- 1Department of Rehabilitation, Tianjin University Tianjin Hospital, Tianjin, China
- 2Tianjin Key Laboratory of Exercise Physiology and Sports Medicine, Institute of Sport, Exercise and Health, Tianjin University of Sport, Tianjin, China
Physical therapy is gaining recognition as an effective therapeutic approach in the realm of peripheral nerve injury (PNI) research. This article seeks to provide a comprehensive review of the latest advancements, applications, and mechanisms of action of four physical therapy modalities—ultrasound, electrical stimulation, photobiomodulation, and aerobic exercise—in the context of PNI. Ultrasound, characterized by its mechanical and thermal effects, is widely regarded as an effective non-invasive or minimally invasive method for neural modulation. Electrical stimulation therapy, a prevalent technique in PNI treatment, entails the application of electric currents to stimulate nerve and muscle tissues, thereby facilitating nerve regeneration and mitigating muscle atrophy. Photobiomodulation, a process that influences cell metabolism through the absorption of photon energy, is closely associated with neural regeneration in the field of rehabilitation medicine. Additionally, aerobic exercise, a popular form of physical activity, serves to enhance blood circulation and improve neuronal function. The article discusses various physical therapy methods for peripheral nerve injuries, including hyperbaric oxygen therapy, magnetic therapy, and biofeedback therapy, in addition to traditional approaches. Despite advancements, challenges in nerve injury treatment persist, such as the need for standardized treatment protocols, consideration of individual variations, and assessment of long-term effectiveness. Future research is needed to address these issues. In summary, this article offers theoretical and empirical evidence supporting the utilization of physical therapy in the management of PNI. This research aims to promote further research and clinical practice in this field, contributing to enhancing patient quality of life and recovery outcomes.
1 Introduction
Peripheral Nerve Injury (PNI) involves harm to nerves located outside the brain and spinal cord, typically resulting from direct trauma or indirect factors such as accidents, combat, or complications arising from illnesses (1). In Europe and America, traumatic peripheral nerve injuries affect hundreds of thousands of patients each year (2). In addition to a high incidence rate, PNI exhibits the following characteristics: slow growth, with regenerating nerves progressing at a rate of ~1 mm per day, and a complex direction of regeneration that frequently results in nerve misrouting and looping (3). This slow growth rate can cause the corresponding target organs to be in a state of denervation for a long time, leading to a certain degree of motor dysfunction, sensory dysfunction, and autonomic dysfunction, with a poor prognosis. Studies show that only 10%−25% (4) of those with nerve injuries fully recover their functions. Furthermore, in addition to inducing lesions at the site of trauma, It also results in abnormal communication between the central nervous system and the periphery, causing varying degrees of pathology, affecting mobility and bringing emotional and economic burdens (5).
PNI, due to tissue damage, suturing, and fixation, often leads to peripheral tissue edema and adhesions, and even muscle contractures and joint stiffness, ultimately resulting in severe impairment of limb function. Physical factor therapy, such as electrical, ultrasound, and photobiomodulation, can effectively alleviate these symptoms. Furthermore, various physical factor therapies also promote nerve regeneration to some extent, but their specific mechanisms of enhancement are currently unclear, making it difficult to accurately select the appropriate physical factor therapy and set the right parameters. In addition, aerobic exercise, as a safe and effective rehabilitation method, plays a significant role in facilitating the recovery from PNI. Similar to physical factor therapy, exercise therapy lacks standardized treatment protocols, particularly in terms of planned intensity, duration, and timing of initiation. Additionally, various other physical therapies, including magnetic therapy, such as magnetic therapy, hyperbaric oxygen therapy, and cryotherapy, have been utilized in the treatment of PNI with favorable outcomes.
The objective of this review is to comprehensively outline the advancements in research and potential mechanisms of various physical therapies for enhancing the functional rehabilitation of PNI. Specifically, this review will concentrate on the impacts of ultrasound, electrical stimulation, photobiomodulation, and other physical modalities, along with the role of aerobic exercise in PNI recovery. Ultrasound, characterized as a mechanical wave, possesses the ability to accurately transmit energy into tissues at significant depths in both spatial and temporal domains (6). Its thermal properties make it an advantageous modality for non-invasive or minimally invasive neuromodulation procedures. Electrical stimulation therapy, a common physical factor therapy in PNI, utilizes electrical currents to stimulate nerves and muscle tissues. This method can facilitate nerve regeneration and reduce muscle atrophy (7). Photobiomodulation is intricately associated with nerve regeneration due to its photochemical and photobiological mechanisms that influence cellular processes, as well as its capacity to stimulate the secretion of neurotrophic factors (8). Aerobic exercise, a prevalent form of physical activity, is known to enhance cardiovascular function and oxygen delivery, potentially mitigating muscle atrophy and spinal alterations following PNI (Table 1). This review also introduces other physical factor therapies used in PNI (Figure 1). In response to the shortcomings of various therapies and the current inadequacy of physiotherapy in the treatment of PNI, we propose a strategy of integrated physiotherapy, which we believe is promising in the treatment of PNI.
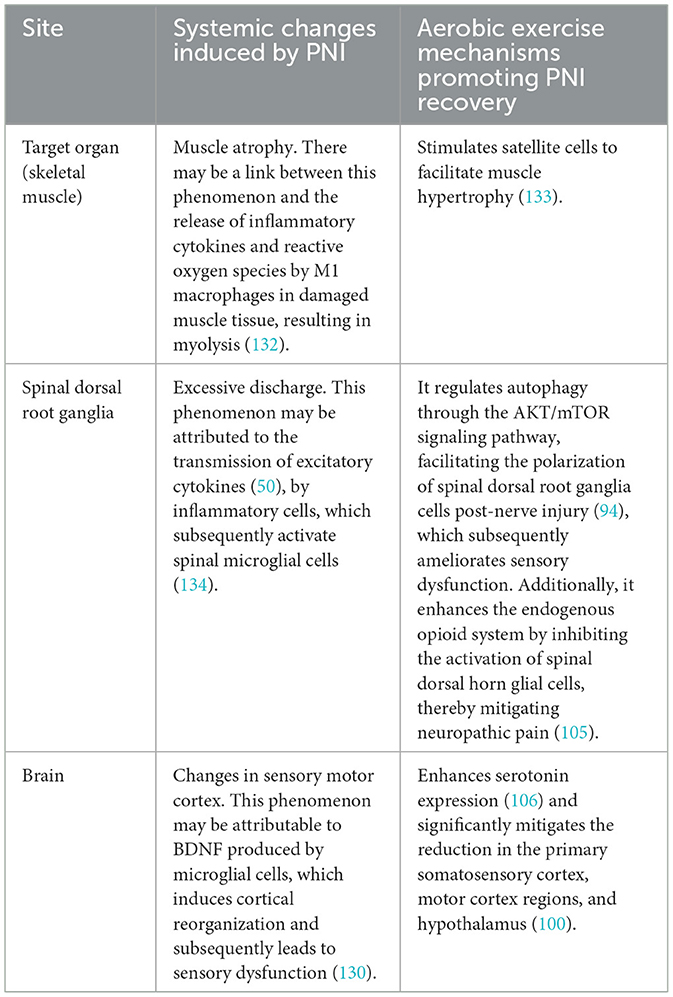
Table 1. The systemic alterations following PNI and the mechanisms through which aerobic exercise facilitates PNI recovery.
2 Search strategy and selection criteria
We conducted a detailed search in the PubMed database using the following keywords: “peripheral nerve injury”, “peripheral nerve regeneration”, “functional recovery”, “mechanisms”, “ultrasound”, “electrical stimulation”, “photobiomodulation”, “low-intensity laser”, and “aerobic exercise”. Our search focused on articles published between 2019 and July 2024. However, to encompass literature of significant importance, we also included seminal works published prior to this period. After removing duplicates, we initially screened the titles and abstracts of each article. We then reviewed the full texts to exclude studies that did not pertain to peripheral nerve injury and physical therapy.
3 The impact of ultrasound on peripheral nerve injury
3.1 The application of ultrasound in peripheral nerve injury
Ultrasound (US) is a high-frequency sound wave that surpasses the human auditory threshold of 20 kHz and is frequently employed in medical imaging for safe and non-invasive clinical procedures (9). Furthermore, as a mechanical vibratory wave, the mechanical energy produced by US can be absorbed by biological tissues to achieve therapeutic purposes. In rehabilitation medicine, the commonly used frequencies are 800–1,000 kHz (10). The exploration of US as a therapeutic modality has roots in ancient times. In 1929 (11), the irradiation of a frog's sciatic nerve by US has been observed to induce minor twitches in the gastrocnemius muscle. This phenomenon has led scholars to investigate the potential of US in promoting the healing of fractures, tendons, ligaments, and other soft tissues. Within the realm of peripheral nerve regeneration, US is specifically referred to as Low-Intensity Pulsed Ultrasound (LIPUS). This modality holds promise for neural regulation (12, 13). In 1958, researchers (14) identified that high-intensity US possesses the ability to mitigate motor disorders through its thermal properties. This discovery underscored the considerable promise of high-intensity US's thermal effects for treating Parkinson's disease and chronic pain. Furthermore, studies have indicated that US may impact brain function, with evidence suggesting an inhibitory influence on visual cortex activity in felines. In 2008, Tyler et al. (15) conducted a study on the effects of LIPUS on neuronal activity. Their findings indicated that LIPUS has the capability to stimulate neuron and network activity remotely and non-invasively, implying that US can serve as an effective tool for modulating brain circuit activity from a distance. Several studies have examined the influence of US on PNI. Affonso conducted a study in 2002 (16) investigating the impact of pulsed US on rat sciatic nerve transection injuries, revealing that US stimulation led to accelerated nerve regeneration.
Research (17) shows that LIPUS treatment enhances functional performance in PNI. After 1 or 4 weeks of daily 5-min sessions at 1 MHz frequency, 140 mW/cm2 intensity, and a 20% duty cycle, the tibialis anterior muscle's CMAP rose by 21.88%, showing improved neuromuscular reinnervation. While myelin sheath thickness increased after 4 weeks compared to the control, there was no significant change in wet muscle weight ratio, suggesting LIPUS may have limited impact on muscle atrophy. Numerous research studies have employed the Sciatic Functional Index (SFI) as a tool for assessing the efficacy and recuperation of sciatic nerve function in rats subjected to models involving sciatic nerve injury (18, 19). The study revealed that the SFI was appreciably elevated in the LIPUS treatment group in comparison to the control group, demonstrating diverse recovery outcomes across different parameters. Selecting the right US parameters is vital for PNI treatment. Jiang et al. (20) found that a low-dose US (250 mW/cm2) was more effective than medium (500 mW/cm2) and high-dose (750 mW/cm2) US in treating sciatic nerve injury. The low-dose group exhibited longer axonal growth, thicker cross-sectional area, and myelin sheath, with consistent results across electron microscopy, toluidine blue staining, and more organized muscle fibers in Masson staining. This study shows that low-dose US enhances nerve regeneration better than higher doses. Low-intensity pulsed ultrasound at 0.3 W/cm2 boosts Schwann cell growth in vitro and is advised for early application on injured nerves, especially in low-serum environments, for optimal results. An animal study (21) showed that 140 mW/cm2 ultrasound significantly increased myelin fiber density by 26.59% and myelin cross-sectional area by 38% compared to controls, despite no change in axonal area.
3.2 Mechanisms of ultrasound therapy for peripheral nerve injury
The biological mechanisms of US therapy encompass mechanical effects, thermal effects, and the physicochemical effects induced by both (cavitation effects). Firstly, because nerve cell membranes contain numerous sensitive ion channels, when US vibration acts on nerve cells, it causes changes in membrane potential thereby affecting nerve signal conduction. Research findings suggest that US with varying parameters can selectively activate mechanically sensitive sodium and potassium channels, contributing to the diverse effects of US on the nervous system (22). Secondly, US on the body can generate a thermal effect, which is the result of acoustic energy converting into heat energy. Finally, the cavitation effect of US refers to the formation of microbubbles of gas in liquids (23), which subsequently oscillate or rupture under appropriate parameters, thereby affecting surrounding tissues or cells. Research on cell interactions suggests a Bilayer Sonophore (BLS) model, which proposes that US impacts intercellular communication by causing deformation of intracellular gas bubbles. This alteration may lead to changes in biochemical signaling pathways, ultimately affecting cell morphology and functionality (24). Using this model, it has been shown that LIPUS modulates cell motility and morphology through the inhibition of MET activation. These findings imply a reciprocal relationship between the intracellular MET signaling pathway and exposure to LIPUS (25) (Figure 2A).
