- 1Shanghai Key Laboratory of Psychotic Disorders, Shanghai Mental Health Center, Shanghai Jiao Tong University School of Medicine, Shanghai, China
- 2College of Education, Shanghai Normal University, Shanghai, China
- 3Department of Psychology, Qiqihar Mental Health Center, Qiqihar, China
- 4Guangji Hospital of Suzhou, Suzhou, China
- 5Department of Psychiatry, Tongji Hospital of Tongji University, Shanghai, China
- 6Nathan S. Kline Institute for Psychiatric Research, New York, NY, USA
- 7Shanghai Key Laboratory of Magnetic Resonance, Department of Physics, East China Normal University, Shanghai, China
- 8Key Laboratory for the Genetics of Developmental and Neuropsychiatric Disorders, Bio-X Institutes, Ministry of Education, Shanghai Jiao Tong University, Shanghai, China
- 9Brain Science and Technology Research Center, Shanghai Jiao Tong University, Shanghai, China
- 10CAS Key Laboratory of Behavioral Science and MRI Research Center, Institute of Psychology, Chinese Academy of Sciences, Beijing, China
The amygdala and the dorsolateral prefrontal cortex (DLPFC) play important roles in “emotion dysregulation,” which has a profound impact on etiologic research of generalized anxiety disorder (GAD). The present study analyzed both eyes-open and eyes-closed resting state functional MRI (rs-fMRI) of 43 subjects (21 GAD patients with medicine free and 22 matched healthy controls). The amygdala and the DLPFC were defined as regions of interest (ROI) to analyze functional connectivity (FC) in GAD patients compared with healthy controls. The main findings revealed GAD patients had increased FC between the amygdala and the temporal pole compared to healthy controls, which was found in both eyes-open and eyes-closed rs-fMRI. And altered FC between the ROIs and brain regions that mainly belonged to the default mode network (DMN) were found. These findings suggest that the abnormal FC between the amygdala and the temporal pole may contribute to the pathophysiology of GAD, and provide insights into the current understanding of the emotion dysregulation of anxiety disorders.
Introduction
Anxiety disorders are the most common of all mental disorders with 30% prevalence in the population, and they significantly contribute to the economic burden of disease (Andlin-Sobocki and Wittchen, 2005; Kessler et al., 2005; Bereza et al., 2009). Among anxiety disorders, generalized anxiety disorder (GAD) is the most common type (Roy-Byrne and Wagner, 2004; Lieb et al., 2005; Kroenke et al., 2007). GAD is characterized by excessive and continuous worry, anxiety, and apprehension. It may also produce distress and/or functional impairments.
Previous studies have argued that “emotional dysregulation,” the inability to control or regulate emotional responses, may be responsible for the development of GAD. This hypothesis is grounded in the observation that individuals with GAD concentrate their attention on threatening thoughts. This cognitive model has been widely adopted for understanding GAD (Mathews and MacLeod, 1985; Bar-Haim et al., 2007; Amir et al., 2009; Behar et al., 2009).
The dorsolateral prefrontal cortex (DLPFC), an important region for performing cognitive operations during the regulation of emotional responses, has been shown to play a key role in the pathophysiology of GAD (MacDonald et al., 2000; Miller and Cohen, 2001; Blasi et al., 2007; Meyer et al., 2011; Moon et al., 2015). Altered activation of the DLPFC in patients with GAD has been associated with emotional dysregulation and attention deficit. Functional MRI studies have reported increased activity of the DLPFC under affective stroop and emotion reappraisal tasks in patients with GAD (Ball et al., 2012; Blair et al., 2012). Additionally, functional abnormalities of the amygdala, known as the most prominent “fear-circuit” structure in the brain that plays a central role in automatic affective processing, have been found in most anxiety disorders (LeDoux, 2000; Anderson et al., 2003; Ohman, 2005; Etkin and Wager, 2007; Adolphs, 2008; Shin and Liberzon, 2010; Linares et al., 2012). The amygdala has also demonstrated responsibility for facilitating perceptual processing and bottom-up emotional control in individuals with GAD (LeDoux, 2000; Davis and Whalen, 2001; Phelps, 2006). Models of emotional regulation have therefore focused primarily on the DLPFC and the amygdala. Accordingly, we assume that individuals with GAD display aberrant FC seeded from the amygdala and the DLPFC compared to healthy controls.
Studies have shed less light on the relationship between the temporal cortex, especially the temporal pole, and GAD. Although the function of the temporal pole is not well understood, a damaged temporal pole can impair ability to use experiential knowledge and therefore may cause affective symptoms (Funnell, 2001). The temporal cortex binds complex, highly processed perceptual inputs to visceral emotional responses (Olson et al., 2007). The temporal pole is located at the end of the ventral visual stream and is strongly interconnected with the amygdala (Nakamura and Kubota, 1996; Stefanacci and Amaral, 2002). It integrates conceptual knowledge and meaning with semantic, visual and auditory information (Carlson et al., 2014), and influences emotions via top-down modulations (Pehrs et al., 2015). Studies have reported altered functional connectivity (FC) between the temporal pole and the amygdala in anxiety disorders (Aghajani et al., 2014; Modi et al., 2015). This finding has prompted us to explore the FC between the temporal pole and the amygdala in GAD patients. Therefore, one of our hypotheses is that altered FC between these two areas is attributed to the etiology of GAD.
Accordingly, the amygdala and the DLPFC will be defined as regions of interest (ROIs) to explore in GAD patients. Because the number of volumes in the eyes-open resting state fMRI (rs-fMRI) we collected was too small, we also analyzed the eyes-closed rs-fMRI using the same protocol to improve the reliability of our results.
Materials and Methods
Participants
All participants received the Mini-International Neuropsychiatric Interview (MINI), Chinese version (Si et al., 2009). Twenty two GAD patients who met the criteria for DSM-IV (Association, 2000) and who were not found to have lifetime psychosis, substance dependence or severe somatic diseases were recruited from the psychological outpatient clinic at the Shanghai Mental Health Center. We excluded patients who had comorbid moods or other anxiety disorders. Twenty one healthy controls were recruited from local communities and Shanghai Jiao Tong University. Controls were matched for gender, age, education level, and did not meet DSM-IV criteria for lifetime mood, anxiety, psychotic, or substance dependence disorders. Forty three participants were enrolled in total for this study and according to the Edinburgh Inventory (Oldfield, 1971), all of them are right-handed adults free of psychotropic medications for at least 2 weeks before enrollment. The study was conducted between August 2011 and November 2012.
