Cortico-Striato-Thalamo-Cortical Circuitry, Working Memory, and Obsessive–Compulsive Disorder
- 1Department of Psychiatry and Behavioral Neurosciences, Brain Imaging Research Division, Wayne State University School of Medicine, Detroit, MI, USA
- 2Department of Psychiatry, Hospital for Sick Children, University of Toronto, Toronto, ON, Canada
- 3Department of Psychiatry, University of Michigan, Ann Arbor, MI, USA
Brain network dysfunction is emerging as a central biomarker of interest in psychiatry, in large part, because psychiatric conditions are increasingly seen as disconnection syndromes. Understanding dysfunctional brain network profiles in task-active states provides important information on network engagement in an experimental context. This in turn may be predictive of many of the cognitive and behavioral deficits associated with complex behavioral phenotypes. Here we investigated brain network profiles in youth with obsessive-compulsive disorder (OCD), contrasting them with a group of age-comparable controls. Network interactions were assessed during simple working memory: in particular, we focused on the modulation by the dorsal anterior cingulate cortex (dACC) of cortical, striatal, and thalamic regions. The focus on the dACC was motivated by its hypothesized role in the pathophysiology of OCD. However, its task-active network signatures have not been investigated before. Network interactions were modeled using psychophysiological interaction, a simple directional model of seed to target brain interactions. Our results indicate that OCD is characterized by significantly increased dACC modulation of cortical, striatal, and thalamic targets during working memory, and that this aberrant increase in OCD patients is maintained regardless of working memory demand. The results constitute compelling evidence of dysfunctional brain network interactions in OCD and suggest that these interactions may be related to a combination of network inefficiencies and dACC hyper-activity that has been associated with the phenotype.
Introduction
Obsessive-compulsive disorder (OCD) is a commonly occurring childhood and adolescent-onset neuropsychiatric disorder. It is characterized by obsessions (recurrent and persistent thoughts that typically induce marked distress) and compulsions (repetitive behaviors aimed at alleviating distress). OCD represents the upper extreme of an underlying continuous trait distribution encompassing obsessive-compulsive behaviors common in the general population that are heritable and cross traditional diagnostic boundaries. Thus, OCD represents a clinical “end-point” for a commonly observed trait (~45% of adolescents report OCD symptoms) (Berg et al., 1988; Apter et al., 1996). The 1-year incidence of OCD and sub-clinical OCD in adolescents is ~0.7 and 8.4%, respectively (Valleni-Basile et al., 1996). These relatively high rates of incidence and the association with a trait evident in the general population emphasize the importance of characterizing biological mechanisms underlying OCD. In this report, we aim to characterize these biological mechanisms by investigating brain network interactions in OCD and their differences from typical healthy controls.
Understanding brain network profiles and brain network dysfunction is a central theme of interest in clinical neuroscience. As suggested by the National Institute of Mental Health (Insel et al., 2010), such a focus may lead to an enhanced understanding of specific bio-behavioral impairments that underpin the emergence of complex behavioral phenotypes which are classified as psychiatric disorders. Indeed, understanding network dysfunction, in particular, is emerging as a leading framework for characterizing the neural substrates of multiple psychiatric conditions (Friston, 1998; Stephan et al., 2006; Almeida et al., 2009; Shaw et al., 2009; Diwadkar, 2012; Schmidt et al., 2013).
Obsessive-compulsive disorder, like most neuropsychiatric conditions, often has its origins in childhood and adolescence when brain network function is still maturing (Paus et al., 2008). Ensuing disordered neurodevelopmental trajectories (in the absence of adaptive responses) may in turn mediate the continued expression of symptoms through adolescence and into early adulthood (Tottenham and Sheridan, 2009). Furthermore, the complex patterns of OCD symptoms are linked to the inability to disengage behaviors from intrusive thoughts, implying aberrantly increased inhibitory control (Bari and Robbins, 2013). These patterns are highly suggestive of dysfunctions in control mechanisms within relevant brain networks (Piras et al., 2013). In this context, the role of the dorsal anterior cingulate cortex (dACC) assumes significance.
The dACC is positioned as a principal control region in the brain (Paus, 2001) that by itself, or through its mediation of cortical-striatal networks, exercises aspects of cognitive and motor control (Bakshi et al., 2011). The region has been of particular interest in OCD: glutamate dysregulation in the anterior cingulate and striatum has been implicated in pediatric OCD patients (Rosenberg et al., 2000, 2004). Altered glutamate concentrations may be linked to dysfunctional fMRI responses during tasks of behavioral engagement and disengagement. For instance, during conflict processing and action monitoring, OCD subjects evince higher activation in regions including the anterior cingulate cortex and the striatum (Fitzgerald et al., 2005; Maltby et al., 2005; Marsh et al., 2014) that may provide functional expressions of dACC dysfunction in the illness. A question of interest is whether these hyper-activations in the dACC are associated with dysfunctional network profiles.
Network models of fMRI have been applied in OCD. However, a principle focus of network-analyses of in vivo imaging data has been on the classification of resting state functional connectivity within (and across) cortical, limbic, striatal, and cerebellar networks (Harrison et al., 2009; Peng et al., 2014). These analyses have been notable as they have revealed categorical and developmental distinctions in resting state functional connectivity (rsFC) between OCD and typical controls in frontal, striatal and thalamic (FSTC) circuits (Fitzgerald et al., 2011). rsFC results are not directly informative about dysfunctional dACC-related profiles in a task-active state. For instance, the relationship between resting state functional connectivity (rsFC) and task-dependent functional interactions between regions remains uncertain (Stephan, 2004) and experimental analyses of within subject data have been equivocal (Rehme et al., 2013). Thus rsFC and the low-frequency bold signals it correlates between provide a complimentary snapshot of pathology; task-active analyses of functional network interactions are important for assessing a measure of network dynamics. Moreover, a separate question of interest is whether dysfunctional activation and brain network profiles in OCD are observed in tasks not involving conflict monitoring. Such evidence will provide strong support for general network based dysfunction in the disorder extending beyond highly circumscribed behavioral domains.
We had two principal aims in this study (summarized in Figure 1): (a) to investigate network profiles originating in the dACC in the task-active state using psychophysiological interaction (PPI) (Friston et al., 1997; O’Reilly et al., 2012), PPI is a simple framework within the general linear model for investigating contextual modulation of targets (e.g., regions within FSTC) by a seed (e.g., dACC) in a task-active context; (b) to investigate these profiles during parametrically manipulated verbal working memory, (Casey et al., 1995; Diwadkar et al., 2011, 2013), a domain that provides a rich window for investigating normal and dysfunctional activation and network profiles in the FSTC.
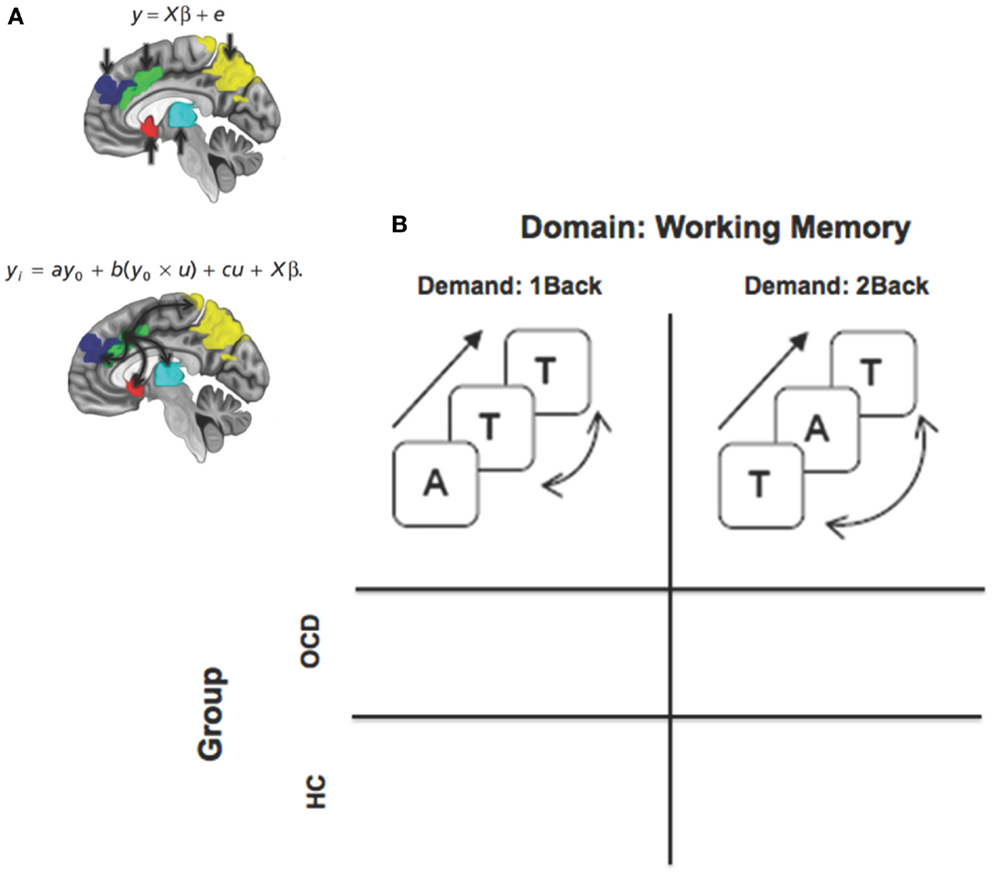
Figure 1. A framework for assessing dysfunctional activation and dACC-related network profiles of cortical, striatal, and thalamic networks in OCD. (A) The two panels depictive activation-based and seed-based approaches to identifying function and dysfunction. The equations represent basic linear model formalisms for each class of models. Note the convolution term (y0 × u) in the PPI based model that accounts for seed (y0 = dACC) modulation of targets in the task-oriented (u = working memory > rest) context. The regions of interest are schematically depicted on the mid-sagittal surface. The second figure schematically depicts the modulatory effects of the dACC assessed using psychophysiological interaction. (B) The factorial design space used for the study that assessed the effects of task-demand (1Back vs. 2Back) crossed with group.
