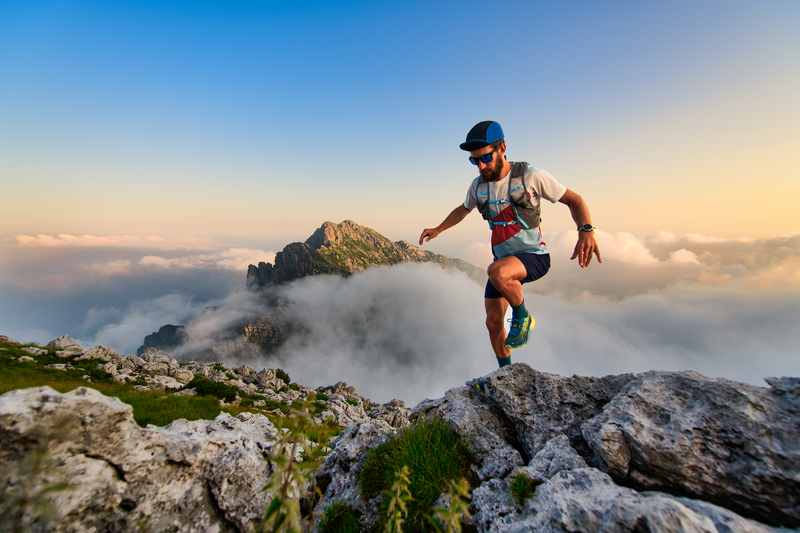
94% of researchers rate our articles as excellent or good
Learn more about the work of our research integrity team to safeguard the quality of each article we publish.
Find out more
OPINION article
Front. Neurol. , 28 June 2024
Sec. Neuro-Otology
Volume 15 - 2024 | https://doi.org/10.3389/fneur.2024.1426213
This article is part of the Research Topic Impact of Vestibular Dysfunction Studies on Space Flight Health Challenges View all 3 articles
The space race set in motion by the Cold War intensified in the 1960s, with the Soviet Union first paving the path to manned space flight in 1961 (1). In high relevance to astronauts' mission readiness (2–5), experiments conducted in this decade, contrasting persons with functioning and bilaterally non-functioning labyrinths, solidified the contributions of the vestibular inputs to production of spatial perception and motion sickness during exposure to passive motion or microgravity (6). However, all forms of labyrinthine deactivation are not created equal. For example, patients may undergo total vestibular nerve section as a treatment for vertigo due to eighth nerve neuroma or Ménière's disease (7, 8); others may for varying causes sustain selective damage within the vestibular end-organs while retaining functioning nerves, as may be the case for candidates of vestibular implants (9, 10). Selective inactivation of otolithic signals may even be induced environmentally under microgravity in space. Examining information processing in the central vestibular mechanism known as velocity storage in various contexts of labyrinthine inactivation may shed further light on health and perceptual anomalies during or following space flight.
Activated by head rotation, large-field visual motion, or proprioceptive cues for continuous rotation, velocity storage is a central neural circuit that sustains a rotational velocity estimate of ongoing self-motion (11–16). The mechanism serves a working memory-like function of self-motion and spatial orientation to shape ocular and postural reflexes in the brainstem and presumably perception in the cerebral cortex (13–15, 17–19).
The key to understanding the working of velocity storage has been in eye movement measurement. Nystagmus, such as occurs during the vestibulo-ocular reflex (VOR), is an automatic eye movement made in response to signals that indicate self-rotation relative to the surrounding world. Nystagmus facilitates the acquisition of visual information with the eyes moving in opposition to and in compensation for the detected rotation (slow phases) but for quick “resetting” interruptions (fast phases; Figure 1A). The presence of a common, sub-cerebral cortical mechanism for multimodal sensory integration had long been presumed based on experimental observations such as: (a) coordinated shaping, rather than a simple superposition, of nystagmus of different sensory origins takes place during combined stimulation (11); (b) the nystagmic reaction to either visual (optokinetic) or vestibular stimulation is subject to a “central inertia,” demonstrated with a prolonged response or after-response (11); (c) optokinetic nystagmus (OKN) can be induced in animals with visual cortical lesions (11, 20); and (d) bilateral vestibular nerve sections degrade OKN and abolish optokinetic after-nystagmus (OKAN) (21, 22).
Figure 1. Characteristics of nystagmus and examples of unusual stimuli that can induce nystagmus. (A) Sawtooth pattern of eye position change over time during nystagmus. Slow-phase movements are directed against, thus in compensation for, the detected head rotation, whereas fast-phase movements facilitate maintenance of eye position within the oculomotor range. (B) Arthrokinetic stimulation. (C) Circular treadmill locomotion. (D) OVAR. (E) Whole-body sinusoidal PWR (a). As a variation in human testing, a disorienting sensation and motion sickness may be induced with a discrete, rather than continuous, head tilt while seated in a rotating chair (b). Note that in (B, C), the head stays spatially stationary, and when conducted in darkness, the stimuli can generate nystagmus without labyrinthine or visual input. In (D, E), at steady state, the semicircular canals do not signal the rotation continuing about the axis indicated by the dotted line.
Animal experiments in the 1970s demonstrated that spiking activity in certain second-order vestibular neurons correlated with the strength of either vestibularly- or optokinetically-induced nystagmus (23–25). With this discovery, the functional operation of the central processing system that soon came to be known as velocity storage became a valuable focus of rigorous model-based testing of behavioral and physiological data (13–15, 26, 27), These neurons belong to the vestibular-only (VO) class, so-termed to differentiate from other secondary vestibular neurons that display saccade or eye position sensitivity (28). Importantly, while the decay time constant of the primary afferent activity after the cessation of head angular acceleration, contributed by the semicircular canal endolymph's inertia, amounts only to 3–6 s, the activity of VO neurons is typically characterized with a time constant of 10–40 s, corresponding to the aforementioned “central inertia” (25, 29–32).
