- 1School of Biomedical Sciences, The University of Queensland, St Lucia, QLD, Australia
- 2Queensland Brain Institute, The University of Queensland, St Lucia, QLD, Australia
Huntington's disease (HD) is a debilitating neurodegenerative condition characterized by motor, cognitive and psychiatric abnormalities. Immune dysregulation, prominently featuring increased immune activity, plays a significant role in HD pathogenesis. In addition to the central nervous system (CNS), systemic innate immune activation and inflammation are observed in HD patients, exacerbating the effects of the Huntingtin (HTT) gene mutation. Recent attention to sex differences in HD symptom severity underscores the need to consider gender as a biological variable in neurodegenerative disease research. Understanding sex-specific immune responses holds promise for elucidating HD pathophysiology and informing targeted treatment strategies to mitigate cognitive and functional decline. This perspective will highlight the importance of investigating gender influence in HD, particularly focusing on sex-specific immune responses predisposing individuals to disease.
Introduction
Huntington's disease (HD) is the most common inherited neurodegenerative disease, characterized by uncontrolled choreatic movements, behavioral and psychiatric disturbances, and dementia (1–3). While symptoms typically emerge in middle age, they can manifest at any point from infancy to old age (3). The mutation responsible for HD is an expanded cytosine-adenine-guanine (CAG) trinucleotide repeat in the huntingtin (Htt) gene leading to an abnormally long polyglutamine tail that confers a toxic gain of function (1, 3). As it is an autosomal dominant inherited disease, there is an equal penetrance and prevalence in men and women in the population (4). Notably however, recent findings from a large American cohort study comprising 3,707 HD individuals revealed a slightly higher prevalence among females compared to males (7.05 vs. 6.10 per 100,000 persons, respectively), suggesting a possible relationship between sex and HD (5). Moreover, evidence from various animal models and epidemiologic cohorts suggests that sex may contribute to the variability in disease manifestation between men and women with HD (4).
Sex differences in HD
Sex differences in HD are multifaceted, encompassing various aspects of disease progression and symptomatology. Notably, its role in disease anticipation has been observed, with paternal transmission resulting in earlier onset and faster progression compared to maternal transmission due to CAG repeat instability primarily during spermatogenesis (6, 7). Consequently, large expansions in CAG repeats predominantly occur through paternal transmission, while offspring of affected mothers are more likely to show no change or contractions in CAG length (8). Clinical observations from large-scale studies, such as the REGISTRY (European Huntington's Disease Network) and ENROLL-HD databases, consistently report that females with HD present with more symptoms, exhibit a slightly more severe phenotype, and experience a faster rate of progression, particularly in motor and functional domains (9, 10). Moreover, another study based on the REGISTRY database found that motor symptoms have a more pronounced impact on functional ability and independence in women with HD compared to men (7, 11, 12). Furthermore, analyses of the PREDICT-HD using REGISTRY and ENROLL-HD databases demonstrated that depression is more prevalent and severe in females with HD (9, 10, 13).
Sex hormones, for example testosterone, have also been suggested to have neuroprotective effects. Reduced plasma testosterone levels in males with HD correlate with increased disease severity and cognitive impairment (14), while decreased plasma testosterone levels in females are associated with depression (6, 11, 15). In contrast to human studies, animal studies suggest that estrogen exerts neuroprotective effects against mechanisms underlying HD development, specifically oxidative stress and glutamate excitotoxicity (4, 16). Specifically, 17β-estradiol has been shown to protect against markers of oxidative stress in models of HD, with females exhibiting less severe motor dysfunction compared to males (17, 18). However, it remains unclear which sex hormones are neuroprotective and which are neurotoxic. More research is needed to understand the role of sex hormones in HD pathologies.
However, contradicting results regarding sex-related differences in HD have been reported. A study conducted by the Huntington Study Group found no significant sex-related effects on function, motor, cognitive and behavioral domains in a large cohort of HD patients (6, 19). While hormonal differences may not be the direct contributor of the sex-specific symptoms or disease progression, sex steroids can regulate the transcription of genes relevant to immune cell development, immune responses, and immune signaling pathways (20). Emerging evidence suggests that immune cell size, morphology, and function, as well as glial cells and inflammation markers may exhibit sex-specific characteristics, highlighting the intricate interplay between sex hormones, immune responses, and HD pathology.
Neuroinflammation in HD
Neuroinflammation is a major hallmark for HD and is characterized by the reactive morphology of glial cells: microglia, astrocytes, and oligodendrocytes. These cells are vital for regulating neuronal activity and maintaining optimal neuronal function (21). Despite their crucial roles, activated microglia and reactive astrocytes have been implicated in HD pathology, perpetuating a chronic inflammatory state by upregulating proinflammatory genes, ultimately, contributing to neuronal death observed in HD progression (21).
Microglia expressing mutant Htt (mHtt) in HD play a neurotoxic role by inducing a proinflammatory response, characterized by increased expression of Interleukin (IL)-6, Tumor Necrosis Factor (TNF)-α and reactive oxygen species (ROS) (22). Similarly, reactive astrocytes in HD produce proinflammatory and harmful molecules such as TNF-α, IL-6, ROS, and mHtt aggregates, exacerbating neuroinflammation (22). Dysfunction of astrocyte in HD, marked by deficits in calcium signaling, iron homeostasis and neurotransmitter clearance, further contributes to disease pathology (22, 23).