Materials and Methods
Participants
Eighteen participants with a diagnosis of OCD and 27 controls participated in the fMRI studies (see Table 1). All participants and their parents were interviewed with the Schedule for Schizophrenia and Affective Disorders for School-Aged Children-Present and Lifetime Version and Schedule for Obsessive-Compulsive and Other Behavioral Syndromes (Wolff and Wolff, 1991; Kaufman et al., 1997). The lifetime (maximum) and current severity of OCD were assessed in the patients with a modified version of the Children’s Yale-Brown Obsessive Compulsive Disorder Scale (Goodman et al., 1989; Scahill et al., 1997). Lifetime and current axis I diagnoses were made independently by two clinicians (David R. Rosenberg, Gregory L. Hanna) using all sources of information according to DSM-IV criteria. All patients with OCD had a total lifetime CY-BOCS score of at least 20. Exclusion criteria for patients and controls included lifetime history of psychosis, bipolar disorder, substance abuse or dependence, anorexia or bulimia nervosa, epilepsy, head injury with sustained loss of consciousness, Huntington’s disease, dyskinesia, chronically disabling medical illness, autism, mental retardation, or a score >15 on the lifetime version of the Social Communication Questionnaire. Controls were free of all psychiatric illness. Legal guardians provided written informed consent prior and children gave written assent prior to participating in the study. The Human Subjects Investigative committee at Wayne State University and the University of Michigan approved the protocol and all methods therein.
fMRI
Gradient echo EPI fMRI data acquisition was conducted at Vaitkevicius Magnetic Resonance Centre on a 3T Siemens Verio system using a 12-channel volume head coil (TR: 2.6 s, TE: 29 ms, FOV: 256 mm × 256 mm, acquisition matrix: 128 × 128, 36 axial slices, voxel dimensions: 2 mm × 2 mm × 3 mm). In addition, a 3D T1-weighted anatomical MRI image was acquired (TR: 2200 ms, TI: 778 ms, TE: 3 ms, flip-angle = 13°, FOV: 256 mm × 256 mm, 256 axial slices of thickness = 1.0 mm, matrix = 256 × 256). A neuroradiologist reviewed all scans to rule out clinically significant abnormalities.
During fMRI, subjects were positioned with adjustable padded restraints employed for head stabilization. Stimuli were rear-projected using an IFIS-SA presentation system (MRI Devices), and subjects responded with a button box unit. During fMRI, subjects participated in an established verbal n-back paradigm (Casey et al., 1995). Parametric working memory load was varied between maintaining 0, 1, or 2 items in memory (0-, 1-, or 2-Back; see Figure 1 insets). Runs were blocked by condition. During each block (30 s), letters were projected in sequence (presentation time: 500 ms; ISI: 2500 ms; 10 letters per block) on a screen; subjects signaled with a two-choice optical response box if the presented letter was a target or not. The paradigm cycled between rest (20 s), 0-, 1-, and 2-Back epochs (three blocks each). The experiment was controlled using presentation (Neurobehavioral Systems Inc.).
fMRI Processing
fMRI data were processed in SPM8 using typical methods. All images were manually oriented to the AC-PC line, realigned to correct for head movement, spatially normalized to the MNI (Montreal Neurological Institute) template brain and resliced (2 mm × 2 mm × 2 mm). Low frequency components were removed using a low-pass filter (128 s) and images were spatially smoothed using a Gaussian filter (8 mm full-width half maximum; FWHM). An autoregressive AR(1) model was used to account for serial correlation, and regressors modeled as a 30 s boxcar vectors (for each of the task-related conditions) were convolved with a canonical hemodynamic reference waveform.
Subjects’ head motion was within accepted limits (<4 mm). Furthermore, in all first level models, the effects of motion were modeled by including the six motion parameters as covariates of no interest. First-level contrasts (1Back > 0Back; 2Back > 0Back) were used to assess the effects of memory load on activation.
PPI (implemented in SPM8) was employed to model dACC modulation of FSTC targets during working memory (Friston et al., 1997; Honey et al., 2005). For each subject, time series from the effects of interest contrast (p < 0.05) were extracted from the dACC peak (including Brodmann areas 32 and the supra-genual aspects of BA24) (Palomero-Gallagher et al., 2008). The extracted time series (the wave form of which provides an estimate of the continuous physiological response of the dACC) was subsequently convolved with the two contrasts of interest reflecting effects of differential memorial load, specifically, 1-Back > 0-Back (low load) and 2-Back > 0-Back (high load). The resultant interaction term was positively weighted to assess the facilitating influence of the dACC on FSTC targets (with the slope of the effect parametrically encoded in the convolution term and reflecting the degree of modulation).
For all activation or network analyses, first level maps (activation or PPI) from each subject were submitted to a second-level random effects analyses of variance with group modeled as an independent factor and memory load as non-independent factor. The factorial design permitted assessment of intra-group load-related effects, as well as between-group differences at varying levels of memory load.
All second level analyses were spatially thresholded in the FSTC regions of interest using deterministic anatomical masks defined in stereotactic space (Maldjian et al., 2003). These maps constitute anatomical representations in stereotactic space representing each of the regions of interest and are widely employed to spatially localize activations in neuroimaging research. Images were corrected using cluster level correction (cluster extent thresholds, pc < 0.05) derived from 104 Monte Carlo simulations from voxels across the individual regions of interest (Ward, 2000). Individual voxel peaks in significant clusters are reported in terms of Montreal Neurological Institute coordinates.
Results
Results are organized to sequentially present evidence of (1) dysfunctional activation profiles and (2) dysfunctional brain network profiles in OCD and HC:
(1a) We first show load-related effects on activation profiles within both HC and OCD. These results provide evidence of within-group effects of parametric increases in working memory load on FSTC.
(1b) Next we present between-group results showing aberrantly increased activation profiles in FSTC in OCD patients compared to HC at both levels of memory load. These results demonstrate that OCD participants more extensively activate FSTC than HC at both levels of memory load.
(2) We show between group results assessing dysfunctional brain network profiles in OCD compared to HC. These results indicate aberrantly increased modulation of FSTC by the dACC in OCD, especially at the lower level of memory load.
Load-Related Effects on Activation Profiles
Figure 2 depicts clusters (pc < 0.05) in FSTC showing increased within-group activation in response to increases in memory load (cluster relevant information in Table 2). In both groups, increased memory load results in increased recruitment of frontal and parietal regions, and the dACC. These results are unsurprising for the HC group. They are highly consistent with previous assessments of activation profiles in this circuit in HC (Braver et al., 1997; Cohen et al., 1997; Diwadkar et al., 2000), showing increased recruitment in brain circuits committed to implementing working memory related functions. The results in OCD are notable as they demonstrate that the memory effect exerts within-group effects consistent with HC. This is important evidence that FSTC in OCD is sensitive to load-related variations in working memory and that the overall implementation of the task generates load-related effects on activation profiles. Notable is an absence of load-related activation effects in the striatum or the thalamus, regions not typically implicated in core memory-related processing. The basal ganglia contribute to cortical-striatal processing loops that sub-serve complex processing, by supplementing prefrontal function (Hazy et al., 2006; Calzavara et al., 2007; Voytek and Knight, 2010). The thalamus forms cortical-thalamic processing units that integrate information from cortical and striatal loops to modulate complex behavior, but has generally not been sensitive to load-related variations in working memory (Haber and Calzavara, 2009).
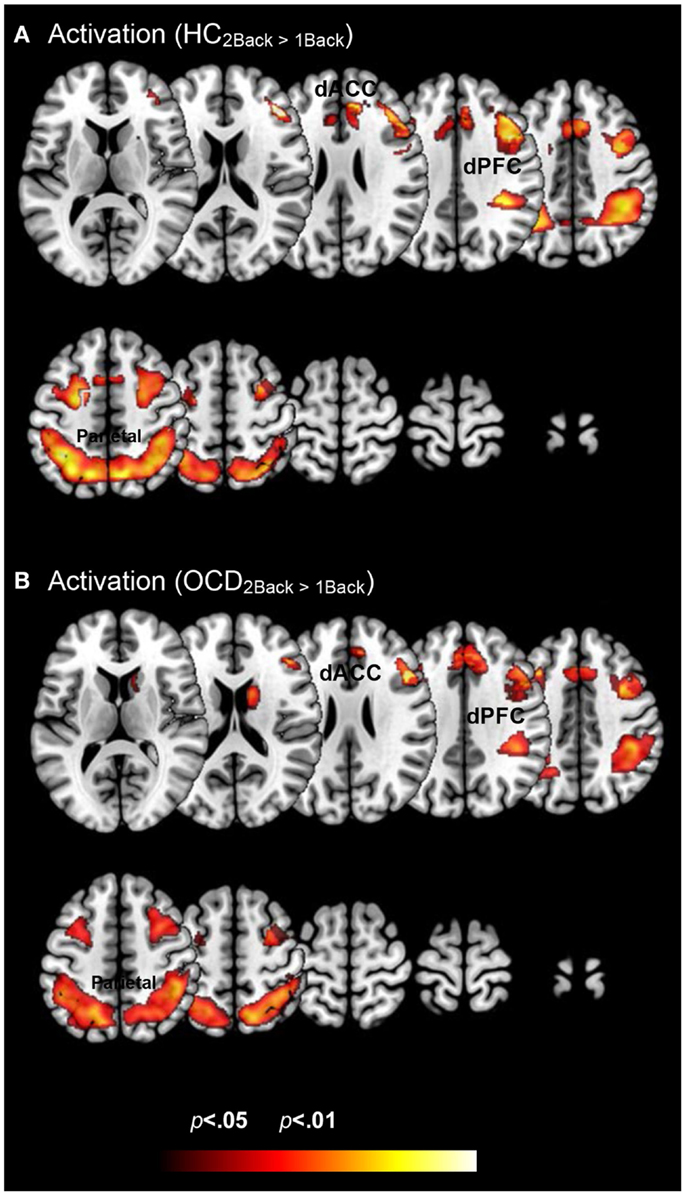
Figure 2. Within group changes in activation profiles as a function of load are depicted on identical ascending mosaics of axial views. The significant clusters (p < 0.05, cluster level) show significant increases in activation with increases in working memory related load. As seen, these increases are evident within both (A) healthy control and (B) OCD groups. These activation profiles establish within group effects of memory load across previously implicated load sensitive working memory related regions. These include dorsolateral prefrontal cortex (dPFC), the dorsal anterior cingulate (dACC), and the parietal cortex.
