- 1 Department of Psychology, The Ohio State University, Columbus, OH, USA
- 2 Beckman Institute for Advanced Science and Technology and Department of Psychology, University of Illinois at Urbana-Champaign, Champaign, IL, USA
- 3 Department of Psychology, University of Pittsburgh, Pittsburgh, PA, USA
- 4 Department of Kinesiology and Community Health, University of Illinois at Urbana-Champaign, Champaign, IL, USA
A growing body of literature provides evidence for the prophylactic influence of cardiorespiratory fitness on cognitive decline in older adults. This study examined the association between cardiorespiratory fitness and recruitment of the neural circuits involved in an attentional control task in a group of healthy older adults. Employing a version of the Stroop task, we examined whether higher levels of cardiorespiratory fitness were associated with an increase in activation in cortical regions responsible for imposing attentional control along with an up-regulation of activity in sensory brain regions that process task-relevant representations. Higher fitness levels were associated with better behavioral performance and an increase in the recruitment of prefrontal and parietal cortices in the most challenging condition, thus providing evidence that cardiorespiratory fitness is associated with an increase in the recruitment of the anterior processing regions. There was a top-down modulation of extrastriate visual areas that process both task-relevant and task-irrelevant attributes relative to the baseline. However, fitness was not associated with differential activation in the posterior processing regions, suggesting that fitness enhances attentional function by primarily influencing the neural circuitry of anterior cortical regions. This study provides novel evidence of a differential association of fitness with anterior and posterior brain regions, shedding further light onto the neural changes accompanying cardiorespiratory fitness.
Introduction
Healthy lifestyle factors are increasingly being recognized to play a critical role in the maintenance of cognitive and brain functioning through the adult lifespan (Hertzog et al., 2009). The recognition of a positive association between fitness and several aspects of cognitive functioning in healthy older adults (Colcombe et al., 2003, 2006; Erickson et al., 2009b; Voss et al., 2010), individuals with neurodegenerative disorders (Prakash et al., 2007, 2010a); children (Chaddock et al., 2010); and many other populations has led to an escalation in the focus of exercise-cognition as a topic of research in the last decade. We, in our laboratory, have systematically been examining the influence of aerobic exercise on cognitive functioning of older adults (Kramer et al., 1999; Colcombe and Kramer, 2003; Colcombe et al., 2004, 2006; Erickson et al., 2009b) and have provided evidence for a significant improvement in the cognitive abilities of healthy older adults who have participated in a 6-month aerobic exercise training intervention, compared to a non-aerobic control group. We have evidence of an increase in fitness being associated with increased volumes in the frontal and temporal cortices (Colcombe et al., 2003, 2006), and more recent, specific evidence of increased volume in the hippocampi of older adults (Erickson et al., 2009b). We have also provided evidence that increased fitness levels and participation in aerobic exercise training is associated with greater neural recruitment of regions involved in executive function including the prefrontal and the parietal cortices (Colcombe et al., 2004); and increased functional connectivity between many regions of the default-mode network (Voss et al., 2010). Extending this work, we are now interested in examining the additional changes in neural recruitment that may accompany higher levels of fitness, thereby positively influencing cognitive performance.
In this study, our goal was to examine whether higher levels of cardiorespiratory fitness are associated with a differential recruitment of the anterior and posterior processing regions during performance on a modified version of the Stroop task. The Stroop task is particularly interesting because of its involvement of both the prefrontal regions in exerting top-down control and the subsequent recruitment of the posterior processing regions in order to perform the task (Bench et al., 1993; Bush et al., 1998; Brown et al., 1999; Banich et al., 2000, 2001; Milham et al., 2001; Erickson et al., 2009a). To examine associations with posterior processing regions, we localized ventral visual areas that were sensitive to color processing and word processing in older adults and examined whether activation in these stimuli-sensitive regions in older adults during the Stroop task was associated with increasing levels of cardiorespiratory fitness, in a top-down modulation fashion.
To examine association with anterior, prefrontal processing regions, we used a modification of the original color-word Stroop task. Similar to previous versions of the Stroop task that have been employed in many neuroimaging studies (Banich et al., 2000, 2001; Milham et al., 2001; Langenecker et al., 2004), participants were asked to respond to the color of the ink in which the word was printed, while ignoring the meaning of the word. In contrast to other tasks, we presented our participants with two versions of the incongruent trials (incongruent-eligible and incongruent-ineligible), in addition to the congruent and neutral trials (for a detailed description, see the Materials and Methods section). On incongruent-eligible trials, the incongruent color-word was part of a set of colors that the participant could respond with (red, green, or purple), printed in an incongruent ink-color (e.g., the word RED printed in green ink, when red, green, and purple are the possible ink-colors). These trials thus involved conflict at both the response and non-response levels (Milham et al., 2001; Liu et al., 2006; Erickson et al., 2009a; Prakash et al., 2010b). An incongruent-ineligible stimulus, in contrast, was any color-word other than red, green, or purple printed in an incongruent color (e.g., the word BLUE printed in green ink, when red, green, and purple are the possible ink-colors). For both these trials, the participants were asked to make responses (see Figure 1). The incongruent-ineligible condition, because of a mismatch of the identity of the word with the color in which it was printed, produced increased interference relative to the neutral and the congruent conditions, however, was less challenging than the incongruent-eligible condition, in which the word was one of the possible responses that the participant could make.
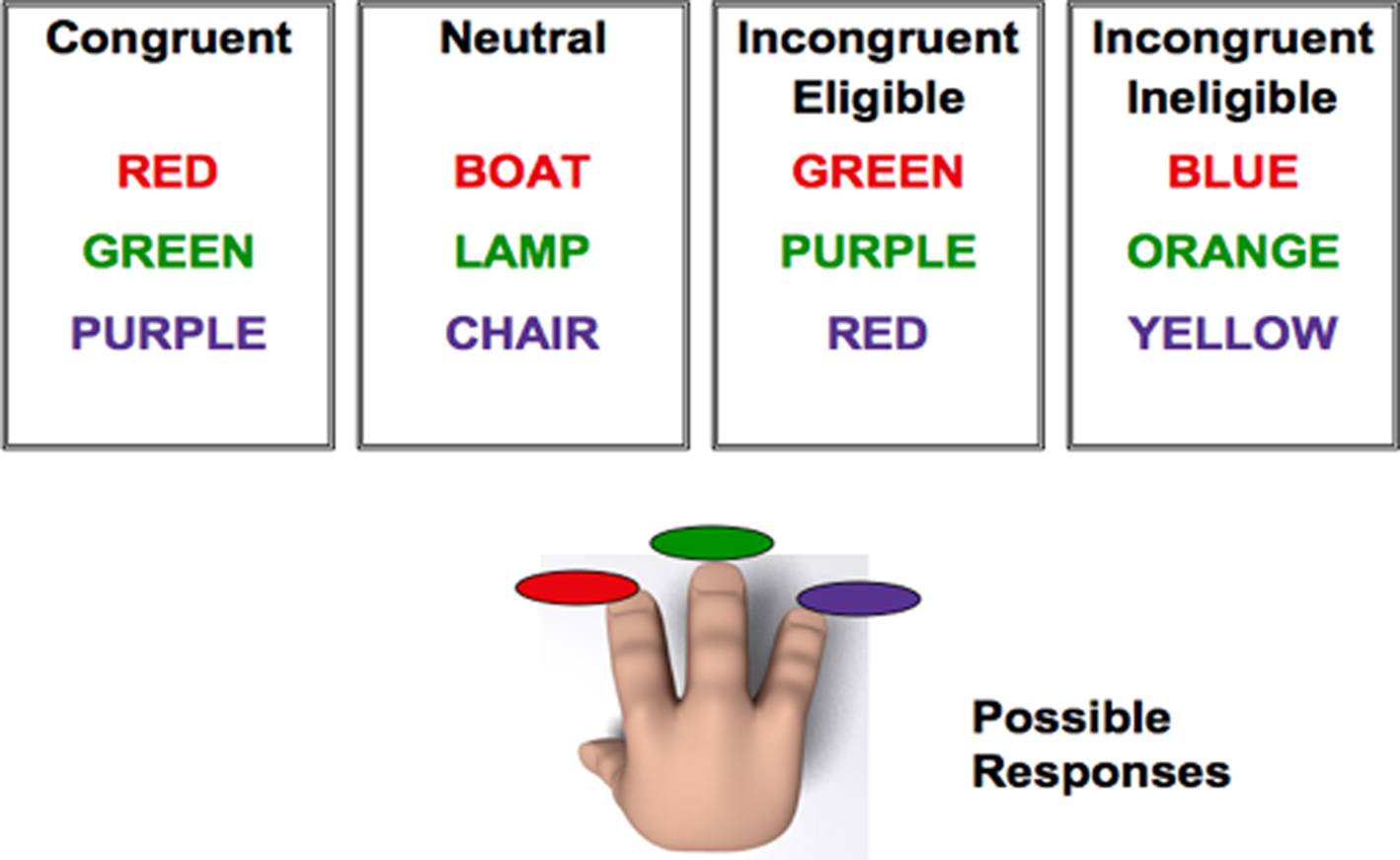
Figure 1. Pictorial representation of the modified version of the Stroop task used in the study. Participants could only respond to three ink-colors: red, green, or purple. For the incongruent-eligible condition (third box) the word was one of the ink-colors that the participant could respond with (red, green, or purple) but printed in an incongruent ink-color (such as GREEN printed in red ink). For the incongruent-ineligible condition (fourth box), the word could be any color-word other than red, green, or purple printed in an incongruent ink-color (such as the word BLUE printed in red ink).Reprinted from Prakash et al. (2010a), Copyright (2010), with permission from Elsevier.
