. It is important to note there is limited data on the head-to-head testing of tcVNS with taVNS and these studies should be explored.
In the following sections, we will focus on tVNS, given that these techniques have been subject to intensive study. The key difference between taVNS and tcVNS is the branch of the VN which is putatively targeted. taVNS targets the auricular branch of the VN, a subsidiary from both left and right VN main bundles that innervates the ear on the same side (Peuker and Filler, 2002). In contrast, tcVNS targets the cervical branch of the VN (Barbanti et al., 2015; Gaul et al., 2016; Silberstein et al., 2016b; Frangos and Komisaruk, 2017). It remains unclear whether neck and ear stimulation produce similar biological and end organ effects.
tVNS can also be associated with indirect sporadic effects, due to rare afferent-efferent vagal reflexes via the NTS. For instance, tVNS may sometimes cause a reflexive cough, colloquially known as Arnold's ear-cough reflex. Other vegetative reflexes, such as the ear-gag reflex, ear-lacrimation reflex, ear-syncope reflex, and vaso-vagal reflex can also be observed, albeit relatively rarely (Tekdemir et al., 1998; Ellrich, 2011; Napadow et al., 2012). Overall, tVNS is associated with fewer side effects in comparison to iVNS, which has the potential to be associated with increased tolerability. In addition, portable devices are relatively easy to handle and are more cost effective than implantable devices (Morris et al., 2016).
Modes of Application
Long-Term Stimulation in Clinical Trials and Intervention Studies
Epilepsy
The effect of tVNS on pharmacoresistant epilepsy has been investigated in several studies. An early pilot study (Stefan et al., 2012) demonstrated that seizure frequency was reduced in five out of seven patients after 9 months of tVNS therapy, and that tVNS was well-tolerated. This reduction was also observable in a larger sample size over a 12 months period (Aihua et al., 2014). Another 6 months pilot study (He et al., 2013a) demonstrated seizure frequency reductions in 9 out of 14 children. A more recent 20-week placebo-controlled clinical trial of 76 patients with epilepsy (Bauer et al., 2016) reported that tVNS decreased seizure frequency. However, only about half of the patients were classified as responders—defined as seizure frequency reduction >25%. In a more recent prospective study of 20 patients (Barbella et al., 2018), only one third derived clinical benefit from tVNS. In a randomized clinical trial of 47 patients with epilepsy, Rong et al. reported that after 24 weeks of daily treatment 16% were seizure free and 38% had reduced seizure frequency (Rong et al., 2014). A larger-scale clinical trial of tVNS in epilepsy is pending, as the evidence regarding efficacy is currently insufficient for routine clinical care (Boon et al., 2018). Although the mechanisms of VNS in epilepsy are not fully understood, it is suggested that the nuclei of the brainstem are involved. The NTS has direct or indirect projections toward the locus coeruleus (LC) and raphe nuclei, which are suggested to be associated with seizures through noradrenergic and serotonergic neurons, exerting an antiepileptic effect. In particular, antiepileptic effects have been associated with an increase in norepinephrine (Krahl and Clark, 2012; Panebianco et al., 2016). Another theory suggests that VNS can activate inhibitory structures in the brain, with an increase in free gamma-aminobutyric acid (GABA) levels in cerebrospinal fluid and GABA-A receptor density in the hippocampus of patients who responded favorably (Marrosu et al., 2003). In recent years, the idea has grown that inflammation is involved in the development of seizures and epilepsy and, therefore, activation of anti-inflammatory pathways through VNS could explain antiepileptic effects (Krahl and Clark, 2012; Bonaz et al., 2013; Panebianco et al., 2016). Early work in rats indicated that the recruitment of vagal C-fibers is necessary for the suppression of seizures, by activating the C-fibers of the Vagal nerve (Woodbury and Woodbury, 1990), mediating GABA and glycine levels.
Depression
A placebo-controlled pilot study of patients with depression (Hein et al., 2013) found that 2 weeks of tVNS decreased depression severity using validated measures. This finding was replicated later in a larger patient sample, although this non-randomized study identified only about one third of the patients enrolled as tVNS responders (Rong et al., 2016). Neuroimaging studies in mild to moderately depressed patients have demonstrated that tVNS altered functional brain connectivity in the default mode network (Fang et al., 2016; Liu et al., 2016) and led to insula activation that was correlated with the clinical effectiveness of tVNS treatment (Fang et al., 2017). Furthermore, a decrease in functional connectivity between the bilateral medial hypothalamus and rostral anterior cingulate cortex (rACC) (Tu et al., 2018), as well as an increase of functional connectivity between the left nucleus accumbens and bilateral rACC (Wang et al., 2018) during 4 weeks of tVNS treatment were reported. Another potential mechanism, by which tVNS may exert an antidepressant action, is the modulation of inflammatory processes that are currently discussed (Rawat et al., 2019; also see Pavlov and Tracey, 2012). A previous review has summarized existing research on tVNS in depression in greater detail (Kong et al., 2018 also see Lv et al., 2019).
Tinnitus
A third clinical field in which several tVNS studies exist is tinnitus. One pilot study (Lehtimäki et al., 2013) found that 10 days of tVNS, combined with sound therapy, ameliorated patient-reported tinnitus severity and attenuated their auditory event-related field signal on magnetoencephalography (MEG). Another pilot study similarly observed a clinically meaningful amelioration of patient-reported tinnitus severity in four out of 10 patients after 20 days of combined tVNS and sound therapy (De Ridder et al., 2014). This has been replicated in a larger sample (30 patients), 15 of which were classified as responders to combined tVNS and sound therapy (Shim et al., 2015). However, a further pilot study administering tVNS (without sound therapy) for 6 months did not show any clinically meaningful effect (Kreuzer et al., 2014). It appears that the VNS generates improvements in patients with tinnitus due to the suppression of auditory, limbic and other areas of the brain involved in the generation / perception of tinnitus through the ascending auditory and vagal pathways (Yakunina et al., 2018). The rationale for the treatment of tinnitus using tVNS is build around the idea, that tVNS together with the presentation of tones can boost neuronal plasticity: the joint use of VNS and tones produces a reduction in the activity of the gamma band in the left auditory cortex, as well as the phase coherence between the cortex. Auditory and areas associated with tinnitus distress, including the cingulate cortex (Vanneste et al., 2017).
Other Clinical Conditions
The effect of tVNS on a variety of other diseases has been explored. A pilot study of tVNS in schizophrenia found no effect on symptom severity (Hasan et al., 2015). Moreover, many potential targets for treatment via tVNS have been suggested, including attention deficit hyperactivity disorder (ADHD) (Beste et al., 2016), autism spectrum disorders (Jin and Kong, 2016), Alzheimer's dementia (Jacobs et al., 2015), post-operative cognitive dysfunction (Xiong et al., 2009), increased risk of type II diabetes (Huang et al., 2014), preterm infants with oromotor dysfunction (Badran et al., 2020), chronic stroke patients (Capone et al., 2017), coronary insufficiency (Afanasiev et al., 2016) and chronic migraine (Straube et al., 2015). The idea that tVNS might be a promising treatment in Alzheimer's dementia has received support through recent evidence that tVNS can recover impaired microglia function in a mouse model of Alzheimer's dementia (Kaczmarczyk et al., 2017; Huffman et al., 2019), and there is an ongoing clinical trial of tVNS as a treatment for mild cognitive impairment (NCT03359902). For ADHD, trigeminal nerve stimulation (TNS) has been suggested as a complementary treatment to tVNS, and a recent study found promising clinical improvements (McGough et al., 2019). A study in patients with chronic pelvic pain (Napadow et al., 2012) found that tVNS ameliorated patient-reported pain intensity and anxiety. Antinociceptive effects of tVNS have been replicated in some studies but not in others, and its effect has remained inconsistent between studies and individuals (Laqua et al., 2014; Usichenko et al., 2017a,b; De Icco et al., 2018; Janner et al., 2018).
Whilst the above studies assume that the effects of tVNS are primarily mediated by central neuromodulation, i.e., effects on neurotransmission and neuroplasticity in the brain, tVNS-induced cardiovagal and cardiosympathetic effects have also been reported, and a number of studies have focused on the clinical potential of these effects. For example, a number of studies have found tVNS to reduce sympathetic nerve activity, indexed through resting muscle sympathetic activity (Clancy et al., 2014; Murray et al., 2016b; Ylikoski et al., 2017). However, cardiac effects of tVNS may be related to stimulation parameters, such as pulse width and stimulation frequency (Badran et al., 2018c) and there remains to date unexplained inter-individual variations in the clinical response to these parameters in the treatment of cardiovascular disease (Murray et al., 2016a).
Taken together, these studies indicate that tVNS has the potential to treat a wide range of clinical conditions. One of the key challenges for its further development appears to be the lack of inter-individual consistency in treatment success. Those differences are currently not well-understood, and may relate to anatomical differences, physiological state, and stimulation parameters. Table 1 provides an overview of the stimulation parameters that have been deployed in various studies along with other characteristics of those reports1.
Acute/Short-Term Stimulation in Experimental Trials
Alongside clinical trials and intervention studies, tVNS has gained increasing interest as a tool for neuromodulation in experimental studies. Based on evidence that vagal activity is related to a host of psychological and physiological processes, tVNS promises deeper insights by enabling active manipulation of VN activity. Predominantly, these studies are characterized by short stimulation periods, addressing the immediate effects. Psychological targets have been broad (though not all of them are sensitive to tVNS), including: experimentally induced worry (Burger et al., 2019a); post-error slowing (Sellaro et al., 2015b); attention to fearful faces (Verkuil and Burger, 2019); associative memory (Jacobs et al., 2015) or single-item word memory (Giraudier et al., 2020; Mertens et al., 2020); extinction of fear responses or fear conditioning (Burger et al., 2016, 2017, 2018, 2019b; Genheimer et al., 2017; Szeska et al., 2020); implicit spiritual self-representations (Finisguerra et al., 2019); flow experience (Colzato et al., 2018b); response selection during sequential action (Jongkees et al., 2018) or during action cascading processes (Steenbergen et al., 2015); the recognition of emotions in faces or bodies (Colzato et al., 2017; Sellaro et al., 2018; Koenig et al., 2019); divergent thinking (Colzato et al., 2018a); conflict-triggered adjustment of cognitive control (Fischer et al., 2018); auditory selective attention (Rufener et al., 2018) or visual selective attention (Ventura-Bort et al., 2018); inhibitory control (Beste et al., 2016; Borges et al., 2020); automatic motor inhibition (Keute et al., 2018); cognitive flexibility (Borges et al., 2020; Tona et al., 2020); prosocial behavior (Sellaro et al., 2015a) and reward sensitivity (Neuser et al., 2019).
Other more physiologically oriented studies have investigated the influence of tVNS on cardiac activity (Brock et al., 2017; De Couck et al., 2017; Lamb et al., 2017; Gancheva et al., 2018; Borges et al., 2019; Bretherton et al., 2019; Koenig et al., 2019; Paleczny et al., 2019; Tobaldini et al., 2019; Tran et al., 2019); autonomic outflow (Sclocco et al., 2017); sympathetic nerve activity (Clancy et al., 2014) or cardiac baroreflex sensitivity (Antonino et al., 2017); atrial fibrillation (Stavrakis et al., 2015); cardiac mechanical function (Tran et al., 2019); vagal sensory evoked potentials (Fallgatter et al., 2003, 2005; Polak et al., 2009; Leutzow et al., 2013); persistent hiccups (Schulz-Stübner and Kehl, 2011); visual bistable perception (Keute et al., 2019a); nociceptive neuromodulation (Napadow et al., 2012; Busch et al., 2013; Laqua et al., 2014; Usichenko et al., 2017b; Janner et al., 2018); tumor necrosis factor-alpha (Brock et al., 2017); hepatic energy metabolism (Gancheva et al., 2018); whole blood culture-derived cytokines and chemokines (Lerman et al., 2016); salivary hormones (Ventura-Bort et al., 2018; Koenig et al., 2019; Warren et al., 2019); pupil diameter (Warren et al., 2019); gastroduodenal or gastrointestinal motility (Frøkjaer et al., 2016; Juel et al., 2017); muscle activity in the gastrointestinal tract (Hong et al., 2019), gastric frequency (Teckentrup et al., 2020); electroencephalography (Hyvärinen et al., 2015; Keute et al., 2018; Lewine et al., 2019) and event-related potentials (Lewine et al., 2019), specifically the P3/P300 event-related potential (Ventura-Bort et al., 2018; Warren et al., 2019); cortical excitability (Capone et al., 2015) and changes in blood-oxygen-level-dependent (BOLD) functional Magnetic Resonance Imaging (fMRI) (Kraus et al., 2007, 2013; Dietrich et al., 2008; Frangos et al., 2015; Frangos and Komisaruk, 2017; Garcia et al., 2017; Yakunina et al., 2017, 2018; Badran et al., 2018b; Peng et al., 2018; Sclocco et al., 2019, 2020). Ultrahigh field (7T) fMRI studies with enhanced spatiotemporal resolution have clearly demonstrated tVNS stimulus-evoked activation of the ipsilateral NTS, the primary synapse for vagus nerve traffic to the brain (Garcia et al., 2017; Sclocco et al., 2019, 2020). Cases reports illustrate the use of tVNS in the treatment of a patient with persistent geotropic direction-changing positional nystagmus (Cha et al., 2016) or insomnia (Yu et al., 2017). Table 2 summarizes the characteristics of these studies on acute/short-term tVNS.
Proposed Checklist for Minimum Reporting Items
Based on the review of the existing literature, we propose a set of minimum reporting items for tVNS publications in Table 3. Important to note, these are not suggested to replace existing standards or guidelines when reporting observational studies (von Elm et al., 2008) or clinical trials (Moher et al., 2001). Figure 2 provides a graphical overview of the specific tVNS reporting items.
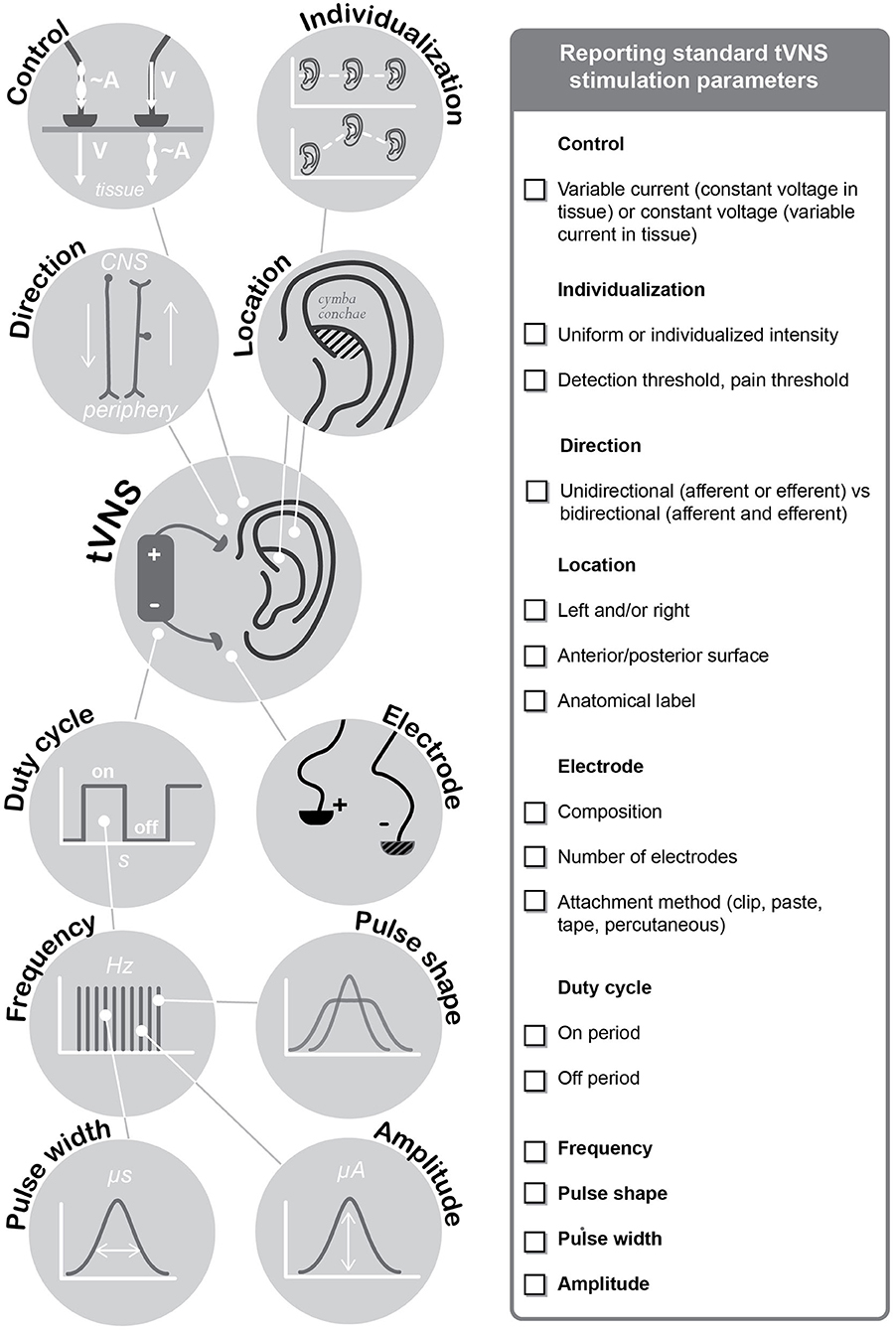
Figure 2. Minimum Reporting Standards for Research on Transcutaneous Vagus Nerve Stimulation (Version 2020).
In regards to stimulation level reporting, our general guidance (consistent with recommended reporting practices for other techniques, e.g., Woods et al., 2016; Bikson et al., 2019) is to fully describe the dose and any further details of electrode design that may impact tolerability. As with other reporting items, how and what details should be reported is guided by the principle of reproducibility. Dose is defined as all parameters of the device (hardware and programming) that govern the pattern of current flow through the body including to the nominal nerve target (Peterchev et al., 2012). For electrical stimulation dose encompasses: (1) all aspects of the stimulation waveform (e.g., pulse shape such as square, frequency); (2) details of electrode contact with the skin (e.g., size, shape, location). Factors that go into selecting dose, on a trial or subject basis (such as titration to sensation) are critical to report, but the actual dose applied should also be reported (Peterchev et al., 2012). It is important that complete details of dose be reported, not simply those aspects of dose the investigators think are important to outcomes (or important to mention). It is also important to recognize that referencing a technique by a name of classification does not fully describe dose since the same name may be used to describe different protocols (Guleyupoglu et al., 2013; Bikson et al., 2019). Nor is it sufficient to describe dose by referring to prior publications when those publications did not fully describe dose, when those prior works described a range of approaches broader than tested in the present study, or when any modifications (even incremental) were made. Finally, careful attention should be paid to the use of nomenclature (Bikson et al., 2019) that is not definitive in describing the dose (e.g., unipolar, anodal), may apply to different aspects of the dose (e.g., pulse duty cycle or train duty cycle) or mis-applying terminology (biphasic vs. bipolar).
Details of electrode design, preparation and application that are no genuine part of dose are likewise critical to allow consistent dosing. For example, the critical interface is the contact surface between the tissue and electrolyte e.g., hydrogel, paste (for a non-invasive electrode), or metal (for a percutaneous electrode). This needs to be described for every electrode, including electrodes that are considered less important for outcomes (e.g., so called “return” or “reference” electrodes). Other aspects of the electrode, such as materials and thickness, are equally important for reproducibility, including, for example, electrochemical stability (Merrill et al., 2005) and tolerability (Minhas et al., 2010; Khadka et al., 2018).
