- 1School of Medicine, Duke University, Durham, NC, United States
- 2Department of Medicine, Duke University, Durham, NC, United States
- 3Department of Neurobiology, Duke University, Durham, NC, United States
As far back as we can remember, we eat. In fact, we eat before we can remember. Our first meal is amniotic fluid. We swallow it during the first trimester of gestation, and with that, we expose our gut to a universe of molecules. These early molecules have a profound influence on gut and brain function. For example, the taste of the amniotic fluid changes based on the mother’s diet. Indeed, recent findings suggest that food preferences begin in utero. Likewise, a baby’s first exposure to bacteria, previously thought to be during birth, appears to be in utero as well. And just as postnatal food and microbiota are implicated in brain function and dysfunction, prenatal nutrients and microbes may have a long-lasting impact on the development of the gut-brain neural circuits processing food, especially considering their plasticity during this vulnerable period. Here, we use current literature to put forward concepts needed to understand how the gut first meets the brain, and how this encounter may help us remember food.
Introduction
Before we are born, we must learn to hear, smell, taste—and eat. The first meal a human consumes is not its mother’s milk or formula, but instead amniotic fluid ingested by the fetus in utero. That first meal introduces the brain and the gut, establishing communication which will be sustained throughout its lifespan.
During gestation, the fetus recognizes the scent of its amniotic fluid (Marlier et al., 1998; Schaal et al., 1998), and even its taste (Lipchock et al., 2011). After the first ingestion, this fluid has a profound effect on the morphological development of the gastrointestinal tract (Mulvihill et al., 1985; Bohórquez et al., 2011). How this first meal influences our ability to sense food remains unexplored. Only in recent years have the means through which the gut senses chemical components of food become evident (Jang et al., 2007).
Soon after the discovery of taste receptors in the tongue (Adler et al., 2000), it became clear that those receptors were not confined to the tongue, but are also prominently expressed throughout the gastrointestinal epithelium (Rozengurt and Sternini, 2007). Those taste receptors are located in specialized intestinal sensory epithelial cells, including enteroendocrine cells. And just like other sensory epithelial cells, enteroendocrine cells are innervated (Bohórquez et al., 2015). This synapse is the first link, the first pathway, from the gut to the brain. It serves as a sensory portal for the transduction of stimuli from the gut lumen, and as a path for pathogens to access the peripheral and central nervous systems. We draw information from other sensory systems to infer potential implications of early sensory stimuli on the development and function of gut-brain sensory transduction (Figure 1). These lines, rather than being an exhaustive account, should serve as a foundation to stimulate the development of knowledge of the gut-brain sensory transduction field.
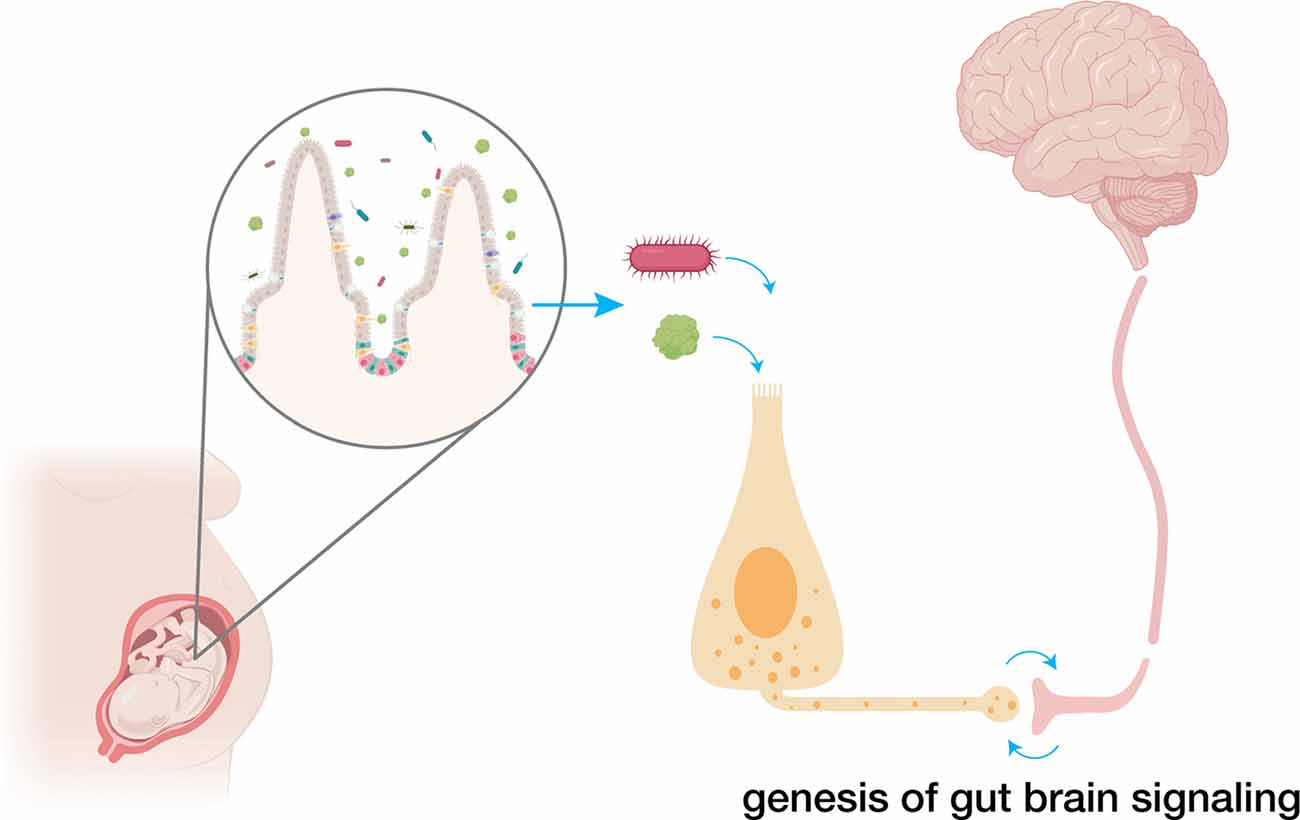
Figure 1. Early exposure to amniotic fluid, nutrients and the microbiota influences gut-brain circuit development. Prior to birth, fetal swallowing exposes its gut to amniotic fluid, containing both nutrients and a developing microbiome. There, nutrients, microbiota and their metabolites come in contact with developing gut sensor cells—enteroendocrine cells—which form synapses with vagal nodose neurons. Dysregulation of the development of this gut-brain neural circuitry can have profound, prolonged effects on later health. Created with BioRender.