Besides vestibular or optokinetic means, and indeed in the absence of actual motion or visual motion, an illusion of body rotation (circular vection) and corresponding compensatory nystagmus can be induced in a stationary person when a rotating surround is passively followed with an extended arm (Figure 1B) (12) or when the person steps along a fictitious, circular trajectory on a counter-rotating floor (Figure 1C) (16). Compensatory nystagmus is also generated in the absence of vision or steady activation of coplanar semicircular canals when the subject is continuously rotated about a tilted axis (off-vertical axis rotation—OVAR; Figure 1D) (33–35) or when sinusoidally pitched or rolled during earth-vertical axis rotation (pitch/roll while rotating—PWR/RWR; Figure 1E) (36, 37).
That nystagmus can be generated and sustained without coplanar optokinetic or semicircular canal activation indicates that the velocity storage mechanism actively reconstitutes self-motion signals from multimodal sensory inputs. Additionally, the stored estimate of self-motion can be dynamically transformed such that the outcome nystagmus tends to align to the gravito-inertial field. For example, horizontal OKN induced while tilted laterally gives way to OKAN with a vertical component (38–40). Similarly, the per-rotatory nystagmus in response to off-center rotation with forward or backward tangential motion develops an out-of-plane, vertical component as the centripetal acceleration tilts the gravito-inertial field (41). Thus, velocity storage is also equipped with orienting properties to act as a “neural gyroscope” (40, 42, 43), making it more fitting to be identified as “working memory-like” rather than mere storage.
The properties of velocity storage are nearly exclusively characterized by the dynamics of slow-phase eye velocity of nystagmus. However, many of so-characterized properties have also been demonstrated in the activity of VO neurons (29, 44, 45). Insight into the working of velocity storage has been conversely derived from VO neuron activity as well. For example, while most VO neurons receive convergent inputs from different semicircular canal and otolith afferents, many show preferences to activation near or orthogonal to the plane of a specific push-pull canal pair (29, 46). Thus, VO neurons appear to collectively code the three-dimensional action of velocity storage using a coordinate system consistent with the geometric alignment of the semicircular canals and extraocular muscles, which is common across lateral- or frontal-eyed species (29, 47). Further, while vestibular afferents cannot differentiate active from passive motion, VO neurons reportedly show different responses (48–51). Accordingly, velocity storage may be activated and controlled differently during active and passive motion.
Velocity storage is under the inhibitory control of the cerebellar nodulus and, likely, the adjacent caudal uvula. Damage to these areas destabilizes velocity storage, triggering periodic alternating nystagmus and elongating the time constant of the decay of the VOR nystagmus during a rotational test (52–55). Such damage also results in loss of compensatory nystagmus during OVAR and sinusoidal PWR/RWR (56, 57) as well as in a compromised ability to reorient eye velocity to the gravito-inertial field (55, 57, 58). Reversible unilateral inactivation of the nodulus induces spontaneous nystagmus in darkness with contralaterally-directed slow phases and abnormal eye velocity orientation to gravity (59). The nodulus and caudal uvula target a wide variety of cell types in the vestibular nuclei, including VO neurons (60). Nodular micro-electrical stimulation results in shortening of the decay time constant of the VOR or OKAN with ipsilateral slow phases (60, 61), but may also yield further different effects depending on the duration and exact location of the stimulation (59, 62).
Lastly, velocity storage is malleable. For example, repeated rotation in darkness shortens the VOR decay time constant (decreased “central inertia”) in a long-retained effect known as vestibular habituation (63–65). The spatial orientation properties of velocity storage, such as observed in eye movements outside the stimulus plane during the VOR, can also be modified (66, 67), possibly to facilitate adaptation to a new gravito-inertial environment (68–70). Relatedly, the spatial tuning of VO neurons can be modified with prolonged tilt (71). As the cerebellum plays a major role in motor learning and calibration (72, 73), the malleability of velocity storage likely depends on the nodulus and uvula (64). Mal de débarquement syndrome, a chronic illness primarily characterized by a persistent illusory perception of self-motion, is thought to result from a failure in velocity storage to readapt to a normal acceleration environment after adapting to passive motion (74–76).
Studies conducted in humans and animals with bilaterally non-functioning labyrinths, including those from the 1960s, found that these subjects were immune to motion sickness when exposed to otherwise highly provocative vestibular tests, such as PWR/RWR, OVAR, and other unusual motion stimuli (6, 77–79). Such immunity merits re-examination in parallel with patterns of velocity storage activation (Table 1). Differences and similarities in perceptual experiences are also considered when illuminating information is available.
Vestibular habituation, identified with a shortened velocity storage time constant, reportedly reduces susceptibility to motion sickness (101, 102). The GABA-B agonist baclofen has been indicated to also reduce the velocity storage time constant and motion sickness susceptibility to RWR, but reversibly (103, 104). Given that bilateral vestibular nerve sections result in loss of OKAN (21, 22), and that loss of or impaired vestibular functions due to ototoxicity or other causes significantly reduces OKAN (80–83), the immunity to motion sickness to provocative motion stimuli in labyrinthine-defective individuals may be explained by their loss of or reduced ability to store velocity signals. However, motion sickness and circular vection can still be induced with head movements in a rotating visual environment after vestibular neurectomy (7). The effect of baclofen on visually induced motion sickness is not known.