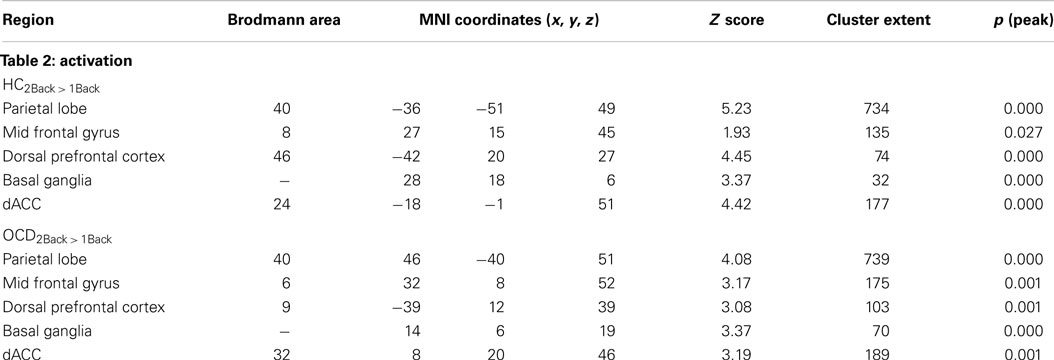
Table 2. The table provides information on clusters of significance and peaks within where each of the groups showed increased activation to variations in memory load (Figure 2).
Between-Group Results Showing Aberrantly Increased Activation Profiles in FSTC in OCD Patients
Figure 3 depicts clusters (pc < 0.05) in FSTC showing increased activation in OCD (relative to HC) at each level of memory load (cluster relevant information in Table 3). Several effects are evident. Dysfunctional activation profiles are observed in the frontal and parietal cortices and in the dACC at both levels of load. Absent is evidence of dysfunctional activation profiles in the striatum or the thalamus. Moreover, dysfunction in activation profiles scales as a function of memory load: Increased memory demand leads to increased activation in cortical regions. These analyses are consistent with previous studies in FSTC in OCD participants in other behavioral domains such as conflict monitoring that are closely associated with behavioral phenotypes in the illness (Huyser et al., 2011). As one of our study aims was to assess whether hyper-activation in FSTC constitutes a domain-general property of brain regions in OCD, these analyses extend the findings beyond the domain of conflict processing and suggest that multiple tasks engaging FSTC are sensitive for detecting activation-related dysfunction.
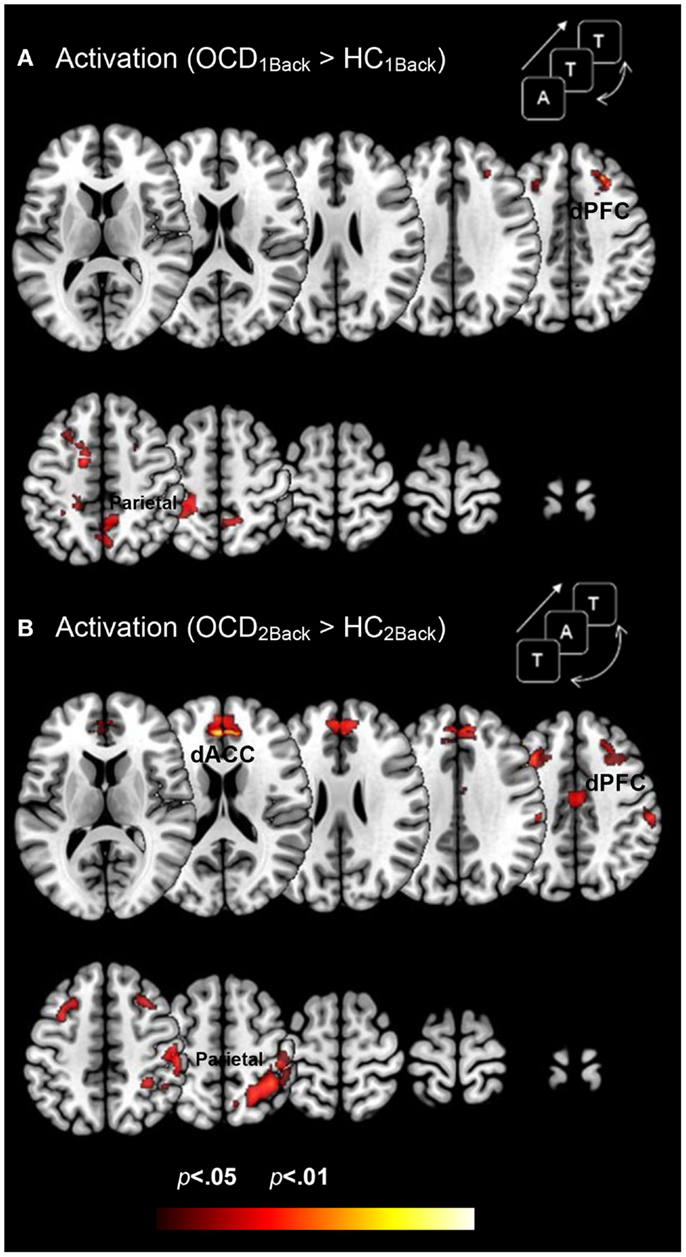
Figure 3. Dysfunctional activation profiles in OCD (relative to controls) are depicted for both (A) the 1Back level of memory and (B) the 2Back level of memory load. Increased activation in OCD (p < 0.05, cluster level) is depicted on identical ascending mosaics of axial views. These activation profiles indicate increased activation in dorsolateral prefrontal cortex (dPFC), the dorsal anterior cingulate (dACC), and the parietal cortex in OCD. Notably the degree of dysfunctional activation in OCD scales as a function of memory load. We speculate that the parametric demands as expressed in dysfunctional activation profiles load disproportionately in OCD participants. As will be seen, brain network profiles in OCD do not strictly follow activation patterns, evidence that signatures of network interactions may complement psychopathology revealed in activation models.
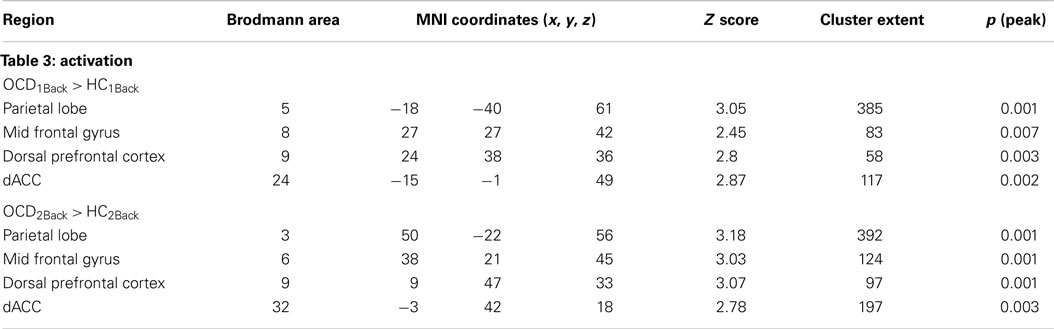
Table 3. The table provides information regarding clusters of significant and significant peaks showing dysfunctional activation profiles in OCD (compared to HC) at each level of memory load (Figure 3).
Between Group Results Assessing Dysfunctional Brain Network Profiles in OCD
Figure 4 depicts clusters (pc < 0.05) in FSTC showing increased modulation by the dACC in OCD (relative to HC) at each level of memory load (cluster relevant information in Table 4). We highlight several notable effects. First, dysfunctional network profiles in OCD form a pattern that is distinct and complimentary to that observed in activation profiles. OCD is characterized by increased dACC related modulation at the 1Back level of load but not the 2Back level, suggesting that the degree of dACC modulation (and the mechanisms that can be inferred from it) do not scale with load. We speculate (see Discussion) that this effect may be related to aberrantly increased dACC modulation at the 1Back level itself. The hyper-modulation may reflect inefficiencies in control-related network function or hyper-activity of the dACC, or both. Second, dysfunctional modulation of the striatum is evident, with significantly increased dACC modulation of the caudate and putamen observed at the 1Back level (Figure 4A). This effect also constitutes a complementary pattern of dysfunction from activation in OCD where profiles in the striatum appeared normal (Figure 3).