The First Enteroendocrine Cell
What is known about enteroendocrine cell developmental origin can be traced to the 1960s. Anthony Pearse discovered that seemingly unrelated endocrine cells in different organs appeared to have a common origin—the neural crest (Pearse, 1969). This grouping of cells, known as amine precursor uptake and decarboxylation (APUD) cells, has been heavily debated (Le Douarin and Teillet, 1973; Andrew et al., 1998; Barker and Clevers, 2007; Barker et al., 2008) and eventually fell out of favor. Nonetheless, like other sensory epithelial cells of endodermal origin, enteroendocrine cells have prominent neuronal features. They are electrically excitable (Rogers et al., 2011), form synapses (Bohórquez et al., 2015), and release neuropeptides and neurotransmitters in response to stimuli (Hartenstein et al., 2017). Because of the mounting evidence that these cells act as true epithelial transducers, further exploration will elucidate the precise origin of these cells.
The first enteroendocrine cells appear early in gestation, during gut tube development. At this point the cells remain mitotically active (Crosnier, 2005; Penkova et al., 2010; Hartenstein et al., 2017). Upon folding of the gut epithelium into crypts and villi, most cells are differentiated, and further proliferation occurs from the Lgr5-expressing adult stem cells of the crypts (Barker et al., 2007). The differentiation of an intestinal stem cell into an enteroendocrine cell is dependent on Delta-Notch signaling (Micchelli and Perrimon, 2006). Low levels of Notch influence enteroendocrine differentiation, while high levels of Notch promote an enterocyte fate. Then, in the presence of low Notch activity, enteroendocrine cell fate is driven by acheate-scute (ac-sc) complex genes that encode bHLH transcription factors and the enteroendocrine cell fate promoter Prospero, among others (Yin and Xi, 2018).
The role of ac-sc complex gene expression in driving neuronal differentiation was established in Drosophila decades ago (Cubas et al., 1991; Skeath and Carroll, 1991). Since then, ac-sc complex gene expression has been found to promote enteroendocrine cell fate (Bardin et al., 2010). Just recently, Chen and colleagues used a Drosophila model to propose that Delta-Notch signaling guides enterocyte generation from intestinal stem cells as the default mode, and that, paralleling neuronal differentiation, transient Sc expression is required to trigger enteroendocrine cell formation (Chen et al., 2018). In mammals, the bHLH transcription factor family is commonly known to be pro-neural, but certain bHLH transcription factors including Math1 (Atoh1), Neurogenin3, and NeuroD have also been identified as pro-endocrine. Math1 is expressed in cells destined to become secretory cells (Yang et al., 2001). Then, neurogenin3 expression is required for intestinal enteroendocrine cell development (Jenny et al., 2002; Schonhoff et al., 2004). NeuroD controls terminal differentiation of specific enteroendocrine cells (Naya et al., 1997; Mutoh et al., 1998). In parallel or downstream to ac-sc and bHLH signaling, Prospero functions to commit intestinal stem cell differentiation to enteroendocrine cells (Zeng and Hou, 2015). Though the discovery of its function in enteroendocrine cells is recent, Prospero has long been studied as a transcription factor predominantly found in the nervous system (Manning and Doe, 1999).
At a more macroscopic level, the similarities between the differentiation of enteroendocrine cells and neural cells persist. It has been suggested that enteroendocrine cells can be generated from intestinal stem cells using one of three pathways: terminal differentiation into an enteroendocrine cell, asymmetric division into an intestinal stem cell and an enteroendocrine cell, or symmetric division into two enteroendocrine cells (Zeng and Hou, 2015). Experiments from Chen and colleagues in Drosophila suggest that intestinal stem cells actually yield enteroendocrine progenitor cells, which then terminally divide into two enteroendocrine cells (Chen et al., 2018). This proposed process of cell division mirrors ganglion mother cells in Drosophila neuroblasts: each neuroblast divides exactly once prior to terminal differentiation into neurons or glial cells (Homem and Knoblich, 2012). Regardless of method of division, intestinal stem cells are located basally; similarly, sensory organ progenitors of Drosophila that give rise to sensory neurons are located sub-epidermally. And in both, polarity is acquired after differentiation (Hartenstein et al., 2017). Thus, when Notch activity is low, ac-sc complex genes, bHLH transcription factors and Prospero signaling drive enteroendocrine cell development from intestinal stem cells by inducing the formation of an enteroendocrine progenitor cell that divides into enteroendocrine cells.
When the Gut First Meets the Brain
The discovery that enteroendocrine cells are innervated opened a path for gut sensory transduction. Such signals could travel from the gut lumen to the brain, via the vagus nerve. The vagus is the primary integrator of visceral sensory information, and vagal innervation of the gastrointestinal tract is critical to various homeostatic processes including satiety and visceral pain signaling (Berthoud, 2008; Ratcliffe et al., 2011b). Additionally, the vagus has been shown to be critical in the communication of the microbiome with the central nervous system. Chronic exposure to Lactobaccillus rhamnosus has anxiolytic effects in mice that are eliminated by subdiaphragmatic vagotomy (Bravo et al., 2011).