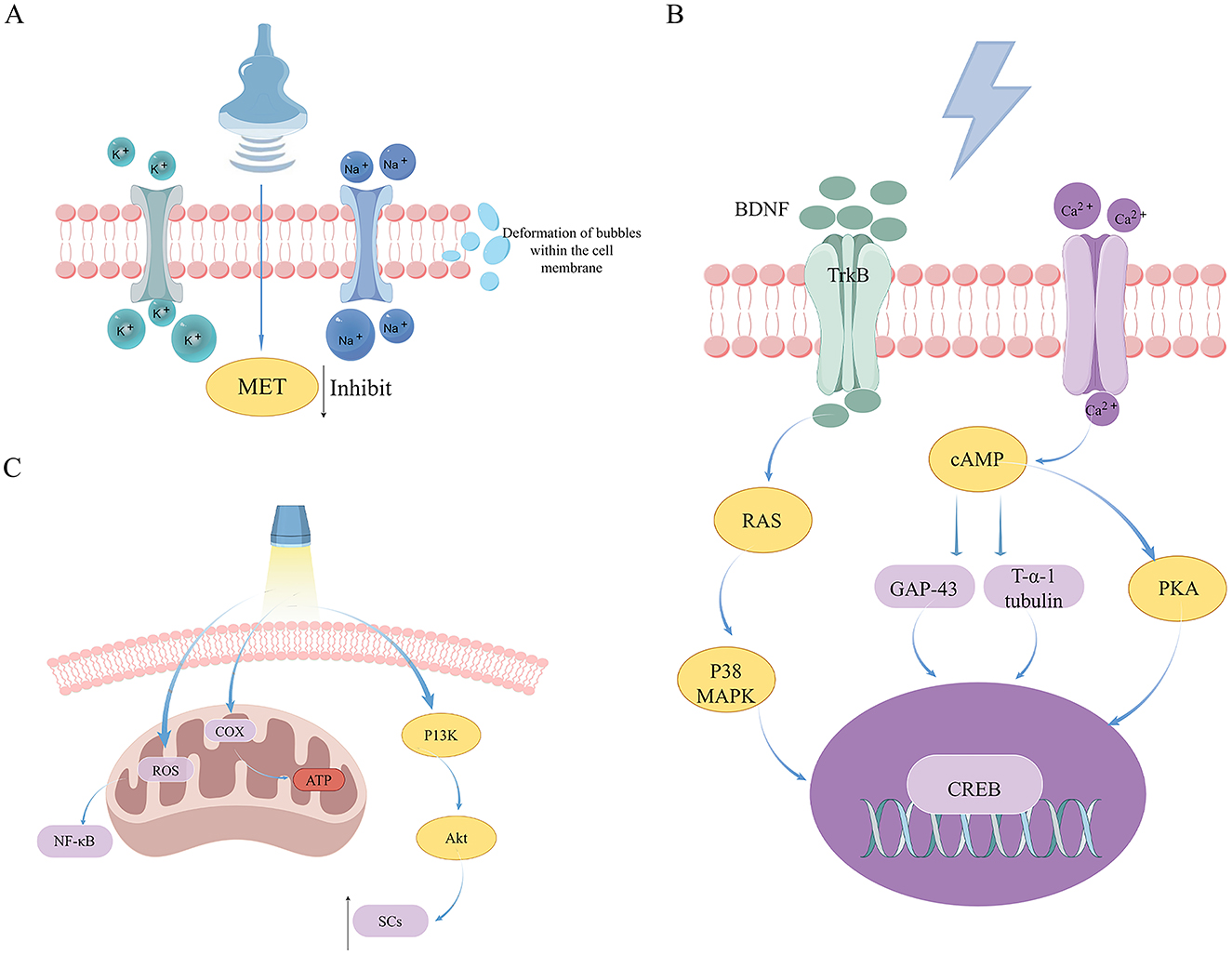
Figure 2. An examination of the mechanisms underlying US, ES, and PBM in the therapeutic management of peripheral nerve injuries. (A) The mechanisms of US therapy in treating PNI. (B) The mechanisms of ES therapy in treating PNI. (C) The mechanisms of PBM therapy in treating PNI.
In the context of PNI treatment in the US, the impact of US is predominantly observed in neuroglial cells, inflammatory cells, and NGF. Neuroglial cells play a vital role as mediators in the process of peripheral nerve regeneration, with particular emphasis on the research surrounding Schwann cells (SCs). In a study conducted by Crisci and Ferreira (16), it was determined that LIPUS stimulation increases the activity of SCs, thereby expediting the early restoration of myelin sheaths. Ren et al. (26) suggested that LIPUS enhances Schwann cell proliferation and nerve regeneration by activating the GSK-3β/β-catenin pathway. GSK-3β, a cytoplasmic kinase, influences developmental signaling and regulates Cyclin D1 transcription through β-catenin phosphorylation, facilitating SC proliferation. Nonetheless, the exact influence of GSK-3β on PNI remains ambiguous, as some research suggests that LIPUS facilitates axon regeneration by suppressing GSK-3β activity (27). It is hypothesized that targeted inhibition of GSK-3β can promote neurite outgrowth, while its overexpression may hinder neurite extension (28). Certain hypotheses suggest that nerve regeneration induced by LIPUS occurs via axonal mediation, without reliance on the proliferation of SCs. The utilization of LIPUS on dorsal root ganglion neurons has been shown to enhance axonal growth by a factor of two, potentially by stimulating the Netrin-1/DCC pathway (29). Subsequent to PNI, activated SCs can stimulate immune reactions; studies have demonstrated that LIPUS reduces the expression of cytokines involved in inflammation TNF-α and IL-6 in rats seven days following nerve crush injury, suggesting that LIPUS may attenuate pro-inflammatory reactions during the process of peripheral nerve regeneration (21). The results of the studies show that LIPUS has a significant inhibitory effect on the mature IL-1β in both in vitro and in vivo settings, as well as an increase in macrophage autophagy levels (30, 31). Furthermore, the research indicates that LIPUS significantly enhances the expression of M2 macrophage-associated genes while suppressing M1 macrophage-related genes, suggesting a potential role in regulating inflammation following PNI. Neurotrophic factors have been shown to protect nerve, axon regeneration, and myelinogenesis following PNI (32, 33). Augmenting the efficacy of NGF could serve as a promising therapeutic strategy for promoting nerve regeneration; studies indicate (34) LIPUS significantly increases NGF-induced neurite lengths, attributed to LIPUS-enhanced ERK1/2 and cAMP activates cyclic AMP response element-binding protein CREB phosphorylation induced by NGF, as well as enhanced expression of Trx-1. The findings indicate that LIPUS may augment NGF-induced neuronal growth by activating the ERK1/2-CREB-Trx-1 signaling pathways through mechanical means.
In summary, LIPUS aids PNI recovery through complex mechanisms such as enhancing glial cell activity, modulating immune responses, and activating nerve growth factors. These actions offer new therapeutic insights for nerve repair. However, more research and clinical trials are necessary to fully assess its clinical effectiveness and optimal application.
4 The impact of electrical stimulation on peripheral nerve injury
4.1 The application of electrical stimulation in peripheral nerve injury
Electrical stimulation (ES) is a viable and efficacious therapeutic modality for PNI, demonstrating considerable potential for promoting nerve regeneration. Extensive research has been conducted on ES as a physical therapy for PNI, with historical roots dating back to the early nineteenth century when it's potential to mitigate functional deficits in affected organs was recognized. In 1841 (35), the study revealed that ES had the capability to elicit muscle contractions in denervated frog legs and mitigate muscle atrophy. Many animal experiments have shown that ES can promote nerve regeneration. In 1952, Hoffman et al. (36) applied ES at frequencies ranging from 50 to 100 Hz to the sciatic nerve of rats with partially severed L5 nerve roots, resulting in an observed increase in axonal sprouting velocity following ES application. It was hypothesized by researchers that ES triggered retrograde signals in neuronal cell bodies, resulting in an expedited axoplasmic synthesis and improved axonal sprouting. ES has demonstrated efficacy in promoting nerve axon regeneration in animal models, and more recently, researchers have successfully translated this approach to human populations with peripheral nerve injuries, yielding favorable therapeutic results. In 2009, Gordon et al. (37) treated patients with median nerve injuries postoperatively with ES. A notable increase in axon regeneration was noted at the 6–8 month mark in comparison to the control group, accompanied by enhancements in MUNE, motor latency, and sensory nerve conduction velocity. In a study conducted in 2020, Power (38) observed that postoperative ES led to improvements in electromyographic indices, grip strength, and pinch strength among patients diagnosed with cubital tunnel syndrome.
With the progression of research into ES for the treatment of PNI, uncertainties associated with ES parameters have also become evident. A 1 h, 20 Hz non-invasive electrical stimulation (ES) is a widely used treatment in animal studies and clinical trials, known for its therapeutic benefits (39, 40). Additionally, Brushart et al. (41) found that this stimulation improved nerve regeneration at the junction but didn't speed up growth. Lu et al. (42) examined transcutaneous ES at 1, 2, 20, and 200 Hz with 1 mA intensity, finding that higher frequencies reduced regeneration. In the 2 Hz group, rats had smaller axonal areas, thicker myelin, higher density, and more blood vessels. Furthermore, the timing and length of ES are crucial elements; nonetheless, research using mouse models (43) showed no notable difference in results between 1 h and 10 min ES sessions (16 Hz, 100 μs). Roh et al. (43) further substantiated these findings, indicating that a 10 min duration of ES is adequate to elicit therapeutic effects comparable to those observed with 1 h ES in mouse models of nerve transection and repair. This data indicates that axonal regrowth might not solely depend on the length of ES, but also on other elements, possibly the number of triggered. Traditionally, ES has been administered immediately following surgical procedures. However, recent investigations have explored the application of ES in preoperative contexts to augment its efficacy. This preoperative application of ES is referred to as Conditioned Electrical Stimulation (CES) (44). In contrast, postoperative ES (PES) has been shown to facilitate nerve regeneration by minimizing misdirection at the neural junction. CES accelerates axonal extension. Senger et al. (45) compared one-week CES with immediate PES before nerve repair surgery and found that CES-treated axons extended further than those in the PES group, with significant improvements in sensory recovery and motor reinnervation, possibly due to reduced inflammation. In 2018, Barber et al. (46) demonstrated that intraoperative 1 h sessions of 20 Hz ES significantly enhanced shoulder function in patients with spinal accessory nerve traction injuries. Additionally, the intervention led to improved electrophysiological scores, thereby underscoring the therapeutic value of perioperative ES application.
4.2 The mechanism of electrical stimulation therapy for peripheral nerve injury
The mechanism by which ES facilitates recovery in PNI is highly intricate, encompassing a multitude of factors. Principally, to establish a regenerative microenvironment, the majority of nerve stimulators position two electrodes at the termini of the injured nerve, thereby generating an intrinsic electric field at the site of injury. Typically, the anode electrode is situated at the proximal end, while the cathode electrode is placed at the distal end. Regeneration-related factors such as NGF are polarized and electrophoretically migrated toward the cathode under the influence of the built-in electric field. This concentration gradient can guide growth cones of nerves toward the cathode, thereby promoting peripheral nerve regeneration. The intrinsic electric field enhances axoplasmic flow (47), thereby promoting the transport of protein synthesis from neuronal cell bodies to nerve terminals. ES influences the neuron's membrane potential, triggering the opening of Ca2+ channels and a subsequent influx of Ca2+ ions (48). This influx then activates several proteins, such as adenylyl cyclase (AC), which in turn stimulates the production of cAMP. cAMP enhances the production of growth-associated protein 43 (GAP-43), actin, and Tα-1 tubulin. Concurrently, CREB through the mediation of protein kinase A (PKA), thereby promoting the assembly of cellular scaffolding and facilitating the elongation of growth cones from proximal remnants (49, 50) increased calcium levels can also trigger the upregulation of BDNF and its receptor, TrkB, which collectively influence various downstream effects of ES. Furthermore, an alternative route explains how ES activates CREB via the p38 MAPK signaling pathway (Figure 2B). Research shows that p38 MAPK activation is crucial for BDNF synthesis in the rat hippocampus (51), highlighting the significant function of p38 MAPK in the nervous system, both centrally and PNS.