Neuronally, while the ability to store velocity signals appears to depend on the wellbeing of the primary afferents, there is no evidence that VO neurons undergo anterograde transneuronal degeneration after vestibular nerve sections (105, 106). Neuronal activities ascribed to velocity storage functions that survive labyrinthine deactivation remain to be identified. Behaviorally however, nystagmus and circular vection can be induced in labyrinthine-defective individuals during fictitious circular stepping around in darkness (Figure 1C) (16), providing strong evidence that reconstitution of self-motion velocity signals and their storage are separate processes.
Motion sickness is typically induced in a context of passive rather than active motion (107, 108). Likewise, VO neurons are reportedly more sensitive to passive than active head movements (48–51). Participation of these neurons in the vestibular-autonomic circuits is not known (109–113), but motion sickness susceptibility is likely not directly increased as a simple consequence of increased VO neuron activation. For example, while congruent optokinetic and vestibular inputs can synergistically activate VO neurons and incongruent ones antagonistically (25–27, 29, 114), vision of a stationary surround (i.e., optokinetic input congruent with physical motion) protects against motion sickness and perceptual disorientation during PWR/RWR or OVAR (79, 115–120). Curiously, under microgravity in space or during parabolic flight, active head movements with vision of a stationary surround is more provocative than the same movements without vision (3, 96).
Also curiously, while PWR/RWR is highly provocative of motion sickness and perceptually disorienting on earth, it is not so under microgravity in space or during parabolic flight (3, 94, 95). Mirroring this environmental inactivation of otolithic signals, after bilateral utricular nerve section and saccular macula destruction, previously susceptible squirrel monkeys reportedly become immune to motion sickness while unrestrained inside a rotating cage (89), a condition that likely generates provocativeness through a PWR/RWR-like mechanism. By contrast, semicircular canal deactivation precipitated by ototoxicity, sparing otolithic functions, has also been shown to induce immunity to motion sickness in unrestrained squirrel monkeys in a rotating cage (121). Thus, the provocativeness of PWR/RWR appears to arise from central integration of both the semicircular canal and otolithic signals. By parallel, the ability to generate nystagmus during sinusoidal PWR/RWR is lost in macaque monkeys after selective deactivation of the semicircular canal function with canal plugging (36, 85). The counterpart effect of selective deactivation of the otolithic input does not seem to have been experimentally tested, but nystagmus generation during sinusoidal PWR/RWR is also thought to require both otolithic and semicircular canal inputs (57, 67, 85).
The involvement of the cerebellar nodulus and uvula provides another parallel between velocity storage and motion sickness. The nodulo-uvular involvement in motion sickness production has long been suspected based on observations that nodulo-uvular lesions rendered experimental animals immune to motion sickness (97, 98). Doubts raised against this view (99, 100) may be partially addressed by considering the acute vs. chronic effects of such lesions and the presence of parallel vestibular-cerebellar circuits that can produce counteracting autonomic effects (52, 53, 111, 113, 122, 123). It is presumably within such complex neural interactions that learning takes place so that evoked symptoms of motion sickness and future susceptibility diminish even when the provocative situation is unchanged (4, 70). Motion sickness is said to be most severe when the orientation and autonomic regulation systems are undergoing rapid recalibration (4). Whether understanding the neural basis of velocity storage malleability improves the predictability of motion sickness susceptibility to specific stimuli or situations remains to be seen. The physiology of and the circuitries that control and are controlled by VO neurons likely serve a focal point of future studies (19, 113), an approach thus far possible chiefly in animal-based experiments.
Clues from various forms of labyrinthine and central loss of vestibular function connect spatial perception, motion sickness, and velocity storage as parallel phenomena. Similar partially overlapping parallelisms from different perspectives have previously been suggested (19, 113). A possible pitfall of such thinking is that these phenomena may be just that—parallel but unrelated. No matter, the paths to fill the knowledge gaps are largely unpaved and promise abundant scientific opportunities. Continuing development in space exploration and technology behooves us to advance the field.
JM: Writing – original draft, Writing – review & editing.
The author(s) declare that no financial support was received for the research, authorship, and/or publication of this article.
The author declares that the research was conducted in the absence of any commercial or financial relationships that could be construed as a potential conflict of interest.
All claims expressed in this article are solely those of the authors and do not necessarily represent those of their affiliated organizations, or those of the publisher, the editors and the reviewers. Any product that may be evaluated in this article, or claim that may be made by its manufacturer, is not guaranteed or endorsed by the publisher.
OKAN, optokinetic after-nystagmus; OKN, optokinetic nystagmus; OVAR, off-vertical axis rotation; PWR, pitch while rotating; RWR, roll while rotating; VO, vestibular-only; VOR, vestibulo-ocular reflex.
1. Dunbar B. NASA: 60 Years and Counting—Human Spaceflight. National Aeronautics and Space Administration (2018). Available online at: https://www.nasa.gov/specials/60counting/spaceflight.html (accessed March 6, 2024).
2. Clark B. Visual space perception as influenced by unusual vestibular stimulation. Hum Fact. (1963) 5:265–74. doi: 10.1177/001872086300500307
3. Graybiel A, Miller EF, Homick JL. Individual differences in susceptibility to motion sickness among six Skylab astronauts. Acta Astronaut. (1975) 2:155–74. doi: 10.1016/0094-5765(75)90051-X
4. Lackner JR, Dizio P. Space motion sickness. Exp Brain Res. (2006) 175:377–99. doi: 10.1007/s00221-006-0697-y
5. Clément G, Skinner A, Lathan C. Distance and size perception in astronauts during long-duration spaceflight. Life. (2013) 3:524–37. doi: 10.3390/life3040524
6. Graybiel A. Functional disturbances of vestibular origin of significance in space flight. In:Bjurstedt H, , editor. Proceedings of the Second International Symposium on Basic Environmental Problems of Man in Space. Vienna: Springer (1967). p. 8–32.