Discussion
Having proposed a set of reporting standards, we will now address some of the outstanding issues, which in our view, future tVNS studies have to objectively and systematically address. These issues have all been examined in previous studies to a greater or lesser extent, but given the lack of reporting standards, no definite conclusions can yet be drawn. It is our hope that having provided these standards, clear answers will become apparent in the years to come. Here we will subsequently discuss issues related to safety, confounding, stimulation parameters, underlying physiology including studies on biomarkers and translational studies.
Safety and Tolerability
In line with our recommendations of providing standardized information on stimulation parameters etc., we encourage the standardized reporting of adverse events as suggested by Redgrave et al. (2018). A systematic literature review on the safety and tolerability of tVNS has evaluated 51 studies, independent of the area of application (Redgrave et al., 2018). The authors report that the most prevalent side effect was local skin irritation from electrode placement, occurring in about 18% of included subjects following long-term stimulation. Nevertheless, it is important to note that 89 studies were not included in this review as these studies had not reported safety or tolerability data and when approached the authors didn't respond to a formal request to provide data.
Potential Confounding Variables
Alongside transparent reporting of stimulation parameters and adverse events, important confounding variables need to be considered and reported. The inter-individual variability in the neurophysiological and behavioral response to tVNS is high and the reasons for this are poorly understood. A diverse array of factors including, but not limited to age and comorbidities, subjects' ear and tissue morphology and innervation, neurotransmitter balances and brain state, may contribute to inter-individual differences in tVNS response. Based on studies using tVNS, iVNS, and other electrical stimulation techniques, we suggest that investigators consider the following variables that can influence the responsiveness to tVNS and can confound the results in their studies.
Age
Increasing age affects both parasympathetic and sympathetic activity (e.g., Kuo et al., 1999). For example, age is associated with marked changes at hormonal level, which in turn affect acetylcholine-mediated parasympathetic autonomic activity, which is affected by tVNS (Moodithaya and Avadhany, 2012; Krause and Cohen Kadosh, 2014). Furthermore, sensitivity to electrical transcutaneous stimulation is lower in older age-groups (Kemp et al., 2014).
Sex
In animal studies VNS has greater effects in females, probably because of the effect of oestrogens to the muscarinic acetylcholine in the central nervous system (Du et al., 1994). Similar effects should be expected in human subjects due to both hormonal levels and the gender- and age-dependent differences in the functions of the autonomic nervous system (Koenig and Thayer, 2016; Koenig et al., 2017). Differences in the neuronal pathways and neuronal sensitivity may exist and therefore affect response to tVNS (De Couck et al., 2017; Janner et al., 2018).
Medical Conditions
Neurotransmitter levels may differ between individuals according to specific medication intake and medical condition. This was shown to cause research subjects to respond differently to stimulation due to a certain dose-response relationship that interacts with initial neurotransmitter levels (Ziemann et al., 2002; Falkenberg et al., 2012). Therefore, to avoid confounds in experiments, we recommend to control for (or exclude) individuals with psychological or psychiatric conditions (e.g., Homma et al., 1993; Salman, 2015) and medication use that affects neurotransmitter systems (unless those study populations are directly relevant to the research question).
Ear and Tissue Anatomy
Different ear sizes and skin properties, such as impedance, water content, structure, and subcutaneous fat thickness as well as auricular anatomy of the vagus innervation may cause different current distributions and require different current strengths to achieve the same current flow (Maffiuletti et al., 2008; Cakmak, 2019). Consequently, physiological and behavioral effects may vary.
Time of the Day /Different State
The brain does not always respond stereotypically to stimulation, as response may depend on the current state of activity (Silvanto et al., 2008), level of fatigue, wakefulness, attention, or mood (Sztajzel et al., 2008; Steenbergen et al., 2020). Controlling for brain state, for instance, by employing a focused behavioral task, or applying stimulation only during a particular brain state, e.g., based on patterns of electroencephalographic (EEG) activity (Brázdil et al., 2019), may potentially improve responsiveness. This may also extend to physiological states in general, such as respiratory phase (Napadow et al., 2012; Garcia et al., 2017; Sclocco et al., 2019).
Adherence
Especially in neuropsychiatric populations, adherence must be controlled. Dependent on the population non-adherence rates up to 50% (Perkins, 2002) have been reported from pharmaceutical trials and it must be assumed that the same numbers will occur. Such non-adherence rates have e.g., reported for the tVNS schizophrenia trial (Hasan et al., 2015) and the adherence should be recorded and analyzed in all future tVNS trials.
Control Condition
Control condition is tVNS tested against sham stimulation (actual stimulation of the earlobe, for example), or no stimulation. Further, authors should report on placebo/expectations effects and which attempts were made to control for this influence. Very recently, problems with the wrongful placement of electrodes in sham-stimulation, in particular the possibility to stimulate muscle zones with potential effects, have been discussed (Cakmak et al., 2017; Liugan et al., 2018).
In future, it is important that researchers are aware of sources of variability that may affect tVNS response, especially in studies using heterogeneous populations, and that they select their desired research population with caution. Furthermore, tracking potential confounds may allow the investigators to control for them in the analysis and to understand outliers within the data. This approach may help to understand factors explaining heterogeneity in the efficacy and response to tVNS.
Left or Right? A Question of Laterality in VNS Targeting
Anecdotally during the development of iVNS, theoretical concerns emerged regarding cardiac safety when implanting electrodes on the right cervical VN in comparison to the left. This theory was only explored in one iVNS trial exploring both left and right iVNS for chronic heart failure which demonstrated equal safety profiles (Premchand et al., 2014). Animal studies suggest that right sided iVNS has stronger cardiac effects (Ng et al., 2001; Yoo et al., 2016). Due to this uncertainty, an important constraint when applying taVNS is the choice of the ear side during stimulation. Individual stimuli delivered to the right cervical VN have two-fold inhibition effects on heart beating cycle, compared to identical stimuli delivered to the left nerve (Brown and Eccles, 1934). The reason is that efferent vagal fibers affecting the sinoatrial node of the heart are thought to be right-lateralized (Nemeroff et al., 2006). Studies in rats have shown that vagal fibers originating in the right dorsal nucleus and the right ambiguous nucleus further inert the region of the syno-atrial nodule, while the fibers of the left dorsal motor nucleus and the projected ambiguous further inert into the atrioventricular nodule region (Brack et al., 2004). Despite the possible side effects of right sided vagal stimulation, a possible treatment for heart failure has been developed using a tcVNS device, measuring the heart rate, that shuts down when bradycardia is detected (De Ferrari and Schwartz, 2011). However, for reasons outlined above, and possibly because a clinical trial showed no arrhythmic effects of tVNS when stimulating the left VN (Kreuzer et al., 2012), taVNS is almost exclusively applied to the left ear. Yet, these concerns have been challenged (Chen et al., 2015). For instance, studies in rodent models have not shown deleterious cardiac side effects (Krahl et al., 2003, also see Ay et al., 2011; He et al., 2013b). A study in healthy human participants has shown that taVNS can be applied to the right ear without associated cardiac side effects (De Couck et al., 2017). Similarly, studies in patients with chronic heart failure (Premchand et al., 2014; Wang et al., 2015b) did not report cardiac side effects suggesting that bilateral or right-lateralized taVNS is not associated with an excess rate of adverse effects. Furthermore, varying the intensity of taVNS has been shown not to impact on cardiac vagal activity in healthy adults (Borges et al., 2019). Critically, to the best of our knowledge, no systematic safety studies to date have directly compared stimulation sites and duration of stimulation to examine possible cardiac adverse effects.
The possibility of safely stimulating both, the left and right VN simultaneously, is of interest. In terms of using tVNS to increase noradrenaline release, it is plausible to suggest that bilateral stimulation may improve efficacy. Animal experimental data suggest a very wide spectrum of effects, critically dependent on stimulation parameters as well as on the duration of stimuli trains (Levy et al., 1969; Slenter et al., 1984) phase-locking the heart beat to the vagal stimuli (Jalife et al., 1983) through the interaction of neural and muscular reflexes (Brooks and Lange, 1977). It has been shown that tVNS activates brain regions with ipsi and against lateral differences—such as the nucleus of the solitary tract, the amygdala or the nucleus accumbens (Frangos et al., 2015). LC projections to the cortex are mainly ipsilateral (Aston-Jones and Waterhouse, 2016), and noradrenaline levels are increased in both hemispheres after iVNS in rats. It has also been shown that depending on the currents applied (iVNS), different neuronal populations are recruited, and moreover that noradrenaline release in different target areas is also current-dependent (Roosevelt et al., 2006). Thus, hypothetical by stimulating both ears simultaneously, a summation effect could potentially be attained to reach the desired effects (also see Clancy et al., 2014). This idea should be objectively evaluated in the future, since pain threshold in some patients can be as low as 0.5 mA when auricular stimulation is carried out, and therapeutic effects could require higher stimulation currents (>1.0 mA) (Yakunina et al., 2017).
Current-Controlled vs. Voltage-Controlled Stimulation
In this iteration of the consensus, we would like to particularly focus on one particular technical aspect, namely the proper reporting on whether current-controlled or voltage-controlled stimulation is used. In principle current or voltage control settings can be used for tVNS; however, effects of and on the electrode/tissue boundary have to be accounted for (Merrill et al., 2005; Kaniusas et al., 2019a). As is generally the case in neuromodulation (Butson and McIntyre, 2005; Merrill et al., 2005; Vargas Luna et al., 2013), the current-controlled reliably defines the current in the body (e.g., excitable auricular tissue) independent of the highly variable electrode/tissue boundary. However, in the case of voltage-controlled tVNS, the resulting current in the tissue depends strongly on the electrode-skin boundary properties which then influence the resulting stimulation efficiency. The impact of current-controlled vs. voltage-control on the effectiveness will depend on multiple factors including electrode design. For instance, needle electrodes (for example in percutaneous tVNS) act typically as polarizable electrodes so that the boundary is predominantly capacitive, whereas surface electrodes (for example in taVNS) can act as non-polarizable electrodes with a predominantly resistive boundary. One theoretical concern with current-controlled stimulation is that conditions of unexpected high impedance at the electrode-skin interface will result in an associated increase in stimulator output voltage (i.e., needed to overcome this resistance in providing a prescribed current). The maximum voltage is limited by stimulator output compliance voltage. In a situation where the impedance suddenly changes, which can result from the electrode becoming displaced or (partially) detached and then reattached to the skin, a current controlled device may transiently produce a current above the target level (as its internal circuit adjust to the lower impedance load), which in turn can result in an unpleasant shock. This can be readily addressed with robust and motion-free application of current electrodes (e.g., ear clip electrodes, reliable adhesive electrodes), protocols that are cognisant of factors such as when stimulators are powered (Badran et al., 2019), or stimulators that are designed with a rapid accommodation time.
In the case of the voltage application, the polarization voltage is limited by the applied voltage. In addition, any potential detachment of the voltage electrode leads to an even reduced polarization voltage and thus reduced risks of unwanted transients. Consequently, voltage-controlled stimulation may be limited by current changes in situations where electrode-skin contact is not reliable. In addition to adverse events that can result from current flow through the body (e.g., pricking/itching), as with any electrical stimulation, adverse events may result from excessive electrochemical reactions at the electrode-electrolyte interface (Kaniusas, 2019). Specifically, if electrochemical products at the electrode-electrolyte interface reach the skin, skin irritation may ensue. Protocols to limit this include using charge-balanced waveforms (Sooksood et al., 2009, 2010), judicious selection of metal and electrolyte materials (Merrill et al., 2005; Khadka et al., 2018), minimizing total stimulation time at a given location, or ensuring the electrolyte provides sufficient separation between the metal and skin (Minhas et al., 2010).
Empirical Evidence for the Use of Certain Stimulation Parameters
Currently, the popularity of one tVNS device, tVNS Technologies GmbH (Erlangen, Germany), led to a common yet poorly argued parametric setting. Given the lack of flexibility of this device regarding changing the parameters, a signal with a pulse width between 200 and 300 μs at 25 Hz, and a duty cycle of 30-s on, 30-s off has frequently been adopted in studies. However, other parameters have been used in research with tVNS as well, which may explain in part the heterogeneity observed in findings from studies using tVNS (Borges et al., 2019). Consequently, the lack of knowledge regarding optimal stimulation parameters can be seen as a general limitation in this research field (Borges et al., 2019; Butt et al., 2020). Despite an understanding of the importance of the various stimulation parameters in optimizing the efficacy of tVNS, dose-response studies remain scarce. Recently, Badran et al. (2018c) systematically tested the effect of three variations in pulse width and frequency, respectively, on HR and found that a pulse width of 500 μs, if combined with a frequency of 10 Hz, produced the strongest decrease in HR compared to other parameter combinations. However, as HR is the result of mixed inputs from the sympathetic and parasympathetic (vagus) nerves, the effect of tVNS on HR may not necessarily correlate with the outcome of interest (Goldberger et al., 2019). Therefore, we advocate caution when interpreting these results. Some efforts have been made to understand how changing specific stimulation parameters influences the physiological effects of tVNS. Borges et al. (2019) tested the effect of different intensities on cardiac VN activity (Malik, 1996) in three experiments. They also compared different methods to define current intensity regarding cardiac vagal activity, namely presetting the same current intensity for all study participants throughout the experiment (set method) and instructing the study participants to freely choose an intensity (free stimulation method). Cardiac vagal activity increased during tVNS when compared to resting measurement. However, this increase was not related to stimulation intensity, the method of stimulation, or whether the stimulation was active or sham. De Couck et al. (2017) investigated the effect of stimulation side (right, left ear, or sham), and session duration (10 min or 1 h) on heart rate variability (HRV). They found very specific effects related to heart rate variability components such as standard deviation of the RR intervals (SDNN) as well as low frequency (LF) and LF/high frequency (HF) ratio. However, tVNS had no effects on parameters that serve as an index of cardiac vagal activity, such as root mean square of successive differences in RR intervals (RMSSD) (Malik, 1996). Changes in the frequency domain components of HRV (LH, HF, and LF/HF ratio) were also observed with 1 h of tVNS at the right tragus (Tran et al., 2019). It was also reported that the magnitude and direction of tVNS-induced changes in LF/HF ratio is dependent on resting LF/HF ratio (Bretherton et al., 2019). The greatest effects of tVNS were observed in individuals with the lowest cardiac vagal activity at rest. Regarding stimulation location, Yakunina et al. (2017) compared the effects on brain activation of stimulation carried out at the inner tragus, inferoposterior wall of the ear canal, cymba conchae, and earlobe (sham). Among these areas, only tragus and cymba conchae stimulation activated areas thought to be part of the vagal pathway, such as the NTS. Importantly, the strongest activation of vagally innervated areas was seen during cymba conchae stimulation. These results are consistent with anatomical studies suggesting that the auricular branch of the VN innervates primarily the cymba conchae and the tragus (Peuker and Filler, 2002). Interestingly, a recent study by Sclocco et al. found that stimulation frequency also significantly modulates BOLD fMRI response in NTS, as well as other brainstem nuclei such as LC and raphe nucleus (Sclocco et al., 2020), with 100 Hz stimulation demonstrating enhanced activation in healthy adult volunteers. As anatomy is fundamental to providing effective tVNS (Badran et al., 2018a, also see Burger and Verkuil, 2018), further studies are warranted to delineate the exact anatomical basis of tVNS, in order to better guide future trials.
To summarize, the choice of stimulation parameters, mainly linked to pulse width, frequency, side and location of the stimulation, may influence effects of tVNS on both autonomic and cognitive processes. However, attempts to investigate the effects of individual tVNS stimulation parameters have primarily focussed on presumed physiological effects of tVNS rather than cognitive processes. Furthermore, despite first attempts to address the effects of parametrization, it is not clear what cognitive or autonomic processes have a parametric-specific effect, and this could explain the high heterogeneity of findings in studies using tVNS. Thus, it is time to carry out further studies that aim at understanding the parametric-specific effects of tVNS in order to optimize this tool for different applications.
Potential Biomarkers of Effective Stimulation
The neural mechanisms mediating the effects of tVNS are still poorly understood and, consequently, no clear consensus exists about potential biomarkers that could shed light on the efficacy of tVNS in general, or those guiding a choice in stimulation parameters. In this section, we briefly summarize findings concerning potential biomarkers related to vagal activity (for a detailed review about biomarkers of tVNS, see (Burger et al., 2020a) and finish with some remarks on methodological aspects that may be relevant when assessing biomarkers in tVNS research.
Heart Rate Variability
Some authors have proposed that the beneficial effects of tVNS may rely on increased activity of the VN per se (Gidron et al., 2018). Therefore, tVNS-related changes in vagal activity—measured by vagally-mediated HRV measures (vm-HRV) (Thayer and Lane, 2000; Kuo et al., 2005) may be informative to its efficacy. Animal research has consistently found that VNS, particularly to the right VN, increases vm-HRV measures (e.g., Huang et al., 2010; Sun et al., 2013). However, the relation between iVNS and HRV measures is less clear in humans (see Burger et al., 2020a for further details). Similar to reports using iVNS, findings on the modulatory effects of tVNS on vm-HRV measures are heterogeneous. Some studies showed an increase of vm-HRV measures after tVNS (Lamb et al., 2017; Bretherton et al., 2019; Sclocco et al., 2019; Tran et al., 2019), but others showed no effects (Weise et al., 2015; Antonino et al., 2017; De Couck et al., 2017; Burger et al., 2019a,b), or showed a decrease of vm-HRV parameters in individuals with high resting vagal activity (Bretherton et al., 2019) and two other studies during both, active and sham stimulation (Borges et al., 2019, 2020). A potential limitation of vm-HRV measures as a biomarker for tVNS is that the mechanism influencing vm-HRV (i.e., efferent vagal activation) may differ from the mechanistic target of tVNS (i.e., afferent vagal activation), and little is known about the interrelation of these two vagal pathways, i.e., much is known about cervical vagal feedback loops, but not much is known regarding auricular to cervical loops.
Metabolic Markers of Vagal Stimulation
The VN is a key part of the autonomic nervous system and transmits information between the peripheral organs and the brain to support homeostasis (de Lartigue, 2016). Although vagal stimulation primarily targets afferent fibers, preclinical and human work points to efferent effects as well that are mediated via the brain. In animal studies, there is conclusive evidence for reduced food intake and weight loss following iVNS (Roslin and Kurian, 2001; Val-Laillet et al., 2010; Gil et al., 2011; Banni et al., 2012). In rodents, a closed-loop VNS system implanted on the stomach wall substantially reduced food intake and delayed weight gain (Yao et al., 2018) demonstrating the modulatory role of negative feedback signals. In human studies, the vital role of the VN in modulating food intake, energy metabolism, and glycemic control has been demonstrated more recently (Burneo et al., 2002; Pardo et al., 2007; Shikora et al., 2013; Ikramuddin et al., 2014; Cork, 2018). Notably, taVNS has been shown to decrease the frequency of action potentials in human gastric muscle cells (Hong et al., 2019; Teckentrup et al., 2020) suggesting that an electrogastrogram could be used to non-invasively track successful vagal stimulation. Taken together, these results highlight that stimulating vagal afferents may elicit efferent effects on key markers of energy homeostasis that could be used as a positive control outcome.