SCs play a pivotal role in the recovery of PNI. A substantial number of SCs are longitudinally aligned to form Büngner's bands, which facilitate axonal regeneration. Additionally, SCs are capable of forming myelin and secreting neurotrophic factors, thereby establishing an optimal microenvironment for axonal regeneration (52, 53). Nevertheless, the precise function of SCs at these junctions remains to be fully elucidated (54). Earlier studies have shown that the movement of SCs can be influenced by an external electric field (55), with pronounced anodal movement observed at a field strength of 100 mV/mm (56). Investigations employing RNA-seq have examined the differential gene expression between ES and unstimulated rat SCs (55). Research has pinpointed the MAPK pathway as the most notably enhanced pathway during ES, indicating its vital importance in controlling SCs movement. Furthermore, as previously noted, ES activates the CREB via the MAPK pathway, thereby designating SCs and the MAPK pathway as significant research targets for enhancing PNI recovery through ES. The secretion of NGF is a critical function of SCs in facilitating PNI recovery. Researchers (57) have found that ES can enhance NGF release from cultured SCs, with an electric field (1 Hz, 5 V/cm) increasing NGF release by 4.1 times. Furthermore, pharmacological interventions have shown that ES can induce Ca2+ influx through T-type voltage-gated calcium channels and mobilize calcium ions from both 1,4,5-trisphosphate-sensitive stores and caffeine/ryanodine-sensitive stores, indicating that ES-induced NGF release by SCs is calcium-dependent. As prototypical glial cells in the PNS, SCs actively remove myelin debris via phagocytosis during the early phase of Wallerian degeneration (58). This process constitutes a critical factor that impedes nerve growth, disrupts the regenerative microenvironment, and obstructs myelin regeneration (59). Therefore, the elimination of myelin debris is essential for nerve regeneration. Li et al. (32, 33) have demonstrated that NGF expedites the disintegration of degenerating nerves and facilitates the removal of myelin debris during Wallerian degeneration. These NGF-mediated effects are modulated via the p75NTR/AMPK/mTOR signaling pathway, which enhances SCs autophagy and promotes autophagic flux. Macrophages also play an important role in regulating their phagocytic activity within damaged lesion regions by secreting inflammatory cytokines and chemokines. McLean et al. (60) found that ES can promote macrophage proliferation and largely polarize them toward an anti-inflammatory M2 phenotype, but the molecular mechanisms involved in this transition are not clear. It has been suggested that (61) increased BDNF levels at lesion sites in spinal cord injury patients could polarize macrophages, and we speculate that a similar mechanism operates in the PNS.
In short, ES aids PNI recovery by enhancing axonal regeneration, influencing neuronal and Schwann cell functions, and boosting nerve growth factor release. While treatments are still being refined, ES presents promising prospects for nerve repair.
5 The impact of photobiomodulation on peripheral nerve injury
5.1 The application of photobiomodulation in peripheral nerve injury
Photobiomodulation (PBM) is extensively utilized within the field of rehabilitation medicine. Notably, Low-Level Laser Therapy (LLLT) is frequently employed in the management of PNI (8). Lasers can produce powerful, single-color, coherent, and tightly focused light beams with extremely pure frequencies, making them very useful for biomedical applications (62). Sometimes referred to as PBM, constitutes a form of low-intensity light therapy (63). Medical professionals have effectively utilized PBM to treat a variety of challenging health conditions, including non-healing wounds, chronic diabetic ulcers, spinal and nervous system injuries, and chronic pain management (64). In 1987, Rochkind et al. (65) investigated the effects of LLLT on the sciatic nerve in rats. Subsequent research has demonstrated that transcutaneous PBM therapy facilitates the post-traumatic and post-surgical regeneration of injured nerve fibers (66–69). Over the past few years, scientists have more often employed this technique on individuals with nerve damage with LLLT being favored over other physical treatments, especially for carpal and cubital tunnel syndromes (70). In 2014, Fusakul et al. (71) evaluated the efficacy of LLLT in patients with mild to moderate carpal tunnel syndrome and observed significant improvements in the LLLT treatment group compared to the control group, especially in terms of grip strength and electrophysiological assessments. Tascioglu et al. (72) investigated the effectiveness of LLLT for managing carpal tunnel syndrome. Their findings indicated that LLLT did not demonstrate superior effectiveness compared to the control group in terms of symptom improvement, electromyography, and US parameters. This lack of significant difference may be attributed to potential issues related to the selection of laser parameters.
The selection of appropriate parameters is essential for effective treatment, with primary considerations including wavelength, power density, energy density, and the duration of laser application. A review of both animal studies and clinical trials suggests a preference for laser wavelengths in the range of 630–905 nm, predominantly within the infrared spectrum, and energy densities spanning from single to triple digits (70). In 2004, Gigo et al. (73) carried out research to examine the impact of different laser treatments on rats with severed median nerves. The study utilized three types of laser emissions: continuous wave (808 nm), pulsed wave (905 nm), and a combination of both. Through the examination of muscle mass and nerve morphology in muscles innervated by the median nerve, the researchers determined that the combination of pulsed and continuous laser therapy produced the most favorable functional outcomes. Hsieh et al. (74) explored the effects of LLLT on neuropathic pain in rats with sciatic nerve compression injuries, finding that using 660 nm, 9 J/cm2 of LLLT significantly reduced the overexpression of HIF-1α, TNF-α, and IL-1β, markedly improved the mechanical withdrawal threshold and the functional indices of the sciatic, tibial, and peroneal nerves, and activated VEGF and NGF. Therefore, they identified LLLT as an innovative clinical approach for ameliorating tissue hypoxia/ischemia and inflammation in compressive neuropathies, as well as for facilitating nerve regeneration. In 2014, Wang et al. (75) conducted a comparative study on the effects of three different energy densities (3, 8, and 15 J/cm2) of laser therapy on a rat model of sciatic nerve transection, all with a wavelength of 808 nm, a laser power output of 170 mW, and a power density of 44.7 mW/cm2. By examining the functional status, morphological changes, and neuronal growth in rats, researchers discovered that 808 nm LLLT at lower energy densities (3 J/cm2 and 8 J/cm2) could facilitate post-compression sciatic nerve regeneration.
5.2 The mechanism of photobiomodulation in treating peripheral nerve injuries
Laser therapy, a specialized form of PBM, is characterized by its high directivity, brightness, and monochromaticity. Additionally, it exhibits superior coherence due to the uniform frequency and directionality of the light waves (76). Its biological properties encompass photochemical reactions, thermal effects, pressure effects, and electromagnetic effects, each of which are vital in the regulation of biological tissues (77). In the field of medicine, LLLT is frequently employed as an adjunctive treatment during post-operative rehabilitation to facilitate the recovery of muscular, neural, and joint functions, among other physiological processes (78). LLLT is efficiently absorbed by tissues with minimal attenuation, enabling it to modulate DNA activity and ATP production, thus impacting mitochondrial function. The primary mechanism of laser-induced biological modulation involves the activation of COX within the mitochondrial respiratory chain. The energy derived from absorbed photons during laser treatment augments COX activity, thereby enhancing ATP synthesis. The resultant ATP has the potential to reactivate damaged cells and ameliorate metabolic dysfunctions (79). Experiments have shown that LLLT generates mitochondrial Reactive Oxygen Species (ROS), which in turn trigger the redox-sensitive nuclear transcription factor NF-κB (80) (Figure 2C), a protein that regulates immune responses and enhances synaptic plasticity (81). This process facilitates oxygen entry into cells, thereby restoring cellular respiration and generating reactive oxygen species. Furthermore, LLLT has been shown to alleviate pain and inflammation, prevent tissue necrosis, and effectively reduce nervous system degeneration (82).
Analogous to the effects observed with US and ES, PBM has been shown to promote SCs growth. Li et al. (83) investigated the impact of varying output powers of LLLT at constant wavelengths on the regeneration of rat facial nerves. Their findings indicated that the groups subjected to 250 and 500 mW exhibited enhanced proliferation of SCs, whereas the 1,000 mW group demonstrated an increased rate of SCs apoptosis. It was also determined that the 250 mW laser facilitated Akt phosphorylation through the activation of PI3K, thereby inhibiting SC apoptosis mediated by Akt phosphorylation. These findings are consistent with prior research outcomes (84, 85). Similarly, LLLT has been shown to attenuate the levels of inflammatory mediators, a phenomenon that has also been observed in cases of severe injury to the DRG. Chen et al. (86) demonstrated that LLLT markedly alleviated pain and sensitivity to thermal stimuli in rats subjected to DRG compression. Furthermore, LLLT treatment was associated with a significant reduction in the expression levels of the TNF-α and IL-1β. By enhancing the secretion of chemokine ligand 2 (CCL2) in DRG, Oliveira et al. (87) propose that PBM can modulate macrophage polarization and secretion states. This study concludes that 810 nm PBM facilitates the interaction between neurons and macrophages, resulting in a positive feedback network that accelerates nerve repair. Neurogenic inflammation induces the activation of glial cells within the DRG, resulting in the subsequent production of pro-inflammatory cytokines and chemokines. This process contributes to the development of both peripheral and central sensitization (88). In a rodent model of neuropathic pain, Oliveira et al. (87) observed that PBM led to a reduction in the levels of the nociceptive mediator substance P and TRPV1. Additionally, PBM decreased the temperature of the paws and legs. In PNI, PBM may regulate thermoregulation, neurogenic inflammation, and thermal sensitivity. Zhang et al. (30, 31) demonstrated that LLLT attenuated M1 macrophage-specific markers but enhanced M2 macrophage-specific markers in a rat model of spinal cord injury. This finding substantiates that LLLT facilitates the secretion of diverse neurotrophic factors through the activation of the PKA-CREB pathway in macrophages, thereby promoting axonal regeneration. BDNF and NGF play a critical role in neuronal survival and regeneration. In recent studies (89), 632.8 nm irradiation at a power density of 5 mW and an energy density of 10 J/cm2 significantly enhanced the expression of BDNF and NGF. This upregulation was observed to commence immediately post-irradiation and persisted, with levels continuing to escalate until day 21.
In summary, LLLT shows promise for treating PNI by boosting mitochondrial function, modulating the immune response, and aiding nerve regeneration. Future improvements in its parameters and protocols could enhance its clinical effectiveness.
6 The impact of aerobic exercise on peripheral nerve injury
6.1 The application of aerobic exercise in peripheral nerve injury
Axon regeneration in response to aerobic exercise has been reported to be inconclusive. This inconsistency may be attributed to the lack of standardized treatment protocols, particularly concerning variations in the intensity, duration, and timing of exercise initiation. Using rat muscle creatine content and muscle weight, Hines (90) showed that physical activity can accelerate and improve recovery from peripheral nerve paralysis in 1942. Aerobic exercise, a prevalent form of physical activity, has since been extensively investigated for its effects on PNI rehabilitation. In 1974, Herbison et al. (91) conducted a study on neurohistological outcomes in rats, concluding that high-intensity swimming (2 h per day) may not facilitate the repair of reinnervated muscles. They further suggested that engaging in high-load exercise during the early stages of reinnervation could pose significant risks. Clinical applications and translational research pertaining to aerobic exercise have been investigated. Notably, in 2003, Mennen et al. (92) demonstrated that sensory retraining combined with exercise contraction practices following nerve injury surgery in humans led to enhanced functional recovery.