This study was approved by the Research Ethics Committee of Shanghai Mental Health Center, China (SMHC-IRB 201217). Written informed consent was acquired from every participant.
All participants were informed of the safety and eligibility criteria for fMRI scanning: no neurological conditions and no implanted ferrous metal. The Hamilton Rating Scale for Anxiety (HAMA) (Hamilton, 1959) and Hamilton Rating Scale for Depression (HAMD) (Hamilton, 1967) were administered to all participants on the day of scanning. Demographic and clinical characteristics of the 43 participants are shown in Table 1.
Image Acquisition
Images were obtained using a Siemens Trio 3.0 Tesla MRI scanner (Siemens, Erlangen, Germany) with a standard 12-channel head coil. Restraining foam pads was used to reduce head motion and earplugs were used to reduce scanner noise. High-resolution T1-weighted anatomical images (repetition time (TR) = 1900 ms, echo time (TE) = 2.46 ms, flip angle = 9 degrees, 32 transverse slices, field of view (FOV) = 240 × 240 mm, matrix = 256 × 256, slice thickness = 1 mm) were acquired using a magnetization prepared rapid gradient-echo sequence. Resting-state functional MRI data were acquired using a single-shot, gradient-recalled echo planar imaging sequence (TR = 2000 ms, TE = 25 ms, flip angle = 90 degrees). 32 transverse slices (FOV = 240 × 240 mm, matrix = 64 × 64, slice thickness = 5 mm) resulting in a total of 80/157 volumes and a scan time of 164/314 s, respectively, in eyes-open and eyes-closed rs-fMRI. During the scan, participants were instructed to stay aware. After the scan, the technicians would check the quality of structural images. If any abnormalities were found in the images, participants were re-scanned.
Data Processing and Analysis
Demographic and Clinical Data Analysis
Using Statistical Product and Service Solutions software 17.0 (SPSS, Inc., Chicago, Illinois), we conducted analysis of age, gender, years of education, HAMA, and HAMD. Independent sample t-tests for continuous variables and chi-square tests for categorical variables were used.
Resting-State fMRI Analysis
The Data Processing Assistant for Resting-State fMRI 2.0 (DPARSFA2.0, http://restfmri.net/forum/) (Chao-Gan and Yu-Feng, 2010), which works with the Statistical Parametric Mapping Software (SPM8, http://www.fil.ion.ucl.ac.uk/spm) (Friston et al., 1994) was used to analyze the rs-fMRI data. Preprocessing was completed in 7 steps: (1) Convert DICOM data to NIFTI format and remove first 10 time points of the image; (2) Slice timing correction and realignment of image; (3) Parallel movements in any direction >2.5 mm, or rotary movements >2.5 degree were excluded and subjects using a threshold of frame-wise displacement >0.5 mm (Power et al., 2012) were also excluded; (4) Spatial normalization to the standard Montreal Neurological Institute (MNI) echo-planar imaging template and the resampled voxel size was 3 × 3 × 3 mm; (5) Conduct Friston 24-parameter correction (Yan et al., 2013) to minimize the effect of head motion; (6) Smoothing with a Gaussian kernel of 8-mm full-width at half-maximum (FWHM); (7) After linear detrending, the functional data was band-pass filtered (pass frequence band: 0.01–0.1 Hz) to reduce the effects of low-frequency drift as well as high-frequency respiratory and cardiac noise (Biswal et al., 1995).
According to previous studies (Cieslik et al., 2013; Comte et al., 2014; Cui et al., 2016), the bilateral amygdala (MNI: 32,−2,−26;−28, 4,−22) and the bilateral DLPFC (MNI: 30, 43, 23;−51, 27, 30) were defined as ROIs. The peak voxel of each ROI and a 6 mm-radius sphere were selected to proceed with the FC analysis. The Pearson correlation coefficients were calculated between the ROI and the other voxels of the whole brain. Fisher's r-to-z transformation was used to convert correlation coefficients into z-scores so that the correlation coefficient would improve the normality of the data (Hampson et al., 2002; Chao-Gan and Yu-Feng, 2010; Song et al., 2011), and generate FC maps. Voxel-wise two-sample t-tests were conducted to compare group differences between GAD patients and controls. Spearman correlation analysis was performed in GAD patients to investigate the correlation between FC and disease severity (HAMA score). Both t-tests and correlation analysis were conducted under the BrainMask_61*73*61. As correction for multiple comparisons, a corrected threshold of p < 0.05 (two-tailed) was derived from a combined threshold of p < 0.005 for individual voxel with a cluster size >53 voxels. These threshold were determined using the 3dFWHM and 3dClustSim program in AFNI software (https://afni.nimh.nih.gov/afni, parameters: single voxel p < 0.005, 2000 Monte Carlo iterations, estimated FWHM = 9.5 mm, the BrainMask_61*73*61 was used as mask in estimation of smoothness and correction). Additionally, age, years of education, HAMD score and intracranial volume (ICV) were modeled as covariates. The analyses above were conducted in both eyes-open and eyes-closed rs-fMRI.
In order to improve the reliability of these results, we calculated mean frame-wise displacement for each group and conducted t-tests between matched groups in SPSS.
Results
Demographic and Clinical Characteristics
Compared with the healthy control group, the HAMA scores and HAMD scores were significantly different in GAD patients (P < 0.05). Except that, there is no significant difference between GAD patients and healthy controls.
Resting-State fMRI Results
Functional Connectivity
Compared with healthy controls, GAD patients showed increased FC between the left amygdala and the temporal pole both in eyes-open and eyes-closed rs-fMRI (P < 0.005 to define cluster, AlphaSim correction, cluster size >53 voxels, overall p < 0.05).
In eyes-open rs-fMRI, there was increased connectivity between the left amygdala and the inferior frontal gyrus in the GAD group compared with the control group (P < 0.005 to define cluster, AlphaSim correction, cluster size >53 voxels, overall p < 0.05). (Figure 1, Table 2) There was no significant abnormal FC between GAD subjects and controls seeded from the DLPFC.
