- 1Stress Neurophysiology Unit, French Armed Forces Biomedical Research Institute, Brétigny-sur-Orge, France
- 2CNES, Paris, France
- 3APEMAC/EPSAM, ER 4360, Metz, France
- 4VCR, École de Psychologues Praticiens, Catholic Institute of Paris, EA Religion, Culture et Société, Paris, France
- 5Department of Performance Psychology, Institute of Psychology, German Sport University Cologne, Cologne, Germany
- 6UFR STAPS, Normandie Université Caen, Caen, France
- 7VERT-EX, Caen, France
- 8CHU Caen, Caen, France
- 9French Military Health Service Academy, Paris, France
Introduction: Long-duration space missions will be a real challenge for maintaining astronauts' adaptability. Research on transcutaneous vagus nerve stimulation (taVNS) is expanding rapidly, and its modalities constitute a major research challenge. A growing number of reviews stress the need to validate biomarkers for monitoring effects to enhance our understanding of the processes by which taVNS acts. Heart rate variability (HRV) appears to be a relevant candidate that informs on the autonomic nervous system (ANS). This is a promising technique to minimize the pathogenic effects of such large-scale missions and thus might be a relevant countermeasure. This study aimed to investigate the impact of taVNS on cognitive, psychological, and physiological functioning, including ANS functioning, and the benefits of increasing the number of taVNS sessions.
Method: A total of 44 healthy participants were randomly assigned to one of the two cross-over protocols: a single session protocol (one taVNS and one sham simulation) or a repeated session protocol (three taVNS and three sham simulations). Cognitive, psychological, and physiological measures were performed before (pre) and after (post) each intervention. Sleep monitoring was only recorded before the first and after the last intervention in each protocol. For the repeated session protocol only, participants were allocated to two groups according to their parasympathetic activation gain during the three interventions: high parasympathetic delta (HPd) and low parasympathetic delta (LPd).
Results: Participants in the repeated session protocol increased their HRV, cognitive performance, and sleep efficiency. In particular, taVNS induced higher parasympathetic activation and cardiac flexibility compared to the sham simulation in the repeated session protocol. Nevertheless, the perception of stress may indicate a nocebo effect of the repeated session. The HPd profile had higher interoceptive awareness, HRV highlighted by non-linear measures, and cognitive performance, but presented a decrease in some indicators of sleep efficiency compared to the LPd profile.
Conclusion: taVNS seems to induce positive health outcomes, especially when the stimulation is repeated three times per week. Our findings highlight the benefits of parasympathetic activation during taVNS on psychophysiological and cognitive functioning. Further research is needed to validate these results on a large sample, using longitudinal measures over several months. This intervention appears promising as a countermeasure to extreme missions and occupations.
1 Introduction
Space exploration is undergoing a major revolution, with the launch of private enterprise, the first flights by space tourists, and a return to the Moon in the next few years. All these latest achievements have the ultimate goal of enabling human space travel to Mars. Nevertheless, sending humans into space requires an understanding of the effects of microgravity, cosmic radiation, and confinement during the 3-year mission. Far beyond the Van Allen belt, Mars is the most distant destination of all the planets in our solar system, where no human ever thought they would see the color of land. This is no longer a utopia dreamed up in a science fiction book. The ultimate challenge for future research is to explore countermeasures to maintain the adaptive capacities of crews during long missions. Thus, this study explores a new technique that improves the vagal tone to enhance an astronaut's adaptive abilities. The importance of the parasympathetic nervous system (PNS) tone in stress adaptation, particularly in extreme environments, has been recently demonstrated (1, 2). This activity regulates activation to changes in stress, and its level at rest has been associated with better post-challenge recovery capacities. These findings are part of recent data highlighting the role of high parasympathetic tone in psycho-physio-cognitive adaptation. Increasing parasympathetic tone could, therefore, be a relevant approach, one being vagus nerve stimulation (VNS).
1.1 Vagus nerve stimulation
The vagus nerve (VN) is the 10th cranial nerve. Anatomically, the VN has an afferent or sensory component and an efferent or motor component, which play an important role in maintaining homeostasis (3–5). The VN leaves the brainstem through the retro-olivary sulcus and the skull through the jugular foramen with nerves IX and XI. Thus, the nerve crosses the neck in the carotid sheath (between the carotid artery and jugular vein), the upper chest along the trachea, the lower chest and diaphragm along the esophagus, and then the abdominal cavity (6). Along the way, its branches innervate various structures such as the larynx, pharynx, heart, lungs, and gastrointestinal tract. In the brainstem, sensory afferent fibers terminate in the nucleus tractus solitarius, which then sends fibers that connect directly or indirectly to various brain regions. These regions include the dorsal raphe nuclei, locus coeruleus, amygdala, hypothalamus, thalamus, and orbitofrontal cortex. The VN fibers can be characterized as three types based on their diameter and myelination, inducing different excitation thresholds in response to a stimulus (7). Type A fibers are the first to be activated, and are large in diameter, highly myelinated, and have the lowest recruitment threshold (0.02–0.2 mA). Type B fibers are the second to be activated, and are intermediate in diameter and myelinated, with a medium intensity threshold (0.04–0.6 mA). Type C fibers are the smallest, are non-myelinated, and have the highest recruitment threshold (>2 mA). Several technologies have been developed for stimulating the vagal system. The VNS using an invasive VNS (iVNS) was a revolution for the treatment of drug-refractory epilepsy (1994 in Europe and 1997 in North America) and for treatment-resistant chronic depression (2005). Nevertheless, iVNS requires a costly and invasive surgical procedure involving the implantation of a helical bipolar electrode in the left cervical VN and a connection to a pulse generator, which is most often placed in a left infraclavicular subcutaneous pocket. Although this procedure can improve the health of many patients, the presence of numerous side effects necessitates a prior benefit/risk assessment (8). Transcutaneous auricular VNS (taVNS) enables non-invasive stimulation of the VN without surgery and avoids the side effects of iVNS. Recently developed, it targets the auricular branch of the vagus nerve (ABVN) skin receptor field in the outer ear. The human outer ear is supplied by three sensory nerves: the auriculotemporal nerve, the great auricular nerve, and the ABVN, which provide somatosensory innervation to the outer ear (9). More specifically, two auricular areas have been recognized as targeting the ABVN, namely, the cymba conchae and the tragus. The study conducted by Peuker and Filler (10) was the first anatomical study to report the complete nerve supply pathway and origin of each auricular branch in 14 human ears. The tragus is 45% innervated by the ABVN, while the cymba conchae has 100% of its fibers originating from the ABVN (10). Other studies using functional magnetic resonance imaging have shown that taVNS induces, unlike sham stimulation, higher activity in the nucleus tractus solitarius, left prefrontal cortex, and cingulate areas (11–14). Stimulation parameters for taVNS can differ in terms of current intensity (mA), pulse width (μs), frequency (Hz), duty cycle (s), and session duration (min) (15).
