- 1Department of Rehabilitation, The First Affiliated Hospital of Nanchang University, Nanchang, China
- 2Queen Mary College, Nanchang University, Nanchang, China
- 3The First Clinical Medical College, Nanchang University, Nanchang, China
Visual field defects (VFDs) represent a prevalent complication stemming from neurological and ophthalmic conditions. A range of factors, including tumors, brain surgery, glaucoma, and other disorders, can induce varying degrees of VFDs, significantly impacting patients’ quality of life. Over recent decades, functional imaging has emerged as a pivotal field, employing imaging technology to illustrate functional changes within tissues and organs. As functional imaging continues to advance, its integration into various clinical aspects of VFDs has substantially enhanced the diagnostic, therapeutic, and management capabilities of healthcare professionals. Notably, prominent imaging techniques such as DTI, OCT, and MRI have garnered widespread adoption, yet they possess unique applications and considerations. This comprehensive review aims to meticulously examine the application and evolution of functional imaging in the context of VFDs. Our objective is to furnish neurologists and ophthalmologists with a systematic and comprehensive comprehension of this critical subject matter.
1 Introduction
Visual field defects (VFDs) denote impairments within the visual field. Umerous diseases have the potential to impact the visual field, such as retinal detachment (1), glaucoma (2, 3), retinal vein obstruction (4, 5), retinal pigmentary degeneration (6, 7), and brain tumors (8, 9) or cerebrovascular disorders (10). The majority of these defects result from the infiltration of adjacent tissue by diseases, with tumor compression being the most prevalent causative factor (11, 12). Using glaucoma as an example, it constituted a significant portion of global blindness in 2010, affecting 2.1 million individuals, which accounted for 6.5% of the 34 million blind people worldwide. Additionally, out of the 191 million individuals with visual impairments globally, 4.2 million (2.2%) experienced visual impairment due to glaucoma. Projections indicate that the prevalence of glaucoma is anticipated to rise to 112 million cases by the year 2040. It’s noteworthy that alongside the ongoing pandemic, the novel coronavirus has also been explored for potential associations with VFDs (13). VFDs are increasingly emerging as a pressing concern (14).
The traditional method for diagnosing VFDs primarily depends on visual field measurement techniques. These techniques encompass two major technologies, dynamic and static, which are sometimes used in combination. However, it’s important to recognize that visual field measurement has its constraints, as it can only identify the underlying pathological factors responsible for vision impairment. Visual measurement methods are typically employed for initial assessments and preliminary evaluations (14, 15).
However, within the realm of contemporary science, a multitude of researchers are diligently exploring inventive avenues to enhance the diagnosis, treatment, monitoring, and prognosis of VFDs. Among these initiatives, the incorporation of cutting-edge technology, notably functional imaging, has risen to the forefront as a prominent focal point (16, 17).
The progress in medical imaging has introduced a multitude of innovative techniques that have gained broad acceptance and utility in clinical settings. These encompass technologies like functional magnetic resonance imaging (fMRI), single photon emission computed tomography (SPECT), positron emission computed tomography (PET), among others. These state-of-the-art methodologies play a pivotal role in visualizing anatomical structures, evaluating the functionality and metabolic processes of human tissues and organs, and unveiling molecular markers (18, 19). Functional imaging, a significant branch of medical imaging, is closely associated with neuroimaging and places a greater emphasis on the exploration of brain function mechanisms (20). As an illustration, functional brain imaging, utilizing techniques like positron emission tomography and magnetic resonance imaging, creates maps that highlight regional changes in brain activity. This approach offers a distinctive metabolic perspective on the inherent functioning of brain systems (21). Given the close connection between the causes of VFDs and the brain, functional imaging plays a significant role in this field (17, 22). Hence, we conduct a comprehensive review of the role of functional imaging throughout the entire clinical course of VFDs, along with the latest breakthroughs, to offer researchers a clear and comprehensive perspective.
2 Materials and methods
2.1 Textual analysis
A full-text search on PubMed was conducted using “visual field defect” and “functional imaging” as keywords. Based on the article types, clinical trials and randomized controlled trials were chosen for examination, resulting in a total of 74 search results. Out of these, 26 studies were identified as having strong associations with VFDs and functional imaging. The screening criteria encompassed various factors, including but not limited to:
1. The utilization of functional imaging technology in the examination and diagnosis of VFDs.
2. The application of functional imaging technology in supporting the treatment of VFDs.
3. Using functional imaging technology to predict the future prognosis of VFDs.
Upon selecting these relevant studies, it was observed that while numerous papers discussed the connection between VFDs and functional imaging, there were no review papers specifically addressing the application of functional imaging in the context of VFDs. Therefore, this review, building upon the outlined criteria and with an eye on current research trends, conducts a detailed analysis of the 26 research articles.
2.2 Bibliometric analysis
Our data are from the Web of Science Core collection (23), which is the foremost database integration platform for accessing global academic information. Our indexing strategy is outlined as follows: TS = (“Visual field defect “or” Visual field defects “) AND (“functional imaging “or” MRI “or” fMRI “or” OCT “or” DWI “or” DSI “or” DTI “or” OCTA “or” SD-OCT “or” iMRI “or” DTIT “or” Diffusion tensor“). We included articles and reviews while excluding other document types such as conference papers and books. Additionally, for the sake of convenience and ensuring the reliability of subsequent statistical analyses, we filtered out non-English literature. We downloaded the data and imported it into bibliometrix (24) for bibliometric analysis, focusing on the hot keywords in this field.
3 Results
Following the analysis and organization of the articles, we found that neurological disorders and ophthalmological diseases (particularly glaucoma), are the most common causes of VFD from a prevalence standpoint (25). Clinically, these two diseases are also frequently encountered by physicians. Therefore, in order to enhance the practical value of this article for clinical doctors, we employed the two primary causes of VFDs– neurological diseases and ophthalmic diseases – as our classification criteria. We meticulously categorized the diagnosis and utilization of functional imaging technology for different diseases within these two classifications.
3.1 Diseases of nervous system
The primary principle underlying VFDs induced by neurological diseases is often associated with defects or compression of the optic nerve. Neurological conditions, including epilepsy surgery, brain tumors, and post-stroke events, frequently have an impact on patients’ visual fields, resulting in VFDs (26–28). Numerous researchers have made efforts to employ functional imaging diagnostic technology to facilitate the supplementary diagnosis and treatment of VFDs stemming from nervous system diseases.
3.1.1 OCT and its associated functional imaging
Optical Coherence Tomography (OCT) is considered to be a valuable tool in assisting patients with VFDs. OCT merges the power of light and computer image processing, offering micron-level resolution and millimeter-level depth of penetration. This technology allows for cross-sectional scanning of the retina, providing detailed tomography images that aid in the assessment and diagnosis of visual field issues (29–31). In recent years, OCT has made significant advancements and found widespread application in ophthalmology-related assessments. For instance, Suh et al. explored the relationship between MRI and OCT, utilizing parameters such as macular ganglion cell and inner plexiform layer (GCIPL) and axonal retinal nerve fiber layer (RNFL). Their research aimed to evaluate the diagnostic value of both imaging techniques in cases of VFDs attributed to pituitary masses (32).
Furthermore, Rashid et al. employed OCT to detect alterations in macular ganglion cells and the inner plexiform layer (GCIPL) as well as the axonal retinal nerve fiber layer (RNFL). Their study sought to uncover the connections between these changes and the onset of VFDs. GCIPL and RNFL offer straightforward and dependable objective measurements, and are thus regarded as having predictive value for visual function (33).
Jeon et al. conducted a comparative analysis of retinal thickness measured using OCT and mass biometrics measured through MRI in patients experiencing temporal VFDs due to mass compression. Their findings indicated that, in cases of temporal VFDs caused by chiasmal compressive mass, MRI outperformed OCT in predicting postoperative VFDs recovery (34). Yoneoka et al. performed nasal endoscopic surgery on patients with visual symptoms attributed to pituitary tumors. During perioperative diagnostic MRI and ophthalmological assessments, they examined RNFL and ganglion cell complex thickness (GCC) using OCT as potential predictors of visual field prognosis (35).
Optical Coherence Tomography Angiography (OCTA) is a non-invasive blood flow detection technology developed as an extension of OCT. This advanced technique acquires clear fundus images by calculating variations in the movement of red blood cells within retinal blood vessels using computer algorithms. OCTA enables the precise visualization of lesions at different levels and locations within the eye, offering a detailed view of retinal vascular structures (36, 37). Agarwal et al. employed OCTA to assess morphological alterations in the posterior retinal choroidal vessels and compared these findings with results from clinical examinations and other imaging techniques. Their study revealed that OCTA can effectively depict changes in the microvascular structure of retinal vessels and serves as a sensitive tool for detecting HIV retinopathy (38).