Vagal innervation of the gastrointestinal tract begins early in embryonic life. In one study of fetal mice, vagal sensory fibers reached the stomach by embryonic day 12, the duodenum by day 14, and the distal small intestine by day 16 (Ratcliffe et al., 2011a). By visualizing vagal fibers with DiI applied to the fetal nodose ganglia, vagal fibers appear to be attracted to Netrin-1 expressed by the developing foregut through deleted in colorectal cancer receptors (Ratcliffe et al., 2006). Attractive signaling through neurotrophins including brain-derived neurotrophic factor (BDNF) and repulsive signaling through Slit/Robo are also critical to the prenatal development of sensory vagal innervation of the gastrointestinal tract.
BDNF is crucial for synapse regulation (Lu et al., 2014). Centrally, hippocampal BDNF expression and neurogenesis is dependent on the vagus nerve (O’Leary et al., 2018). Peripherally, neurotrophin-3, neurotropin-4 and BDNF promote vagal innervation of the gastrointestinal tract. In knockout mice of neutrotropin-3 or neurotrophin-4, severely reduced vagal innervation is observed in the esophagus (Raab et al., 2003) and small intestine (Fox et al., 2001), respectively. In a homozygous BDNF knockout, postnatal day 0 mice exhibit altered morphology and reduced density of stomach vagal afferent structures such as intramuscular arrays and intraganglionic laminar endings compared to wild-type controls (Murphy and Fox, 2010). BDNF is known to be expressed in the muscle layers of the GI tract (Murphy and Fox, 2010), and indeed, limited studies have suggested that enteroendocrine cells of higher vertebrates express neurotrophic factors including BDNF and neurotropin-3 (Lucini et al., 2002). In vitro, when a murine enteroendocrine cell is co-cultured with a sensory neuron there is a clear affinity. The neuroepithelial circuit is recapitulated; enteroendocrine cells synapse with vagal nodose neurons in vitro, and time-lapse footage suggests that enteroendocrine cells guide the process (Bohórquez et al., 2015).
Repulsive signaling also works to counter attractive signaling and restrict vagal afferents from entering the bowel wall; Slit/Robo signaling is the most well-studied pathway. Transcripts encoding Robo1-2 have been identified in the embryonic and adult nodose ganglia; Slits1–3 in the embryonic and adult gut (Goldberg et al., 2013). In the same study, co-cultures with mouse nodose ganglia and Slit2 secreting cells demonstrated chemorepulsion of nodose neurites by Slit2. Similarly, Slit/Robo signaling functions in enteroendocrine cell differentiation and development. In Drosophila, enteroendocrine cells release Slit1, which acts on Robo2 receptors in intestinal stem cells to limit differentiation to an endocrine fate, acting as a negative feedback control (Biteau and Jasper, 2014). Although the specific relationship of enteroendocrine-secreted Slit and vagal innervation of the gastrointestinal tract has not been established, juxtaposing these studies suggests a correlation. The repulsion-attraction mechanisms in enteroendocrine cells could serve as beacons for proper innervation of the intestinal mucosa.
Limited studies also suggest that vagal innervation of the gastrointestinal tract promotes survival and proliferation of enteroendocrine cells. In mice and calves, vagotomy reduces the density of enteroendocrine cells in the stomach (Qian et al., 1999; Soehartono et al., 2002). Although the moment of synapse formation between the vagus nerve and enteroendocrine cells remains unknown, the early development of vagal innervation and similarities in signaling molecules suggest that the gut and brain contact early in embryonic life. An early, synaptic, gut-brain connection could help us understand how we process early gut sensory stimulants—nutrients and bacteria.
The Gut’s First Feeling: Nutrients and the Microbiome
The first drink of a mother’s milk is not a newborn’s first meal. Longitudinal, ultrasonographic studies of healthy, developing fetuses have demonstrated that as early as gestational week 12, newborn babies swallow amniotic fluid and by 18 weeks, gestational suckling begins (de Vries et al., 1985; Miller et al., 2003). Amniotic fluid contains the essential nutrients—glucose, fructose, fatty acids and amino acids—that the fetus will need to sense and absorb postnatally to survive. In fact, amniotic fluid provides a significant portion of nutrition to the fetus, and the inability to swallow is a risk factor for low birth weight and poor gastrointestinal development (Mulvihill et al., 1985; Bagci et al., 2016). However, the process of swallowing does more than allow the fetus to absorb the nutrients in amniotic fluid. Swallowing exposes the fetus’s orosensory and post-oral sensor cells—enteroendocrine cells—to nutrients and bacterial ligands that influence the development of gut-brain communication.
Early Nutrient Sensing
The development of flavor memories begins in utero and influences later food preferences. In response to sweet solutions, ovine fetuses increase their rate of swallowing, demonstrating motivated behavior for rewarding substances (El-Haddad et al., 2002). In a well-designed, randomized, controlled trial, babies whose mothers drank carrot juice during the last trimester of gestation or the first 3 months of lactation were more accepting of carrots at 6 months of age (Mennella et al., 2001). In a subsequent study, formula fed infants were randomized to receive palatable cow milk-based formula or unpalatable protein hydrolysate formula at varying ages and durations. They found a sensitive period—prior to 4 months of age—when exposure to a flavor determines its hedonic tone (Mennella et al., 2011).
Both gustatory and post-oral signaling pathways contribute to the findings in these studies in fetuses and infants, but work in adult mice has shown that post-oral signaling, likely via enteroendocrine cells, can potently drive preference independent of taste. Intragastric sugar infusions can rapidly stimulate the intake and preference for non-nutritive solutions in mice (Sclafani and Ackroff, 2012). Furthermore, sweet blind Trmp5 knockout mice can develop a preference for sucrose in a dopamine-dependent pathway based solely on its caloric value (de Araujo et al., 2008). Therefore, post-oral stimuli and their interactions with enteroendocrine cells are also likely to significantly influence the development and function of gut-brain neural circuits.