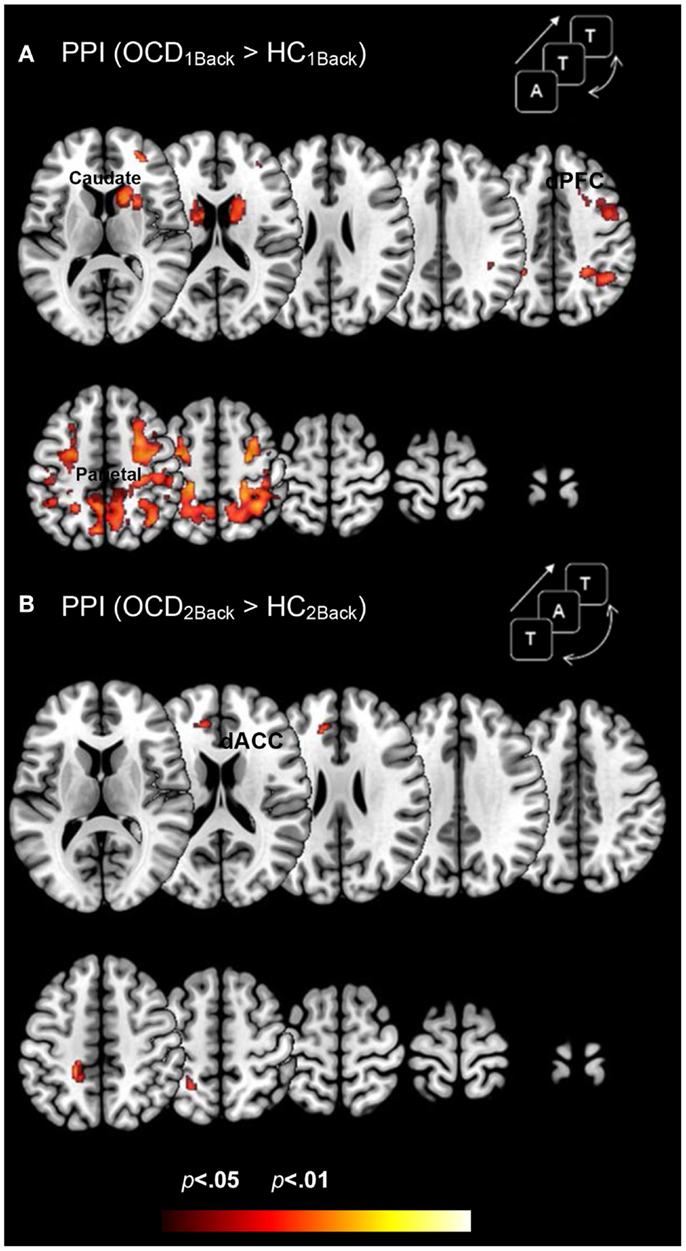
Figure 4. Dysfunctional brain network profiles in OCD (relative to controls) are depicted for both (A) the 1Back level of memory and (B) the 2Back level of memory load. The clusters depict significantly increased dACC-modulation of cortical and striatal targets in OCD compared to typical controls (p < 0.05, cluster level) depicted on identical ascending mosaics of axial views. These brain network profiles complement dysfunctional activation profiles (Figure 3). Note the implication of the caudate, not implicated in dysfunctional activation. The increased modulation by the dACC may reflect increased control-related inputs demanded in OCD to sub-serve network function associated with this fundamental domain. The lack of a parametric effect may reflect the fact that dACC related network engagement is already aberrantly increased at the 1Back level. Indeed, OCD participants did not show an increase in dACC modulation going from the 1Back to the 2Back level of demand (whereas HC participants did).
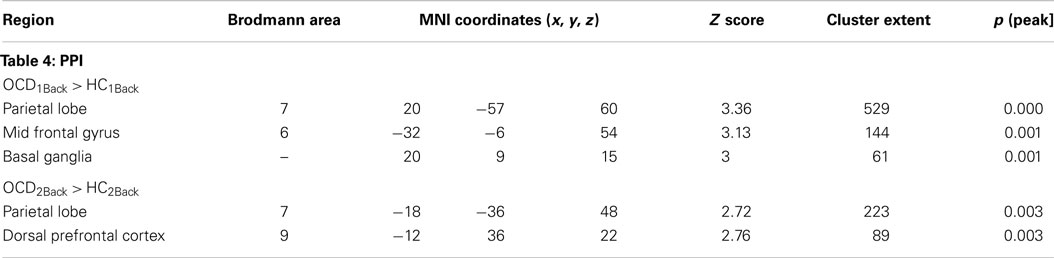
Table 4. The table provides information regarding clusters of significant and significant peaks showing dysfunctional network profiles in OCD (compared to HC) at each level of memory load (Figure 4).
Discussion
We conducted a simple investigation of brain activation and network profiles in a group of OCD youth and age-comparable controls. Participants were assessed with fMRI using a simple working memory paradigm with variable demands (Figure 1). Three principle results are highlighted across both classes of analyses: Activation Profiles: (1a) Activation profiles were highly sensitive to increases in memory load within each group (Figure 2). (1b) Youth with OCD were characterized by aberrantly increased recruitment of frontal and parietal regions (but not striatal or thalamic regions) during both levels of working memory. The degree of hyper-activation scaled as a function of working memory demand (Figure 3). Network Profiles: (2) Compared to HC, youth with OCD were characterized by increased dACC modulation of frontal, parietal, and striatal regions, particular at lower levels of working memory load (Figure 4).
Taken together, these results establish that OCD is characterized by dysfunction in core FSTC regions, detectable using both activation- and network-based analyses of fMRI signals. We suggest that the network-based analyses are notable for being the first to demonstrate dysfunctional network signatures of the dACC, a region closely associated with OCD related pathophysiology. Moreover, these profiles observed using a basic working memory paradigm, suggest that FSTC deficits are a basic pathophysiologic mechanism underlying OCD, are detectable with a multiplicity of tasks, and affect frontal, striatal, and thalamic circuits. In the remainder of the paper, we discuss the putative mechanisms that may underpin these observations and the implications for OCD related pathology and function.
Cingulate, Frontal, Striatal, and Thalamic Regions: A Critical Circuit Sub-Serving Complex Function
The regions targeted in this investigation collectively form core sub-circuits that implement function in a multiplicity of higher-order domains including working memory (Owen et al., 2005; Diwadkar et al., 2011), sustained attention (Fan et al., 2005; Langner and Eickhoff, 2013; Diwadkar et al., 2014), and cognitive control (Carter et al., 1999; Anderson et al., 2008). These functional sub-circuits are also underpinned by dense patterns of anatomical connectivity. The dorsal-prefrontal cortex and the basal ganglia share topographically mapped monosynaptic connections (Calzavara et al., 2007) that may explain co-activation patterns frequently observed in fMRI studies. Descending connections from cortical regions including the prefrontal cortex and sensory, motor, and frontal regions synapse on multiple thalamic nuclei including the ventral and posteromedial complexes (Ray and Price, 1993; Klein et al., 2010; Li et al., 2013) leading to the notion of “cortical-thalamic processing units” (Briggs and Usrey, 2008). The dACC is uniquely positioned from an anatomical standpoint, with connections to frontal and motor regions, to play a mediating influence in control related mechanisms (Paus, 2001). Each of these regions appears to be relatively specialized for highly sophisticated functions.
The dorsal-prefrontal cortex sub-serves working memory in multiple ways. Phasic activity in prefrontal neurons is strongly correlated with the temporary maintenance of memoranda in working memory (Vijayraghavan et al., 2007), suggestive of a direct link between neuronal responses and overt behavior. Moreover, the prefrontal cortex sub-serves goal-directed behavior in multiple domains (including working memory) through direct “command” signals to multiple cortical and sub-cortical targets (Crowe et al., 2013; Funahashi and Andreau, 2013). The anatomical positions of the basal ganglia allow the structure to receive inputs from multiple uni- and heteromodal regions (Ragsdale and Graybiel, 1990). Thus the structure serves as a critical node in multiple network pathways, playing executive and supporting roles in several behavioral domains. Along with the prefrontal cortex, the basal ganglia appear to exert attention-related modulation of working memory related function (Herrero et al., 2002; McNab and Klingberg, 2008). The thalamus is considered a principle gateway to the cortex (McAlonan et al., 2008), engaged in filtering of massive sensory inputs, particularly in the visual domain, and sending extensive outputs to cortical and sub-cortical regions (Haber and Calzavara, 2009). The structure also plays essential computational roles by integrating network activity essential for modulating behaviors. Many of the psychological domains that are underpinned by regional function are implicated in OCD. Thus pediatric OCD patients in particular show deficits in sustained attention (Lucke et al., 2014), executive function and working memory (Melloni et al., 2012), and cognitive control and metacognition (Koch and Exner, 2015). It is therefore not surprising that frontal, striatal, and thalamic circuits have been identified as central to potential interventions in OCD (Burguiere et al., 2015).
Hyper-activation in OCD during Working Memory: Possible Mechanisms and Relationship with Other Disorders
Though working memory deficits are generally seen as secondary to the core pathology of OCD (Harkin and Kessler, 2011), our activation results provide good convergence with recent reports. Memory load-related hyper-activation in frontal-parietal regions has been proposed as an intermediate phenotype for OCD, where the hyper-activation has been labeled as compensatory (Nakao et al., 2009; Koch et al., 2012; de Vries et al., 2013). Effects on dACC activation have, however, been equivocal; previous studies have shown a reverse effect of complexity on dACC activation in OCD, with disengagement of the structure following load related effects. Nevertheless, our results provide good conceptual overlap with studies in pathology that have linked hyper-activation under conditions of task compliance with regional efficiency. This concept of inefficiency finds pronounced expression in the schizophrenia spectrum, where disease-related effects (that have been associated with dopamine dysfunction) are presumed to affect the “duty cycle” of task-relevant brain regions including the prefrontal cortex and the striatum (Callicott et al., 2003; Manoach, 2003; Jansma et al., 2004; Meisenzahl et al., 2007; Diwadkar et al., 2012). These inefficiencies might imply that neuronal pools (that form one electrophysiological origin of the fMRI signal) (Logothetis and Wandell, 2004) engage in excess excitatory firing responses when demand is exerted on FSTC. Moreover, inefficiencies provide a window into the “scalability” of brain regions in response to demand. In other words, the functioning limits of FSTC in OCD may be compromised such that excessive cognitive demand may stretch FSTC ability to sub-serve function. In this view, FSTC hyper-activation during working memory far, from being a peripheral correlate of OCD, is a central mechanism underlying the illness, and a primary intermediate phenotype as previously proposed (de Vries et al., 2013).