3.1.2 MRI and its associated functional imaging
Functional imaging has played a significant role in supporting the treatment of VFDs and certain associated surgical procedures. One such technique, MRI, utilizes magnetic fields and radio waves to generate a cross-sectional image of a patient’s body (39).
Beam imaging of the operating room (OR) has demonstrated its effectiveness in preventing VFDs when conducted within interventional MRI (iMRI) setups. Vakharia et al. observed that the implementation of OR tractography with overlay, conducted outside of an iMRI suite, can be effectively employed during approaches to the temporal horn of the lateral ventricle. However, it is worth noting that this approach carries a 5% risk of VFDs (40). David et al. conducted a study in which they conducted preoperative and postoperative MRI scans, including T1-MPRAGE and diffusion tensor imaging, along with motor field measurements in patients with temporal lobe epilepsy, following Goldmann criteria. Their goal was to elucidate the tissue-specific aspects of VFDs severity, employing analyses at both the voxel and network levels. Additionally, they explored the imaging correlations before and after amygdalotomy (41). Saeki comprehensively analyzed the distinct clinical presentations observed in patients with Rathke’s cleft cyst (RCC) using MRI models. Among these manifestations, VFDs emerged as one of the most significant. This exploration of the growth mechanism and treatment options for these cysts is pivotal for effectively monitoring and managing patients with RCC (42). Cho et al. put forth a hypothesis supporting the idea that the central 15° of the visual field corresponds to the posterior 25% of the visual cortex. They arrived at this conclusion by studying patients with reliable static perimetry that revealed homonymous hemianopias and employing high-resolution brain imaging. Their aim was to enhance the accuracy of lesion range assessment through MRI (43).
fMRI represents an innovative technology built upon the foundation of MRI. It is a neuroimaging technique that leverages magnetic resonance imaging to assess hemodynamic changes triggered by neuronal activity, primarily within the brain or spinal cord (44, 45). Diffusion Tensor Imaging (DTI), a novel approach for characterizing brain structure, is a specialized variant of MRI (46, 47). The progress in DTI and fMRI over the recent decades has significantly improved our comprehension of brain structure and function in patients with central nervous system diseases (48, 49).
In a study conducted by Cho et al., they attempted to establish a correlation between VFDs in patients with ischemic stroke and the location of lesions identified in MRI scans. However, their attempts to utilize MRI for assessing the progression of VFDs in patients yielded unsatisfactory results. The authors proposed that the limited effectiveness of their approach may be attributed to the subtle radiological characteristics of these lesions, making accurate radiological assessment either unattainable or exceeding the scope of the visual field testing protocol (50). Ying et al. conducted a study in which they administered structural imaging and resting-state fMRI scans both before and after surgery in patients with suprasellar tumors that presented with VFDs. Their objective was to investigate alterations in both the structure and function of the visual cortex at various time points before and after the decompression of the optic nerve (51).
Souza et al. conducted preoperative and postoperative DTI scans in epilepsy patients to assess changes in their visual field (52). James et al. employed DTI to track Meyer’s loop (ML) with the aim of preventing postoperative VFDs following anterior temporal lobectomy (ATL) for drug-resistant temporal lobe epilepsy (TLE) (53). Cui et al. assisted in anterior temporal lobectomy using DTI, optical radiation mapping, micro neuronavigation, and intraoperative magnetic resonance imaging to minimize the risk of VFDs (54). Souza et al., by combining DTI and Humphrey perimetry in patients, established correlations between post-TLE surgery Visual Radiation (OR) microstructure and VFDs, proposing that postoperative OR tractography following DTI could aid in VFDs evaluation (55). Yin et al. evaluated the incidence of VFDs by detecting radiation injury to the optic nerve through DTI in patients who underwent laser interstitial thermal therapy-amygdalohippocampectomy (LITT-AHE) for medically refractory mesial temporal lobe epilepsy (MTLE) (56). Quigg et al. utilized MRI to investigate the impact of volume changes on the Visual Field Defect Ratio (VFDR) and to comprehend the relationship between VFDR, epilepsy remission, and driving status 3 years after Stereotactic Radiosurgery (57). Tong et al. used optic nerve radiation-DTI during neuronavigational-guided surgery for occipital arteriovenous malformations, aiming to reduce the severity of postoperative VFDs and assess factors contributing to visual field preservation (58). Faust et al. conducted fiber tracking examinations using DTI on patients with tumors in the temporal lobe axis prior to surgery. After merging the DTI data with the corresponding magnetized fast gradient echo (MP-RAGE) images, they achieved tumor reconstruction and visualization of neural fibers. Their findings indicated that this approach is beneficial in reducing the likelihood of VFDs (59). Yogarajah et al. (60) employed diffusion tensor beam imaging to segment patients’ white matter and evaluate the risk of VFDs on an individual basis following anterior temporal lobectomy. Voets et al. (61) utilized DTI to assess the penetration of a laser catheter into the optical radiation and further complemented the evaluation of optic nerve injury. Lilja et al. (62) delved into the application of DTI as a method for the objective assessment of anterior visual pathway damage resulting from pituitary adenoma. They posited that DTI could offer objective data, identify early indicators of damage, and function as an additional diagnostic tool to help determine surgical indications for pituitary adenoma cases. Winston et al. (63) suggested that DTI can be harnessed to map the visual radiation and categorize the risk in patients before anterior temporal lobotomy (ATLR). This approach integrated bundle display into interventional MRI to facilitate guided surgery (63).
Diffusion Spectrum Imaging (DSI) is a recently developed MRI technique capable of mapping intricate fibrous structures in tissues with high angular resolution by imaging the spectroscopy of tissue water diffusion. This advanced method is particularly valuable for detecting visual pathways (64). Liang et al. examined the clinical utility of DSI for the quantitative assessment of visual pathway irregularities, with the aim of predicting the extent of VFDs in patients with pituitary adenoma. Their findings led to the conclusion that DSI can offer quantitative data to identify visual abnormalities and may serve as a potential diagnostic tool for assessing the degree of VFDs in pituitary adenoma cases (65).
Kim et al. uncovered that alterations in early resting-state functional connectivity (RSFC), as assessed by fMRI, could serve as a predictive factor for the recovery of VFDs in patients with acute stroke (66). Rutland et al. (67) harnessed 7-T diffusion-weighted MRI (DWI) for probability-based imaging of optic tracts and radiation to investigate microstructural damage caused by pituitary macroadenomas. The distinct dispersion characteristics of ultrahigh field 7-T DWI allowed for the characterization of optic tract injuries in patients experiencing cross-compression prior to surgery, offering valuable insights into the prognosis of vision recovery. In another study, van Lanen et al. (68) developed a quantitative scoring method to evaluate VFDs following anterior temporal lobectomy using static visual field tests. They evaluated the feasibility of this approach for clinical applications.
Furthermore, numerous other methods have gained widespread use in this field. Pruckner et al. adopted a multimodal approach to assess visual outcomes following epilepsy surgery. They presented clinical and neuroimaging evidence that suggested anterior temporal lobectomy (ATL) carried a greater risk and led to more severe postoperative VFDs compared to temporal selective amygdalohippocampectomy (tsSAHE) (69). Winston et al. reported that incorporating optic nerve radiography during anterior temporal lobectomy (ATLR) for refractory temporal lobe epilepsy (TLE) had the potential to reduce the severity of postoperative VFDs without impacting seizure outcomes or hippocampal resection (70).
On the whole, MRI has relatively low spatial resolution, especially when assessing microscopic structural changes in the visual pathway, compared to other imaging modalities like OCT. In one study, researchers found it difficult to assess the extent of brain lesions with conventional MRI, with 11.7% of cerebral palsy patients having normal conventional MRI findings (71). In contrast, OCT can provide higher spatial resolution, allowing detection and assessment of microscopic changes in visual pathway structures like thinning of the ganglion cell layer (GCL) and inner plexiform layer (IPL), and demonstrate visual field defects caused by brain lesions (72). However, it should be noted that MRI can provide valuable information about the extent of white matter tract injury, especially for assessing white matter damage (73). Moreover, compared to OCT, a unique advantage of MRI is its capability for comprehensive, three-dimensional analysis of brain structure and neural pathways.