Oligodendrocytes are the myelinating glia of the CNS that play crucial roles in rapid transmission of electrical signals between neurons and providing trophic support for neurons (24–26). In mouse models of HD, early structural impairments in myelin have been observed, accompanied by significant reductions in the expression of myelin-related genes in striatal and cortical tissues, as well as isolated oligodendrocytes from HD mice (24, 27–30). These myelination deficits likely impair the transmission of information across neuronal circuits, as seen by motor performance impairments in mice expressing mHTT specifically in oligodendroglia cells and the rescue of various HD-related pathologies when it is genetically excised from HD mice (24, 27, 31). While the functional roles of microglia, astrocytes, and oligodendrocytes in HD progression have been extensively reviewed (32, 33), emerging evidence suggests sex differences in these glial cells may impact neurodegenerative disease outcomes. Here, we focus on the sex-specific role of these cells in HD progression, a topic that has not been extensively discussed previously (Figure 1).
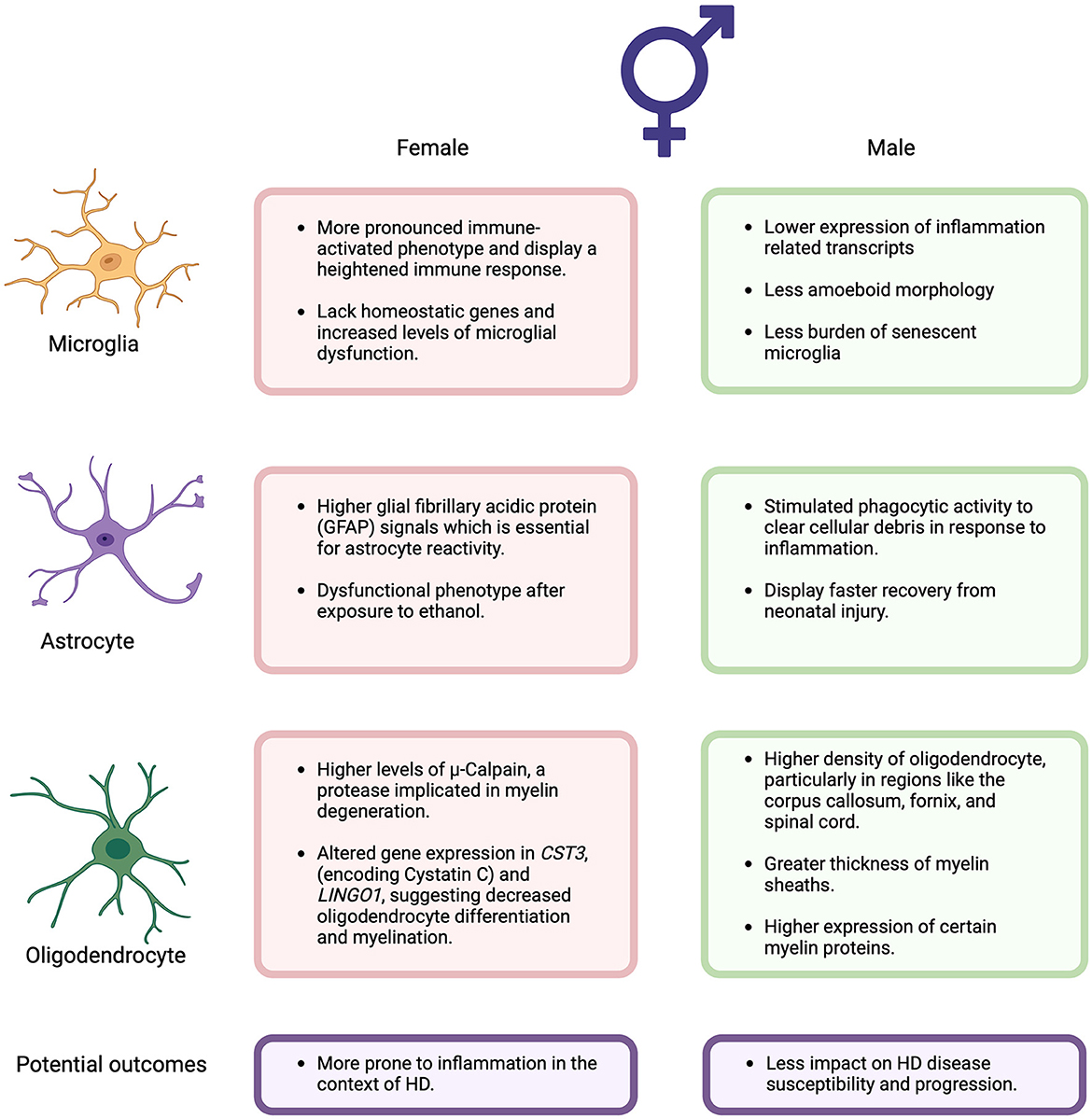
Figure 1. Summary of the sex differences in the potential functional roles of microglia, astrocytes, and oligodendrocytes associated with Huntington's Disease discussed in this perspective. Figure created using BioRender (Biorender.com).