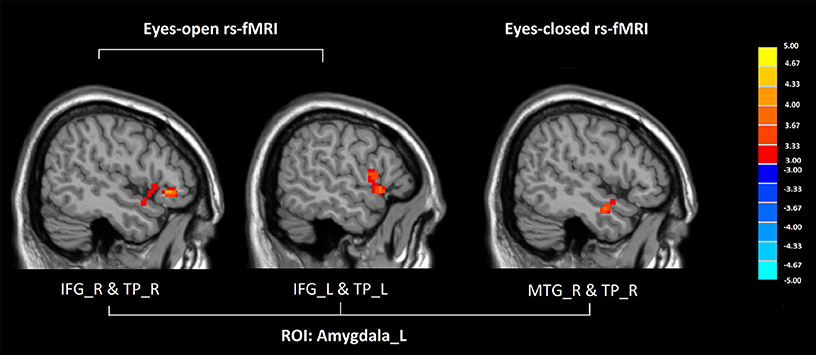
Figure 1. Altered functional connectivity seeded from the left amygdala in GAD, compared with HC (P < 0.005 to define cluster, AlphaSim correction, cluster size >53 voxels, overall p < 0.05). Hot colors indicate increased functional connectivity in GAD compared with HC. (GAD, generalized anxiety disorder; HC, healthy control; rs-fMRI, resting state fMRI; IFG, inferior frontal gyrus; TP, temporal gyrus; MTG, middle temporal gyrus; L, left; R, right).
In eyes-closed rs-fMRI, we found increased FC in the left amygdala with the middle temporal gyrus (P < 0.005 to define cluster, AlphaSim correction, cluster size >53 voxels, overall p < 0.05). Decreased FC between the left DLPFC and the precuneus, the lingual gyrus, the calcarine sulcus, and the cerebellar vermis was detected in GAD subjects compared with healthy controls (P < 0.005 to define cluster, AlphaSim correction, cluster size >53 voxels, overall p < 0.05). There was decreased connectivity between the right DLPFC and the medial prefrontal cortex (mPFC), the dorsal anterior cingulate cortex (dACC), the middle temporal gyrus, the angular gyrus, the precuneus, the calcarine sulcus, and the cerebellar vermis in GAD subjects compared with healthy controls (P < 0.005 to define cluster, AlphaSim correction, cluster size >53 voxels, overall p < 0.05). (Figure 2, Table 2).
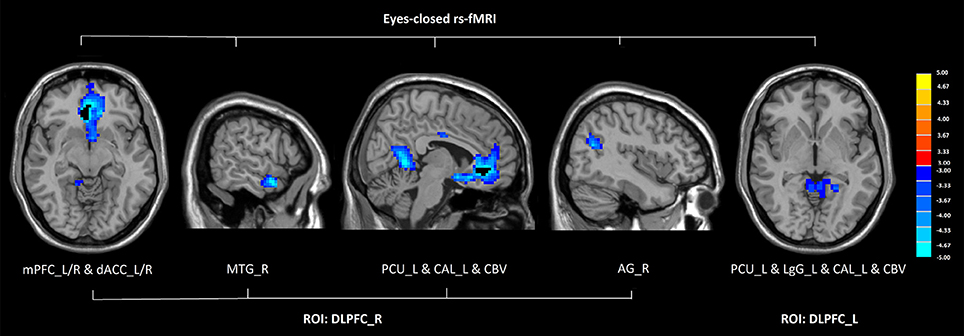
Figure 2. Altered functional connectivity seeded from the bilateral DLPFC in GAD, compared with HC (P < 0.005 to define cluster, AlphaSim correction, cluster size >53 voxels, overall p < 0.05). Hot and cold colors indicate increased and decreased FC in GAD compared with HC. (DLPFC, dorsolateral prefrontal cortex; GAD, generalized anxiety disorder; HC, healthy control; rs-fMRI, resting state fMRI; mPFC, medial prefrontal cortex; dACC, dorsal anterior cingulate cortex; MTG, middle temporal gyrus; PCU, precuneus; CAL, calcarine sulcus; CBV, cerebellar vermis; AG, angular gyrus; LgG, lingual gyrus; L, left; R, right).
Correlation Analysis of FC and Illness Severity
In eyes-open rs-fMRI, the HAMA score had a significant negative correlation with the FC between the left amygdala and the superior frontal gyrus in GAD subjects (P < 0.005 to define cluster, AlphaSim correction, cluster size >53 voxels, overall p < 0.05). The FC between the right amygdala and the fusiform gyrus, the superior/middle occipital gyrus, and the cerebellum was positively correlated to the HAMA score in GAD subjects (P < 0.005 to define cluster, AlphaSim correction, cluster size >53 voxels, overall p < 0.05). We found that the HAMA score was positively correlated with the FC between the DLPFC and the inferior frontal gyrus, the supplementary motor area (SMA), and the cerebellum in GAD subjects (P < 0.005 to define cluster, AlphaSim correction, cluster size >53 voxels, overall p < 0.05). (Table 3, Supplement Figure 1)
In eyes-closed rs-fMRI, the HAMA score had a significant positive correlation with the FC between the right DLPFC and the lingual gyrus, the cuneus, the fusiform gyrus, the inferior/middle occipital gyrus, and the cerebellum in GAD subjects (P < 0.005 to define cluster, AlphaSim correction, cluster size >53 voxels, overall p < 0.05). (Table 3, Supplement Figure 1).
Additionally, both in eyes-open and eyes-closed rs-fMRI, we did not find a significant difference in frame-wise displacement between GAD patients and healthy controls [p = 0.926 (eyes-open), 0.271 (eyes-closed)].
Discussion
In this study, we conducted analysis to characterize alterations in FC that may show the pathological basis of GAD using both the eyes-open and the eyes-closed rs-fMRI. While exploring FC seeded from the amygdala and the DLPFC, we found: (1) Patients with GAD showed increased FC between the left amygdala and the temporal pole compared with health controls. (2) In both eyes-open and eyes-closed conditions, the brain regions showed altered FC with amygdala/DLPFC were primarily from the default mode network (DMN).
Increased FC between the amygdala and the temporal pole was detected by both eyes-open and eyes-closed rs-fMRI. This finding may illustrate that the altered FC may contribute to the etiology of GAD. Previous findings indicate that the temporal pole plays a role in both social and emotional processing, including specific recognition, theory of mind (Wong and Gallate, 2012), memory (Damasio et al., 1996), and encodes similarity relations among different concepts (Patterson et al., 2007). It is also thought to be involved in access to knowledge during “mentalizing,” which refers to the attribution of intentions and other mental states (Frith and Frith, 2003). The amygdala is one of the most investigated structures of the brain, especially in the context of emotional processing. The amygdala is marked as the most prominent “fear-circuit” structure, and hyperactivation of the amygdala is found in most anxiety disorders (Etkin and Wager, 2007; Shin and Liberzon, 2010; Linares et al., 2012). And it has been studied extensively within the context of fear conditioning and extinction as key processes for the pathophysiology of anxiety disorders. The FC between the amygdala and the temporal pole may reflect integration of emotional regulation with knowledge during stimuli perception and mentalizing in healthy subjects. This neural process is presumably disrupted in GAD, which is consistent with observations of deficits in socio-emotional behaviors (Aghajani et al., 2014). The increased connectivity between the temporal pole and the amygdala in GAD patients may be responsible for why stimuli more easily evoke anxiety in GAD patients.