In the two recent studies in which we employed this task (Erickson et al., 2009a; Prakash et al., 2010a), we demonstrated that both older and younger adults find the incongruent-eligible condition to be more challenging than the incongruent-ineligible condition. In addition, we found greater right DLPFC recruitment in the incongruent-eligible condition for younger adults (Erickson et al., 2009a; Prakash et al., 2010b; for similar findings also see Milham et al., 2001), suggesting increased cortical utilization in younger adults in the presence of increasing cognitive demands. Such an amplification in the recruitment of task-related areas in response to increasing task demands can broadly be conceptualized as evidence for neuronal flexibility, such that younger adults in the presence of increasing levels of task complexity are able to up-regulate the cortical resources resulting in superior performance (DiGirolamo et al., 2001; Erickson et al., 2009a). In contrast, older adults demonstrated greater recruitment of neural resources at lower levels of conflict, leaving fewer resources for the more challenging condition. Thus, older adults at lower task demands demonstrate the additional recruitment of neural resources seen in younger adults in response to challenging task demands (Prakash et al., 2010a). These results are consistent with findings of other studies (DiGirolamo et al., 2001; Milham et al., 2002; Reuter-Lorenz and Mikels, 2006) and suggest that while older adults recruit the PFC regions to compensate for decreases in other regions (Davis et al., 2008), this additional recruitment might come with a cost of reduced capabilities to enhance cortical resources in response to increasing levels of task demands, resulting in a decline in cognitive performance.
Thus, one possible means through which cardiorespiratory fitness might benefit task performance would be to increase recruitment of cortical resources in task-related regions in response to increases in task demands, rather than a general increase in PFC recruitment across all task conditions. In this study, we examined the association between cardiorespiratory fitness and prefrontal recruitment during the two incongruent conditions in a group of healthy older adults. A positive association between fitness and increase in the neural recruitment between the two incongruent conditions (eligible > ineligible) in the prefrontal cortices would provide evidence for an enhanced capacity to up-regulate regions of the prefrontal cortex with increasing levels of fitness. However, if fitness were associated with increased activation of the PFC in all conditions of the Stroop task, it would suggest a more general increase in the utilization of the PFC with increasing levels of aerobic fitness.
Materials and Methods
Research Participants
Seventy community-dwelling (mean age = 65; SD = 5 years; 46 males and 24 females) older adults were recruited to participate in this study. Participants were recruited via advertisements in the local media, promotional flyers and announcements to local senior citizen agencies. All participants were required to satisfy a number of inclusionary criteria: between 60 and 75 years of age, capable of performing physical exercise, personal physician’s consent to participate in exercise testing, successful completion of graded maximal exercise test without evidence of cardiac abnormalities, a score greater than 51 on the mMMSE (maximum score = 57; Stern et al., 1987), corrected (near and far) acuity 20/40 or better, a score < 4 on the Geriatric Depression Scale (GDS, Sheikh and Yesavage, 1986), no history of psychiatric or neurological conditions, no implanted devices that could interfere with the MR signal, and no other contraindications for participating in an MR environment. Participants were also administered the two verbal subtests of the Kaufman Brief Intelligence Test (K-BIT) to assess crystallized intelligence (Kaufman and Kaufman, 1990). The University of Illinois Institutional Review Board approved the study and all participants provided informed consent.
Cardiorespiratory Fitness Assessment
Two measures of cardiorespiratory fitness were employed in order to assess the aerobic capacity of older adults. All participants had to be cleared by their personal physician to participate in the graded exercise test that constituted walking on a treadmill and for the subsequent Rockport VO2 testing. For the maximal graded exercise test, participants were asked to walk at a pace which was slightly faster (and therefore challenging) than their normal walking speed. This typically ranged between 2.5 and 3.8 mph, with 90% of the sample walking at 2.5 mph or above. This testing speed was to be reached within the last 30 s of the 3-min warm-up and thereafter stayed the same for the remainder of the test. The grade of the treadmill began at 0% and stayed there throughout the 3-min warm-up and for the first 2-min stage of the test. The second stage (minutes 3 and 4 of the test) was performed at 2% grade and the incline was increased every 2 min by 2–3% depending upon the subject’s respiratory exchange ratio (RER). If the participant’s RER was ≥1.0, the increase remained at 2%. If RER was <1.0 the increase was 3%. Using these same guidelines, the participant’s RER was reevaluated during each stage to determine the degree of the incline. Measurements of oxygen uptake, heart rate, and blood pressure were continuously monitored by a cardiologist, nurse, and exercise test technician. Oxygen uptake (VO2) was measured from expired air samples taken at 30-s intervals until a peak VO2 was attained at the point of test termination based on participants’ reaching volitional exhaustion. This represented 100% of the sample. Examination of ratings of perceived exertion (RPE) as assessed by the Borg’s (1998) RPE Scale at test completion indicated that 85% of participants reported an RPE of 17 (very hard) or greater (i.e., maximal intensity) at test termination. VO2 peak was defined as the highest recorded VO2 value when two of three criteria were satisfied: (1) a plateau in VO2 peak between two or more workloads; (2) a respiratory exchange ratio >1.00; and (3) a heart rate equivalent to their age predicted maximum (i.e., 220 − age). Average VO2 peak of our participants was 21.91 mL/kg (SD = 4.38). We also employed a sub-maximal field test, the Rockport Fitness Walking Test (Kline et al., 1987) to assess aerobic endurance capacity of our participants. The average VO2 – estimated from the Rockport test was 19.96 for our participants (SD = 6.80). The two measures of fitness were correlated at 0.57 (p < 0.0001), thus suggesting a high degree of coherence between the two measures. For later analyses with cognitive data, we calculated a z-composite score of the two VO2 peak measures (zFIT) by averaging across both VO2 peak and VO2 – estimated from the Rockport test. The creation of a composite measure from multiple measures of the same construct serves to reduce error variance.
Task Design
Subjects were scanned in a 3 Tesla MRI system while they performed a modified version of the Stroop color-word test (Milham et al., 2001; Erickson et al., 2009a; Prakash et al., 2010a). Participants were instructed to respond to the color in which the word on screen was printed rather than responding to the semantic meaning of the word. In addition to the neutral (e.g., the word LAMP printed in red ink) and the congruent (e.g., the word RED printed in red ink) conditions, we had two types of incongruent conditions (incongruent-eligible and incongruent-ineligible) enabling us to study the effect of increases in conflict on behavioral performance and brain processes in older adults. The paradigm was a three-choice manual response task, in which participants were asked to respond to the three ink-colors (red, green, or purple) using their right hand. An incongruent-eligible stimulus was one of the words from the set of colors that the participant could respond with (red, green, or purple) printed in an incongruent ink-color (e.g., the word RED printed in green ink). An incongruent-ineligible stimulus, in contrast, was any color-word other than red, green, or purple printed in an incongruent color (e.g., the word BLUE printed in green ink). For both trial types, participants were asked to make responses. The main difference between the two incongruent conditions was that in the eligible condition the actual color-word was a part of the response set with which the participant could respond and resulted in greater response conflict. In the ineligible trials, the color-word was not a part of the response set (Figure 1).
A total of 144 stimuli (36 of each type) were presented to each participant for a period of 1 s per trial with a 1.5-s response window and a 3-s stimulus-onset asynchrony (SOA). A crosshair (+) was presented on the screen during all inter-stimulus intervals. We employed an event-related stimulus design with a 40% jitter, such that the timing between trials varied, in order to optimize the stimulus sequence and timing. Each stimulus type was first-order counterbalanced across the entire run, which lasted for 9 min. Stimulus sequence and timing were generated with optseq21 (see also Dale 1999).
To localize color-sensitive and word-sensitive regions in the ventral visual cortex, subjects’ performed two brief localizer scans. For localizing color-sensitive regions, we presented 30-s contrast-matched, flashing black and white and color checkerboards to our participants followed by a 30-s fixation cross. The order of the blocks was BW, FIX, COL, FIX and was presented twice to each participant. In order to localize word-sensitive regions, we presented our participants with 30-s length-matched blocks of words (e.g., COURT), strings (e.g., ETHYNL), and non-words (e.g., PEETH), followed by a 30-s fixation cross. The order of the blocks was WORD, FIX, STRING, FIX, NON-WORD, FIX and was again presented twice to each participant.
fMRI Acquisition and Processing
Participants were scanned in a 3T Siemens Allegra head-only scanner. All stimuli were presented using MRI-safe fiber optic goggles (Resonance Technologies, Inc.). T2* weighted images were acquired using a fast echo-planar imaging (EPI) sequence (64 × 64 matrix, 4-mm slice thickness, TR = 1500 ms, TE = 26 ms, flip angle = 60°). A total of 380 volumes were acquired for the Stroop task, 150 volumes for the color localizer scan, and 220 volumes for the word localizer scan. Anatomical, T1-weighted images were acquired using a 3D MPRAGE (Magnetization Prepared Rapid Gradient Echo Imaging) protocol with 144 contiguous axial slices, collected in ascending fashion parallel to the anterior and posterior commissures, echo time (TE) = 3.87 ms, repetition time (TR) = 1800 ms, field of view (FOV) = 256 mm, acquisition matrix 192 mm × 192 mm, slice thickness = 1.3 mm, and flip angle = 8°. All functional images were motion corrected using a rigid-body algorithm in MCFLIRT (Jenkinson, 2003), and temporally smoothed with a Gaussian high-pass (Stroop task = 80 s cut-off; localizer scans = 120 s cut-off) filter. Spatial smoothing was done with a 7-mm (full width at half maximum, FWHM) 3-dimensional Gaussian kernel. Following this, all high-resolution T1-weigthed images were skull stripped using a robust deformable brain extraction technique (BET, Smith, 2002). These skull-stripped images for each participant were spatially registered using a 12-parameter affine transformation to a study-specific template in stereotaxic space that was specifically created for the study in order to avoid systematic registration error. This was done by: (a) warping each participant’s high-resolution scan to MNI space; (b) creating an average of these registered images; and (c) spatially smoothing the average image with a 10-mm (FWHM) Gaussian kernel. This study-specific template was subsequently used for spatial registration of the fMRI data.