The Fetal Microbiome
The amniotic fluid that constitutes a fetus’s first meal contains more than nutrients—it also carries microbiota. Recently, the dogma that the uterus is an immune-privileged environment has been challenged. If the fetus is exposed to microbes, studying intestinal sensation of nutrients in isolation would be only part of the puzzle. It is crucial to assess how a prenatal microbiome could affect gut-brain neural circuit development.
Microbiota play a critical role in the embryogenesis of many lower species. In lumbricid earthworms, marine sponges and caridean shrimp, for example, symbiotic bacteria are selectively recruited and vertically transmitted to developing embryos to support embryogenesis and protect them from pathogen colonization (Gil-Turnes et al., 1989; Ereskovsky et al., 2005; Davidson and Stahl, 2008). The same dependence on microbiota in higher species has not been established, but increasing evidence points toward vertical transmission of commensal prenatal microbes in higher vertebrates. In turkeys, microbial structures are clearly observed in the embryo’s intestinal tract well before hatching (Bohórquez, 2010). Jiménez and colleagues established the possibility of vertical transmission from mother to fetal amniotic fluid in mice (Jiménez et al., 2005). They isolated labeled Enterococcus faecium in the amniotic fluid of pregnant mice orally inoculated with the same strain; the bacterium was not isolated in control mice. In the same study, PCR analysis of umbilical cord blood of healthy mouse neonates delivered at term by cesarean section identified bacteria belonging to species including Enterococcus faecium, Propionibacterium acnes, Staphylococcus epidermidis and Streptococcus sanguinis (Jiménez et al., 2005). This was one of the first studies to suggest the presence of prenatal microbiota in healthy pregnancies.
Furthermore, in humans, studies have established distinct microbiota populations in the amniotic fluid, meconium, placenta and umbilical cord (Jiménez et al., 2008; Aagaard et al., 2014; Ardissone et al., 2014; Collado et al., 2016). In one study, over 5 in 10 human infants delivered at greater than 33 weeks of gestation had evidence of intestinal colonization by 16S rRNA amplification and sequencing of meconium samples (Ardissone et al., 2014). In this and corroborating studies, shared features and specific genera of microbiota between the meconium, amniotic fluid, and the placenta have been observed (Ardissone et al., 2014; Collado et al., 2016). The process of swallowing non-sterile amniotic fluid likely inoculates the healthy fetal gut with a developing microbiome.
Early Influences of the Microbiome on Development and Disease
The birthing process further colonizes the newborn gut with microbial flora. Any disruption—including changes in birthing method and maternal perinatal antibiotics—can increase the risk of disease later in life. Using obesity as an example, in a study of 436 mother child-dyads followed for 7 years after birth, the risk for obesity was 84% greater in children whose mothers had taken late term antibiotics and 46% greater in children born by cesarean section (Mueller et al., 2015). In a subsequent study, the association between childhood obesity and cesarean section remained after controlling for maternal obesity (Mueller et al., 2017). Shortly after birth, feeding continues to expose the newborn’s gut to bacteria. And just as differences in diet alter a newborn’s perception of nutrients, the microbiome of breast and formula fed infants may also be affected. Breast feeding has been associated with an increased abundance of bifidiobacteria species, decreased microbial diversity, and increased stability of the microbiota population (Bezirtzoglou et al., 2011; Kashtanova et al., 2016). Alterations of the gut microbiota in this sensitive time have also been shown to contribute to disease; antibiotics administered in early infancy have also been associated with an increased risk for obesity (Bailey et al., 2014; Cox et al., 2014).
The study of germ-free animals has allowed researchers to investigate the role of the microbiota in gut-brain signaling and development (Luczynski et al., 2016). Gnotobiotic zebrafish have reduced levels of serotonin-positive enteroendocrine cells despite normal levels of intestinal epithelial cells, and numbers can be restored by inoculation with a complete microbiome (Bates et al., 2006). In a mouse model of pre-term infants, inoculation of pre-term mice with the microbiota of pre-term human infants who were adequately gaining weight resulted in improved villus height and crypt depth, increased cell proliferation, increased density of Paneth and goblet cells, and improved tight junctions (Yu et al., 2016). Microbiota are also essential for normal brain development. In one study, germ free mice demonstrate increased motor activity, reduced anxiety-like behavior, and increased striatal levels of synaptophysin (a synaptic vesicle maturation and synaptogenesis marker) and PSD-95 (marker for maturation of excitatory synapses). Early colonization of these germ-free mice with microbiota normalized several behavior patterns and synaptophysin and PSD-95 levels, suggesting early microbiota is crucial for normal brain development and behavior (Diaz Heijtz, 2016). Carlson and colleagues recently demonstrated associations between microbial composition and cognitive functioning, as measured by the Mullen Scales of Early Learning, in developmentally normal infants (Carlson et al., 2018). Gut-brain sensory neural circuitry could link the microbiome to its early effects on the development of the gut, the brain, and disease.
Conclusion
Recently, gut-brain biology has become an attractive scientific field. Most of the work has focused on correlative observations between gut microbiota and the brain. But emerging literature is revealing the neural circuits linking the gut surface and the brain. The discovery of synapses in enteroendocrine cells has provided a new avenue of exploration in how nutrients and microbiota signals in the gut modulate brain function and behaviors. It has also allowed us to look at the development of gut-brain neural circuits from a new perspective. There are significant parallels between enteroendocrine cell and sensory neuron development and differentiation. At an early gestational age, we begin to perceive the outside world through innervated sensory epithelial cells. Enteroendocrine cells are included in this family now. What we feel, hear, taste, smell and eat in utero influences our development and later integration into the world at birth. Alterations in the development of these sensory neuroepithelial circuits are potential targets for gut and brain disorders linked to visceral hypersensitivity. Some of those include obesity, anorexia, autism, chronic abdominal pain and irritable bowel disease. After all, when the gut first meets the brain may be when our brain learns to feel food in the gut.