The mode of aerobic exercise, the timing of exercise initiation, and the intensity of exercise constitute significant areas of contemporary research interest. In a 2017 study, Arbat et al. (93) investigated the impact of forced, passive, and voluntary exercise on PNI. Their findings indicated that exercise intensity, rather than the mode of exercise, prevents motor neuron synaptic stripping following axonal rupture. In 2022, Bai et al. (94) conducted a study on the timing of exercise initiation, specifically analyzing the functional recovery of nerve-injured rats subjected to low-intensity, late-initiated treadmill exercise. Their findings indicate that late-initiated treadmill exercise facilitates sensory motor function recovery, prevents muscle atrophy, and promotes nerve regeneration following autologous transplantation repair of long-gap nerve transection. In 2023, Camuzard et al. (95) classified animal models into four distinct groups: continuous exercise, preoperative exercise, postoperative exercise, and no exercise. Their findings indicated that continuous or early exercise markedly enhances functional outcomes by directly influencing muscle innervation, although it exerts minimal effects on nerve regeneration. In 2022, Di Palma et al. (96) conducted a study on nerve-injured rats, categorizing them into groups based on exercise intensity: fast running, slow running, and intermittent running. Moderate-intensity intermittent running significantly enhances functional recovery by promoting axon sprouting and inducing muscle autophagy, thus stabilizing muscle-nerve interactions.
6.2 The mechanisms of aerobic exercise on peripheral nerve injury
PNI results in a 70% decrease in skeletal muscle cross-sectional area within a 2-month period (97). Furthermore, PNI can result in the atrophy of sensory receptors, leading to subsequent sensory deficits. Additionally, PNI not only leads to localized spinal cord lesions, but it also triggers apoptosis of motor neurons. This neuronal apoptosis is attributed to the impaired transfer of essential nutrient factors to the neuron cell bodies following the injury (98). Lower motor neurons in the spinal cord are less efficient at transmitting information to upper motor neurons in the cerebral cortex as a result of apoptosis of anterior horn motor neurons. Consequently, motor commands originating from the brain fail to reach target organs, such as skeletal muscles, and peripheral sensory information is not conveyed to the brain. This disruption results in impaired cortical sensitivity and motor function (99). EEG findings indicate (100) a reduction in cortical sensitivity among patients with PNI. Aerobic exercise has been demonstrated to enhance blood flow velocity, improve oxygen delivery, stimulate the release of neurotrophic factors, and activate neuronal pathways. Consequently, these physiological changes contribute to systemic improvements in the function of organs, the spinal cord, and the brain (Figure 3), thereby facilitating recovery from injury and enhancing overall functional capacity.
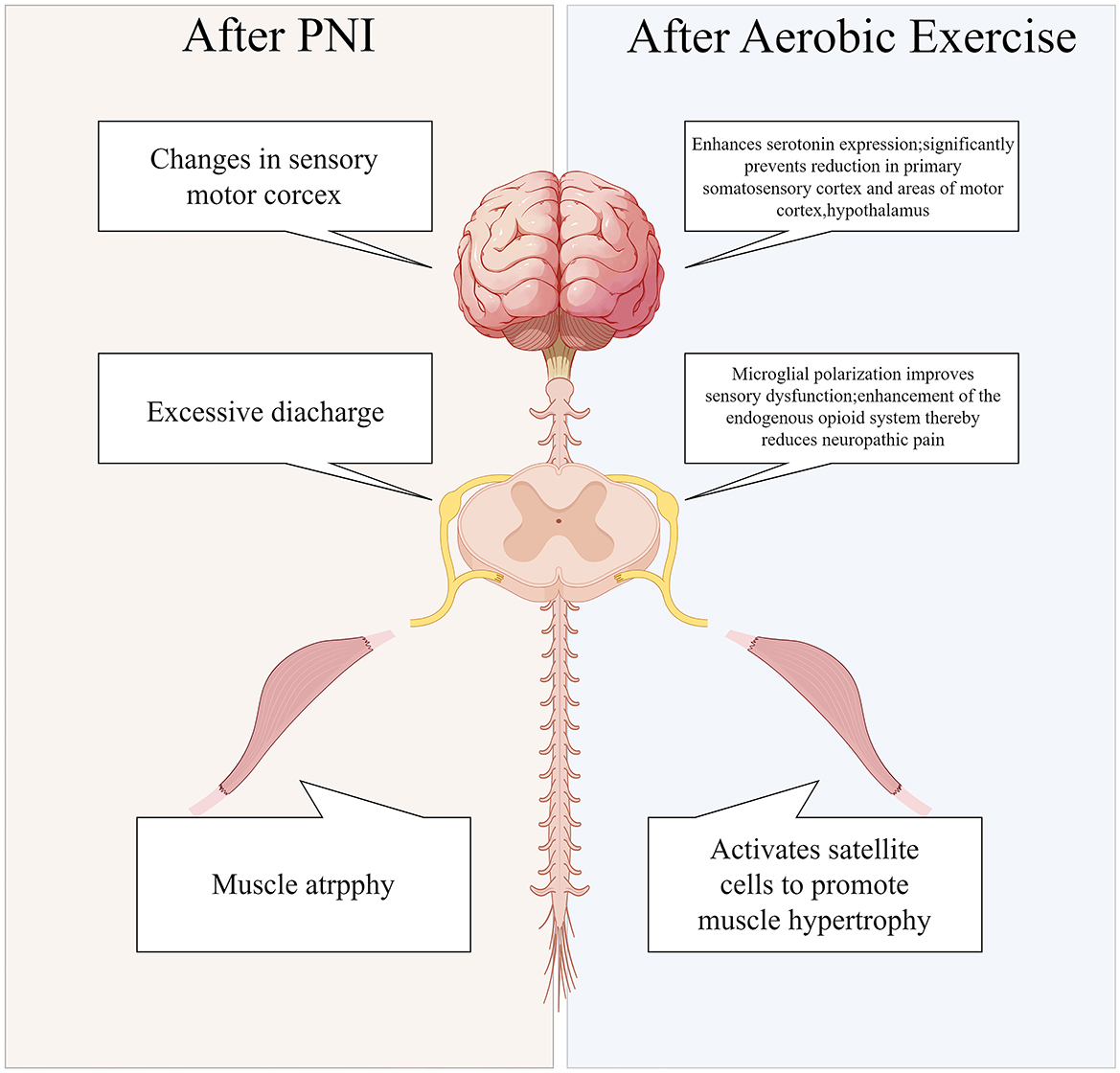
Figure 3. Systemic alterations in peripheral nerve injuries pre-and post-aerobic exercise intervention.
A considerable body of evidence consistently demonstrates that aerobic exercise markedly enhances the neuroimmune response in cases of traumatic PNI (101). Aerobic exercise can (102) significantly shift macrophages from a pro-inflammatory to an anti-inflammatory phenotype, thereby reducing the levels of pro-inflammatory cytokines and alleviating adverse reactions following PNI. Pro-inflammatory cytokines are frequently released at the site of post-traumatic PNI. Findings indicate a significant reduction in the levels of TNF-α following aerobic exercise. These findings indicate that aerobic exercise is pivotal in modulating anti-inflammatory immune responses. Consequently, aerobic exercise exerts substantial anti-inflammatory effects through the regulation of macrophage polarization and TNF-α modulation. As well as providing key insights into the development of targeted rehabilitation programs for PNI, this research provides a theoretical foundation for the application of exercise rehabilitation in the treatment of PNI. Following PNI, BDNF is secreted by activated microglia in the spinal cord. The elevated levels of BDNF subsequently modulate GABA neuronal inhibition, resulting in sensory hypersensitivity (103). Following aerobic exercise, in mice, BDNF expression in the dorsal horn of the spinal cord is reduced (104), which subsequently reverses microglial activation and enhances autophagy flux. Furthermore, as a result of neural injury induced by exercise training, BDNF modulates autophagy via the AKT/mTOR signaling pathway, and polarizes spinal cord microglia (94). In conclusion, exercise training has been demonstrated to effectively mitigate pain-related behaviors in mice. Furthermore, it has been observed that exercise training attenuates the expression of BDNF in the spinal cord dorsal horn following neural injury, thereby reversing sensory hypersensitivity. Additionally, aerobic exercise has been associated with a reduction in levels of GFAP, a marker of astrocytes (105). The promotion of autophagy within glial cells seems to be supported by aerobic exercise. Furthermore, Physical activity has also been shown to enhance serotonin production (106), a neurotransmitter that plays a crucial role in regulating emotions, sleep, and overall brain function, and is also implicated in post-PNI analgesic mechanisms. Despite this, aerobic exercise does not appear to affect BDNF levels consistently across studies. In human research, the majority of experiments indicate that aerobic exercise elevates serum BDNF concentrations (107, 108). Nevertheless, BDNF levels have been reported to be reduced or unchanged following aerobic exercise in a smaller subset of studies (109, 110). The observed trend in BDNF variation appears to exhibit a gender-related pattern. In comparison to control groups, aerobic exercise is associated with an increase in BDNF levels in male patients, while it is associated with a decrease in BDNF levels in female patients (111). Research indicates that testosterone can modulate the expression of BDNF and its receptor, TrkB, in motor neurons. Exercise has been shown to induce a sustained elevation in testosterone levels, which may subsequently result in prolonged upregulation of BDNF and TrkB expression. A similar phenomenon has been observed in ES treatment for PNI (112), where it was found that the related neural regeneration cellular mechanisms require androgen receptor signaling. Empirical evidence from animal studies corroborates these findings, as a result of aerobic exercise, serum BDNF concentrations significantly increased (113–115).
Aerobic exercise significantly impacts PNI rehabilitation by regulating neuroimmune responses, reducing inflammation, enhancing neuroglial autophagy, promoting neuronal activation through increased neurotrophic factor release, and improving blood flow and oxygen supply. Despite some debate over BDNF trends, aerobic exercise is crucial in PNI recovery and supports the creation of personalized rehabilitation programs.
7 The application of other physical therapies in peripheral nerve injury
Table 2 summarizes several studies with significant impact on PNI treatment from the four physical methods discussed earlier. When it comes to rehabilitation therapy for PNI, in addition to well-known physical therapies such as US, ES, PBM, and aerobic exercise, new technologies and methods have emerged in recent years that have shown beneficial treatment effects in clinical practice (Table 3).
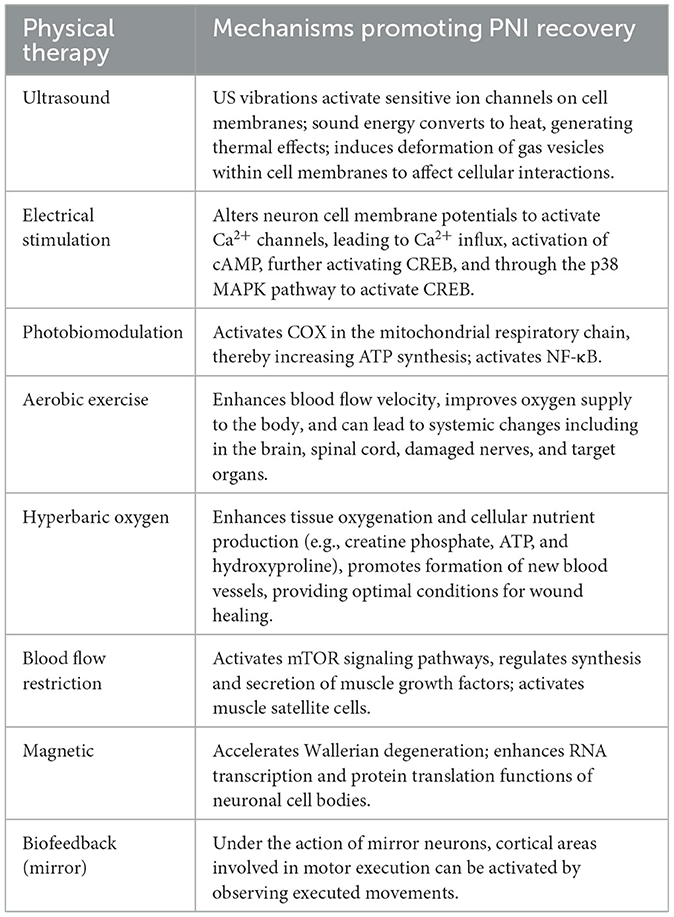
Table 3. An overview of the mechanisms through which various physical therapy modalities facilitate PNI recovery.