Noradrenergic-Related Processes and Markers
One potential mechanism by which tVNS may exert its effect is through the activation of the LC norepinephrine (LC-NE) system (Van Leusden et al., 2015; Hansen, 2019). Evidence pointing to a modulatory role of VN activity on LC-NE system activity comes from neuroimaging studies (Dietrich et al., 2008; Kraus et al., 2013; Frangos et al., 2015; Yakunina et al., 2017) and from studies relating vagal activity with physiological markers of LC-NE system activity, such as the P300 amplitude of event-related potentials (ERPs) (Murphy et al., 2011) see for review (Nieuwenhuis et al., 2005), salivary alpha amylase(sAA; Ehlert et al., 2006; Warren et al., 2017), and pupil dilation (Rajkowski, 1993; Joshi et al., 2016; Warren et al., 2017). For instance, De Taeye et al. observed that epileptic patients that responded favorably to iVNS therapy showed an increase in the P300 amplitude during VNS (De Taeye et al., 2014) see also (Neuhaus et al., 2007; Schevernels et al., 2016; Wostyn et al., 2017). In healthy participants, however, evidence for the modulatory effects of tVNS on the P300 amplitude has been mixed. Some studies found enhancing effects (Rufener et al., 2018; Ventura-Bort et al., 2018; Lewine et al., 2019), but others found no modulation of the P300 (Warren et al., 2017; Fischer et al., 2018). In terms of pupil dilation, although evidence about the relation between VNS and pupil dilatation is rather scarce, findings in animals (Bianca and Komisaruk, 2007; Mridha et al., 2019) and humans (but see Schevernels et al., 2016; Jodoin et al., 2018) seem to point to increased dilation of the pupil under active iVNS compared to no stimulation. By contrast, in four recent tVNS studies, no modulation of pupil dilation in response to the stimulation was found (Keute et al., 2019b; Warren et al., 2019; Burger et al., 2020b). Finally, recent studies have investigated the effects of tVNS on sAA levels as a potential marker of central NE release (Ehlert et al., 2006; Warren et al., 2017). Similar to P300 and pupil dilation, studies exploring tVNS effects on sAA level changes have shown inconsistent results. Some showed increased sAA levels following tVNS, but not after sham stimulation (Fischer et al., 2018; Ventura-Bort et al., 2018; Warren et al., 2019). Three recent studies did not show any sAA changes in response to tVNS (Koenig et al., 2019; Giraudier et al., 2020; D'Agostini et al. under review), Also, documented improvements of sleep quality with tVNS (Bretherton et al., 2019) are inconsistent with LC activation, which is the main brainstem nucleus that promotes arousal.
Taken together, there is currently no reliable vagal or noradrenergic biomarker of tVNS that produces replicable results across studies. It is likely that the reasons for this are multifactorial [see for a detailed discussion, (Burger et al., 2020a)]. Firstly, many studies included relatively small sample sizes, and the reported effects may have been underpowered. Secondly, baseline differences in tonic noradrenergic activation may also have an important influence on the efficacy (Murphy et al., 2011; Gee et al., 2014; van Kempen et al., 2019). Finally, stimulation settings, including stimulation sites (tragus vs. cymba concha; left vs. right ear) and parameters such as stimulation interval (30 s ON/OFF vs. continuous stimulation), intensity set-up (fixed or variable across participants), pulse widths, stimulation timing, among others, are not kept constant across experiments, impeding, to some extent, a full comparison of the results across labs. These changes might not be arbitrary, given that some of the settings may favor the efficacy of tVNS (e.g., stimulation of cymba conchae compared to the tragus (Yakunina et al., 2017); continuous vs. intermittent stimulation (Ventura-Bort et al., 2018); and long compared to short stimulation duration (Warren et al., 2019). We hope that the aforementioned standards may help overcome these challenges and improve the current knowledge about potential tVNS biomarkers.
Functional Neuroimaging
In comparison to HRV, pupil dilation and sAA, functional magnetic resonance imaging fMRI provides the possibility to confirm involvement of the central noradrenergic system by looking directly at LC and NTS activation as well as activation of possible target areas. Consequently, neuroimaging studies have tried to assess the modulatory role of VN activity on the LC-NE system activity in healthy adults (Kraus et al., 2007, 2013; Dietrich et al., 2008; Frangos et al., 2015; Yakunina et al., 2017; Badran et al., 2018b; Peng et al., 2018; Sclocco et al., 2019, 2020) and interictal migraine patients (Garcia et al., 2017; Zhang et al., 2019). The following results in functional activation are based on the comparison between real and sham stimulation at varying stimulation locations across the studies (see Table 1). Three studies that examined activation using 1.5T imaging (Dietrich et al., 2008) did not report any sham stimulation, therefore their results were based only on active stimulation compared to pre-stimulation baseline (N = 4). The authors found increased activation in the left LC as well as an increase in functional activation in the left thalamus (Dietrich et al., 2008). Conversely, a decrease in functional activation in limbic and temporal brain areas (N = 6) (Kraus et al., 2007) as well as in the LC and the NTS (n = 8) (Kraus et al., 2013) has also been shown. However, the reported functional activation of these three studies might have to be interpreted with caution due to the low sample sizes. Additionally, it is possible that the spatial precision afforded in data acquisition and data processing was not sufficient in these studies to reliably detect activations in LC and the NTS which are only a few millimeters wide. Furthermore, none of these studies reported information on the MRI head coil or smoothing kernel used which makes it difficult to assess Signal-to-Noise Ratio (SNR) and spatial precision of the results (Kraus et al., 2007, 2013; Dietrich et al., 2008). Studies with larger sample sizes using 3T scanners have shown functional activation in NTS (Frangos et al., 2015; Garcia et al., 2017; Yakunina et al., 2017; Sclocco et al., 2020), in the bilateral amygdala and left parahippocampal gyrus (Peng et al., 2018), which corresponds to the results of Frangos et al. (2015) that showed an increase in activation in the contralateral amygdala, nucleus accumbens and anterior thalamic nuclei. Moreover, a gradual increase and maximal activation in NTS during post-stimulation was observed (Frangos et al., 2015). In addition to increased NTS activation, post stimulation effects, immediately after exhalatory-gated auricular vagal afferent nerve stimulation (eRAVANS), led to increased response to trigeminal sensory afference in nucleus raphe centralis and LC (Garcia et al., 2017). Yakunina et al. (2017) showed bilateral LC and NTS activation in unsmoothed data and indicated that this was also observed during real stimulation by placing electrodes at the inner surface of the tragus. Badran et al. (2018b) were not able to replicate these effects. However, this study used lower resolution fMRI (voxel size of 3 mm3), which may explain the lack of activation observed in the NTS and LC. Some studies have also reported a decrease in functional activation in the bilateral hypothalamus and throughout the hippocampal formation in healthy adults (Frangos et al., 2015) as well as in the bilateral LC in interictal migraine patients (Zhang et al., 2019). This heterogeneous pattern of functional activations reported highlights once again the challenge of comparing and interpreting results from fMRI tVNS studies using different devices, electrodes, stimulation locations and session durations, as already previously reviewed (e.g., Yakunina et al., 2017; Peng et al., 2018). It is furthermore equally important to consider the varying stimulation and rest phases of study designs in different studies (e.g., 0.5 s pulse during each exhalation phase of respiration Garcia et al., 2017; 7 min on/2 min off stimulation Frangos et al., 2015; 30 s on/60 s off stimulation Yakunina et al., 2017; Peng et al., 2018), which might have affected the ability to detect functional activations in NTS and LC target areas. As there is considerable one possible focus in using tVNS fMRI to study evoked activation in NTS and other neurotransmitter source nuclei following stimulation, the fMRI studies of Yakunina et al. (2017) and Sclocco et al. (2019) used high resolution (e.g., 2.75 mm, and 1.2 mm isotropic resolution, respectively) and small Gaussian smoothing kernels (e.g., 2 mm), provide promising spatial precision in their methodological approach. Moreover, both studies compared results across various stimulation locations and observed most convincing LC activations using the left cymba conchae as an active stimulation location, which is in line with left cymba conchae being considered a good target for eliciting LC activation (Peuker and Filler, 2002). Both Yakunina et al. (2017) and Sclocco et al. (2019) provided details on co-registration methods and demonstrated sufficient spatial precision in data processing. The latter study used ultra-high-resolution fMRI at 7 Tesla with multi-band factor 2 to further increase SNR and demonstrated that exhalatory-gated tVNS enhanced NTS and LC/raphe targeting. Similar to Garcia et al. (2017), they observed increased activation in the LC as well as both dorsal and median raphe nuclei and in contrast to previous studies, they implemented short duration stimulation events (1s) extended over many minutes of time (Sclocco et al., 2019). Whilst many 3T fMRI studies may lack sufficient spatial precision to answer the question whether tVNS can target NTS and LC, recent studies suggest that larger sample sizes can also show NTS and LC response at this lower field strength (Sclocco et al., 2020), and previous 3T studies are more numerous and provide the strongest support that tVNS may indeed be a suitable tool for targeting the LC-NE system. Disorder specific brain circuits have been discussed as potential targets for tVNS in depression (Iseger et al., 2020) and tinnitus (Yakunina et al., 2018).
Taken together, when validating tVNS effects in various populations with the use of the most direct biomarker at hand for the LC-NE system, i.e., fMRI—a number of methodological considerations should be kept in mind over and above the usual need for appropriate stimulation parameters. Specifically, given that both NTS and LC span only a few millimeters, the extent of smoothing across studies should be considered. Frangos et al. (2015) pointed out the concerns of applying spatial smoothing to brainstem nuclei. Choosing a too high smoothing factor [e.g., 6 mm (Peng et al., 2018) or 8 mm (Yakunina et al., 2017)] could lead to an increased likelihood of false positives or to no observable activation in brainstem nuclei and thus, some chose to forgo smoothing brainstem data (Frangos et al., 2015; Yakunina et al., 2017). Similarly, ultra-high-resolution fMRI in the range of 1–2 mm voxel sizes also at higher field strengths seems warranted as well as customized high-precision spatial post-processing approaches optimized for the LC-NE system [see (Liu et al., 2017) for a review]. In addition to using comparable set-ups across studies, further research should also try to incorporate structural measures of the LC-NA system such as neuromelanin (NM)—sensitive magnetic resonance imaging (MRI) to anatomically identify the LC in vivo (Sasaki et al., 2006; Betts et al., 2017, 2019; Hämmerer et al., 2018; Priovoulos et al., 2018; Liu et al., 2019; Trujillo et al., 2019; Ye et al., 2020). Finally, the increased susceptibility of brainstem fMRI for low SNR and high physiological noise (Sclocco et al., 2018) could be counteracted by appropriate imaging paradigms as well as denoising or noise-control approaches (Brooks et al., 2013; Sclocco et al., 2018). If these recommendations are kept in mind, fMRI carries great potential as a more precise and direct tool for identifying activation in the LC-NE system using tVNS and in future may help to differentiate between tVNS responders vs. non-responders.
Toward Circuit-Based tVNS: Translational Approaches
Despite the growing interest in tVNS and in particular taVNS in clinical applications, many human studies remain in explorative frameworks and are typically confined to indirect readouts or neuronal activity of indirect fMRI responses (Yakunina et al., 2017; Burger et al., 2020a). Besides, imaging of small pontine nuclei such as the LC, NTS, or the raphe nucleus can be challenging in humans using MRI/fMRI, even at the purely anatomical level (Betts et al., 2019). Animal experimentation, on the other hand, can employ invasive techniques that allow researchers to gain detailed insights in molecular, anatomical, and neurophysiological mechanisms involved in VNS therapy. Thus, animal models enable a systematic investigation to be undertaken not only in terms of specificity of their readouts, such as cellular activity or level of neuromodulators, but also in terms of delineating parameter space for effective stimulation.
Effectiveness of VNS stimulation can be detected either directly with high temporal resolution i.e., in vivo electrophysiology as well as calcium imaging or more indirectly, after stimulation, using immunostaining or mRNA probes against immediate early genes products (C-Fos, Arc, Egr1) available in several animal models (Groves et al., 2005; Manta et al., 2009; Ay et al., 2016; Hulsey et al., 2017). Activation of the NTS, LC, and raphe nucleus after VNS has also been monitored via extracellular electrophysiological recordings (Groves et al., 2005; Manta et al., 2009; Hulsey et al., 2017). Hulsey and colleagues mapped the stimulation space using LC neuron spiking activity as an output variable. Although this study was performed using invasive VNS, it was clearly shown that the application of low currents (0.1–1.2 mA) induced LC neuron firing, but higher currents (>1.2 mA) also activated neighboring Me5 neurons (Hulsey et al., 2017). This finding is of particular importance since different neuronal populations with distinct axonal projection could be potentially recruited depending on the set of stimulation parameters chosen. Also such findings can explain the broad scope of responses seen in human studies under sub-optimal parameters. Electrophysiological modulation in LC output regions has also been recorded upon VNS (Dorr and Debonnel, 2006; Manta et al., 2009; Alexander et al., 2017; Beaumont et al., 2017). These changes in neuronal activity in LC efferents have also been associated with long-lasting changes in the synaptic proteome in the amygdala and piriform cortex (Alexander et al., 2017). It is worth mentioning that similar studies are absent in the case of the promising non-invasive taVNS in animal models.
Despite Hulsey's rigorous approach toward parameter space exploration, a very rigid set of stimulation parameters is commonly used in animal models as well as in human studies. These involve current intensities varying between 0.25 and 1 mA, pulse frequency ranging between 20 and 30 Hz, a pulse width of 330–500 μs and a duty cycle of 30 s stimulation followed by a 5 min resting phase for 30–60 min (Manta et al., 2009; He et al., 2013b; Jiang et al., 2016; Vázquez-Oliver et al., 2020). Nevertheless, animal research offers the possibility to easily explore new sets of parameters such as variable waveforms or summation effects of multiple stimulation locations (Ay et al., 2016; Kaniusas et al., 2019a). In this respect, biphasic waveforms have been lately proposed since they can lead to larger recruitment of nerve fibers compared to monophasic waveforms (Kaniusas et al., 2019a). Monophasic, biphasic and triphasic stimulation patterns for different bursts lengths were recently compared (Kaniusas et al., 2020). This aspect of being able to manipulate the waveform, therefore, may allow us to tailor the strength of our stimulation depending on the specific disease condition. Furthermore, the majority of reports fail to provide a convincing rationale behind their parameter selection (Hosoi et al., 2000; Huston et al., 2007), stating them as “customized” and thus hindering the optimization of these stimulation parameters (Noller et al., 2019). Given that tVNS finds its application in a range of conditions, just as in the human studies noted above, it will be of prime importance to scrutinize factors such as the stimulation parameters, the anatomical location to deliver the electrical stimulation on the VN, and the design of the electrodes (Noller et al., 2019). Overall, optimization of stimulation parameters derived from animal research may provide an essential basis for optimal tVNS in human patients.
Nevertheless, electrophysiological read-outs might not always be the most suitable output signal to tune stimulation parameters. Even though specific stimulation parameters can evoke robust neuronal spiking, it can also lead to neurotransmitter depletion at the terminals (Yavich et al., 2005). Therefore, higher spiking rates do not necessarily translate into increasing levels of neuromodulators at the extracellular space. Thus, when spike rate is used as the only output optimization variable, the final results can be skewed. In this context, neurochemical approaches became a potent tool that is routinely implemented in animal models but is still far from being applicable in humans. Pioneering studies in the neurochemistry field using microdialysis identified glutamate release in the NTS of cats as a likely mode of vagal neurotransmission (Allchin et al., 1994). Since noradrenergic, cholinergic, or serotonergic activation downstream of the NTS likely mediates therapeutic effects of VNS, synaptic exhaustion can lead to a ceiling of neurotransmitter/neuromodulator levels at lower stimulation frequency as determined by microdialysis (Roosevelt et al., 2006; Follesa et al., 2007; Raedt et al., 2011; Manta et al., 2013). Nevertheless, if real-time feedback is intended for optimization of stimulation parameters using neuromodulator concentration as the output variable, the temporal resolution of microdialysis is too low. Here, electrochemical methods such as cyclic voltammetry and amperometry can be a suitable alternative given their subsecond time resolution (Kile et al., 2012). In particular, neuromodulators such as dopamine, adrenaline, noradrenaline, ATP, and serotonin can be electrochemically detected via fast-scan cyclic voltammetry or amperometry in vivo (Heien et al., 2004; John and Jones, 2007; Gourine et al., 2008; Njagi et al., 2010). Thus, fast electrochemical detection of neuromodulator concentration can help to optimize tVNS parameters for a personalized intervention in different pathologies (Mirza et al., 2019). Bringing together both, stimulation optimization and high-speed detection of neuromodulator release, will help to dissect the complex brain state dependence seen in human studies. It is worth noting that using neurotransmitter concentration as an output variable for stimulation parameter optimization can be easily implemented in animal models, with the advantage of multiple recordings in different regions simultaneously and high-density channel recordings (Zhang et al., 2018; Tomagra et al., 2019). Yet, due to its invasive nature, application in humans is precluded, which emphasizes the need for preclinical research on non-human primates.
A unique opportunity in animal research compared to humans will be the dissection of afferent and efferent pathways on a cellular and molecular level. Early retrograde tracing studies have helped us to understand how the auricular branch of the VN innervates brainstem nuclei (Jacquin et al., 1982; Takemura et al., 1987). The auriculotemporal nerve and auricular branch of the VN are thought to predominantly project to the NTS, dorsal vagal nucleus, motor nucleus of the VN, and paratrigeminal nucleus. A picture emerged where most innervation to these nuclei show a strong ipsilateral profile, although the area postrema is a notable exception, as it has bilateral innervation (Kalia and Sullivan, 1982). Despite these pioneering studies, new genetic and viral approaches in animals will continue to unlock the main cellular connectivity pathways involved in tVNS (Nassi et al., 2015). Such connectivity schemes are likely to guide mechanistic approaches to optimally stimulate neuromodulatory systems and better anticipate off-targets effects. It is worthwhile to note that the auricular branch of the VN stimulation zone is innervated by sympathetic nerves as well. It has been therefore suggested that several sympathetic pathways could be stimulated while stimulating the auricular branch of the VN, which might lead to an activation of the NTS via the LC (Cakmak, 2019). This suggestion is novel since unidirectional NTS to LC activation is usually considered (Cakmak, 2019). On the other hand, there is accumulating evidence showing that the LC itself is not a functional neuroanatomical unit, but instead has multiple modules that differ in their projection targets and activity dynamics (Chandler et al., 2019). For example, circuits analysis using viral tracing, optogenetics, and chemogenetics have unraveled specific LC modules/circuits involved in analgesia, explorative behavior, or aversive learning modulation (Hirschberg et al., 2017; Borodovitsyna et al., 2018; Chandler et al., 2019). Moreover, it has been shown that NA is released in the hippocampus after 0.5 mA current stimulation but not in the cortex, while both structures are flooded by the neuromodulator when threshold current crosses more than 1.0 mA (Roosevelt et al., 2006). This exciting finding highlights: (1) the possibility of targeting different networks based on stimulation parameters and (2) the importance of understanding susceptibility of sub-circuits within the noradrenergic system regarding the stimulation parameters as well as different pathologies or brain states.
Animal research will reveal complex neuroanatomical connectivity implicated in taVNS with LC/NTS modularity. As a corollary, researchers will have to keep in mind that parameter optimization should be tuned specifically to the disease or brain state to be modulated, taking into account specific functional neuroanatomy. High throughput recording techniques such as calcium imaging and high-density in vivo electrophysiology coupled to molecular genetics and viral tracing will be needed together in this quest (Nassi et al., 2015; Schwarz et al., 2015; Totah et al., 2019). In addition, high-density channel electrochemical methods, behavioral studies and specific transgenic models of disease will also be required to provide a general view on tVNS/taVNS effects at the organismic level during normal conditions and in disease (Zhang et al., 2016, 2018; Tomagra et al., 2019; Vázquez-Oliver et al., 2020).