7. Johnson WH, Sunahara FA, Landolt JP. Importance of the vestibular system in visually induced nausea and self-vection. J Vestib Res. (1999) 9:83–7. doi: 10.3233/VES-1999-9202
8. Thai-Van H, Bounaix MJ, Fraysse B. Menière's disease: pathophysiology and treatment. Drugs. (2001) 61:1089–102. doi: 10.2165/00003495-200161080-00005
9. Sluydts M, Curthoys I, Vanspauwen R, Papsin BC, Cushing SL, Ramos A, et al. Electrical vestibular stimulation in humans: a narrative review. Audiol Neurootol. (2020) 25:6–24. doi: 10.1159/000502407
10. Strupp M, Brandt T, Dieterich M. “Bilateral Vestibulopathy,” Vertigo and Dizziness. Cham: Springer International Publishing (2023). p. 99–118.
11. ter Braak J. Untersuchungen über optokinetischen Nystagmus. Arch Neerl Physiol Homme Anim. (1936) 21:309–76.
12. Brandt Th, Büchele W, Arnold F. Arthrokinetic nystagmus and ego-motion sensation. Exp Brain Res. (1977) 30:331–8. doi: 10.1007/BF00237260
13. Cohen B, Matsuo V, Raphan T. Quantitative analysis of the velocity characteristics of optokinetic nystagmus and optokinetic after-nystagmus. J Physiol. (1977) 270:321–44. doi: 10.1113/jphysiol.1977.sp011955
14. Raphan T, Cohen B, Matsuo V. A velocity-storage mechanism responsible for optokinetic nystagmus (OKN), optokinetic after-nystagmus (OKAN) and vestibular nystagmus. In:Baker R, Berthoz A, , editors. Control of Gaze by Brain Stem Neurons. Developments in Neuroscience. Amsterdam: Elsevier (1977). p. 37–47.
15. Robinson DA. Vestibular and optokinetic symbiosis: an example of explaining by modelling. In:Baker R, Berthoz A, , editors. Control of Gaze by Brain Stem Neurons. Developments in Neuroscience. Amsterdam: Elsevier (1977). p. 49–58.
16. Bles W, Jong JMV, Wit GD. Somatosensory compensation for loss of labyrinthine function. Acta Otolaryngol. (1984) 97:213–21. doi: 10.3109/00016488409130982
17. Bertolini G, Ramat S, Laurens J, Bockisch CJ, Marti S, Straumann D, et al. Velocity storage contribution to vestibular self-motion perception in healthy human subjects. J Neurophysiol. (2011) 105:209–23. doi: 10.1152/jn.00154.2010
18. Haggerty SE, Wu AR, Sienko KH, Kuo AD. A shared neural integrator for human posture control. J Neurophysiol. (2017) 118:894–903. doi: 10.1152/jn.00428.2016
19. Lackner JR, DiZio P. Velocity storage: its multiple roles. J Neurophysiol. (2020) 123:1206–15. doi: 10.1152/jn.00139.2019
20. Pasik P, Pasik T, Krieger HP. Effects of cerebral lesions upon opto-kinetic nystagmus in monkeys. J Neurophysiol. (1959) 22:297–304. doi: 10.1152/jn.1959.22.3.297
21. Cohen B, Uemura T, Takemori S. Effects of labyrinthectomy on optokinetic nystagmus (OKN) and optokinetic after-nystagmus (OKAN). Int J Equilib Res. (1973) 3:88–93.
22. Collewijn H. Impairment of optokinetic (after-)nystagmus by labyrinthectomy in the rabbit. Exp Neurol. (1976) 52:146–56. doi: 10.1016/0014-4886(76)90207-7
23. Waespe W, Henn V. Behaviour of secondary vestibular units during optokinetic nystagmus and after-nystagmus in alert monkeys. Pflugers Arch. (1976) 362:R50.
24. Waespe W, Henn V. Vestibular nuclei activity during optokinetic after-nystagmus (OKAN) in the alert monkey. Exp Brain Res. (1977) 30:323–30. doi: 10.1007/BF00237259
25. Waespe W, Henn V. Neuronal activity in the vestibular nuclei of the alert monkey during vestibular and optokinetic stimulation. Exp Brain Res. (1977) 27:523–38. doi: 10.1007/BF00239041
26. Waespe W, Henn V. Conflicting visual-vestibular stimulation and vestibular nucleus activity in alert monkeys. Exp Brain Res. (1978) 33:203–11. doi: 10.1007/BF00238060
27. Waespe W, Henn V. The velocity response of vestibular nucleus neurons during vestibular, visual, and combined angular acceleration. Exp Brain Res. (1979) 37:337–47. doi: 10.1007/BF00237718
28. Fuchs AF, Kimm J. Unit activity in vestibular nucleus of the alert monkey during horizontal angular acceleration and eye movement. J Neurophysiol. (1975) 38:1140–61. doi: 10.1152/jn.1975.38.5.1140
29. Yakushin SB, Raphan T, Cohen B. Coding of velocity storage in the vestibular nuclei. Front Neurol. (2017) 8:386. doi: 10.3389/fneur.2017.00386
30. Fernandez C, Goldberg JM. Physiology of peripheral neurons innervating semicircular canals of the squirrel monkey. II. Response to sinusoidal stimulation and dynamics of peripheral vestibular system J Neurophysiol. (1971) 34:661–75. doi: 10.1152/jn.1971.34.4.66.1
31. Goldberg JM, Fernandez C. Physiology of peripheral neurons innervating semicircular canals of the squirrel monkey. I. Resting discharge and response to constant angular accelerations. J Neurophysiol. (1971) 34:635–60. doi: 10.1152/jn.1971.34.4.635
32. Dai M, Klein A, Cohen B, Raphan T. Model-based study of the human cupular time constant. J Vestib Res. (1999) 9:293–301. doi: 10.3233/VES-1999-9407
33. Guedry FE. Orientation of the rotation-axis relative to gravity: its influence on nystagmus and the sensation of rotation. Acta Otolaryngol. (1965) 60:30–48. doi: 10.3109/00016486509126986
34. Benson AJ, Bodin MA. Interaction of linear and angular accelerations on vestibular receptors in man. Aerosp Med. (1966) 37:144–54.