Oxygen is essential for the proper functioning of peripheral nerves, as it is required by nerve cells for energy production within mitochondria (116). Traumatic PNI disrupts the blood supply, thereby diminishing oxygen delivery to the nerves and adjacent tissues. The initial manifestations of local acute ischemia initiate a series of cascade reactions that facilitate angiogenesis (116), inflammation, and cell proliferation. Hyperbaric oxygen therapy (HBOT) augments tissue oxygenation and facilitates the synthesis of cellular nutrients, including phosphocreatine, ATP, and hydroxyproline, thereby promoting angiogenesis and creating optimal conditions for wound healing. Experimental studies have demonstrated that this therapeutic approach is advantageous for peripheral nerve regeneration, attributable to its intrinsic physical properties. Bilsev et al. (117) examined the efficacy of HBOT in patients with upper limb nerve injuries following nerve repair. Their findings indicated superior functional recovery and enhanced electrophysiological outcomes in the HBOT group compared to the control group.
Another therapeutic approach, blood flow restriction (BFR), was employed by researchers who applied a pressure of 130–170 mmHg to the upper arm of a pianist suffering from an upper limb nerve injury (118). A significant increase in forearm and upper arm girth, as well as improved grip strength, was observed after this intervention, following the performance. BFR exercise has the potential to significantly enhance the recovery of residual nerve symptoms in pianists. A critical component of this mechanism is the activation of mTOR signaling (119), which contributes to muscle growth factor secretion and synthesis. Additionally, BFR exercise promotes skeletal muscle hypertrophy through the activation of satellite cells (120).
Emerging evidence indicates that magnetic fields exert a substantial influence on neuronal development. Pulsed magnetic fields (PMF) have been shown to promote nerve regeneration more safely and effectively than static magnetic fields (SMF) (121). Pulsed electromagnetic fields (PEMFs) have been shown to accelerate Wallerian degeneration in distal nerve remnants, thereby creating a conducive microenvironment for the rapid growth of proximal axons into the bands of Büngner. PEMF facilitates RNA transcription and protein synthesis within nerve cell bodies, accelerates the cytoplasmic transport of structural proteins, and promotes axonal regeneration by concentrating neurotrophic factors at injury sites and distal nerve segments. Additionally, PEMF inhibits the formation of collagen fibers, thereby reducing mechanical resistance to axonal regeneration (122). Bademoglu et al. (123) investigated the short-term and long-term electrophysiological and functional regeneration effects of PEMF on sciatic nerve injuries. Their findings indicated significantly greater functional improvements in the intervention groups during weeks 1 and 2 compared to the control groups. However, the effects were not statistically significant in weeks 2, 4, and 6, suggesting that PEMF may be particularly beneficial during the initial stages of recovery (124). Establishing parameters for magnetic stimulation presents significant challenges, given that intense magnetic fields have the potential to harm cellular structures, leading to genetic mutations and DNA damage.
Biofeedback therapy (BFT) presents physiological conditions in readily interpretable visual and auditory formats, thereby facilitating conscious mental control and psychological training to modulate abnormal physiological responses for the regulation of bodily functions, as well as for the prevention and treatment of diseases. In recent years, mirror feedback therapy, a more straightforward variant of biofeedback therapy, has been employed in the management of patients with PNI. The principle posits that cortical regions implicated in motor execution can be activated through the observation of action execution, a phenomenon attributed to the functionality of the mirror neuron system (MNS). This activation has been shown to influence sensory and motor control following PNI (125). Hsu and others (125, 126) conducted a 12-week intervention on patients with injuries ranging from the elbow to the mid-palm. Their findings indicate that mirror therapy may result in superior therapeutic outcomes in terms of hand movement and functional performance for patients with nerve injuries, compared to traditional sensory reeducation treatments. However, it is noteworthy that no significant improvement in sensory function was observed.
8 Conclusions and future prospects
An overview of the historical development and therapeutic applications of US, ES, PBM, and aerobic exercise in the treatment of PNI. It delves into the mechanisms of action and their effects on neuroglial cells, NGF, and the immune system. These physical therapies collectively demonstrate the capacity to promote neuroglial cell proliferation, modulate inflammatory responses, and enhance the secretion of NGF. Nevertheless, the signaling pathways through which they facilitate nerve regeneration may differ, necessitating further investigation into their specific mechanisms.
However, each modality of physical therapy presents specific limitations. For example, in the case of US therapy, the variability in soft tissue types, injury patterns, and tissue thicknesses complicates the standardization of its parameters for application in human nerve injuries. And there is a paucity of literature demonstrating the efficacy of US in enhancing sensory function. In addition, the inertial cavitation of high-intensity US may cause irreversible damage to peripheral nerve axons (127). ES for PNI encompasses various methodologies, including traditional approaches such as transcutaneous electrical nerve stimulation (TENS) and implanted ES. TENS is constrained by limited penetration and suboptimal targeting (128), whereas implanted stimulation is associated with high costs and complex surgical procedures. The commonly used 20 Hz low-frequency therapy in clinical practice is ineffective at stimulating deep nerves, despite its ability to activate neuronal activity. In contrast, medium-frequency (1,000–100,000 Hz) electrical stimulation can penetrate soft tissue without causing discomfort. Therefore, interferential current is an ideal option for treating peripheral nerve injuries (129), as it passes through the skin and generates a targeted low-frequency envelope that precisely stimulates the injury site. PBM encompasses intricate irradiation parameters, techniques, methods, and treatment durations, yet it is constrained by limited clinical research. Furthermore, PBM frequently encounters challenges in penetrating human skin, thereby complicating the identification of appropriate phototherapy applications for PNI regeneration and functional recovery (70). The majority of clinical treatments focus on conditions such as carpal tunnel syndrome and cubital tunnel syndrome, which affect the skin's superficial layers. Most previous studies investigating aerobic exercise in the context of PNI have predominantly utilized animal models. Consequently, future research needs to evaluate the applicability of these findings to human subjects. Among PNI patients, particularly those suffering from traumatic injuries, adherence to aerobic exercise regimens remains low. This is further complicated by ongoing debates regarding the optimal parameters and timing of such interventions. Furthermore, physical factor therapies facilitate the promotion of various NGF, such as BDNF, which contribute to the establishment of conducive microenvironments for nerve regeneration. Recent studies suggest that cortical remodeling and somatosensory hypersensitivity following PNI may be associated with these neurotrophic factors (130).
Following nerve injury, spinal dorsal horn BDNF levels are upregulated, enhancing pro-inflammatory responses and pain sensitivity. These observations indicate that the restoration of neurotrophic factor levels via physical exercise may elicit anti-inflammatory effects. Austin et al. (131) observed a significant reduction in spinal BDNF levels in animals subjected to exercise compared to control groups, suggesting that aerobic exercise may confer beneficial effects through the observed decrease in BDNF levels. Consequently, the integration of aerobic exercise with physical factor therapies is recommended. Extensive research has demonstrated that aerobic exercise exerts positive effects on neuroimmunity and analgesia, consistently resulting in reduced inflammation and alleviated symptoms of pain hypersensitivity. Thus, aerobic exercise functions as an intervention to promote early immune responses and mitigate late-stage sensory abnormalities in the treatment of PNI. Integrating various physical therapy modalities can significantly improve treatment outcomes. For example, in cases of long-segment nerve injuries, postoperative swimming training has been demonstrated to enhance upper limb muscle function and volume in rats subjected to 20 mm brachial plexus nerve transplantation (95), although it exerts minimal effects on nerve tissue morphology. Combining aerobic exercise with physical factor therapy has the potential to synergistically enhance functional recovery and nerve regeneration. In a similar vein, addressing the limitations associated with the low penetration of TENS and PBM, we speculate that combining coherent ES with high penetration and US therapy could yield superior results.
Future research can enhance physical therapy for peripheral nerve injury by: (1) optimizing experimental design and standardization to improve research comparability and effectiveness; (2) exploring multimodal therapies, such as combining ultrasound, electrical stimulation, phototherapy, and exercise to boost nerve repair; and (3) conducting in-depth studies on the molecular and cellular mechanisms of nerve repair, focusing on nerve growth factors and inflammation. These efforts will refine therapy strategies and support clinical application.
Author contributions
X-LC: Writing – review & editing. X-XZ: Writing – original draft, Writing – review & editing. S-YL: Writing – review & editing. Y-JL: Writing – review & editing. ND: Writing – review & editing. M-QL: Writing – review & editing. Q-WL: Writing – review & editing. QL: Writing – review & editing.
Funding
The author(s) declare that financial support was received for the research and/or publication of this article. This work was supported by Natural Science Foundation of Tianjin City (22JCYBJC00210), Project leader: QL; Natural Science Foundation of Tianjin City (22JCYBJC00220), Project leader: X-LC.
Conflict of interest
The authors declare that the research was conducted in the absence of any commercial or financial relationships that could be construed as a potential conflict of interest.
Generative AI statement
The author (s) declare that no Gen AI was used in the creation of this manuscript.
Publisher's note
All claims expressed in this article are solely those of the authors and do not necessarily represent those of their affiliated organizations, or those of the publisher, the editors and the reviewers. Any product that may be evaluated in this article, or claim that may be made by its manufacturer, is not guaranteed or endorsed by the publisher.