Conclusions
Given that the VN has been implicated in the pathophysiology of a number of disorders across many disciplines and phenomena on a behavioral and psychological level, VNS, and particularly non-invasive tVNS, has generated considerable interest. Whilst the mechanisms by which tVNS exerts psychological and physiological effects are increasingly, and more completely, understood, many early studies have been beset by inconsistencies around reporting. The development of internationally agreed consensus guidelines around reporting of tVNS studies should address these issues. Whilst tVNS represents a potential treatment option in many disorders and an interesting tool for experimental research, it needs to be studied in an objective and robust manner before its true place as a neuroimmunomodulatory intervention can be determined.
Author's Note
If you are interested in being part of the tVNS consensus group and our efforts to strengthen tVNS research methodology, please contact the corresponding author. Finding consensus among so many authors involved is certainly a challenge. We therefore like these recommendations to be considered as work in progress.
Author Contributions
All authors listed have made a substantial, direct and intellectual contribution to the work, and approved it for publication.
Funding
The specific work presented here, did not receive any funding. DH is supported by the CBBS NeuroNetzwerk 17, SFB (Sonderforschungsbereich) 1315, Project B6, Research Training Group 2413 ‘SynAge’ TP12, and ARUK SRF2018B-004. DQ acknowledges support from the Novo Nordisk Foundation (Excellence grant: NNF16OC0019856) and the Research Council of Norway (301767). VN acknowledges financial support via the US National Institutes for Health (NIH), Office of the Director (OT2-OD023867 to VN); National Center for Complementary and Integrative Health (NCCIH), NIH (P01-AT009965 to VN).
Conflict of Interest
EK and SK are employed by company SzeleSTIM GmbH. JS received honoraria from SzeleSTIM GmbH and owns patents in the field of the auricular vagus nerve stimulation. EK, SK, and JS are shareholders of SzeleSTIM GmbH.
The remaining authors declare that the research was conducted in the absence of any commercial or financial relationships that could be construed as a potential conflict of interest.
The reviewer TS declared a shared affiliation, with no collaboration, with one of the authors HJ, to the handling editor at the time of review.
Footnotes
1. ^The review of studies is based on a PubMed search using the keywords “transcutaneous vagus nerve stimulation” OR “tVNS”
References
Afanasiev, S. A., Pavliukova, E. N., Kuzmichkina, M. A., Rebrova, T. Y., Anfinogenova, Y., Likhomanov, K. S., et al. (2016). Nonpharmacological correction of hypersympatheticotonia in patients with chronic coronary insufficiency and severe left ventricular dysfunction. Ann. Noninvasive Electrocardiol. 21, 548–556. doi: 10.1111/anec.12349
Aihua, L., Lu, S., Liping, L., Xiuru, W., Hua, L., and Yuping, W. (2014). A controlled trial of transcutaneous vagus nerve stimulation for the treatment of pharmacoresistant epilepsy. Epilepsy Behav. 39, 105–110. doi: 10.1016/j.yebeh.2014.08.005
Alexander, G. M., Huang, Y. Z., Soderblom, E. J., He, X.-P., Moseley, M. A., and McNamara, J. O. (2017). Vagal nerve stimulation modifies neuronal activity and the proteome of excitatory synapses of amygdala/piriform cortex. J. Neurochem. 140, 629–644. doi: 10.1111/jnc.13931
Allchin, R. E., Batten, T. F., McWilliam, P. N., and Vaughan, P. F. (1994). Electrical stimulation of the vagus increases extracellular glutamate recovered from the nucleus tractus solitarii of the cat by in vivo microdialysis. Exp. Physiol. 79, 265–268. doi: 10.1113/expphysiol.1994.sp003761
Antonino, D., Teixeira, A. L., Maia-Lopes, P. M., Souza, M. C., Sabino-Carvalho, J. L., Murray, A. R., et al. (2017). Non-invasive vagus nerve stimulation acutely improves spontaneous cardiac baroreflex sensitivity in healthy young men: a randomized placebo-controlled trial. Brain Stimul. 10, 875–881. doi: 10.1016/j.brs.2017.05.006
Aston-Jones, G., and Waterhouse, B. (2016). Locus coeruleus: from global projection system to adaptive regulation of behavior. Brain Res. 1645, 75–78. doi: 10.1016/j.brainres.2016.03.001
Ay, I., Nasser, R., Simon, B., and Ay, H. (2016). Transcutaneous cervical vagus nerve stimulation ameliorates acute ischemic injury in rats. Brain Stimul. 9, 166–173. doi: 10.1016/j.brs.2015.11.008
Ay, I., Sorensen, A. G., and Ay, H. (2011). Vagus nerve stimulation reduces infarct size in rat focal cerebral ischemia: an unlikely role for cerebral blood flow. Brain Res. 1392, 110–115. doi: 10.1016/j.brainres.2011.03.060
Badran, B. W., Brown, J. C., Dowdle, L. T., Mithoefer, O. J., LaBate, N. T., Coatsworth, J., et al. (2018a). Tragus or cymba conchae? Investigating the anatomical foundation of transcutaneous auricular vagus nerve stimulation (taVNS). Brain Stimul. 11, 947–948. doi: 10.1016/j.brs.2018.06.003
Badran, B. W., Dowdle, L. T., Mithoefer, O. J., LaBate, N. T., Coatsworth, J., Brown, J. C., et al. (2018b). Neurophysiologic effects of transcutaneous auricular vagus nerve stimulation (taVNS) via electrical stimulation of the tragus: a concurrent taVNS/fMRI study and review. Brain Stimul. 11, 492–500. doi: 10.1016/j.brs.2017.12.009
Badran, B. W., Jenkins, D. D., Cook, D., Thompson, S., Dancy, M., DeVries, W. H., et al. (2020). Transcutaneous auricular vagus nerve stimulation-paired rehabilitation for oromotor feeding problems in newborns: an open-label pilot study. Front. Hum. Neurosci. 14:77. doi: 10.3389/fnhum.2020.00077
Badran, B. W., Mithoefer, O. J., Summer, C. E., LaBate, N. T., Glusman, C. E., Badran, A. W., et al. (2018c). Short trains of transcutaneous auricular vagus nerve stimulation (taVNS) have parameter-specific effects on heart rate. Brain Stimul. 11, 699–708. doi: 10.1016/j.brs.2018.04.004
Badran, B. W., Yu, A. B., Adair, D., Mappin, G., DeVries, W. H., Jenkins, D. D., et al. (2019). Laboratory administration of transcutaneous auricular vagus nerve stimulation (taVNS): technique, targeting, and considerations. J. Visual. Exp. 143:984. doi: 10.3791/58984
Banni, S., Carta, G., Murru, E., Cordeddu, L., Giordano, E., Marrosu, F., et al. (2012). Vagus nerve stimulation reduces body weight and fat mass in rats. PLoS ONE. 7:e44813. doi: 10.1371/journal.pone.0044813
Barbanti, P., Grazzi, L., Egeo, G., Padovan, A. M., Liebler, E., and Bussone, G. (2015). Non-invasive vagus nerve stimulation for acute treatment of high-frequency and chronic migraine: an open-label study. J. Headache Pain 16:61. doi: 10.1186/s10194-015-0542-4
Barbella, G., Cocco, I., Freri, E., Marotta, G., Visani, E., Franceschetti, S., et al. (2018). Transcutaneous vagal nerve stimulatio (t-VNS): an adjunctive treatment option for refractory epilepsy. Seizure 60, 115–119. doi: 10.1016/j.seizure.2018.06.016
Bauer, S., Baier, H., Baumgartner, C., Bohlmann, K., Fauser, S., Graf, W., et al. (2016). Transcutaneous vagus nerve stimulation (tVNS) for treatment of drug-resistant epilepsy: a randomized, double-blind clinical trial (cMPsE02). Brain Stimul. 9, 356–363. doi: 10.1016/j.brs.2015.11.003
Beaumont, E., Campbell, R. P., Andresen, M. C., Scofield, S., Singh, K., Libbus, I., et al. (2017). Cervical vagus nerve stimulation augments spontaneous discharge in second- and higher-order sensory neurons in the rat nucleus of the solitary tract. Am. J. Physiol Heart Circ. Physiol. 313, H354–H367. doi: 10.1152/ajpheart.00070.2017
Beste, C., Steenbergen, L., Sellaro, R., Grigoriadou, S., Zhang, R., Chmielewski, W., et al. (2016). Effects of concomitant stimulation of the GABAergic and norepinephrine system on inhibitory control—a study using transcutaneous vagus nerve stimulation. Brain Stimul. 9, 811–818. doi: 10.1016/j.brs.2016.07.004
Betts, M. J., Cardenas-Blanco, A., Kanowski, M., Jessen, F., and Düzel, E. (2017). In vivo MRI assessment of the human locus coeruleus along its rostrocaudal extent in young and older adults. Neuroimage 163, 150–159. doi: 10.1016/j.neuroimage.2017.09.042
Betts, M. J., Kirilina, E., Otaduy, M. C. G., Ivanov, D., Acosta-Cabronero, J., Callaghan, M. F., et al. (2019). Locus coeruleus imaging as a biomarker for noradrenergic dysfunction in neurodegenerative diseases. Brain 142, 2558–2571. doi: 10.1093/brain/awz193
Bianca, R., and Komisaruk, B. R. (2007). Pupil dilatation in response to vagal afferent electrical stimulation is mediated by inhibition of parasympathetic outflow in the rat. Brain Res. 1177, 29–36. doi: 10.1016/j.brainres.2007.06.104
Bikson, M., Esmaeilpour, Z., Adair, D., Kronberg, G., Tyler, W. J., Antal, A., et al. (2019). Transcranial electrical stimulation nomenclature. Brain Stimul. 12, 1349–1366. doi: 10.1016/j.brs.2019.07.010
Bonaz, B., Picq, C., Sinniger, V., Mayol, J. F., and Clarençon, D. (2013). Vagus nerve stimulation: from epilepsy to the cholinergic anti-inflammatory pathway. Neurogastroenterol. Motil. 25, 208–221. doi: 10.1111/nmo.12076
Boon, P., De Cock, E., Mertens, A., and Trinka, E. (2018). Neurostimulation for drug-resistant epilepsy: a systematic review of clinical evidence for efficacy, safety, contraindications and predictors for response. Curr. Opin. Neurol. 31, 198–210. doi: 10.1097/WCO.0000000000000534
Borges, U., Knops, L., Laborde, S., Klatt, S., and Raab, M. (2020). Transcutaneous vagus nerve stimulation may enhance only specific aspects of the core executive functions. A randomized crossover trial. Front. Neurosci. 14:523. doi: 10.3389/fnins.2020.00523
Borges, U., Laborde, S., and Raab, M. (2019). Influence of transcutaneous vagus nerve stimulation on cardiac vagal activity: not different from sham stimulation and no effect of stimulation intensity. PLoS ONE 14:e0223848. doi: 10.1371/journal.pone.0223848
Borodovitsyna, O., Flamini, M. D., and Chandler, D. J. (2018). Acute stress persistently alters locus coeruleus function and anxiety-like behavior in adolescent rats. Neuroscience 373, 7–19. doi: 10.1016/j.neuroscience.2018.01.020
Brack, K. E., Coote, J. H., and Ng, G. A. (2004). Interaction between direct sympathetic and vagus nerve stimulation on heart rate in the isolated rabbit heart. Exp. Physiol. 89, 128–139. doi: 10.1113/expphysiol.2003.002654
Brázdil, M., DoleŽalová, I., Koritáková, E., Chládek, J., Roman, R., Pail, M., et al. (2019). EEG Reactivity predicts individual efficacy of vagal nerve stimulation in intractable epileptics. Front. Neurol. 10:392. doi: 10.3389/fneur.2019.00392
Bretherton, B., Atkinson, L., Murray, A., Clancy, J., Deuchars, S., and Deuchars, J. (2019). Effects of transcutaneous vagus nerve stimulation in individuals aged 55 years or above: potential benefits of daily stimulation. Aging 11, 4836–4857. doi: 10.18632/aging.102074
Brock, C., Brock, B., Aziz, Q., Møller, H. J., Pfeiffer Jensen, M., Drewes, A. M., et al. (2017). Transcutaneous cervical vagal nerve stimulation modulates cardiac vagal tone and tumor necrosis factor-alpha. Neurogastroenterol. Motil. 29:e12999. doi: 10.1111/nmo.12999
Brooks, C. M., and Lange, G. (1977). Interaction of myogenic and neurogenic mechanisms that control heart rate. Proc. Natl. Acad. Sci. U.S.A. 74, 1761–1762. doi: 10.1073/pnas.74.4.1761
Brooks, J. C. W., Faull, O. K., Pattinson, K. T. S., and Jenkinson, M. (2013). Physiological noise in brainstem fMRI. Front. Hum. Neurosci. 7:623. doi: 10.3389/fnhum.2013.00623
Brown, G. L., and Eccles, J. C. (1934). The action of a single vagal volley on the rhythm of the heart beat. J. Physiol. 82, 211–241. doi: 10.1113/jphysiol.1934.sp003176
Burger, A. M., D'Agostini, M., Verkuil, B., and Diest, I. V. (2020a). Moving beyond belief: a narrative review of potential biomarkers for transcutaneous vagus nerve stimulation. Psychophysiology 57:e13571. doi: 10.1111/psyp.13571
Burger, A. M., Diest, I. V., Does, W. V., der Hysaj, M., Thayer, J. F., Brosschot, J. F., et al. (2018). Transcutaneous vagus nerve stimulation and extinction of prepared fear: a conceptual non-replication. Sci. Rep. 8:11471. doi: 10.1038/s41598-018-29561-w
Burger, A. M., Van der Does, W., Brosschot, J. F., and Verkuil, B. (2020b). From ear to eye? No effect of transcutaneous vagus nerve stimulation on human pupil dilation: a report of three studies. Biol. Psychol. 152:107863. doi: 10.1016/j.biopsycho.2020.107863
Burger, A. M., Van der Does, W., Thayer, J. F., Brosschot, J. F., and Verkuil, B. (2019a). Transcutaneous vagus nerve stimulation reduces spontaneous but not induced negative thought intrusions in high worriers. Biol. Psychol. 142, 80–89. doi: 10.1016/j.biopsycho.2019.01.014
Burger, A. M., Van Diest, I., Van der Does, W., Korbee, J. N., Waziri, N., Brosschot, J. F., et al. (2019b). The effect of transcutaneous vagus nerve stimulation on fear generalization and subsequent fear extinction. Neurobiol. Learn. Mem. 161, 192–201. doi: 10.1016/j.nlm.2019.04.006
Burger, A. M., and Verkuil, B. (2018). Transcutaneous nerve stimulation via the tragus: are we really stimulating the vagus nerve? Brain Stimul. 11, 945–946. doi: 10.1016/j.brs.2018.03.018
Burger, A. M., Verkuil, B., Fenlon, H., Thijs, L., Cools, L., Miller, H. C., et al. (2017). Mixed evidence for the potential of non-invasive transcutaneous vagal nerve stimulation to improve the extinction and retention of fear. Behav. Res. Ther. 97, 64–74. doi: 10.1016/j.brat.2017.07.005
Burger, A. M., Verkuil, B., Van Diest, I., Van der Does, W., Thayer, J. F., and Brosschot, J. F. (2016). The effects of transcutaneous vagus nerve stimulation on conditioned fear extinction in humans. Neurobiol. Learn. Mem. 132, 49–56. doi: 10.1016/j.nlm.2016.05.007
Burneo, J. G., Faught, E., Knowlton, R., Morawetz, R., and Kuzniecky, R. (2002). Weight loss associated with vagus nerve stimulation. Neurology 59, 463–464. doi: 10.1212/WNL.59.3.463
Busch, V., Zeman, F., Heckel, A., Menne, F., Ellrich, J., and Eichhammer, P. (2013). The effect of transcutaneous vagus nerve stimulation on pain perception – an experimental study. Brain Stimul. 6, 202–209. doi: 10.1016/j.brs.2012.04.006
Butson, C. R., and McIntyre, C. C. (2005). Tissue and electrode capacitance reduce neural activation volumes during deep brain stimulation. Clin. Neurophysiol. 116, 2490–2500. doi: 10.1016/j.clinph.2005.06.023
Butt, M. F., Albusoda, A., Farmer, A. D., and Aziz, Q. (2020). The anatomical basis for transcutaneous auricular vagus nerve stimulation. J. Anat. 236, 588–611. doi: 10.1111/joa.13122
Cakmak, Y. O., Apaydin, H., Kiziltan, G., Gunduz, A., Ozsoy, B., Urey, H., et al. (2017). Rapid alleviation of parkinson's disease symptoms via electrostimulation of intrinsic auricular muscle zones. Front. Hum. Neurosci. 11:338. doi: 10.3389/fnhum.2017.00338
Cakmak, Y. O. (2019). Concerning auricular vagal nerve stimulation: occult neural networks. Front. Hum. Neurosci. 13:421. doi: 10.3389/fnhum.2019.00421
Capone, F., Assenza, G., Di Pino, G., Musumeci, G., Ranieri, F., Florio, L., et al. (2015). The effect of transcutaneous vagus nerve stimulation on cortical excitability. J. Neural Transm. 122, 679–685. doi: 10.1007/s00702-014-1299-7
Capone, F., Miccinilli, S., Pellegrino, G., Zollo, L., Simonetti, D., Bressi, F., et al. (2017). Transcutaneous vagus nerve stimulation combined with robotic rehabilitation improves upper limb function after stroke. Neural Plasticity 2017:7876507. doi: 10.1155/2017/7876507