The default mode network (DMN) is defined as the set of regions in the brain that are consistently more activated during resting condition than other brain networks (Fox and Raichle, 2007). It is often described as a unitary, homogeneous system that is largely involved in the integration of autobiographical memories and in self-monitoring, in the retrieval and manipulation of past events in an effort to solve problems and develop future plans, and in emotion regulation (Greicius et al., 2003). When a task requires attention, however, the activation of such network is suppressed. Deficits in DMN suppression are reported in several mental illnesses, notably anxiety disorders (Anticevic et al., 2012). Our results showed altered FC between the amygdala and several regions of the brain including the temporal pole, the middle temporal gyrus, which belong to the DMN, in the GAD group compared with the control group. Altered FC was also found between the DLPFC and brain regions of the DMN, such as the mPFC, the angular gyrus, and the precuneus in the GAD group.
The middle temporal gyrus is regarded as an important brain structure in the integration of memory, audiovisual association, object-recognition and visual perception (Li et al., 2013; Shao et al., 2013). The middle temporal gyrus was found to have increased FC between the amygdala. This finding may reflect an increased predisposition for inaccurate interpretation of stimuli (Pannekoek et al., 2013). However, the FC between the DLPFC and the middle temporal gyrus, and the mPFC was decreased in GAD patients compared with healthy controls. The DLPFC is involved in the function of working memory, executive functions, emotion regulation, subjective feelings, and self-awareness (Craig, 2002; Critchley et al., 2004). The mPFC is widely known to be crucial for emotion regulation, especially for controlling negative emotional responses (Etkin et al., 2009). The angular gyrus, which is also a part of the DMN, has been implicated in affective regulation associated with empathic response, anxiety, and mood (Leung et al., 2013). The precuneus is implicated in episodic memory, visuospatial processing, self-reflection and aspects of consciousness (Fox et al., 2015; Hannawi et al., 2015; Kwok and Macaluso, 2015). The decreased FC between the DLPFC and the brain regions in the DMN may explain uncontrolled emotional regulation in GAD patients. This finding was consistent with our previous study and the models of “bottom-up” and “top-down” emotional processing (Phillips et al., 2003, 2008; Ochsner and Gross, 2005; Phan et al., 2005; Goldin et al., 2008; Cui et al., 2016).
The lingual gyrus, the cuneus, and the fusiform gyrus all belong to the visual network (VN). Functional abnormalities of these regions reflect excessive vigilance as a hallmark of anxiety disorders. The VN is associated with pathological memories and planning a response to potentially threatening stimuli (Bremner et al., 1999). The dACC, a part of the salience network (SN), plays a central role in detecting emotional salience and triggering cognitive control via FC with the DLPFC (Sridharan et al., 2008; Bressler and Menon, 2010). Both the DLPFC and the dorsal ACC are implicated in emotional regulation circuits (Bush et al., 2000; Brühl et al., 2014). Therefore, the decreased FC between the DLPFC and the VN/SN may be related to the loss of emotional regulation from the DLPFC in GAD patients. The cerebellum is linked with the cerebrum, brainstem, and spinal cord through efferent and afferent fibers, and the cerebellar vermis is connected to the amygdala anatomically in animals (De Bellis et al., 2002). Recently, more and more studies have reported the cerebellum is functionally related to expressing fear and processing fear memory (Supple et al., 1987; Sacchetti et al., 2005). Cerebellar cognitive affective syndrome was observed in patients with cerebellar damage (Stoodley, 2012). The DLPFC is involved in executive control (Habas et al., 2009; O'Reilly et al., 2010; Yeo et al., 2011), so the connectivity between the cerebellum and the DLPFC may mediate anxiety (Caulfield et al., 2016). And it supports our finding that the decreased FC between the DLPFC and the cerebellum in GAD patients compared with healthy controls.
Regarding the underlying mechanism, the temporal lobe, especially the temporal pole, may be the emphasis for future treatment of GAD. Although there some studies reported the effect of transcranial direct current stimulation (tDSC) and repetitive transcranial magnetic stimulation (rTMS) in curing GAD, the outcomes are various. According to our findings, we prefer the temporal pole as the stimulated target. By decreasing the related abnormal FC may remit anxiety in GAD patients.
Conclusion and Limitations
In conclusion, our study found altered FC seeded from the amygdala and the DLPFC in GAD patients using eyes-open and eyes-closed rs-fMRI. We found that the increased FC between the amygdala and the temporal pole may be underlying the neural pathophysiology of GAD. We hope these findings will shed light on the current understanding of GAD and on advanced therapeutic interventions.
A limitation in this study is that we were only able to acquire 164 s in the eyes-open resting state fMRI data and the reliability of FC analysis under this condition is limited (Shehzad et al., 2009; Thomason et al., 2011; Braun et al., 2012; Li et al., 2012). Nonetheless, the consistency between eyes-open and eyes-closed conditions alleviates this concern and provides support for our conclusion. Additionally, as fMRI data was acquired using the parameters TR = 2s, slices = 30, band pass filtering in the range 0.01 < f < 0.1 Hz, cardiac and respiratory fluctuations may still reduce the specificity of low frequency fluctuations to functional connected regions (Lowe et al., 1998). Future research will focus on MRI follow-up and will explore changes in neuroimaging of GAD.
Author Contributions
WL: manuscript, data gathering and analysis. HC, ZZ, QH, LZ, JM, and HL: manuscript and data gathering. LK, JJ, QG, and YZ: manuscript and analysis. JL and QL: data gathering and quality control. JW and ZY: manuscript, administration, and editing. CL: research plan development, manuscript, administration, and editing.