Author Contributions
KB and DB designed the scope of the review. KB wrote the first draft of the manuscript. DB edited the manuscript.
Funding
This work was supported by the National Institutes of Health grants to DB K01 DK-103832, R03 DK-114500, The Hartwell Foundation and The Dana Foundation. KB is a Howard Hughes Medical Institute Medical Research Fellow.
Conflict of Interest Statement
The authors declare that the research was conducted in the absence of any commercial or financial relationships that could be construed as a potential conflict of interest.
References
Aagaard, K., Ma, J., Antony, K. M., Ganu, R., Petrosino, J., and Versalovic, J. (2014). The Placenta harbors a unique microbiome. Sci. Transl. Med. 6:237ra65. doi: 10.1126/scitranslmed.3008599
Adler, E., Hoon, M. A., Mueller, K. L., Chandrashekar, J., Ryba, N. J., and Zuker, C. S. (2000). A novel family of mammalian taste receptors. Cell 100, 693–702. doi: 10.1016/s0092-8674(00)80705-9
Andrew, A., Kramer, B., and Rawdon, B. B. (1998). The origin of gut and pancreatic neuroendocrine (APUD) cells–The last word? J. Pathol. 186, 117–118. doi: 10.1002/(sici)1096-9896(1998100)186:2<117::aid-path152>3.0.co;2-j
Ardissone, A. N., de la Cruz, D. M., Davis-Richardson, A. G., Rechcigl, K. T., Li, N., Drew, J. C., et al. (2014). Meconium microbiome analysis identifies bacteria correlated with premature birth. PLoS One 9:e90784. doi: 10.1371/journal.pone.0090784
Bagci, S., Brosens, E., Tibboel, D., De Klein, A., Ijsselstijn, H., Wijers, C. H. W., et al. (2016). More than fetal urine: enteral uptake of amniotic fluid as a major predictor for fetal growth during late gestation. Eur. J. Pediatr. 175, 825–831. doi: 10.1007/s00431-016-2713-y
Bailey, L. C., Forrest, C. B., Zhang, P., Richards, T. M., Livshits, A., and DeRusso, P. A. (2014). Association of antibiotics in infancy with early childhood obesity. JAMA Pediatr. 168, 1063–1069. doi: 10.1001/jamapediatrics.2014.1539
Bardin, A. J., Perdigoto, C. N., Southall, T. D., Brand, A. H., and Schweisguth, F. (2010). Transcriptional control of stem cell maintenance in the Drosophila intestine. Development 137, 705–714. doi: 10.1242/dev.039404
Barker, N., and Clevers, H. (2007). Tracking down the stem cells of the intestine: strategies to identify adult stem cells. Gastroenterology 133, 1755–1760. doi: 10.1053/j.gastro.2007.10.029
Barker, N., van de Wetering, M., and Clevers, H. (2008). The intestinal stem cell. Genes Dev. 22, 1856–1864. doi: 10.1101/gad.1674008
Barker, N., Van Es, J. H., Kuipers, J., Kujala, P., Van Den Born, M., Cozijnsen, M., et al. (2007). Identification of stem cells in small intestine and colon by marker gene Lgr5. Nature 449, 1003–1007. doi: 10.1038/nature06196
Bates, J. M., Mittge, E., Kuhlman, J., Baden, K. N., Cheesman, S. E., and Guillemin, K. (2006). Distinct signals from the microbiota promote different aspects of zebrafish gut differentiation. Dev. Biol. 297, 374–386. doi: 10.1016/j.ydbio.2006.05.006
Berthoud, H. R. (2008). Vagal and hormonal gut-brain communication: from satiation to satisfaction. Neurogastroenterol. Motil. 20, 64–72. doi: 10.1111/j.1365-2982.2008.01104.x
Bezirtzoglou, E., Tsiotsias, A., and Welling, G. W. (2011). Microbiota profile in feces of breast- and formula-fed newborns by using fluorescence in situ hybridization (FISH). Anaerobe 17, 478–482. doi: 10.1016/j.anaerobe.2011.03.009
Biteau, B., and Jasper, H. (2014). Slit/Robo signaling regulates cell fate decisions in the intestinal stem cell lineage of Drosophila. Cell Rep. 7, 1867–1875. doi: 10.1016/j.celrep.2014.05.024
Bohórquez, D. V. (2010). Nutritional Influences on the Ultra-Structural Development of the Small Intestinal Epithelium of the Perinatal Turkey Embryo and Poult. [dissertation]. Raleigh, NC: North Carolina State University.