1.2 taVNS benefits across the body
Over the past few years, the literature has demonstrated the beneficial effects of taVNS in several domains: psychological disorders (e.g., depression, anxiety, fear, schizophrenia, and post-traumatic stress disorder), psychiatric disorders (e.g., epilepsy, headache, brain injury, stroke, and post-stroke rehabilitation), neurodegenerative (e.g., Parkinson's disease and cognitive decline), cognitive (e.g., cognitive disorders and disorders of consciousness), developmental (e.g., psychomotor retardation), genetic (e.g., Prader–Willi syndrome), cardiovascular (e.g., atrial fibrillation, myocardial infarction, heart failure, arrhythmia, and tachycardia), chronic diseases (e.g., diabetes, glucose intolerance, chronic pain, obesity, and dystonia), gastrointestinal (e.g., gastrointestinal dysfunction, postoperative bowel obstruction, inflammatory bowel disease, and colon cancer), and tinnitus treatment (8, 16, 17). Furthermore, taVNS improves many psychological functions, including wellbeing, alertness, and cognitive performance, in conjunction with a reduction in negative mood (18) and an improvement in learning (19). Recently, several studies have also highlighted its benefit in the treatment of COVID-19 (20–22). More specifically, taVNS is said to improve sleep quality, particularly in individuals who have insomnia (23–26).
1.3 HRV as a biomarker of taVNS effect
Heart rate variability (HRV) has become widely accepted as an objective marker of an individual's response to stimuli (27–29). Currently, the literature emphasizes the role of parasympathetic activity as a brake on stress (30–34). Porges (35) noted that HRV reflects both chronic stress and vulnerability to stress. Environmental responses are consistent with changes in activity in the sympathetic, parasympathetic, and enteric components of the visceral motor system that govern smooth muscle, cardiac muscle, and glands. These systems play a major role in sympathovagal balance and, therefore, contribute to the maintenance of adaptive homeostasis with interindividual differences (34). Thus, variations in HRV may reflect the body's and mind's resistance to a psychological or physical stressor. The cardiac biosignals could be an index of the heart's adaptability to changing environmental conditions. This balance has mainly been assessed by short-term temporal measurements, based on the variation between each consecutive heartbeat. In particular, those reflecting rapid beat-to-beat changes, such as the root mean square of successive differences (RMSSDs) and the percentage of successive normal sinus RR intervals separated by more than 50 ms (pNN50), have been shown to be reliable indices of parasympathetic vagal modulation (36, 37). Moreover, high-frequency power (HF, 0.15–0.40 Hz) reflects vagal-mediated HRV in the frequency domain when the respiratory rate is between 9 and 24 cycles per min (38). Thus, variation in HRV may reflect the body's and mind's resistance to a psychological or physical stressor, and cardiac biosignals could be an index of the heart's adaptability to changing environmental conditions that may be relevant for studying taVNS mechanisms.
1.4 Cognitive influence on adaptation
Cardiac vagal activity would also constitute a measure of the efficiency of an individual's executive functioning. Recently, a new neurocognitive test was developed and named “MindPulse” (39). The purpose of this test is to highlight the elements underlying perceptual-motor decision-making, in particular attentional and executive functions. More generally, this would enable an assessment of an individual's decision-making abilities. These are essential in many areas of everyday life, as well as for crews working in extreme environments. The balance between speed and precision in perceptual-motor decision-making is at stake in adaptation to a potentially changing environment (40). Decision-making is a fundamental adaptive process that enables an individual to choose one of several options (41, 42). The most advantageous option in the short and medium term remains the goal. This neurobiological process involves several cognitive, affective, and motivational functions associated with the activation of brain networks that rely on coordinated brain structures, among which the prefrontal cortex, amygdala, insula, and nucleus accumbens play key roles (43, 44). This also depends on the context in which individuals find themselves and their own internal needs and feelings (45). The influence of the individual's affective state on decision-making has been explored, notably by somatic marker theory (46). Somatic markers and the environment influence the speed of decision-making processes (45). In addition, the combination of an individual's emotional, motivational, and cognitive states, as well as characteristics specific to the environment, are the elements that enable an individual to choose rapidly one option over another (47, 48). These abilities are particularly sensitive to sleep deprivation (41, 49). They appear to be particularly affected in astronauts on space missions. Studies report a decrease in problem-solving ability, attention, memory (i.e., working, short-term, and spatial), learning, attention, and reaction times (50–56). These changes appear to be linked to sleep deprivation and circadian desynchronization problems faced by astronauts, as well as to stressors inherent in the environment, notably monotony (57–60). Decision-making is, therefore, the result of an interplay between different processes that may imply the vagal mind–body axis functioning.
1.5 Objectives of the study
Despite these promising data, the available studies highlight the existence of significant methodological shortcomings. The most important of these are the absence of consensus on optimal parameters for vagal stimulation, taVNS prescription procedures for intervention, and heterogeneity between clinical studies. A growing number of reviews emphasize the importance of validating biomarkers for taVNS monitoring effects to enhance our understanding of the processes involved in taVNS. These needs concern not only the patient but also the healthy subject under occupational stress, whose complaints in relation to thymia, sleep, efficiency, and wellbeing currently still have too few validated clinical responses. In particular, the restorative value of sleep has received little attention regarding the benefits of taVNS.
The issue of session repetition raises questions about the optimal number of taVNS sessions for the improvement target. Several studies demonstrate no effect of taVNS on the clinical target studied (61–63). More and more reviews are highlighting the need to use biomarkers to not only strengthen the understanding of taVNS efficacy but also to move toward a consensus methodology for taVNS use (15, 64–68). The article by Farmer et al., published in 2020, aimed to provide recommendations for future studies (69).
Therefore, this study aims to investigate the impact of taVNS on the quality of cognitive adaptation, parasympathetic tone, and sleep quality. An additional aim is to compare the benefits of single sessions with the application of repeated sessions. In light of the available literature on the optimal number of sessions (70–74), the focus is on three sessions over a week (73) to study the impact of repeating taVNS compared with a single taVNS session. Thus, this study proposes the following objectives: (1) to evaluate the impact of taVNS vs. sham intervention in the repeated sessions on cognitive (principal criteria) and physiological functioning compared to a single session; (2) to evaluate the impact of repeated sessions on sleep quality compared to a single session; (3) to evaluate the impact of taVNS vs. sham intervention on psychological functioning among the repeated sessions; and (4) to evaluate RMSSD modifications during repeated taVNS intervention as a biomarker of efficacity.
2 Materials and methods
2.1 Design
The present study (ID-RCB: 2022-A02512-41) was approved by the Committee for the Protection of Individuals (CPP Nord Ouest I, Rouen, France) and was conducted according to the standards of the Declaration of Helsinki. After comprehensive verbal and written presentations, all participants gave their written consent to participate.
2.2 Participants
A total of 44 healthy subjects (24 women and 20 men) were recruited for this study. Their health status was confirmed by a clinical history.
Demographics are given as mean ± standard deviation. The mean age was 30.09 ± 5.11 years (ranging from 24 to 43). Among the 24 women, 5 (20.83%) were using contraception (i.e., contraceptive pills). Only 10 of the participants were smokers (22.72%), and five participants were taking medication for hypertension, iron supplementation, salbutamol, and antihistamines. In total, 19 participants had a medical history including minor surgery, ear infections, tympanic perforation, and salivary gland infections. The average height was 172.56 ± 7.72 centimeters, and the average weight was 68.90 ± 12.12 kg.
Among subjects, 25 participants were single (56.81%), and 19 were part of a couple (43.18%), of which one had a child (2.27%). In total, 37 (84.09%) participants reported that they encountered major personal events and 32 (72.72%) reported facing professional stressful events. One (2.27%) participant had finished high school, 10 (22.72%) had a bachelor's degree, 25 had a master's degree (56.81%), and 8 (18.18%) had a PhD.
Table 1 reports sociodemographic characteristics.
2.3 Inclusion and exclusion criteria
The inclusion criteria included the following: (1) aged between 24 and 40 years old; (2) no anxiety or depressive disorders assessed by the Hospital Anxiety and Depression Scale (HAD, (75)); (3) no history of head trauma; (4) absence of a metallic implant; (5) no recent ear trauma; and (6) no facial or ear pain.
