- 1Neurology Unit, Department of Medicine, Faculty of Sciences, University of Fribourg, Fribourg, Switzerland
- 2Psychiatry Unit, Department of Medicine, Faculty of Sciences, University of Fribourg, Fribourg, Switzerland
- 3Laboratory of Cognitive Neurorehabilitation, Department of Clinical Neurosciences, Medical School, University of Geneva, Geneva, Switzerland
Deficits in inhibitory control, the ability to suppress ongoing or planned motor or cognitive processes, contribute to many psychiatric and neurological disorders. The rehabilitation of inhibition-related disorders may therefore benefit from neuroplasticity-based training protocols aiming at normalizing inhibitory control proficiency and the underlying brain networks. Current literature on training-induced behavioral and brain plasticity in inhibitory control suggests that improvements may follow either from the development of automatic forms of inhibition or from the strengthening of top-down, controlled inhibition. Automatic inhibition develops in conditions of consistent and repeated associations between inhibition-triggering stimuli and stopping goals. Once established, the stop signals directly elicit inhibition, thereby bypassing slow, top-down executive control and accelerating stopping processes. In contrast, training regimens involving varying stimulus-response associations or frequent inhibition failures prevent the development of automatic inhibition and thus strengthen top-down inhibitory processes rather than bottom-up ones. We discuss these findings in terms of developing optimal inhibitory control training regimens for rehabilitation purposes.
Inhibitory Control
Measures of Inhibitory Control
Inhibitory control refers to the ability to suppress ongoing or planned motor or cognitive processes and enables adapting to rapidly changing situations (Aron et al., 2004; Aron, 2007). While in the current review, we focus on the inhibitory control of motor responses, it is worth noting that a large variety of experimental paradigms involving—at least partly—inhibition processes have been used in training studies [e.g., Flanker, Stroop, or antisaccade tasks; reviewed in Aron (2011)]. Because these tasks involve many different components in addition to inhibitory control [attention, working memory (WM), etc], they have been presented as concerning the more general “executive control” construct. Further work on training-induced plasticity of inhibition focused on training self-control (e.g., Muraven, 2010). The stop-signal task (SST; Lappin and Eriksen, 1966) and the Go/NoGo task (Donders, 1969) involve very directly motor inhibitory control and were thus primarily used to train this aspect.
The SST consists of a speeded discrimination task in which responses to the stimuli have to be canceled when a stop signal is presented (Logan et al., 1984; Band et al., 2003). The shorter the delay between the stimulus and the stop signal, the higher is the probability of successfully inhibiting an ongoing response. SST performance is usually indexed by stop-signal reaction time (SSRT) calculated as the delay from the stop signal at which the stopping process successfully suppresses the motor response to the preceding go signal (e.g., Logan, 1994). By contrast, in the Go/NoGo task, participants have to respond as fast as possible to a set of stimuli, while withholding their responses to another set of stimuli. The number of responses to NoGo stimuli (false alarms) and reaction times to Go stimuli index inhibition performance.
Neural Bases of Inhibitory Control
Converging neuroimaging and clinical data indicate that inhibitory control depends on a cortico-subcortical network comprising the inferior frontal gyrus (IFG), the pre-supplementary motor area (preSMA), and the basal ganglia (for reviews see, e.g., Aron, 2007; Chambers et al., 2009; Chikazoe, 2010). The inhibitory processes typically take place around 100–200 ms after the onset of the stop-stimulus (Falkenstein et al., 1995, 1999; Rubia et al., 2001; Aron et al., 2004). Current models of inhibitory control suggest that action elicitation (“Go”) and cancelation (“Stop”) processes are mostly independent up to their final stage, where they interact to ultimately suppress prepotent or actual behavioral responses if a stop signal was presented (Hanes et al., 1998; Aron et al., 2007; Boucher et al., 2007). Once processed by early sensory cortices, the information about the Go signals is relayed to the premotor area, which activates primary motor areas via the striatum, pallidum, and thalamus (Aron and Poldrack, 2006). The information about Stop signals is relayed to the inferior frontal cortices and/or the pre-SMA; these structures generate the inhibition via the basal ganglia input nuclei [notably the subthalamic nucleus (STN)], which connects to the globus pallidus mediating response inhibition by suppressing the final stages of the Go process (Aron et al., 2007; Chambers et al., 2009; Aron, 2011).
Domain-General Inhibitory Control Mechanism
Latent variables analyses suggest that in addition to task-specific processes, there is a common, domain-general inhibitory control mechanism involved across all inhibitory tasks (Brocki and Bohlin, 2004; Friedman and Miyake, 2004), and contributing to many higher-order cognitive abilities including, for example, abstract reasoning, the resolution of complex problem, and decision making (Chiappe et al., 2000; Houde et al., 2000; Handley et al., 2004; Viskontas et al., 2004; Cain, 2006; De Neys and Everaerts, 2008). The existence of a domain-general inhibitory control mechanism is further supported by functional neuroimaging evidence for the involvement of the same fronto-striatal network in the inhibition of various cognitive and motor processes. The pre-SMA, IFG, and STN have indeed been shown to support the inhibition of eye movements (Chikazoe et al., 2007), linguistic processes (Xue et al., 2006, 2008), thoughts, memories and even emotions (Jonides et al., 1998; Depue et al., 2007; Dillon and Pizzagalli, 2007; Mitchell et al., 2007). Of note, however, whether this network is solely involved in inhibitory control or more general attentional phenomena remains debated (e.g., Hampshire et al., 2010).
Plasticity of Inhibitory Control
With regard to the considerable research efforts having addressed the neural underpinnings of inhibitory control, whether this function can be improved with training and whether the underlying brain networks are subject to plastic changes remain surprisingly unknown.