35. Cohen B, Suzuki JI, Raphan T. Role of the otolith organs in generation of horizontal nystagmus: effects of selective labyrinthine lesions. Brain Res. (1983) 276:159–64. doi: 10.1016/0006-8993(83)90558-9
36. Raphan T, Cohen B, Suzuki J-I, Henn V. Nystagmus generated by sinusoidal pitch while rotating. Brain Res. (1983) 276:165–72. doi: 10.1016/0006-8993(83)90559-0
37. Hess BJM, Angelaki DE. Angular velocity detection by head movements orthogonal to the plane of rotation. Exp Brain Res. (1993) 95:77–83. doi: 10.1007/BF00229656
38. Clément G, Lathan CE. Effects of static tilt about the roll axis on horizontal and vertical optokinetic nystagmus and optokinetic after-nystagmus in humans. Exp Brain Res. (1991) 84:335–41. doi: 10.1007/BF00231454
39. Dai MJ, Raphan T, Cohen B. Spatial orientation of the vestibular system: dependence of optokinetic after-nystagmus on gravity. J Neurophysiol. (1991) 66:1422–39. doi: 10.1152/jn.1991.66.4.1422
40. Raphan T, Cohen B. Organizational principles of velocity storage in three dimensions: the effect of gravity on cross-coupling of optokinetic after-nystagmus. Ann N Y Acad Sci. (1988) 545:74–92. doi: 10.1111/j.1749-6632.1988.tb19556.x
41. Merfeld DM, Young LR, Tomko DL, Paige GD. Spatial orientation of VOR to combined vestibular stimuli in squirrel monkeys. Acta Otolaryngol Suppl. (1991) 481:287–92. doi: 10.3109/00016489109131403
42. Angelaki DE, Hess BJ. Inertial representation of angular motion in the vestibular system of rhesus monkeys. I. Vestibuloocular reflex. J Neurophysiol. (1994) 71:1222–49. doi: 10.1152/jn.1994.71.3.1222
43. Raphan T, Dai M, Cohen B. Spatial orientation of the vestibular system. Ann N Y Acad Sci. (1992) 656:140–57. doi: 10.1111/j.1749-6632.1992.tb25205.x
44. Reisine H, Raphan T. Neural basis for eye velocity generation in the vestibular nuclei of alert monkeys during off-vertical axis rotation. Exp Brain Res. (1992) 92:209–26. doi: 10.1007/BF00227966
45. Blazquez PM, Highstein SM. Visual-vestibular interaction in vertical vestibular only neurons. Neuroreport. (2007) 18:1403–6. doi: 10.1097/WNR.0b013e3282cdeedd
46. Yakushin SB, Raphan T, Cohen B. Spatial properties of central vestibular neurons. J Neurophysiol. (2006) 95:464–78. doi: 10.1152/jn.00459.2005
47. Simpson JI, Graf W. Eye-muscle geometry and compensatory eye movements in lateral-eyed and frontal-eyed animals. Ann N Y Acad Sci. (1981) 374:20–30. doi: 10.1111/j.1749-6632.1981.tb30856.x
48. Gdowski GT, McCrea RA. Integration of vestibular and head movement signals in the vestibular nuclei during whole-body rotation. J Neurophysiol. (1999) 82:436–49. doi: 10.1152/jn.1999.82.1.436
49. McCrea RA, Gdowski GT, Boyle R, Belton T. Firing behavior of vestibular neurons during active and passive head movements: vestibulo-spinal and other non-eye-movement related neurons. J Neurophysiol. (1999) 82:416–28. doi: 10.1152/jn.1999.82.1.416
50. Roy JE, Cullen KE. Selective processing of vestibular reafference during self-generated head motion. J Neurosci. (2001) 21:2131–42. doi: 10.1523/JNEUROSCI.21-06-02131.2001
51. Cullen KE. Vestibular processing during natural self-motion: implications for perception and action. Nat Rev Neurosci. (2019) 20:346–63. doi: 10.1038/s41583-019-0153-1
52. Jeong H-S, Oh JY, Kim JS, Kim J, Lee AY, Oh S-Y. Periodic alternating nystagmus in isolated nodular infarction. Neurology. (2007) 68:956–7. doi: 10.1212/01.wnl.0000257111.24769.d2
53. Oh Y-M, Choi K-D, Oh S-Y, Kim JS. Periodic alternating nystagmus with circumscribed nodular lesion. Neurology. (2006) 67:399. doi: 10.1212/01.wnl.0000219818.35451.10
54. Waespe W, Cohen B, Raphan T. Dynamic modification of the vestibulo-ocular reflex by the nodulus and uvula. Science. (1985) 228:199–202. doi: 10.1126/science.3871968
55. Wearne S, Raphan T, Cohen B. Control of spatial orientation of the angular vestibuloocular reflex by the nodulus and uvula. J Neurophysiol. (1998) 79:2690–715. doi: 10.1152/jn.1998.79.5.2690
56. Angelaki DE, Hess BJ. Lesion of the nodulus and ventral uvula abolish steady-state off-vertical axis otolith response. J Neurophysiol. (1995) 73:1716–20. doi: 10.1152/jn.1995.73.4.1716
57. Angelaki DE, Hess BJ. Inertial representation of angular motion in the vestibular system of rhesus monkeys. II. Otolith-controlled transformation that depends on an intact cerebellar nodulus. J Neurophysiol. (1995) 73:1729–51. doi: 10.1152/jn.1995.73.5.1729