Cha, W. W., Song, K., and Lee, H. Y. (2016). Persistent geotropic direction-changing positional nystagmus treated with transcutaneous vagus nerve stimulation. Brain Stimul. 9, 469–470. doi: 10.1016/j.brs.2016.03.011
Chakravarthy, K., Chaudhry, H., Williams, K., and Christo, P. J. (2015). Review of the uses of vagal nerve stimulation in chronic pain management. Curr. Pain Headache Rep. 19:54. doi: 10.1007/s11916-015-0528-6
Chandler, D. J., Jensen, P., McCall, J. G., Pickering, A. E., Schwarz, L. A., and Totah, N. K. (2019). Redefining noradrenergic neuromodulation of behavior: impacts of a modular locus coeruleus architecture. J. Neurosci. 39, 8239–8249. doi: 10.1523/JNEUROSCI.1164-19.2019
Chen, M., Yu, L., Ouyang, F., Liu, Q., Wang, Z., Wang, S., et al. (2015). The right side or left side of noninvasive transcutaneous vagus nerve stimulation: based on conventional wisdom or scientific evidence? Int. J. Cardiol. 187, 44–45. doi: 10.1016/j.ijcard.2015.03.351
Clancy, J. A., Mary, D. A., Witte, K. K., Greenwood, J. P., Deuchars, S. A., and Deuchars, J. (2014). Non-invasive vagus nerve stimulation in healthy humans reduces sympathetic nerve activity. Brain Stimul. 7, 871–877. doi: 10.1016/j.brs.2014.07.031
Colzato, L. S., Ritter, S. M., and Steenbergen, L. (2018a). Transcutaneous vagus nerve stimulation (tVNS) enhances divergent thinking. Neuropsychologia 111, 72–76. doi: 10.1016/j.neuropsychologia.2018.01.003
Colzato, L. S., Sellaro, R., and Beste, C. (2017). Darwin revisited: the vagus nerve is a causal element in controlling recognition of other's emotions. Cortex 92, 95–102. doi: 10.1016/j.cortex.2017.03.017
Colzato, L. S., Wolters, G., and Peifer, C. (2018b). Transcutaneous vagus nerve stimulation (tVNS) modulates flow experience. Exp. Brain Res. 236, 253–257. doi: 10.1007/s00221-017-5123-0
Cork, S. C. (2018). The role of the vagus nerve in appetite control: implications for the pathogenesis of obesity. J. Neuroendocrinol. 30:e12643. doi: 10.1111/jne.12643
Cristancho, P., Cristancho, M. A., Baltuch, G. H., Thase, M. E., and O'Reardon, J. P. (2011). Effectiveness and safety of vagus nerve stimulation for severe treatment-resistant major depression in clinical practice after FDA approval: outcomes at 1 year. J. Clin. Psychiatry 72, 1376–1382. doi: 10.4088/JCP.09m05888blu
De Couck, M., Cserjesi, R., Caers, R., Zijlstra, W. P., Widjaja, D., Wolf, N., et al. (2017). Effects of short and prolonged transcutaneous vagus nerve stimulation on heart rate variability in healthy subjects. Auton. Neurosci. 203, 88–96. doi: 10.1016/j.autneu.2016.11.003
De Ferrari, G. M., and Schwartz, P. J. (2011). Vagus nerve stimulation: from pre-clinical to clinical application: challenges and future directions. Heart Fail. Rev. 16, 195–203. doi: 10.1007/s10741-010-9216-0
De Icco, R., Martinelli, D., Bitetto, V., Fresia, M., Liebler, E., Sandrini, G., et al. (2018). Peripheral vagal nerve stimulation modulates the nociceptive withdrawal reflex in healthy subjects: a randomized, cross-over, sham-controlled study. Cephalalgia 38, 1658–1664. doi: 10.1177/0333102417742347
de Lartigue, G. (2016). Role of the vagus nerve in the development and treatment of diet-induced obesity. J. Physiol. 594, 5791–5815. doi: 10.1113/JP271538
De Ridder, D., Vanneste, S., Engineer, N. D., and Kilgard, M. P. (2014). Safety and efficacy of vagus nerve stimulation paired with tones for the treatment of tinnitus: a case series. Neuromodulation 17, 170–179. doi: 10.1111/ner.12127
De Taeye, L., Vonck, K., van Bochove, M., Boon, P., Van Roost, D., Mollet, L., et al. (2014). The P3 event-related potential is a biomarker for the efficacy of vagus nerve stimulation in patients with epilepsy. Neurotherapeutics 11, 612–622. doi: 10.1007/s13311-014-0272-3
Desbeaumes Jodoin, V., Richer, F., Miron, J.-P., Fournier-Gosselin, M.-P., and Lespérance, P. (2018). Long-term sustained cognitive benefits of vagus nerve stimulation in refractory depression. J. ECT 34, 283–290. doi: 10.1097/YCT.0000000000000502
Dietrich, S., Smith, J., Scherzinger, C., Hofmann-Preiß, K., Freitag, T., Eisenkolb, A., et al. (2008). A novel transcutaneous vagus nerve stimulation leads to brainstem and cerebral activations measured by functional MRI / funktionelle magnetresonanztomographie zeigt aktivierungen des hirnstamms und weiterer zerebraler strukturen unter transkutaner vagusnervstimulation. Biomed. Tech/Biomed. Eng. 53, 104–111. doi: 10.1515/BMT.2008.022
Dorr, A. E., and Debonnel, G. (2006). Effect of vagus nerve stimulation on serotonergic and noradrenergic transmission. J. Pharmacol. Exp. Ther. 318, 890–898. doi: 10.1124/jpet.106.104166
Du, X. J., Dart, A. M., and Riemersma, R. A. (1994). Sex differences in the parasympathetic nerve control of rat heart. Clin. Exp. Pharmacol. Physiol. 21, 485–493. doi: 10.1111/j.1440-1681.1994.tb02545.x
Ehlert, U., Erni, K., Hebisch, G., and Nater, U. (2006). Salivary alpha-amylase levels after yohimbine challenge in healthy men. J. Clin. Endocrinol. Metab. 91, 5130–5133. doi: 10.1210/jc.2006-0461
Ellrich, J. (2011). Transcutaneous vagus nerve stimulation. Eur. Neurol. Rev. 6, 254–256. doi: 10.17925/ENR.2011.06.04.254
Falkenberg, L. E., Westerhausen, R., Specht, K., and Hugdahl, K. (2012). Resting-state glutamate level in the anterior cingulate predicts blood-oxygen level-dependent response to cognitive control. Proc. Natl Acad. Sci. U. S. A. 109, 5069–5073. doi: 10.1073/pnas.1115628109
Fallgatter, A. J., Ehlis, A.-C., Ringel, T. M., and Herrmann, M. J. (2005). Age effect on far field potentials from the brain stem after transcutaneous vagus nerve stimulation. Int. J. Psychophysiol. 56, 37–43. doi: 10.1016/j.ijpsycho.2004.09.007
Fallgatter, A. J., Neuhauser, B., Herrmann, M. J., Ehlis, A.-C., Wagener, A., Scheuerpflug, P., et al. (2003). Far field potentials from the brain stem after transcutaneous vagus nerve stimulation. J. Neural Transm. 110, 1437–1443. doi: 10.1007/s00702-003-0087-6
Fang, J., Egorova, N., Rong, P., Liu, J., Hong, Y., Fan, Y., et al. (2017). Early cortical biomarkers of longitudinal transcutaneous vagus nerve stimulation treatment success in depression. Neuroimage Clin. 14, 105–111. doi: 10.1016/j.nicl.2016.12.016
Fang, J., Rong, P., Hong, Y., Fan, Y., Liu, J., Wang, H., et al. (2016). Transcutaneous vagus nerve stimulation modulates default mode network in major depressive disorder. Biol. Psychiatry 79, 266–273. doi: 10.1016/j.biopsych.2015.03.025
Ferrari, G. M. D., Crijns, H. J. G. M., Borggrefe, M., Milasinovic, G., Smid, J., Zabel, M., et al. (2011). Chronic vagus nerve stimulation: a new and promising therapeutic approach for chronic heart failure. Eur. Heart J. 32, 847–855. doi: 10.1093/eurheartj/ehq391
Finisguerra, A., Crescentini, C., and Urgesi, C. (2019). Transcutaneous vagus nerve stimulation affects implicit spiritual self-representations. Neuroscience 412, 144–159. doi: 10.1016/j.neuroscience.2019.05.059
Fischer, R., Ventura-Bort, C., Hamm, A., and Weymar, M. (2018). Transcutaneous vagus nerve stimulation (tVNS) enhances conflict-triggered adjustment of cognitive control. Cogn. Affect. Behav. Neurosci. 18, 680–693. doi: 10.3758/s13415-018-0596-2
Follesa, P., Biggio, F., Gorini, G., Caria, S., Talani, G., Dazzi, L., et al. (2007). Vagus nerve stimulation increases norepinephrine concentration and the gene expression of BDNF and bFGF in the rat brain. Brain Res. 1179, 28–34. doi: 10.1016/j.brainres.2007.08.045
Frangos, E., Ellrich, J., and Komisaruk, B. R. (2015). Non-invasive access to the vagus nerve central projections via electrical stimulation of the external ear: FMRI evidence in humans. Brain Stimul. 8, 624–636. doi: 10.1016/j.brs.2014.11.018
Frangos, E., and Komisaruk, B. R. (2017). Access to vagal projections via cutaneous electrical stimulation of the neck: FMRI evidence in healthy humans. Brain Stimul. 10, 19–27. doi: 10.1016/j.brs.2016.10.008
Frøkjaer, J. B., Bergmann, S., Brock, C., Madzak, A., Farmer, A. D., Ellrich, J., et al. (2016). Modulation of vagal tone enhances gastroduodenal motility and reduces somatic pain sensitivity. Neurogastroenterol. Motil. 28, 592–598. doi: 10.1111/nmo.12760
Gancheva, S., Bierwagen, A., Markgraf, D. F., Bönhof, G. J., Murphy, K. G., Hatziagelaki, E., et al. (2018). Constant hepatic ATP concentrations during prolonged fasting and absence of effects of cerbomed nemos® on parasympathetic tone and hepatic energy metabolism. Mol. Metab. 7, 71–79. doi: 10.1016/j.molmet.2017.10.002
Garcia, R. G., Lin, R. L., Lee, J., Kim, J., Barbieri, R., Sclocco, R., et al. (2017). Modulation of brainstem activity and connectivity by respiratory-gated auricular vagal afferent nerve stimulation in migraine patients. Pain 158, 1461–1472. doi: 10.1097/j.pain.0000000000000930
Gaul, C., Diener, H.-C., Silver, N., Magis, D., Reuter, U., Andersson, A., et al. (2016). Non-invasive vagus nerve stimulation for PREVention and Acute treatment of chronic cluster headache (PREVA): a randomised controlled study. Cephalalgia 36, 534–546. doi: 10.1177/0333102415607070
Gee, J. W., de Knapen, T., and Donner, T. H. (2014). Decision-related pupil dilation reflects upcoming choice and individual bias. Proc. Natl. Acad. Sci. U.S.A. 111, E618–E625. doi: 10.1073/pnas.1317557111
Genheimer, H., Andreatta, M., Asan, E., and Pauli, P. (2017). Reinstatement of contextual conditioned anxiety in virtual reality and the effects of transcutaneous vagus nerve stimulation in humans. Sci. Rep. 7:17886. doi: 10.1038/s41598-017-18183-3
Gidron, Y., Deschepper, R., De Couck, M., Thayer, J. F., and Velkeniers, B. (2018). The vagus nerve can predict and possibly modulate non-communicable chronic diseases: introducing a neuroimmunological paradigm to public health. J. Clin. Med. 7:371. doi: 10.3390/jcm7100371
Gil, K., Bugajski, A., and Thor, P. (2011). Electrical vagus nerve stimulation decreases food consumption and weight gain in rats fed a high-fat diet. J. Physiol. Pharmacol. 62, 637–646.
Giraudier, M., Ventura-Bort, C., and Weymar, M. (2020). Transcutaneous vagus nerve stimulation (tVNS) improves high confidence recognition memory but not emotional word processing. Front. Psychol. 11:1276. doi: 10.3389/fpsyg.2020.01276
Goadsby, P. J., Grosberg, B. M., Mauskop, A., Cady, R., and Simmons, K. A. (2014). Effect of noninvasive vagus nerve stimulation on acute migraine: an open-label pilot study. Cephalalgia 34, 986–993. doi: 10.1177/0333102414524494
Goldberger, J. J., Arora, R., Buckley, U., and Shivkumar, K. (2019). Autonomic nervous system dysfunction. J. Am. College Cardiol. 73:64. doi: 10.1016/j.jacc.2018.12.064
Gourine, A. V., Dale, N., Korsak, A., Llaudet, E., Tian, F., Huckstepp, R., et al. (2008). Release of ATP and glutamate in the nucleus tractus solitarii mediate pulmonary stretch receptor (Breuer–Hering) reflex pathway. J. Physiol. 586, 3963–3978. doi: 10.1113/jphysiol.2008.154567
Groves, D. A., Bowman, E. M., and Brown, V. J. (2005). Recordings from the rat locus coeruleus during acute vagal nerve stimulation in the anaesthetised rat. Neurosci. Lett. 379, 174–179. doi: 10.1016/j.neulet.2004.12.055
Guleyupoglu, B., Schestatsky, P., Edwards, D., Fregni, F., and Bikson, M. (2013). Classification of methods in transcranial electrical stimulation (tES) and evolving strategy from historical approaches to contemporary innovations. J. Neurosci. Methods 219, 297–311. doi: 10.1016/j.jneumeth.2013.07.016
Hämmerer, D., Callaghan, M. F., Hopkins, A., Kosciessa, J., Betts, M., Cardenas-Blanco, A., et al. (2018). Locus coeruleus integrity in old age is selectively related to memories linked with salient negative events. Proc. Natl. Acad. Sci. U.S.A. 115, 2228–2233. doi: 10.1073/pnas.1712268115
Hansen, N. (2019). Memory reinforcement and attenuation by activating the human locus coeruleus via transcutaneous vagus nerve stimulation. Front. Neurosci. 12:955. doi: 10.3389/fnins.2018.00955
Harden, C. L., Pulver, M. C., Ravdin, L. D., Nikolov, B., Halper, J. P., and Labar, D. R. (2000). A pilot study of mood in epilepsy patients treated with vagus nerve stimulation. Epilepsy Behav. 1, 93–99. doi: 10.1006/ebeh.2000.0046
Hasan, A., Wolff-Menzler, C., Pfeiffer, S., Falkai, P., Weidinger, E., Jobst, A., et al. (2015). Transcutaneous noninvasive vagus nerve stimulation (tVNS) in the treatment of schizophrenia: a bicentric randomized controlled pilot study. Eur. Arch. Psychiatry Clin. Neurosci. 265, 589–600. doi: 10.1007/s00406-015-0618-9
He, W., Jing, X., Wang, X., Rong, P., Li, L., Shi, H., et al. (2013a). Transcutaneous auricular vagus nerve stimulation as a complementary therapy for pediatric epilepsy: a pilot trial. Epilepsy Behav. 28, 343–346. doi: 10.1016/j.yebeh.2013.02.001
He, W., Jing, X.-H., Zhu, B., Zhu, X.-L., Li, L., Bai, W.-Z., et al. (2013b). The auriculo-vagal afferent pathway and its role in seizure suppression in rats. BMC Neurosci. 14:85. doi: 10.1186/1471-2202-14-85
Heien, M. L. A. V., Johnson, M. A., and Wightman, R. M. (2004). Resolving neurotransmitters detected by fast-scan cyclic voltammetry. Anal. Chem. 76, 5697–5704. doi: 10.1021/ac0491509
Hein, E., Nowak, M., Kiess, O., Biermann, T., Bayerlein, K., Kornhuber, J., et al. (2013). Auricular transcutaneous electrical nerve stimulation in depressed patients: a randomized controlled pilot study. J. Neural Transm. 120, 821–827. doi: 10.1007/s00702-012-0908-6
Hirschberg, S., Li, Y., Randall, A., Kremer, E. J., and Pickering, A. E. (2017). Functional dichotomy in spinal- vs prefrontal-projecting locus coeruleus modules splits descending noradrenergic analgesia from ascending aversion and anxiety in rats. ELife 6:e29808. doi: 10.7554/eLife.29808.027
Homma, S., Yamazaki, Y., and Karakida, T. (1993). Blood pressure and heart rate relationships during cervical sympathetic and vagus nerve stimulation in streptozotocin diabetic rats. Brain Res. 629, 342–344. doi: 10.1016/0006-8993(93)91343-Q
Hong, G.-S., Pintea, B., Lingohr, P., Coch, C., Randau, T., Schaefer, N., et al. (2019). Effect of transcutaneous vagus nerve stimulation on muscle activity in the gastrointestinal tract (transVaGa): a prospective clinical trial. Int. J. Colorectal Dis. 34, 417–422. doi: 10.1007/s00384-018-3204-6
Hosoi, T., Okuma, Y., and Nomura, Y. (2000). Electrical stimulation of afferent vagus nerve induces IL-1beta expression in the brain and activates HPA axis. Am. J. Physiol. Regul. Integr. Comp. Physiol. 279, R141–R147. doi: 10.1152/ajpregu.2000.279.1.R141
Hou, P. W., Hsu, H. C., Lin, Y. W., Tang, N. Y., Cheng, C. Y., and Hsieh, C. L. (2015). The history mechanism, and clinical application of auricular therapy in traditional Chinese medicine. Evid Based Complement. Alternat. Med. 2015:495684. doi: 10.1155/2015/495684
Howland, R. H. (2014). Vagus nerve stimulation. Curr. Behav. Neurosci. Rep. 1, 64–73. doi: 10.1007/s40473-014-0010-5
Huang, F., Dong, J., Kong, J., Wang, H., Meng, H., Spaeth, R. B., et al. (2014). Effect of transcutaneous auricular vagus nerve stimulation on impaired glucose tolerance: a pilot randomized study. BMC Complement. Alternat. Med. 14:203. doi: 10.1186/1472-6882-14-203
Huang, J., Wang, Y., Jiang, D., Zhou, J., and Huang, X. (2010). The sympathetic-vagal balance against endotoxemia. J. Neural Transm. 117, 729–735. doi: 10.1007/s00702-010-0407-6
Huffman, W. J., Subramaniyan, S., Rodriguiz, R. M., Wetsel, W. C., Grill, W. M., and Terrando, N. (2019). Modulation of neuroinflammation and memory dysfunction using percutaneous vagus nerve stimulation in mice. Brain Stimul. 12, 19–29. doi: 10.1016/j.brs.2018.10.005