References
1. Li J, Yao Y, Wang Y, Xu J, Zhao D, Liu M, et al. Modulation of the crosstalk between schwann cells and macrophages for nerve regeneration: a therapeutic strategy based on a multifunctional tetrahedral framework nucleic acids system. Adv Mater. (2022) 34:e2202513. doi: 10.1002/adma.202270320
2. Lavorato A, Raimondo S, Boido M, Muratori L, Durante G, Cofano F, et al. Mesenchymal stem cell treatment perspectives in peripheral nerve regeneration: systematic review. Int J Mol Sci. (2021) 22:572. doi: 10.3390/ijms22020572
3. Li C, Liu SY, Pi W, Zhang PX. Cortical plasticity and nerve regeneration after peripheral nerve injury. Neural Regen Res. (2021) 16:1518–23. doi: 10.4103/1673-5374.303008
4. Karsy M, Watkins R, Jensen MR, Guan J, Brock AA, Mahan MA, et al. Trends and cost analysis of upper extremity nerve injury using the national (nationwide) inpatient sample. World Neurosurg. (2019) 123:e488–500. doi: 10.1016/j.wneu.2018.11.192
5. Hussain G, Wang J, Rasul A, Anwar H, Qasim M, Zafar S, et al. Current status of therapeutic approaches against peripheral nerve injuries: a detailed story from injury to recovery. Int J Biol Sci. (2020) 16:116–34. doi: 10.7150/ijbs.35653
6. Feng B, Chen L, Ilham SJ. A review on ultrasonic neuromodulation of the peripheral nervous system: enhanced or suppressed activities? Appl Sci-Basel. (2019) 9:1637. doi: 10.3390/app9081637
7. Hu M, Hong L, Liu C, Hong S, He S, Zhou M, et al. Electrical stimulation enhances neuronal cell activity mediated by schwann cell derived exosomes. Sci Rep. (2019) 9:4206. doi: 10.1038/s41598-019-41007-5
8. Rosso MPDO, Buchaim DV, Kawano N, Furlanette G, Pomini KT, Buchaim RL, et al. Photobiomodulation therapy (pbmt) in peripheral nerve regeneration: a systematic review. Bioengineering. (2018) 5:44. doi: 10.3390/bioengineering5020044
9. Acheta J, Stephens S, Belin S, Poitelon Y. Therapeutic low-intensity ultrasound for peripheral nerve regeneration—a schwann cell perspective. Front Cell Neurosci. (2021) 15:812588. doi: 10.3389/fncel.2021.812588
10. Liu X, Zou D, Hu Y, He Y, Lu J. Research progress of low-intensity pulsed ultrasound in the repair of peripheral nerve injury. Tissue Eng Part B Rev. (2023) 29:414–28. doi: 10.1089/ten.teb.2022.0194
11. Tyler WJ, Lani SW, Hwang GM. Ultrasonic modulation of neural circuit activity. Curr Opin Neurobiol. (2018) 50:222–31. doi: 10.1016/j.conb.2018.04.011
12. Jeremias JS, Camanho GL, Bassit AC, Forgas A, Ingham SJ, Abdalla RJ, et al. Low-intensity pulsed ultrasound accelerates healing in rat calcaneus tendon injuries. J Orthop Sports Phys Ther. (2011) 41:526–31. doi: 10.2519/jospt.2011.3468
13. Lai WC, Iglesias BC, Mark BJ, Wang D. Low-intensity pulsed ultrasound augments tendon, ligament, and bone-soft tissue healing in preclinical animal models: a systematic review. Arthroscopy. (2021) 37:2318–33. doi: 10.1016/j.arthro.2021.02.019
14. Fry FJ, Ades HW, Fry WJ. Production of reversible changes in the central nervous system by ultrasound. Science. (1958) 127:83–4. doi: 10.1126/science.127.3289.83
15. Tyler WJ, Tufail Y, Finsterwald M, Tauchmann ML, Olson EJ, Majestic C, et al. Remote excitation of neuronal circuits using low-intensity, low-frequency ultrasound. PLoS ONE. (2008) 3:e3511. doi: 10.1371/journal.pone.0003511
16. Crisci AR, Ferreira AL. Low-intensity pulsed ultrasound accelerates the regeneration of the sciatic nerve after neurotomy in rats. Ultrasound Med Biol. (2002) 28:1335–41. doi: 10.1016/S0301-5629(02)00576-8
17. Kawai H, Ito A, Kawaguchi A, Nagai-Tanima M, Nakahara R, Xu S, et al. Ultrasound therapy for a week promotes regeneration and reduces pro-inflammatory macrophages in a rat sciatic nerve autograft model. Sci Rep. (2023) 13:11494. doi: 10.1038/s41598-023-38630-8
18. Chen WZ, Qiao H, Zhou W, Wu J, Wang ZB. Upgraded nerve growth factor expression induced by low-intensity continuous-wave ultrasound accelerates regeneration of neurotometicly injured sciatic nerve in rats. Ultrasound Med Biol. (2010) 36:1109–17. doi: 10.1016/j.ultrasmedbio.2010.04.014
19. Akhlaghi Z, Mobarakeh JI, Mokhtari M, Behnam H, Rahimi AA, Khajeh HM, et al. The effects of altered ultrasound parameters on the recovery of sciatic nerve injury. Iran Biomed J. (2012) 16:107–12. doi: 10.6091/ibj.942.2012
20. Jiang W, Wang Y, Tang J, Peng J, Wang Y, Guo Q, et al. Low-intensity pulsed ultrasound treatment improved the rate of autograft peripheral nerve regeneration in rat. Sci Rep. (2016) 6:22773. doi: 10.1038/srep22773
21. Ito A, Wang T, Nakahara R, Kawai H, Nishitani K, Aoyama T, et al. Ultrasound therapy with optimal intensity facilitates peripheral nerve regeneration in rats through suppression of pro-inflammatory and nerve growth inhibitor gene expression. PLoS ONE. (2020) 15:e234691. doi: 10.1371/journal.pone.0234691
22. Zhong YX, Liao JC, Liu X, Tian H, Deng LR, Long L, et al. Low intensity focused ultrasound: a new prospect for the treatment of parkinson's disease. Ann Med. (2023) 55:2251145. doi: 10.1080/07853890.2023.2251145
23. Baker KG, Robertson VJ, Duck FA. A review of therapeutic ultrasound: biophysical effects. Phys Ther. (2001) 81:1351–8. doi: 10.1093/ptj/81.7.1351
24. Lu CT, Zhao YZ, Wu Y, Tian XQ, Li WF, Huang PT, et al. Experiment on enhancing antitumor effect of intravenous epirubicin hydrochloride by acoustic cavitation in situ combined with phospholipid-based microbubbles. Cancer Chemother Pharmacol. (2011) 68:343–8. doi: 10.1007/s00280-010-1489-4
25. Li P, Zhang J, Li F, Yu Y, Chen Y. Low-intensity ultrasound enhances the chemosensitivity of hepatocellular carcinoma cells to cisplatin via altering the mir-34a/c-met axis. Int J Mol Med. (2019) 44:135–44. doi: 10.3892/ijmm.2019.4205
26. Ren C, Chen X, Du N, Geng S, Hu Y, Liu X, et al. Low-intensity pulsed ultrasound promotes schwann cell viability and proliferation via the gsk-3beta/beta-catenin signaling pathway. Int J Biol Sci. (2018) 14:497–507. doi: 10.7150/ijbs.22409
27. Ren C, Li JM, Lin X. Lipus enhance elongation of neurites in rat cortical neurons through inhibition of gsk-3beta. Biomed Environ Sci. (2010) 23:244–9. doi: 10.1016/S0895-3988(10)60059-1
28. Kim WY, Zhou FQ, Zhou J, Yokota Y, Wang YM, Yoshimura T, et al. Essential roles for gsk-3s and gsk-3-primed substrates in neurotrophin-induced and hippocampal axon growth. Neuron. (2006) 52:981–96. doi: 10.1016/j.neuron.2006.10.031
29. Ventre DM, Cluff A, Gagnon C, Diaz VD, Koppes RA, Koppes AN, et al. The effects of low intensity focused ultrasonic stimulation on dorsal root ganglion neurons and schwann cells in vitro. J Neurosci Res. (2021) 99:374–91. doi: 10.1002/jnr.24700
30. Zhang B, Chen H, Ouyang J, Xie Y, Chen L, Tan Q, et al. Sqstm1-dependent autophagic degradation of pkm2 inhibits the production of mature il1b/il-1beta and contributes to lipus-mediated anti-inflammatory effect. Autophagy. (2020) 16:1262–78. doi: 10.1080/15548627.2019.1664705
31. Zhang J, Sun J, Zheng Q, Hu X, Wang Z, Liang Z, et al. Low-level laser therapy 810-nm up-regulates macrophage secretion of neurotrophic factors via pka-creb and promotes neuronal axon regeneration in vitro. J Cell Mol Med. (2020) 24:476–87. doi: 10.1111/jcmm.14756
32. Li R, Li DH, Zhang HY, Wang J, Li XK, Xiao J, et al. Growth factors-based therapeutic strategies and their underlying signaling mechanisms for peripheral nerve regeneration. Acta Pharmacol Sin. (2020) 41:1289–300. doi: 10.1038/s41401-019-0338-1
33. Li R, Li D, Wu C, Ye L, Wu Y, Yuan Y, et al. Nerve growth factor activates autophagy in schwann cells to enhance myelin debris clearance and to expedite nerve regeneration. Theranostics. (2020) 10:1649–77. doi: 10.7150/thno.40919
34. Zhao L, Feng Y, Hu H, Shi A, Zhang L, Wan M, et al. Low-intensity pulsed ultrasound enhances nerve growth factor-induced neurite outgrowth through mechanotransduction-mediated erk1/2-creb-trx-1 signaling. Ultrasound Med Biol. (2016) 42:2914–25. doi: 10.1016/j.ultrasmedbio.2016.07.017
35. Tian T, Moore AM, Ghareeb PA, Boulis NM, Ward PJ. A perspective on electrical stimulation and sympathetic regeneration in peripheral nerve injuries. Neurotrauma Rep. (2024) 5:172–80. doi: 10.1089/neur.2023.0133
36. Hoffman H. Acceleration and retardation of the process of axon-sprouting in partially devervated muscles. Aust J Exp Biol Med Sci. (1952) 30:541–66. doi: 10.1038/icb.1952.52
37. Gordon T, Amirjani N, Edwards DC, Chan KM. Brief post-surgical electrical stimulation accelerates axon regeneration and muscle reinnervation without affecting the functional measures in carpal tunnel syndrome patients. Exp Neurol. (2010) 223:192–202. doi: 10.1016/j.expneurol.2009.09.020
38. Power HA, Morhart MJ, Olson JL, Chan KM. Postsurgical electrical stimulation enhances recovery following surgery for severe cubital tunnel syndrome: a double-blind randomized controlled trial. Neurosurgery. (2020) 86:769–77. doi: 10.1093/neuros/nyz322
39. Al-Majed AA, Neumann CM, Brushart TM, Gordon T. Brief electrical stimulation promotes the speed and accuracy of motor axonal regeneration. J Neurosci. (2000) 20:2602–8. doi: 10.1523/JNEUROSCI.20-07-02602.2000
40. Singh B, Xu QG, Franz CK, Zhang R, Dalton C, Gordon T, et al. Accelerated axon outgrowth, guidance, and target reinnervation across nerve transection gaps following a brief electrical stimulation paradigm. J Neurosurg. (2012) 116:498–512. doi: 10.3171/2011.10.JNS11612
41. Brushart TM, Hoffman PN, Royall RM, Murinson BB, Witzel C, Gordon T, et al. Electrical stimulation promotes motoneuron regeneration without increasing its speed or conditioning the neuron. J Neurosci. (2002) 22:6631–8. doi: 10.1523/JNEUROSCI.22-15-06631.2002
42. Lu MC, Ho CY, Hsu SF, Lee HC, Lin JH, Yao CH, et al. Effects of electrical stimulation at different frequencies on regeneration of transected peripheral nerve. Neurorehabil Neural Repair. (2008) 22:367–73. doi: 10.1177/1545968307313507
43. Roh J, Schellhardt L, Keane GC, Hunter DA, Moore AM, Snyder-Warwick AK, et al. Short-duration, pulsatile, electrical stimulation therapy accelerates axon regeneration and recovery following tibial nerve injury and repair in rats. Plast Reconstr Surg. (2022) 149:681e−90e. doi: 10.1097/PRS.0000000000008924
44. Juckett L, Saffari TM, Ormseth B, Senger JL, Moore AM. The effect of electrical stimulation on nerve regeneration following peripheral nerve injury. Biomolecules. (2022) 12:1856. doi: 10.3390/biom12121856
45. Senger JB, Chan A, Chan KM, Kwan-Wong T, Acton L, Olson J, et al. Conditioning electrical stimulation is superior to postoperative electrical stimulation in enhanced regeneration and functional recovery following nerve graft repair. Neurorehabil Neural Repair. (2020) 34:299–308. doi: 10.1177/1545968320905801
46. Barber B, Seikaly H, Ming CK, Beaudry R, Rychlik S, Olson J, et al. Intraoperative brief electrical stimulation of the spinal accessory nerve (best spin) for prevention of shoulder dysfunction after oncologic neck dissection: a double-blinded, randomized controlled trial. J Otolaryngol-Head Neck Surg. (2018) 47:7. doi: 10.1186/s40463-017-0244-9