A parallel explanation for hyper-activity is that it reflects glutamate-related dysfunction that affects how relevant regions are recruited for a task (Wu et al., 2012, 2013; Stewart et al., 2013; Pauls et al., 2014). As a principle excitatory neurotransmitter, glutamate exerts substantial effects on brain function, particularly in the excitatory model. Glutamate dysfunction in OCD can alter the neurochemical-electrophysiological relationship that sub-serves BOLD-based activation. A more complete assessment of the Glutamate-fMRI relationship will require assessment of both classes of signals acquired within subjects. This is an ongoing endeavor in our studies that involves multi-modal acquisition of fMRI and MRS data within subjects.
Hyper-Modulation of FSTC by the dACC: Novel Evidence of Dysfunctional Network Profiles
Relatively few studies have assessed connectivity in the task-active state in OCD. Psychophysiological interactions provide a straightforward model of directional effects of seed regions on their targets in a task-related context, providing a window into network interactions. This window is considered intermediate between functional and effective connectivity (Friston, 2011). The interpretations of PPI are constrained by the choice of seeds and the hypothesized role(s) ascribed to the seed. Toward that end, our choice of the dACC was motivated by its role in cognitive control of brain networks (Carter et al., 1999; Paus, 2001; Bakshi et al., 2011).
The dACC plays an integral role in tasks of explicit cognitive control including response conflict and response monitoring (Braver et al., 2001; van Veen et al., 2001), and choice selection (Eshel et al., 2007). The dACC may serve to amplify task-relevant signals to heteromodal association regions of the cortex (Egner and Hirsch, 2005; Sohn et al., 2007). Thus, control processes from the dACC may influence the activity of core working memory systems, and the degree of this modulation may reflect the efficiency of interaction between control and working memory systems. Increased control-related modulation in part reflects decreased efficiency. This hyper-modulation by the dACC may strongly suggest inefficient control-related network profiles in OCD. These effects are again consistent with observed evidence in other disorders, for example, in the schizophrenia spectrum where increased dACC related modulation is strongly associated with the illness and risk for the illness (Bakshi et al., 2011). The absence of a parametric effect on dACC modulation appears related to highly increased aberrant dACC modulation at the lower level of demand in OCD: no intra-group increases in dACC modulation were observed in OCD as memory load increases. As such, the network effects constitute complementary signatures of FSTC dysfunction in OCD.
Limitations and Conclusion
Brain network profiles will constitute an important frontier in the search for mechanisms and endophenotypes, and their evidence is an important expression of the goals advocated by Research Domain Criteria (RDoC: Insel et al., 2010). While our sample size (though small) is comparable to several other published studies, it nevertheless precludes us from assessing the role of co-morbid diagnoses and medication effects within OCD youth. These are important clinical questions, and an expansion of this sample is ongoing, and may permit more detailed assessment of our observed effects.
The specific neurochemical and molecular bases of these effects are obscured by the interpretational limits of both the fMRI signal (Logothetis, 2008) that cannot distinguish between a multiplicity of neuronal contributions to the hemodynamic response, and by the relatively limited class of inferences that can be drawn from the application of PPI analyses (Stephan, 2004). Moreover, these technical challenges are compounded by the fundamental limitation in understanding the correlates of brain structure and function: the fact that functional characteristics of brain networks exist in a regressive relationship with their structural substrates (Park and Friston, 2013). Thus, the same underlying structural networks can give rise to a multiplicity of functions and dysfunctions. Nevertheless, our results (and other studies we have cited) promise to reveal mechanisms of disease-related dysfunction as expressed in brain profiles. An understanding of putative mechanisms is a necessary precursor of treatment and cure. Therefore we propose that studies such as ours (and future extensions) will provide better elucidation of disease mechanisms than currently exist.
Conflict of Interest Statement
The authors declare that the research was conducted in the absence of any commercial or financial relationships that could be construed as a potential conflict of interest.
Acknowledgments
This work was supported by the National Institute of Mental Health (MH059299), the Children’s Hospital of Michigan Foundation, the Prechter World Bipolar Foundation, the Lyckaki-Young Fund from the State of Michigan, the Miriam Hamburger Endowed Chair of Child Psychiatry, the Paul and Anita Strauss Endowment, the Donald and Mary Kosch Foundation, Detroit Wayne County Authority, and Gateway Community Health. The funding agencies played no role in the analyses or reporting of the data.
Abbreviations
dACC, dorsal anterior cingulate cortex; FSTC, frontal striatal thalamic circuits; OCD, obsessive-compulsive disorder; PPI, psychophysiological interaction.
References
Almeida, J. R., Versace, A., Mechelli, A., Hassel, S., Quevedo, K., Kupfer, D. J., et al. (2009). Abnormal amygdala-prefrontal effective connectivity to happy faces differentiates bipolar from major depression. Biol. Psychiatry 66, 451–459. doi: 10.1016/j.biopsych.2009.03.024
Pubmed Abstract | Pubmed Full Text | CrossRef Full Text | Google Scholar
Anderson, J. R., Fincham, J. M., Qin, Y., and Stocco, A. (2008). A central circuit of the mind. Trends Cogn. Sci. 12, 136–143. doi:10.1016/j.tics.2008.01.006
Apter, A., Fallon, T. J. Jr., King, R. A., Ratzoni, G., Zohar, A. H., Binder, M., et al. (1996). Obsessive-compulsive characteristics: from symptoms to syndrome. J. Am. Acad. Child Adolesc. Psychiatry 35, 907–912. doi:10.1097/00004583-199607000-00016
Pubmed Abstract | Pubmed Full Text | CrossRef Full Text | Google Scholar
Bakshi, N., Pruitt, P., Radwan, J., Keshavan, M. S., Rajan, U., Zajac-Benitez, C., et al. (2011). Inefficiently increased anterior cingulate modulation of cortical systems during working memory in young offspring of schizophrenia patients. J. Psychiatr. Res. 45, 1067–1076. doi:10.1016/j.jpsychires.2011.01.002
Pubmed Abstract | Pubmed Full Text | CrossRef Full Text | Google Scholar
Bari, A., and Robbins, T. W. (2013). Inhibition and impulsivity: behavioral and neural basis of response control. Prog. Neurobiol. 108, 44–79. doi:10.1016/j.pneurobio.2013.06.005
Pubmed Abstract | Pubmed Full Text | CrossRef Full Text | Google Scholar
Berg, C. Z., Whitaker, A., Davies, M., Flament, M. F., and Rapoport, J. L. (1988). The survey form of the Leyton obsessional inventory-child version: norms from an epidemiological study. J. Am. Acad. Child Adolesc. Psychiatry 27, 759–763. doi:10.1097/00004583-198811000-00017
Braver, T. S., Barch, D. M., Gray, J. R., Molfese, D. L., and Snyder, A. (2001). Anterior cingulate cortex and response conflict: effects of frequency, inhibition and errors. Cereb. Cortex 11, 825–836. doi:10.1093/cercor/11.9.825
Pubmed Abstract | Pubmed Full Text | CrossRef Full Text | Google Scholar
Braver, T. S., Cohen, J. D., Nystrom, L. E., Jonides, J., Smith, E. E., and Noll, D. C. (1997). A parametric study of prefrontal cortex involvement in human working memory. Neuroimage 5, 49–62. doi:10.1006/nimg.1996.0247
Pubmed Abstract | Pubmed Full Text | CrossRef Full Text | Google Scholar
Briggs, F., and Usrey, W. M. (2008). Emerging views of corticothalamic function. Curr. Opin. Neurobiol. 18, 403–407. doi:10.1016/j.conb.2008.09.002
Pubmed Abstract | Pubmed Full Text | CrossRef Full Text | Google Scholar
Burguiere, E., Monteiro, P., Mallet, L., Feng, G., and Graybiel, A. M. (2015). Striatal circuits, habits, and implications for obsessive-compulsive disorder. Curr. Opin. Neurobiol. 30C, 59–65. doi:10.1016/j.conb.2014.08.008
Pubmed Abstract | Pubmed Full Text | CrossRef Full Text | Google Scholar