Hulsey, D. R., Riley, J. R., Loerwald, K. W., Rennaker, R. L., Kilgard, M. P., and Hays, S. A. (2017). Parametric characterization of neural activity in the locus coeruleus in response to vagus nerve stimulation. Exp. Neurol. 289, 21–30. doi: 10.1016/j.expneurol.2016.12.005
Huston, J. M., Gallowitsch-Puerta, M., Ochani, M., Ochani, K., Yuan, R., Rosas-Ballina, M., et al. (2007). Transcutaneous vagus nerve stimulation reduces serum high mobility group box 1 levels and improves survival in murine sepsis. Crit.Care Med. 35, 2762–2768. doi: 10.1097/01.CCM.0000288102.15975.BA
Hyvärinen, P., Yrttiaho, S., Lehtimäki, J., Ilmoniemi, R. J., Mäkitie, A., Ylikoski, J., et al. (2015). Transcutaneous vagus nerve stimulation modulates tinnitus-related beta- and gamma-band activity. Ear Hear. 36, e76–e85. doi: 10.1097/AUD.0000000000000123
Ikramuddin, S., Blackstone, R. P., Brancatisano, A., Toouli, J., Shah, S. N., Wolfe, B. M., et al. (2014). Effect of reversible intermittent intra-abdominal vagal nerve blockade on morbid obesity: the recharge randomized clinical trial. JAMA 312, 915–922. doi: 10.1001/jama.2014.10540
Iseger, T. A., van Bueren, N. E. R., Kenemans, J. L., Gevirtz, R., and Arns, M. (2020). A frontal-vagal network theory for major depressive disorder: implications for optimizing neuromodulation techniques. Brain Stimul. 13, 1–9. doi: 10.1016/j.brs.2019.10.006
Jacobs, H. I. L., Riphagen, J. M., Razat, C. M., Wiese, S., and Sack, A. T. (2015). Transcutaneous vagus nerve stimulation boosts associative memory in older individuals. Neurobiol. Aging 36, 1860–1867. doi: 10.1016/j.neurobiolaging.2015.02.023
Jacquin, M. F., Semba, K., Rhoades, R. W., and Egger, M. D. (1982). Trigeminal primary afferents project bilaterally to dorsal horn and ipsilaterally to cerebellum, reticular formation, and cuneate, solitary, supratrigeminal and vagal nuclei. Brain Res. 246, 285–291. doi: 10.1016/0006-8993(82)91177-5
Jalife, J., Slenter, V. A., Salata, J. J., and Michaels, D. C. (1983). Dynamic vagal control of pacemaker activity in the mammalian sinoatrial node. Circ. Res. 52, 642–656. doi: 10.1161/01.RES.52.6.642
Janner, H., Klausenitz, C., Gürtler, N., Hahnenkamp, K., and Usichenko, T. I. (2018). Effects of electrical transcutaneous vagus nerve stimulation on the perceived intensity of repetitive painful heat stimuli: a blinded placebo- and sham-controlled randomized crossover investigation. Anesthesia Analgesia 126, 2085–2092. doi: 10.1213/ANE.0000000000002820
Jiang, Y., Li, L., Ma, J., Zhang, L., Niu, F., Feng, T., et al. (2016). Auricular vagus nerve stimulation promotes functional recovery and enhances the post-ischemic angiogenic response in an ischemia/reperfusion rat model. Neurochem. Int. 97, 73–82. doi: 10.1016/j.neuint.2016.02.009
Jin, Y., and Kong, J. (2016). Transcutaneous vagus nerve stimulation: a promising method for treatment of autism spectrum disorders. Front. Neurosci. 10:609. doi: 10.3389/fnins.2016.00609
Jodoin, V. D., Lespérance, P., Nguyen, D. K., Fournier-Gosselin, M.-P., Richer, F., and Centre Hospitalier de l'Université de Montréal, Canada. (2018). Effects of vagus nerve stimulation on pupillary function. Int. J. Psychophysiol. 98(3 Pt 1), 455–459. doi: 10.1016/j.ijpsycho.2015.10.001
John, C. E., and Jones, S. R. (2007). “Fast scan cyclic voltammetry of dopamine and serotonin in mouse brain slices,” in Electrochemical Methods for Neuroscience, eds A. C. Michael and L. M. Borland (Francis:CRC Press/Taylor). Available onlie at: http://www.ncbi.nlm.nih.gov/books/NBK2579/
Johnson, R. L., and Wilson, C. G. (2018). A review of vagus nerve stimulation as a therapeutic intervention. J. Inflamm. Res. 11, 203–213. doi: 10.2147/JIR.S163248
Jongkees, B. J., Immink, M. A., Finisguerra, A., and Colzato, L. S. (2018). Transcutaneous vagus nerve stimulation (tVNS) enhances response selection during sequential action. Front. Psychol. 9:1159. doi: 10.3389/fpsyg.2018.01159
Joshi, S., Li, Y., Kalwani, R. M., and Gold, J. I. (2016). Relationships between pupil diameter and neuronal activity in the locus coeruleus, colliculi, and cingulate cortex. Neuron 89, 221–234. doi: 10.1016/j.neuron.2015.11.028
Juel, J., Brock, C., Olesen, S., Madzak, A., Farmer, A., Aziz, Q., et al. (2017). Acute physiological and electrical accentuation of vagal tone has no effect on pain or gastrointestinal motility in chronic pancreatitis. J. Pain Res. 10, 1347–1355. doi: 10.2147/JPR.S133438
Kaczmarczyk, R., Tejera, D., Simon, B. J., and Heneka, M. T. (2017). Microglia modulation through external vagus nerve stimulation in a murine model of Alzheimer's disease. J. Neurochem. 146, 76–85. doi: 10.1111/jnc.14284
Kalia, M., and Sullivan, J. M. (1982). Brainstem projections of sensory and motor components of the vagus nerve in the rat. J. Comp. Neurol. 211, 248–265. doi: 10.1002/cne.902110304
Kampusch, S., Kaniusas, E., and Széles, J. C. (2013). “New approaches in multi-punctual percutaneous stimulation of the auricular vagus nerve,” in 2013 6th International IEEE/EMBS Conference on Neural Engineering (NER) (San Diego, CA), 263–266. doi: 10.1109/NER.2013.6695922
Kaniusas, E. (2019). Biomedical Signals and Sensors III: Linking Electric Biosignals and Biomedical Sensors. Available online at: https://www.springer.com/de/book/9783319749167
Kaniusas, E., Kampusch, S., Tittgemeyer, M., Panetsos, F., Gines, R. F., Papa, M., et al. (2019a). Current directions in the auricular vagus nerve stimulation II – an engineering perspective. Front. Neurosci. 13:772. doi: 10.3389/fnins.2019.00772
Kaniusas, E., Kampusch, S., Tittgemeyer, M., Panetsos, F., Gines, R. F., Papa, M., et al. (2019b). Current directions in the auricular vagus nerve stimulation I – a physiological perspective. Front. Neurosci. 13:854. doi: 10.3389/fnins.2019.00854
Kaniusas, E., Samoudi, A. M., Kampusch, S., Bald, K., Tanghe, E., Martens, L., et al. (2020). Stimulation pattern efficiency in percutaneous auricular vagus nerve stimulation: experimental versus numerical data. IEEE Trans. Biomed. Eng. 67, 1921–1935. doi: 10.1109/TBME.2019.2950777
Kemp, J., Després, O., Pebayle, T., and Dufour, A. (2014). Age-related decrease in sensitivity to electrical stimulation is unrelated to skin conductance: an evoked potentials study. Clin. Neurophysiol. 125, 602–607. doi: 10.1016/j.clinph.2013.08.020
Keute, M., Boehrer, L., Ruhnau, P., Heinze, H.-J., and Zaehle, T. (2019a). Transcutaneous vagus nerve stimulation (tVNS) and the dynamics of visual bistable perception. Front. Neurosci. 13:227. doi: 10.3389/fnins.2019.00227
Keute, M., Demirezen, M., Graf, A., Mueller, N. G., and Zaehle, T. (2019b). No modulation of pupil size and event-related pupil response by transcutaneous auricular vagus nerve stimulation (taVNS). Sci. Rep. 9:11452. doi: 10.1038/s41598-019-47961-4
Keute, M., Ruhnau, P., Heinze, H.-J., and Zaehle, T. (2018). Behavioral and electrophysiological evidence for GABAergic modulation through transcutaneous vagus nerve stimulation. Clin. Neurophysiol. 129, 1789–1795. doi: 10.1016/j.clinph.2018.05.026
Khadka, N., Borges, H., Zannou, A. L., Jang, J., Kim, B., Lee, K., et al. (2018). Dry tDCS: Tolerability of a novel multilayer hydrogel composite non-adhesive electrode for transcranial direct current stimulation. Brain Stimul. 11, 1044–1053. doi: 10.1016/j.brs.2018.07.049
Kile, B. M., Walsh, P. L., McElligott, Z. A., Bucher, E. S., Guillot, T. S., Salahpour, A., et al. (2012). Optimizing the temporal resolution of fast-scan cyclic voltammetry. ACS Chem. Neurosci. 3, 285–292. doi: 10.1021/cn200119u
Koenig, J., Parzer, P., Haigis, N., Liebemann, J., Jung, T., Resch, F., et al. (2019). Effects of acute transcutaneous vagus nerve stimulation on emotion recognition in adolescent depression. Psychol. Med. 1–10. doi: 10.1017/S0033291719003490. [Epub ahead of print].
Koenig, J., Rash, J. A., Campbell, T. S., Thayer, J. F., and Kaess, M. (2017). A meta-analysis on sex differences in resting-state vagal activity in children and adolescents. Front. Physiol. 8:582. doi: 10.3389/fphys.2017.00582
Koenig, J., and Thayer, J. F. (2016). Sex differences in healthy human heart rate variability: a meta-analysis. Neurosci. Biobehav. Rev. 64, 288–310. doi: 10.1016/j.neubiorev.2016.03.007
Kong, J., Fang, J., Park, J., Li, S., and Rong, P. (2018). Treating depression with transcutaneous auricular vagus nerve stimulation: state of the art and future perspectives. Front. Psychiatry 9:20. doi: 10.3389/fpsyt.2018.00020
Koopman, F. A., Chavan, S. S., Miljko, S., Grazio, S., Sokolovic, S., Schuurman, P. R., et al. (2016). Vagus nerve stimulation inhibits cytokine production and attenuates disease severity in rheumatoid arthritis. Proc. Natl. Acad. Sci. U.S.A. 113, 8284–8289. doi: 10.1073/pnas.1605635113
Krahl, S. E., and Clark, K. B. (2012). Vagus nerve stimulation for epilepsy: a review of central mechanisms. Surg. Neurol. Int. 3, S255–S259. doi: 10.4103/2152-7806.103015
Krahl, S. E., Senanayake, S. S., and Handforth, A. (2003). Right-sided vagus nerve stimulation reduces generalized seizure severity in rats as effectively as left-sided. Epilepsy Res. 56, 1–4. doi: 10.1016/s0920-1211(03)00122-0
Kraus, T., Hösl, K., Kiess, O., Schanze, A., Kornhuber, J., and Forster, C. (2007). BOLD fMRI deactivation of limbic and temporal brain structures and mood enhancing effect by transcutaneous vagus nerve stimulation. J. Neural Transm. 114, 1485–1493. doi: 10.1007/s00702-007-0755-z
Kraus, T., Kiess, O., Hösl, K., Terekhin, P., Kornhuber, J., and Forster, C. (2013). CNS BOLD fMRI effects of sham-controlled transcutaneous electrical nerve stimulation in the left outer auditory canal – a pilot study. Brain Stimul. 6, 798–804. doi: 10.1016/j.brs.2013.01.011
Krause, B., and Cohen Kadosh, R. (2014). Not all brains are created equal: the relevance of individual differences in responsiveness to transcranial electrical stimulation. Front. Syst. Neurosci. 8:25. doi: 10.3389/fnsys.2014.00025
Kreuzer, P. M., Landgrebe, M., Husser, O., Resch, M., Schecklmann, M., Geisreiter, F., et al. (2012). Transcutaneous vagus nerve stimulation: retrospective assessment of cardiac safety in a pilot study. Front. Psychiatry 3:70. doi: 10.3389/fpsyt.2012.00070
Kreuzer, P. M., Landgrebe, M., Resch, M., Husser, O., Schecklmann, M., Geisreiter, F., et al. (2014). Feasibility, safety and efficacy of transcutaneous vagus nerve stimulation in chronic tinnitus: an open pilot study. Brain Stimul. 7, 740–747. doi: 10.1016/j.brs.2014.05.003
Kuo, T. B., Lin, T., Yang, C. C., Li, C. L., Chen, C. F., and Chou, P. (1999). Effect of aging on gender differences in neural control of heart rate. Am. J. Physiol. 277, H2233–H2239. doi: 10.1152/ajpheart.1999.277.6.H2233
Kuo, T. B. J., Lai, C. J., Huang, Y.-T., and Yang, C. C. H. (2005). Regression analysis between heart rate variability and baroreflex-related vagus nerve activity in rats. J. Cardiovasc. Electrophysiol. 16, 864–869. doi: 10.1111/j.1540-8167.2005.40656.x
Lamb, D. G., Porges, E. C., Lewis, G. F., and Williamson, J. B. (2017). Non-invasive vagal nerve stimulation effects on hyperarousal and autonomic state in patients with posttraumatic stress disorder and history of mild traumatic brain injury: preliminary evidence. Front. Med. 4:124. doi: 10.3389/fmed.2017.00124
Lange, G., Janal, M. N., Maniker, A., Fitzgibbons, J., Fobler, M., Cook, D., et al. (2011). Safety and efficacy of vagus nerve stimulation in fibromyalgia: a phase I/II proof of concept trial. Pain Med. 12, 1406–1413. doi: 10.1111/j.1526-4637.2011.01203.x
Lanska, D. J. (2002). Corning and vagal nerve stimulation for seizures in the 1880s. Neurology 58, 452–459. doi: 10.1212/WNL.58.3.452
Laqua, R., Leutzow, B., Wendt, M., and Usichenko, T. (2014). Transcutaneous vagal nerve stimulation may elicit anti- and pro-nociceptive effects under experimentally-induced pain—a crossover placebo-controlled investigation. Auton. Neurosci. Basic Clin. 185, 120–122. doi: 10.1016/j.autneu.2014.07.008
Lehtimäki, J., Hyvärinen, P., Ylikoski, M., Bergholm, M., Mäkel,ä, J. P., Aarnisalo, A., et al. (2013). Transcutaneous vagus nerve stimulation in tinnitus: a pilot study. Acta Oto Laryngol. 133, 378–382. doi: 10.3109/00016489.2012.750736
Lerman, I., Hauger, R., Sorkin, L., Proudfoot, J., Davis, B., Huang, A., et al. (2016). Noninvasive transcutaneous vagus nerve stimulation decreases whole blood culture-derived cytokines and chemokines: a randomized, blinded, healthy control pilot trial: noninvasive vagus nerve stimulation modulates peripheral inflammation. Neuromodulation 19, 283–290. doi: 10.1111/ner.12398
Leutzow, B., Lange, J., Gibb, A., Schroeder, H., Nowak, A., Wendt, M., et al. (2013). Vagal sensory evoked potentials disappear under the neuromuscular block – an experimental study. Brain Stimul. 6, 812–816. doi: 10.1016/j.brs.2013.03.005
Levine, Y. A., Koopman, F., Faltys, M., Zitnik, R., and Tak, P.-P. (2014). Neurostimulation of the cholinergic antiinflammatory pathway in rheumatoid arthritis and inflammatory bowel disease. Bioelectron. Med. 1, 34–43. doi: 10.15424/bioelectronmed.2014.00008
Levy, M. N., Martin, P. J., Lano, T., and Zieske, H. (1969). Paradoxical effect of vagus nerve stimulation on heart rate in dogs. Circ. Res. 25, 303–314. doi: 10.1161/01.RES.25.3.303
Lewine, J. D., Paulson, K., Bangera, N., and Simon, B. J. (2019). Exploration of the impact of brief noninvasive vagal nerve stimulation on EEG and event-related potentials: impact of nVNS on brain electrophysiology. Neuromodulation 22, 564–572. doi: 10.1111/ner.12864
Liporace, J., Hucko, D., Morrow, R., Barolat, G., Nei, M., Schnur, J., et al. (2001). Vagal nerve stimulation: adjustments to reduce painful side effects. Neurology 57, 885–886. doi: 10.1212/WNL.57.5.885
Liu, J., Fang, J., Wang, Z., Rong, P., Hong, Y., Fan, Y., et al. (2016). Transcutaneous vagus nerve stimulation modulates amygdala functional connectivity in patients with depression. J. Affect. Disord. 205, 319–326. doi: 10.1016/j.jad.2016.08.003
Liu, K. Y., Acosta-Cabronero, J., Cardenas-Blanco, A., Loane, C., Berry, A. J., Betts, M. J., et al. (2019). In vivo visualization of age-related differences in the locus coeruleus. Neurobiol. Aging 74, 101–111. doi: 10.1016/j.neurobiolaging.2018.10.014
Liu, K. Y., Marijatta, F., Hämmerer, D., Acosta-Cabronero, J., Düzel, E., and Howard, R. J. (2017). Magnetic resonance imaging of the human locus coeruleus: a systematic review. Neurosci. Biobehav. Rev. 83, 325–355. doi: 10.1016/j.neubiorev.2017.10.023
Liugan, M., Zhang, M., and Cakmak, Y. O. (2018). Neuroprosthetics for auricular muscles: neural networks and clinical aspects. Front. Neurol. 8:752. doi: 10.3389/fneur.2017.00752
Lv, H., Zhao, Y., Chen, J., Wang, D., and Chen, H. (2019). Vagus nerve stimulation for depression: a systematic review. Front. Psychol. 10:64. doi: 10.3389/fpsyg.2019.00064
Maffiuletti, N. A., Herrero, A. J., Jubeau, M., Impellizzeri, F. M., and Bizzini, M. (2008). Differences in electrical stimulation thresholds between men and women. Ann. Neurol. 63, 507–512. doi: 10.1002/ana.21346
Malik, M. (1996). Heart rate variability. Standards of measurement, physiological interpretation, and clinical use: task force of the European Society of Cardiology and the North American Society for Pacing and Electrophysiology. Annals Noninv. Electrocardiol. 1, 151–181. doi: 10.1111/j.1542-474X.1996.tb00275.x
Manta, S., Dong, J., Debonnel, G., and Blier, P. (2009). Enhancement of the function of rat serotonin and norepinephrine neurons by sustained vagus nerve stimulation. J. Psychiatry Neurosci. 34, 272–280.