Sex differences in neuroinflammation
Sex differences in microglia
Independent of hormonal signals, microglia from female mice have been found to be neuroprotective, limiting the damage caused by acute focal cerebral (34). However, emerging evidence suggests that microglia from mature females adopt a more immune-activated phenotype, consistent with the finding that females have a stronger immune response than males (35–37). This is supported by studies of the aging brain where microglia in females have higher expression of inflammation-related transcripts compared to males (38, 39). Additionally, female rats exhibit a greater proportion of microglia with an amoeboid morphology and thicker processes in specific brain regions such as the hippocampus, amygdala, and parietal cortex (35, 40). Moreover, female mice demonstrate enriched microglia-specific gene expression in the hippocampus relative to males, with higher expression of genes associated with inflammation and apoptosis in female-derived microglia (35, 41). Furthermore, studies indicate that female mice lack homeostatic genes across different life stages (juveniles, adults and in old age), leading to increased levels of microglial dysfunction in aged females compared to males (39, 42). Aged females also possess a greater burden of senescent microglia, which may contribute to the development of neurodegenerative diseases and a more rapid and severe disease course in females (39, 42, 43). While research on sex differences in microglia in HD remains limited compared to other neurodegenerative diseases like Alzheimer's disease (AD), studies in AD have shown that aged female donors display higher expression of gene signatures related to inflammatory response and proinflammatory immune responses in microglia nuclei compared to males (44). Additionally, functional studies involving microglial transplants and analyses of microglial Ca2+ signaling and process motility suggest that female mice exhibit more rapid aging of microglia than males (38, 45). These findings indicate that female-derived microglia may be more prone to proinflammation activation and dysfunction, potentially contributing to the progression of neurodegenerative diseases like HD.
Sex differences in astrocytes
Astrocytes have also been found to have sex differences in their response to inflammation, which may have implications in HD progression. Upregulation of the intermediate filament glial fibrillary acidic protein (GFAP) is essential for the transition of astrocytes to a reactive state (46). A recent study identified a significant elevation in plasma GFAP concentration in HD patients with the HTT mutation, suggesting its association with HD progression (47). Female mice were found to have significantly higher GFAP signals than males (48, 49), indicating that female astrocytes may be more prone to inflammation than males in the context of HD. In contrast, in vitro studies have shown that female astrocytes exhibit enhanced expression of anti-inflammatory cytokine, interferon-gamma-inducible protein 10 (IP-10/CXCL10), in response to Lipopolysaccharides (LPS) (50). Whereas male astrocytes show enhanced expression of pro-inflammatory markers including IL-6, TNF-α and IL-1β, after LPS treatment (50). Nevertheless, the sex-dependent changes in astrocytes contributing to neuroprotection or neurotoxicity are complex and still largely unknown.
Recent studies have revealed sex differences in the functional role of astrocyte such as phagocytosis. In vitro studies have shown that male-derived astrocytes are stimulated to phagocytose brain-derived cellular debris, to re-establish tissue homeostasis in response to inflammation, whereas phagocytic activity is inhibited in female-derived astrocytes (38, 51). Moreover, phagocytic responses in primary astrocytes obtained from HD mouse models progressively decrease over time (52). Exposure to ethanol induces a dysfunctional phenotype in female astrocytes but not in males (38, 53). Conversely, primary cultures from newborn rats suggest that female astrocytes may exhibit greater resistance to oxygen-glucose deprivation-induced cell death, indicating a better metabolic adaptation response to insults (38, 54). Furthermore after in vivo neonatal hypoxia-ischemia, female astrocytes demonstrate enhanced mitochondrial metabolism compared to males (38, 55), although males display faster recovery from the neonatal injury (38, 55). These findings suggest that sex differences in astrocytes may have significant functional consequences for HD progression, particularly in response to non-homeostatic disease states.
Sex differences in oligodendrocytes
Oligodendrocytes display numerous sex differences in neurodegenerative diseases, potentially impacting disease susceptibility and progression. Firstly, studies in rats have shown higher density of oligodendrocytes in male rats compared to females in regions such as the corpus callosum, fornix and spinal cord (38, 56). This difference is associated with greater thickness of myelin sheaths and higher expression of certain myelin proteins in males, along with a shorter lifespan of female oligodendrocytes (38, 56). Moreover, females exhibit significantly higher transcriptional and translational levels of μ-Calpain, a protease implicated in the degeneration of myelin, compared to males (56). In AD, single-cell transcriptome analyses have revealed sex differences in oligodendrocytes. Males with AD display increased pathology associated with global transcriptional activation in oligodendrocytes, a phenomenon not observed in females (38, 57). Additionally, female oligodendrocyte precursors demonstrate a down-regulation shift in response to pathology that is absent in male cells (38, 57).