With advances in MRI techniques such as high-field MRI, fMRI, and DTI, our understanding of the pathophysiology of VFDs have been further enhanced. High-field MRI utilizing stronger magnetic fields can provide higher signal-to-noise ratio and better resolution, which helps better image deep brain structures and visualize smaller structures more clearly. This allows more accurate observation of the anatomical and morphologic characteristics of various structures along the visual pathway, including the optic chiasm and lateral geniculate nuclei, by examining changes in these anatomical structures to gain deeper insight into the pathophysiological mechanisms of visual field defects (74). fMRI can monitor blood flow changes in active brain areas to understand functional maps and networks in the brain, which is critical for studying the function of visual processing regions and brain plasticity in VFDs patients (75). DTI is another specialized MRI technique that measures the diffusion properties of water molecules in brain tissue. Since water molecules diffuse more easily along the direction of neural fiber bundles, DTI can be used to map the white matter fiber tracts in the brain, including the visual radiations (76). DTI can also more precisely quantify and assess the integrity of vision-related nerve fibers using various diffusion coefficients like FA, l1, l23 and MD to predict whether visual field defects will occur (77).
3.2 Ophthalmic diseases
Eye diseases are indeed significant contributors to VFDs. The issue of excessive eye strain in modern society is becoming increasingly severe, leading to a rising incidence of various eye conditions. Among the most common are glaucoma, retinal damage, and various other visual problems that can result in VFDs. Regular eye check-ups and eye health practices are crucial for preventing and managing these conditions (78, 79). Numerous studies have made efforts to employ functional imaging techniques for investigating complications associated with VFDs in eye diseases, mainly OCT and its related functional imaging techniques.OCT is also a widely utilized tool for the detection of various eye diseases. For instance, Ohguro et al. used measurements of RNFL and VFDs, determined through OCT, to differentiate between cases of naso-optic hypoplasia (NOH) with NOH-like temporal VFDs, which is associated with NOH, and cases of glaucoma (80). Kallab et al. (81) conducted measurements to investigate the focal structure–function correlation of capillary density (CD). They utilized OCT to measure the structural thickness of the retinal nerve fiber layer around the optic disc (RNFL-T), in addition to employing OCT-angiography (OCT-A) or a combination of both techniques. Their aim was to compare VFDs in patients at various stages of glaucoma, ranging from early to late (81).
Spectral Domain Optical Coherence Tomography (SD-OCT) represents the second generation of OCT technology. When compared to the first-generation Time-Domain OCT technology, it boasts clear advantages in terms of imaging speed, signal-to-noise ratio, and sensitivity. As a result, SD-OCT plays a pivotal role in the fields of ophthalmic imaging and functional imaging (82, 83). As a result of its advantages, SD-OCT has seen increased usage in recent years. Miki et al. utilized Spectralis SD-OCT to detect the rate of RNFL loss in eyes with VFDs. Their findings suggest that measuring SD-OCT RNFL loss rates may serve as a valuable tool for identifying patients at a high risk of experiencing visual field loss (84). Wandji et al. employed SD-OCT to identify the presence of buried optic disc drusen (BODD). Additionally, they utilized Garway-Heath imaging to identify the associated VFDs. Their study focused on comparing the relationship between the presence of buried optic disc drusen and the resulting VFDs (85).
Various imaging techniques have been explored for assessing visual function and disorders. For instance, Kwon investigated the correlation between parameters related to the Foveal Avascular Zone (FAZ) and central vision function. This was achieved by measuring the FAZ area, circumference, roundness, and parafoveal vessel density using OCTA images. Such studies help to advance our understanding of the relationship between structural features of the eye and visual function (86). Carvalho et al. employed fMRI-based neural modeling to investigate hypothesized alterations in population receptive fields (pRFs) linked to VFDs in glaucoma. Their study revealed that local pRFs in the visual areas were associated with masking the VFDs, thereby making it challenging for patients to detect changes in their VFDs. This research sheds light on the neural mechanisms involved in VFDs perception and the impact of pRFs on visual awareness (87). In summary, the OCT series of functional imaging techniques have predominantly found applications in the realm of eye diseases.
The studies mentioned above have been compiled and summarized in Table 1 for reference and easy access. These investigations have contributed to our understanding of the various aspects of VFDs and their associations with different conditions, offering valuable insights for clinical diagnosis and management.Early diagnosis and accurate differentiation of ophthalmic diseases is the most important step in preventing and halting their onset and progression. Different imaging modalities have their own merits in early diagnosis of various diseases and their differentiation. OCT has demonstrated good performance in early glaucoma detection. By conducting a global region assessment using Cirrus OCT, a moderate consistency (κ = 0.420) was found between Cirrus OCT retinal nerve fiber layer thickness (RNFLT) measurements and neuroretinal rim area (RA) classifications. Additionally, by comparing results from Cirrus OCT between normal and early open-angle glaucoma groups, Cirrus OCT parameters were found to have good diagnostic ability for glaucoma with high sensitivity and specificity (88). MRI can detect early brain changes in glaucoma, showing microstructural abnormalities in the visual pathway and changes and atrophy in the central visual system, providing important information for early diagnosis and helping diagnose and monitor the progression of glaucomatous damage (89). For diabetic retinopathy, OCT can detect characteristic changes associated with early diabetic retinopathy such as macular edema, which is crucial for discovering diabetic retinopathy early on (90). MRI can serve as a potential screening method and possible quantitative physiological biomarker for early signs of diabetic retinopathy in type 2 diabetes patients. Studies show MRI can provide quantitative information on the early status of diabetic retinopathy by measuring retinal oxygenation levels (91). OCT can also aid in diagnosing early age-related macular degeneration (AMD) lesions by observing thickness and morphological changes in the macular area. Additionally, OCT image parameters are associated with the degree and progression of AMD lesions (92). MRI has shown some limitations in detecting early AMD lesions. Specifically, early AMD did not show significant associations with brain MRI changes like white matter lesions (WML), subcortical white matter volume (SW) and ventricular volume (VE), so MRI is less utilized for early detection of AMD (93).
3.3 Bibliometrics analysis
We retrieved studies published between January 1, 1999, and December 31, 2023, from Web of science core collection and employed a combined quantitative and qualitative approach to data analysis. Furthermore, we conducted a comprehensive search of the entire literature. The data was then structured to identify the keywords used in the literature based on their frequency and timeline. This helps identify current research hotspots and aids in predicting future trends in the field of functional imaging. However, the conclusions drawn from bibliometric analysis depend on the quantity and quality of the considered literature. Also, as research outcomes in some fields might manifest through alternative mediums like patents, bibliometric analysis may not comprehensively represent the entire discipline. Therefore, as a macroscopic analytical method, while bibliometric analysis has its limitations, it also offers unique insights into the development of functional imaging research.
The results of the bibliometric analysis (94–96), as depicted in Figure 1, indicate that OCT is the most widely used and popular functional imaging method, accounting for the highest percentage among all keyword searches, with a 5% share. Following OCT, the MRI series of technologies, with “MRI” as a keyword accounting for 3%, and “fMRI” as a keyword accounting for 1%, represent the second most commonly used group of functional imaging methods.