Manta, S., El Mansari, M., Debonnel, G., and Blier, P. (2013). Electrophysiological and neurochemical effects of long-term vagus nerve stimulation on the rat monoaminergic systems. Int. J. Neuropsychopharmacol. 16, 459–470. doi: 10.1017/S1461145712000387
Marrosu, F., Serra, A., Maleci, A., Puligheddu, M., Biggio, G., and Piga, M. (2003). Correlation between GABAA receptor density and vagus nerve stimulation in individuals with drug-resistant partial epilepsy. Epilepsy Res. 55, 59–70. doi: 10.1016/S0920-1211(03)00107-4
McGough, J. J., Sturm, A., Cowen, J., Tung, K., Salgari, G. C., Leuchter, A. F., et al. (2019). Double-blind, sham-controlled, pilot study of trigeminal nerve stimulation for attention-deficit/hyperactivity disorder. J. Am. Acad. Child Adolesc. Psychiatry 58, 403–411.e3. doi: 10.1016/j.jaac.2018.11.013
Merrill, D. R., Bikson, M., and Jefferys, J. G. R. (2005). Electrical stimulation of excitable tissue: design of efficacious and safe protocols. J. Neurosci. Methods 141, 171–198. doi: 10.1016/j.jneumeth.2004.10.020
Mertens, A., Naert, L., Miatton, M., Poppa, T., Carrette, E., Gadeyne, S., et al. (2020). Transcutaneous vagus nerve stimulation does not affect verbal memory performance in healthy volunteers. Front. Psychol. 11:551. doi: 10.3389/fpsyg.2020.00551
Mertens, A., Raedt, R., Gadeyne, S., Carrette, E., Boon, P., and Vonck, K. (2018). Recent advances in devices for vagus nerve stimulation. Expert Rev. Med. Devices 15, 527–539. doi: 10.1080/17434440.2018.1507732
Minhas, P., Bansal, V., Patel, J., Ho, J. S., Diaz, J., Datta, A., et al. (2010). Electrodes for high-definition transcutaneous DC stimulation for applications in drug delivery and electrotherapy, including tDCS. J. Neurosci. Methods 190, 188–197. doi: 10.1016/j.jneumeth.2010.05.007
Mirza, K. B., Golden, C. T., Nikolic, K., and Toumazou, C. (2019). Closed-loop implantable therapeutic neuromodulation systems based on neurochemical monitoring. Front. Neurosci. 13:808. doi: 10.3389/fnins.2019.00808
Moher D. Schulz K. F. Altman D. CONSORT Group Consolidated Standards of Reporting Trials (2001). The CONSORT statement: revised recommendations for improving the quality of reports of parallel-group randomized trials. JAMA 285, 1987–1991. doi: 10.1001/jama.285.15.1987
Moodithaya, S., and Avadhany, S. T. (2012). Gender differences in age-related changes in cardiac autonomic nervous function. J. Aging Res. 2012:679345. doi: 10.1155/2012/679345
Morris, G. L., Gloss, D., Buchhalter, J., Mack, K. J., Nickels, K., and Harden, C. (2013). Evidence-based guideline update: vagus nerve stimulation for the treatment of epilepsy. Epilepsy Curr. 13, 297–303. doi: 10.5698/1535-7597-13.6.297
Morris, J., Straube, A., Diener, H.-C., Ahmed, F., Silver, N., Walker, S., et al. (2016). Cost-effectiveness analysis of non-invasive vagus nerve stimulation for the treatment of chronic cluster headache. J. Headache Pain 17:43. doi: 10.1186/s10194-016-0633-x
Mourdoukoutas, A. P., Truong, D. Q., Adair, D. K., Simon, B. J., and Bikson, M. (2018). High-resolution multi-scale computational model for non-invasive cervical vagus nerve stimulation. Neuromodulation 21, 261–268. doi: 10.1111/ner.12706
Mridha, Z., de Gee, J. W., Shi, Y., Alkashgari, R., Williams, J., Suminski, A., et al. (2019). Graded recruitment of pupil-linked neuromodulation by parametric stimulation of the vagus nerve | bioRxiv [Preprint]. doi: 10.1101/2019.12.28.890111
Murphy, P. R., Robertson, I. H., Balsters, J. H., and O'connell, R. G. (2011). Pupillometry and P3 index the locus coeruleus-noradrenergic arousal function in humans. Psychophysiology 48, 1532–1543. doi: 10.1111/j.1469-8986.2011.01226.x
Murray, A. R., Atkinson, L., Mahadi, M. K., Deuchars, S. A., and Deuchars, J. (2016a). The strange case of the ear and the heart: the auricular vagus nerve and its influence on cardiac control. Auton. Neurosci. Basic Clin. 199, 48–53. doi: 10.1016/j.autneu.2016.06.004
Murray, A. R., Clancy, J. A., Deuchars, S. A., and Deuchars, J. (2016b). Transcutaneous vagus nerve stimulation (tVNS) decreases sympathetic nerve activity in older healthy human subjects. FASEB J. 30(1 Suppl), 754.3. doi: 10.1136/heartjnl-2016-309890.215
Napadow, V. (2019). When a white horse is a horse: embracing the overlap between acupuncture and neuromodulation. J. Altern. Complement. Med. 24, 621–623. doi: 10.1089/acm.2018.29047.vtn
Napadow, V., Edwards, R. R., Cahalan, C. M., Mensing, G., Greenbaum, S., Valovska, A., et al. (2012). Evoked pain analgesia in chronic pelvic pain patients using respiratory-gated auricular vagal afferent nerve stimulation. Pain Med. 13, 777–789. doi: 10.1111/j.1526-4637.2012.01385.x
Nassi, J. J., Cepko, C. L., Born, R. T., and Beier, K. T. (2015). Neuroanatomy goes viral! Front. Neuroanat. 9:80. doi: 10.3389/fnana.2015.00080
Nemeroff, C. B., Mayberg, H. S., Krahl, S. E., McNamara, J., Frazer, A., Henry, T. R., et al. (2006). VNS therapy in treatment-resistant depression: clinical evidence and putative neurobiological mechanisms. Neuropsychopharmacology 31, 1345–1355. doi: 10.1038/sj.npp.1301082
Nesbitt, A. D., Marin, J. C. A., Tompkins, E., Ruttledge, M. H., and Goadsby, P. J. (2015). Initial use of a novel noninvasive vagus nerve stimulator for cluster headache treatment. Neurology 84, 1249–1253. doi: 10.1212/WNL.0000000000001394
Neuhaus, A. H., Luborzewski, A., Rentzsch, J., Brakemeier, E. L., Opgen-Rhein, C., Gallinat, J., et al. (2007). P300 is enhanced in responders to vagus nerve stimulation for treatment of major depressive disorder. J. Affect. Disord. 100, 123–128. doi: 10.1016/j.jad.2006.10.005
Neuser, M. P., Teckentrup, V., Kühnel, A., Hallschmid, M., Walter, M., and Kroemer, N. B. (2019). Vagus nerve stimulation increases vigor to work for rewards. BioRxiv [Preprint]. 789982. doi: 10.1101/789982
Ng, G. A., Brack, K. E., and Coote, J. H. (2001). Effects of direct sympathetic and vagus nerve stimulation on the physiology of the whole heart—a novel model of isolated langendorff perfused rabbit heart with intact dual autonomic innervation. Exp. Physiol. 86, 319–329. doi: 10.1113/eph8602146
Nieuwenhuis, S., Aston-Jones, G., and Cohen, J. D. (2005). Decision making, the P3, and the locus coeruleus-norepinephrine system. Psychol. Bull. 131, 510–532. doi: 10.1037/0033-2909.131.4.510
Njagi, J., Chernov, M. M., Leiter, J. C., and Andreescu, S. (2010). Amperometric detection of dopamine in vivo with an enzyme based carbon fiber microbiosensor. Anal. Chem. 82, 989–996. doi: 10.1021/ac9022605
Noller, C. M., Levine, Y. A., Urakov, T. M., Aronson, J. P., and Nash, M. S. (2019). Vagus nerve stimulation in rodent models: an overview of technical considerations. Front. Neurosci. 13:911. doi: 10.3389/fnins.2019.00911
Paleczny, B., Seredyński, R., and Ponikowska, B. (2019). Inspiratory- and expiratory-gated transcutaneous vagus nerve stimulation have different effects on heart rate in healthy subjects: preliminary results. Clin. Auton. Res. doi: 10.1007/s10286-019-00604-0. [Epub ahead of print].
Panebianco, M., Zavanone, C., Dupont, S., Restivo, D. A., and Pavone, A. (2016). Vagus nerve stimulation therapy in partial epilepsy: a review. Acta Neurol. Belgica 116, 241–248. doi: 10.1007/s13760-016-0616-3
Pardo, J., Sheikh, S., Kuskowski, M., Surerus-Johnson, C., Hagen, M., Lee, J., et al. (2007). Weight loss during chronic, cervical vagus nerve stimulation in depressed patients with obesity. Int. J. Obesity 31, 1756–1759. doi: 10.1038/sj.ijo.0803666
Pavlov, V. A., and Tracey, K. J. (2012). The vagus nerve and the inflammatory reflex–linking immunity and metabolism. Nat. Rev. Endocrinol. 8, 743–754. doi: 10.1038/nrendo.2012.189
Peng, L., Mu, K., Liu, A., Zhou, L., Gao, Y., Shenoy, I. T., et al. (2018). Transauricular vagus nerve stimulation at auricular acupoints kindey (CO10), yidan (CO11), liver (CO12) and shenmen (TF4) can induce auditory and limbic cortices activation measured by fMRI. Hear. Res. 359, 1–12. doi: 10.1016/j.heares.2017.12.003
Penry, J. K., and Dean, J. C. (1990). Prevention of intractable partial seizures by intermittent vagal stimulation in humans: preliminary results. Epilepsia 31, S40–S43. doi: 10.1111/j.1528-1157.1990.tb05848.x
Perkins, D. O. (2002). Predictors of noncompliance in patients with schizophrenia. J. Clin. Psychiatry 63, 1121–1128. doi: 10.4088/JCP.v63n1206
Peterchev, A. V., Wagner, T. A., Miranda, P. C., Nitsche, M. A., Paulus, W., Lisanby, S. H., et al. (2012). Fundamentals of transcranial electric and magnetic stimulation dose: definition, selection, and reporting practices. Brain Stimul. 5, 435–453. doi: 10.1016/j.brs.2011.10.001
Peuker, E. T., and Filler, T. J. (2002). The nerve supply of the human auricle. Clin. Anat. 15, 35–37. doi: 10.1002/ca.1089
Polak, T., Markulin, F., Ehlis, A.-C., Langer, J. B. M., Ringel, T. M., and Fallgatter, A. J. (2009). Far field potentials from brain stem after transcutaneous vagus nerve stimulation: optimization of stimulation and recording parameters. J. Neural Transm. 116, 1237–1242. doi: 10.1007/s00702-009-0282-1
Premchand, R. K., Sharma, K., Mittal, S., Monteiro, R., Dixit, S., Libbus, I., et al. (2014). Autonomic regulation therapy via left or right cervical vagus nerve stimulation in patients with chronic heart failure: results of the ANTHEM-HF trial. J. Cardiac Failure 20, 808–816. doi: 10.1016/j.cardfail.2014.08.009
Priovoulos, N., Jacobs, H. I. L., Ivanov, D., Uludag, K., Verhey, F. R. J., and Poser, B. A. (2018). High-resolution in vivo imaging of human locus coeruleus by magnetization transfer MRI at 3T and 7T. Neuroimage 168, 427–436. doi: 10.1016/j.neuroimage.2017.07.045
Raedt, R., Clinckers, R., Mollet, L., Vonck, K., El Tahry, R., Wyckhuys, T., et al. (2011). Increased hippocampal noradrenaline is a biomarker for efficacy of vagus nerve stimulation in a limbic seizure model. J. Neurochem. 117, 461–469. doi: 10.1111/j.1471-4159.2011.07214.x
Rajkowski, J. (1993). Correlations between locus coeruleus (LC) neural activity, pupil diameter and behavior in monkey support a role of LC in attention. Soc. Neurosc. Available online at: https://ci.nii.ac.jp/naid/10021384962/en/
Rawat, J. K., Roy, S., Singh, M., Guatam, S., Yadav, R. K., Ansari, M. N., et al. (2019). Transcutaneous vagus nerve stimulation regulates the cholinergic anti-inflammatory pathway to counteract 1, 2-dimethylhydrazine induced colon carcinogenesis in albino wistar rats. Front. Pharmacol. 10:353. doi: 10.3389/fphar.2019.00353
Redgrave, J., Day, D., Leung, H., Laud, P. J., Ali, A., Lindert, R., et al. (2018). Safety and tolerability of transcutaneous vagus nerve stimulation in humans; a systematic review. Brain Stimul. 11, 1225–1238. doi: 10.1016/j.brs.2018.08.010
Rong, P., Liu, A., Zhang, J., Wang, Y., He, W., Yang, A., et al. (2014). Transcutaneous vagus nerve stimulation for refractory epilepsy: a randomized controlled trial. Clin. Sci. doi: 10.1042/CS20130518. [Epub ahead of print].
Rong, P., Liu, J., Wang, L., Liu, R., Fang, J., Zhao, J., et al. (2016). Effect of transcutaneous auricular vagus nerve stimulation on major depressive disorder: a nonrandomized controlled pilot study. J. Affect. Disord. 195, 172–179. doi: 10.1016/j.jad.2016.02.031
Roosevelt, R. W., Smith, D. C., Clough, R. W., Jensen, R. A., and Browning, R. A. (2006). Increased extracellular concentrations of norepinephrine in cortex and hippocampus following vagus nerve stimulation in the rat. Brain Res. 1119, 124–132. doi: 10.1016/j.brainres.2006.08.048
Roslin, M., and Kurian, M. (2001). The use of electrical stimulation of the vagus nerve to treat morbid obesity. Epilepsy Behav. 2, S11–S16. doi: 10.1006/ebeh.2001.0213
Rufener, K. S., Geyer, U., Janitzky, K., Heinze, H.-J., and Zaehle, T. (2018). Modulating auditory selective attention by non-invasive brain stimulation: differential effects of transcutaneous vagal nerve stimulation and transcranial random noise stimulation. Eur. J. Neurosci. 48, 2301–2309. doi: 10.1111/ejn.14128
Salman, I. M. (2015). Cardiovascular autonomic dysfunction in chronic kidney disease: a comprehensive review. Curr. Hypertens. Rep. 17:59. doi: 10.1007/s11906-015-0571-z
Sasaki, M., Shibata, E., Tohyama, K., Takahashi, J., Otsuka, K., Tsuchiya, K., et al. (2006). Neuromelanin magnetic resonance imaging of locus ceruleus and substantia nigra in Parkinson's disease. Neuroreport 17, 1215–1218. doi: 10.1097/01.wnr.0000227984.84927.a7
Sator-Katzenschlager, S. M., Scharbert, G., Kozek-Langenecker, S. A., Szeles, J. C., Finster, G., Schiesser, A. W., et al. (2004). The short- and long-term benefit in chronic low back pain through adjuvant electrical versus manual auricular acupuncture. Anesthesia Analgesia 98, 1359–1364. doi: 10.1213/01.ANE.0000107941.16173.F7
Schevernels, H., van Bochove, M. E., De Taeye, L., Bombeke, K., Vonck, K., Van Roost, D., et al. (2016). The effect of vagus nerve stimulation on response inhibition. Epilepsy Behav. 64, 171–179. doi: 10.1016/j.yebeh.2016.09.014
Schulz-Stübner, S., and Kehl, F. (2011). Treatment of persistent hiccups with transcutaneous phrenic and vagal nerve stimulation. Intensive Care Med. 37, 1048–1049. doi: 10.1007/s00134-011-2150-3
Schwarz, L. A., Miyamichi, K., Gao, X. J., Beier, K. T., Weissbourd, B., DeLoach, K. E., et al. (2015). Viral-genetic tracing of the input–output organization of a central noradrenaline circuit. Nature 524, 88–92. doi: 10.1038/nature14600
Sclocco, R., Beissner, F., Bianciardi, M., Polimeni, J. R., and Napadow, V. (2018). Challenges and opportunities for brainstem neuroimaging with ultrahigh field MRI. Neuroimage 168, 412–426. doi: 10.1016/j.neuroimage.2017.02.052
Sclocco, R., Garcia, R. G., Gabriel, A., Kettner, N. W., Napadow, V., and Barbieri, R. (2017). “Respiratory-gated auricular vagal afferent nerve stimulation (RAVANS) effects on autonomic outflow in hypertension,” in 2017 39th Annual International Conference of the IEEE Engineering in Medicine and Biology Society (EMBC) (Jeju), 3130–3133. doi: 10.1109/EMBC.2017.8037520
Sclocco, R., Garcia, R. G., Kettner, N. W., Fisher, H. P., Isenburg, K., Makarovsky, M., et al. (2020). Stimulus frequency modulates brainstem response to respiratory-gated transcutaneous auricular vagus nerve stimulation. Brain Stimul. 13, 970–978. doi: 10.1016/j.brs.2020.03.011
Sclocco, R., Garcia, R. G., Kettner, N. W., Isenburg, K., Fisher, H. P., Hubbard, C. S., et al. (2019). The influence of respiration on brainstem and cardiovagal response to auricular vagus nerve stimulation: a multimodal ultrahigh-field (7T) fMRI study. Brain Stimul. 12, 911–921. doi: 10.1016/j.brs.2019.02.003
Sellaro, R., de Gelder, B., Finisguerra, A., and Colzato, L. S. (2018). Transcutaneous vagus nerve stimulation (tVNS) enhances recognition of emotions in faces but not bodies. Cortex 99, 213–223. doi: 10.1016/j.cortex.2017.11.007
Sellaro, R., Steenbergen, L., Verkuil, B., van IJzendoorn, M. H., and Colzato, L. S. (2015a). Transcutaneous vagus nerve stimulation (tVNS) does not increase prosocial behavior in cyberball. Front. Psychol. 6:499. doi: 10.3389/fpsyg.2015.00499
Sellaro, R., van Leusden, J. W. R., Tona, K.-D., Verkuil, B., Nieuwenhuis, S., and Colzato, L. S. (2015b). Transcutaneous vagus nerve stimulation enhances post-error slowing. J. Cogn. Neurosci. 27, 2126–2132. doi: 10.1162/jocn_a_00851
Shikora, S., Toouli, J., Herrera, M. F., Kulseng, B., Zulewski, H., Brancatisano, R., et al. (2013). Vagal blocking improves glycemic control and elevated blood pressure in obese subjects with type 2 diabetes mellitus. J. Obes. 2013:245683. doi: 10.1155/2013/245683
Shim, H. J., Kwak, M. Y., An, Y.-H., Kim, D. H., Kim, Y. J., and Kim, H. J. (2015). Feasibility and safety of transcutaneous vagus nerve stimulation paired with notched music therapy for the treatment of chronic tinnitus. J. Audiol. Otol. 19, 159–167. doi: 10.7874/jao.2015.19.3.159
Silberstein, S. D., Calhoun, A. H., Lipton, R. B., Grosberg, B. M., Cady, R. K., Dorlas, S., et al. (2016a). Chronic migraine headache prevention with noninvasive vagus nerve stimulation: the EVENT study. Neurology 87, 529–538. doi: 10.1212/WNL.0000000000002918
Silberstein, S. D., Mechtler, L. L., Kudrow, D. B., Calhoun, A. H., McClure, C., Saper, J. R., et al. (2016b). Non-invasive vagus nerve stimulation for the acute treatment of cluster headache: findings from the randomized, double-blind, sham-controlled ACT1 study. Headache 56, 1317–1332. doi: 10.1111/head.12896
Silvanto, J., Muggleton, N., and Walsh, V. (2008). State-dependency in brain stimulation studies of perception and cognition. Trends Cogn. Sci. 12, 447–454. doi: 10.1016/j.tics.2008.09.004
Slenter, V. A., Salata, J. J., and Jalife, J. (1984). Vagal control of pacemaker periodicity and intranodal conduction in the rabbit sinoatrial node. Circ. Res. 54, 436–446. doi: 10.1161/01.RES.54.4.436
Sooksood, K., Stieglitz, T., and Ortmanns, M. (2009). “Recent advances in charge balancing for functional electrical stimulation,” in Conference Proceedings: Annual International Conference of the IEEE Engineering in Medicine and Biology Society. IEEE Engineering in Medicine and Biology Society. Annual Conference (Minneapolis), 2009, 5518–5521. doi: 10.1109/IEMBS.2009.5333181
Sooksood, K., Stieglitz, T., and Ortmanns, M. (2010). An active approach for charge balancing in functional electrical stimulation. IEEE Trans. Biomed. Circ. Syst. 4, 162–170. doi: 10.1109/TBCAS.2010.2040277
Stavrakis, S., Humphrey, M. B., Scherlag, B. J., Hu, Y., Jackman, W. M., Nakagawa, H., et al. (2015). Low-level transcutaneous electrical vagus nerve stimulation suppresses atrial fibrillation. J. Am. Coll. Cardiol. 65, 867–875. doi: 10.1016/j.jacc.2014.12.026
Steenbergen, L., Colzato, L. S., and Maraver, M. J. (2020). Vagal signaling and the somatic marker hypothesis: the effect of transcutaneous vagal nerve stimulation on delay discounting is modulated by positive mood. Int. J. Psychophysiol. 148, 84–92. doi: 10.1016/j.ijpsycho.2019.10.010
Steenbergen, L., Sellaro, R., Stock, A.-K., Verkuil, B., Beste, C., and Colzato, L. S. (2015). Transcutaneous vagus nerve stimulation (tVNS) enhances response selection during action cascading processes. Eur. Neuropsychopharmacol. 25, 773–778. doi: 10.1016/j.euroneuro.2015.03.015
Stefan, H., Kreiselmeyer, G., Kerling, F., Kurzbuch, K., Rauch, C., Heers, M., et al. (2012). Transcutaneous vagus nerve stimulation (t-VNS) in pharmacoresistant epilepsies: a proof of concept trial. Epilepsia 53, e115–e118. doi: 10.1111/j.1528-1167.2012.03492.x
Straube, A., Ellrich, J., Eren, O., Blum, B., and Ruscheweyh, R. (2015). Treatment of chronic migraine with transcutaneous stimulation of the auricular branch of the vagal nerve (auricular t-VNS): A randomized, monocentric clinical trial. J. Headache Pain 16:543. doi: 10.1186/s10194-015-0543-3
Sun, P., Zhou, K., Wang, S., Li, P., Chen, S., Lin, G., et al. (2013). Involvement of MAPK/NF-κB signaling in the activation of the cholinergic anti-inflammatory pathway in experimental colitis by chronic vagus nerve stimulation. PLoS ONE 8:e69424. doi: 10.1371/journal.pone.0069424
Szeska, C., Richter, J., Wendt, J., Weymar, M., and Hamm, A. O. (2020). Promoting long-term inhibition of human fear responses by non-invasive transcutaneous vagus nerve stimulation during extinction training. Sci. Rep. 10:1529. doi: 10.1038/s41598-020-58412-w
Sztajzel, J., Jung, M., and Bayes de Luna, A. (2008). Reproducibility and gender-related differences of heart rate variability during all-day activity in young men and women. Ann. Noninvasive Electrocardiol. 13, 270–277. doi: 10.1111/j.1542-474X.2008.00231.x
Takemura, M., Sugimoto, T., and Sakai, A. (1987). Topographic organization of central terminal region of different sensory branches of the rat mandibular nerve. Exp. Neurol. 96, 540–557. doi: 10.1016/0014-4886(87)90217-2
Teckentrup, V., Neubert, S., Santiago, J. C. P., Hallschmid, M., Walter, M., and Kroemer, N. B. (2020). Non-invasive stimulation of vagal afferents reduces gastric frequency. Brain Stimul. 13, 470–473. doi: 10.1016/j.brs.2019.12.018
Tekdemir, I., Aslan, A., and Elhan, A. (1998). A clinico-anatomic study of the auricular branch of the vagus nerve and Arnold's ear-cough reflex. Surg. Radiol. Anat. 20, 253–257. doi: 10.1007/s00276-998-0253-5
Thayer, J. F., and Lane, R. D. (2000). A model of neurovisceral integration in emotion regulation and dysregulation. J. Affect. Disord. 61, 201–216. doi: 10.1016/S0165-0327(00)00338-4
Thayer, J. F., and Lane, R. D. (2009). Claude bernard and the heart-brain connection: further elaboration of a model of neurovisceral integration. Neurosci. Biobehav. Rev. 33, 81–88. doi: 10.1016/j.neubiorev.2008.08.004
Tobaldini, E., Toschi-Dias, E., Appratto de Souza, L., Rabello Casali, K., Vicenzi, M., Sandrone, G., et al. (2019). Cardiac and peripheral autonomic responses to orthostatic stress during transcutaneous vagus nerve stimulation in healthy subjects. J. Clin. Med. 8:496. doi: 10.3390/jcm8040496
Tomagra, G., Picollo, F., Battiato, A., Picconi, B., De Marchis, S., Pasquarelli, A., et al. (2019). Quantal release of dopamine and action potential firing detected in midbrain neurons by multifunctional diamond-based microarrays. Front. Neurosci. 13:288. doi: 10.3389/fnins.2019.00288
Tona, K.-D., Revers, H., Verkuil, B., and Nieuwenhuis, S. (2020). Noradrenergic regulation of cognitive flexibility: no effects of stress, transcutaneous vagus nerve stimulation, and atomoxetine on task-switching in humans. J. Cogn. Neurosci. 32:1881–1895. doi: 10.1162/jocn_a_01603
Totah, N. K. B., Logothetis, N. K., and Eschenko, O. (2019). Noradrenergic ensemble-based modulation of cognition over multiple timescales. Brain Res. 1709, 50–66. doi: 10.1016/j.brainres.2018.12.031
Tran, N., Asad, Z., Elkholey, K., Scherlag, B. J., Po, S. S., and Stavrakis, S. (2019). Autonomic neuromodulation acutely ameliorates left ventricular strain in humans. J. Cardiovasc. Transl. Res. 12, 221–230. doi: 10.1007/s12265-018-9853-6
Trujillo, P., Petersen, K. J., Cronin, M. J., Lin, Y.-C., Kang, H., Donahue, M. J., et al. (2019). Quantitative magnetization transfer imaging of the human locus coeruleus. Neuroimage 200, 191–198. doi: 10.1016/j.neuroimage.2019.06.049
Tu, Y., Fang, J., Cao, J., Wang, Z., Park, J., Jorgenson, K., et al. (2018). A distinct biomarker of continuous transcutaneous vagus nerve stimulation treatment in major depressive disorder. Brain Stimul. 11, 501–508. doi: 10.1016/j.brs.2018.01.006
Usichenko, T., Hacker, H., and Lotze, M. (2017a). Transcutaneous auricular vagal nerve stimulation (taVNS) might be a mechanism behind the analgesic effects of auricular acupuncture. Brain Stimul. 10, 1042–1044. doi: 10.1016/j.brs.2017.07.013
Usichenko, T., Laqua, R., Leutzow, B., and Lotze, M. (2017b). Preliminary findings of cerebral responses on transcutaneous vagal nerve stimulation on experimental heat pain. Brain Imaging Behav. 11, 30–37. doi: 10.1007/s11682-015-9502-5
Uthman, B. M., Wilder, B. J., Penry, J. K., Dean, C., Ramsay, R. E., Reid, S. A., et al. (1993). Treatment of epilepsy by stimulation of the vagus nerve. Neurology 43, 1338–1345. doi: 10.1212/WNL.43.7.1338
Val-Laillet, D., Biraben, A., Randuineau, G., and Malbert, C. H. (2010). Chronic vagus nerve stimulation decreased weight gain, food consumption and sweet craving in adult obese minipigs. Appetite 55, 245–252. doi: 10.1016/j.appet.2010.06.008
Valsalva, A. M. (1704). De Aura Humana Tractatus and Trajecti ad Rhenum Urecht. Utrecht:Trajecti ad Rhenum.