Funding
Funding for this study was provided by the National Natural Science Foundation of China (81071098, 81270023, 81571756)(to CL and ZY), Shanghai Health System Leadership in Health Research Program (XBR2011005) (to CL), Shanghai Health Bureau Project (2013SY003) (to JW), the Science and Technology Commission of Shanghai Municipality (13dz2260500, 15411950201, 14411961400, 20154Y0080) (to CL, ZZ, and JW), Shanghai Clinical Center for Mental Disorders (2014), National Key Clinical Disciplines at Shanghai Mental Health Center (Office of Medical Affairs, Ministry of Health, 2011-873; OMA-MH, 2011-873)(to HC), Beijing Nova Program for Science and Technology (XXJH2015B079)(to ZY), Pfizer Investigator Initiation Research Fund (WI173560)(to CL) and Chinese Government Scholarship (CSC NO.201606230115)(to WL).
Conflict of Interest Statement
The authors declare that the research was conducted in the absence of any commercial or financial relationships that could be construed as a potential conflict of interest.
Acknowledgments
The authors wish to thank all the subjects for their participation in the study.
Supplementary Material
The Supplementary Material for this article can be found online at: http://journal.frontiersin.org/article/10.3389/fnhum.2016.00549/full#supplementary-material
References
Adolphs, R. (2008). Fear, faces, and the human amygdala. Curr. Opin. Neurobiol. 18, 166–172. doi: 10.1016/j.conb.2008.06.006
Aghajani, M., Veer, I. M., van Tol, M. J., Aleman, A., van Buchem, M. A., Veltman, D. J., et al. (2014). Neuroticism and extraversion are associated with amygdala resting-state functional connectivity. Cogn. Affect. Behav. Neurosci. 14, 836–848. doi: 10.3758/s13415-013-0224-0
Amir, N., Beard, C., Burns, M., and Bomyea, J. (2009). Attention modification program in individuals with generalized anxiety disorder. J. Abnorm. Psychol. 118, 28–33. doi: 10.1037/a0012589
Anderson, A. K., Christoff, K., Panitz, D., De Rosa, E., and Gabrieli, J. D. (2003). Neural correlates of the automatic processing of threat facial signals. J. Neurosci. 23, 5627–5633.
Andlin-Sobocki, P., and Wittchen, H. U. (2005). Cost of anxiety disorders in Europe. Eur. J. Neurol. 12(Suppl. 1), 39–44. doi: 10.1111/j.1468-1331.2005.01196.x
Anticevic, A., Cole, M. W., Murray, J. D., Corlett, P. R., Wang, X.-J., and Krystal, J. H. (2012). The role of default network deactivation in cognition and disease. Trends Cogn. Sci. 16, 584–592. doi: 10.1016/j.tics.2012.10.008
Association, A. P. (2000). Diagnostic and Statistical Manual of Mental Disorders. 4. Washington, DC: American Psychiatric Association.
Ball, T. M., Sullivan, S., Flagan, T., Hitchcock, C. A., Simmons, A., Paulus, M. P., et al. (2012). Selective effects of social anxiety, anxiety sensitivity, and negative affectivity on the neural bases of emotional face processing. Neuroimage 59, 1879–1887. doi: 10.1016/j.neuroimage.2011.08.074
Bar-Haim, Y., Lamy, D., Pergamin, L., Bakermans-Kranenburg, M. J., and van IJzendoorn, M. H. (2007). Threat-related attentional bias in anxious and nonanxious individuals: a meta-analytic study. Psychol. Bull. 133, 1–24. doi: 10.1037/0033-2909.133.1.1
Behar, E., DiMarco, I. D., Hekler, E. B., Mohlman, J., and Staples, A. M. (2009). Current theoretical models of generalized anxiety disorder (GAD): conceptual review and treatment implications. J. Anxiety Disord. 23, 1011–1023. doi: 10.1016/j.janxdis.2009.07.006
Bereza, B. G., Machado, M., and Einarson, T. R. (2009). Systematic review and quality assessment of economic evaluations and quality-of-life studies related to generalized anxiety disorder. Clin. Ther. 31, 1279–1308. doi: 10.1016/j.clinthera.2009.06.004
Biswal, B., Zerrin Yetkin, F. Z., Haughton, V. M., and Hyde, J. S. (1995). Functional connectivity in the motor cortex of resting human brain using echo-planar mri. Magn. Reson. Med. 34, 537–541. doi: 10.1002/mrm.1910340409
Blair, K. S., Geraci, M., Smith, B. W., Hollon, N., DeVido, J., Otero, M., et al. (2012). Reduced dorsal anterior cingulate cortical activity during emotional regulation and top-down attentional control in generalized social phobia, generalized anxiety disorder, and comorbid generalized social phobia/generalized anxiety disorder. Biol. Psychiatry 72, 476–482. doi: 10.1016/j.biopsych.2012.04.013
Blasi, G., Goldberg, T. E., Elvevåg, B., Rasetti, R., Bertolino, A., Cohen, J., et al. (2007). Differentiating allocation of resources and conflict detection within attentional control processing. Eur. J. Neurosci. 25, 594–602. doi: 10.1111/j.1460-9568.2007.05283.x
Braun, U., Plichta, M. M., Esslinger, C., Sauer, C., Haddad, L., Grimm, O., et al. (2012). Test–retest reliability of resting-state connectivity network characteristics using fMRI and graph theoretical measures. Neuroimage 59, 1404–1412. doi: 10.1016/j.neuroimage.2011.08.044
Bremner, J. D., Narayan, M., Staib, L. H., Southwick, S. M., McGlashan, T., and Charney, D. S. (1999). Neural correlates of memories of childhood sexual abuse in women with and without posttraumatic stress disorder. Am. J. Psychiatry 156, 1787–1795.