Furthermore, a single-cell RNA-seq analysis in AD identified sex differences in gene expression patterns of oligodendrocytes. CST3, encoding Cystatin C, is upregulated in neurons and oligodendrocytes of AD males but downregulated in AD females (58). Cystatin C is involved in membrane structure and cellular signaling, contributing to blood brain barrier integrity and reduced permeability (59). Reduced levels of CSF cystatin C have been linked to increased susceptibility to neurodegeneration (60). A recent single-nucleus RNAseq study from HD and control post-mortem brain tissues showed that expression of CST3 is downregulated in the oligodendrocytes and oligodendrocyte precursors (OPCs) of the HD caudate (61). Thus, as cystatin C is downregulated in female oligodendrocytes, this suggests that females are more vulnerable to AD pathology and likely other neurodegenerative diseases, like HD (58). Additionally, Leucine-rich repeat and immunoglobulin-like domain-containing nogo receptor-interacting protein 1 (LINGO1) were found to be upregulated in AD females but not in males (58). LINGO1 is a negative modulator of neuronal survival, axonal integrity and myelination (62, 63). As AD females exhibit upregulated levels of LINGO1, they may have decreased oligodendrocyte differentiation and myelination (63). The single-nucleus RNAseq study from HD and control post-mortem brain tissues also showed that expression of LINGO1 is up-regulated in the oligodendrocytes and OPCs of the HD caudate and cingulate (61). Altogether, these studies indicate that oligodendrocytes differentiation and myelination, as well as the response to pathology, are sex-dependent, with females being more susceptible to these changes and therefore to neurodegenerative diseases, such as HD.
Potential sex-specific treatment for HD
Sex-specific responses to treatments in HD highlight the importance of considering sex as a biological variable in therapeutic development. While studies have found no sex differences in the safety and efficacy of certain treatments like tetrabenazine, for managing chorea (6, 64–66), there may be variations in dosage and treatment duration between males and females. For example, preclinical studies in zQ175 HD mice have shown that both sexes benefit from mGluR5 negative allosteric modulator treatment, but females required a longer treatment duration for optimal efficacy (6, 67). Regarding the treatment of mood disorders, it has been reported from the PREDICT-HD study that females with HD are more likely to use anxiolytics and antidepressants than males at early disease stages of HD (68), possibly due to greater disease severity and progression.
Emerging evidence suggests that targeting glial cell dysfunction, with consideration for sex-specific differences, may offer novel interventions in treating HD patients. Inhibition of phagocytosis activity in female astrocytes (38, 51), coupled with progressively reduced phagocytic responses in both R6/2 and zQ175 HD mouse models (52), underscores the potential benefits of modulating astrocytic phagocytosis for female HD patients. Tibolone, a synthetic steroid used for menopausal symptoms (69), has shown promise in stimulating phagocytosis activity in both sexes, with greater effect observed in females (38). Importantly, tibolone's mechanism of action varies between male and female cells, indicating potential sex-specific therapeutic strategies (51).
While therapeutic approaches targeting oligodendrocytes in HD are currently lacking, research suggests that females may have decreased oligodendrocyte differentiation and myelination, rendering them potentially more susceptible to HD progression (63). Repurposing existing drugs that promote oligodendrocyte progenitor cell differentiation and myelination, such as clemastine fumarate, could offer therapeutic avenues for HD. Clemastine fumarate has demonstrated myelin repair potential in multiple sclerosis patients, indicating a possible role in slowing neurodegenerative disease progression (70, 71). However, further research is needed to evaluate its efficacy and safety for HD treatment.
Conclusion
In conclusion, sex differences in HD present a complex and multifaceted landscape. While female HD patients often exhibit a higher prevalence and more severe disease symptoms, contradictory results from animal models and clinical studies underscore the need for deeper investigation. The role of sex differences in neuroinflammation, particularly the heightened proinflammatory response and sensitivity to dysregulation observed in female microglia and oligodrocytes, may contribute to the worsened disease phenotype in females. In contrast, while astrocytes display sex-specific changes, there is limited knowledge about the functional consequences of these changes in neurodegeneration. However, the conflicting nature of existing research highlights the necessity for additional studies to fully elucidate the impact of sex differences in neuroinflammation on HD progression.
Current pharmacological interventions hold promise in modulating glial function, potentially offering sex-specific effects. Nonetheless, a deeper understanding of their mechanisms of action and optimisation for different patient populations is imperative. Future drug development endeavors may benefit from a focus on designing treatments that specifically target glial cells, with careful consideration for sex-specific differences. Ultimately, advancing our understanding of sex differences in HD and developing tailored therapeutic strategies hold the key to improving outcomes for all patients affected by this debilitating neurodegenerative disease.
Data availability statement
The original contributions presented in the study are included in the article/supplementary material, further inquiries can be directed to the corresponding author.
Author contributions
GR-J: Data curation, Writing – original draft, Writing – review & editing. JL: Conceptualization, Supervision, Writing – original draft, Writing – review & editing. TW: Funding acquisition, Supervision, Writing – original draft, Writing – review & editing. JF: Conceptualization, Data curation, Supervision, Visualization, Writing – original draft, Writing – review & editing.
Funding
The author(s) declare that financial support was received for the research, authorship, and/or publication of this article. This work was supported by a National Health and Medical Research Council grant (2009957 to TW).
Conflict of interest
The authors declare that the research was conducted in the absence of any commercial or financial relationships that could be construed as a potential conflict of interest.