47. Kanbayashi T, Yamauchi T, Miyaji Y, Sonoo M. Interaction of cathodal and anodal stimulations in nerve conduction studies. Muscle Nerve. (2019) 59:713–6. doi: 10.1002/mus.26467
48. Knott EP, Assi M, Pearse DD. Cyclic amp signaling: a molecular determinant of peripheral nerve regeneration. Biomed Res Int. (2014) 2014:651625. doi: 10.1155/2014/651625
49. Gumy LF, Yeo GS, Tung YC, Zivraj KH, Willis D, Coppola G, et al. Transcriptome analysis of embryonic and adult sensory axons reveals changes in mrna repertoire localization. RNA. (2011) 17:85–98. doi: 10.1261/rna.2386111
50. Chu XL, Song XZ, Li Q, Li YR, He F, Gu XS, et al. Basic mechanisms of peripheral nerve injury and treatment via electrical stimulation. Neural Regen Res. (2022) 17:2185–93. doi: 10.4103/1673-5374.335823
51. Katoh-Semba R, Kaneko R, Kitajima S, Tsuzuki M, Ichisaka S, Hata Y, et al. Activation of p38 mitogen-activated protein kinase is required for in vivo brain-derived neurotrophic factor production in the rat hippocampus. Neuroscience. (2009) 163:352–61. doi: 10.1016/j.neuroscience.2009.06.011
52. Gomez-Sanchez JA, Patel N, Martirena F, Fazal SV, Mutschler C, Cabedo H, et al. Emerging role of hdacs in regeneration and ageing in the peripheral nervous system: repair schwann cells as pivotal targets. Int J Mol Sci. (2022) 23:2996. doi: 10.3390/ijms23062996
53. Ramesh R, Manurung Y, Ma KH, Blakely TJ, Won S, Moreno-Ramos OA, et al. Jun regulation of injury-induced enhancers in schwann cells. J Neurosci. (2022) 42:6506–17. doi: 10.1523/JNEUROSCI.2533-21.2022
54. Alvarez-Suarez P, Gawor M, Proszynski TJ. Perisynaptic schwann cells—the multitasking cells at the developing neuromuscular junctions. Semin Cell Dev Biol. (2020) 104:31–8. doi: 10.1016/j.semcdb.2020.02.011
55. Yao L, Li Y, Knapp J, Smith P. Exploration of molecular pathways mediating electric field-directed schwann cell migration by rna-seq. J Cell Physiol. (2015) 230:1515–24. doi: 10.1002/jcp.24897
56. Mckasson MJ, Huang L, Robinson KR. Chick embryonic schwann cells migrate anodally in small electrical fields. Exp Neurol. (2008) 211:585–7. doi: 10.1016/j.expneurol.2008.02.015
57. Huang J, Ye Z, Hu X, Lu L, Luo Z. Electrical stimulation induces calcium-dependent release of ngf from cultured schwann cells. Glia. (2010) 58:622–31. doi: 10.1002/glia.20951
58. Gomez-Sanchez JA, Carty L, Iruarrizaga-Lejarreta M, Palomo-Irigoyen M, Varela-Rey M, Griffith M, et al. Schwann cell autophagy, myelinophagy, initiates myelin clearance from injured nerves. J Cell Biol. (2015) 210:153–68. doi: 10.1083/jcb.201503019
59. Brosius LA, Chung WS, Sloan SA, Carson GA, Zhou L, Lovelett E, et al. Schwann cells use tam receptor-mediated phagocytosis in addition to autophagy to clear myelin in a mouse model of nerve injury. Proc Natl Acad Sci U S A. (2017) 114:E8072–80. doi: 10.1073/pnas.1710566114
60. Mclean NA, Verge VM. Dynamic impact of brief electrical nerve stimulation on the neural immune axis-polarization of macrophages toward a pro-repair phenotype in demyelinated peripheral nerve. Glia. (2016) 64:1546–61. doi: 10.1002/glia.23021
61. Ji XC, Dang YY, Gao HY, Wang ZT, Gao M, Yang Y, et al. Local injection of lenti-bdnf at the lesion site promotes m2 macrophage polarization and inhibits inflammatory response after spinal cord injury in mice. Cell Mol Neurobiol. (2015) 35:881–90. doi: 10.1007/s10571-015-0182-x
62. Fonseca AS, Moreira TO, Paixao DL, Farias FM, Guimaraes OR, de Paoli S, et al. Effect of laser therapy on dna damage. Lasers Surg Med. (2010) 42:481–8. doi: 10.1002/lsm.20921
63. Cotler HB, Chow RT, Hamblin MR, Carroll J. The use of low level laser therapy (lllt) for musculoskeletal pain. MOJ Orthop Rheumatol. (2015) 2:00068. doi: 10.15406/mojor.2015.02.00068
64. Mussttaf RA, Jenkins D, Jha AN. Assessing the impact of low level laser therapy (lllt) on biological systems: a review. Int J Radiat Biol. (2019) 95:120–43. doi: 10.1080/09553002.2019.1524944
65. Rochkind S, Barrnea L, Razon N, Bartal A, Schwartz M. Stimulatory effect of he-ne low dose laser on injured sciatic nerves of rats. Neurosurgery. (1987) 20:843–7. doi: 10.1227/00006123-198706000-00004
66. Hamilton GF, Robinson TK, Ray RH. The effects of helium-neon laser upon regeneration of the crushed peroneal nerve. J Orthop Sports Phys Ther. (1992) 15:209–14. doi: 10.2519/jospt.1992.15.5.209
67. Rochkind S, Ouaknine GE. New trend in neuroscience: low-power laser effect on peripheral and central nervous system (basic science, preclinical and clinical studies). Neurol Res. (1992) 14:2–11. doi: 10.1080/01616412.1992.11740003
68. Shamir MH, Rochkind S, Sandbank J, Alon M. Double-blind randomized study evaluating regeneration of the rat transected sciatic nerve after suturing and postoperative low-power laser treatment. J Reconstr Microsurg. 17:133–7. doi: 10.1055/s-2001-12702
69. Miloro M, Halkias LE, Mallery S, Travers S, Rashid RG. Low-level laser effect on neural regeneration in gore-tex tubes. Oral Surg Oral Med Oral Pathol Oral Radiol Endod. (2002) 93:27–34. doi: 10.1067/moe.2002.119518
70. Muniz XC, de Assis A, de Oliveira B, Ferreira L, Bilal M, Iqbal H, et al. Efficacy of low-level laser therapy in nerve injury repair-a new era in therapeutic agents and regenerative treatments. Neurol Sci. (2021) 42:4029–43. doi: 10.1007/s10072-021-05478-7
71. Fusakul Y, Aranyavalai T, Saensri P, Thiengwittayaporn S. Low-level laser therapy with a wrist splint to treat carpal tunnel syndrome: a double-blinded randomized controlled trial. Lasers Med Sci. (2014) 29:1279–87. doi: 10.1007/s10103-014-1527-2
72. Tascioglu F, Degirmenci NA, Ozkan S, Mehmetoglu O. Low-level laser in the treatment of carpal tunnel syndrome: clinical, electrophysiological, and ultrasonographical evaluation. Rheumatol Int. (2012) 32:409–15. doi: 10.1007/s00296-010-1652-6
73. Gigo-Benato D, Russo TL, Tanaka EH, Assis L, Salvini TF, Parizotto NA, et al. Effects of 660 and 780 nm low-level laser therapy on neuromuscular recovery after crush injury in rat sciatic nerve. Lasers Surg Med. (2010) 42:673–82. doi: 10.1002/lsm.20978
74. Hsieh YL, Chou LW, Chang PL, Yang CC, Kao MJ, Hong CZ, et al. Low-level laser therapy alleviates neuropathic pain and promotes function recovery in rats with chronic constriction injury: possible involvements in hypoxia-inducible factor 1alpha (hif-1alpha). J Comp Neurol. (2012) 520:2903–16. doi: 10.1002/cne.23072
75. Wang CZ, Chen YJ, Wang YH, Yeh ML, Huang MH, Ho ML, et al. Low-level laser irradiation improves functional recovery and nerve regeneration in sciatic nerve crush rat injury model. PLoS ONE. (2014) 9:e103348. doi: 10.1371/journal.pone.0103348
76. Marble CB, Yakovlev VV. Biomedical optics applications of advanced lasers and nonlinear optics. J Biomed Opt. (2020) 25:1–9. doi: 10.1117/1.JBO.25.4.040902
77. Zhang F, Li Q, Qin W, Ren W, Zhu P, Jin Q, et al. A study of the biological effects of low-level light. Lasers Med Sci. (2024) 39:74. doi: 10.1007/s10103-024-04018-x
78. Chang WD, Wu JH, Wang HJ, Jiang JA. Therapeutic outcomes of low-level laser therapy for closed bone fracture in the human wrist and hand. Photomed Laser Surg. (2014) 32:212–8. doi: 10.1089/pho.2012.3398
79. Morries LD, Cassano P, Henderson TA. Treatments for traumatic brain injury with emphasis on transcranial near-infrared laser phototherapy. Neuropsychiatr Dis Treat. (2015) 11:2159–75. doi: 10.2147/NDT.S65809
80. Chen AC, Arany PR, Huang YY, Tomkinson EM, Sharma SK, Kharkwal GB, et al. Low-level laser therapy activates nf-kb via generation of reactive oxygen species in mouse embryonic fibroblasts. PLoS ONE. (2011) 6:e22453. doi: 10.1371/journal.pone.0022453
81. Xu F, Han L, Wang Y, Deng D, Ding Y, Zhao S, et al. Prolonged anesthesia induces neuroinflammation and complement-mediated microglial synaptic elimination involved in neurocognitive dysfunction and anxiety-like behaviors. BMC Med. (2023) 21:7. doi: 10.1186/s12916-022-02705-6
82. Wu YH, Wang J, Gong DX, Gu HY, Hu SS, Zhang H, et al. Effects of low-level laser irradiation on mesenchymal stem cell proliferation: a microarray analysis. Lasers Med Sci. (2012) 27:509–19. doi: 10.1007/s10103-011-0995-x
83. Li B, Wang X. Photobiomodulation enhances facial nerve regeneration via activation of pi3k/akt signaling pathway-mediated antioxidant response. Lasers Med Sci. (2022) 37:993–1006. doi: 10.1007/s10103-021-03344-8
84. Huang HT, Sun ZG, Liu HW, Ma JT, Hu M. Erk/mapk and pi3k/akt signal channels simultaneously activated in nerve cell and axon after facial nerve injury. Saudi J Biol Sci. (2017) 24:1853–8. doi: 10.1016/j.sjbs.2017.11.027
85. Dong L, Li R, Li D, Wang B, Lu Y, Li P, et al. Fgf10 enhances peripheral nerve regeneration via the preactivation of the pi3k/akt signaling-mediated antioxidant response. Front Pharmacol. (2019) 10:1224. doi: 10.3389/fphar.2019.01224
86. Chen YJ, Wang YH, Wang CZ, Ho ML, Kuo PL, Huang MH, et al. Effect of low level laser therapy on chronic compression of the dorsal root ganglion. PLoS ONE. (2014) 9:e89894. doi: 10.1371/journal.pone.0089894
87. de Oliveira ME, Da SJ, Brioschi ML, Chacur M. Effects of photobiomodulation therapy on neuropathic pain in rats: evaluation of nociceptive mediators and infrared thermography. Lasers Med Sci. (2021) 36:1461–7. doi: 10.1007/s10103-020-03187-9
88. Matsuda M, Huh Y, Ji RR. Roles of inflammation, neurogenic inflammation, and neuroinflammation in pain. J Anesth. (2019) 33:131–9. doi: 10.1007/s00540-018-2579-4
89. Gomes LE, Dalmarco EM, Andre ES. The brain-derived neurotrophic factor, nerve growth factor, neurotrophin-3, and induced nitric oxide synthase expressions after low-level laser therapy in an axonotmesis experimental model. Photomed Laser Surg. (2012) 30:642–7. doi: 10.1089/pho.2012.3242
90. Chiaramonte R, Pavone V, Testa G, Pesce I, Scaturro D, Musumeci G, et al. The role of physical exercise and rehabilitative implications in the process of nerve repair in peripheral neuropathies: a systematic review. Diagnostics. (2023) 13:364. doi: 10.3390/diagnostics13030364
91. Herbison GJ, Jaweed MM, Ditunno JF. Effect of swimming on reinnervation of rat skeletal muscle. J Neurol Neurosurg Psychiatry. (1974) 37:1247–51. doi: 10.1136/jnnp.37.11.1247
92. Mennen U. End-to-side nerve suture in clinical practice. Hand Surg. (2003) 8:33–42. doi: 10.1142/S0218810403001352
93. Arbat-Plana A, Navarro X, Udina E. Effects of forced, passive, and voluntary exercise on spinal motoneurons changes after peripheral nerve injury. Eur J Neurosci. (2017) 46:2885–92. doi: 10.1111/ejn.13739
94. Bai J, Geng B, Wang X, Wang S, Yi Q, Tang Y, et al. Exercise facilitates the m1-to-m2 polarization of microglia by enhancing autophagy via the bdnf/akt/mtor pathway in neuropathic pain. Pain Physician. (2022) 25:E1137–51.