Bressler, S. L., and Menon, V. (2010). Large-scale brain networks in cognition: emerging methods and principles. Trends Cogn. Sci. 14, 277–290. doi: 10.1016/j.tics.2010.04.004
Brühl, A. B., Hänggi, J., Baur, V., Rufer, M., Delsignore, A., Weidt, S., et al. (2014). Increased cortical thickness in a frontoparietal network in social anxiety disorder. Hum. Brain Mapp. 35, 2966–2977. doi: 10.1002/hbm.22378
Bush, G., Luu, P., and Posner, M. I. (2000). Cognitive and emotional influences in anterior cingulate cortex. Trends Cogn. Sci. 4, 215–222. doi: 10.1016/S1364-6613(00)01483-2
Carlson, T. A., Simmons, R. A., Kriegeskorte, N., and Slevc, L. R. (2014). The emergence of semantic meaning in the ventral temporal pathway. J. Cogn. Neurosci. 26, 120–131. doi: 10.1162/jocn_a_00458
Caulfield, M. D., Zhu, D. C., McAuley, J. D., and Servatius, R. J. (2016). Individual differences in resting-state functional connectivity with the executive network: support for a cerebellar role in anxiety vulnerability. Brain Struct. Funct. 221, 3081–3093. doi: 10.1007/s00429-015-1088-6
Chao-Gan, Y., and Yu-Feng, Z. (2010). DPARSF: a MATLAB toolbox for “pipeline” data analysis of resting-state fMRI. Front. Syst. Neurosci. 4:13. doi: 10.3389/fnsys.2010.00013
Cieslik, E. C., Zilles, K., Caspers, S., Roski, C., Kellermann, T. S., Jakobs, O., et al. (2013). Is there “one” DLPFC in cognitive action control? Evidence for heterogeneity from co-activation-based parcellation. Cereb. Cortex 23, 2677–2689. doi: 10.1093/cercor/bhs256
Comte, M., Schön, D., Coull, J. T., Reynaud, E., Khalfa, S., Belzeaux, R., et al. (2014). Dissociating bottom-up and top-down mechanisms in the cortico-limbic system during emotion processing. Cereb. Cortex 26, 144–155. doi: 10.1093/cercor/bhu185
Craig, A. D. (2002). How do you feel? Interoception: the sense of the physiological condition of the body. Nat. Rev. Neurosci. 3, 655–666. doi: 10.1038/nrn894
Critchley, H. D., Wiens, S., Rotshtein, P., and Öhman, A., Dolan, R. J. (2004). Neural systems supporting interoceptive awareness. Nat. Neurosci. 7, 189–195. doi: 10.1038/nn1176
Cui, H., Zhang, J., Liu, Y., Li, Q., Li, H., Zhang, L., et al. (2016). Differential alterations of resting-state functional connectivity in generalized anxiety disorder and panic disorder. Hum. Brain Mapp. 37, 1459–1473. doi: 10.1002/hbm.23113
Damasio, H., Grabowski, T. J., Tranel, D., Hichwa, R. D., and Damasio, A. R. (1996). A neural basis for lexical retrieval. Nature 380, 499–505. doi: 10.1038/380499a0
Davis, M., and Whalen, P. J. (2001). The amygdala: vigilance and emotion. Mol. Psychiatry 6, 13–34. doi: 10.1038/sj.mp.4000812
De Bellis, M. D., Keshavan, M. S., Shifflett, H., Iyengar, S., Dahl, R. E., Axelson, D. A., et al. (2002). Superior temporal gyrus volumes in pediatric generalized anxiety disorder. Biol. Psychiatry 51, 553–562. doi: 10.1016/S0006-3223(01)01375-0
Etkin, A., Prater, K. E., Schatzberg, A. F., Menon, V., and Greicius, M. D. (2009). Disrupted amygdalar subregion functional connectivity and evidence of a compensatory network in generalized anxiety disorder. Arch. Gen. Psychiatry 66, 1361–1372. doi: 10.1001/archgenpsychiatry.2009.104
Etkin, A., and Wager, T. D. (2007). Functional neuroimaging of anxiety: a meta-analysis of emotional processing in PTSD, social anxiety disorder, and specific phobia. Am. J. Psychiatry 164, 1476–1488. doi: 10.1176/appi.ajp.2007.07030504
Fox, K. C. R., Spreng, R. N., Ellamil, M., Andrews-Hanna, J. R., and Christoff, K. (2015). The wandering brain: meta-analysis of functional neuroimaging studies of mind-wandering and related spontaneous thought processes. Neuroimage 111, 611–621. doi: 10.1016/j.neuroimage.2015.02.039
Fox, M. D., and Raichle, M. E. (2007). Spontaneous fluctuations in brain activity observed with functional magnetic resonance imaging. Nat. Rev. Neurosci. 8, 700–711. doi: 10.1038/nrn2201
Friston, K. J., Holmes, A. P., Worsley, K. J., Poline, J. P., Frith, C. D., and Frackowiak, R. S. (1994). Statistical parametric maps in functional imaging: a general linear approach. Hum. Brain Mapp. 2, 189–210. doi: 10.1002/hbm.460020402
Frith, U., and Frith, C. D. (2003). Development and neurophysiology of mentalizing. Philos. Trans. R. Soc. Lond. B Biol. Sci. 358, 459–473. doi: 10.1098/rstb.2002.1218
Funnell, E. (2001). Evidence for scripts in semantic dementia: implications for theories of semantic memory. Cogn. Neuropsychol. 18, 323–341. doi: 10.1080/02643290042000134
Goldin, P. R., McRae, K., Ramel, W., and Gross, J. J. (2008). The neural bases of emotion regulation: reappraisal and suppression of negative emotion. Biol. Psychiatry 63, 577–586. doi: 10.1016/j.biopsych.2007.05.031
Greicius, M. D., Krasnow, B., Reiss, A. L., and Menon, V. (2003). Functional connectivity in the resting brain: a network analysis of the default mode hypothesis. Proc. Natl. Acad. Sci. U.S.A. 100, 253–258. doi: 10.1073/pnas.0135058100
Habas, C., Kamdar, N., Nguyen, D., Prater, K., Beckmann, C. F., Menon, V., et al. (2009). Distinct cerebellar contributions to intrinsic connectivity networks. J. Neurosci. 29, 8586–8594. doi: 10.1523/JNEUROSCI.1868-09.2009
Hamilton, M. (1959). The assessment of anxiety states by rating. Br. J. Med. Psychol. 32, 50–55. doi: 10.1111/j.2044-8341.1959.tb00467.x
Hamilton, M. (1967). Development of a rating scale for primary depressive illness. Br. J. Soc. Clin. Psychol. 6, 278–296. doi: 10.1111/j.2044-8260.1967.tb00530.x
Hampson, M., Peterson, B. S., Skudlarski, P., Gatenby, J. C., and Gore, J. C. (2002). Detection of functional connectivity using temporal correlations in MR images. Hum. Brain Mapp. 15, 247–262. doi: 10.1002/hbm.10022