Broadly defined, “plasticity” refers to experience-dependent modifications of the behavior and of its underlying anatomo-functional brain organization. These modifications support the acquisition of new skills, the improvement of already acquired abilities and the recovery of functional deficits or disorders (e.g., Kelly and Garavan, 2005). Training-induced behavioral and brain plastic changes have been demonstrated at the level of various executive functions. For instance, training WM or planning abilities improves performance (Dahlin et al., 2008; Jaeggi et al., 2008; Li et al., 2008; Von Bastian et al., 2013) and modifies the activity of fronto-parietal or medial frontal brain network, respectively (Beauchamp et al., 2003; Olesen et al., 2004; Dahlin et al., 2008; Klingberg, 2010).
While the plasticity of WM training has received much attention within the field of executive functions, only few studies investigated plasticity of inhibitory control (see Table 1). Hypotheses about the neuroplastic changes underlying inhibitory control, therefore, are only beginning to emerge. The literature so far documents two main mechanisms of training-induced behavioral and brain plasticity of inhibitory control: the development of bottom-up, automatic forms of inhibition and the optimization of top-down, controlled forms of inhibition (Figure 1).
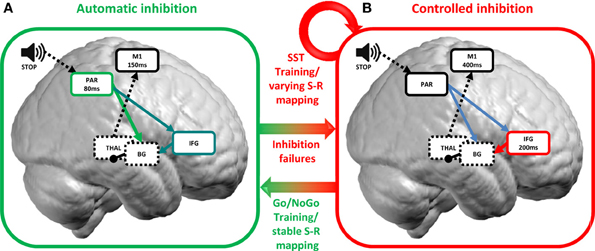
Figure 1. Two mechanisms of training-induced plasticity of inhibitory control. Inhibition stimuli are conveyed to sensory areas processing stimuli features at ca. 80 ms within parietal brain regions (Hyde et al., 2008; Spierer et al., 2008). (A) In conditions of stable S-R mapping, as for the Go/NoGo task, participants switch from a controlled to an automatic inhibition mode with training. Automatic inhibition develops in parietal areas at ca. 80 ms and shortcuts top-down inputs from the IFG (green arrow; although see Lenartowicz et al., 2011 for evidence of a role for the IFG in automatic inhibition; blue arrows) in turn leading to faster inhibition (ca. 150 ms; calculated as the mean RT −100 ms which corresponds to the latency of M1 initiation before motor execution; Thorpe and Fabre-Thorpe, 2001). (B) When S-R mapping varies (as in e.g., SST), top-down, controlled inhibition is modulated by training around 200 ms in the IFG. The IFG then activates subcortical basal ganglia (red arrow) which in turn inhibits the thalamocortical output and suppresses motor execution in M1. Error commission allows shifting from fast automatic to slow top-down controlled forms of inhibition. PAR, parietal; M1, primary motor cortex; IFG, inferior frontal gyrus; BG, basal ganglia; THAL, thalamus; S-R mapping, stimulus-response mapping. Arrows indicate excitatory connections and rounds inhibitory connections. Full lines indicate cortical structures and dashed lines indicate subcortical structures.
The Development of Automatic, Bottom-Up Forms of Inhibition
In a series of psychophysical experiments on inhibitory control training, Verbruggen and Logan (2008) showed that if Go and NoGo stimuli were respectively consistently associated with Go and NoGo goals during inhibitory control training with a classical Go/NoGo task, automatic (i.e., unintentional) forms of inhibition develop with practice. Based on evidence that when Go/NoGo stimulus-response mapping rules are reversed after a practice phase, response to Go stimuli are slowed, the authors advance that with training, automatic processes progressively replaced top-down controlled processes to inhibit prepotent motor responses. After the training, NoGo goals were automatically activated by NoGo stimuli and thus higher-order, top-down forms of control were no longer required to resolve the Go/NoGo task. In turn, inhibition was faster and Go/NoGo performance improved overall (see also Shiffrin and Schneider, 1977).
Recent work from our group provided insights into the neural mechanisms underlying the development of automatic forms of inhibitory control. Using a training paradigm involving stable stimulus-response mapping rules, we contrasted event-related evoked potentials (ERPs) to “Go” and “NoGo” stimuli between the beginning vs. the end of a 40 min auditory spatial Go/NoGo training and during passive listening of the same stimuli before vs. after the training session (Manuel et al., 2010). Consistent with the behavioral studies by Verbruggen and Logan (2008), we showed that training improved Go/NoGo performance. During the active training session, electrophysiological responses to NoGo, but not Go, stimuli engaged distinct brain networks at the beginning vs. the end of the training ca. 80 ms after stimulus onset, driven by a decrease in the activity of left parietal cortices. This modulation in brain activity correlated positively with the behavioral improvement in inhibitory control. As for the processing of the Go and NoGo stimuli in passive listening conditions, the training modulated ERPs in response to Go stimuli at ca. 50 ms, due to decreased right anterior temporo-parietal activity. These results support the automaticity hypothesis by Logan and colleagues: Driven by repeated and stable associations between NoGo stimuli and response withholding, inhibitory control training resulted in a progressive disengagement of frontal top-down inputs for resolving the inhibitory task in favor of fast automatic forms of inhibition (Shiffrin and Schneider, 1977; Logan, 1988; Verbruggen and Logan, 2008). Supporting this hypothesis, Manuel et al. (2010) reported that automatic inhibition was implemented within parietal areas, a region interfacing sensory representations of the feature discriminating auditory spatial Go and NoGo stimuli and response-related motor commands (Deiber et al., 1991, 1996; Decety et al., 1992; Andersen et al., 1997). Interestingly, Lenartowicz et al. (2011) reported a slowing down of response time for Go stimuli that were previously paired with a stop stimulus in a training phase. This automatically triggered inhibition was accompanied by an increase in IFG activity, suggesting that automatic inhibition may still be mediated by higher-order frontal areas. However, Manuel et al. (2010) used a Go/NoGo task and Lenartowicz et al. (2011) an SST; the inhibitory mechanisms in the two tasks might partly differ because in SST, ongoing motor responses have to be inhibited while in Go/NoGo, participants have to suppress prepotent responses.