Exclusion criteria included the following: (1) no endocrinal pathology or treatment (e.g., hyperthyroidism, diabetes, hypertension, and sex reassignment); (2) pregnancy and breastfeeding; (3) anti-inflammatory treatment; (4) treatments interfering with heart rate (e.g., beta-blockers, calcium channel blockers, and α1 receptor agonists); (5) psychotropic treatment; (6) psychiatric or psychic disorders; (7) cardiovascular disorders; (8) alcohol dependence; (9) recent use of illicit drugs; (10) active implantable device (e.g., pacemaker); (11) personal or family history of epileptic seizures; (12) persons deprived of their liberty, persons hospitalized without consent, and persons admitted to a health or social institution for purposes other than research (L-1121-5 to L-1121-8-1; (13) minors (under 18 years old); and (14) protected adults and adults unable to express their consent who are not under protective supervision.
2.4 Experimental design
The study took place at the Hôtel Dieu Hospital (Paris, France) in June and July 2023. This is a single-center, cross-over, interventional, exploratory study to evaluate the potential of taVNS on cognitive and psychophysiological functioning. Participants were randomized into two cross-over protocols:
- Single session (n = 22): two interventions composed of one taVNS (A, taVNS*1) and one sham intervention per week (A', sham*1).
- Repeated session (n = 22): six interventions composed of three taVNS (B, C, D, taVNS*3) and three sham interventions per week (B', C', D', sham*3), scheduled every 2 or 3 days.
For each protocol, two stimulations were administered in a randomized order to compare the effects of within-subject vagal stimulation: taVNS (active) and sham (control); sham (control) and taVNS (active). Thus, depending on the randomization group, subjects started with either the taVNS or sham condition.
A washout period between each condition was 1 week, whatever the protocol (Figure 1).
Cognitive, psychological, and physiological data were assessed at each intervention, before (pre-intervention) and after (post-intervention) the stimulation protocol (i.e., taVNS or sham), except for the repeated session (taVNS*3, sham*3), in which the cognitive test was performed twice during the first and third interventions.
Sleep stages were monitored the night before the first intervention and the night after the last intervention for the repeated sessions protocol. Sleep diaries were completed in the same time period.
2.5 Data collection
A 15-item sociodemographic questionnaire was developed to collect general information on the participant's family situation, medical history, current health status, and hobbies.
2.5.1 Psychological measurements
A 32-item Multidimensional Assessment of Interoceptive Awareness (MAIA) questionnaire that evaluates interoceptive awareness was used. The scale is divided into eight sub-factors that measure awareness of uncomfortable, comfortable, and neutral body sensations, the response to sensations of pain and discomfort, the ability to regulate attention to body sensations, and awareness of mind–body integration (i.e., noticing, not being distracted, not worrying, attention regulation, emotional awareness, self-regulation, body listening, and trusting) (76).
A 14-item perceived stress scale (PSS) that assesses the subjective stress level was used (77).
2.5.2 Cognition measurements
Cognitive performance was evaluated using the MindPulse test, developed by Suarez et al. (39), which assesses decision-making. Subjects were seated in front of a computer screen that showed images requiring a response (instructions were displayed on the screen). The test battery consisted of three tests of increasing complexity. Each test began with a learning phase consisting of four trials, followed by a test phase. Different image packages were used for the learning and test phases. Each image was presented only once to avoid any learning effect.
The first test consisted of a Releasing Reaction Time Task (RRT). The participant was prompted to click down on a mouse button and maintain the pressure until a stimulus (an image presented in the center of the screen on a white background) appeared. At this point, the button should be released. Then, the screen remained blank for between 2 and 7s before another prompt appeared for the participant to press the mouse button. When a new image was shown on the screen, the mouse was meant to be released as fast as possible. The participant could practice as many times as necessary.
The second 1 Choice Releasing Reaction Time (1CRRT) test consisted of a Go/No-Go task. In the present study, the participant was instructed to only respond immediately (release the mouse button) to images of a certain color (white or gray, chosen randomly at the beginning of the test). If the image did not satisfy the color criteria, the subject was meant to maintain the pressure on the button until prompted to release it (after 3s). The third 2 Choice Releasing Reaction Time (2CRRT) test also consisted of a Go/No-Go task. In this case, the participant was asked to only respond to an image that met two criteria (the other color from the one-choice task and an animate or inanimate object, chosen randomly).
The computer recorded reaction time (RT) and response quality (the number and type of errors) for each trial. RT was divided into three main components: simple reaction time (SRT), executive speed (ES), and reaction to difficulty (RD). Figure 2 presents an overview of the MindPulse battery of tests.
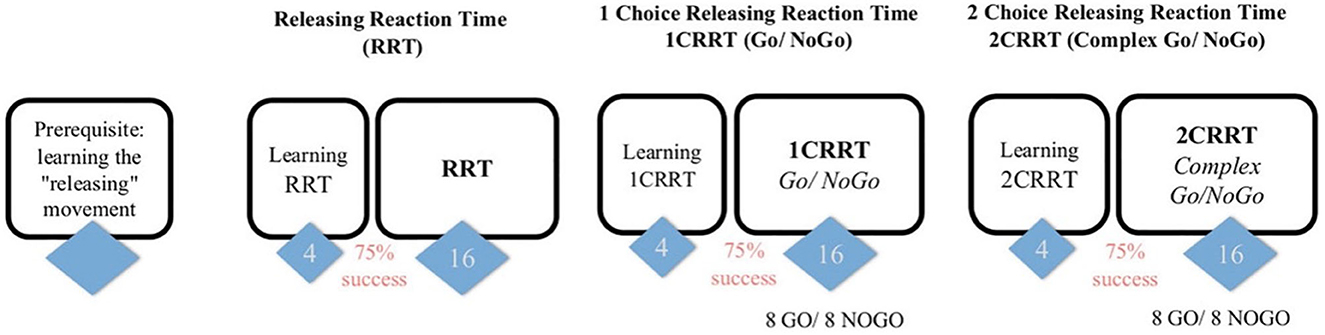
Figure 2. Overview of the MindPulse test battery. Figure reproduced from Suarez et al. (39). The test begins with training trials to learn the releasing movement. It is followed by three tests of increasing complexity. The first Releasing Reaction Task (RRT) consists of 16 trials. The second 1 Choice Releasing Reaction Task (1CRRT), and the third 2 Choice Releasing Reaction Task (2CRRT) tests each consist of eight Go and eight No-Go trials.
2.5.3 Physiological measurements
2.5.3.1 Sleep monitoring
Sleep stages were recorded using a DREEM v2 Headband. The device records the total recording time (TRT), total sleep time (TST), sleep onset latency (SOL), wake after sleep onset (WASO), total awake time during recording (WAKE), minutes of N1 sleep stages (N1 min), minutes of N2 sleep stages (N2 min), minutes of N3 sleep stages (N3 min), minutes of non-rapid eye movement sleep stages (NREM min), minutes of rapid eye movement sleep stages (REM min), percentage of TST in N1 sleep stages (N1%), percentage of TST in N2 sleep stages (N2%), percentage of TST in N3 sleep stages (N3%), percentage of TST in NREM sleep stages (NREM%), percentage of TST in REM sleep stages (REM%), sleep efficiency (SE), sleep onset (SO), latency to persistent sleep (LPS), mean respiration rate during the night (RRm), mean respiration rate during WAKE epochs (RR WAKE), mean respiration rate during N1 epochs (RR N1), mean respiration rate during N2 epochs (RR N2), mean respiration rate during N3 epochs (RR N3), and mean respiration rate during REM epochs (RR REM).