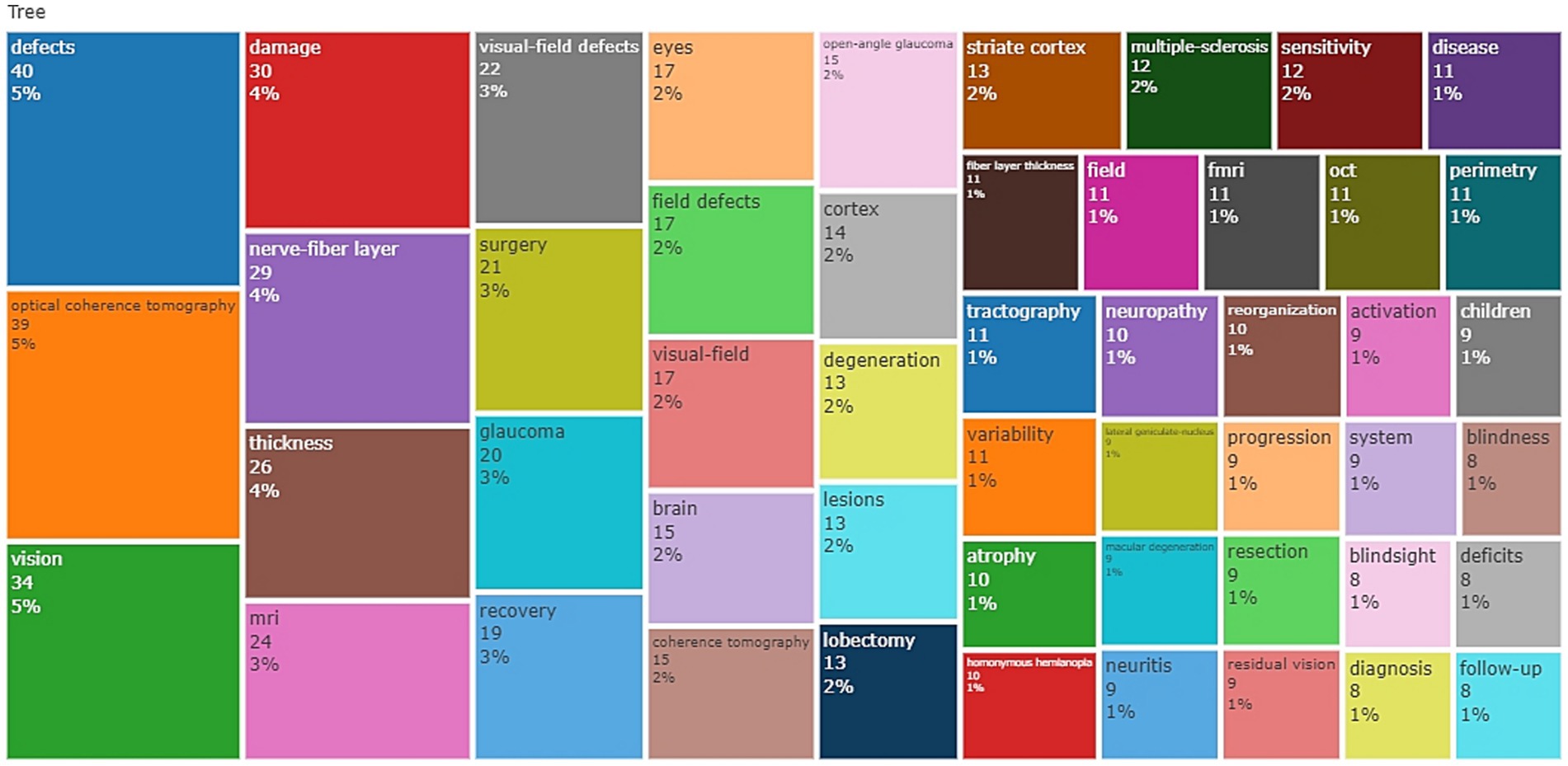
Figure 1. Treemap of the keywords, this figure presents the word frequency analysis of keywords in related fields.
Moreover, the analysis of keywords not only reflects the application of different functional imaging techniques but also provides insights into the conditions causing VFDs and the utilization of imaging technologies. In alignment with our prior detailed literature analysis, the appearance of the keyword “Surgery” with a frequency of 3% suggests that functional imaging techniques are more frequently employed in surgical contexts. The frequency of the keyword “Glaucoma” also reached 3%, indicating a higher occurrence of VFDs caused by glaucoma and a greater need for the application of functional imaging technology.
Furthermore, a significant number of keywords related to the brain and the nervous system appeared, reinforcing our earlier classification of diseases into neurological diseases and eye diseases. This suggests that functional imaging techniques are frequently utilized in both of these disease categories.
Figure 2 reveals that in recent years, there has been significant interest in conditions such as dysthyroid optic neuropathy, idiopathic intracranial hypertension, and nerve sheath meningioma. These conditions have captured considerable attention and research focus within the field of functional imaging.
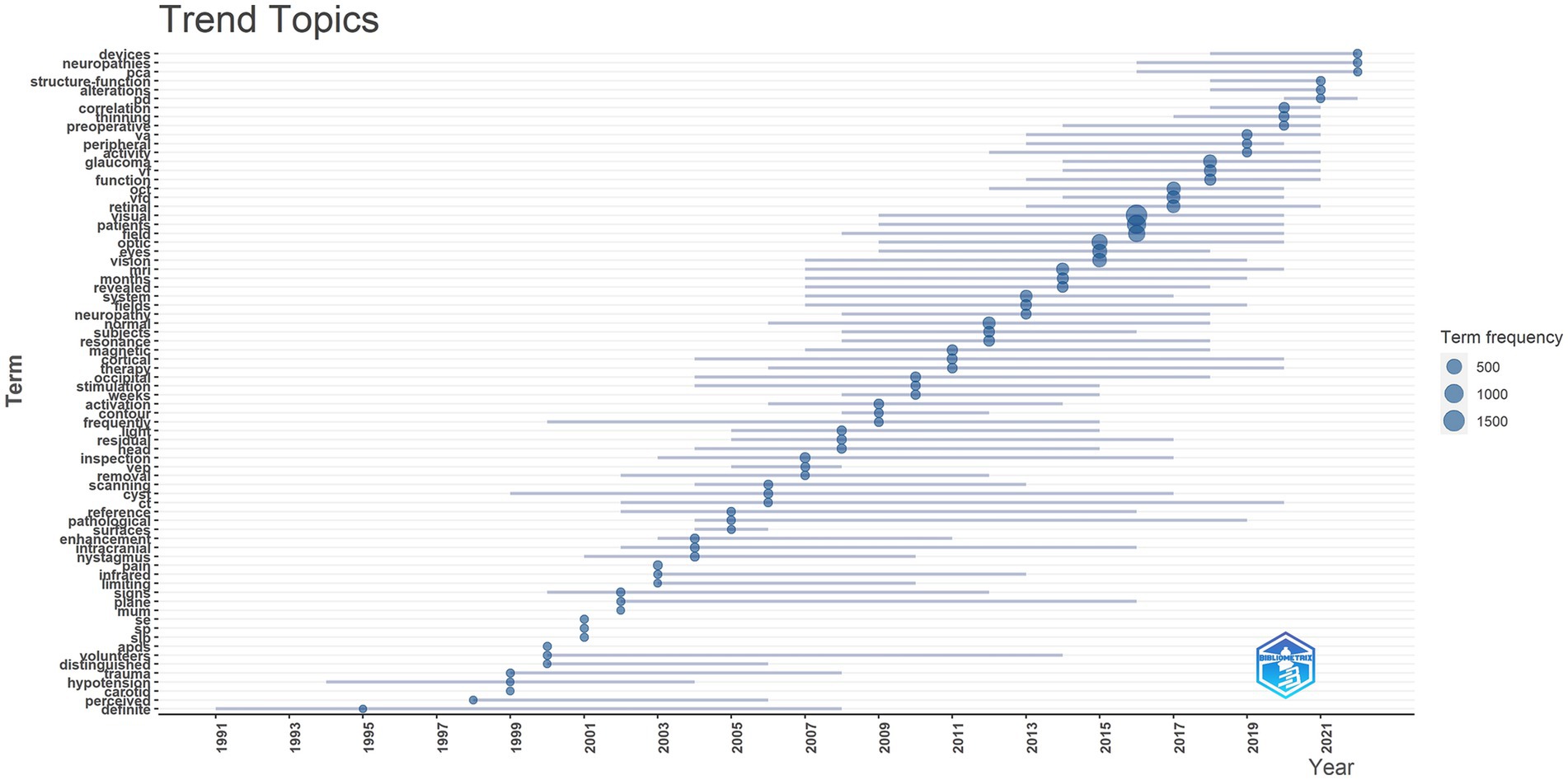
Figure 2. Trend topics, this figure shows the hot spot changes of keywords in related fields over time.
Furthermore, the data highlights that OCTA is among the most advanced and cutting-edge technologies currently in use. This indicates that OCTA is at the forefront of functional imagi ng and has garnered substantial interest and development in recent years.
4 Discussion
With the continuous advancement of science and technology, the realm of functional imaging technology is constantly evolving and expanding. These innovative techniques are finding increasing utility in the realm of VFDs disease. Following a comprehensive review of existing clinical studies, we conducted a macro-economic analysis of this field, revealing that OCT stands as the most commonly employed tool, closely followed by MRI. Among the various MRI-related tools, fMRI is widely embraced, while OCTA emerges as the cutting-edge and state-of-the-art technology within the OCT domain.
OCT is mainly used for disease assessment, and DTI and MRI series are often used to assist surgical operations and evaluate the progression of patients’ conditions. With the continuous advancement of MRI technology, especially the introduction of high-field MRI, the understanding of the pathophysiology of visual field defects has been enhanced. High-field MRI refers to the use of magnetic resonance imaging devices with higher magnetic field strength (97, 98). The advantage of this technology is to improve the signal-to-noise ratio and contrast of images, allowing doctors to distinguish subtle structural changes more clearly. In assessing the microstructure of the visual pathway, high-field MRI can more accurately reveal details of neurons, fiber bundles, and other microstructures. In addition, high-field MRI can provide richer functional information, such as quantitative measurements of brain activity and metabolism, which are crucial for understanding the pathogenesis and pathophysiological changes of visual field defects (98–100). As one of the high-field MRI technologies, DTI focuses on the directional diffusion of water molecules in tissues, providing detailed quantitative information about neural fiber bundles, revealing the degree and location of damage to neural fiber bundles (101, 102). Application of DTI during epileptic surgery or other vision-related surgeries helps reduce the risk of postoperative visual field defects (103). Compared to MRI, DTI may have higher sensitivity and specificity in assessing abnormalities in visual function. It has been widely used to study vision-related diseases such as retinitis pigmentosa, glaucoma, etc. Results demonstrate that DTI can detect structural and functional abnormalities in the optical pathway, further assisting diagnosis and assessment of disease progression (104). MRI, with relatively high spatial resolution, can provide detailed information and high-resolution images of tissue structure and organ morphology from the perspective of overall anatomy (105, 106). Together, they help surgeons more accurately locate and protect important visual pathway structures during surgical interventions, reduce surgical risks, and provide important evidence for personalized treatment plans.