Bohórquez, D. V., Bohórquez, N. E., and Ferket, P. R. (2011). Ultrastructural development of the small intestinal mucosa in the embryo and turkey poult: a light and electron microscopy study. Poult. Sci. 90, 842–855. doi: 10.3382/ps.2010-00939
Bohórquez, D. V., Shahid, R. A., Erdmann, A., Kreger, A. M., Wang, Y., Calakos, N., et al. (2015). Neuroepithelial circuit formed by innervation of sensory enteroendocrine cells. J. Clin. Invest. 125, 782–786. doi: 10.1172/JCI78361
Bravo, J. A., Forsythe, P., Chew, M. V., Escaravage, E., Savignac, H. M., Dinan, T. G., et al. (2011). Ingestion of Lactobacillus strain regulates emotional behavior and central GABA receptor expression in a mouse via the vagus nerve. Proc. Natl. Acad. Sci. U S A 108, 16050–16055. doi: 10.1073/pnas.1102999108
Carlson, A. L., Xia, K., Azcarate-Peril, M. A., Goldman, B. D., Ahn, M., Styner, M. A., et al. (2018). Infant gut microbiome associated with cognitive development. Biol. Psychiatry 83, 148–159. doi: 10.1016/j.biopsych.2017.06.021
Chen, J., Xu, N., Wang, C., Huang, P., Huang, H., Jin, Z., et al. (2018). Transient scute activation via a self-stimulatory loop directs enteroendocrine cell pair specification from self-renewing intestinal stem cells. Nat. Cell Biol. 20, 152–161. doi: 10.1038/s41556-017-0020-0
Collado, M. C., Rautava, S., Aakko, J., Isolauri, E., and Salminen, S. (2016). Human gut colonisation may be initiated in utero by distinct microbial communities in the placenta and amniotic fluid. Sci. Rep. 6:23129. doi: 10.1038/srep23129
Cox, L. M., Yamanishi, S., Sohn, J., Alekseyenko, A. V., Leung, J. M., Cho, I., et al. (2014). Altering the intestinal microbiota during a critical developmental window has lasting metabolic consequences. Cell 158, 705–721. doi: 10.1016/j.cell.2014.05.052
Crosnier, C. (2005). Delta-Notch signalling controls commitment to a secretory fate in the zebrafish intestine. Development 132, 1093–1104. doi: 10.1242/dev.01644
Cubas, P., De Celis, J. F., Campuzano, S., and Modolell, J. (1991). Proneural clusters of achaete-scute expression and the generation of sensory organs in the Drosophila imaginal wing disc. Genes Dev. 5, 996–1008. doi: 10.1101/gad.5.6.996
Davidson, S. K., and Stahl, D. A. (2008). Selective recruitment of bacteria during embryogenesis of an earthworm. ISME J. 2, 510–518. doi: 10.1038/ismej.2008.16
de Araujo, I. E., Oliveira-Maia, A. J., Sotnikova, T. D., Gainetdinov, R. R., Caron, M. G., Nicolelis, M. A. L., et al. (2008). Food reward in the absence of taste receptor signaling. Neuron 57, 930–941. doi: 10.1016/j.neuron.2008.01.032
de Vries, J. I. P., Visser, G. H. A., and Prechtl, H. F. R. (1985). The emergence of fetal behaviour. II. Quantitative aspects. Early Hum. Dev. 12, 99–120. doi: 10.1016/0378-3782(85)90174-4
Diaz Heijtz, R. (2016). Fetal, neonatal and infant microbiome: perturbations and subsequent effects on brain development and behavior. Semin. Fetal Neonatal Med. 21, 410–417. doi: 10.1016/j.siny.2016.04.012
El-Haddad, M. A., Ismail, Y., Guerra, C., Day, L., and Ross, M. G. (2002). Effect of oral sucrose on ingestive behavior in the near-term ovine fetus. Am. J. Obstet. Gynecol. 187, 898–901. doi: 10.1067/mob.2002.127722
Ereskovsky, A. V., Gonobobleva, E., and Vishnyakov, A. (2005). Morphological evidence for vertical transmission of symbiotic bacteria in the viviparous sponge Halisarca dujardini Johnston (Porifera, Demospongiae, Halisarcida). Mar. Biol. 146, 869–875. doi: 10.1007/s00227-004-1489-1
Fox, E. A., Phillips, R. J., Baronowsky, E. A., Byerly, M. S., Jones, S., and Powley, T. L. (2001). Neurotrophin-4 deficient mice have a loss of vagal intraganglionic mechanoreceptors from the small intestine and a disruption of short-term satiety. J. Neurosci. 21, 8602–8615. doi: 10.1523/jneurosci.21-21-08602.2001
Gil-Turnes, M., Hay, M., and Fenical, W. (1989). Symbiotic marine bacteria chemically defend crustacean embryos from a pathogenic fungus. Science 246, 116–118. doi: 10.1126/science.2781297
Goldberg, D., Borojevic, R., Anderson, M., Chen, J. J., Gershon, M. D., and Ratcliffe, E. M. (2013). Slit/Robo-mediated chemorepulsion of vagal sensory axons in the fetal gut. Dev. Dyn. 242, 9–15. doi: 10.1002/dvdy.23898
Hartenstein, V., Takashima, S., Hartenstein, P., Asanad, S., and Asanad, K. (2017). bHLH proneural genes as cell fate determinants of entero-endocrine cells, an evolutionarily conserved lineage sharing a common root with sensory neurons. Dev. Biol. 431, 36–47. doi: 10.1016/j.ydbio.2017.07.013
Homem, C. C. F., and Knoblich, J. A. (2012). Drosophila neuroblasts: a model for stem cell biology. Development 139, 4297–4310. doi: 10.1242/dev.080515
Jang, H. J., Kokrashvili, Z., Theodorakis, M. J., Carlson, O. D., Kim, B. J., Zhou, J., et al. (2007). Gut-expressed gustducin and taste receptors regulate secretion of glucagon-like peptide-1. Proc. Natl. Acad. Sci. U S A 104, 15069–15074. doi: 10.1073/pnas.0706890104
Jenny, M., Uhl, C., Roche, C., Duluc, I., Guillermin, V., Guillemot, F., et al. (2002). Neurogenin3 is differentially required for endocrine cell fate specification in the intestinal and gastric epithelium. EMBO J. 21, 6338–6347. doi: 10.1093/emboj/cdf649
Jiménez, E., Fernández, L., Marín, M. L., Martín, R., Odriozola, J. M., Nueno-Palop, C., et al. (2005). Isolation of commensal bacteria from umbilical cord blood of healthy neonates born by cesarean section. Curr. Microbiol. 51, 270–274. doi: 10.1007/s00284-005-0020-3
Jiménez, E., Marín, M. L., Martín, R., Odriozola, J. M., Olivares, M., Xaus, J., et al. (2008). Is meconium from healthy newborns actually sterile? Res. Microbiol. 159, 187–193. doi: 10.1016/j.resmic.2007.12.007
Kashtanova, D. A., Popenko, A. S., Tkacheva, O. N., Tyakht, A. B., Alexeev, D. G., and Boytsov, S. A. (2016). Association between the gut microbiota and diet: fetal life, early childhood and further life. Nutrition 32, 620–627. doi: 10.1016/j.nut.2015.12.037
Le Douarin, N. M., and Teillet, M. A. (1973). The migration of neural crest cells to the wall of the digestive tract in avian embryo. J. Embryol. Exp. Morphol. 30, 31–48.