2.5.3.2 HRV
Heartbeat interval data (RR) were recorded using the gold-standard ECG data in an integrated system and software package (Biopac MP160 System Inc., California, USA) at a sampling frequency of 2,000 Hz. Each participant wore a 3-lead ECG (BN-EL45-LEADS3) configuration placed on the right chest, the left chest, and the lower left chest. An alcohol pad was used to remove the top layer of dead skin cells. Three self-adhesive disposable electrodes (2.5 × 2.5 cm) were placed on the abovementioned areas. Electrodes were connected to a Biopac BIONOMADIX ECG2-R. The positive lead was attached to the left chest electrode, the negative lead was attached to the right chest electrode (lead II derivation), and the ground electrode was attached to the lower left chest. RR data were recorded for a 10-min period in a sitting position at each intervention, except during the first and last interventions, in which a 10-min period in a standing position was added.
The HRV analysis followed guidelines reported in previous studies (38, 78), which take into account potential circadian variation, and used the PyHRV Python library (79). The following data were recorded: weight, height, waist-to-hip ratio, smoking habits, time of most recent alcohol intake (>24 h), time of most recent caffeine (coffee/tea) intake (>2 h), time of most recent meal (>2 h), time of most recent physical activity (>24 h), and quality of sleep on the day of the experiment and the preceding day.
Raw ECG data were filtered between 3 and 45 Hz using a finite impulse response band-pass filter. The order of the filter was set at 600 (0.3 times the sampling frequency). R peaks were automatically detected using the BioSPPy Python library (80). Hamilton segmentation was performed on the filtered signal, followed by R-peak correction with tolerance set to 0.05. R waves were manually examined to ensure correct detection. If an ECG sequence was overly noisy when visualizing the superposition of all QRS complexes, the time interval was manually removed to improve data quality. RR intervals were automatically detected with the hrvanalysis module using linear interpolation and manually corrected for artifacts and ectopic beats.
2.5.3.3 Time-domain analysis
Time-domain HRV metrics included the mean heart rate (mean HR), the mean interbeat interval (RR), the standard deviation of the normal-to-normal RR interval (SDNN), the root mean square of successive differences between adjacent RR intervals (RMSSD), and the percentage of adjacent NN intervals that differ from each other by more than 50 ms (pNN50).
2.5.3.4 Frequency-domain analysis
Frequency-domain HRV metrics complemented time-domain metrics and included oscillatory components of heart rate dynamics. Spectral density was estimated using Welch's method: low frequencies (i.e., sympathetic and parasympathetic activities) in the range of 0.04–0.15 Hz and high frequencies (i.e., parasympathetic activity) in the range of 0.15–0.4 Hz.
2.5.3.5 Non-linear analysis
Non-linear HRV metrics reflect dynamic and chaotic internal states that other metrics cannot reflect. The most representative metrics were used: the poincaré plot (i.e., graphical representation of the correlation between successive interbeat intervals), SD1 (standard deviation of instantaneous interbeat interval variability), SD2 (standard deviation of continuous, long-term RR variability), α1 (detrended fluctuation analysis self-similarity parameter that represented short-term fluctuations), α2 (detrended fluctuation analysis self-similarity parameter that represented long-term fluctuations), and sample entropy (i.e., regularity and complexity of time series).
2.6 Intervention
Independent of the intervention being the sham or taVNS stimulation, the subject's ears were inspected to ensure that the area was free of wounds and jewelry. The experimenter confirmed with the subject that there were no skin-related contraindications at the stimulation site, including sunburns, cuts, lesions, or open wounds. An alcohol preparation pad (70% isopropyl alcohol) was used to gently rub the target site, both internally and externally, to decrease skin resistance and increase conductance. The electrodes of the tVNS® L device (tVNS Technologies, Germany) were positioned in the left ear (i.e., even if the literature did not reveal any contraindication to lateral stimulation; see Farmer et al. (69) for a review and recommendations), at the level of the cymba conchae in the taVNS stimulation, or turned upside down and placed at the level of the earlobe in the sham stimulation (i.e., the earlobe is supposed to not contain vagal innervation and is considered relevant to compare its effect with the tragus located in the ABVN). White medical adhesive tape was used to secure the electrodes in place (Figure 3). The experimenter ensured that this was not uncomfortable.
The stimulation protocol then began. The experimenter explained that subjects would randomly feel an input in their ear and that two different protocols would be applied depending on how they felt: (1) If they felt nothing (sham), they should remain seated for 20 min in a half-seated position. (2) If they felt a sensory input (taVNS), the perceptual threshold would be determined before the participant remained seated for 20 min in a half-seated position. To determine the perception threshold, 5-s stimulation trials were applied, starting at 0.1 mA and increasing with each trial at a rate of 0.2 mA. Participants rated their current sensation (“How intense is the pain induced by the stimulation?”), ranging from 0 (“no sensation at all”) to 10 (“the strongest sensation imaginable”), until stabilizing at approximately 5 (“slight stinging”). In practice, in the event of a “stinging” or “tickling” sensation, the stimulation intensity was reduced by 50% and the previous step was repeated. If no “stinging” or “tickling” sensation was reported, the stimulation intensity was increased by 50%, and the initial step was repeated until a minimum of four “yes” responses to the “prickly” or “tickling” sensation were recorded. The intensity (in mA) applied was the value at which the subject said their fourth “yes” response after saying “no” (69, 81).
Accordingly, electrical stimulation was applied to the subject's left ear via a titanium electrode placed at the level of the cymba conchae in the taVNS condition or using the same electrode turned upside down and placed at the level of the earlobe in the sham condition. In the taVNS condition, parameters were predefined with a frequency of 25 Hz, pulse widths between 200 and 300 μs, and alternating intervals of 30-s stimulation ON and 30-s stimulation OFF. In the sham condition, no stimulation was delivered. At the same time as the intervention, an ECG (MP160, Biopac) was recorded to assess its implications as a biomarker of the response to vagal stimulation. After the taVNS or sham interventions, the experimenter inspected the ear for any redness or irritation at the stimulation site and noted the observations.
2.7 Statistical methods
Statistics were computed for all outcome measures. Data analyses were performed with JASP (Amsterdam, version 0.16.3), an open-source software package that is used for both classical and Bayesian analyses. Descriptive statistics were expressed as mean ± SD. The Shapiro–Wilk test was used to determine whether the data were normally distributed. When the analysis was significant, effect sizes were reported.
To compare the single and repeated sessions, deltas between the first and last sham and taVNS stimulations were calculated for each cognitive, psychological, and physiological variable. ANOVA two-way or Kruskal–Wallis signed rank analyses were used according to the normal distribution to evaluate the impact of the intervention (i.e., sham and taVNS) and session (i.e., single and repeat) on the cognitive and physiological deltas. Similar analysis strategies were used to evaluate the impact of time (i.e., pre-intervention and post-intervention) and intervention (i.e., sham and taVNS) on sleep monitoring. To explore the impact of the intervention on perceived subjective stress among the repeated sessions, we used repeated measures ANOVA.