van Kempen, J., Loughnane, G. M., Newman, D. P., Kelly, S. P., Thiele, A., O'Connell, R. G., et al. (2019). Behavioural and neural signatures of perceptual decision-making are modulated by pupil-linked arousal. ELife 8:e42541. doi: 10.7554/eLife.42541
Van Leusden, J. W. R., Sellaro, R., and Colzato, L. S. (2015). Transcutaneous vagal nerve stimulation (tVNS): a new neuromodulation tool in healthy humans? Front. Psychol. 6:102. doi: 10.3389/fpsyg.2015.00102
Vanneste, S., Martin, J., Rennaker, R. L., and Kilgard, M. P. (2017). Pairing sound with vagus nerve stimulation modulates cortical synchrony and phase coherence in tinnitus: an exploratory retrospective study. Sci. Rep. 7:17345. doi: 10.1038/s41598-017-17750-y
Vargas Luna, J. L., Krenn, M., Cortés, J. A., and Mayr, W. (2013). Comparison of current and voltage control techniques for neuromuscular electrical stimulation in the anterior thigh. Biomed. Tech. 58:1–2. doi: 10.1515/bmt-2013-4021
Vázquez-Oliver, A., Brambilla-Pisoni, C., Domingo-Gainza, M., Maldonado, R., Ivorra, A., and Ozaita, A. (2020). Auricular transcutaneous vagus nerve stimulation improves memory persistence in naïve mice and in an intellectual disability mouse model. Brain Stimul. 13, 494–498. doi: 10.1016/j.brs.2019.12.024
Ventura-Bort, C., Wirkner, J., Genheimer, H., Wendt, J., Hamm, A. O., and Weymar, M. (2018). Effects of transcutaneous vagus nerve stimulation (tVNS) on the P300 and alpha-amylase level: a pilot study. Front. Hum. Neurosci. 12:202. doi: 10.3389/fnhum.2018.00202
Verkuil, B., and Burger, A. M. (2019). Transcutaneous vagus nerve stimulation does not affect attention to fearful faces in high worriers. Behav. Res. Ther. 113, 25–31. doi: 10.1016/j.brat.2018.12.009
Vieira, A., Reis, A. M., Matos, L. C., Machado, J., and Moreira, A. (2018). Does auriculotherapy have therapeutic effectiveness? an overview of systematic reviews. Complement. Ther. Clin. Pract. 33, 61–70. doi: 10.1016/j.ctcp.2018.08.005
von Elm, E., Altman, D. G., Egger, M., Pocock, S. J., Gøtzsche, P. C., Vandenbroucke, J. P., et al. (2008). The strengthening the reporting of observational studies in epidemiology (STROBE) statement: guidelines for reporting observational studies. J. Clin. Epidemiol. 61, 344–349. doi: 10.1016/j.jclinepi.2007.11.008
Wang, D.-W., Yin, Y.-M., and Yao, Y.-M. (2016). Vagal modulation of the inflammatory response in sepsis. Int. Rev. Immunol. 35, 415–433. doi: 10.3109/08830185.2015.1127369
Wang, Z., Zhou, X., Sheng, X., Yu, L., and Jiang, H. (2015a). Unilateral low-level transcutaneous electrical vagus nerve stimulation: a novel noninvasive treatment for myocardial infarction. Int. J. Cardiol. 190, 9–10. doi: 10.1016/j.ijcard.2015.04.087
Wang, Z., Zhou, X., Sheng, X., Yu, L., and Jiang, H. (2015b). Noninvasive vagal nerve stimulation for heart failure: was it practical or just a stunt? Int. J. Cardiol. 187, 637–638. doi: 10.1016/j.ijcard.2015.03.430
Wang, Z.engjian, Fang, J., Liu, J., Rong, P., Jorgenson, K., Park, J., Lang, C., et al. (2018). Frequency-dependent functional connectivity of the nucleus accumbens during continuous transcutaneous vagus nerve stimulation in major depressive disorder. J. Psychiatric Res. 102, 123–131. doi: 10.1016/j.jpsychires.2017.12.018
Warren, C. M., Tona, K. D., Ouwerkerk, L., van Paridon, J., Poletiek, F., van Steenbergen, H., et al. (2019). The neuromodulatory and hormonal effects of transcutaneous vagus nerve stimulation as evidenced by salivary alpha amylase, salivary cortisol, pupil diameter, and the P3 event-related potential. Brain Stimul. 12, 635–642. doi: 10.1016/j.brs.2018.12.224
Warren, C. M., van den Brink, R. L., Nieuwenhuis, S., and Bosch, J. A. (2017). Norepinephrine transporter blocker atomoxetine increases salivary alpha amylase. Psychoneuroendocrinology 78, 233–236. doi: 10.1016/j.psyneuen.2017.01.029
Weise, D., Adamidis, M., Pizzolato, F., Rumpf, J.-J., Fricke, C., and Classen, J. (2015). Assessment of brainstem function with auricular branch of vagus nerve stimulation in Parkinson's disease. PLoS ONE 10:e0120786. doi: 10.1371/journal.pone.0120786
Woodbury D. M. Woodbury J. W. (1990), Effects of vagal stimulation on experimentally induced seizures in rats. Epilepsia 31, S7–S19. doi: 10.1111/j.1528-1157.1990.tb05852.x
Woods, A. J., Antal, A., Bikson, M., Boggio, P. S., Brunoni, A. R., Celnik, P., et al. (2016). A technical guide to tDCS, and related non-invasive brain stimulation tools. Clin. Neurophysiol. 127, 1031–1048. doi: 10.1016/j.clinph.2015.11.012
Wostyn, S., Staljanssens, W., De Taeye, L., Strobbe, G., Gadeyne, S., Van Roost, D., et al. (2017). EEG derived brain activity reflects treatment response from vagus nerve stimulation in patients with epilepsy. Int. J. Neural Syst. 27:1650048. doi: 10.1142/S0129065716500489
Xiong, J., Xue, F. S., Liu, J. H., Xu, Y. C., Liao, X., Zhang, Y. M., et al. (2009). Transcutaneous vagus nerve stimulation may attenuate postoperative cognitive dysfunction in elderly patients. Med. Hypoth. 73, 938–941. doi: 10.1016/j.mehy.2009.06.033
Yakunina, N., Kim, S. S., and Nam, E.-C. (2017). Optimization of transcutaneous vagus nerve stimulation using functional MRI. Neuromodulation 20, 290−300. doi: 10.1111/ner.12541
Yakunina, N., Kim, S. S., and Nam, E.-C. (2018). BOLD fMRI effects of transcutaneous vagus nerve stimulation in patients with chronic tinnitus. PLoS ONE 13:e0207281. doi: 10.1371/journal.pone.0207281
Yang, G., Xue, F., Sun, C., Liao, X., and Liu, J. (2017). Vagal nerve stimulation: a potentially useful adjuvant to treatment of sepsis. J Anesth Perioper Med. (2017). doi: 10.24015/JAPM.2017.0012
Yao, G., Kang, L., Li, J., Long, Y., Wei, H., Ferreira, C. A., et al. (2018). Effective weight control via an implanted self-powered vagus nerve stimulation device. Nat. Commun. 9:5349. doi: 10.1038/s41467-018-07764-z
Yavich, L., Jäkälä, P., and Tanila, H. (2005). Noradrenaline overflow in mouse dentate gyrus following locus coeruleus and natural stimulation: real-time monitoring by in vivo voltammetry. J. Neurochem. 95, 641–650. doi: 10.1111/j.1471-4159.2005.03390.x
Ye, R., Rua, C., O'Callaghan, C., Jones, P. S., Hezemans, F., Kaalund, S. S., et al. (2020). An in vivo probabilistic atlas of the human locus coeruleus at ultra-high field. BioRxiv [Preprint]. 932087. doi: 10.1101/2020.02.03.932087
Ylikoski, J., Lehtimäki, J., Pirvola, U., Mäkitie, A., Aarnisalo, A., Hyvärinen, P., et al. (2017). Non-invasive vagus nerve stimulation reduces sympathetic preponderance in patients with tinnitus. Acta Oto Laryngol. 137, 426–431. doi: 10.1080/00016489.2016.1269197
Yoo, P. B., Liu, H., Hincapie, J. G., Ruble, S. B., Hamann, J. J., and Grill, W. M. (2016). Modulation of heart rate by temporally patterned vagus nerve stimulation in the anesthetized dog. Physiol. Rep. 4:e12689. doi: 10.14814/phy2.12689
Yu, L., Huang, B., Po, S. S., Tan, T., Wang, M., Zhou, L., et al. (2017). Low-level tragus stimulation for the treatment of ischemia and reperfusion injury in patients with ST-segment elevation myocardial infarction: a proof-of-concept study. JACC Cardiovasc. Interv. 10, 1511–1520. doi: 10.1016/j.jcin.2017.04.036
Yuan, H., and Silberstein, S. D. (2016a). Vagus nerve and vagus nerve stimulation, a comprehensive review: Part I. Headache 56, 71–78. doi: 10.1111/head.12647
Yuan, H., and Silberstein, S. D. (2016b). Vagus nerve and vagus nerve stimulation, a comprehensive review: Part II. Headache 56, 259–266. doi: 10.1111/head.12650
Zabara, J. (1992). Inhibition of experimental seizures in canines by repetitive vagal stimulation. Epilepsia 33, 1005–1012. doi: 10.1111/j.1528-1157.1992.tb01751.x
Zhang, S., Song, Y., Jia, J., Xiao, G., Yang, L., Sun, M., et al. (2016). “An implantable microelectrode array for dopamine and electrophysiological recordings in response to L-dopa therapy for Parkinson's disease,” in Conference Proceedings: …Annual International Conference of the IEEE Engineering in Medicine and Biology Society. IEEE Engineering in Medicine and Biology Society. Annual Conference (Orlando), 2016, 1922–1925. doi: 10.1109/EMBC.2016.7591098
Zhang, S., Song, Y., Wang, M., Xiao, G., Gao, F., Li, Z., et al. (2018). Real-time simultaneous recording of electrophysiological activities and dopamine overflow in the deep brain nuclei of a non-human primate with Parkinson's disease using nano-based microelectrode arrays. Microsyst. Nanoeng. 4, 1–9. doi: 10.1038/micronano.2017.70
Zhang, Y., Liu, J., Li, H., Yan, Z., Liu, X., Cao, J., et al. (2019). Transcutaneous auricular vagus nerve stimulation at 1 Hz modulates locus coeruleus activity and resting state functional connectivity in patients with migraine: an fMRI study. Neuroimage Clin. 24:101971. doi: 10.1016/j.nicl.2019.101971
Keywords: transcutaneous vagus nerve stimulation, minimum reporting standards, guidelines & recommendations, transcutaneous auricular vagus nerve stimulation, transcutaneous cervical vagus nerve stimulation
Citation: Farmer AD, Strzelczyk A, Finisguerra A, Gourine AV, Gharabaghi A, Hasan A, Burger AM, Jaramillo AM, Mertens A, Majid A, Verkuil B, Badran BW, Ventura-Bort C, Gaul C, Beste C, Warren CM, Quintana DS, Hämmerer D, Freri E, Frangos E, Tobaldini E, Kaniusas E, Rosenow F, Capone F, Panetsos F, Ackland GL, Kaithwas G, O'Leary GH, Genheimer H, Jacobs HIL, Van Diest I, Schoenen J, Redgrave J, Fang J, Deuchars J, Széles JC, Thayer JF, More K, Vonck K, Steenbergen L, Vianna LC, McTeague LM, Ludwig M, Veldhuizen MG, De Couck M, Casazza M, Keute M, Bikson M, Andreatta M, D'Agostini M, Weymar M, Betts M, Prigge M, Kaess M, Roden M, Thai M, Schuster NM, Montano N, Hansen N, Kroemer NB, Rong P, Fischer R, Howland RH, Sclocco R, Sellaro R, Garcia RG, Bauer S, Gancheva S, Stavrakis S, Kampusch S, Deuchars SA, Wehner S, Laborde S, Usichenko T, Polak T, Zaehle T, Borges U, Teckentrup V, Jandackova VK, Napadow V and Koenig J (2021) International Consensus Based Review and Recommendations for Minimum Reporting Standards in Research on Transcutaneous Vagus Nerve Stimulation (Version 2020). Front. Hum. Neurosci. 14:568051. doi: 10.3389/fnhum.2020.568051
Received: 31 May 2020; Accepted: 01 September 2020;
Published: 23 March 2021.
Edited by:
Yusuf Ozgur Cakmak, University of Otago, New ZealandReviewed by:
Chunhong Liu, Capital Medical University, ChinaTeresa Schuhmann, Maastricht University, Netherlands
Copyright © 2021 Farmer, Strzelczyk, Finisguerra, Gourine, Gharabaghi, Hasan, Burger, Jaramillo, Mertens, Majid, Verkuil, Badran, Ventura-Bort, Gaul, Beste, Warren, Quintana, Hämmerer, Freri, Frangos, Tobaldini, Kaniusas, Rosenow, Capone, Panetsos, Ackland, Kaithwas, O'Leary, Genheimer, Jacobs, Van Diest, Schoenen, Redgrave, Fang, Deuchars, Széles, Thayer, More, Vonck, Steenbergen, Vianna, McTeague, Ludwig, Veldhuizen, De Couck, Casazza, Keute, Bikson, Andreatta, D'Agostini, Weymar, Betts, Prigge, Kaess, Roden, Thai, Schuster, Montano, Hansen, Kroemer, Rong, Fischer, Howland, Sclocco, Sellaro, Garcia, Bauer, Gancheva, Stavrakis, Kampusch, Deuchars, Wehner, Laborde, Usichenko, Polak, Zaehle, Borges, Teckentrup, Jandackova, Napadow and Koenig. This is an open-access article distributed under the terms of the Creative Commons Attribution License (CC BY). The use, distribution or reproduction in other forums is permitted, provided the original author(s) and the copyright owner(s) are credited and that the original publication in this journal is cited, in accordance with accepted academic practice. No use, distribution or reproduction is permitted which does not comply with these terms.
*Correspondence: Julian Koenig, anVsaWFuLmtvZW5pZyYjeDAwMDQwO21lZC51bmktaGVpZGVsYmVyZy5kZQ==