Behavioral Analyses
The behavioral data [both reaction time (RT) and error rates] were analyzed using one-way analysis of variances (ANOVAs). Each ANOVA had four levels (congruent, neutral, incongruent-eligible, and incongruent-ineligible). We then used partial correlations (pr) in order to examine the association between cardiorespiratory fitness and behavioral measures (RT and error rates) for each of the trial types (congruent, neutral, response-eligible, and response-ineligible), after removing variance associated with age, gender, education, and K-BIT (Verbal). We included gender as a covariate in all our analyses examining the effects of cardiorespiratory fitness as there have been a number of studies that have showed that males have higher levels of fitness in comparison to females (Meyer et al., 2004; Lotscher et al., 2007) and given that there was a disproportionate representation of females in our sample, we did not want our results to be driven by the effects of gender. Also, given the wide age range of our elderly participants (60–75 years), we included age as a covariate in all our analyses.
fMRI Univariate Analyses
Stroop task
Following pre-processing, the functional neuroimaging data collected during the presentation of the Stroop task, were convolved with a double-gamma function to model the response for each condition. Only correct trials were included in the model. This first-level analysis, done separately for each participant, resulted in voxel-wise parameter estimate maps for the entire brain for each condition and for the direct comparison between the conditions (incongruent-eligible > neutral, incongruent-ineligible > neutral, eligible > ineligible, and ineligible > eligible). These parameter estimate maps and variance maps were then forwarded to a whole-head second level analysis whereby inter-participant variability was treated as a random variable. In order to examine association of fitness with cortical recruitment during the Stroop task, independent of age, gender, education, verbal intelligence, and gray matter volume, we included our main variable of interest, cardiorespiratory fitness, along with covariates of no interest – age, gender, education, verbal intelligence, and gray matter volume in the model, thus treating cardiorespiratory fitness as a continuous variable. This was done in order to identify cortical regions that contained variance which could be explained by our variable of interest, that is, cardiorespiratory fitness, after accounting for variance explained by the demographic variables. Given that prior studies have demonstrated a relationship between fitness levels and volume of prefrontal regions (Colcombe et al., 2003, 2006) in older adults, in this study, we wanted to isolate the variance associated with the processing capacity of these regions unconfounded by differences in volume. To correct for the confound of anatomical differences which may manifest itself as functional activations in between-subject comparisons, we inserted on a voxel-wise basis, the 3D gray matter partial volume information for each subject at the higher-level analysis as a covariate. This analysis resulted in functional z-stat maps that were independent of anatomical differences and likely represented true functional differences. This higher-level analysis resulted in three voxel-wise parameter estimate maps of interest for each condition – one representing the group mean, the second representing cortical regions that showed a positive association with cardiorespiratory fitness and the third map displaying cortical regions demonstrating a negative relationship with cardiorespiratory fitness. Given our aims, we were primarily interested in examining whether higher levels of fitness in our aging sample would be associated with a greater difference in the activation patterns of the two incongruent conditions. That is, our main contrast of interest was the incongruent-eligible > incongruent-ineligible condition. In order to ensure that the cortical areas demonstrating an increased activation in this contrast truly represented cortical activation and not deactivation, we masked the parameter estimate maps of this contrast with the parameter estimate map of the incongruent-eligible condition. This was done primarily to remove any cortical regions that demonstrated less deactivation in the incongruent-eligible condition relative to the incongruent-ineligible condition rather than more activation. All Z statistic images were thresholded using clusters determined by a voxel-wise threshold of z > 2.33 (p < 0.01) and a corrected cluster-wise threshold of p < 0.05.
Localizer tasks
Localizer scans were presented to the participants in order to localize areas of the ventral visual cortex that were sensitive to the respective stimuli. Each participant’s hemodynamic response function was modeled for task-dependent change using a double-gamma convolution. This first-level analysis resulted in voxel-wise parameter estimate maps for the entire brain for each condition and for the direct comparison between the conditions (color > b/w AND word > string + non-word). These parameter estimate maps and variance maps were then forwarded to a whole-head second level analysis whereby inter-participant variability was treated as a random variable.
In order to localize color areas, the linear contrast of color > black/white checkerboard was employed. This resulted in a bilateral area of activation in the ventral visual cortex that was significantly more active to color checkerboards than to black and white checkerboards or to words, strings or non-words (see Figure 2). This region of interest (ROI) was thus found to be sensitive to color processing, and was used as a mask to locate subject-specific peaks in functional space. That is, for each of the participants in the study, we took a local maxima defined in functional space in this ROI and created a 10-mm sphere around that peak in order to obtain a participant-specific color-sensitive area (CSA). Similarly, to localize a word-processing region, we employed the linear contrast of word > string + non-word that resulted in a cluster of activation spanning the left middle and inferior temporal cortices with a peak in the temporal fusiform cortex (MNI co-ordinates: −40 −38 −20). This region is similar to the regions known to be involved in processing of word stimuli (Cohen et al., 2000; Jobard et al., 2003). This cluster too was converted to a binary mask, to define a local maximum for each participant in functional space. Local peaks were subsequently used to make subject-specific word-sensitive ROI’s (WSA). Both the word-sensitive area (WSA) and the CSA for each participant were first used to extract mean percent signal change in conditions of the localizer tasks (color checkerboard, black and white checkerboard, word, string, and non-words). We then used these regions as ROIs in the Stroop task to investigate the modulation of these regions during varying levels of attentional control.
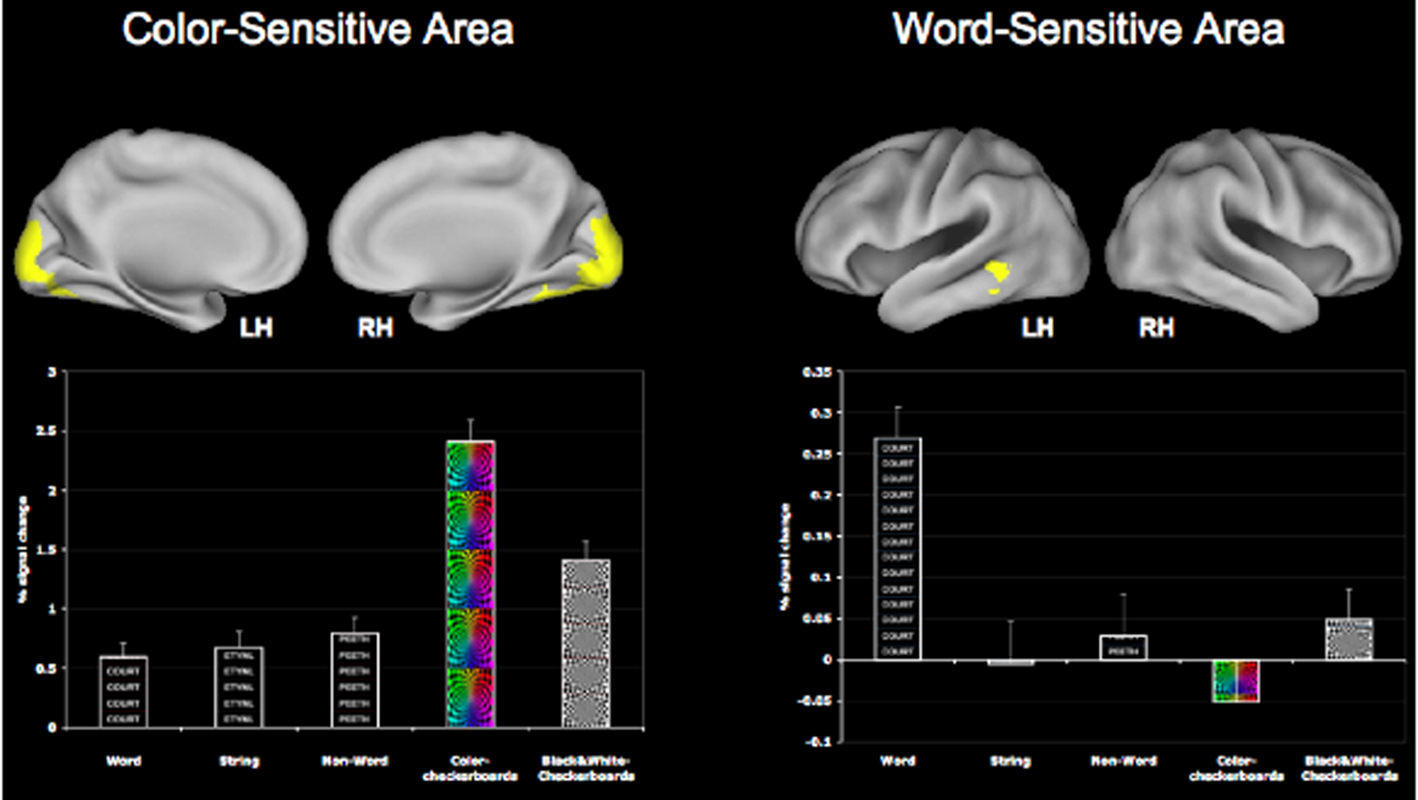
Figure 2. Greater sensitivity of the CSA and the WSA for color and word stimuli respectively. The CSA was identified using the contrast of COL > B/W checkerboard, while the WSA was identified using the contrast of WORD > STRING + NON-WORD.
Modulation of the CSA and WSA during Stroop task performance
To investigate the modulation of the ventral visual cortical areas during the Stroop task, we used the ROI’s generated from the localizer scans (both CSA and WSA) to examine activation in the CSA and WSA during performance on the Stroop task. In other words, we applied CSA and WSA to the individual parameter estimates of the congruent, neutral, incongruent-eligible, and incongruent-ineligible trials and then extracted mean percent signal change from these ROI’s during all four Stroop conditions (congruent, neutral, incongruent-eligible, and incongruent-ineligible). The percent signal change extracted for these ROI’s was used to examine association with behavioral data and cardiorespiratory fitness using partial correlations, controlling for the effects of age, gender, education, and verbal intelligence.