95. Camuzard O, Lu JC, Abbadi SE, Chang TN, Chuang DC. The impact of exercise on motor recovery after long nerve grafting-experimental rat study. J Reconstr Microsurg. (2023) 39:508–16. doi: 10.1055/s-0043-1761207
96. Di Palma M, Ambrogini P, Lattanzi D, Brocca L, Bottinelli R, Cuppini R, et al. The impact of different exercise protocols on rat soleus muscle reinnervation and recovery following peripheral nerve lesion and regeneration. Front Physiol. (2022) 13:948985. doi: 10.3389/fphys.2022.948985
97. Willand MP, Chiang CD, Zhang JJ, Kemp SW, Borschel GH, Gordon T, et al. Daily electrical muscle stimulation enhances functional recovery following nerve transection and repair in rats. Neurorehabil Neural Repair. (2015) 29:690–700. doi: 10.1177/1545968314562117
98. Ramachandran VS, Rogers-Ramachandran D. synaesthesia in phantom limbs induced with mirrors. Proc Biol Sci. (1996) 263:377–86. doi: 10.1098/rspb.1996.0058
99. Selles RW, Schreuders TA, Stam HJ. Mirror therapy in patients with causalgia (complex regional pain syndrome type ii) following peripheral nerve injury: two cases. J Rehabil Med. (2008) 40:312–4. doi: 10.2340/16501977-0158
100. Kong Y, Kuss M, Shi Y, Fang F, Xue W, Shi W, et al. Exercise facilitates regeneration after severe nerve transection and further modulates neural plasticity. Brain Behav Immun Health. (2022) 26:100556. doi: 10.1016/j.bbih.2022.100556
101. Sleijser-Koehorst M, Koop MA, Coppieters MW, Lutke SI, Radisic N, Hooijmans CR, et al. The effects of aerobic exercise on neuroimmune responses in animals with traumatic peripheral nerve injury: a systematic review with meta-analyses. J Neuroinflammation. (2023) 20:104. doi: 10.1186/s12974-023-02777-y
102. Lesnak JB, Sluka KA. Mechanism of exercise-induced analgesia: what we can learn from physically active animals. Pain Rep. (2020) 5:e850. doi: 10.1097/PR9.0000000000000850
103. Cichon J, Blanck T, Gan WB, Yang G. Activation of cortical somatostatin interneurons prevents the development of neuropathic pain. Nat Neurosci. (2017) 20:1122–32. doi: 10.1038/nn.4595
104. Almeida C, Demaman A, Kusuda R, Cadetti F, Ravanelli MI, Queiroz AL, et al. Exercise therapy normalizes bdnf upregulation and glial hyperactivity in a mouse model of neuropathic pain. Pain. (2015) 156:504–13. doi: 10.1097/01.j.pain.0000460339.23976.12
105. Sumizono M, Sakakima H, Otsuka S, Terashi T, Nakanishi K, Ueda K, et al. The effect of exercise frequency on neuropathic pain and pain-related cellular reactions in the spinal cord and midbrain in a rat sciatic nerve injury model. J Pain Res. (2018) 11:281–91. doi: 10.2147/JPR.S156326
106. Lopez-Alvarez VM, Puigdomenech M, Navarro X, Cobianchi S. Monoaminergic descending pathways contribute to modulation of neuropathic pain by increasing-intensity treadmill exercise after peripheral nerve injury. Exp Neurol. (2018) 299:42–55. doi: 10.1016/j.expneurol.2017.10.007
107. Griffin EW, Mullally S, Foley C, Warmington SA, O'Mara SM, Kelly AM, et al. Aerobic exercise improves hippocampal function and increases bdnf in the serum of young adult males. Physiol Behav. (2011) 104:934–41. doi: 10.1016/j.physbeh.2011.06.005
108. Briken S, Rosenkranz SC, Keminer O, Patra S, Ketels G, Heesen C, et al. Effects of exercise on irisin, bdnf and il-6 serum levels in patients with progressive multiple sclerosis. J Neuroimmunol. (2016) 299:53–8. doi: 10.1016/j.jneuroim.2016.08.007
109. Swift DL, Johannsen NM, Myers VH, Earnest CP, Smits JA, Blair SN, et al. The effect of exercise training modality on serum brain derived neurotrophic factor levels in individuals with type 2 diabetes. PLoS ONE. (2012) 7:e42785. doi: 10.1371/journal.pone.0042785
110. Enette L, Vogel T, Merle S, Valard-Guiguet AG, Ozier-Lafontaine N, Neviere R, et al. Effect of 9 weeks continuous vs. Interval aerobic training on plasma bdnf levels, aerobic fitness, cognitive capacity and quality of life among seniors with mild to moderate alzheimer's disease: a randomized controlled trial. Eur Rev Aging Phys Act. (2020) 17:2. doi: 10.1186/s11556-019-0234-1
111. Mcgregor CE, English AW. The role of bdnf in peripheral nerve regeneration: activity-dependent treatments and val66met. Front Cell Neurosci. (2018) 12:522. doi: 10.3389/fncel.2018.00522
112. Thompson NJ, Sengelaub DR, English AW. Enhancement of peripheral nerve regeneration due to treadmill training and electrical stimulation is dependent on androgen receptor signaling. Dev Neurobiol. (2014) 74:531–40. doi: 10.1002/dneu.22147
113. Fahimi A, Baktir MA, Moghadam S, Mojabi FS, Sumanth K, Mcnerney MW, et al. Physical exercise induces structural alterations in the hippocampal astrocytes: exploring the role of bdnf-trkb signaling. Brain Struct Funct. (2017) 222:1797–808. doi: 10.1007/s00429-016-1308-8
114. Jin JJ, Ko IG, Kim SE, Hwang L, Lee MG, Kim DY, et al. Age-dependent differences of treadmill exercise on spatial learning ability between young- and adult-age rats. J Exerc Rehabil. (2017) 13:381–6. doi: 10.12965/jer.1735070.535
115. Maejima H, Kanemura N, Kokubun T, Murata K, Takayanagi K. Exercise enhances cognitive function and neurotrophin expression in the hippocampus accompanied by changes in epigenetic programming in senescence-accelerated mice. Neurosci Lett. (2018) 665:67–73. doi: 10.1016/j.neulet.2017.11.023
116. Andre-Levigne D, Pignel R, Boet S, Jaquet V, Kalbermatten DF, Madduri S, et al. Role of oxygen and its radicals in peripheral nerve regeneration: from hypoxia to physoxia to hyperoxia. Int J Mol Sci. (2024) 25. doi: 10.3390/ijms25042030
117. Ince B, Ismayilzada M, Arslan A, Dadaci M. Does hyperbaric oxygen therapy facilitate peripheral nerve recovery in upper extremity injuries? A prospective study of 74 patients. Eur J Trauma Emerg Surg. (2022) 48:3997–4003. doi: 10.1007/s00068-022-01920-3
118. Yasuda T, Sato Y, Nakajima T. Is blood flow-restricted training effective for rehabilitation of a pianist with residual neurological symptoms in the upper limbs? A case study. J Phys Ther Sci. (2021) 33:612–7. doi: 10.1589/jpts.33.612
119. Gundermann DM, Walker DK, Reidy PT, Borack MS, Dickinson JM, Volpi E, et al. Activation of mtorc1 signaling and protein synthesis in human muscle following blood flow restriction exercise is inhibited by rapamycin. Am J Physiol-Endocrinol Metab. (2014) 306:E1198–204. doi: 10.1152/ajpendo.00600.2013
120. Nielsen JL, Aagaard P, Bech RD, Nygaard T, Hvid LG, Wernbom M, et al. Proliferation of myogenic stem cells in human skeletal muscle in response to low-load resistance training with blood flow restriction. J Physiol. (2012) 590:4351–61. doi: 10.1113/jphysiol.2012.237008
121. De Pedro JA, Perez-Caballer AJ, Dominguez J, Collia F, Blanco J, Salvado M. Pulsed electromagnetic fields induce peripheral nerve regeneration and endplate enzymatic changes. Bioelectromagnetics. (2005) 26:20–7. doi: 10.1002/bem.20049
122. Tai YK, Ng C, Purnamawati K, Yap J, Yin JN, Wong C, et al. Magnetic fields modulate metabolism and gut microbiome in correlation with pgc-1alpha expression: follow-up to an in vitro magnetic mitohormetic study. FASEB J. (2020) 34:11143–67. doi: 10.1096/fj.201903005RR
123. Bademoglu G, Erdal N, Uzun C, Tasdelen B. The effects of pulsed electromagnetic field on experimentally induced sciatic nerve injury in rats. Electromagn Biol Med. (2021) 40:408–19. doi: 10.1080/15368378.2021.1907403
124. Zmyslony M, Palus J, Jajte J, Dziubaltowska E, Rajkowska E. Dna damage in rat lymphocytes treated in vitro with iron cations and exposed to 7 mt magnetic fields (static or 50 hz). Mutat Res. (2000) 453:89–96. doi: 10.1016/S0027-5107(00)00094-4
125. Chen YH, Siow TY, Wang JY, Lin SY, Chao YH. Greater cortical activation and motor recovery following mirror therapy immediately after peripheral nerve repair of the forearm. Neuroscience. (2022) 481:123–33. doi: 10.1016/j.neuroscience.2021.11.048
126. Hsu HY, Chen PT, Kuan TS, Yang HC, Shieh SJ, Kuo LC, et al. A touch-observation and task-based mirror therapy protocol to improve sensorimotor control and functional capability of hands for patients with peripheral nerve injury. Am J Occup Ther. (2019) 73:1287729571p−2p. doi: 10.5014/ajot.2018.027763
127. Puleo C, Cotero V. Noninvasive neuromodulation of peripheral nerve pathways using ultrasound and its current therapeutic implications. Cold Spring Harb Perspect Med. (2020) 10:a034215. doi: 10.1101/cshperspect.a034215
128. Botzanowski B, Donahue MJ, Ejneby MS, Gallina AL, Ngom I, Missey F, et al. Noninvasive stimulation of peripheral nerves using temporally-interfering electrical fields. Adv Healthc Mater. (2022) 11:e2200075. doi: 10.1002/adhm.202200075
129. Guo W, He Y, Zhang W, Sun Y, Wang J, Liu S, et al. A novel non-invasive brain stimulation technique: “temporally interfering electrical stimulation”. Front Neurosci. (2023) 17:1092539. doi: 10.3389/fnins.2023.1092539
130. Huang L, Jin J, Chen K, You S, Zhang H, Sideris A, et al. Bdnf produced by cerebral microglia promotes cortical plasticity and pain hypersensitivity after peripheral nerve injury. PLoS Biol. (2021) 19:e3001337. doi: 10.1371/journal.pbio.3001337
131. Austin PJ, Moalem-Taylor G. The neuro-immune balance in neuropathic pain: involvement of inflammatory immune cells, immune-like glial cells and cytokines. J Neuroimmunol. (2010) 229:26–50. doi: 10.1016/j.jneuroim.2010.08.013
132. Xiang Y, Dai J, Xu L, Li X, Jiang J, Xu J, et al. Research progress in immune microenvironment regulation of muscle atrophy induced by peripheral nerve injury. Life Sci. (2021) 287:120117. doi: 10.1016/j.lfs.2021.120117
133. Bazgir B, Fathi R, Rezazadeh VM, Mozdziak P, Asgari A. Satellite cells contribution to exercise mediated muscle hypertrophy and repair. Cell J. (2017) 18:473–84. doi: 10.22074/cellj.2016.4714
Keywords: peripheral nerve regeneration, ultrasound, electricity, photobiomodulation, aerobic exercise
Citation: Chu X-L, Zhao X-X, Liu S-Y, Li Y-J, Ding N, Liu M-Q, Li Q-W and Li Q (2025) Research progress in different physical therapies for treating peripheral nerve injuries. Front. Neurol. 16:1508604. doi: 10.3389/fneur.2025.1508604
Received: 31 October 2024; Accepted: 28 February 2025;
Published: 07 April 2025.
Edited by:
Yaxian Wang, Nantong University, ChinaReviewed by:
Xiaolei Liu, University of Pennsylvania, United StatesChunrui Yang, The Second Hospital of Tianjin Medical University, China
Copyright © 2025 Chu, Zhao, Liu, Li, Ding, Liu, Li and Li. This is an open-access article distributed under the terms of the Creative Commons Attribution License (CC BY). The use, distribution or reproduction in other forums is permitted, provided the original author(s) and the copyright owner(s) are credited and that the original publication in this journal is cited, in accordance with accepted academic practice. No use, distribution or reproduction is permitted which does not comply with these terms.
*Correspondence: Qing-Wen Li, bGVlcXcxMTAxQDE2My5jb20=; Qi Li, TGlxaV84MkAxMjYuY29t
†These authors share first authorship