In recent years, OCT-related technologies, especially the second-generation SD-OCT, have improved scanning speed, enhanced imaging resolution and depth, and integrated more closely with artificial intelligence, achieving significant progress in addressing VFDs caused by ophthalmic diseases (107–109). Another important technology, OCTA, has certain advantages in detecting early changes in VFDs. Traditional OCT mainly generates images by measuring the reflectivity of light, primarily reflecting structural information of tissues. In contrast, OCTA generates images by detecting blood flow signals, providing more detailed information about vascular structures (110). This allows OCTA to detect early changes more sensitively in VFDs, such as changes in local blood flow, microcirculation disorders, and changes in vascular density, which may be difficult to observe in traditional OCT images. Additionally, OCTA can provide information on the three-dimensional vascular structure and differentiate vascular distribution at different depths through scans, aiding in more accurate localization of VFD lesions (36, 111–113). However, OCTA also has some limitations and challenges. For example, it is highly dependent on the flow rate, and signal loss may occur in low perfusion states or low blood flow velocities. Additionally, OCTA has limitations in resolution and depth, restricting its application in certain situations (36, 114).
Overall, these technologies almost cover all aspects of the patient treatment process, playing important clinical roles in diagnosis, treatment, and prognosis. They significantly strengthen patient care, making monitoring and assessing unforeseen risks possible. Regarding specific diseases, literature analysis suggests that functional imaging techniques may see greater adoption in surgical contexts, with a growing demand for their application in the domain of glaucoma. Recent attention has also been directed towards disorders such as dysthyroid optic neuropathy, idiopathic intracranial hypertension, and nerve sheath meningiomas. These conditions have garnered substantial interest and research focus within the functional imaging field.
5 Conclusion
In conclusion, functional imaging techniques such as DTI, OCT, and MRI have become invaluable tools in the diagnosis, treatment, and management of visual field defects. Each modality provides unique insights, yet also possesses its own limitations and considerations. As the field continues to progress, the integration of functional imaging into clinical practice will further enhance our understanding and care of patients with VFDs. Nevertheless, additional research is warranted to optimize and standardize protocols across institutions. Overall, this review highlights the vital role of functional imaging in advancing the care of visual field defects through refined characterization of structure–function relationships.
Author contributions
WC: Writing – original draft. JL: Writing – original draft. TJ: Writing – original draft. ML: Writing – review & editing.
Funding
The author(s) declare financial support was received for the research, authorship, and/or publication of this article. This work was supported by the Science and Technology Department of Jiangxi Province (No. 20202BABL216063).
Acknowledgments
We thank the Science and Technology Department of Jiangxi Province for the fund support.
Conflict of interest
This study was conducted in the absence of any commercial or financial relationship that could be interpreted as a potential conflict of interest.
Publisher’s note
All claims expressed in this article are solely those of the authors and do not necessarily represent those of their affiliated organizations, or those of the publisher, the editors and the reviewers. Any product that may be evaluated in this article, or claim that may be made by its manufacturer, is not guaranteed or endorsed by the publisher.
References
1. Steel, D. Retinal detachment. BMJ Clin Evid. (2014) 2014:710 https://www.ncbi.nlm.nih.gov/pmc/articles/PMC3940167/
2. Jonas, JB, Aung, T, Bourne, RR, Bron, AM, Ritch, R, and Panda-Jonas, S. Glaucoma. Lancet. (2017) 390:2183–93. doi: 10.1016/S0140-6736(17)31469-1
3. Michau, TM. Equine Glaucoma. Vet Clin North Am Equine Pract. (2017) 33:519–40. doi: 10.1016/j.cveq.2017.07.005
4. Hayreh, SS. Ocular vascular occlusive disorders: natural history of visual outcome. Prog Retin Eye Res. (2014) 41:1–25. doi: 10.1016/j.preteyeres.2014.04.001
5. Patel, A, Nguyen, C, and Lu, S. Central retinal vein occlusion: a review of current evidence-based treatment options. Middle East Afr J Ophthalmol. (2016) 23:44–8. doi: 10.4103/0974-9233.173132
6. Hartong, DT, Berson, EL, and Dryja, TP. Retinitis pigmentosa. Lancet. (2006) 368:1795–809. doi: 10.1016/S0140-6736(06)69740-7
7. Abd El-Aziz, MM, El-Ashry, MF, Chan, WM, Chong, KL, Barragan, I, Antinolo, G, et al. A novel genetic study of Chinese families with autosomal recessive retinitis pigmentosa. Ann Hum Genet. (2007) 71:281–94. doi: 10.1111/j.1469-1809.2006.00333.x
8. Moller, MW, Andersen, MS, Glintborg, D, Pedersen, CB, Halle, B, Kristensen, BW, et al. Pituitary adenoma. Ugeskr Laeger. (2019) 181:V05180331
9. Semple, PL, Webb, MK, de Villiers, JC, and Laws, ER Jr. Pituitary apoplexy. Neurosurgery. (2005) 56:65–73; discussion 72-3. doi: 10.1227/01.NEU.0000144840.55247.38
10. Pula, JH, and Yuen, CA. Eyes and stroke: the visual aspects of cerebrovascular disease. Stroke Vasc Neurol. (2017) 2:210–20. doi: 10.1136/svn-2017-000079
11. Soussain, C, Malaise, D, and Cassoux, N. Primary vitreoretinal lymphoma: a diagnostic and management challenge. Blood. (2021) 138:1519–34. doi: 10.1182/blood.2020008235