To evaluate RMSSD modifications during repeated taVNS, the mean of the three values of RMSSD recorded during taVNS intervention was used to calculate a delta between the mean of RMSSD and RMSSD of the first pre-intervention. The median (=11.43) was used to differentiate our population on the RMSSD delta. Thus, we elaborated two profiles based on the parasympathetic gain during taVNS compared to before the first intervention. The first one, the high parasympathetic delta (HPd), had the most parasympathetic activation during the taVNS intervention, and the second one, the low parasympathetic delta (LPd), had the lowest parasympathetic activation during the taVNS intervention. Following the establishment of parasympathetic profiles, repeated measures ANOVA was used to evaluate the impact of parasympathetic activation profiles during taVNS on cognitive, psychological, and physiological functioning.
Holm post-hoc analyses were performed when the p-value was significant. Bayesian analyses were performed by applying equivalent analyses to ANOVA analyses. The Bayesian factor was calculated if no significant effect was detected. A low value was understood as supporting the null hypothesis, and a high value indicated evidence in favor of the alternative hypothesis (Supplementary material). For all analyses, statistical significance was set at a p-value of < 0.05. A p-value between 0.05 and 0.07 was considered evidence of a trend.
3 Results
3.1 Cognition and physiological functioning among single vs. repeated sessions
3.1.1 Cognition
A trend to session effects was found for the simple reaction time dispersion [F(1, 84) = 3.515, p = 0.064, w2 = 0.028] and outliers' answers [F(1, 84) = 3.240, p = 0.075, η2 = 0.037].
Participants in the repeated session had a higher simple reaction time dispersion but lower outliers' answers than those in the single session.
3.1.2 HRV
A significant session effect was found for HR [F(1, 84) = 4.371, p = 0.042, w2 = 0.036]. A significant session*intervention effect was found for SDNN [F(1, 84) = 6.203, p = 0.015, η2 = 0.067], RMSSD [F(1, 84) = 6.261, p = 0.014, η2 = 0.068], HF [F(1, 84) = 3.872, p = 0.052, η2 = 0.043], SD1 [F(1, 84) = 6.251, p = 0.014, η2 = 0.068], and SD2 [F(1, 84) = 5.758, p = 0.019, η2 = 0.062]. A trend for a significant session effect was found for the RR interval [F(1, 84) = 3.487, p = 0.065, η2 = 0.038].
Post-hoc analyses revealed that participants in the repeated session had a higher RMSSD (p = 0.035), HF (p = 0.052), and SD1 (p = 0.035) in the taVNS condition compared to the sham condition. Participants in the sham condition had a higher SDNN (p = 0.025) and a lower SD2 (p = 0.027) in the repeated session compared to those in the single session. Moreover, subjects in the repeated intervention had a higher HR and tended to have lower RR intervals compared to those in the single intervention group.
Table 2 presents a summary of the cognitive and physiological differences among interventions.
3.2 Exploration of psychological and physiological functioning among repeated sessions
3.2.1 Sleep measurements among repeated sessions
3.2.1.1 Sleep monitoring
Significant time*intervention effects were found for the TRT [F(1, 38) = 1.938, p = 0.013, η2 = 0.137], TST [F(1, 38) = 7.018, p = 0.012, η2 = 0.142], and LPS [F(1, 38) = 6.520, p = 0.015, η2 = 0.143]. A trend to a time*intervention effect was found for the WASO [F(1, 38) = 3.716, p = 0.061, η2 = 0.083] and NREM (min) [F(1, 38) = 3.892, p = 0.056, η2 = 0.084].
Post-hoc analyses revealed that participants in the repeated session who received the taVNS decreased their TRT (p = 0.022) and TST (p = 0.028) post-intervention compared to pre-intervention. They also decreased their TRT (p = 0.036) and TST (p = 0.030) post-interventions compared to the sham condition. Participants who received the taVNS tended to decrease their WASO (p = 0.068), NREM (min) (p = 0.062), and LPS (p = 0.072) post-interventions compared to those who received the sham stimulation.
Table 3 presents a summary of the sleep monitoring differences among interventions.
3.2.2 Impact of interventions on the perception of stress across time
3.2.2.1 Subjective stress
There was a significant effect of time [F(5, 210) = 2.921, p = 0.014, η2 = 0.046], a significant effect of time*intervention [F(5, 210) = 2.347, p = 0.042, η2 = 0.037], and a significant intervention effect [F(1, 42) = 7.964, p = 0.007, η2 = 0.040] on perceived stress.
Post-hoc analyses revealed that participants perceived more subjective stress post-second intervention compared to pre-first intervention (p = 0.005). At post-first intervention, those who received the taVNS stimulation had a higher perception of stress compared to those who received the sham stimulation (p = 0.044). Similar results were found. At post-second intervention, participants who received the sham stimulation had lower levels of subjective stress compared to those who received the taVNS stimulation at pre- (p = 0.017) and post- (p = 0.005) first interventions and pre-second intervention (p = 0.030). In addition, those who received the sham stimulation tended to have a higher perception of subjective stress at the post-third intervention compared to the post-second intervention (p = 0.074). Moreover, participants who received the taVNS intervention had a higher perception of stress than those who received the sham intervention. Table 4 presents the stress perception across time among interventions.
3.2.3 Impact of parasympathetic activation during taVNS
3.2.3.1 Cognition
Significant intervention effects were found for reaction to difficulty index [F(1, 20) = 5.538, p = 0.029, η2 = 0.097], Go/No-Go speed [F(1, 20) = 4.521, p = 0.046, η2 = 0.123], and a trend for the simple reaction time dispersion [F(1, 20) = 3.797, p = 0.066, η2 = 0.077].
The HPd profile had a lower reaction to the difficulty index and a higher Go/No-Go speed than the LPd profile. The HPd profile tended to have a higher simple reaction time dispersion than the LPd profile (Figure 4).
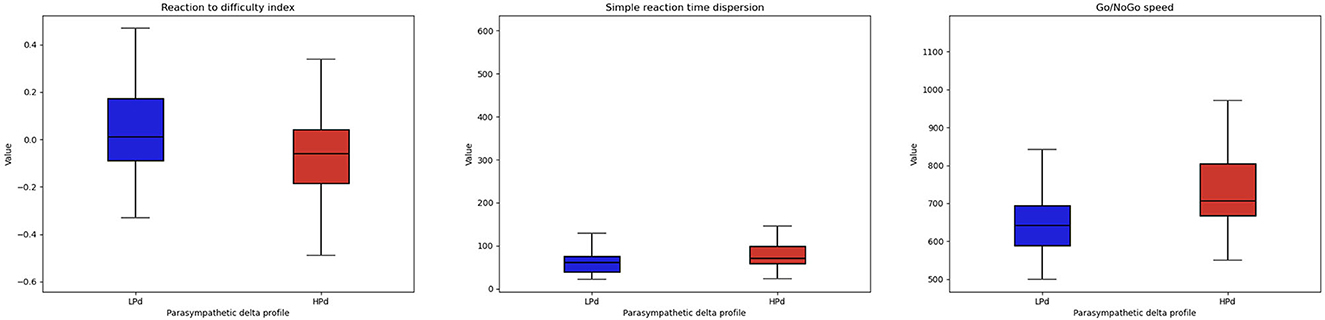
Figure 4. Cognitive performance among profiles. The HPa profile has a better cognitive performance at the MindPulse test compared to the LPa profile.
3.2.3.2 Interoception
A significant intervention group effect [F(1, 20) = 6.834, p = 0.017, η2 = 0.060] was found for trusting.
The HPd profile had higher interoceptive trust compared to the LPd profile (Figure 5).