The author(s) declared that they were an editorial board member of Frontiers, at the time of submission. This had no impact on the peer review process and the final decision.
Publisher's note
All claims expressed in this article are solely those of the authors and do not necessarily represent those of their affiliated organizations, or those of the publisher, the editors and the reviewers. Any product that may be evaluated in this article, or claim that may be made by its manufacturer, is not guaranteed or endorsed by the publisher.
References
1. Finkbeiner S. Huntington's disease. Cold Spring Harb Perspect Biol. (2011) 3:a007476. doi: 10.1101/cshperspect.a007476
2. Roos RA. Huntington's disease: a clinical review. Orphanet J Rare Dis. (2010) 5:1–8. doi: 10.1186/1750-1172-5-40
4. Smith KM, Dahodwala N. Sex differences in Parkinson's disease and other movement disorders. Exp Neurol. (2014) 259:44–56. doi: 10.1016/j.expneurol.2014.03.010
5. Bruzelius E, Scarpa J, Zhao Y, Basu S, Faghmous JH, Baum A. Huntington's disease in the United States: variation by demographic and socioeconomic factors. Mov. Disord. (2019) 34:858–65. doi: 10.1002/mds.27653
6. Rocha NP, Teixeira AL, Furr Stimming E. Sex differences in huntington's disease: considerations for clinical care and research trials. In:Thomas EA, Parkin GM, , editors. Biomarkers for Huntington's Disease: Improving Clinical Outcomes. Cham: Springer (2023), p. 459–72. doi: 10.1007/978-3-031-32815-2_19
7. Zielonka D, Stawinska-Witoszynska B. Gender differences in non-sex linked disorders: insights from Huntington's disease. Front Neurol. (2020) 11:571. doi: 10.3389/fneur.2020.00571
8. Aziz NA, van Belzen MJ, Coops ID, Belfroid RD, Roos RA. Parent-of-origin differences of mutant HTT CAG repeat instability in Huntington's disease. Eur J Med Genet. (2011) 54:e413–8. doi: 10.1016/j.ejmg.2011.04.002
9. Hentosh S, Zhu L, Patino J, Furr JW, Rocha NP, Furr Stimming E. Sex differences in Huntington's disease: evaluating the enroll-HD database. Mov Disord Clin Pract. (2021) 8:420–6. doi: 10.1002/mdc3.13178
10. Zielonka D, Marinus J, Roos RA, De Michele G, Di Donato S, Putter H, et al. The influence of gender on phenotype and disease progression in patients with Huntington's disease. Parkinsonism Relat Disord. (2013) 19:192–7. doi: 10.1016/j.parkreldis.2012.09.012
11. Meoni S, Macerollo A, Moro E. Sex differences in movement disorders. Nat Rev Neurol. (2020) 16:84–96. doi: 10.1038/s41582-019-0294-x
12. Zielonka D, Ren M, De Michele G, Roos RA, Squitieri F, Bentivoglio AR, et al. The contribution of gender differences in motor, behavioral and cognitive features to functional capacity, independence and quality of life in patients with Huntington's disease. Parkinsonism Relat Disord. (2018) 49:42–7. doi: 10.1016/j.parkreldis.2018.01.006
13. Epping EA, Mills JA, Beglinger LJ, Fiedorowicz JG, Craufurd D, Smith MM, et al. Characterization of depression in prodromal Huntington disease in the neurobiological predictors of HD (PREDICT-HD) study. J Psychiatr Res. (2013) 47:1423–31. doi: 10.1016/j.jpsychires.2013.05.026
14. Markianos M, Panas M, Kalfakis N, Vassilopoulos D. Plasma testosterone in male patients with Huntington's disease: relations to severity of illness and dementia. Ann Neurol. (2005) 57:520–5. doi: 10.1002/ana.20428
15. Markianos M, Panas M, Kalfakis N, Vassilopoulos D. Plasma testosterone, dehydroepiandrosterone sulfate, and cortisol in female patients with Huntington's disease. Neuroendocrinol Lett. (2007) 28:199–203.