12. Vroonen, L, Daly, A, and Beckers, A. Management of prolactinoma. Rev Med Suisse. (2013) 9:1522–6.
13. Gold, DM, and Galetta, SL. Neuro-ophthalmologic complications of coronavirus disease 2019 (COVID-19). Neurosci Lett. (2021) 742:135531. doi: 10.1016/j.neulet.2020.135531
14. Wall, M. Perimetry and visual field defects. Handb Clin Neurol. (2021) 178:51–77. doi: 10.1016/B978-0-12-821377-3.00003-9
15. Hu, R, Racette, L, Chen, KS, and Johnson, CA. Functional assessment of glaucoma: uncovering progression. Surv Ophthalmol. (2020) 65:639–61. doi: 10.1016/j.survophthal.2020.04.004
16. Alwashmi, K, Meyer, G, and Rowe, FJ. Audio-visual stimulation for visual compensatory functions in stroke survivors with visual field defect: a systematic review. Neurol Sci. (2022) 43:2299–321. doi: 10.1007/s10072-022-05926-y
17. Urbanski, M, Coubard, OA, and Bourlon, C. Visualizing the blind brain: brain imaging of visual field defects from early recovery to rehabilitation techniques. Front Integr Neurosci. (2014) 8:74. doi: 10.3389/fnint.2014.00074
18. Christiansen, L, and Siebner, HR. Tools to explore neuroplasticity in humans: combining interventional neurophysiology with functional and structural magnetic resonance imaging and spectroscopy. Handb Clin Neurol. (2022) Springer 184:105–19. doi: 10.1016/B978-0-12-819410-2.00032-1
19. Roalf, DR, and Gur, RC. Functional brain imaging in neuropsychology over the past 25 years. Neuropsychology. (2017) Springer 31:954–71. doi: 10.1037/neu0000426
20. Shallice, T. Functional imaging and neuropsychology findings: how can they be linked? NeuroImage. (2003) 20:S146–54. doi: 10.1016/j.neuroimage.2003.09.023
21. Raichle, ME, and Mintun, MA. Brain work and brain imaging. Annu Rev Neurosci. (2006) 29:449–76. doi: 10.1146/annurev.neuro.29.051605.112819
22. Winston, GP. Epilepsy surgery, vision, and driving: what has surgery taught us and could modern imaging reduce the risk of visual deficits? Epilepsia. (2013) 54:1877–88. doi: 10.1111/epi.12372
23. van Eck, NJ, and Waltman, L. Software survey: VOSviewer, a computer program for bibliometric mapping. Scientometrics. (2010) 84:523–38. doi: 10.1007/S11192-009-0146-3
24. Campra, M, Riva, P, Oricchio, G, and Brescia, V. Bibliometrix analysis of medical tourism. Health Serv Manag Res. (2022) 35:172–88. doi: 10.1177/09514848211011738
25. Agarwal, A, and Kedar, S. Prognosis and treatment of visual field defects. Semin Neurol. (2015) 35:549–56. doi: 10.1055/s-0035-1563573
26. Armstrong, RA. Visual problems associated with traumatic brain injury. Clin Exp Optom. (2018) 101:716–26. doi: 10.1111/cxo.12670
27. Vakharia, VN, Diehl, B, and Tisdall, M. Visual field defects in temporal lobe epilepsy surgery. Curr Opin Neurol. (2021) 34:188–96. doi: 10.1097/WCO.0000000000000905
28. van Baarsen, KM, Porro, GL, and Wittebol-Post, D. Epilepsy surgery provides new insights in retinotopic organization of optic radiations. A systematic review. Curr Opin Ophthalmol. (2009) 20:490–4. doi: 10.1097/ICU.0b013e3283313c02
29. Asam, JS, Polzer, M, Tafreshi, A, Hirnschall, N, and Findl, O. Anterior Segment OCT In: JF Bille, editor. High resolution imaging in microscopy and ophthalmology: New Frontiers in biomedical optics. Cham (CH): Springer (2019)
30. Aumann, S, Donner, S, Fischer, J, and Muller, F. Optical coherence tomography (OCT): principle and technical realization In: JF Bille, editor. High resolution imaging in microscopy and ophthalmology: New Frontiers in biomedical optics. Cham (CH): Springer (2019)
31. Rozot, P, Baikoff, G, Lutun, E, and Wei, J. Evaluation of capsular block syndrome with an anterior segment OCT. J Fr Ophtalmol. (2005) 28:309–11. doi: 10.1016/S0181-5512(05)81059-9
32. Suh, H, Choi, H, and Jeon, H. The radiologic characteristics and retinal thickness are correlated with visual field defect in patients with a pituitary mass. J Neuroophthalmol. (2021) 41:e541–7. doi: 10.1097/WNO.0000000000001011
33. Rashid, AS, Rashid, D, Yang, G, Link, H, Gauffin, H, and Huang-Link, Y. Homonymous visual field defect and retinal thinning after occipital stroke. Brain Behav. (2021) 11:e2345. doi: 10.1002/brb3.2345
34. Jeon, H, Suh, HB, Kim, TY, and Choi, HY. Predictive value of OCT and MRI for postoperative visual recovery in patients with chiasmal compressive lesions. Eur J Ophthalmol. (2022) 32:2982–7. doi: 10.1177/11206721211073216
35. Yoneoka, Y, Hatase, T, Watanabe, N, Jinguji, S, Okada, M, Takagi, M, et al. Early morphological recovery of the optic chiasm is associated with excellent visual outcome in patients with compressive chiasmal syndrome caused by pituitary tumors. Neurol Res. (2015) 37:1–8. doi: 10.1179/1743132814Y.0000000407
36. de Carlo, TE, Romano, A, Waheed, NK, and Duker, JS. A review of optical coherence tomography angiography (OCTA). Int J Retina Vitreous. (2015) 1:5. doi: 10.1186/s40942-015-0005-8
37. Koutsiaris, AG, Batis, V, Liakopoulou, G, Tachmitzi, SV, Detorakis, ET, and Tsironi, EE. Optical coherence tomography angiography (OCTA) of the eye: a review on basic principles, advantages, disadvantages and device specifications. Clin Hemorheol Microcirc. (2022) 83:247–71. doi: 10.3233/CH-221634
38. Agarwal, A, Invernizzi, A, Acquistapace, A, Riva, A, Agrawal, R, Jain, S, et al. Analysis of Retinochoroidal vasculature in human immunodeficiency virus infection using spectral-domain OCT angiography. Ophthalmol Retina. (2017) 1:545–54. doi: 10.1016/j.oret.2017.03.007
40. Vakharia, VN, Vos, SB, Winston, GP, Gutman, MJ, Wykes, V, McEvoy, AW, et al. Intraoperative overlay of optic radiation tractography during anteromesial temporal resection: a prospective validation study. J Neurosurg. (2022) 136:543–52. doi: 10.3171/2020.12.JNS203437
41. David, B, Eberle, J, Delev, D, Gaubatz, J, Prillwitz, CC, Wagner, J, et al. Multi-scale image analysis and prediction of visual field defects after selective amygdalohippocampectomy. Sci Rep. (2021) 11:1444. doi: 10.1038/s41598-020-80751-x
42. Saeki, N, Sunami, K, Sugaya, Y, and Yamaura, A. MRI findings and clinical manifestations in Rathke's cleft cyst. Acta Neurochir. (1999) 141:1055–61. doi: 10.1007/s007010050482
43. Cho, J, Liao, E, and Trobe, JD. Correlation of macular sparing and homonymous paracentral scotomas with MRI lesions in posterior cerebral artery infarction. J Neuroophthalmol. (2022) 42:367–71. doi: 10.1097/WNO.0000000000001603
44. Amaro, E Jr, and Barker, GJ. Study design in fMRI: basic principles. Brain Cogn. (2006) 60:220–32. doi: 10.1016/j.bandc.2005.11.009
45. Smitha, KA, Akhil Raja, K, Arun, KM, Rajesh, PG, Thomas, B, Kapilamoorthy, TR, et al. Resting state fMRI: a review on methods in resting state connectivity analysis and resting state networks. Neuroradiol J. (2017) 30:305–17. doi: 10.1177/1971400917697342
46. Corroenne, R, Arthuis, C, Kasprian, G, Mahallati, H, Ville, Y, Millischer Bellaiche, AE, et al. Diffusion tensor imaging of fetal brain: principles, potential and limitations of promising technique. Ultrasound Obstet Gynecol. (2022) 60:470–6. doi: 10.1002/uog.24935
47. Sammartino, F, and Hodaie, M. Diffusion tensor imaging of the basal ganglia for functional neurosurgery applications. Prog Neurol Surg. (2018) 33:62–79. doi: 10.1159/000480766
48. Filippi, M, and Agosta, F. Diffusion tensor imaging and functional MRI. Handb Clin Neurol. (2016) 136:1065–87. doi: 10.1016/B978-0-444-53486-6.00056-9
49. Khalil, M, Siddiqui, K, Baig, A, and Shamim, MS. Role of diffusion tensor imaging for brain tumour resection. J Pak Med Assoc. (2022) 72:1667–9. doi: 10.47391/JPMA.22-88
50. Cho, J, Liao, E, and Trobe, JD. Accuracy of visual fields in localizing MRI lesions in posterior cerebral artery infarction. J Neuroophthalmol. (2022) 42:360–6. doi: 10.1097/WNO.0000000000001602
51. Ying, J, Yuan, T, Jin, L, Li, C, Gui, S, Wang, R, et al. Brain morphometric and functional magnetic resonance imaging study on patients with visual field defects resulting from Suprasellar tumors: preoperative and postoperative assessment. World Neurosurg. (2020) 134:e353–9. doi: 10.1016/j.wneu.2019.10.060
52. de Souza, J, Ayub, G, Nogueira, M, Zanao, T, Lopes, TM, Pimentel-Silva, LR, et al. Temporopolar amygdalohippocampectomy: seizure control and postoperative outcomes. J Neurosurg. (2020) 134:1044–53. doi: 10.3171/2020.3.JNS192624
53. James, JS, Radhakrishnan, A, Thomas, B, Madhusoodanan, M, Kesavadas, C, Abraham, M, et al. Diffusion tensor imaging tractography of Meyer's loop in planning resective surgery for drug-resistant temporal lobe epilepsy. Epilepsy Res. (2015) 110:95–104. doi: 10.1016/j.eplepsyres.2014.11.020
54. Cui, Z, Ling, Z, Pan, L, Song, H, Chen, X, Shi, W, et al. Optic radiation mapping reduces the risk of visual field deficits in anterior temporal lobe resection. Int J Clin Exp Med. (2015) 8:14283–95.