The neuroimaging data were analyzed using a statistical parametric approach using FSL version 4.12 and FEAT (fMRI Expert Analysis Tool) Version 5.98. Mixed-effects analysis was performed using FLAME (FMRIB’s Local Analysis of Mixed Effects, Beckmann et al., 2003; Woolrich et al., 2004). SPSS 16.0 was used for further statistical analyses.
Rendering
All cortical renderings were performed using the Caret Software3. Group statistical maps were mapped onto the Probabilistic Average Landmark and Surface-based (PALS) atlas using the multi-fiducial mapping technique (Van Essen, 2005).
Results
Behavioral Results
Reaction time
Reaction time and accuracy rates of all participants were recorded while they performed the Stroop task in the scanner. For RT analyses, only correct trials were included. Table 1 presents the mean RT and accuracy data for all conditions of the Stroop task. An ANOVA showed that the effect of condition was significant [F(3,276) = 14.169, p < 0.01]. Post hoc paired t-tests showed a significant difference between the incongruent-eligible condition and the congruent, neutral and the incongruent-ineligible conditions (p < 0.01, for all t-tests). Participants thus responded more slowly on the eligible trials as compared to the ineligible trials. Therefore, these results suggest that the incongruent-eligible condition involving conflict at the response and non-response levels was the most challenging condition requiring greater attentional control as compared to the incongruent-ineligible condition.
In order to examine the association of cardiorespiratory fitness, we conducted a series of partial correlations between fitness and RT data on each of the conditions of the Stroop task, after removing variance associated with age, gender, education, and verbal intelligence. Consistent with our previous research (Colcombe and Kramer, 2003; Colcombe et al., 2004), we found that higher levels of fitness were associated with faster RT on the most challenging condition of the Stroop task, the incongruent-eligible condition (pr = −0.20, p < 0.05, one-tailed).
Error rates
Similar to the RT data, we first examined the main effect of trial type on the error rates in the Stroop task using a one-way ANOVA. We found a main effect of trial type on error rates [F(3, 276) = 9.852, p < 0.01]. Post hoc analyses revealed that the incongruent-eligible and the incongruent-ineligible condition differed significantly from one-another and from the congruent and the neutral conditions (p < 0.01 for all t-tests). Participants made more errors on the incongruent-eligible trials as compared to the incongruent-ineligible trials (Table 1).
To examine fitness effects, we again employed partial correlations and found that fitness was differentially associated with error rates in the incongruent-eligible condition, after removing variance associated with age, gender, education, and verbal intelligence (pr = −0.289, p < 0.01, one-tailed). The correlations with other conditions of the Stroop task were not significant. Taken together, both the RT and accuracy data suggests that older adults performed worse on the incongruent-eligible trials compared to the less demanding task conditions. Our results also provide evidence for an association of cardiorespiratory fitness and cognitive improvements in the most challenging condition.
Univariate Results
Cardiorespiratory fitness and recruitment of anterior processing regions
Consistent with our previous work with this paradigm examining differential recruitment of cortical regions in response to the incongruent-eligible trials relative to the incongruent-ineligible trials (Prakash et al., 2010a), we found that older adults demonstrated an inability to up-regulate cortical resources in response to the more demanding condition of the Stroop task (Figure 3). Table 2 lists the cortical areas that were recruited by the elderly during the two incongruent conditions greater than the neutral condition. As can be seen from the tables as well as Figure 3, the cortical areas recruited in the two incongruent conditions relative to neutral trials were similar for the older adults. A direct contrast between the two conditions did not yield any significant activation.
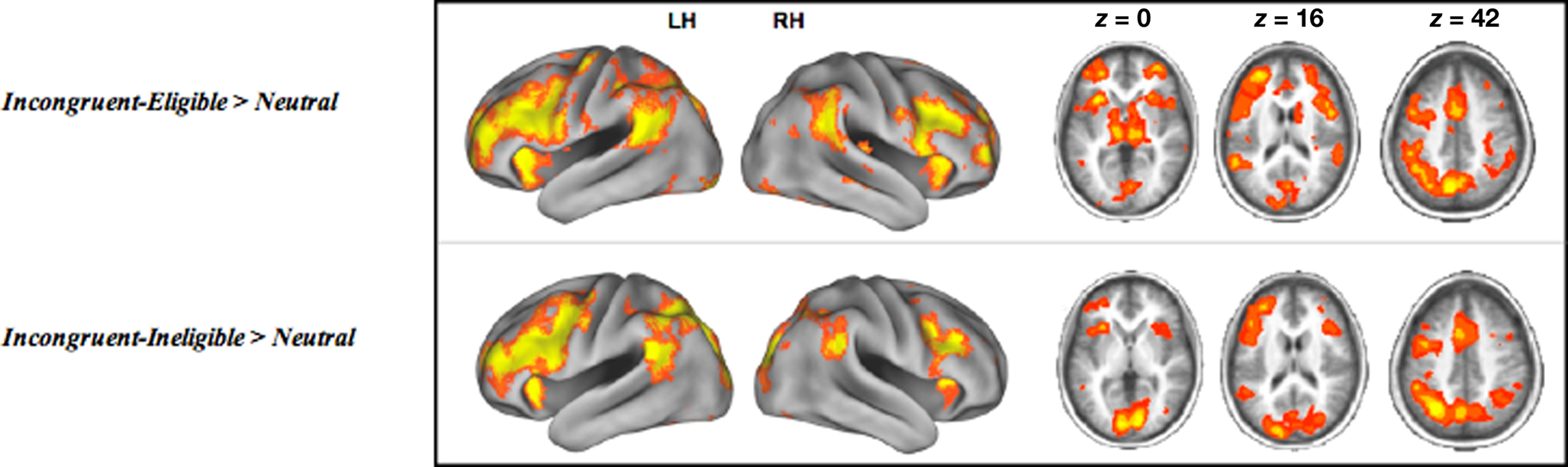
Figure 3. Cortical regions active during the incongruent-eligible > neutral contrast and incongruent-ineligible > neutral contrast. All axial slices are presented in neurological orientation (L = L, R = R).
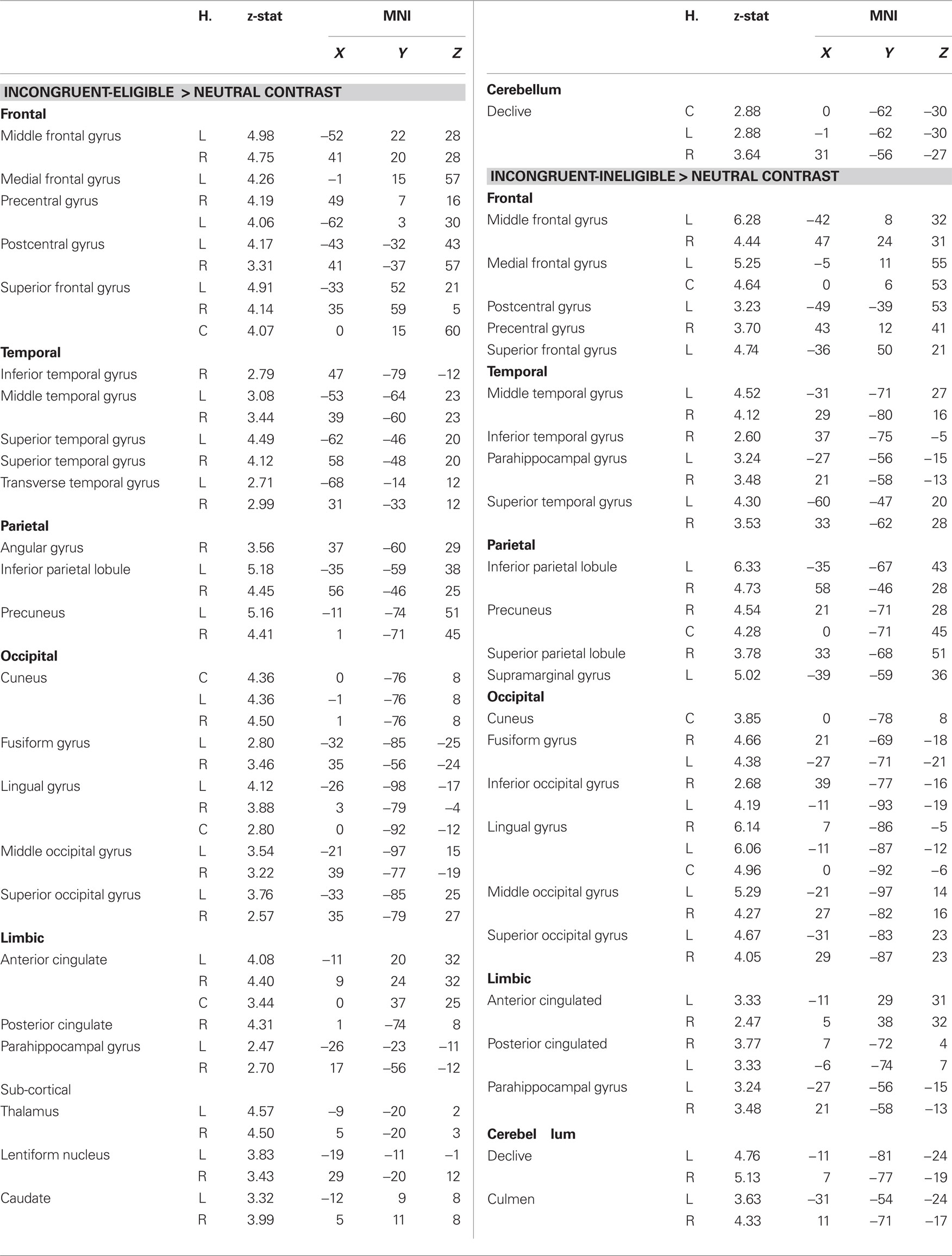
Table 2. Cortical regions activated during the incongruent-eligible > neutral contrast and incongruent-ineligible > neutral contrast.