Hannawi, Y., Lindquist, M. A., Caffo, B. S., Sair, H. I., and Stevens, R. D. (2015). Resting brain activity in disorders of consciousness A systematic review and meta-analysis. Neurology 84, 1272–1280. doi: 10.1212/WNL.0000000000001404
Kessler, R. C., Berglund, P., Demler, O., Jin, R., Merikangas, K. R., and Walters, E. E. (2005). Lifetime prevalence and age-of-onset distributions of DSM-IV disorders in the national comorbidity survey replication. Arch. Gen. Psychiatry 62, 593–602. doi: 10.1001/archpsyc.62.6.593
Kroenke, K., Spitzer, R. L., Williams, J. B., Monahan, P. O., and Lowe, B. (2007). Anxiety disorders in primary care: prevalence, impairment, comorbidity, and detection. Ann. Intern. Med. 146, 317–325. doi: 10.7326/0003-4819-146-5-200703060-00004
Kwok, S. C., and Macaluso, E. (2015). Immediate memory for “when, where and what:” short-delay retrieval using dynamic naturalistic material. Hum. Brain Mapp. 36, 2495–2513. doi: 10.1002/hbm.22787
LeDoux, J. E. (2000). Emotion circuits in the brain. Annu. Rev. Neurosci. 23, 155–184. doi: 10.1146/annurev.neuro.23.1.155
Leung, M.-K., Chan, C. C. H., Yin, J., Lee, C.-F., So, K.-F., and Lee, T. M. C. (2013). Increased gray matter volume in the right angular and posterior parahippocampal gyri in loving-kindness meditators. Soc. Cogn. Affect. Neurosci. 8, 34–39. doi: 10.1093/scan/nss076
Li, R., Wang, S., Zhu, L., Guo, J., Zeng, L., Gong, Q., et al. (2013). Aberrant functional connectivity of resting state networks in transient ischemic attack. PLoS ONE 8:e71009. doi: 10.1371/journal.pone.0071009
Li, Z., Kadivar, A., Pluta, J., Dunlop, J., and Wang, Z. (2012). Test–retest stability analysis of resting brain activity revealed by blood oxygen level-dependent functional MRI. J. Magn. Reson. Imaging 36, 344–354. doi: 10.1002/jmri.23670
Lieb, R., Becker, E., and Altamura, C. (2005). The epidemiology of generalized anxiety disorder in Europe. Eur. Neuropsychopharmacol. 15, 445–452. doi: 10.1016/j.euroneuro.2005.04.010
Linares, I. M., Trzesniak, C., Chagas, M. H., Hallak, J. E., Nardi, A. E., and Crippa, J. A. (2012). Neuroimaging in specific phobia disorder: a systematic review of the literature. Rev. Bras. Psiquiatr. 34, 101–111. doi: 10.1016/S1516-4446(12)70017-X
Lowe, M. J., Mock, B. J., and Sorenson, J. A. (1998). Functional connectivity in single and multislice echoplanar imaging using resting-state fluctuations. Neuroimage 7, 119–132. doi: 10.1006/nimg.1997.0315
MacDonald, A. W. III, Cohen, J. D., Stenger, V. A., and Carter, C. S. (2000). Dissociating the role of the dorsolateral prefrontal and anterior cingulate cortex in cognitive control. Science 288, 1835–1838. doi: 10.1126/science.288.5472.1835
Mathews, A., and MacLeod, C. (1985). Selective processing of threat cues in anxiety states. Behav. Res. Ther. 23, 563–569. doi: 10.1016/0005-7967(85)90104-4
Meyer, T., Qi, X. L., Stanford, T. R., and Constantinidis, C. (2011). Stimulus selectivity in dorsal and ventral prefrontal cortex after training in working memory tasks. J. Neurosci. 31, 6266–6276. doi: 10.1523/JNEUROSCI.6798-10.2011
Miller, E. K., and Cohen, J. D. (2001). An integrative theory of prefrontal cortex function. Annu. Rev. Neurosci. 24, 167–202. doi: 10.1146/annurev.neuro.24.1.167
Modi, S., Kumar, M., Kumar, P., and Khushu, S. (2015). Aberrant functional connectivity of resting state networks associated with trait anxiety. Psychiatry Res. 234, 25–34. doi: 10.1016/j.pscychresns.2015.07.006
Moon, C. M., Kang, H. K., and Jeong, G. W. (2015). Metabolic change in the right dorsolateral prefrontal cortex and its correlation with symptom severity in patients with generalized anxiety disorder: proton magnetic resonance spectroscopy at 3 Tesla. Psychiatry Clin. Neurosci. 69, 422–430. doi: 10.1111/pcn.12279
Nakamura, K., and Kubota, K. (1996). The primate temporal pole: its putative role in object recognition and memory. Behav. Brain Res. 77, 53–77. doi: 10.1016/0166-4328(95)00227-8
Ochsner, K. N., and Gross, J. J. (2005). The cognitive control of emotion. Trends Cogn. Sci. 9, 242–249. doi: 10.1016/j.tics.2005.03.010
Ohman, A. (2005). The role of the amygdala in human fear: automatic detection of threat. Psychoneuroendocrinology 30, 953–958. doi: 10.1016/j.psyneuen.2005.03.019
Oldfield, R. C. (1971). The assessment and analysis of handedness: the Edinburgh inventory. Neuropsychologia 9, 97–113. doi: 10.1016/0028-3932(71)90067-4
Olson, I. R., Plotzker, A., and Ezzyat, Y. (2007). The Enigmatic temporal pole: a review of findings on social and emotional processing. Brain 130(Pt 7), 1718–1731. doi: 10.1093/brain/awm052
O'Reilly, J. X., Beckmann, C. F., Tomassini, V., Ramnani, N., and Johansen-Berg, H. (2010). Distinct and overlapping functional zones in the cerebellum defined by resting state functional connectivity. Cereb. Cortex 20, 953–965. doi: 10.1093/cercor/bhp157
Pannekoek, J. N., Veer, I. M., van Tol, M.-J., van der Werff, S. J. A., Demenescu, L. R., Aleman, A., et al. (2013). Resting-state functional connectivity abnormalities in limbic and salience networks in social anxiety disorder without comorbidity. Eur. Neuropsychopharmacol. 23, 186–195. doi: 10.1016/j.euroneuro.2012.04.018
Patterson, K., Nestor, P. J., and Rogers, T. T. (2007). Where do you know what you know? The representation of semantic knowledge in the human brain. Nat. Rev. Neurosci. 8, 976–987. doi: 10.1038/nrn2277
Pehrs, C., Zaki, J., Schlochtermeier, L. H., Jacobs, A. M., Kuchinke, L., and Koelsch, S. (2015). The temporal pole top-down modulates the ventral visual stream during social cognition. Cereb. Cortex. doi: 10.1093/cercor/bhv226. [Epub ahead of print].