55. de Souza, J, Ayub, G, Pereira, PC, Vasconcellos, JPC, Yasuda, C, Joaquim, AF, et al. Fractional anisotropy of the optic radiations correlates with the visual field after epilepsy surgery. Neuroradiology. (2019) 61:1425–36. doi: 10.1007/s00234-019-02281-2
56. Yin, D, Thompson, JA, Drees, C, Ojemann, SG, Nagae, L, Pelak, VS, et al. Optic radiation Tractography and visual field deficits in laser interstitial thermal therapy for Amygdalohippocampectomy in patients with mesial temporal lobe epilepsy. Stereotact Funct Neurosurg. (2017) 95:107–13. doi: 10.1159/000454866
57. Quigg, M, Barbaro, NM, Ward, MM, Chang, EF, Broshek, DK, Langfitt, JT, et al. Visual field defects after radiosurgery versus temporal lobectomy for mesial temporal lobe epilepsy: findings of the ROSE trial. Seizure. (2018) 63:62–7. doi: 10.1016/j.seizure.2018.10.017
58. Tong, X, Wu, J, Lin, F, Cao, Y, Zhao, Y, Jin, Z, et al. Visual field preservation in surgery of occipital arteriovenous malformations: a prospective study. World Neurosurg. (2015) 84:1423–36. doi: 10.1016/j.wneu.2015.06.069
59. Faust, K, and Vajkoczy, P. Distinct displacements of the optic radiation based on tumor location revealed using preoperative diffusion tensor imaging. J Neurosurg. (2016) 124:1343–52. doi: 10.3171/2015.3.JNS141584
60. Yogarajah, M, Focke, NK, Bonelli, S, Cercignani, M, Acheson, J, Parker, GJ, et al. Defining Meyer's loop-temporal lobe resections, visual field deficits and diffusion tensor tractography. Brain. (2009) 132:1656–68. doi: 10.1093/brain/awp114
61. Voets, NL, Alvarez, I, Qiu, D, Leatherday, C, Willie, JT, Sotiropoulos, S, et al. Mechanisms and risk factors contributing to visual field deficits following stereotactic laser Amygdalohippocampotomy. Stereotact Funct Neurosurg. (2019) 97:255–65. doi: 10.1159/000502701
62. Lilja, Y, Gustafsson, O, Ljungberg, M, Starck, G, Lindblom, B, Skoglund, T, et al. Visual pathway impairment by pituitary adenomas: quantitative diagnostics by diffusion tensor imaging. J Neurosurg. (2017) 127:569–79. doi: 10.3171/2016.8.JNS161290
63. Winston, GP, Daga, P, Stretton, J, Modat, M, Symms, MR, McEvoy, AW, et al. Optic radiation tractography and vision in anterior temporal lobe resection. Ann Neurol. (2012) 71:334–41. doi: 10.1002/ana.22619
64. Tian, L, Yan, H, and Zhang, D. Diffusion spectrum magnetic resonance imaging. Beijing Da Xue Xue Bao. (2009) 41:716–20.
65. Liang, L, Lin, H, Lin, F, Yang, J, Zhang, H, Zeng, L, et al. Quantitative visual pathway abnormalities predict visual field defects in patients with pituitary adenomas: a diffusion spectrum imaging study. Eur Radiol. (2021) 31:8187–96. doi: 10.1007/s00330-021-07878-x
66. Kim, TH, Shin, SD, Song, KJ, Hong, KJ, Ro, YS, Song, SW, et al. Chest compression fraction between mechanical compressions on a reducible stretcher and manual compressions on a standard stretcher during transport in out-of-hospital cardiac arrests: the ambulance stretcher innovation of Asian cardiopulmonary resuscitation (ASIA-CPR) pilot trial. Prehosp Emerg Care. (2017) 21:636–44. doi: 10.1080/10903127.2017.1317892
67. Rutland, JW, Padormo, F, Yim, CK, Yao, A, Arrighi-Allisan, A, Huang, KH, et al. Quantitative assessment of secondary white matter injury in the visual pathway by pituitary adenomas: a multimodal study at 7-tesla MRI. J Neurosurg. (2019) 132:333–42. doi: 10.3171/2018.9.JNS182022
68. van Lanen, R, Hoeberigs, MC, Bauer, NJC, Haeren, RHL, Hoogland, G, Colon, A, et al. Visual field deficits after epilepsy surgery: a new quantitative scoring method. Acta Neurochir. (2018) 160:1325–36. doi: 10.1007/s00701-018-3525-9
69. Pruckner, P, Nenning, KH, Fischmeister, FPS, Yildirim, MS, Schwarz, M, Reitner, A, et al. Visual outcomes after anterior temporal lobectomy and transsylvian selective amygdalohippocampectomy: a quantitative comparison of clinical and diffusion data. Epilepsia. (2023) 64:705–17. doi: 10.1111/epi.17490
70. Winston, GP, Daga, P, White, MJ, Micallef, C, Miserocchi, A, Mancini, L, et al. Preventing visual field deficits from neurosurgery. Neurology. (2014) 83:604–11. doi: 10.1212/WNL.0000000000000685
71. Bax, M, Tydeman, C, and Flodmark, O. Clinical and MRI correlates of cerebral palsy: the European cerebral palsy study. JAMA. (2006) 296:1602–8. doi: 10.1001/jama.296.13.1602
72. Jacobson, L, Lennartsson, F, and Nilsson, M. Retinal ganglion cell topography predicts visual field function in spastic cerebral palsy. Dev Med Child Neurol. (2020) 62:1100–6. doi: 10.1111/dmcn.14545
73. Scheck, SM, Boyd, RN, and Rose, SE. New insights into the pathology of white matter tracts in cerebral palsy from diffusion magnetic resonance imaging: a systematic review. Dev Med Child Neurol. (2012) 54:684–96. doi: 10.1111/j.1469-8749.2012.04332.x
74. Rutland, JW, Schefflein, J, Arrighi-Allisan, AE, Ranti, D, Ladner, TR, Pai, A, et al. Measuring degeneration of the lateral geniculate nuclei from pituitary adenoma compression detected by 7T ultra-high field MRI: a method for predicting vision recovery following surgical decompression of the optic chiasm. J Neurosurg. (2020) 132:1747–56. doi: 10.3171/2019.2.JNS19271
75. Prabhakaran, GTT, Al-Nosairy, KOO, Tempelmann, C, Thieme, H, and Hoffmann, MBB. Mapping visual field defects with fMRI - impact of approach and experimental conditions. Front Neurosci. (2021) 15:745886. doi: 10.3389/fnins.2021.745886
76. Koenraads, Y, Porro, GL, Braun, KPJ, Groenendaal, F, de Vries, LS, and van der Aa, NE. Prediction of visual field defects in newborn infants with perinatal arterial ischemic stroke using early MRI and DTI-based tractography of the optic radiation. Eur J Paediatr Neurol. (2016) 20:309–18. doi: 10.1016/j.ejpn.2015.11.010
77. Weinstein, M, Ben-Sira, L, Moran, A, Berger, I, Marom, R, Geva, R, et al. The motor and visual networks in preterm infants: an fMRI and DTI study. Brain Res. (2016) 1642:603–11. doi: 10.1016/j.brainres.2016.04.052
78. Bertaud, S, Aragno, V, Baudouin, C, and Labbe, A. Primary open-angle glaucoma. Rev Med Interne. (2019) 40:445–52. doi: 10.1016/j.revmed.2018.12.001
79. Reddy, SV, and Husain, D. Panretinal photocoagulation: a review of complications. Semin Ophthalmol. (2018) 33:83–8. doi: 10.1080/08820538.2017.1353820
80. Ohguro, H, Ohguro, I, Tsuruta, M, Katai, M, and Tanaka, S. Clinical distinction between nasal optic disc hypoplasia (NOH) and glaucoma with NOH-like temporal visual field defects. Clin Ophthalmol. (2010) 4:547–55.