Increasing levels of cardiorespiratory fitness, however, were associated with a greater difference in cortical recruitment between the two incongruent conditions as demonstrated by a positive association between the cortical regions displayed in Figure 4 and higher levels of fitness. We found that a contrast of eligible > ineligible trials resulted in greater magnitude of activation in the bilateral prefrontal cortices, including the middle and inferior frontal gyri, along with the left superior parietal lobule for higher levels of fitness, providing evidence for the fact that cardiorespiratory fitness is associated with greater recruitment of PFC and parietal resources in response to increases in task demands in older adults (Table 3; Figure 4). The increased recruitment seen in the contrast of the two incongruent conditions (eligible > ineligible) with higher levels of fitness was driven by the greater recruitment in the incongruent-eligible condition and less recruitment in the incongruent-ineligible condition.
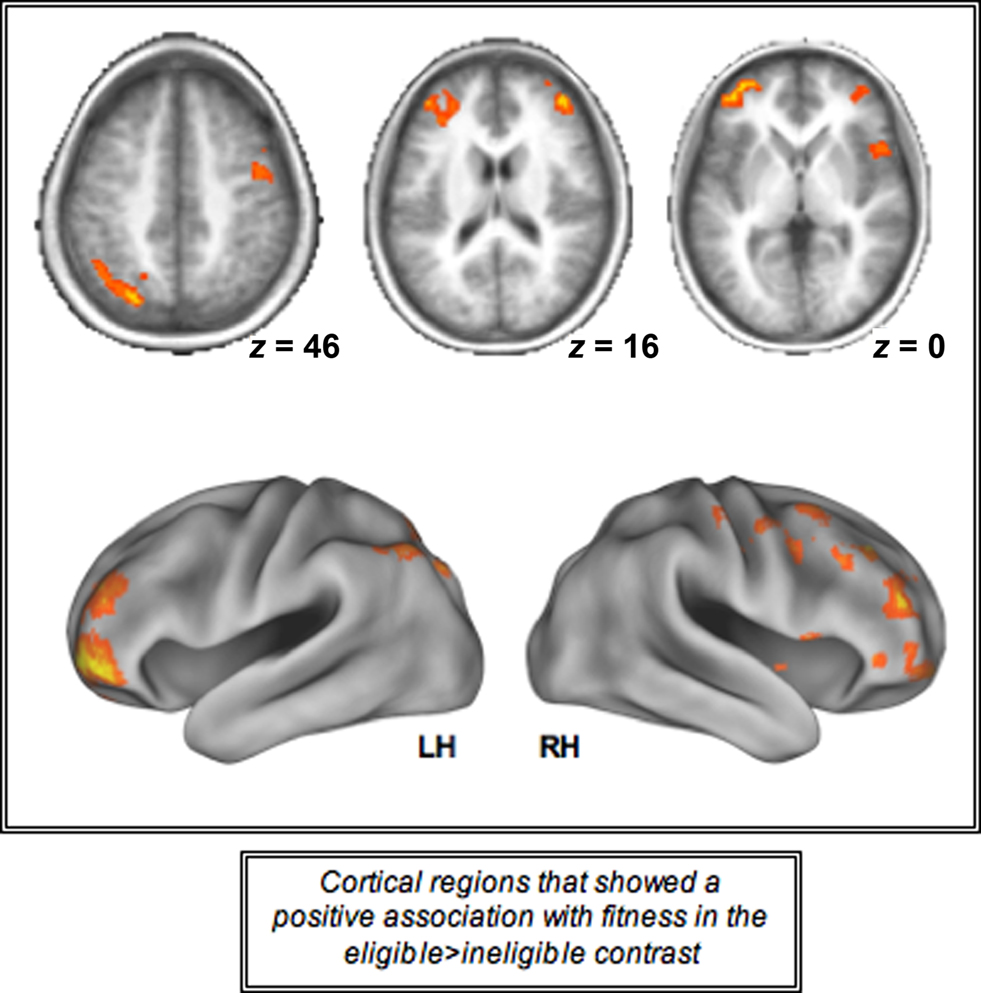
Figure 4. Cortical regions activated during the incongruent-eligible > incongruent-ineligible contrast that showed a positive association with cardiorespiratory fitness. All axial slices are presented in neurological orientation (L = L, R = R).
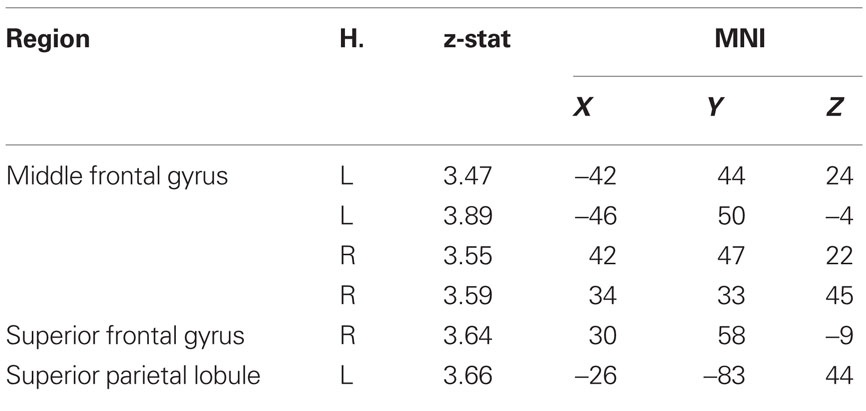
Table 3. Local maxima of cortical regions identified during the incongruent-eligible > incongruent-ineligible contrast that showed a positive association with cardiorespiratory fitness.
Modulation of the ventral visual areas during the Stroop task
One of the other primary goals of this study was to examine the modulation of the posterior regions during the Stroop task and its subsequent association with fitness. For this, we localized areas of the ventral visual cortex that were sensitive to color and word stimuli separately. The contrast of color > b/w resulted in a bilateral cluster of activation in the occipital lobule, with a peak in the left occipital fusiform gyrus. Similarly, for the contrast of word > string + non-word, we found a cluster in the left middle temporal cortex that demonstrated greater sensitivity to words than other stimuli (Figure 2). We then took subject peaks in these clusters, to create a 10-mm ROI for each participant in order to independently identify regions of maximum sensitivity for the color and word stimuli. Using paired t-tests, we found the CSA to be more sensitive to color checkerboards than to black/white checkerboards, words, strings or non-words (p < 0.01), while the WSA was more sensitive to words than any of the other stimuli used in the localizer scans (p < 0.01).
To examine modulation of the color and word-sensitive regions during the Stroop task, we used these regions as ROI’s and extracted percent signal change in all four conditions of the Stroop task. There were two main findings from this set of analyses. Firstly, using paired samples t-tests we found increased activation in the CSA relative to the WSA for all conditions of the Stroop task (p < 0.01). Secondly, we also found that older adults demonstrated a modulation of both the color- and word-sensitive regions for all conditions of the Stroop task relative to baseline (Figure 5). These results thus suggest that presentation of embedded stimuli, such as the ones employed in the Stroop task, result in a modulation of both the task-relevant and the task-irrelevant attribute. However, we did not find an increasing modulation of the CSA with respect to increasing attentional demands. Models of selective attention (Lavie et al., 2004) predict that with increasing conflict, areas of the ventral visual cortex responsible for processing both task-relevant and task-irrelevant dimensions show selective enhancement. However, in our study, older adults failed to show such a selective enhancement of the CSA with increasing cognitive load, though the WSA did show greater magnitude of activation for the incongruent conditions relative to the congruent and the neutral condition (Figure 5).
Interestingly, we found that cardiorespiratory fitness was not associated with increased or decreased activation in the CSA and the WSA during any of the conditions of the Stroop task. Thus, fitness was not associated with a greater recruitment of the ventral visual areas during performance on the Stroop task.
Discussion
Previous findings have provided evidence of greater neuronal plasticity in older adults with higher levels of cardiorespiratory fitness (Colcombe et al., 2004, 2006; Erickson et al., 2009b). In the present study, we were interested in determining whether fitness was differentially associated with increased activation in the prefrontal and parietal cortices and the ventral visual areas, two broad regions known to play a vital role in top-down control. The process of top-down modulation underlies much of the goal-directed activity in which we engage (Frith, 2001; Bar, 2003) and works primarily by selectively focusing attention on relevant stimuli and filtering out distracting task-irrelevant stimuli. Evidence from single-cell physiology, functional neuroimaging, and EEG data, suggests simultaneous activation of the prefrontal, parietal, and specialized posterior cortical regions during top-down modulation (Corbetta et al., 1990; Barcelo et al., 2000; Kastner and Ungerleider, 2001; Pessoa et al., 2003). Further, multivariate analyses, generating functional and effective connectivity maps of interacting brain regions, also provides evidence for the correlated activation patterns between the prefrontal cortices and the ventral visual areas (Rissman et al., 2004; Gazzaley et al., 2007). In the context of a modified version of a Stroop task, we report that higher levels of cardiorespiratory fitness were associated with increased recruitment of the anterior, and not the posterior processing regions. Further, the increase in neural recruitment with higher levels of fitness seen in anterior regions was not present for all conditions of the Stroop task, but was more selectively found for the most challenging condition, suggesting that fitness is not associated with a general increase in recruitment of the anterior processing regions, but is associated with a more selective up-regulation of such regions in response to increasing task demands. Juxtaposed with our previous aging research (Prakash et al., 2010a), along with that of other research studies demonstrating an inability of older adults to recruit additional regions of the attentional network in response to increasing task demands (Milham et al., 2001; Nagel et al., 2009), suggests that higher fitness might be one lifestyle factor through which older adults could potentially demonstrate a more youth-like, load-dependent modulation of the prefrontal and parietal sites.