Callicott, J. H., Egan, M. F., Mattay, V. S., Bertolino, A., Bone, A. D., Verchinksi, B., et al. (2003). Abnormal fMRI response of the dorsolateral prefrontal cortex in cognitively intact siblings of patients with schizophrenia. Am. J. Psychiatry 160, 709–719. doi:10.1176/appi.ajp.160.4.709
Pubmed Abstract | Pubmed Full Text | CrossRef Full Text | Google Scholar
Calzavara, R., Mailly, P., and Haber, S. N. (2007). Relationship between the corticostriatal terminals from areas 9 and 46, and those from area 8A, dorsal and rostral premotor cortex and area 24c: an anatomical substrate for cognition to action. Eur. J. Neurosci. 26, 2005–2024. doi:10.1111/j.1460-9568.2007.05825.x
Pubmed Abstract | Pubmed Full Text | CrossRef Full Text | Google Scholar
Carter, C. S., Botvinick, M. M., and Cohen, J. D. (1999). The contribution of the anterior cingulate cortex to executive processes in cognition. Rev. Neurosci. 10, 49–57. doi:10.1515/REVNEURO.1999.10.1.49
Pubmed Abstract | Pubmed Full Text | CrossRef Full Text | Google Scholar
Casey, B. J., Cohen, J. D., Jezzard, P., Turner, R., Noll, D. C., Trainor, R. J., et al. (1995). Activation of prefrontal cortex in children during a nonspatial working memory task with functional MRI. Neuroimage 2, 221–229. doi:10.1006/nimg.1995.1029
Pubmed Abstract | Pubmed Full Text | CrossRef Full Text | Google Scholar
Cohen, J. D., Perlstein, W. M., Braver, T. S., Nystrom, L. E., Noll, D. C., Jonides, J., et al. (1997). Temporal dynamics of brain activation during a working memory task. Nature 386, 604–608. doi:10.1038/386604a0
Pubmed Abstract | Pubmed Full Text | CrossRef Full Text | Google Scholar
Crowe, D. A., Goodwin, S. J., Blackman, R. K., Sakellaridi, S., Sponheim, S. R., Macdonald, A. W. III, et al. (2013). Prefrontal neurons transmit signals to parietal neurons that reflect executive control of cognition. Nat. Neurosci. 16, 1484–1491. doi:10.1038/nn.3509
Pubmed Abstract | Pubmed Full Text | CrossRef Full Text | Google Scholar
de Vries, F. E., De Wit, S. J., Cath, D. C., Van Der Werf, Y. D., Van Der Borden, V., Van Rossum, T. B., et al. (2013). Compensatory frontoparietal activity during working memory: an endophenotype of obsessive-compulsive disorder. Biol. Psychiatry 76, 878–887. doi:10.1016/j.biopsych.2013.11.021
Pubmed Abstract | Pubmed Full Text | CrossRef Full Text | Google Scholar
Diwadkar, V. A. (2012). Adolescent risk pathways toward schizophrenia: sustained attention and the brain. Curr. Top. Med. Chem. 12, 2339–2347. doi:10.2174/156802612805289962
Pubmed Abstract | Pubmed Full Text | CrossRef Full Text | Google Scholar
Diwadkar, V. A., Bakshi, N., Gupta, G., Pruitt, P., White, R., and Eickhoff, S. B. (2014). Dysfunction and dysconnection in cortical-striatal networks during sustained attention: genetic risk for schizophrenia or bipolar disorder and its impact on brain network function. Front. Psychiatry 5:50. doi:10.3389/fpsyt.2014.00050
Pubmed Abstract | Pubmed Full Text | CrossRef Full Text | Google Scholar
Diwadkar, V. A., Carpenter, P. A., and Just, M. A. (2000). Collaborative activity between parietal and dorso-lateral prefrontal cortex in dynamic spatial working memory revealed by fMRI. Neuroimage 12, 85–99. doi:10.1006/nimg.2000.0586
Pubmed Abstract | Pubmed Full Text | CrossRef Full Text | Google Scholar
Diwadkar, V. A., Meintjes, E. M., Goradia, D., Dodge, N. C., Warton, C., Molteno, C. D., et al. (2013). Differences in cortico-striatal-cerebellar activation during working memory in syndromal and nonsyndromal children with prenatal alcohol exposure. Hum. Brain Mapp. 34, 1931–1945. doi:10.1002/hbm.22042
Pubmed Abstract | Pubmed Full Text | CrossRef Full Text | Google Scholar
Diwadkar, V. A., Pruitt, P., Goradia, D., Murphy, E., Bakshi, N., Keshavan, M. S., et al. (2011). Fronto-parietal hypo-activation during working memory independent of structural abnormalities: conjoint fMRI and sMRI analyses in adolescent offspring of schizophrenia patients. Neuroimage 58, 234–241. doi:10.1016/j.neuroimage.2011.06.033
Pubmed Abstract | Pubmed Full Text | CrossRef Full Text | Google Scholar
Diwadkar, V. A., Pruitt, P., Zhang, A., Radwan, J., Keshavan, M. S., Murphy, E., et al. (2012). The neural correlates of performance in adolescents at risk for schizophrenia: inefficiently increased cortico-striatal responses measured with fMRI. J. Psychiatr. Res. 46, 12–21. doi:10.1016/j.jpsychires.2011.09.016
Pubmed Abstract | Pubmed Full Text | CrossRef Full Text | Google Scholar
Egner, T., and Hirsch, J. (2005). Cognitive control mechanisms resolve conflict through cortical amplification of task-relevant information. Nat. Neurosci. 8, 1784–1790. doi:10.1038/nn1594
Pubmed Abstract | Pubmed Full Text | CrossRef Full Text | Google Scholar
Eshel, N., Nelson, E. E., Blair, R. J., Pine, D. S., and Ernst, M. (2007). Neural substrates of choice selection in adults and adolescents: development of the ventrolateral prefrontal and anterior cingulate cortices. Neuropsychologia 45, 1270–1279. doi:10.1016/j.neuropsychologia.2006.10.004
Pubmed Abstract | Pubmed Full Text | CrossRef Full Text | Google Scholar
Fan, J., Mccandliss, B. D., Fossella, J., Flombaum, J. I., and Posner, M. I. (2005). The activation of attentional networks. Neuroimage 26, 471–479. doi:10.1016/j.neuroimage.2005.02.004
Fitzgerald, K. D., Welsh, R. C., Gehring, W. J., Abelson, J. L., Himle, J. A., Liberzon, I., et al. (2005). Error-related hyperactivity of the anterior cingulate cortex in obsessive-compulsive disorder. Biol. Psychiatry 57, 287–294. doi:10.1016/j.biopsych.2004.10.038
Pubmed Abstract | Pubmed Full Text | CrossRef Full Text | Google Scholar
Fitzgerald, K. D., Welsh, R. C., Stern, E. R., Angstadt, M., Hanna, G. L., Abelson, J. L., et al. (2011). Developmental alterations of frontal-striatal-thalamic connectivity in obsessive-compulsive disorder. J. Am. Acad. Child Adolesc. Psychiatry 50, e933. doi:10.1016/j.jaac.2011.06.011
Pubmed Abstract | Pubmed Full Text | CrossRef Full Text | Google Scholar
Friston, K. J. (1998). The disconnection hypothesis. Schizophr. Res. 30, 115–125. doi:10.1016/S0920-9964(97)00140-0
Friston, K. J. (2011). Functional and effective connectivity: a review. Brain Connect. 1, 13–36. doi:10.1089/brain.2011.0008
Pubmed Abstract | Pubmed Full Text | CrossRef Full Text | Google Scholar
Friston, K. J., Buechel, C., Fink, G. R., Morris, J., Rolls, E., and Dolan, R. J. (1997). Psychophysiological and modulatory interactions in neuroimaging. Neuroimage 6, 218–229. doi:10.1006/nimg.1997.0291
Pubmed Abstract | Pubmed Full Text | CrossRef Full Text | Google Scholar
Funahashi, S., and Andreau, J. M. (2013). Prefrontal cortex and neural mechanisms of executive function. J. Physiol. Paris 107, 471–482. doi:10.1016/j.jphysparis.2013.05.001
Pubmed Abstract | Pubmed Full Text | CrossRef Full Text | Google Scholar
Goodman, W. K., Price, L. H., Rasmussen, S. A., Mazure, C., Delgado, P., Heninger, G. R., et al. (1989). The Yale-Brown obsessive compulsive scale. II. Validity. Arch. Gen. Psychiatry 46, 1012–1016. doi:10.1001/archpsyc.1989.01810110048007
Pubmed Abstract | Pubmed Full Text | CrossRef Full Text | Google Scholar
Haber, S. N., and Calzavara, R. (2009). The cortico-basal ganglia integrative network: the role of the thalamus. Brain Res. Bull. 78, 69–74. doi:10.1016/j.brainresbull.2008.09.013
Pubmed Abstract | Pubmed Full Text | CrossRef Full Text | Google Scholar
Harkin, B., and Kessler, K. (2011). The role of working memory in compulsive checking and OCD: a systematic classification of 58 experimental findings. Clin. Psychol. Rev. 31, 1004–1021. doi:10.1016/j.cpr.2011.06.004
Pubmed Abstract | Pubmed Full Text | CrossRef Full Text | Google Scholar
Harrison, B. J., Soriano-Mas, C., Pujol, J., Ortiz, H., Lopez-Sola, M., Hernandez-Ribas, R., et al. (2009). Altered corticostriatal functional connectivity in obsessive-compulsive disorder. Arch. Gen. Psychiatry 66, 1189–1200. doi:10.1001/archgenpsychiatry.2009.152
Pubmed Abstract | Pubmed Full Text | CrossRef Full Text | Google Scholar
Hazy, T. E., Frank, M. J., and O’reilly, R. C. (2006). Banishing the homunculus: making working memory work. Neuroscience 139, 105–118. doi:10.1016/j.neuroscience.2005.04.067
Pubmed Abstract | Pubmed Full Text | CrossRef Full Text | Google Scholar
Herrero, M. T., Barcia, C., and Navarro, J. M. (2002). Functional anatomy of thalamus and basal ganglia. Childs Nerv. Syst. 18, 386–404. doi:10.1007/s00381-002-0604-1
Pubmed Abstract | Pubmed Full Text | CrossRef Full Text | Google Scholar