16. Weaver CE Jr, Park-Chung M, Gibbs TT, Farb DH. 17β-Estradiol protects against NMDA-induced excitotoxicity by direct inhibition of NMDA receptors. Brain Res. (1997) 761:338–41. doi: 10.1016/S0006-8993(97)00449-6
17. Heron P, Daya S. 17β-Estradiol protects against quinolinic acid-induced lipid peroxidation in the rat brain. Metab Brain Dis. (2000) 15:247–74. doi: 10.1023/a:1011119107765
18. Túnez I, Collado JA, Medina FJ, Peña J, Muñoz MC, Jimena I, et al. 17 β-Estradiol may affect vulnerability of striatum in a 3-nitropropionic acid-induced experimental model of Huntington's disease in ovariectomized rats. Neurochem Int. (2006) 48:367–73. doi: 10.1016/j.neuint.2005.11.011
19. Marder K, Zhao H, Myers R, Cudkowicz M, Kayson E, Kieburtz K, et al. Rate of functional decline in Huntington's disease. Neurology. (2000) 54:452. doi: 10.1212/WNL.54.2.452
20. van Lunzen J, Altfeld M. Sex differences in infectious diseases–common but neglected. J Infect Dis. (2014) 209(suppl_3):S79–80. doi: 10.1093/infdis/jiu159
21. Palpagama TH, Waldvogel HJ, Faull RL, Kwakowsky A. The role of microglia and astrocytes in Huntington's disease. Front Mol Neurosci. (2019) 12:258. doi: 10.3389/fnmol.2019.00258
22. Saba J, Couselo FL, Bruno J, Carniglia L, Durand D, Lasaga M, et al. Neuroinflammation in Huntington's disease: a starring role for astrocyte and microglia. Curr Neuropharmacol. (2022) 20:1116. doi: 10.2174/1570159X19666211201094608
23. Khakh BS, Beaumont V, Cachope R, Munoz-Sanjuan I, Goldman SA, Grantyn R. Unravelling and exploiting astrocyte dysfunction in Huntington's disease. Trends Neurosci. (2017) 40:422–37. doi: 10.1016/j.tins.2017.05.002
24. Wilton DK, Stevens B. The contribution of glial cells to Huntington's disease pathogenesis. Neurobiol Dis. (2020) 143:104963. doi: 10.1016/j.nbd.2020.104963
25. Meyer N, Richter N, Fan Z, Siemonsmeier G, Pivneva T, Jordan P, et al. Oligodendrocytes in the mouse corpus callosum maintain axonal function by delivery of glucose. Cell Rep. (2018) 22:2383–94. doi: 10.1016/j.celrep.2018.02.022
26. Philips T, Rothstein JD. Oligodendroglia: metabolic supporters of neurons. J Clin Invest. (2017) 127:3271–80. doi: 10.1172/JCI90610
27. Bardile CF, Garcia-Miralles M, Caron N, Langley S, Teo RTY, Petretto E, et al. A43 intrinsic mutant HTT-mediated defects in oligodendroglia cells contribute to myelin deficits and behavioural abnormalities in huntington disease. BMJ. (2018) 25:24–34. doi: 10.1136/jnnp-2018-EHDN.41
28. Garcia-Miralles M, Yusof NABM, Tan JY, Radulescu CI, Sidik H, Tan LJ, et al. Laquinimod treatment improves myelination deficits at the transcriptional and ultrastructural levels in the YAC128 mouse model of Huntington disease. Mol Neurobiol. (2019) 56:4464–78. doi: 10.1007/s12035-018-1393-1
29. Jin J, Peng Q, Hou Z, Jiang M, Wang X, Langseth AJ, et al. Early white matter abnormalities, progressive brain pathology and motor deficits in a novel knock-in mouse model of Huntington's disease. Hum Mol Genet. (2015) 24:2508–27. doi: 10.1093/hmg/ddv016
30. Teo RTY, Hong X, Yu-Taeger L, Huang Y, Tan LJ, Xie Y, et al. Structural and molecular myelination deficits occur prior to neuronal loss in the YAC128 and BACHD models of Huntington disease. Hum Mol Genet. (2016) 25:2621–32. doi: 10.1093/hmg/ddw122
31. Huang B, Wei W, Wang G, Gaertig MA, Feng Y, Wang W, et al. Mutant huntingtin downregulates myelin regulatory factor-mediated myelin gene expression and affects mature oligodendrocytes. Neuron. (2015) 85:1212–26. doi: 10.1016/j.neuron.2015.02.026
32. Lee JD, Lo MW, Fung JN, Woodruff TM. Neuroinflammation in Huntington's disease. In:Peplow PV, Martinez B, Gennarelli TA, , editors. Neurodegenerative Diseases Biomarkers: Towards Translating Research to Clinical Practice. New York, NY: Springer (2022), p. 215–33. doi: 10.1007/978-1-0716-1712-0_9
33. Ferrari Bardile C, Garcia-Miralles M, Caron NS, Rayan NA, Langley SR, Harmston N, et al. Intrinsic mutant HTT-mediated defects in oligodendroglia cause myelination deficits and behavioral abnormalities in Huntington disease. Proc Nat Acad Sci. (2019) 116:9622–7. doi: 10.1073/pnas.1818042116
34. Villa A, Gelosa P, Castiglioni L, Cimino M, Rizzi N, Pepe G, et al. Sex-specific features of microglia from adult mice. Cell Rep. (2018) 23:3501–11. doi: 10.1016/j.celrep.2018.05.048