Lipchock, S. V., Reed, D. R., and Mennella, J. A. (2011). The gustatory and olfactory systems during infancy: implications for development of feeding behaviors in the high-risk neonate. Clin. Perinatol. 38, 627–641. doi: 10.1016/j.clp.2011.08.008
Lu, B., Nagappan, G., and Lu, Y. (2014). “BDNF and synaptic plasticity, cognitive function and dysfunction,” in Neurotrophic Factors, eds G. R. Lewin and B. D. Carter (Berlin, Heidelberg: Springer Berlin Heidelberg), 223–250.
Lucini, C., Maruccio, L., de Girolamo, P., Vega, J. A., and Castaldo, L. (2002). Localisation of neurotrophin–Containing cells in higher vertebrate intestine. Anat. Embryol. 205, 135–140. doi: 10.1007/s00429-002-0237-x
Luczynski, P., McVey Neufeld, K. A., Oriach, C. S., Clarke, G., Dinan, T. G., and Cryan, J. F. (2016). Growing up in a bubble: using germ-free animals to assess the influence of the gut microbiota on brain and behavior. Int. J. Neuropsychopharmacol. 19:pyw020. doi: 10.1093/ijnp/pyw020
Manning, L., and Doe, C. Q. (1999). Prospero distinguishes sibling cell fate without asymmetric localization in the Drosophila adult external sense organ lineage. Development 126, 2063–2071.
Marlier, L., Schaal, B., and Soussignan, R. (1998). Neonatal responsiveness to the odor of amniotic and lacteal fluids: A test of perinatal chemosensory continuity. Child Dev. 69, 611–623. doi: 10.2307/1132193
Mennella, J. A., Jagnow, C. P., and Beauchamp, G. K. (2001). Prenatal and Postnatal Flavor Learning by Human Infants. Pediatrics 107:E88. doi: 10.1542/peds.107.6.e88
Mennella, J. A., Lukasewycz, L. D., Castor, S. M., and Beauchamp, G. K. (2011). The timing and duration of a sensitive period in human flavor learning: a randomized trial. Am. J. Clin. Nutr. 93, 1019–1024. doi: 10.3945/ajcn.110.003541
Micchelli, C. A., and Perrimon, N. (2006). Evidence that stem cells reside in the adult Drosophila midgut epithelium. Nature 439, 475–479. doi: 10.1038/nature04371
Miller, J. L., Sonies, B. C., and Macedonia, C. (2003). Emergence of oropharyngeal, laryngeal and swallowing activity in the developing fetal upper aerodigestive tract: An ultrasound evaluation. Early Hum. Dev. 71, 61–87. doi: 10.1016/s0378-3782(02)00110-x
Mueller, N. T., Mao, G., Bennet, W. L., Hourigan, S. K., Dominguez-Bello, M. G., Appel, L. J., et al. (2017). Does vaginal delivery mitigate or strengthen the intergenerational association of overweight and obesity? Findings from the Boston Birth Cohort. Int. J. Obes. 41, 497–501. doi: 10.1038/ijo.2016.219
Mueller, N. T., Whyatt, R., Hoepner, L., Oberfield, S., Dominguez-Bello, M. G., Widen, E. M., et al. (2015). Prenatal exposure to antibiotics, cesarean section and risk of childhood obesity. Int. J. Obes. 39, 665–670. doi: 10.1038/ijo.2014.180
Mulvihill, S. J., Stone, M. M., Debas, H. T., and Fonkalsrud, E. W. (1985). The role of amniotic fluid in fetal nutrition. J. Pediatr. Surg. 20, 668–672. doi: 10.1016/s0022-3468(85)80021-x
Murphy, M. C., and Fox, E. A. (2010). Mice deficient in brain-derived neurotrophic factor have altered development of gastric vagal sensory innervation. J. Comp. Neurol. 518, 2934–2951. doi: 10.1002/cne.22372
Mutoh, H., Naya, F. J., Tsai, M. J., and Leiter, A. B. (1998). The basic helix-loop-helix protein beta2 interacts with p300 to coordinate differentiation of secretin-expressing enteroendocrine cells. Genes Dev. 12, 820–830. doi: 10.1101/gad.12.6.820
Naya, F. J., Huang, H. P., Qiu, Y., Mutoh, H., DeMayo, F. J., Leiter, A. B., et al. (1997). Diabetes, defective pancreatic morphogenesis and abnormal enteroendocrine differentiation in BETA2/NeuroD-deficient mice. Genes Dev. 11, 2323–2334. doi: 10.1101/gad.11.18.2323
O’Leary, O. F., Ogbonnaya, E. S., Felice, D., Levone, B. R., Conroy, L. C., Fitzgerald, P., et al. (2018). The vagus nerve modulates BDNF expression and neurogenesis in the hippocampus. Eur. Neuropsychopharmacol. 28, 307–316. doi: 10.1016/j.euroneuro.2017.12.004
Pearse, A. G. (1969). The calcitonin secreting C cells and their relationship to the APUD cell series. J. Endocrinol. 45, 13–14.