58. Cohen B, John P, Yakushin SB, Buettner-Ennever J, Raphan T. The nodulus and uvula: source of cerebellar control of spatial orientation of the angular vestibulo-ocular reflex. Ann N Y Acad Sci. (2002) 978:28–45. doi: 10.1111/j.1749-6632.2002.tb07553.x
59. Sheliga BM, Yakushin SB, Silvers A, Raphan T, Cohen B. Control of spatial orientation of the angular vestibulo-ocular reflex by the nodulus and uvula of the vestibulocerebellum. Ann N Y Acad Sci. (1999) 871:94–122. doi: 10.1111/j.1749-6632.1999.tb09178.x
60. Meng H, Blázquez PM, Dickman JD, Angelaki DE. Diversity of vestibular nuclei neurons targeted by cerebellar nodulus inhibition. J Physiol. (2014) 592:171–88. doi: 10.1113/jphysiol.2013.259614
61. Solomon D, Cohen B. Stimulation of the nodulus and uvula discharges velocity storage in the vestibulo-ocular reflex. Exp Brain Res. (1994) 102:57–68. doi: 10.1007/BF00232438
62. Heinen SJ, Oh DK, Keller EL. Characteristics of nystagmus evoked by electrical stimulation of the uvular/nodular lobules of the cerebellum in monkey. J Vestib Res. (1992) 2:235–45. doi: 10.3233/VES-1992-2306
63. Clément G, Tilikete C, Courjon J-H. Retention of habituation of vestibulo-ocular reflex and sensation of rotation in humans. Exp Brain Res. (2008) 190:307–15. doi: 10.1007/s00221-008-1471-0
64. Cohen H, Cohen B, Raphan T, Waespe W. Habituation and adaptation of the vestibuloocular reflex: a model of differential control by the vestibulocerebellum. Exp Brain Res. (1992) 90:526–38. doi: 10.1007/BF00230935
65. Jäger J, Henn V. Habituation of the vestibulo-ocular reflex (VOR) in the monkey during sinusoidal rotation in the dark. Exp Brain Res. (1981) 41:108–14. doi: 10.1007/BF00236599
66. Dai M, Raphan T, Cohen B. Adaptation of the angular vestibulo-ocular reflex to head movements in rotating frames of reference. Exp Brain Res. (2009) 195:553–67. doi: 10.1007/s00221-009-1825-2
67. Maruta J. Lasting alteration of spatial orientation induced by passive motion in rabbits and its possible relevance to mal de débarquement syndrome. Front Neurol. (2023) 14:1110298. doi: 10.3389/fneur.2023.1110298
68. Graybiel A. Angular velocities, angular accelerations, and Coriolis accelerations. In:Gazenko OG, Calvin M, , editors. Foundations of Space Biology and Medicine: Joint USA/USSR Publication. Washington, DC: National Aeronautics and Space Administration/Academy of Sciences of the USSR (1975). p. 247–304.
69. Guedry FE, Graybiel A. Compensatory nystagmus conditioned during adaptation to living in a rotating room. J Appl Physiol. (1962) 17:398–404. doi: 10.1152/jappl.1962.17.3.398
70. Reason JT. Motion sickness adaptation: a neural mismatch model. J R Soc Med. (1978) 71:819–29. doi: 10.1177/014107687807101109
71. Eron JN, Cohen B, Raphan T, Yakushin SB. Adaptation of orientation vectors of otolith-related central vestibular neurons to gravity. J Neurophysiol. (2008) 100:1686–90. doi: 10.1152/jn.90289.2008
73. Raymond JL, Lisberger SG, Mauk MD. The cerebellum: a neuronal learning machine? Science. (1996) 272:1126–31. doi: 10.1126/science.272.5265.1126
74. Dai M, Cohen B, Smouha E, Cho C. Readaptation of the vestibulo-ocular reflex relieves the mal de debarquement syndrome. Front Neurol. (2014) 5:124. doi: 10.3389/fneur.2014.00124
75. Maruta J, Cho C, Raphan T, Yakushin SB. Symptom reduction in mal de débarquement syndrome with attenuation of the velocity storage contribution in the central vestibular pathways. Front Rehabil Sci. (2024) 5:1331135. doi: 10.3389/fresc.2024.1331135
76. Yakushin SB, Raphan T, Cho C. Treatment of gravitational pulling sensation in patients with mal de debarquement syndrome (MdDS): a model-based approach. Front Integr Neurosci. (2022) 16:801817. doi: 10.3389/fnint.2022.801817
77. Dai M, Raphan T, Cohen B. Labyrinthine lesions and motion sickness susceptibility. Exp Brain Res. (2007) 178:477–87. doi: 10.1007/s00221-006-0759-1
78. Johnson WH, Meek J, Graybiel A. Effects of labyrinthectomy on canal sickness in squirrel monkey. Ann Otol Rhinol Laryngol. (1962) 71:289–98. doi: 10.1177/000348946207100201
79. Watanuki K, Takahashi M, Ikeda T. Perception of surrounding space controls posture, gaze, and sensation during Coriolis stimulation. Aviat Space Environ Med. (2000) 71:381–7.