35. Delage C, Rendina DN, Malacon KE, Tremblay M-È, Bilbo SD. Sex differences in microglia as a risk factor for Alzheimer's disease. In:Ferretti MT, Dimech AS, Chadha AS, , editor. Sex and Gender Differences in Alzheimer's Disease. Cambridge, MA: Academic Press (2021), p. 79–104. doi: 10.1016/B978-0-12-819344-0.00008-9
36. Gaillard R, Spinedi E. Sex-and stress-steroids interactions and the immune system: evidence for a neuroendocrine-immunological sexual dimorphism. Domest Anim Endocrinol. (1998) 15:345–52. doi: 10.1016/S0739-7240(98)00028-9
37. Jaillon S, Berthenet K, Garlanda C. Sexual dimorphism in innate immunity. Clin Rev Allergy Immunol. (2019) 56:308–21. doi: 10.1007/s12016-017-8648-x
38. Chowen JA, Garcia-Segura LM. Role of glial cells in the generation of sex differences in neurodegenerative diseases and brain aging. Mech Ageing Dev. (2021) 196:111473. doi: 10.1016/j.mad.2021.111473
39. Mangold CA, Wronowski B, Du M, Masser DR, Hadad N, Bixler GV, et al. Sexually divergent induction of microglial-associated neuroinflammation with hippocampal aging. J Neuroinflammation. (2017) 14:1–19. doi: 10.1186/s12974-017-0920-8
40. Schwarz JM, Sholar PW, Bilbo SD. Sex differences in microglial colonization of the developing rat brain. J Neurochem. (2012) 120:948–63. doi: 10.1111/j.1471-4159.2011.07630.x
41. Hanamsagar R, Alter MD, Block CS, Sullivan H, Bolton JL, Bilbo SD. Generation of a microglial developmental index in mice and in humans reveals a sex difference in maturation and immune reactivity. Glia. (2017) 65:1504–20. doi: 10.1002/glia.23176
42. Doust YV, King AE, Ziebell JM. Implications for microglial sex differences in tau-related neurodegenerative diseases. Neurobiol Aging. (2021) 105:340–8. doi: 10.1016/j.neurobiolaging.2021.03.010
43. Nissen JC. Microglial function across the spectrum of age and gender. Int J Mol Sci. (2017) 18:561. doi: 10.3390/ijms18030561
44. Coales I, Tsartsalis S, Fancy N, Weinert M, Clode D, Owen D, et al. Alzheimer's disease-related transcriptional sex differences in myeloid cells. J Neuroinflammation. (2022) 19:247. doi: 10.1186/s12974-022-02604-w
45. Olmedillas del Moral M, Fröhlich N, Figarella K, Mojtahedi N, Garaschuk O. Effect of caloric restriction on the in vivo functional properties of aging microglia. Front Immunol. (2020) 11:750. doi: 10.3389/fimmu.2020.00750
46. Pekny M, Nilsson M. Astrocyte activation and reactive gliosis. Glia. (2005) 50:427–34. doi: 10.1002/glia.20207
47. You H, Wu T, Du G, Huang Y, Zeng Y, Lin L, et al. Evaluation of blood glial fibrillary acidic protein as a potential marker in Huntington's disease. Front Neurol. (2021) 12:779890. doi: 10.3389/fneur.2021.779890
48. Cordeau P Jr, Lalancette-Hébert M, Weng YC, Kriz J. Live imaging of neuroinflammation reveals sex and estrogen effects on astrocyte response to ischemic injury. Stroke. (2008) 39:935–42. doi: 10.1161/STROKEAHA.107.501460
49. Ullah MF, Ahmad A, Bhat SH, Abu-Duhier FM, Barreto GE, Ashraf GM. Impact of sex differences and gender specificity on behavioral characteristics and pathophysiology of neurodegenerative disorders. Neurosci Biobehav Rev. (2019) 102:95–105. doi: 10.1016/j.neubiorev.2019.04.003
50. Santos-Galindo M, Acaz-Fonseca E, Bellini MJ, Garcia-Segura LM. Sex differences in the inflammatory response of primary astrocytes to lipopolysaccharide. Biol Sex Differ. (2011) 2:7. doi: 10.1186/2042-6410-2-7
51. Crespo-Castrillo A, Garcia-Segura L-M, Arevalo M-A. The synthetic steroid tibolone exerts sex-specific regulation of astrocyte phagocytosis under basal conditions and after an inflammatory challenge. J Neuroinflammation. (2020) 17:1–14. doi: 10.1186/s12974-020-1719-6
52. Wakida NM, Lau AL, Nguyen J, Cruz GMS, Fote GM, Steffan JS, et al. Diminished LC3-associated phagocytosis by Huntington's disease striatal astrocytes. J Huntingtons Dis. (2022) 11:25–33. doi: 10.3233/JHD-210502
53. Wilhelm CJ, Hashimoto JG, Roberts ML, Bloom SH, Andrew MR, Wiren KM. Astrocyte dysfunction induced by alcohol in females but not males. Brain Pathol. (2016) 26:433–51. doi: 10.1111/bpa.12276
54. Liu M, Hurn PD, Roselli CE, Alkayed NJ. Role of P450 aromatase in sex-specific astrocytic cell death. J Cereb Blood Flow Metab. (2007) 27:135–41. doi: 10.1038/sj.jcbfm.9600331
55. Morken TS, Brekke E, Håberg A, Widerøe M, Brubakk A-M, Sonnewald U. Altered astrocyte–neuronal interactions after hypoxia-ischemia in the neonatal brain in female and male rats. Stroke. (2014) 45:2777–85. doi: 10.1161/STROKEAHA.114.005341