Honey, G. D., Pomarol-Clotet, E., Corlett, P. R., Honey, R. A., Mckenna, P. J., Bullmore, E. T., et al. (2005). Functional dysconnectivity in schizophrenia associated with attentional modulation of motor function. Brain 128, 2597–2611. doi:10.1093/brain/awh632
Pubmed Abstract | Pubmed Full Text | CrossRef Full Text | Google Scholar
Huyser, C., Veltman, D. J., Wolters, L. H., De Haan, E., and Boer, F. (2011). Developmental aspects of error and high-conflict-related brain activity in pediatric obsessive-compulsive disorder: a fMRI study with a Flanker task before and after CBT. J. Child Psychol. Psychiatry 52, 1251–1260. doi:10.1111/j.1469-7610.2011.02439.x
Pubmed Abstract | Pubmed Full Text | CrossRef Full Text | Google Scholar
Insel, T., Cuthbert, B., Garvey, M., Heinssen, R., Pine, D. S., Quinn, K., et al. (2010). Research domain criteria (RDoC): toward a new classification framework for research on mental disorders. Am. J. Psychiatry 167, 748–751. doi:10.1176/appi.ajp.2010.09091379
Jansma, J. M., Ramsey, N. F., Van Der Wee, N. J., and Kahn, R. S. (2004). Working memory capacity in schizophrenia: a parametric fMRI study. Schizophr. Res. 68, 159–171. doi:10.1016/S0920-9964(03)00127-0
Pubmed Abstract | Pubmed Full Text | CrossRef Full Text | Google Scholar
Kaufman, J., Birmaher, B., Brent, D., Rao, U., Flynn, C., Moreci, P., et al. (1997). Schedule for affective disorders and schizophrenia for school-age children-present and lifetime version (K-SADS-PL): initial reliability and validity data. J. Am. Acad. Child Adolesc. Psychiatry 36, 980–988. doi:10.1097/00004583-199707000-00021
Pubmed Abstract | Pubmed Full Text | CrossRef Full Text | Google Scholar
Klein, J. C., Rushworth, M. F., Behrens, T. E., Mackay, C. E., De Crespigny, A. J., D’arceuil, H., et al. (2010). Topography of connections between human prefrontal cortex and mediodorsal thalamus studied with diffusion tractography. Neuroimage 51, 555–564. doi:10.1016/j.neuroimage.2010.02.062
Pubmed Abstract | Pubmed Full Text | CrossRef Full Text | Google Scholar
Koch, J., and Exner, C. (2015). Selective attention deficits in obsessive-compulsive disorder: the role of metacognitive processes. Psychiatry Res. 225, 550–555. doi:10.1016/j.psychres.2014.11.049
Pubmed Abstract | Pubmed Full Text | CrossRef Full Text | Google Scholar
Koch, K., Wagner, G., Schachtzabel, C., Peikert, G., Schultz, C. C., Sauer, H., et al. (2012). Aberrant anterior cingulate activation in obsessive-compulsive disorder is related to task complexity. Neuropsychologia 50, 958–964. doi:10.1016/j.neuropsychologia.2012.02.002
Pubmed Abstract | Pubmed Full Text | CrossRef Full Text | Google Scholar
Langner, R., and Eickhoff, S. B. (2013). Sustaining attention to simple tasks: a meta-analytic review of the neural mechanisms of vigilant attention. Psychol. Bull. 139, 870–900. doi:10.1037/a0030694
Pubmed Abstract | Pubmed Full Text | CrossRef Full Text | Google Scholar
Li, W., Qin, W., Liu, H., Fan, L., Wang, J., Jiang, T., et al. (2013). Subregions of the human superior frontal gyrus and their connections. Neuroimage 78, 46–58. doi:10.1016/j.neuroimage.2013.04.011
Pubmed Abstract | Pubmed Full Text | CrossRef Full Text | Google Scholar
Logothetis, N. K. (2008). What we can do and what we cannot do with fMRI. Nature 453, 869–878. doi:10.1038/nature06976
Pubmed Abstract | Pubmed Full Text | CrossRef Full Text | Google Scholar
Logothetis, N. K., and Wandell, B. A. (2004). Interpreting the BOLD signal. Annu. Rev. Physiol. 66, 735–769. doi:10.1146/annurev.physiol.66.082602.092845
Lucke, I. M., Lin, C., Conteh, F., Federline, A., Sung, H., Specht, M., et al. (2014). Continuous performance test in pediatric obsessive-compulsive disorder and tic disorders: the role of sustained attention. CNS Spectr. 1–11. doi:10.1017/S1092852914000467
Pubmed Abstract | Pubmed Full Text | CrossRef Full Text | Google Scholar
Maldjian, J. A., Laurienti, P. J., Kraft, R. A., and Burdette, J. H. (2003). An automated method for neuroanatomic and cytoarchitectonic atlas-based interrogation of fMRI data sets. Neuroimage 19, 1233–1239. doi:10.1016/S1053-8119(03)00169-1
Pubmed Abstract | Pubmed Full Text | CrossRef Full Text | Google Scholar
Maltby, N., Tolin, D. F., Worhunsky, P., O’keefe, T. M., and Kiehl, K. A. (2005). Dysfunctional action monitoring hyperactivates frontal-striatal circuits in obsessive-compulsive disorder: an event-related fMRI study. Neuroimage 24, 495–503. doi:10.1016/j.neuroimage.2004.08.041
Pubmed Abstract | Pubmed Full Text | CrossRef Full Text | Google Scholar
Manoach, D. S. (2003). Prefrontal cortex dysfunction during working memory performance in schizophrenia: reconciling discrepant findings. Schizophr. Res. 60, 285–298. doi:10.1016/S0920-9964(02)00294-3
Pubmed Abstract | Pubmed Full Text | CrossRef Full Text | Google Scholar
Marsh, R., Horga, G., Parashar, N., Wang, Z., Peterson, B. S., and Simpson, H. B. (2014). Altered activation in fronto-striatal circuits during sequential processing of conflict in unmedicated adults with obsessive-compulsive disorder. Biol. Psychiatry 75, 615–622. doi:10.1016/j.biopsych.2013.02.004
Pubmed Abstract | Pubmed Full Text | CrossRef Full Text | Google Scholar
McAlonan, K., Cavanaugh, J., and Wurtz, R. H. (2008). Guarding the gateway to cortex with attention in visual thalamus. Nature 456, 391–394. doi:10.1038/nature07382
Pubmed Abstract | Pubmed Full Text | CrossRef Full Text | Google Scholar
McNab, F., and Klingberg, T. (2008). Prefrontal cortex and basal ganglia control access to working memory. Nat. Neurosci. 11, 103–107. doi:10.1038/nn2024
Pubmed Abstract | Pubmed Full Text | CrossRef Full Text | Google Scholar
Meisenzahl, E. M., Schmitt, G. J., Scheuerecker, J., and Moller, H. J. (2007). The role of dopamine for the pathophysiology of schizophrenia. Int. Rev. Psychiatry 19, 337–345. doi:10.1080/09540260701502468
Pubmed Abstract | Pubmed Full Text | CrossRef Full Text | Google Scholar
Melloni, M., Urbistondo, C., Sedeno, L., Gelormini, C., Kichic, R., and Ibanez, A. (2012). The extended fronto-striatal model of obsessive compulsive disorder: convergence from event-related potentials, neuropsychology and neuroimaging. Front. Hum. Neurosci. 6:259. doi:10.3389/fnhum.2012.00259
Pubmed Abstract | Pubmed Full Text | CrossRef Full Text | Google Scholar
Nakao, T., Nakagawa, A., Nakatani, E., Nabeyama, M., Sanematsu, H., Yoshiura, T., et al. (2009). Working memory dysfunction in obsessive-compulsive disorder: a neuropsychological and functional MRI study. J. Psychiatr. Res. 43, 784–791. doi:10.1016/j.jpsychires.2008.10.013
Pubmed Abstract | Pubmed Full Text | CrossRef Full Text | Google Scholar
O’Reilly, J. X., Woolrich, M. W., Behrens, T. E., Smith, S. M., and Johansen-Berg, H. (2012). Tools of the trade: psychophysiological interactions and functional connectivity. Soc. Cogn. Affect. Neurosci. 7, 604–609. doi:10.1093/scan/nss055
Pubmed Abstract | Pubmed Full Text | CrossRef Full Text | Google Scholar
Owen, A. M., Mcmillan, K. M., Laird, A. R., and Bullmore, E. (2005). N-back working memory paradigm: a meta-analysis of normative functional neuroimaging studies. Hum. Brain Mapp. 25, 46–59. doi:10.1002/hbm.20131
Pubmed Abstract | Pubmed Full Text | CrossRef Full Text | Google Scholar
Palomero-Gallagher, N., Mohlberg, H., Zilles, K., and Vogt, B. (2008). Cytology and receptor architecture of human anterior cingulate cortex. J. Comp. Neurol. 508, 906–926. doi:10.1002/cne.21684
Pubmed Abstract | Pubmed Full Text | CrossRef Full Text | Google Scholar
Park, H. J., and Friston, K. (2013). Structural and functional brain networks: from connections to cognition. Science 342, 1238411. doi:10.1126/science.1238411
Pubmed Abstract | Pubmed Full Text | CrossRef Full Text | Google Scholar
Pauls, D. L., Abramovitch, A., Rauch, S. L., and Geller, D. A. (2014). Obsessive-compulsive disorder: an integrative genetic and neurobiological perspective. Nat. Rev. Neurosci. 15, 410–424. doi:10.1038/nrn3746
Pubmed Abstract | Pubmed Full Text | CrossRef Full Text | Google Scholar
Paus, T. (2001). Primate anterior cingulate cortex: where motor control, drive and cognition interface. Nat. Rev. Neurosci. 2, 417–424. doi:10.1038/35077500
Pubmed Abstract | Pubmed Full Text | CrossRef Full Text | Google Scholar
Paus, T., Keshavan, M., and Giedd, J. N. (2008). Why do many psychiatric disorders emerge during adolescence? Nat. Rev. Neurosci. 9, 947–957. doi:10.1038/nrn2513
Pubmed Abstract | Pubmed Full Text | CrossRef Full Text | Google Scholar