Collectively, these studies suggest that behavioral improvements in inhibitory control with training regimens involving unvarying stimulus-response mappings are supported by the development of fast, automatic forms of response inhibition instead of relying solely on the reinforcement of top-down inputs from frontal areas (Manuel et al., 2010; Figure 1). Of note, the automatization of inhibitory control with practice is at odds with traditional views of inhibitory control as an inherently top-down executive process (Aron et al., 2004). Perhaps counter-intuitively, inhibitory control can be automatic and driven by brain areas usually thought to trigger movements. These results further suggest that top-down executive frontal areas are not always necessarily required to control behavior, or at least that the engagement of these inhibitory areas can be driven automatically by specific stimuli (Lenartowicz et al., 2011).
Modification of Controlled, Top-Down Forms of Inhibition
Based on our findings in Manuel et al. (2010), we hypothesized that when inhibitory control training is based on a task involving inconsistent mappings between stimulus and response, automatic processing would not develop. Rather, only top-down control mechanisms would be constantly solicited during the training phase, and thus ultimately modified by practice (Shiffrin and Schneider, 1977; Verbruggen and Logan, 2008). Such a pattern would, for instance, be induced by training with an SST because in SST, Go goals are engaged in all trials and must only sometimes be subsequently canceled.
To test this hypothesis, we contrasted event-related potentials to Go stimuli at the beginning vs. the end of a 1-h auditory stop signal training session (Manuel et al., 2013). The training improved inhibitory performance as evidenced by a significant decrease in stop-signal reaction time, i.e., the latency of the stop process. At the neurophysiological level, we observed plastic modification of a right lateralized fronto-basal network comprising the IFG, the pre-SMA and the basal ganglia at ca. 200 ms post-stimulus onset, suggesting that top-down executive networks were modified with SST training (Figure 1). Alternatively, such modification could also reflect a change in proactive control strategy since we contrasted responses to Go stimuli which were not consistently paired with going or stopping.
In addition to inconsistent stimulus-response associations, a second parameter may be manipulated to promote the modification of top-down mechanisms over the development of automatic inhibition: the difficulty of the task. An increase in the difficulty to inhibit prepotent or ongoing responses indeed increases inhibition failures (commission errors), which in turn lead to the (re)engagement of controlled forms of inhibition. During inhibitory tasks, post-error shifts to more cautious response modes translate into switches from fast automatic to slow, top-down controlled forms of inhibition (Manuel et al., 2012), which could account for post-error slowing effects (Rabbitt, 1966; Notebaert et al., 2009). Controlled forms of inhibition would be prominently involved in tasks generating many errors and thus training with difficult task would likely modify top-down mechanisms. Consistent with this finding, Benikos et al. (2013) showed that while training on a moderately difficult Go/NoGo task improved inhibitory control performance, training with lower or higher inhibitory demands failed to induce, respectively, an improvement or a decrease in performance. Moreover, Benikos et al. (2013) reported that training-induced improvements in the moderate inhibition load condition followed from an increase in the P3 evoked potential, compatible with a modification of top-down inhibition mechanisms.
Neurophysiological Mechanisms of Training-Induced Functional Changes
While current literature clearly suggests that inhibitory control is subject to fast plastic changes, the underlying neurophysiological mechanisms remain largely unresolved. In the studies reported above, performance improvements were accompanied by a decrease in the activation strength of inhibition-related stimuli (Manuel et al., 2010, 2013). Although very speculative, such pattern of training-induced functional changes might follow from an increase in synaptic efficacy and/or in neural efficiency (Haier et al., 1992), yielding to a decrease of the number of neurons responding during the trained task, which would in turn increase the speed of inhibition processes (Schoups et al., 1998; Poldrack, 2000; Song et al., 2002; Kelly and Garavan, 2005). The refinement of inhibitory control network could also follow from strengthening in the synaptic connections between the neural ensembles involved in the inhibition and in a decrease in the connections with less critical regions (Galvan, 2010). Further studies should also determine whether and how inhibitory control training may induce structural modifications of the involved brain areas. Modification of gray matter volume or density (e.g., following from neuro- or synapto-genesis), as well as changes in neuronal morphology or in glial cells have indeed been demonstrated following the training of many cognitive and motor skills (Zatorre et al., 2012; Thomas and Baker, 2013 for reviews). Similarly, training-induced plasticity has been shown at the level of white matter microstructure (Zatorre et al., 2012).
Generalization of the Effects of Training
We have reported above how specific inhibitory control training parameters promote either the development of automatic forms of inhibition or the modification of top-down controlled inhibition. Critically, each of the mechanisms of training-induced plasticity also determines the extent to which the effects of the training will generalize to other stimuli, conditions, or tasks. One could indeed hypothesize that if top-down control mechanisms are modified by training, the effects of practice would transfer to other untrained conditions or tasks supported by the same fronto-basal brain network. In contrast, because automatic inhibition consists in associations between specific stimuli and inhibition goals, the effects of training regimen promoting the development of automatic forms inhibition would unlikely generalize to untrained stimuli. Training-induced modifications of automatic inhibition processes might be, however, advantageous in the remediation of traits characterized by highly automatized (inappropriate) responses as in, e.g., impulsivity (Marteau et al., 2012).