80. Hain TC, Zee DS. Abolition of optokinetic afternystagmus by aminoglycoside ototoxicity. Ann Otol Rhinol Laryngol. (1991) 100:580–3. doi: 10.1177/000348949110000712
81. Zee DS, Yee RD, Robinson DA. Optokinetic responses in labyrinthine-defective human beings. Brain Res. (1976) 113:423–8. doi: 10.1016/0006-8993(76)90955-0
82. Ireland DJ, Jell RM. Optokinetic after-nystagmus in man after loss or reduction of labyrinthine function - a preliminary report. J Otolaryngol. (1982) 11:86–90.
83. Zasorin NL, Baloh RW, Yee RD, Honrubia V. Influence of vestibulo-ocular reflex gain on human optokinetic responses. Exp Brain Res. (1983) 51:271–4. doi: 10.1007/BF00237202
84. Cheung BS, Howard IP, Money KE. Visually-induced sickness in normal and bilaterally labyrinthine-defective subjects. Aviat Space Environ Med. (1991) 62:527–31.
85. Raphan T, Dai M, Maruta J, Waespe W, Henn V, Suzuki J-I, et al. Canal and otolith afferent activity underlying eye velocity responses to pitching while rotating. Ann NY Acad Sci. (1999) 871:181–94. doi: 10.1111/j.1749-6632.1999.tb09184.x
86. Money KE, Friedberg J. The role of the semicircular canals in causation of motion sickness and nystagmus in the dog. Can J Physiol Pharmacol. (1964) 42:793–801. doi: 10.1139/y64-089
87. Igarashi M, Takahashi M, Kubo T, Alford BR, Wright WK. Effect of off-vertical tilt and macular ablation on postrotatory nystagmus in the squirrel monkey. Acta Otolaryngol. (1980) 90:93–9. doi: 10.3109/00016488009131703
88. Janeke JB, Jongkees LB, Oosterveld WJ. Relationship between otoliths and nystagmus. Acta Otolaryngol. (1970) 69:1–6. doi: 10.3109/00016487009123330
89. Brizzee KR, Igarashi M. Effect of macular ablation on frequency and latency of motion-induced emesis in the squirrel monkey. Aviat Space Environ Med. (1986) 57:1066–70.
90. Igarashi M, Himi T, Kulecz WB, Kobayashi K. Role of otolith endorgans in the genesis of vestibular-visual conflict sickness (pitch) in the squirrel monkey (first report). Aviat Space Environ Med. (1987) 58:A207–211.
91. Dizio P, Lackner JR. Influence of gravitoinertial force level on vestibular and visual velocity storage in yaw and pitch. Vision Res. (1992) 32:111–20. doi: 10.1016/0042-6989(92)90119-4
92. DiZio P, Lackner JR, Evanoff JN. The influence of gravitoinertial force level on oculomotor and perceptual responses to sudden stop stimulation. Aviat Space Environ Med. (1987) 58:A224–230.
93. Oman CM, Pouliot CF, Natapoff A. Horizontal angular VOR changes in orbital and parabolic flight: human neurovestibular studies on SLS-2. J Appl Physiol. (1996) 81:69–81. doi: 10.1152/jappl.1996.81.1.69
94. Lackner JR, Graybiel A. The effective intensity of Coriolis, cross-coupling stimulation is gravitoinertial force dependent: implications for space motion sickness. Aviat Space Environ Med. (1986) 57:229–35.
95. DiZio P, Lackner JR, Evanoff JN. The influence of gravitoinertial force level on oculomotor and perceptual responses to Coriolis, cross-coupling stimulation. Aviat Space Environ Med. (1987) 58:A218–223.
96. Lackner JR, Graybiel A. Head movements in low and high gravitoinertial force environments elicit motion sickness: implications for space motion sickness. Aviat Space Environ Med. (1987) 58:A212–217.
97. Bard P, Woolsey CN, Snider RS, Mountcastle VB, Bromiley RB. Deliniation of central nervous mechanisms involved in motion sickness. Fed Proc. (1947) 6:72.