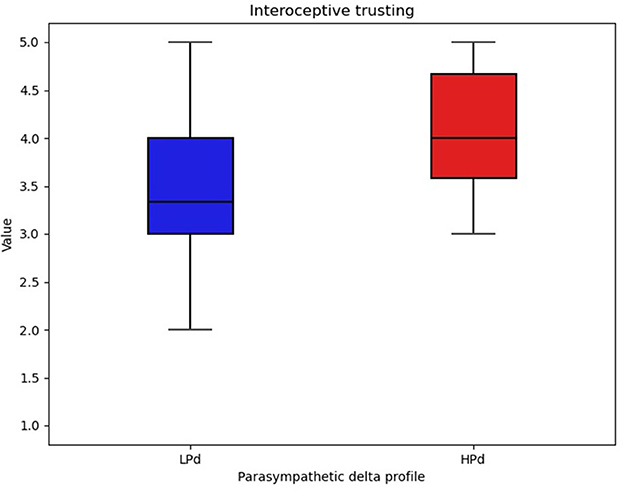
Figure 5. Interoceptive trust among profiles. The HPa profile has a higher trust compared to the LPa profile.
3.2.3.3 HRV
There were significant intervention group effects for SD ratio [F(1, 20) = 4.577, p = 0.045, η2 = 0.121], α1 [F(1, 20) = 5.833, p = 0.025, η2 = 0.167], α2 [F(1, 20) = 4.796, p = 0.041, η2 = 0.148], and SampEn [F(1, 20) = 9.209, p = 0.007, η2 = 0.194].
The HPd profile had a lower SD ratio and α1 as well as a higher α2 and SampEn compared to the LPd profile (Figure 6).
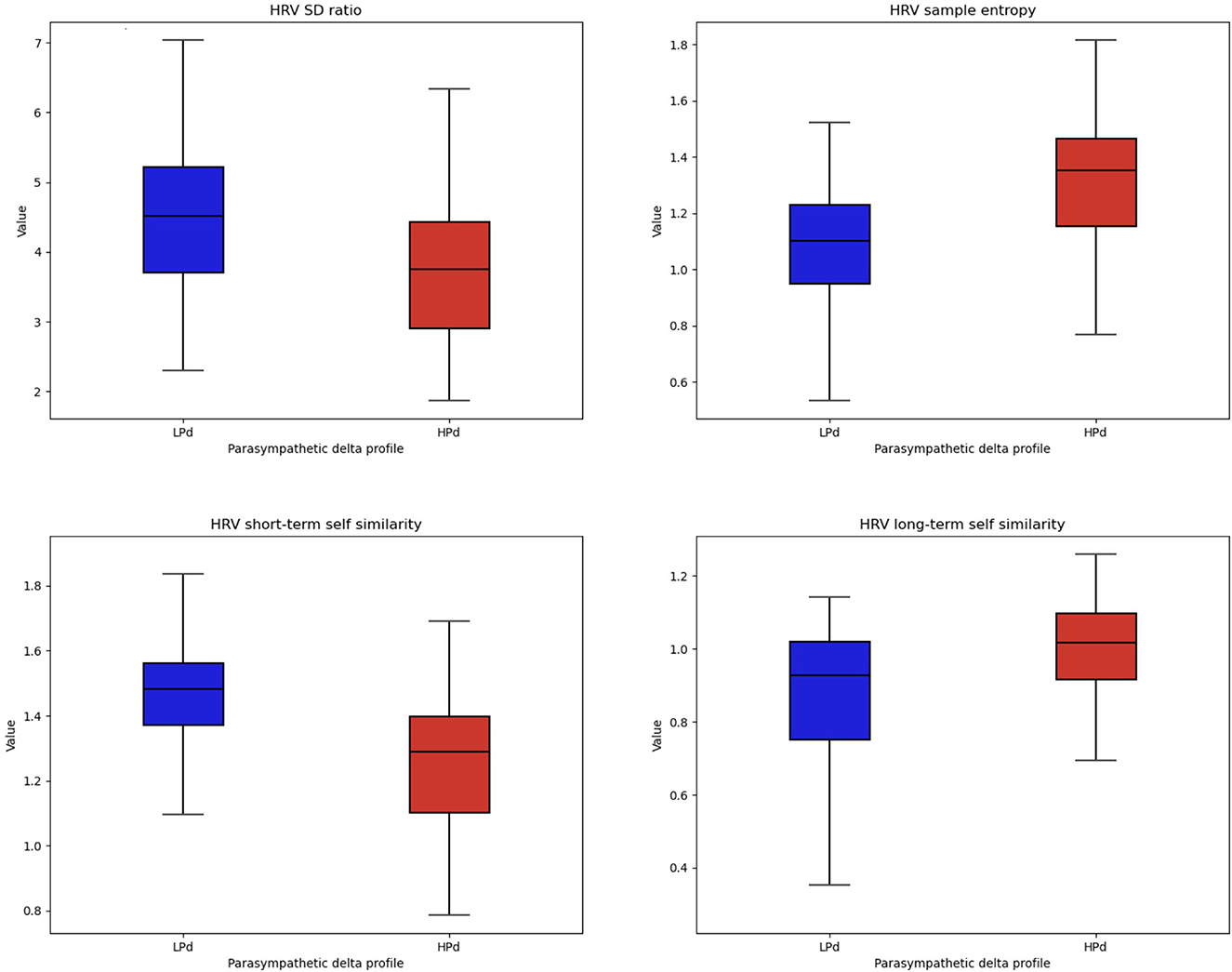
Figure 6. Non-linear HRV indices among profiles. The HPa profile has a higher HRV flexibility and self-similarity compared to the LPa profile.
3.2.3.4 Sleep monitoring
Significant intervention effects were found for WAKE [F(1, 19) = 5.043, p = 0.037, η2 = 0.210] and a trend for RR N1 [F(1, 19) = 3.471, p = 0.078, η2 = 0.154].
The HPd profile had a higher total awake time during recording and tended to have a higher respiration rate during N1 epochs than the LPd profile (Figure 7).
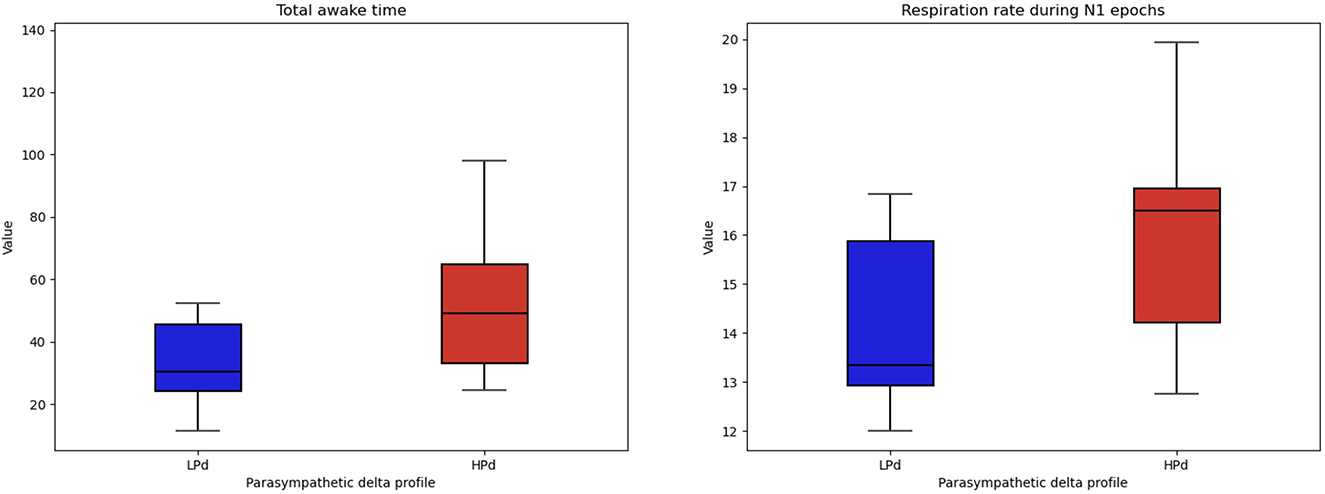
Figure 7. Sleep quality among profiles. The HPa profile has a higher total awake time during recording and tended to have a higher respiration rate during the first stage of sleep compared to the LPa profile.