Empirical support for these assumptions comes from Thorell et al. (2009), who showed that training on a Go/NoGo with consistent S-R mapping rules, improved inhibitory control on the trained Go/NoGo task, but did not transfer to other inhibitory control tasks (SST or Flanker), nor to other executive tasks including, e.g., WM or problem solving tasks. The fact that Go/NoGo training improved performance without transferring to other executive processes indicates that automatic, forms of inhibition, but not top-down controlled inhibition developed. In contrast, SST training led to larger transfer of the effect of training supporting that it modified higher-order top-down processes. Another example of distant transfer of the effects of inhibitory control training with stop signal task comes from Verbruggen et al. (2012), who showed that a brief training on an SST diminished risky behavior during a subsequent monetary gambling task. This study indicates that training executive processes at the motor level (stop signal task) transfer to other, non-motor, decision making tasks. Specifically designed training studies, examining systematically the generalization patterns of training regimens promoting either the development of automatic or controlled inhibition are required to elucidate the question of the transfer of the effects of inhibitory control training.
Several other studies demonstrate that when the domain-general inhibitory control network is modified by training, the effects of the training will influence subsequent complex behavior involving the same inhibitory control component (Houben and Jansen, 2011; Houben et al., 2011, 2012; Veling et al., 2011; Jones and Field, 2013). Houben et al. (2011) trained alcohol drinking participants on a Go/NoGo task. In one group, Go stimuli were consistently paired with alcohol-related stimuli, whereas in the second group, the NoGo stimuli were paired with alcohol-related stimuli. The results revealed that participants in the second group had increased negative attitudes toward alcohol and significantly reduced weekly alcohol consumption. The effects of inhibitory control training have also been tested on food consumption. Houben (2011) trained healthy participant with an SST in which they had to respond as fast as possible to Go stimuli and to withhold their response when a stop signal was presented after a Go signal. In an “inhibition” condition aiming at improving the inhibition of responses to food, high-calorie food items were systematically paired with a stop signal. In an “impulsivity” condition aiming at strengthening impulses to food, the high-calorie food item was never paired with a stop signal. The results revealed that immediately after the training, participants in the “inhibition” condition consumed significantly less high-calorie food than the participants who underwent the “impulsivity” condition. Similar effects were found by Houben and Jansen (2011), who showed that using “chocolate” items as NoGo stimuli decreased subsequent chocolate consumption as compared to conditions where chocolate items were presented as Go stimuli. Finally, Veling et al. (2011) showed that food-related SST training also helped chronic dieters to control behavior to food.
The reciprocal effect has also been confirmed by studies showing that practice on complex tasks involving an inhibitory control component improves performance on basic inhibitory control tasks. For instance, Di Russo et al. (2006) showed that fencing athletes were more proficient than control populations in Go/NoGo tasks.
Rehabilitation of Inhibition-Related Disorders
Evidence for training-induced improvement in inhibitory control and for the modification of the underlying fronto-basal network suggest that practicing inhibition tasks might help recovering for inhibition-related pathologies (e.g., Johnstone et al., 2012). Inhibitory control deficits and abnormal anatomic variations at various levels of the fronto-basal network supporting inhibitory control have indeed been advanced as constituting a causal factor or at least as being strongly associated with the emergence of psychiatric disorders including, e.g., addiction, schizophrenia, compulsive disorders, obesity, or ADHD (Badcock et al., 2002; Aron and Poldrack, 2005; Holroyd et al., 2005; Garavan and Hester, 2007; Ruchsow et al., 2007, 2008; Eisenberg and Berman, 2010; McLoughlin et al., 2010) as well as related pathological traits such as impulsivity (Aron and Poldrack, 2005; Knoch et al., 2006; Chambers et al., 2009).
Future Perspectives
Many questions remain open within the field of inhibitory control plasticity. For instance, the long-term effects of inhibitory control training, and the effects of long training sessions, remain unresolved. Houben et al. (2011) showed evidence for an effect of short Go/NoGo training on drinking behavior up to 1 week following training, suggesting that the improvements may persist over time. Regarding the length of training sessions, there is no direct evidence for a greater improvement with long rather than short training regimen, and the interaction between training duration and the persistence of the effects of training remain to be explored.
We have proposed a few task parameters favoring the reinforcement of controlled rather than automatic inhibitory processes, which in turn would promote the generalization of the effect of inhibitory control training to other conditions and tasks. These proposals remain, however, mostly speculative and need further investigations. In this regard, the relevance of inhibitory control training for the rehabilitation of inhibition-related disorders also needs to be further established.
Collectively, current literature on inhibitory control plasticity suggests that training inhibitory control may constitute a promising tool for the rehabilitation of many inhibition-related psychiatric and neurological disorders.
Conflict of Interest Statement
The authors declare that the research was conducted in the absence of any commercial or financial relationships that could be construed as a potential conflict of interest.
Acknowledgments
This work was supported by a grant from the Swiss National Science Foundation to Lucas Spierer (Grant #320030_143348). We thank Sebastian Dieguez, David Magezi, and the reviewers for their valuable comments on early versions of the manuscript.