Our study involved an examination of the association between anterior and posterior processing regions with fitness in older adults, and though a direct age-related comparison was not performed to examine differences in recruitment of these regions as a function of age, we believe, these results taken together with our previous age-related findings on this task (Prakash et al., 2010a) provide us with some degrees of freedom to make connections to the current literature on aging and tie this work with the current theories and views of the aging mind. Keeping this caveat in mind, there are two important points to consider. First, a number of neuroimaging studies demonstrate an increase of prefrontal activation with age and a concomitant decrease in sensory processing in the visual cortex (Grady et al., 1994; Rypma and D’Esposito, 2000; Madden et al., 2002). Increased activation in the prefrontal cortices in light of declining efficiency of the posterior processing regions has been reported in many studies focusing on selective attention (Madden and Hoffman, 1997; Cabeza et al., 2004), episodic memory (Grady et al., 1995; Gutchess et al., 2005) and has been interpreted as reflecting a process of compensatory scaffolding (Park and Reuter-Lorenz, 2009) whereby the over-activation in the prefrontal cortices is thought to compensate for declines in the posterior processing regions (Davis et al., 2008; Dennis and Cabeza, 2008; Park and Reuter-Lorenz, 2009). Within this context, higher-fit older adults capitalizing on the scaffolding properties of the prefrontal cortex, show a greater recruitment of these regions providing evidence for the neural changes accompanying cardiorespiratory fitness. Importantly, the over-activation of the prefrontal and parietal sites was not seen for all conditions, but was selectively observed for the challenging condition of the Stroop task.
The additional recruitment of the prefrontal and parietal cortices have been observed in older adults during performance on many tasks, and several theories and hypotheses have been postulated speculating the possible functional significance of the additional recruitment (Park et al., 2001, 2004; Cabeza, 2002; Logan et al., 2002; Reuter-Lorenz and Lusting, 2005). One such view, emanating mostly from studies showing a positive relationship between cognitive performance and increased activation (Reuter-Lorenz et al., 1999, 2000; Cabeza et al., 2002; Dolcos et al., 2002) provides support for the compensation hypotheses of neurocognitive aging. In another set of studies, a negative relationship has been reported between cognitive performance and increased activation, supporting the notion that aging results in a deterioration of various cortical regions, resulting in a more non-selective recruitment of these processors (Logan et al., 2002; Colcombe et al., 2005; Erickson et al., 2007). Building on this research, we recently found that while older adults show greater recruitment of the regions comprising the attentional network, they demonstrate an inability to increase activation in the attentional network during conditions of enhanced conflict, like the younger adults (Prakash et al., 2010a). This inability to recruit regions of the cortex in response to increasing task demands possibly results from an over-recruitment of these regions during conditions of low conflict. The results of the present study, presents the possibility that cardiorespiratory fitness could enhance cognitive function by allowing the prefrontal cortices to respond more flexibly to task demands, that is, show an increase in neural recruitment in response to increases in conflict, thereby reflecting the presence of greater “reserve” resources for higher-fit individuals which could be tapped into in light of challenging task demands.
The results of our study provide evidence for a differential impact of cardiorespiratory fitness on anterior and posterior processing regions of the brain. While interesting, these should be interpreted in the context of several limitations and possible alternative explanations. Firstly, we present a cross-sectional examination of an association between fitness and cortical recruitment while controlling for variables known to possibly have an influence on our dependent variables of interest, that is, age, gender, education, and verbal intelligence. Though cross-sectional studies offer an important first step toward the examination of a hypothesized relationship between two variables, such studies are limited by their ability to examine a multitude of variables that could potentially influence the present results. Thus, it is likely that fitness may not have a direct influence on cortical recruitment, but through increased vascularization and synaptic plasticity that are known to be associated with higher levels of fitness (Black et al., 1990; Swain et al., 2003; Cotman et al., 2007), it might result in a greater recruitment of the prefrontal cortices. However, if increased vascularization was the only possible mechanism through which fitness might enhance cortical recruitment, such an effect would then be observed not only for the eligible condition in the anterior regions, but should be present for all conditions of the Stroop task. The lack of brain perfusion data in this study, prevents us from directly examining the effects of fitness on cortical recruitment beyond that of increased vasculature, but future studies measuring both blood flow and BOLD signal should help clarify this confound. On a similar note, individual differences in self-efficacy, arousal, and fatigue might mediate the effects of fitness on cortical recruitment and though our cross-sectional design prevents us from examining the influence of all such variables, we believe, a thorough longitudinal randomized intervention trial will help delineate conclusively the beneficial effects of fitness on cortical recruitment.
Another limitation of the study was the rather homogeneous sample of older adults that was recruited for the current study, which in turn restricts the generalizations that can be made from the results of the study. We included relatively sedentary older adults in our sample. It will be important for future studies to examine whether cortical recruitment in the anterior regions is further enhanced with higher levels of fitness. Further, given the homogeneity in our sample, and the cross-sectional nature of our study, we also failed to find an influence of fitness on the posterior processing regions, which are important for efficient top-down control. It is conceivable that fitness might facilitate better top-down modulation by increasing the functional connectivity of the anterior regions with the posterior regions. In future studies, it would be important to examine the longitudinal effects of an exercise intervention on posterior and anterior systems and how the interaction between these two systems change as a function of the intervention.
Despite these limitations, we believe, our study lends support to an impressive array of animal and human literature that provides critical insights into the mechanistic effects of fitness training on brain plasticity (van Praag et al., 1999, 2005; Cotman and Berchtold, 2002; Colcombe et al., 2004, 2006). For example, in rodents, exercise is known to induce a series of molecular and cellular cascade of events such as cell proliferation, and synaptic plasticity in the hippocampus (Black et al., 1990; Kleim et al., 2002; Swain et al., 2003; Farmer et al., 2004; Christie et al., 2008); increase in the serotonin (Blomstrand et al., 1989), acetylcholine (Fordyce and Farrar, 1991), and dopamine levels (Poulton and Muir, 2005); and an increase in the production of nerve-growth factors like brain derived neurotrophic factor (BDNF, Vaynman et al., 2004), insulin-like growth factor-1 (IGF-1, Trejo et al., 2001; Lopez-Lopez et al., 2004) and vascular endothelial growth factor (VEGF, Fabel et al., 2003), molecules which are known to be necessary in long term potentiation and angiogenesis. Further, recent human work also provides converging evidence of neurobiological changes that might be associated with cardiorespiratory fitness, such as hippocampal volume mediating the association between fitness and behavioral performance (Erickson et al., 2009b); increased fractional anisotropy values in the cingulum and the uncinate fasciculus (Marks et al., 2007); and an increase in the volume of gray and white matter following an aerobic exercise intervention (Colcombe et al., 2006).
In conclusion, the results of this study lend support for the prophylactic influence of cardiorespiratory fitness on cognition. We found that higher levels of fitness were associated with better behavioral performance on the most demanding condition of the Stroop task. This is consistent with our previous work, in which we have reported that the largest benefits of fitness training appear to be for tasks involving higher-level executive control processes (Kramer et al., 1999; Colcombe and Kramer, 2003; Colcombe et al., 2004). In addition, we found that higher levels of fitness differentially impacted the anterior and posterior systems. Older adults, with greater aerobic endurance capacity, showed increased activation in the prefrontal regions that are responsible for imposing attentional control. Though in this study we did not find an association between cardiorespiratory fitness and posterior processing, in the future it will be important to determine how the anterior and posterior systems behave during the Stroop task as a result of aerobic fitness training. It is possible that even though higher-fit older adults are able to engage the natural capacity for plasticity in the prefrontal cortices, exercise training may involve a shift from the scaffolding of the prefrontal cortices to a recruitment of more specialized neural circuitry involving the posterior processing regions.
Conflict of Interest Statement
The authors declare that the research was conducted in the absence of any commercial or financial relationships that could be construed as a potential conflict of interest.
Acknowledgments
This work was supported by the National Institute on Aging (grant numbers RO1 AG25667, and RO1 AG25032), and the Institute for the Study of Aging. The authors thank Nancy Dodge and Holly Tracy for their help with data collection.
Footnotes
References
Banich, M. T., Milhalm, M. P., Atchley, R., Cohen, N. J., Webb, A., Wszalek, T., Kramer, A. F., Liang, Z., Wright, A., Shenker, J., and Magin, J. (2000). fMRI studies of Stroop tasks reveal unique roles of anterior and posterior brain systems in attentional selection. J. Cogn. Neurosci. 12, 988–1000.
Banich, M. T., Milhalm, M. P., Jacobson, B. L., Webb, A., Wszalek, T., and Cohen, N. J. (2001). Attentional selection and the processing of task-irrelevant information: insights from fMRI examinations of the Stroop task. Prog. Brain Res. 134, 450–470.
Bar, M. (2003). A cortical mechanism for triggering top-down facilitation in visual object recognition. J. Cogn. Neurosci. 15, 600–609.
Barcelo, F., Suwazono, S., and Knight, R. T. (2000). Prefrontal modulation of visual processing in humans. Nat. Neurosci. 3, 399–403.
Beckmann, C. F., Jenkinson, M., and Smith, S. M. (2003). General multi-level linear modeling for group analysis in FMRI. Neuroimage 20, 1052–1063.
Bench, C. J., Frith, C. D., Grasby, P. M., Friston, K. J., Paulesu, E., Frackowiak, R. S. J., and Dolan, R. J. (1993). Investigations of the functional anatomy of attention using the Stroop test. Neuropsychologia 31, 907–922.
Black, J. E., Isaacs, K. R., Anderson, B. J., Alcantara, A. A., and Greenough, W. T. (1990). Learning causes synaptogenesis, whereas motor activity causes angiogenesis, in cerebellar cortex of adult rats. Proc. Natl. Acad. Sci. U.S.A. 87, 5568–5572.
Blomstrand, E., Perret, D., Parry-Billings, M., and Newsholme, E. A. (1989). Effect of sustained exercise on plasma amino acid concentrations on 5-hydroxy-tryptamine metabolism in six different brain regions in the rat. Acta Physiol. Scand. 136, 473–481.