Peng, Z., Shi, F., Shi, C., Yang, Q., Chan, R. C., and Shen, D. (2014). Disrupted cortical network as a vulnerability marker for obsessive-compulsive disorder. Brain Struct. Funct. 219, 1801–1812. doi:10.1007/s00429-013-0602-y
Pubmed Abstract | Pubmed Full Text | CrossRef Full Text | Google Scholar
Piras, F., Piras, F., Caltagirone, C., and Spalletta, G. (2013). Brain circuitries of obsessive compulsive disorder: a systematic review and meta-analysis of diffusion tensor imaging studies. Neurosci. Biobehav. Rev. 37, 2856–2877. doi:10.1016/j.neubiorev.2013.10.008
Pubmed Abstract | Pubmed Full Text | CrossRef Full Text | Google Scholar
Ragsdale, C. W. Jr., and Graybiel, A. M. (1990). A simple ordering of neocortical areas established by the compartmental organization of their striatal projections. Proc. Natl. Acad. Sci. U.S.A. 87, 6196–6199. doi:10.1073/pnas.87.16.6196
Pubmed Abstract | Pubmed Full Text | CrossRef Full Text | Google Scholar
Ray, J. P., and Price, J. L. (1993). The organization of projections from the mediodorsal nucleus of the thalamus to orbital and medial prefrontal cortex in macaque monkeys. J. Comp. Neurol. 337, 1–31. doi:10.1002/cne.903370102
Pubmed Abstract | Pubmed Full Text | CrossRef Full Text | Google Scholar
Rehme, A. K., Eickhoff, S. B., and Grefkes, C. (2013). State-dependent differences between functional and effective connectivity of the human cortical motor system. Neuroimage 67, 237–246. doi:10.1016/j.neuroimage.2012.11.027
Pubmed Abstract | Pubmed Full Text | CrossRef Full Text | Google Scholar
Rosenberg, D. R., Macmaster, F. P., Keshavan, M. S., Fitzgerald, K. D., Stewart, C. M., and Moore, G. J. (2000). Decrease in caudate glutamatergic concentrations in pediatric obsessive - compulsive disorder patients taking paroxetine. J. Am. Acad. Child Adolesc. Psychiatry 39, 1096–1103. doi:10.1097/00004583-200009000-00008
Pubmed Abstract | Pubmed Full Text | CrossRef Full Text | Google Scholar
Rosenberg, D. R., Mirza, Y., Russell, A., Tang, J., Smith, J. M., Banerjee, S. P., et al. (2004). Reduced anterior cingulate glutamatergic concentrations in childhood OCD and major depression versus healthy controls. J. Am. Acad. Child Adolesc. Psychiatry 43, 1146–1153. doi:10.1097/01.chi.0000132812.44664.2d
Pubmed Abstract | Pubmed Full Text | CrossRef Full Text | Google Scholar
Scahill, L., Riddle, M. A., Mcswiggin-Hardin, M., Ort, S. I., King, R. A., Goodman, W. K., et al. (1997). Children’s Yale-Brown obsessive compulsive scale: reliability and validity. J. Am. Acad. Child Adolesc. Psychiatry 36, 844–852. doi:10.1097/00004583-199706000-00023
Pubmed Abstract | Pubmed Full Text | CrossRef Full Text | Google Scholar
Schmidt, A., Smieskova, R., Aston, J., Simon, A., Allen, P., Fusar-Poli, P., et al. (2013). Brain connectivity abnormalities predating the onset of psychosis: correlation with the effect of medication. JAMA Psychiatry 70, 903–912. doi:10.1001/jamapsychiatry.2013.117
Pubmed Abstract | Pubmed Full Text | CrossRef Full Text | Google Scholar
Shaw, M. E., Moores, K. A., Clark, R. C., Mcfarlane, A. C., Strother, S. C., Bryant, R. A., et al. (2009). Functional connectivity reveals inefficient working memory systems in post-traumatic stress disorder. Psychiatry Res. 172, 235–241. doi:10.1016/j.pscychresns.2008.07.014
Pubmed Abstract | Pubmed Full Text | CrossRef Full Text | Google Scholar
Sohn, M. H., Albert, M. V., Jung, K., Carter, C. S., and Anderson, J. R. (2007). Anticipation of conflict monitoring in the anterior cingulate cortex and the prefrontal cortex. Proc. Natl. Acad. Sci. U.S.A. 104, 10330–10334. doi:10.1073/pnas.0703225104
Pubmed Abstract | Pubmed Full Text | CrossRef Full Text | Google Scholar
Stephan, K. E. (2004). On the role of general system theory for functional neuroimaging. J. Anat. 205, 443–470. doi:10.1111/j.0021-8782.2004.00359.x
Pubmed Abstract | Pubmed Full Text | CrossRef Full Text | Google Scholar
Stephan, K. E., Baldeweg, T., and Friston, K. J. (2006). Synaptic plasticity and dysconnection in schizophrenia. Biol. Psychiatry 59, 929–939. doi:10.1016/j.biopsych.2005.10.005
Pubmed Abstract | Pubmed Full Text | CrossRef Full Text | Google Scholar
Stewart, S. E., Mayerfeld, C., Arnold, P. D., Crane, J. R., O’dushlaine, C., Fagerness, J. A., et al. (2013). Meta-analysis of association between obsessive-compulsive disorder and the 3’ region of neuronal glutamate transporter gene SLC1A1. Am. J. Med. Genet. B Neuropsychiatr. Genet. 162b, 367–379. doi:10.1002/ajmg.b.32137
Pubmed Abstract | Pubmed Full Text | CrossRef Full Text | Google Scholar
Tottenham, N., and Sheridan, M. A. (2009). A review of adversity, the amygdala and the hippocampus: a consideration of developmental timing. Front. Hum. Neurosci. 3:68. doi:10.3389/neuro.09.068.2009
Pubmed Abstract | Pubmed Full Text | CrossRef Full Text | Google Scholar
Valleni-Basile, L. A., Garrison, C. Z., Waller, J. L., Addy, C. L., Mckeown, R. E., Jackson, K. L., et al. (1996). Incidence of obsessive-compulsive disorder in a community sample of young adolescents. J. Am. Acad. Child Adolesc. Psychiatry 35, 898–906. doi:10.1097/00004583-199607000-00015
Pubmed Abstract | Pubmed Full Text | CrossRef Full Text | Google Scholar
van Veen, V., Cohen, J. D., Botvinick, M. M., Stenger, V. A., and Carter, C. S. (2001). Anterior cingulate cortex, conflict monitoring, and levels of processing. Neuroimage 14, 1302–1308. doi:10.1006/nimg.2001.0923
Pubmed Abstract | Pubmed Full Text | CrossRef Full Text | Google Scholar
Vijayraghavan, S., Wang, M., Birnbaum, S. G., Williams, G. V., and Arnsten, A. F. (2007). Inverted-U dopamine D1 receptor actions on prefrontal neurons engaged in working memory. Nat. Neurosci. 10, 376–384. doi:10.1038/nn1846
Voytek, B., and Knight, R. T. (2010). Prefrontal cortex and basal ganglia contributions to visual working memory. Proc. Natl. Acad. Sci. U.S.A. 107, 18167–18172. doi:10.1073/pnas.1007277107
Pubmed Abstract | Pubmed Full Text | CrossRef Full Text | Google Scholar
Ward, B. D. (2000). Simultaneous Inference for fMRI Data. Milwaukee, WI: Medical College of Wisconsin.
Wolff, R. P., and Wolff, L. S. (1991). Assessment and treatment of obsessive-compulsive disorder in children. Behav. Modif. 15, 372–393. doi:10.1177/01454455910153006
Pubmed Abstract | Pubmed Full Text | CrossRef Full Text | Google Scholar
Wu, K., Hanna, G. L., Easter, P., Kennedy, J. L., Rosenberg, D. R., and Arnold, P. D. (2013). Glutamate system genes and brain volume alterations in pediatric obsessive-compulsive disorder: a preliminary study. Psychiatry Res. 211, 214–220. doi:10.1016/j.pscychresns.2012.07.003
Pubmed Abstract | Pubmed Full Text | CrossRef Full Text | Google Scholar
Wu, K., Hanna, G. L., Rosenberg, D. R., and Arnold, P. D. (2012). The role of glutamate signaling in the pathogenesis and treatment of obsessive-compulsive disorder. Pharmacol. Biochem. Behav. 100, 726–735. doi:10.1016/j.pbb.2011.10.007
Pubmed Abstract | Pubmed Full Text | CrossRef Full Text | Google Scholar
Keywords: dorsal anterior cingulate cortex, obsessive-compulsive disorder, network analysis, working memory, fMRI
Citation: Diwadkar VA, Burgess A, Hong E, Rix C, Arnold PD, Hanna GL and Rosenberg DR (2015) Dysfunctional activation and brain network profiles in youth with obsessive-compulsive disorder: a focus on the dorsal anterior cingulate during working memory. Front. Hum. Neurosci. 9:149. doi: 10.3389/fnhum.2015.00149
Received: 12 November 2014; Accepted: 03 March 2015;
Published online: 17 March 2015.
Edited by:
Baojuan Li, Fourth Military Medical University, ChinaReviewed by:
Dewen Hu, National University of Defense Technology, ChinaLirong Yan, Wuhan General Hospital, China
Copyright: © 2015 Diwadkar, Burgess, Hong, Rix, Arnold, Hanna and Rosenberg. This is an open-access article distributed under the terms of the Creative Commons Attribution License (CC BY). The use, distribution or reproduction in other forums is permitted, provided the original author(s) or licensor are credited and that the original publication in this journal is cited, in accordance with accepted academic practice. No use, distribution or reproduction is permitted which does not comply with these terms.
*Correspondence: Vaibhav A. Diwadkar, Department of Psychiatry and Behavioral Neurosciences, Brain Imaging Research Division, Wayne State University School of Medicine, Suite 5B, 3901 Chrysler Drive, Detroit, MI 48201, USA e-mail: vdiwadka@med.wayne.edu