References
Andersen, R. A., Snyder, L. H., Bradley, D. C., and Xing, J. (1997). Multimodal representation of space in the posterior parietal cortex and its use in planning movements. Annu. Rev. Neurosci. 20, 303–330. doi: 10.1146/annurev.neuro.20.1.303
Aron, A. R. (2007). The neural basis of inhibition in cognitive control. Neuroscientist 13, 214–228. doi: 10.1177/1073858407299288
Aron, A. R. (2011). From reactive to proactive and selective control: developing a richer model for stopping inappropriate responses. Biol. Psychiatry 69, e55–e68. doi: 10.1016/j.biopsych.2010.07.024
Aron, A. R., Durston, S., Eagle, D. M., Logan, G. D., Stinear, C. M., and Stuphorn, V. (2007). Converging evidence for a fronto-basal-ganglia network for inhibitory control of action and cognition. J. Neurosci. 27, 11860–11864. doi: 10.1523/JNEUROSCI.3644-07.2007
Aron, A. R., and Poldrack, R. A. (2005). The cognitive neuroscience of response inhibition: relevance for genetic research in attention-deficit/hyperactivity disorder. Biol. Psychiatry 57, 1285–1292. doi: 10.1016/j.biopsych.2004.10.026
Aron, A. R., and Poldrack, R. A. (2006). Cortical and subcortical contributions to stop signal response inhibition: role of the subthalamic nucleus. J. Neurosci. 26, 2424–2433. doi: 10.1523/JNEUROSCI.4682-05.2006
Aron, A. R., Robbins, T. W., and Poldrack, R. A. (2004). Inhibition and the right inferior frontal cortex. Trends Cogn. Sci. 8, 170–177. doi: 10.1016/j.tics.2004.02.010
Badcock, J. C., Michie, P. T., Johnson, L., and Combrinck, J. (2002). Acts of control in schizophrenia: dissociating the components of inhibition. Psychol. Med. 32, 287–297. doi: 10.1017/S0033291701005128
Band, G. P., Van Der Molen, M. W., and Logan, G. D. (2003). Horse-race model simulations of the stop-signal procedure. Acta. Psychol. (Amst) 112, 105–142. doi: 10.1016/S0001-691800079-3
Beauchamp, M. H., Dagher, A., Aston, J. A., and Doyon, J. (2003). Dynamic functional changes associated with cognitive skill learning of an adapted version of the tower of london task. Neuroimage 20, 1649–1660. doi: 10.1016/j.neuroimage.2003.07.003
Benikos, N., Johnstone, S. J., and Roodenrys, S. J. (2013). Short-term training in the go/nogo task: behavioural and neural changes depend on task demands. Int. J. Psychophysiol. 87, 262–272. doi: 10.1016/j.ijpsycho.2012.12.001
Boucher, L., Palmeri, T. J., Logan, G. D., and Schall, J. D. (2007). Inhibitory control in mind and brain: an interactive race model of countermanding saccades. Psychol. Rev. 114, 376–397. doi: 10.1037/0033-295X.114.2.376
Bowley, C., Faricy, C., Hegarty, B., Johnstone, J. S., Smith, L. J., Kelly, J. P., et al. (2013). The effects of inhibitory control training on alcohol consumption, implicit alcohol-related cognitions and brain electrical activity. Int. J. Psychophysiol. doi: 10.1016/j.ijpsycho.2013.04.011. [Epub ahead of print].
Brocki, K. C., and Bohlin, G. (2004). Executive functions in children aged 6 to 13: a dimensional and developmental study. Dev. Neuropsychol. 26, 571–593. doi: 10.1207/s15326942dn2602_3
Cain, K. (2006). Individual differences in children's memory and reading comprehension: an investigation of semantic and inhibitory deficits. Memory 14, 553–569. doi: 10.1080/09658210600624481
Chambers, C. D., Garavan, H., and Bellgrove, M. A. (2009). Insights into the neural basis of response inhibition from cognitive and clinical neuroscience. Neurosci. Biobehav. Rev. 33, 631–646. doi: 10.1016/j.neubiorev.2008.08.016
Chiappe, P., Hasher, L., and Siegel, L. S. (2000). Working memory, inhibitory control, and reading disability. Mem. Cognit. 28, 8–17. doi: 10.3758/BF03211570
Chikazoe, J. (2010). Localizing performance of go/no-go tasks to prefrontal cortical subregions. Curr. Opin. Psychiatry 23, 267–272. doi: 10.1097/YCO.0b013e3283387a9f
Chikazoe, J., Konishi, S., Asari, T., Jimura, K., and Miyashita, Y. (2007). Activation of right inferior frontal gyrus during response inhibition across response modalities. J. Cogn. Neurosci. 19, 69–80. doi: 10.1162/jocn.2007.19.1.69
Chiu, Y. C., Aron, A. R., and Verbruggen, F. (2012). Response suppression by automatic retrieval of stimulus-stop association: evidence from transcranial magnetic stimulation. J. Cogn. Neurosci. 24, 1908–1918. doi: 10.1162/jocn_a_00247
Cohen, J. R., and Poldrack, R. A. (2008). Automacity in motor sequence learning does not impair response inhibition. Psychon. Bull. Rev. 15, 108–115. doi: 10.3758/PBR.15.1.108
Dahlin, E., Nyberg, L., Backman, L., and Neely, A. S. (2008). Plasticity of executive functioning in young and older adults: immediate training gains, transfer, and long-term maintenance. Psychol. Aging 23, 720–730. doi: 10.1037/a0014296
Decety, J., Kawashima, R., Gulyas, B., and Roland, P. E. (1992). Preparation for reaching: a PET study of the participating structures in the human brain. Neuroreport 3, 761–764. doi: 10.1097/00001756-199209000-00010
Deiber, M. P., Ibanez, V., Sadato, N., and Hallett, M. (1996). Cerebral structures participating in motor preparation in humans: a positron emission tomography study. J. Neurophysiol. 75, 233–247.