Phan, K. L., Fitzgerald, D. A., Nathan, P. J., Moore, G. J., Uhde, T. W., and Tancer, M. E. (2005). Neural substrates for voluntary suppression of negative affect: a functional magnetic resonance imaging study. Biol. Psychiatry 57, 210–219. doi: 10.1016/j.biopsych.2004.10.030
Phelps, E. A. (2006). Emotion and cognition: insights from studies of the human amygdala. Annu. Rev. Psychol. 57, 27–53. doi: 10.1146/annurev.psych.56.091103.070234
Phillips, M. L., Drevets, W. C., Rauch, S. L., and Lane, R. (2003). Neurobiology of emotion perception I: the neural basis of normal emotion perception. Biol. Psychiatry 54, 504–514. doi: 10.1016/S0006-3223(03)00168-9
Phillips, M. L., Ladouceur, C. D., and Drevets, W. C. (2008). A neural model of voluntary and automatic emotion regulation: implications for understanding the pathophysiology and neurodevelopment of bipolar disorder. Mol. Psychiatry 13, 833–857. doi: 10.1038/mp.2008.65
Power, J. D., Barnes, K. A., Snyder, A. Z., Schlaggar, B. L., and Petersen, S. E. (2012). Spurious but systematic correlations in functional connectivity MRI networks arise from subject motion. Neuroimage 59, 2142–2154. doi: 10.1016/j.neuroimage.2011.10.018
Roy-Byrne, P. P., and Wagner, A. (2004). Primary care perspectives on generalized anxiety disorder. J. Clin. Psychiatry 65(Suppl. 13), 20–26.
Sacchetti, B., Scelfo, B., and Strata, P. (2005). The cerebellum: synaptic changes and fear conditioning. Neuroscientist 11, 217–227. doi: 10.1177/1073858405276428
Shao, Y., Wang, L., Ye, E., Jin, X., Ni, W., Yang, Y., et al. (2013). Decreased thalamocortical functional connectivity after 36 hours of total sleep deprivation: evidence from resting state fMRI. PLoS ONE 8:e78830. doi: 10.1371/journal.pone.0078830
Shehzad, Z., Kelly, A. M. C., Reiss, P. T., Gee, D. G., Gotimer, K., Uddin, L. Q., et al. (2009). The resting brain: unconstrained yet reliable. Cereb. Cortex 19, 2209–2229. doi: 10.1093/cercor/bhn256
Shin, L. M., and Liberzon, I. (2010). The neurocircuitry of fear, stress, and anxiety disorders. Neuropsychopharmacology 35, 169–191. doi: 10.1038/npp.2009.83
Si, T.-M., Shu, L., Dang, W.-M., Su, Y.-A., Chen, J.-X., Dong, W.-T., et al. (2009). Evaluation of the reliability and validity of Chinese version of the Mini-International Neuropsychiatric Interview in patients with mental disorders. Chin. Ment. Health J. 23, 493–497. doi: 10.3969/j.issn.1000-6729.2009.07.011
Song, X.-W., Dong, Z.-Y., Long, X.-Y., Li, S.-F., Zuo, X.-N., Zhu, C.-Z., et al. (2011). REST: a toolkit for resting-state functional magnetic resonance imaging data processing. PLoS ONE 6:e25031. doi: 10.1371/journal.pone.0025031
Sridharan, D., Levitin, D. J., and Menon, V. (2008). A critical role for the right fronto-insular cortex in switching between central-executive and default-mode networks. Proc. Natl. Acad. Sci. U.S.A. 105, 12569–12574. doi: 10.1073/pnas.0800005105
Stefanacci, L., and Amaral, D. G. (2002). Some observations on cortical inputs to the macaque monkey amygdala: an anterograde tracing study. J. Comp. Neurol. 451, 301–323. doi: 10.1002/cne.10339
Stoodley, C. J. (2012). The cerebellum and cognition: evidence from functional imaging studies. Cerebellum 11, 352–365. doi: 10.1007/s12311-011-0260-7
Supple, W. F., Leaton, R. N., and Fanselow, M. S. (1987). Effects of cerebellar vermal lesions on species-specific fear responses, neophobia, and taste-aversion learning in rats. Physiol. Behav. 39, 579–586. doi: 10.1016/0031-9384(87)90156-9
Thomason, M. E., Dennis, E. L., Joshi, A. A., Joshi, S. H., Dinov, I. D., Chang, C., et al. (2011). Resting-state fMRI can reliably map neural networks in children. Neuroimage 55, 165–175. doi: 10.1016/j.neuroimage.2010.11.080
Wong, C., and Gallate, J. (2012). The function of the anterior temporal lobe: a review of the empirical evidence. Brain Res. 1449, 94–116. doi: 10.1016/j.brainres.2012.02.017
Yan, C.-G., Cheung, B., Kelly, C., Colcombe, S., Craddock, R. C., Di Martino, A., et al. (2013). A comprehensive assessment of regional variation in the impact of head micromovements on functional connectomics. Neuroimage 76, 183–201. doi: 10.1016/j.neuroimage.2013.03.004
Keywords: amygdala, DLPFC, temporal pole, DMN, functional connectivity, generalized anxiety disorder
Citation: Li W, Cui H, Zhu Z, Kong L, Guo Q, Zhu Y, Hu Q, Zhang L, Li H, Li Q, Jiang J, Meyers J, Li J, Wang J, Yang Z and Li C (2016) Aberrant Functional Connectivity between the Amygdala and the Temporal Pole in Drug-Free Generalized Anxiety Disorder. Front. Hum. Neurosci. 10:549. doi: 10.3389/fnhum.2016.00549
Received: 01 June 2016; Accepted: 14 October 2016;
Published: 04 November 2016.
Edited by:
Baojuan Li, Massachusetts General Hospital, USACopyright © 2016 Li, Cui, Zhu, Kong, Guo, Zhu, Hu, Zhang, Li, Li, Jiang, Meyers, Li, Wang, Yang and Li. This is an open-access article distributed under the terms of the Creative Commons Attribution License (CC BY). The use, distribution or reproduction in other forums is permitted, provided the original author(s) or licensor are credited and that the original publication in this journal is cited, in accordance with accepted academic practice. No use, distribution or reproduction is permitted which does not comply with these terms.
*Correspondence: Jijun Wang, amlqdW53YW5nMjdAMTYzLmNvbQ==
Zhi Yang, eWFuZ3pAcHN5Y2guYWMuY24=
Chunbo Li, Y2h1bmJvX2xpQDE2My5jb20=