Brown, G. G., Kinderman, S. S., Siegle, G. J., Granholm, E., Wong, E. C., and Buxton, R. B. (1999). Brain activation and pupil response during covert performance of the Stroop Color Word task. J. Int. Neuropsychol. Soc. 5, 308–319.
Bush, G., Whalen, P. J., Rosen, B. R., Jenike, M. A., McInerney, S. C., and Rauch, S. L. (1998). The counting Stroop: an interference task specialized for functional neuroimaging – validation study with functional MRI. Hum. Brain Mapp. 6, 270–282.
Cabeza, R. (2002). Hemispheric asymmetry reduction in older adults: the HAROLD model. Psychol. Aging 17, 85–100.
Cabeza, R., Anderson, N. D., Locantore, J. K., and McIntosh, A. R. (2002). Aging gracefully: compensatory brain activity in high-performing older adults. Neuroimage 17, 1394–1402.
Cabeza, R., Daselaar, S. M., Dolcos, F., Prince, S. E., Budde, M., and Nyberg, L. (2004). Task-independent and task-specific age effects on brain activity during working memory, visual attention and episodic retrieval. Cereb. Cortex 14, 364–375.
Chaddock, L., Erickson, K. I., Prakash, R. S., and Kramer, A. F. (2010). Basal ganglia volume is associated with aerobic fitness in preadolescent children. Dev. Neurosci. 32, 249–256.
Christie, B. R., Eadie, B. D., Kannangara, T. S., Robillard, J. M., Shin, J., and Titterness, A. K. (2008). Exercising our brains: how physical activity impacts synaptic plasticity in the dentate gyrus. Neuromol. Med. 10, 47–58.
Cohen, L., Dehaene, S., Naccache, L., Lehericy, S., Dehaene-Lambertz, G., Henaff, M. A., and Michel, F. (2000). The visual word form area: spatial and temporal characterization of an initial stage of reading in normal subjects and posterior split-brain patients. Brain 123, 291–307.
Colcombe, S., and Kramer, A. F. (2003). Fitness effects on the cognitive function of older adults: a meta-analytic study. Psychol. Sci. 14, 125–130.
Colcombe, S. J., Erickson, K. I., Raz, N., Webb, A. G., Cohen, N. J., and McAuley, E. (2003). Aerobic fitness reduces brain tissue loss in aging humans. J. Gerontol. A Biol. Sci. Med. Sci. 55, 176–180.
Colcombe, S. J., Erickson, K. I., Scalf, P. E., Kim, J. S., Prakash, R., McAuley, E., Elavsky, S., Marquez, D. X., Hu, L., and Kramer, A. F. (2006). Aerobic exercise training increases brain volume in aging humans. J. Gerontol. A Biol. Sci. Med. Sci. 61, 1166–1170.
Colcombe, S. J., Kramer, A. F., Erickson, K. I., Scalf, P., McAuley, E., and Cohen, N. J. (2004). Cardiovascular fitness, cortical plasticity, and aging. Proc. Natl. Acad. Sci. U.S.A. 101, 3316–3321.
Colcombe, S. J., Kramer, A. F., Erickson, K. I., and Scalf, P. (2005). The implications of cortical recruitment and brain morphology for individual differences in inhibitory functioning in aging humans. Psychol. Aging 20, 363–375.
Corbetta, M., Miezin, F. M., Dobmeyer, S., Schulman, G. L., and Petersen, S. E. (1990). Attentional modulation of neural processing of shape, color and velocity in humans. Science 248, 1556–1559.
Cotman, C. W., and Berchtold, N. C. (2002). Exercise: a behavioral intervention to enhance brain health and plasticity. Trends Neurosci. 25, 295–301.
Cotman, C. W., Berchtold, N. C., and Christie, L.-A. (2007). Exercise builds brain health: key roles of growth factor cascades and inflammation. Trends Neurosci. 30, 464–472.
Dale, A. M. (1999). Optimal experimental design for event-related fMRI. Hum. Brain Mapp. 8, 109–114.
Davis, S. W., Dennis, N. A., Daselaar, S. M., Fleck, M. S., and Cabeza, R. (2008). Que PASA? The posterior anterior shift in aging. Cereb. Cortex 18,1201–1209.
Dennis, N. A., and Cabeza, R. (2008). “Neuroimaging of healthy cognitive aging,” in Handbook of Aging and Cognition, 3rd Edn., eds F. I. M. Craik and T. A. Salthouse (Mahwah, NJ: Erlbaum), 1–53.
DiGirolamo, G. J., Kramer, A. F., Barad, V., Cepeda, N., Weissman, D. H., Wszalek, T. M., Cohen, N. J., Banich, M., Webb, A., and Beloposky, A. (2001). General and task-specific frontal lobe recruitment in older adults during executive processes: a fMRI investigation of task switching. Neuroreport 12, 2065–2072.
Dolcos, F., Rice, H. J., and Cabeza, R. (2002). Hemispheric asymmetry and aging: right hemisphere decline or hemispheric asymmetry. Neurosci. Biobehav. Rev. 26, 819–825.
Erickson, K. I., Colcombe, S. J., Wadhwa, R., Bherer, L., Peterson, M. S., Scalf, P. E., Kim, J. S., Alvarado, M., and Kramer, A. F. (2007). Training-induced plasticity in older adults: effects of training on hemispheric asymmetry. Neurobiol. Aging 28, 272–283.
Erickson, K. I., Prakash, R. S., Kim, J. S., Sutton, B. P., Colcombe, S. J., and Kramer, A. F. (2009a). Top-down attentional control in spatially coincident stimuli enhances activity in both task-relevant and task-irrelevant regions of cortex. Behav. Brain Res. 197, 186–197.
Erickson, K. I., Prakash, R. S., Voss, M. W., Chaddock, L., Hu, L., Morris, K. S., White, W. M., Wojcicki, T. R., McAuley, E., and Kramer, A. F. (2009b). Aerobic fitness is associated with hippocampal volume in elderly humans. Hippocampus 19, 1030–1039.
Fabel, K., Fabel, K., Tam, B., Kaufer, D., Baiker, A., Simmons, N., Kuo, C. J., and Palmer, T. D. (2003). VEGF is necessary for exercise-induced adult hippocampal neurogenesis. Eur. J. Neurosci. 18, 2803–2812.
Farmer, J., Zhao, X., van Praag, H., Wodtke, K., Gage, F. H., and Christie, B. R. (2004). Effects of voluntary exercise on synaptic plasticity and gene expression in the dentate gyrus of adult male Sprague-Dawley rats in vivo. Neuroscience 124, 71–79.
Fordyce, D. E., and Farrar, R. P. (1991). Enhancement of spatial learning in F344 rats by physical activity and related learning-associated alterations in hippocampal and cortical cholinergic functioning. Behav. Brain Res. 46, 123–133.
Frith, C. (2001). A framework for studying the neural basis of attention. Neuropsychologia 39, 1367–1371.
Gazzaley, A., Rissman, J., Cooney, J., Rutman, A., Seibert, T., Clapp, W., and D’Esposito, M. (2007). Functional interactions between prefrontal and visual association cortex contribute to top-down modulation of visual processing. Cereb. Cortex 17, 125–135.
Grady, C. L., Maisog, J. M., Horwitz, B., Ungerleider, L. G., Mentis, M. J., Salerno, J. A., Pietrini, P., Wagner, E., and Haxby, J. V. (1994). Age-related changes in cortical blood flow activation during visual processing of faces and location. J. Neurosci. 14, 1450–1462.
Grady, C. L., McIntosh, A. R., Horwitz, B., Maisog, J. M., Ungerleider, L. G., Mentis, M. J., Pietrini, P., Schapiro, M. B., and Haxby, J. V. (1995). Age-related reductions in human recognition memory due to impaired encoding. Science 269, 218–221.
Gutchess, A. H., Welsh, R. C., Hedden, T., Bangert, A., Minear, M., and Liu, L. L. (2005). Aging and the neural correlates of successful picture encoding: frontal activations compensate for decreased medial-temporal activity. J. Cogn. Neurosci. 17, 84–96.
Hertzog, C., Kramer, A. F., Wilson, R. S., and Lindenberger, U. (2009). Enrichment effects on adult cognitive development: can the functional capacity of older adults be preserved and enhanced? Psychol. Sci. Public Interest 9, 1–65.
Jenkinson, M. (2003). Fast, automated, N-dimensional phase-unwrapping algorithm. Magn. Reson. Med. 49, 193–197.
Jobard, G., Crivello, F., and Tzourio-Mazoyer, N. (2003). Evaluation of the dual route theory of reading: a meta analysis of 35 neuroimaging studies. Neuroimage 20, 693–712.
Kastner, S., and Ungerleider, L. G. (2001). The neural bases of biased competition in human visual cortex. Neuropsychologia 39, 1263–1276.
Kaufman, A. S., and Kaufman, N. L. (1990). Kaufman Brief Intelligence Test: Manual. Circle Pines, MN: American Guidance Service.
Kleim, J. A., Cooper, N. R., and VandenBerg, P. M. (2002). Exercise induces angiogenesis but does not alter movement representations within rat motor cortex. Brain Res. 934, 1–6.
Kline, G. M., Porcari, J. P., Hintermeister, R., Freedson, P. S., Ward, A., McCarron, R. F., Ross, J., and Rippe, J. M. (1987). Estimation of VO2 Max from a one mile track walk, gender, age, and body weight. Med. Sci. Sports Exerc. 19, 253–259.
Kramer, A. F., Hahn, S., Cohen, N., Banich, M., McAuley, E., Harrison, C., Chason, J., Vakil, E., Bardell, L., Boileau, R. A., and Colcombe, A. (1999). Aging, fitness, and neurocognitive function. Nature 400, 418–419.
Langenecker, S. A., Nielson, K. A., and Rao, S. M. (2004). fMRI of healthy older adults during Stroop interference. Neuroimage 21, 192–200.