Deiber, M. P., Passingham, R. E., Colebatch, J. G., Friston, K. J., Nixon, P. D., and Frackowiak, R. S. (1991). Cortical areas and the selection of movement: a study with positron emission tomography. Exp. Brain Res. 84, 393–402. doi: 10.1007/BF00231461
De Neys, W., and Everaerts, D. (2008). Developmental trends in everyday conditional reasoning: the retrieval and inhibition interplay. J. Exp. Child Psychol. 100, 252–263. doi: 10.1016/j.jecp.2008.03.003
Depue, B. E., Curran, T., and Banich, M. T. (2007). Prefrontal regions orchestrate suppression of emotional memories via a two-phase process. Science 317, 215–219. doi: 10.1126/science.1139560
Dillon, D. G., and Pizzagalli, D. A. (2007). Inhibition of action, thought, and emotion: a selective neurobiological review. Appl. Prev. Psychol. 12, 99–114. doi: 10.1016/j.appsy.2007.09.004
Di Russo, F., Taddei, F., Apnile, T., and Spinelli, D. (2006). Neural correlates of fast stimulus discrimination and response selection in top-level fencers. Neurosci. Lett. 408, 113–118. doi: 10.1016/j.neulet.2006.08.085
Donders, F. C. (1969). On the speed of mental processes. Acta Psychol. (Amst) 30, 412–431. doi: 10.1016/0001-691890065-1
Eisenberg, D. P., and Berman, K. F. (2010). Executive function, neural circuitry, and genetic mechanisms in schizophrenia. Neuropsychopharmacology 35, 258–277. doi: 10.1038/npp.2009.111
Falkenstein, M., Hoormann, J., and Hohnsbein, J. (1999). ERP components in go/nogo tasks and their relation to inhibition. Acta Psychol. (Amst) 101, 267–291. doi: 10.1016/S0001-691800008-6
Falkenstein, M., Koshlykova, N. A., Kiroj, V. N., Hoormann, J., and Hohnsbein, J. (1995). Late ERP components in visual and auditory go/nogo tasks. Electroencephalogr. Clin. Neurophysiol. 96, 36–43. doi: 10.1016/0013-469400182-K
Friedman, N. P., and Miyake, A. (2004). The relations among inhibition and interference control functions: a latent-variable analysis. J. Exp. Psychol. Gen. 133, 101–135. doi: 10.1037/0096-3445.133.1.101
Galvan, A. (2010). Neural plasticity of development and learning. Hum. Brain Mapp. 31, 879–890. doi: 10.1002/hbm.21029
Garavan, H., and Hester, R. (2007). The role of cognitive control in cocaine dependence. Neuropsychol. Rev. 17, 337–345. doi: 10.1007/s11065-007-9034-x
Guerrieri, R., Nederkoorn, C., and Jansen, A. (2008). The interaction between impulsivity and a varied food environment: its influence on food intake and overweight. Int. J. Obes. (Lond) 32, 708–714. doi: 10.1038/sj.ijo.0803770
Guerrieri, R., Nederkoorn, C., and Jansen, A. (2012). Disinhibition is easier learned than inhibition. The effects of (dis)inhibition training on food intake. Appetite 59, 96–99. doi: 10.1016/j.appet.2012.04.006
Haier, R. J., Siegel, B. V. Jr., Maclachlan, A., Soderling, E., Lottenberg, S., et al. (1992). Regional glucose metabolic changes after learning a complex visuospatial/motor task: a positron emission tomographic study. Brain Res. 570, 134–143. doi: 10.1016/0006-899390573-R
Hampshire, A., Chamberlain, S. R., Monti, M. M., Duncan, J., and Owen, A. M. (2010). The role of the right inferior frontal gyrus: inhibition and attentional control. Neuroimage 50, 1313–1319. doi: 10.1016/j.neuroimage.2009.12.109
Handley, S. J., Capon, A., Beveridge, M., Dennis, I., and Evans, J. S. B. (2004). Working memory, inhibitory control and the development of children's reasoning. Think. Reason. 10, 175–195. doi: 10.1080/13546780442000051
Hanes, D. P., Patterson, W. F. 2nd., and Schall, J. D. (1998). Role of frontal eye fields in countermanding saccades: visual, movement, and fixation activity. J. Neurophysiol. 79, 817–834.
Holroyd, C. B., Yeung, N., Coles, M. G., and Cohen, J. D. (2005). A mechanism for error detection in speeded response time tasks. J. Exp. Psychol. Gen. 134, 163–191. doi: 10.1037/0096-3445.134.2.163
Houben, K. (2011). Overcoming the urge to splurge: influencing eating behavior by manipulating inhibitory control. J. Behav. Ther. Exp. Psychiatry 42, 384–388. doi: 10.1016/j.jbtep.2011.02.008
Houben, K., Havermans, R. C., Nederkoorn, C., and Jansen, A. (2012). Beer a no-go: learning to stop responding to alcohol cues reduces alcohol intake via reduced affective associations rather than increased response inhibition. Addiction 107, 1280–1287. doi: 10.1111/j.1360-0443.2012.03827.x
Houben, K., and Jansen, A. (2011). Training inhibitory control. A recipe for resisting sweet temptations. Appetite 56, 345–349. doi: 10.1016/j.appet.2010.12.017
Houben, K., Nederkoorn, C., Wiers, R. W., and Jansen, A. (2011). Resisting temptation: decreasing alcohol-related affect and drinking behavior by training response inhibition. Drug Alcohol Depend. 116, 132–136. doi: 10.1016/j.drugalcdep.2010.12.011
Houde, O., Zago, L., Mellet, E., Moutier, S., Pineau, A., Mazoyer, B., et al. (2000). Shifting from the perceptual brain to the logical brain: the neural impact of cognitive inhibition training. J. Cogn. Neurosci. 12, 721–728. doi: 10.1162/089892900562525
Hyde, K. L., Peretz, I., and Zatorre, R. J. (2008). Evidence for the role of the right auditory cortex in fine pitch resolution. Neuropsychologia 46, 632–639. doi: 10.1016/j.neuropsychologia.2007.09.004
Jaeggi, S. M., Buschkuehl, M., Jonides, J., and Perrig, W. J. (2008). Improving fluid intelligence with training on working memory. Proc. Natl. Acad. Sci. U.S.A. 105, 6829–6833. doi: 10.1073/pnas.0801268105
Jodo, E., and Inoue, K. (1990). Effects of practice on the P300 in a go/nogo task. Electroencephalogr. Clin. Neurophysiol. 76, 249–257. doi: 10.1016/0013-469490019-G
Johnstone, S. J., Roodenrys, S., Blackman, R., Johnston, E., Loveday, K., Mantz, S., et al. (2012). Neurocognitive training for children with and without AD/HD. Atten. Defic. Hyperact. Disord. 4, 11–23. doi: 10.1007/s12402-011-0069-8
Jones, A., and Field, M. (2013). The effects of cue-specific inhibition training on alcohol consumption in heavy social drinkers. Exp. Clin. Psychopharmacol. 21, 8–16. doi: 10.1037/a0030683
Jonides, J., Schumacher, E. H., Smith, E. E., Koeppe, R. A., Awh, E., Reuter-Lorenz, P. A., et al. (1998). The role of parietal cortex in verbal working memory. J. Neurosci. 18, 5026–5034.