Tables 5, 6 present the impact of parasympathetic activation during taVNS on psychophysiological and sleep functioning.
3.3 Side effects
Two subjects (4.54%) reported numbness in their hands immediately after stimulation. One subject (2.27%) experienced tinnitus and a rush of blood to the temples within minutes. Overall, sensations reported in this study ranged from warmth, buzzing, vibration, and tingling to small needles.
4 Discussion
Although a significant amount of research is still required, taVNS interventions offer a promising outcome in clinical medicine to improve the quality of life. This study aimed to investigate the impact of taVNS on the quality of cognitive and psychophysiological functioning and to compare the impacts of the number of sessions. Overall, this study shed light on the following main findings: (1) benefits of three sessions per week of taVNS instead of a single session; (2) positive outcomes of taVNS to improve cognitive, physiological, and psychological functioning as well as sleep quality; (3) taVNS intervention is not suitable for everyone; and (4) the more parasympathetic functioning is increased during the taVNS stimulation, the greater the health benefits.
4.1 The added value of repeated sessions to improve health
The results reveal two types of responses: physiology and cognition. A dose effect has been found in case repeated session (i.e., three interventions per week). The taVNS stimulation induced a higher parasympathetic functioning (RMSSD, HF) and better flexibility of the cardiac biosignal in the short-term variability compared to the sham. Interestingly, compared to the single session, the sham stimulation had a higher sympathetic activation (SDNN) and lower flexibility of the cardiac biosignal in the long-term variability. Moreover, all participants had a lower HRV in the repeated session compared to those in the single session. This last result seems to mainly translate the outcomes of the sham intervention instead of the taVNS intervention. In consequence, the repetition of the placebo intervention induces negative HRV effects.
Nevertheless, cognitive performance, especially through executive functions, is mitigated by the repetition of the interventions. Executive functions refer to the top-down mental processes that support goal-directed behavior (82).
Thus, even if a higher dispersion of the simple reaction time was observed, participants in the repeated session made lower outlier answers compared to those in the single session. A substantial body of empirical evidence exists connecting higher levels of cardiac vagal activity with better executive performance (83, 84). Borges et al. (85) conducted a study to examine the effects of taVNS on specific executive functions, including inhibitory control and cognitive flexibility. They highlighted that the expected association between taVNS and executive functions might be understood by considering the neuroanatomical pathways of the vagus nerve. Executive functions and cardiac vagal activity share overlapping neurological structures, both being regulated by cortical areas, notably the prefrontal cortex (86). Given that the taVNS signal is sent afferently to the prefrontal cortex via the ABVN, cardiac vagal activity could be affected by vagal stimulation (87). This hypothesis requires further study to be validated. Another important issue will be to confirm the dispersion of reaction times observed during repeated sessions. This possible instability and stability of reaction times could signal a decline in attentional and/or motivational functions.
The taVNS stimulation induced a decreased sleep time after the intervention compared to before the intervention. Nevertheless, taVNS stimulation increased their sleep quality with a decreased LPS and a trend for WASO and NREM. The literature among insomnia patients highlighted the use of taVNS to improve sleep (88, 89). Although a previous study showed a decrease in REM and an increase in NREM after VNS administration (90), others showed an increased number of periods in the REM phase (91, 92) in patients with epilepsy. As sleep progresses, parasympathetic vagal tone increases, and sympathetic tone decreases (93). Thus, NREM sleep has been associated with parasympathetic functioning. The ANS response is more complex regarding REM sleep. While REM sleep is composed of two phases, the parasympathetic vagal tone is linked to the tonic phase and the sympathetic tone to the phasic phase (94).
Altogether, these results are in line with the benefits of multiple stimulation sessions to induce better physiological and cognitive functioning.
4.2 Side effects of taVNS
The side effects of taVNS in the clinical population are small, but few are known in a healthy population. No discontinuation of the protocol occurred due to side effects. The sensations reported in this study ranged from warmth, buzzing, vibration, and tingling to small needles. In terms of side effects, two subjects reported numbness in their hands immediately after stimulation, while one experienced tinnitus and a rush of blood to the temples within minutes. These effects were minor and insignificant for the sample as a whole. The most common side effect is skin irritation at the stimulation site, but we had no cases of this in our study. Other side effects occurring in more than 1% of cases include nasopharyngitis, headache, dizziness, nausea/vomiting, and facial droop (64). Consequently, the use of taVNS in restricted environmental contexts is conceivable.
Furthermore, a dose effect on stress perception appears to be induced by repeated sessions of taVNS stimulation. Our results highlight that the taVNS stimulation induces higher levels of perceived stress than the sham stimulation. This finding is notably observed after the first intervention compared to the sham stimulation but also before and after the first intervention, as well as before the second intervention compared to the sham stimulation after the second intervention. This suggests that taVNS could induce a nocebo stress effect, which could imply an electrical trigger sensitization. Further studies are needed to evaluate nocebo hyperalgesia (95). Nevertheless, time induces several modifications in the perception of stress, according to the session. The taVNS stimulation seems to induce an increase in subjective stress compared to the sham stimulation at the beginning, but the sham stimulation tended to have higher levels of subjective stress after the third intervention compared to after the second intervention. As time progresses, the more the curve might be reversed from the last intervention onward. Interventional follow-up over several weeks would have been necessary to confirm or refute a decrease in stress for taVNS compared with sham. If these results were corroborated, it would be necessary to evaluate whether the experimental context of the repeated stimulation session has a nocebo effect per se. Moreover, some participants may experience a sensitization of the nociceptive system, resulting in nociceptive pain (96). Nevertheless, this pain sensation may be a sensory illusion in the central nervous system. Therefore, some individuals may feel pain and hypersensitivity toward the taVNS stimulation.
4.3 Implication of PNS baseline level on the positive outcomes of taVNS stimulation
The HPd profile had better HRV flexibility and self-similarity revealed by non-linear indices compared to the LPd profile. Non-linear indices have the potential to express added behavioral components that traditional temporal and frequential domains cannot capture and be associated with better health outcomes (38, 97).