56. Cerghet M, Skoff RP, Bessert D, Zhang Z, Mullins C, Ghandour MS. Proliferation and death of oligodendrocytes and myelin proteins are differentially regulated in male and female rodents. J Neurosci. (2006) 26:1439–47. doi: 10.1523/JNEUROSCI.2219-05.2006
57. Mathys H, Davila-Velderrain J, Peng Z, Gao F, Mohammadi S, Young JZ, et al. Single-cell transcriptomic analysis of Alzheimer's disease. Nature. (2019) 570:332–7. doi: 10.1038/s41586-019-1195-2
58. Belonwu SA, Li Y, Bunis D, Rao AA, Solsberg CW, Tang A, et al. Sex-stratified single-cell RNA-Seq analysis identifies sex-specific and cell type-specific transcriptional responses in Alzheimer's disease across two brain regions. Mol Neurobiol. (2022) 59:276–93. doi: 10.21203/rs.3.rs-418653/v1
59. Zi M, Xu Y. Involvement of cystatin C in immunity and apoptosis. Immunol Lett. (2018) 196:80–90. doi: 10.1016/j.imlet.2018.01.006
60. Hoghooghi V, Palmer AL, Frederick A, Jiang Y, Merkens JE, Balakrishnan A, et al. Cystatin C plays a sex-dependent detrimental role in experimental autoimmune encephalomyelitis. Cell Rep. (2020) 33:108236. doi: 10.1016/j.celrep.2020.108236
61. Lim RG, Al-Dalahmah O, Wu J, Gold MP, Reidling JC, Tang G, et al. Huntington disease oligodendrocyte maturation deficits revealed by single-nucleus RNAseq are rescued by thiamine-biotin supplementation. Nat Commun. (2022) 13:7791. doi: 10.1038/s41467-022-35388-x
62. Fernandez-Enright F, Andrews JL. Lingo-1: a novel target in therapy for Alzheimer's disease? Neural Regen Res. (2016) 11:88. doi: 10.4103/1673-5374.175048
63. Mi S, Miller RH, Lee X, Scott ML, Shulag-Morskaya S, Shao Z, et al. LINGO-1 negatively regulates myelination by oligodendrocytes. Nat Neurosci. (2005) 8:745–51. doi: 10.1038/nn1460
64. Frank S. Tetrabenazine as anti-chorea therapy in Huntington disease: an open-label continuation study. Huntington Study Group/TETRA-HD Investigators. BMC Neurol. (2009) 9:1–10. doi: 10.1186/1471-2377-9-62
65. Frank S, Ondo W, Fahn S, Hunter C, Oakes D, Plumb S, et al. A study of chorea after tetrabenazine withdrawal in patients with Huntington disease. Clin Neuropharmacol. (2008) 31:127–33. doi: 10.1097/WNF.0b013e3180ca77ea
66. Group HS. Tetrabenazine as antichorea therapy in Huntington disease: a randomized controlled trial. Neurology. (2006) 66:366–72. doi: 10.1212/01.wnl.0000198586.85250.13
67. Li SH, Colson T-LL, Abd-Elrahman KS, Ferguson SS. Metabotropic glutamate receptor 5 antagonism reduces pathology and differentially improves symptoms in male and female heterozygous zQ175 Huntington's mice. Front Mol Neurosci. (2022) 15:801757. doi: 10.3389/fnmol.2022.801757
68. Rowe KC, Paulsen JS, Langbehn DR, Wang C, Mills J, Beglinger LJ, et al. Patterns of serotonergic antidepressant usage in prodromal Huntington disease. Psychiatry Res. (2012) 196:309–14. doi: 10.1016/j.psychres.2011.09.005
69. Del Río JP, Molina S, Hidalgo-Lanussa O, Garcia-Segura LM, Barreto GE. Tibolone as hormonal therapy and neuroprotective agent. Trends Endocrinol Metab. (2020) 31:742–59. doi: 10.1016/j.tem.2020.04.007
70. Green AJ, Gelfand JM, Cree BA, Bevan C, Boscardin WJ, Mei F, et al. Clemastine fumarate as a remyelinating therapy for multiple sclerosis (ReBUILD): a randomised, controlled, double-blind, crossover trial. Lancet. (2017) 390:2481–9. doi: 10.1016/S0140-6736(17)32346-2
Keywords: Huntington (disease), neuroinflammation, sex difference, microglia, oligodendrocytes (OLs), astrocytes
Citation: Risby-Jones G, Lee JD, Woodruff TM and Fung JN (2024) Sex differences in Huntington's disease from a neuroinflammation perspective. Front. Neurol. 15:1384480. doi: 10.3389/fneur.2024.1384480
Received: 09 February 2024; Accepted: 28 May 2024;
Published: 10 June 2024.
Edited by:
Krista DiSano, United States Department of Veterans Affairs, United StatesReviewed by:
Carla Mariana Caruso, National Scientific and Technical Research Council (CONICET), ArgentinaThulani Hansika Palpagama, The University of Auckland, New Zealand
Copyright © 2024 Risby-Jones, Lee, Woodruff and Fung. This is an open-access article distributed under the terms of the Creative Commons Attribution License (CC BY). The use, distribution or reproduction in other forums is permitted, provided the original author(s) and the copyright owner(s) are credited and that the original publication in this journal is cited, in accordance with accepted academic practice. No use, distribution or reproduction is permitted which does not comply with these terms.
*Correspondence: Jenny N. Fung, ai5mdW5nMUB1cS5lZHUuYXU=