Kelly, A. M., and Garavan, H. (2005). Human functional neuroimaging of brain changes associated with practice. Cereb. Cortex 15, 1089–1102. doi: 10.1093/cercor/bhi005
Klingberg, T. (2010). Training and plasticity of working memory. Trends Cogn. Sci. 14, 317–324. doi: 10.1016/j.tics.2010.05.002
Knoch, D., Gianotti, L. R., Pascual-Leone, A., Treyer, V., Regard, M., Hohmann, M., et al. (2006). Disruption of right prefrontal cortex by low-frequency repetitive transcranial magnetic stimulation induces risk-taking behavior. J. Neurosci. 26, 6469–6472. doi: 10.1523/JNEUROSCI.0804-06.2006
Lappin, J. S., and Eriksen, C. W. (1966). Use of a delayed signal to stop a visual reaction-time response. J. Exp. Psychol. 72, 805–811. doi: 10.1037/h0021266
Lenartowicz, A., Verbruggen, F., Logan, G. D., and Poldrack, R. A. (2011). Inhibition-related activation in the right inferior frontal gyrus in the absence of inhibitory cues. J. Cogn. Neurosci. 23, 3388–3399. doi: 10.1162/jocn_a_00031
Li, S. C., Schmiedek, F., Huxhold, O., Rocke, C., Smith, J., and Lindenberger, U. (2008). Working memory plasticity in old age: practice gain, transfer, and maintenance. Psychol. Aging 23, 731–742. doi: 10.1037/a0014343
Logan, G. D. (1988). Automaticity, resources, and memory: theoretical controversies and practical implications. Hum. Factors 30, 583–598.
Logan, G. D. (1994). Spatial attention and the apprehension of spatial relations. J. Exp. Psychol. Hum. Percept. Perform. 20, 1015–1036. doi: 10.1037/0096-1523.20.5.1015
Logan, G. D., and Burkell, J. (1986). Dependance and independance in responding to double stimulation: a comparison of stop, change, and dual-task paradigms. J. Exp. Psychol. 12, 549–563. doi: 10.1037/0096-1523.12.4.549
Logan, G. D., Cowan, W. B., and Davis, K. A. (1984). On the ability to inhibit simple and choice reaction time responses: a model and a method. J. Exp. Psychol. Hum. Percept. Perform. 10, 276–291. doi: 10.1037/0096-1523.10.2.276
Manuel, A. L., Bernasconi, F., Murray, M. M., and Spierer, L. (2012). Spatio-temporal brain dynamics mediating post-error behavioral adjustments. J. Cogn. Neurosci. 24, 1331–1343. doi: 10.1162/jocn_a_00150
Manuel, A. L., Bernasconi, F., and Spierer, L. (2013). Plastic modifications within inhibitory control networks induced by practicing a stop-signal task: an electrical neuroimaging study. Cortex 49, 1141–1147. doi: 10.1016/j.cortex.2012.12.009
Manuel, A. L., Grivel, J., Bernasconi, F., Murray, M. M., and Spierer, L. (2010). Brain dynamics underlying training-induced improvement in suppressing inappropriate action. J. Neurosci. 30, 13670–13678. doi: 10.1523/JNEUROSCI.2064-10.2010
Marteau, T. M., Hollands, G. J., and Fletcher, P. C. (2012). Changing human behavior to prevent disease: the importance of targeting automatic processes. Science 337, 1492–1495. doi: 10.1126/science.1226918
McLoughlin, G., Albrecht, B., Banaschewski, T., Rothenberger, A., Brandeis, D., Asherson, P., et al. (2010). Electrophysiological evidence for abnormal preparatory states and inhibitory processing in adult ADHD. Behav. Brain Funct. 6, 66. doi: 10.1186/1744-9081-6-66
Mitchell, J. P., Heatherton, T. F., Kelley, W. M., Wyland, C. L., Wegner, D. M., and Neil Macrae, C. (2007). Separating sustained from transient aspects of cognitive control during thought suppression. Psychol. Sci. 18, 292–297. doi: 10.1111/j.1467-9280.2007.01891.x
Muraven, M. (2010). Building self-control strength: practicing self-control leads to improved self-control performance. J. Exp. Soc. Psychol. 46, 465–468. doi: 10.1016/j.jesp.2009.12.011
Notebaert, W., Houtman, F., Opstal, F. V., Gevers, W., Fias, W., and Verguts, T. (2009). Post-error slowing: an orienting account. Cognition 111, 275–279. doi: 10.1016/j.cognition.2009.02.002
Olesen, P. J., Westerberg, H., and Klingberg, T. (2004). Increased prefrontal and parietal activity after training of working memory. Nat. Neurosci. 7, 75–79. doi: 10.1038/nn1165
Poldrack, R. A. (2000). Imaging brain plasticity: conceptual and methodological issues–a theoretical review. Neuroimage 12, 1–13. doi: 10.1006/nimg.2000.0596
Rabbitt, P. M. (1966). Errors and error correction in choice-response tasks. J. Exp. Psychol. 71, 264–272. doi: 10.1037/h0022853
Rubia, K., Russell, T., Overmeyer, S., Brammer, M. J., Bullmore, E. T., Sharma, T., et al. (2001). Mapping motor inhibition: conjunctive brain activations across different versions of go/no-go and stop tasks. Neuroimage 13, 250–261. doi: 10.1006/nimg.2000.0685
Ruchsow, M., Groen, G., Kiefer, M., Buchheim, A., Walter, H., Martius, P., et al. (2008). Response inhibition in borderline personality disorder: event-related potentials in a go/nogo task. J. Neural Transm. 115, 127–133. doi: 10.1007/s00702-007-0819-0
Ruchsow, M., Reuter, K., Hermle, L., Ebert, D., Kiefer, M., and Falkenstein, M. (2007). Executive control in obsessive-compulsive disorder: event-related potentials in a go/nogo task. J. Neural Transm. 114, 1595–1601. doi: 10.1007/s00702-007-0779-4
Schapkin, S. A., Falkenstein, M., Marks, A., and Griefahn, B. (2007). Practice-related effects in a go-nogo task. Percept. Mot. Skills 105, 1275–1288.