Penkova, N. I., Baltadjiev, G. A., Koeva, Y. A., Atanassova, P. K., Andonov, V. N., and Trichkova, V. A. (2010). Prenatal and postnatal differentiation of the small intestine in rat. Folia Med. 52, 54–62.
Qian, B. F., el-Salhy, M., Danielsson, A., Shalaby, A., and Axelsson, H. (1999). Effects of unilateral cervical vagotomy on antral endocrine cells in mouse. Histol. Histopathol. 14, 705–709. doi: 10.14670/HH-14.705
Raab, M., Wörl, J., Brehmer, A., and Neuhuber, W. L. (2003). Reduction of NT-3 or TrkC results in fewer putative vagal mechanoreceptors in the mouse esophagus. Auton. Neurosci. Basic Clin. 108, 22–31. doi: 10.1016/j.autneu.2003.08.003
Ratcliffe, E. M., Fan, L., Mohammed, T. J., Anderson, M., Chalazonitis, A., and Gershon, M. D. (2011a). Enteric neurons synthesize netrins and are essential for the development of the vagal sensory innervation of the fetal gut. Dev. Neurobiol. 71, 362–373. doi: 10.1002/dneu.20869
Ratcliffe, E. M., Farrar, N. R., and Fox, E. A. (2011b). Development of the vagal innervation of the gut: Steering the wandering nerve. Neurogastroenterol. Motil. 23, 898–911. doi: 10.1111/j.1365-2982.2011.01764.x
Ratcliffe, E. M., Setru, S. U., Chen, J. J., Li, Z. S., D’Autreaux, F., and Gershon, M. D. (2006). Netrin/DCC-Mediated Attraction of Vagal Sensory Axons to the Fetal Mouse Gut. J. Comp. Neurol. 498, 567–580. doi: 10.1002/cne.21027
Rogers, G. J., Tolhurst, G., Ramzan, A., Habib, A. M., Parker, H. E., Gribble, F. M., et al. (2011). Electrical activity-triggered glucagon-like peptide-1 secretion from primary murine L-cells. J. Physiol. 589, 1081–1093. doi: 10.1113/jphysiol.2010.198069
Rozengurt, E., and Sternini, C. (2007). Taste receptor signaling in the mammalian gut. Curr. Opin. Pharmacol. 7, 557–562. doi: 10.1016/j.coph.2007.10.002
Schaal, B., Marlier, L., and Soussignan, R. (1998). Olfactory function in the human fetus: Evidence from selective neonatal responsiveness to the odor of amniotic fluid. Behav. Neurosci. 112, 1438–1449. doi: 10.1037/0735-7044.112.6.1438
Schonhoff, S. E., Giel-Moloney, M., and Leiter, A. B. (2004). Neurogenin 3-expressing progenitor cells in the gastrointestinal tract differentiate into both endocrine and non-endocrine cell types. Dev. Biol. 270, 443–454. doi: 10.1016/j.ydbio.2004.03.013
Sclafani, A., and Ackroff, K. (2012). Role of gut nutrient sensing in stimulating appetite and conditioning food preferences. Am. J. Physiol. Regul. Integr. Comp. Physiol. 302, R1119–R1133. doi: 10.1152/ajpregu.00038.2012
Skeath, J. B., and Carroll, S. B. (1991). Regulation of achaete-scute gene expression and sensory organ pattern formation in the Drosophila wing. Genes Dev. 5, 984–995. doi: 10.1101/gad.5.6.984
Soehartono, R. H., Kitamura, N., Yamagishi, N., Taguchi, K., Yamada, J., and Yamada, H. (2002). An immunohistochemical study of endocrine cells in the abomasum of vagotomized calf. J. Vet. Med. Sci. 64, 11–15. doi: 10.1292/jvms.64.11
Yang, Q., Bermingham, N. A., Finegold, M. J., and Zoghbi, H. Y. (2001). Requirement of Math1 for secretory cell lineage commitment in the mouse intestine. Science 294, 2155–2158. doi: 10.1126/science.1065718
Yin, C., and Xi, R. (2018). A phyllopod-mediated feedback loop promotes intestinal stem cell enteroendocrine commitment in Drosophila. Stem Cell Reports 10, 43–57. doi: 10.1016/j.stemcr.2017.11.014
Yu, Y., Lu, L., Sun, J., Petrof, E. O., and Claud, E. C. (2016). Preterm infant gut microbiota affects intestinal epithelial development in a humanized microbiome gnotobiotic mouse model. Am. J. Physiol. Gastrointest. Liver Physiol. 311, G521–G532. doi: 10.1152/ajpgi.00022.2016
Keywords: enteroendocrine cell, gut-brain axis, sensory neurodevelopment, fetal microbiome, prenatal nutrition
Citation: Buchanan KL and Bohórquez DV (2018) You Are What You (First) Eat. Front. Hum. Neurosci. 12:323. doi: 10.3389/fnhum.2018.00323
Received: 26 May 2018; Accepted: 25 July 2018;
Published: 13 August 2018.
Edited by:
John R. Lukens, University of Virginia, United StatesReviewed by:
Prajwal Gurung, University of Iowa, United StatesSonia Villapol, Houston Methodist Research Institute, United States
Copyright © 2018 Buchanan and Bohórquez. This is an open-access article distributed under the terms of the Creative Commons Attribution License (CC BY). The use, distribution or reproduction in other forums is permitted, provided the original author(s) and the copyright owner(s) are credited and that the original publication in this journal is cited, in accordance with accepted academic practice. No use, distribution or reproduction is permitted which does not comply with these terms.
*Correspondence: Diego V. Bohórquez, ZGllZ28uYm9ob3JxdWV6QGR1a2UuZWR1