98. Wang SC, Chinn HI. Experimental motion sickness in dogs; importance of labyrinth and vestibular cerebellum. Am J Physiol. (1956) 185:617–23. doi: 10.1152/ajplegacy.1956.185.3.617
99. Uno A, Takeda N, Kitahara T, Sakata Y, Yamatodani A, Kubo T. Effects of vestibular cerebellum lesion on motion sickness in rats. Acta Otolaryngol. (2000) 120:386–9. doi: 10.1080/000164800750000612
100. Miller AD, Wilson VJ. Vestibular-induced vomiting after vestibulocerebellar lesions. Brain Behav Evol. (1983) 23:26–31. doi: 10.1159/000121484
101. Clément G, Deguine O, Bourg M, Pavy-LeTraon A. Effects of vestibular training on motion sickness, nystagmus, and subjective vertical. J Vestib Res. (2007) 17:227–37. doi: 10.3233/VES-2007-175-604
102. Dai M, Kunin M, Raphan T, Cohen B. The relation of motion sickness to the spatial temporal properties of velocity storage. Exp Brain Res. (2003) 151:173–89. doi: 10.1007/s00221-003-1479-4
103. Cohen B, Dai M, Yakushin SB, Raphan T. Baclofen, motion sickness susceptibility and the neural basis for velocity storage. Prog Brain Res. (2008) 171:543–53. doi: 10.1016/S0079-6123(08)00677-8
104. Cohen B, Helwig D, Raphan T. Baclofen and velocity storage: a model of the effects of the drug on the vestibulo-ocular reflex in the rhesus monkey. J Physiol. (1987) 393:703–25. doi: 10.1113/jphysiol.1987.sp016849
105. Waespe W, Schwarz U, Wolfensberger M. Firing characteristics of vestibular nuclei neurons in the alert monkey after bilateral vestibular neurectomy. Exp Brain Res. (1992) 89:311–22. doi: 10.1007/BF00228247
106. Yakushin SB, Raphan T, Büttner-Ennever JA, Suzuki J-I, Cohen B. Spatial properties of central vestibular neurons of monkeys after bilateral lateral canal nerve section. J Neurophysiol. (2005) 94:3860–71. doi: 10.1152/jn.01102.2004
107. Rolnick A, Lubow RE. Why is the driver rarely motion sick? The role of controllability in motion sickness. Ergonomics. (1991) 34:867–79. doi: 10.1080/00140139108964831
108. Bertolini G, Straumann D. Moving in a moving world: a review on vestibular motion sickness. Front Neurol. (2016) 7:14. doi: 10.3389/fneur.2016.00014
109. Cohen B, Dai M, Yakushin SB, Cho C. The neural basis of motion sickness. J Neurophysiol. (2019) 121:973–82. doi: 10.1152/jn.00674.2018
110. Machuca-Márquez P, Sánchez-Benito L, Menardy F, Urpi A, Girona M, Puighermanal E, et al. Vestibular CCK signaling drives motion sickness-like behavior in mice. Proc Natl Acad Sci USA. (2023) 120:e2304933120. doi: 10.1073/pnas.2304933120
111. Balaban CD, Porter JD. Neuroanatomic substrates for vestibulo-autonomic interactions. J Vestib Res. (1998) 8:7–16. doi: 10.3233/VES-1998-8102
112. Yates BJ, Catanzaro MF, Miller DJ, McCall AA. Integration of vestibular and emetic gastrointestinal signals that produce nausea and vomiting: potential contributions to motion sickness. Exp Brain Res. (2014) 232:2455–69. doi: 10.1007/s00221-014-3937-6
113. Oman CM, Cullen KE. Brainstem processing of vestibular sensory exafference: implications for motion sickness etiology. Exp Brain Res. (2014) 232:2483–92. doi: 10.1007/s00221-014-3973-2
114. Keller EL, Precht W. Visual-vestibular responses in vestibular nuclear neurons in the intact and cerebellectomized, alert cat. Neuroscience. (1979) 4:1599–613. doi: 10.1016/0306-4522(79)90023-X
115. Guedry FE. Visual counteraction on nauseogenic and disorienting effects of some whole-body motions: a proposed mechanism. Aviat Space Environ Med. (1978) 49:36–41. doi: 10.21236/ADA038767
116. Brandt T. Motion sickness. In:Brandt T, , editor. Vertigo: Its Multisensory Syndromes. London: Springer London (1991). p. 311–23.
117. Griffin MJ, Newman MM. Visual field effects on motion sickness in cars. Aviat Space Environ Med. (2004) 75:739–48.
118. Leger A, Money KE, Landolt JP, Cheung BS, Rodden BE. Motion sickness caused by rotations about Earth-horizontal and Earth-vertical axes. J Appl Physiol Respir Environ Exerc Physiol. (1981) 50:469–77. doi: 10.1152/jappl.1981.50.3.469
119. Cian C, Ohlmann T, Ceyte H, Gresty MA, Golding JF. Off vertical axis rotation motion sickness and field dependence. Aviat Space Environ Med. (2011) 82:959–63. doi: 10.3357/ASEM.3049.2011
120. Dichgans J, Brandt T. Optokinetic motion sickness and pseudo-Coriolis effects induced by moving visual stimuli. Acta Otolaryngol. (1973) 76:339–48. doi: 10.3109/00016487309121519
121. Igarashi M, McLeod ME, Graybiel A. Clinical pathological correlations in squirrel monkeys after suppression of semicircular canal function by streptomycin sulfate. Acta Otolaryngol. (1966) 1966(Suppl.214):1–28. doi: 10.3109/00016486609121825
122. Balaban CD. Vestibular nucleus projections to the parabrachial nucleus in rabbits: implications for vestibular influences on the autonomic nervous system. Exp Brain Res. (1996) 108:367–81. doi: 10.1007/BF00227260
Keywords: cerebellar nodulus, mal de débarquement, nystagmus, semicircular canals, space flight, spatial orientation, vestibular habituation, vestibulo-ocular reflex (VOR)
Citation: Maruta J (2024) On labyrinthine function loss, motion sickness immunity, and velocity storage. Front. Neurol. 15:1426213. doi: 10.3389/fneur.2024.1426213
Received: 30 April 2024; Accepted: 17 June 2024;
Published: 28 June 2024.
Edited by:
James Robert Lackner, Brandeis University, United StatesCopyright © 2024 Maruta. This is an open-access article distributed under the terms of the Creative Commons Attribution License (CC BY). The use, distribution or reproduction in other forums is permitted, provided the original author(s) and the copyright owner(s) are credited and that the original publication in this journal is cited, in accordance with accepted academic practice. No use, distribution or reproduction is permitted which does not comply with these terms.
*Correspondence: Jun Maruta, anVuLm1hcnV0YUBtc3NtLmVkdQ==
Disclaimer: All claims expressed in this article are solely those of the authors and do not necessarily represent those of their affiliated organizations, or those of the publisher, the editors and the reviewers. Any product that may be evaluated in this article or claim that may be made by its manufacturer is not guaranteed or endorsed by the publisher.
Research integrity at Frontiers
Learn more about the work of our research integrity team to safeguard the quality of each article we publish.