81. Kallab, M, Hommer, N, Schlatter, A, Chua, J, Tan, B, Schmidl, D, et al. Combining vascular and nerve fiber layer thickness measurements to model glaucomatous focal visual field loss. Ann N Y Acad Sci. (2022) 1511:133–41. doi: 10.1111/nyas.14732
82. Renard, JP, Fenolland, JR, and Giraud, JM. Glaucoma progression analysis by spectral-domain optical coherence tomography (SD-OCT). J Fr Ophtalmol. (2019) 42:499–516. doi: 10.1016/j.jfo.2019.03.001
83. Yaqoob, Z, Wu, J, and Yang, C. Spectral domain optical coherence tomography: a better OCT imaging strategy. BioTechniques. (2005) 39:S6–S13. doi: 10.2144/000112090
84. Miki, A, Medeiros, FA, Weinreb, RN, Jain, S, He, F, Sharpsten, L, et al. Rates of retinal nerve fiber layer thinning in glaucoma suspect eyes. Ophthalmology. (2014) 121:1350–8. doi: 10.1016/j.ophtha.2014.01.017
85. Nana Wandji, B, Dugauquier, A, and Ehongo, A. Visual field defects and retinal nerve fiber layer damage in buried optic disc drusen: a new insight. Int J Ophthalmol. (2022) 15:1641–9. doi: 10.18240/ijo.2022.10.12
86. Kwon, J, Choi, J, Shin, JW, Lee, J, and Kook, MS. Glaucoma diagnostic capabilities of foveal avascular zone parameters using optical coherence tomography angiography according to visual field defect location. J Glaucoma. (2017) 26:1120–9. doi: 10.1097/IJG.0000000000000800
87. Carvalho, J, Invernizzi, A, Martins, J, Renken, RJ, and Cornelissen, FW. Local neuroplasticity in adult glaucomatous visual cortex. Sci Rep. (2022) 12:21981. doi: 10.1038/s41598-022-24709-1
88. Suh, MH, Kim, SK, Park, KH, Kim, DM, Kim, SH, and Kim, HC. Combination of optic disc rim area and retinal nerve fiber layer thickness for early glaucoma detection by using spectral domain OCT. Graefes Arch Clin Exp Ophthalmol. (2013) 251:2617–25. doi: 10.1007/s00417-013-2468-3
89. Zhang, P, Wen, W, Sun, XH, and He, S. Selective reduction of fMRI responses to transient achromatic stimuli in the magnocellular layers of the LGN and the superficial layer of the SC of early Glaucoma patients. Hum Brain Mapp. (2016) 37:558–69. doi: 10.1002/hbm.23049
90. Storch, MW, Zinser, G, Lauermann, P, Khattab, MH, Nguyen-Höhl, A, Raddatz, D, et al. Influence of the size of the foveal avascular zone on functional and morphological parameters in patients with early-stage diabetic retinopathy. Clin Ophthalmol. (2022) 16:1207–13. doi: 10.2147/OPTH.S358467
91. Yang, Y, Zhu, XR, Xu, QG, Metcalfe, H, Wang, ZC, and Yang, JK. Magnetic resonance imaging retinal oximetry: a quantitative physiological biomarker for early diabetic retinopathy? Diabet Med. (2012) 29:501–5. doi: 10.1111/j.1464-5491.2011.03440.x
92. Kanagasingam, Y, Bhuiyan, A, Abramoff, MD, Smith, RT, Goldschmidt, L, and Wong, TY. Progress on retinal image analysis for age related macular degeneration. Prog Retin Eye Res. (2014) 38:20–42. doi: 10.1016/j.preteyeres.2013.10.002
93. Grosso, A, Mosley, TH, Klein, R, Couper, DJ, Tikellis, G, Wong, TY, et al. Is early age-related macular degeneration associated with cerebral MRI changes? The atherosclerosis risk in communities study. Am J Ophthalmol. (2007) 143:157–9. doi: 10.1016/j.ajo.2006.07.040
94. Gazzaz, ZJ, Butt, NS, Zubairi, NA, and Malik, AA. Scientometric evaluation of research productivity on diabetes from the Kingdom of Saudi Arabia over the last two decades (2000-2019). J Diabetes Res. (2020) 2020:1–10. doi: 10.1155/2020/1514282
95. Goerlandt, F, and Li, J. Forty years of risk analysis: a Scientometric overview. Risk Anal. (2022) 42:2253–74. doi: 10.1111/risa.13853
96. Tao, X, Hanif, H, Ahmed, HH, and Ebrahim, NA. Bibliometric analysis and visualization of academic procrastination. Front Psychol. (2021) 12:722332. doi: 10.3389/fpsyg.2021.722332
97. Dumoulin, SO, Fracasso, A, van der Zwaag, W, Siero, JCW, and Petridou, N. Ultra-high field MRI: advancing systems neuroscience towards mesoscopic human brain function. NeuroImage. (2018) 168:345–57. doi: 10.1016/j.neuroimage.2017.01.028
98. Hespel, AM, and Cole, RC. Advances in high-field MRI. Vet Clin North Am Small Anim Pract. (2018) 48:11–29. doi: 10.1016/j.cvsm.2017.08.002
99. Balchandani, P, and Naidich, TP. Ultra-high-field MR neuroimaging. AJNR Am J Neuroradiol. (2015) 36:1204–15. doi: 10.3174/ajnr.A4180
100. Di Salle, F, Esposito, F, Elefante, A, Scarabino, T, Volpicelli, A, Cirillo, S, et al. High field functional MRI. Eur J Radiol. (2003) 48:138–45. doi: 10.1016/j.ejrad.2003.08.010
101. Bruno, F, Arrigoni, F, Mariani, S, Patriarca, L, Palumbo, P, Natella, R, et al. Application of diffusion tensor imaging (DTI) and MR-tractography in the evaluation of peripheral nerve tumours: state of the art and review of the literature. Acta Biomed. (2019) 90:68–76. doi: 10.23750/abm.v90i5-S.8326
102. Tae, WS, Ham, BJ, Pyun, SB, Kang, SH, and Kim, BJ. Current clinical applications of diffusion-tensor imaging in neurological disorders. J Clin Neurol. (2018) 14:129–40. doi: 10.3988/jcn.2018.14.2.129
103. Lilja, Y, and Nilsson, DT. Strengths and limitations of tractography methods to identify the optic radiation for epilepsy surgery. Quant Imaging Med Surg. (2015) 5:288–99. doi: 10.3978/j.issn.2223-4292.2015.01.08
104. Kaushik, M, Graham, SL, Wang, CY, and Klistorner, A. A topographical relationship between visual field defects and optic radiation changes in Glaucoma. Invest Ophthalmol Vis Sci. (2014) 55:5770–5. doi: 10.1167/iovs.14-14733
105. Ferda, J, Ferdova, E, Hes, O, Mracek, J, Kreuzberg, B, and Baxa, J. PET/MRI: multiparametric imaging of brain tumors. Eur J Radiol. (2017) 94:A14–25. doi: 10.1016/j.ejrad.2017.02.034
106. Yousaf, T, Dervenoulas, G, and Politis, M. Advances in MRI methodology. Int Rev Neurobiol. (2018) 141:31–76. doi: 10.1016/bs.irn.2018.08.008
107. Bussel, I II, Wollstein, G, and Schuman, JS. OCT for glaucoma diagnosis, screening and detection of glaucoma progression. Br J Ophthalmol. (2014) 98:ii15–9. doi: 10.1136/bjophthalmol-2013-304326
108. Fu, J, Tan, S, Peng, C, Zhou, H, and Wei, S. A comparative study of alteration in retinal layer segmentation alteration by SD-OCT in neuromyelitis optica spectrum disorders: a systematic review and meta-analysis. Adv Ophthalmol Pract Res. (2021) 1:100007. doi: 10.1016/j.aopr.2021.100007
109. Zaharova, E, and Sherman, J. The use of SD-OCT in the differential diagnosis of dots, spots and other white retinal lesions. Eye Brain. Dove Medical Press (2011) 3:69–80. doi: 10.2147/EB.S23208
110. Rocholz, R, Corvi, F, Weichsel, J, Schmidt, S, and Staurenghi, G. OCT angiography (OCTA) in retinal diagnostics In: JF Bille, editor. High resolution imaging in microscopy and ophthalmology: New Frontiers in biomedical optics. Cham (CH): (2019)
111. Aghsaei Fard, M, and Ritch, R. Optical coherence tomography angiography in glaucoma. Ann Transl Med. (2020) 8:1204. doi: 10.21037/atm-20-2828
112. Rao, HL, Pradhan, ZS, Suh, MH, Moghimi, S, Mansouri, K, and Weinreb, RN. Optical coherence tomography angiography in Glaucoma. J Glaucoma. (2020) 29:312–21. doi: 10.1097/IJG.0000000000001463
113. Wylegala, A. Principles of OCTA and applications in clinical neurology. Curr Neurol Neurosci Rep. (2018) 18:96. doi: 10.1007/s11910-018-0911-x
Keywords: functional imaging, visual field defects, MRI, fMRI, OCT, DTI, OCTA
Citation: Cheng W, Liu J, Jiang T and Li M (2024) The application of functional imaging in visual field defects: a brief review. Front. Neurol. 15:1333021. doi: 10.3389/fneur.2024.1333021
Edited by:
Yuan Liu, University of Miami Health System, United StatesReviewed by:
Xiangxiang Liu, Capital Medical University, ChinaXing Ye, Nanjing Brain Hospital Affiliated to Nanjing Medical University, China
Copyright © 2024 Cheng, Liu, Jiang and Li. This is an open-access article distributed under the terms of the Creative Commons Attribution License (CC BY). The use, distribution or reproduction in other forums is permitted, provided the original author(s) and the copyright owner(s) are credited and that the original publication in this journal is cited, in accordance with accepted academic practice. No use, distribution or reproduction is permitted which does not comply with these terms.
*Correspondence: Moyi Li, bGltb3lpMTIzQGFsaXl1bi5jb20=
†These authors have contributed equally to this work and share first authorship