Schoups, A. A., Vogels, O., and Orban, G. A. (1998). Effects of perceptual learning in orientation discrimination on orientation coding in V1. Invest. Ophtalmol. Vis. Sci. 39, 3142.
Shiffrin, R. M., and Schneider, W. (1977). Controlled and automatic human information processing. II. Perceptial learning, automatic attending, and a general theory. Psychol. Rev. 84, 127–190. doi: 10.1037/0033-295X.84.2.127
Song, Y., Ding, Y., Fan, S., and Chen, L. (2002). An event-related potential study on visual perceptual learning under short-term and long-term training conditions. Neuroreport 13, 2053–2057. doi: 10.1097/00001756-200211150-00013
Spierer, L., Murray, M. M., Tardif, E., and Clarke, S. (2008). The path to success in auditory spatial discrimination: electrical neuroimaging responses within the supratemporal plane predict performance outcome. Neuroimage 41, 493–503. doi: 10.1016/j.neuroimage.2008.02.038
Thomas, C., and Baker, C. I. (2013). Teaching an adult brain new tricks: a critical review of evidence for training-dependent structural plasticity in humans. Neuroimage 73, 225–236. doi: 10.1016/j.neuroimage.2012.03.069
Thorell, L. B., Lindqvist, S., Bergman Nutley, S., Bohlin, G., and Klingberg, T. (2009). Training and transfer effects of executive functions in preschool children. Dev. Sci. 12, 106–113. doi: 10.1111/j.1467-7687.2008.00745.x
Thorpe, S. J., and Fabre-Thorpe, M. (2001). Neuroscience. Seeking categories in the brain. Science 291, 260–263. doi: 10.1126/science.1058249
Veling, H., Aarts, H., and Papies, E. K. (2011). Using stop signals to inhibit chronic dieters' responses toward palatable foods. Behav. Res. Ther. 49, 771–780. doi: 10.1016/j.brat.2011.08.005
Verbruggen, F., Adams, R., and Chambers, C. D. (2012). Proactive motor control reduces monetary risk taking in gambling. Psychol. Sci. 23, 805–815. doi: 10.1177/0956797611434538
Verbruggen, F., and Logan, G. D. (2008). Automatic and controlled response inhibition: associative learning in the go/no-go and stop-signal paradigms. J. Exp. Psychol. Gen. 137, 649–672. doi: 10.1037/a0013170
Viskontas, I. V., Morrison, R. G., Holyoak, K. J., Hummel, J. E., and Knowlton, B. J. (2004). Relational integration, inhibition, and analogical reasoning in older adults. Psychol. Aging 19, 581–591. doi: 10.1037/0882-7974.19.4.581
Von Bastian, C. C., Langer, N., Jancke, L., and Oberauer, K. (2013). Effects of working memory training in young and old adults. Mem. Cognit. 41, 611–624. doi: 10.3758/s13421-012-0280-7
Xue, G., Aron, A. R., and Poldrack, R. A. (2008). Common neural substrates for inhibition of spoken and manual responses. Cereb. Cortex 18, 1923–1932. doi: 10.1093/cercor/bhm220
Xue, G., Poldrack, R. A., and Aron, A. R. (2006). Right inferior frontal cortex is involved in inhibiting both manual and vocal responses. Soc. Neurosci. Abstr. 32, 367–365.
Keywords: plasticity, inhibitory control, training, rehabilitation, frontal
Citation: Spierer L, Chavan CF and Manuel AL (2013) Training-induced behavioral and brain plasticity in inhibitory control. Front. Hum. Neurosci. 7:427. doi: 10.3389/fnhum.2013.00427
Received: 08 May 2013; Accepted: 15 July 2013;
Published online: 01 August 2013.
Edited by:
Russell A. Poldrack, University of Texas, USAReviewed by:
Russell A. Poldrack, University of Texas, USAFrederick Verbruggen, University of Exeter, UK
Copyright © 2013 Spierer, Chavan and Manuel. This is an open-access article distributed under the terms of the Creative Commons Attribution License (CC BY). The use, distribution or reproduction in other forums is permitted, provided the original author(s) or licensor are credited and that the original publication in this journal is cited, in accordance with accepted academic practice. No use, distribution or reproduction is permitted which does not comply with these terms.
*Correspondence: Lucas Spierer, Neurology Unit, Medicine Department, University of Fribourg, PER 09, Chemin du Musée 5, CH-1700 Fribourg, Switzerland e-mail: lucas.spierer@unifr.ch