Lavie, N., Hirst, A., DeFockert, J. W., and Viding, E. (2004). Load theory of selective attention and cognitive control. J. Exp. Psychol. 133, 339–354.
Liu, X., Banich, M. T., Jacobson, B. L., and Tanabe, J. L. (2006). Functional dissociation of attentional selection within PFC: response and non-response related aspects of attentional selection as ascertained by fMRI. Cereb. Cortex 16, 827–834.
Logan, J. M., Sanders, A. L., Snyder, A. Z., Morris, J. C., and Buckner, R. L. (2002). Under- recruitment and nonselective recruitment: dissociable neural mechanisms associated with aging. Neuron 33, 827–840.
Lopez-Lopez, C., LeRoith, D., and Torres-Aleman, I. (2004). Insulin-like growth factor I is required for vessel remodeling in the adult brain. Proc. Natl. Acad. Sci. U.S.A. 101, 9833–9838.
Lotscher, F., Loffel, T., Steiner, R., Vogt, M., Klossner, S., Popp, A., Lippuner, K., Hoppeler, H., and Dapp, C. (2007). Biologically relevant sex differences for fitness-related parameters in active octogenarians. Eur. J. Appl. Physiol. 99, 533–540.
Madden, D. J., and Hoffman, J. M. (1997). “Application of positron emission tomography to age-related cognitive changes,” in Brain Imaging in Clinical Psychiatry, eds K. K. R. Krishnan and P. M. Doraiswamy (New York: Marcel Dekker), 575–613.
Madden, D. J., Turkington, T. G., Provenzale, J. M., Denny, L. L., Langley, L. K., Hawk, T. C., and Coleman, R. E. (2002). Aging and attentional guidance during visual search: functional neuroanatomy by positron emission tomography. Psychol. Aging 17, 24–43.
Marks, B. L., Madden, D. J., Bucur, B., Provenzale, J. M., White, L. E., Cabeza, R., and Huettel, S. A. (2007). Role of aerobic fitness and aging on cerebral white matter integrity. Ann. N. Y. Acad. Sci. 1097, 171–174.
Meyer, K., Niemann, S., and Abel, T. (2004). Gender differences in physical activity and fitness—association with self-reported health and health-relevant attitudes in a middle-aged Swiss urban population. J. Public Health 12, 283–290.
Milham, M. P., Banich, M. T., Webb, A., Barad, V., Cohen, N. J., and Wszalek, T. (2001). The relative involvement of anterior cingulate and prefrontal cortex in attentional control depends on nature of conflict. Brain Res. Cogn. 12, 1325–1346.
Milham, M. P., Erickson, K. I., Banich, M. T., Kramer, A. F., Webb, A., and Wszalek, T., and Cohen, N. J. (2002). Attentional control in the aging brain: insights from an fMRI study of the Stroop task. Brain Cogn. 49, 467–473.
Nagel, I. E., Preuschhof, C., Li, S-C., Nyberg, L., Backmann, L., Lindenberger, U., and Heekeren, H. R. (2009). Performance level modulates adult age differences in brain activation during spatial working memory. Proc. Natl. Acad. Sci. U.S.A. 106, 22552–22557.
Park, D. C., Polk, T. A., Mikels, J. A., Taylor, S. F., and Marshuetz, C. (2001). Cerebral aging: integration of brain and behavioral models cognitive function. Dialogues Clin. Neurosci. Cereb. Aging 3, 151–165.
Park, D. C., Polk, T. A., Park, R., Minear, M., Savage, A., and Smith, M. R. (2004). Aging reduces neural specialization in ventral visual cortex. Proc. Natl. Acad. Sci. U.S.A. 101, 13091–13095.
Park, D. C., and Reuter-Lorenz, P. A. (2009). The adaptive brain: aging and neurocognitive scaffolding. Annu. Rev. Psychol. 60, 173–196.
Pessoa, L., Kastner, S., and Underleider, L. G. (2003). Neuroimaging studies of attention: from modulation of sensory processing to top-down control. J. Neurosci. 23, 3990–3998.
Poulton, N. P., and Muir, G. D. (2005). Treadmill training ameliorates dopamine loss but not behavioral deficits in hemi-parkinsonian rats. Exp. Neurol. 193, 181–197.
Prakash, R. S., Erickson, K. I., Colcombe, S. J., Kim, J., Sutton, B., and Kramer, A. F. (2010a). Age-related differences in the involvement of the prefrontal cortex in attentional control. Brain Cogn. 71, 328–335.
Prakash, R. S., Snook, E. M., Motl, R. W., and Kramer, A. F. (2010b). Aerobic fitness is associated with gray matter volume and white matter integrity in multiple sclerosis. Brain Res. 1341, 41–51.
Prakash, R. S., Snook, E. M., Erickson, K. I., Colcombe, S. J., Webb, M. L., Motl, R. W., and Kramer, A. F. (2007). Cardiorespiratory fitness: a predictor of cortical plasticity in multiple sclerosis. Neuroimage 34, 1238–1244.
Reuter-Lorenz, P. A., and Lustig, C. (2005). Brain aging: reorganizing discoveries about the aging mind. Curr. Opin. Neurobiol. 15, 245–251.
Reuter-Lorenz, P. A., and Mikels, J. (2006). “The aging brain: implications of enduring plasticity for behavioral and cultural change,” in Lifespan Development and the Brain: The Perspective of Biocultural Co-Constructivism, eds P. Baltes, P. A. Reuter-Lorenz, and F. Roesler (Cambridge, UK: Cambridge University Press), 255–276.
Reuter-Lorenz, P., Jonides, J., Smith, E. S., Hartley, A., Miller, A., Marscheutz, C., and Koeppe, R. A. (2000). Age differences in the frontal lateralization of verbal and spatial working memory revealed by PET. J. Cogn. Neurosci. 12, 174–187.
Reuter-Lorenz, P., Stanczak, L., and Miller, A. (1999). Neural recruitment and cognitive aging: two hemispheres are better than one, especially as you age. Psychol. Sci. 10, 494–500.
Rissman, J., Gazzaley, A., and D’Esposito, M. (2004). Measuring functional connectivity during distinct stages of a cognitive task. Neuroimage 23, 752–763.
Rypma, B., and D’Esposito, M. (2000). Isolating the neural mechanisms of age-related changes in human working memory. Nat. Neurosci. 3, 509–515.
Sheikh, J. I., and Yesavage, J. A. (1986). “Geriatric Depression Scale (GDS): recent evidence and development of a shorter version,” in Clinical Gerontology: A Guide to Assessment and Intervention, ed. T. L. Brink (New York: The Haworth Press), 165–173.
Stern, Y., Sano, M., Paulsen, J., and Mayeux, R. (1987). Modified mini-mental state examination: validity and reliability. Neurology 37, 179.
Swain, R. A., Harris, A. B., Wiener, E. C., Dutka, M. V., Morris, H. D., Theien, B. E., Konda, S., Engberg, K., Lauterbur, P. C., and Greenough, W. T. (2003). Prolonged exercise induces angiogenesis and increases cerebral blood volume in primary motor cortex of the rat. Neuroscience 117, 1037–1046.
Trejo, J. L., Carro, E., and Torres-Aleman, I. (2001). Circulating insulin-like growth factor mediates exercise-induced increases in the number of new neurons in the adult hippocampus. J. Neurosci. 21, 1628–1634.
Van Essen, D. C. (2005). A population-average, landmark- and surface-based (PALS) atlas of human cerebral cortex. Neuroimage 28, 635–662.
van Praag, H., Kempermann, G., and Gage, F. H. (1999). Running increases cell proliferation and neurogenesis in the adult mouse dentate gyrus. Nat. Neurosci. 2, 266–270.
van Praag, H., Shubert, T., Zhao, C., and Gage, F. H. (2005). Exercise enhances learning and hippocampal neurogenesis in aged mice. J. Neurosci. 25, 8680–8685.
Vaynman, S., Ying, Z., and Gomez-Pinilla, F. (2004). Hippocampal BDNF mediates the efficacy of exercise on synaptic plasticity and cognition. Eur. J. Neurosci. 20, 2580–2590.
Voss, M. W., Erickson, K. I., Prakash, R. S., Chaddock, L., Malkowski, E., Alves, H., Kim, J. S., Morris, K. S., White, S. M., Wójcicki, T. R., Hu, L., Szabo, A., Klamm, E., McAuley, E., and Kramer, A. F. (2010). Functional connectivity: a source of variance in the relationship between cardiorespiratory fitness and cognition. Neuropsychologia 48, 1394–1406.
Keywords: cardiorespiratory fitness, Stroop task, cognitive and attentional control
Citation: Prakash RS, Voss MW, Erickson KI, Lewis JM, Chaddock L, Malkowski E, Alves H, Kim J, Szabo A, White SM, Wójcicki TR, Klamm EL, McAuley E and Kramer AF (2011) Cardiorespiratory fitness and attentional control in the aging brain. Front. Hum. Neurosci. 4:229. doi: 10.3389/fnhum.2010.00229
Received: 25 March 2010;
Accepted: 03 December 2010;
Published online: 14 January 2011.
Edited by:
Donald T. Stuss, Baycrest Centre for Geriatric Care, CanadaReviewed by:
Paul Burgess, University College London, UK;William E. Mcilroy, University of Toronto, Canada
Copyright: © 2011 Prakash, Voss, Erickson, Lewis, Chaddock, Malkowski, Alves, Kim, Szabo, White, Wójcicki, Klamm, McAuley and Kramer. This is an open-access article subject to an exclusive license agreement between the authors and the Frontiers Research Foundation, which permits unrestricted use, distribution, and reproduction in any medium, provided the original authors and source are credited.
*Correspondence: Ruchika Shaurya Prakash, Department of Psychology, The Ohio State University, 225 Psychology Building, 1835 Neil Avenue, Columbus, OH 43210, USA. e-mail: prakash.30@osu.edu