Although the literature is cautious about the interpretation of non-linear indices, our results highlight that high parasympathetic activation reflects the greater complexity of the cardiac signal. These parameters were mostly correlated with the RMSSD (97), the index used to create our profiles, which provides a reflection of parasympathetic activity. One recommendation for assessing the effects of taVNS is to measure efferent VN activity by HRV. Modulation of VN is achieved with taVNS (69). Physiologically, increased efferent VN activity leads to a slowing of heart rate via inhibition of the sinus node by the release of acetylcholine, the VN's main neurotransmitter (38, 78). However, using RMSSD to measure the effect of taVNS on cardiac vagal activity, various studies have found no differences between active and sham pacing (98–101). Nevertheless, Clancy et al. (102) evaluated taVNS on the ANS response. They applied continuous stimulation for 15 min with a pulse width of 200 ms and a pulse frequency of 30 Hz, with the amplitude adjusted to the sensory threshold level (10–50 mA). The authors showed that taVNS significantly increased HRV in healthy participants, indicating a shift in cardiac autonomic function toward parasympathetic predominance. Microneurographic recordings also revealed a significant decrease in the frequency and incidence of muscle sympathetic nerve activity during taVNS. Thus, ECG measurements are considered one of the most promising biomarkers (102, 103). Recently, Machetanz et al. (104) highlighted that RMSSD, SD1, and SDNN could be particularly suitable biomarkers for taVNS adaptation. They also showed a decrease in HRV when stimulation was localized to the cavum conchae rather than the cymba conchae. In another study, Machetanz et al. (105) confirmed a decrease in HRV during the stimulation of the cavum conchae. They emphasized the potential of HRV to optimally define taVNS parameters and targets. Therefore, an increase in HRV in its parasympathetic component might be an efficient candidate for studying the mechanisms of action of taVNS (106) and may also help predict the efficacy of taVNS.
Findings also reported that the HPd profile had a higher level of interoceptive trust compared to the LPd profile. Thus, participants gain confidence in their bodily sensations. Preliminary results have shown that taVNS has the potential to improve interoceptive functioning (107). These authors observed an improvement in interoceptive sensitivity (i.e., subjective evaluation of interoceptive signals), while no difference in interoceptive acuity (i.e., perception and detection of signals coming from the inner body) was demonstrated. The MAIA questionnaire (108) assesses interoceptive sensitivity. Further studies should be carried out to explore the impact of taVNS on the integration of interoceptive information and its relationships with the ANS response.
Furthermore, our results suggest that higher parasympathetic activation during taVNS is associated with better cognitive performance at the MindPulse test (39). The HPd profile had a lower reaction to difficulty index, a higher Go/No-Go speed, and a tendency to increase simple reaction time dispersion compared to the LPd profile. The reaction to difficulty index was developed to measure how an individual may cope with difficulty by accelerating or decelerating (39). Depending on the taVNS stimulation or sham, participants use different strategies to deal with difficulty. In the context of higher parasympathetic activation during the taVNS stimulation, participants slow down instead of going faster for the HPd. The HPd profile seems to be cautious. Overall, given the role of the vagus nerve in cognitive functioning, an increase in parasympathetic HRV may be a mechanistic candidate for the impact of taVNS on executive functions (106). Borges et al. (85) also provided arguments that taVNS may have very specific effects on cognitive processes. In addition, they showed an increase in HF during each of the cognitive flexibility tasks, although HF did not differ between the taVNS and sham conditions. More recently, a study by McIntire et al. (109) evaluated the efficacy of transcutaneous cervical vagal nerve stimulation in attenuating the negative effects of fatigue on cognition and mood. They showed that the intervention group that received stimulation had significantly better results in terms of arousal and cognitive functioning after prolonged 24-h wakefulness. They had significantly lower rates of fatigue than the control group throughout the study (109).
Concerning sleep quality, the HPd profile had a higher total awake time during recording and tended to have a higher respiration rate during the first stage of sleep than the LPd profile. These counter-intuitive results need to be confirmed by further studies.
4.4 taVNS as a countermeasure for challenging missions and professions as astronauts
taVNS is an effortless tool to implement with fewer side effects. Data from space analogs have highlighted the sometimes deleterious, sometimes salutogenic effects of these environments on adaptation (1, 60) and increased operational risks as long-term stress tends to degrade human performance (110). Among astronauts, sleep deprivation is associated with abrupt changes in wake and sleep schedules, the absence of a 24-h light–dark cycle, a high workload, and physical stress. The literature has shown that poor-quality sleep is one of the main factors influencing neuropsychological changes (111). Indeed, sleep disorders are frequently reported (i.e., increased sleep latency, decreased sleep efficiency, and reduced delta sleep duration) (112–115). In recent years, the number of publications proposing effective countermeasures has grown steadily (53, 60, 116–119).
Given the promising results of this study on stress regulation, sleep, and executive functions in healthy adults, taVNS might be a relevant countermeasure to the pathogenic effects of missions. Therefore, there is a need for further studies to better understand the benefits of taVNS in this population, its modalities of use, and the psychophysiological profile of subjects for whom taVNS is most effective. If positive effects on cognitive, psychological, and physiological levels are confirmed in healthy populations, taVNS could be considered a countermeasure for several challenging populations, including those in military and emergency situations.
5 Limitations
This study has several methodological shortcomings. The first is the small sample size and an imbalance between male and female participants and right- and left-handed subjects. Second, our study does not include a control group that does not belong to any of the taVNS or sham conditions. A larger study should include this third control group to monitor the impact of intervention responses. Third, our results are dependent on the conditions in which our participants found themselves at each stage. The lack of control over life events between each of the sessions can constitute a bias. Fourth, psychological and interoceptive data (i.e., collected through questionnaires) are subjective measures. Intelligent sensors would provide more objective measures of subjects' adaptation. Finally, no longitudinal monitoring was carried out outside the intervention period. Regular monitoring beyond the study period would have enabled us to assess the evolution of the observed effects.
6 Conclusion
The present study found the following main findings: (1) benefits of three sessions per week of taVNS instead of a single session; (2) positive outcomes of taVNS to improve physiological and psychological functioning as well as sleep quality; (3) taVNS intervention is not suitable for everyone; and (4) the level of increase in parasympathetic functioning could be an indicator of the positive effects of the taVNS stimulation. Overall, these results offer promising health outcomes for extreme missions and occupations, such as astronauts in light of future space missions. This may be a relevant countermeasure to deal with intense stressors. Further studies are needed to confirm our results.
Data availability statement
The datasets presented in this article are not readily available because they are the owned by the French Health Military Service. Requests to access the datasets should be directed to MT, bWFyaW9uLnRyb3Vzc2VsYXJkJiN4MDAwNDA7Z21haWwuY29t.
Ethics statement
The studies involving humans were approved by CPP Nord Ouest I Université de Rouen- Factulte de Médecine/Pharmacie Bâtiment Stewart 6ème Etage - Rue du Professeur Stewart 76000 ROUEN France. The studies were conducted in accordance with the local legislation and institutional requirements. The participants provided their written informed consent to participate in this study.
Author contributions
BLR: Conceptualization, Data curation, Formal analysis, Investigation, Methodology, Project administration, Supervision, Validation, Visualization, Writing—original draft, review & editing. CM-K: Supervision, Writing—review & editing. AG: Investigation, Writing—review & editing. SJ: Investigation, Writing—review & editing. CV: Investigation, Writing—review & editing. SL: Conceptualization, Writing—review & editing. DC: Writing—review & editing. SB: Supervision, Writing—review & editing. MT: Conceptualization, Investigation, Supervision, Writing—review & editing.
Funding
The author(s) declare financial support was received for the research, authorship, and/or publication of this article. The work was supported by the French Space Agency (CNES; n°4800001159) and the French Military Health Service.
Acknowledgments
The authors wish to thank the volunteers who took part in this study and the data collection team. The authors also would like to thank the French Space Agency (CNES) and the French Government Defense (AID) for their support. We are grateful to the Human Adaptation Institute for their support and thank MindPulse for providing us with their technology. The data used in this study are the property of the French Health Service. Any requests must be filed with and evaluated by the French Military Staff.
Conflict of interest
SB was the head of the VERT-EX company.
The remaining authors declare that the research was conducted in the absence of any commercial or financial relationships that could be construed as a potential conflict of interest.
Publisher's note
All claims expressed in this article are solely those of the authors and do not necessarily represent those of their affiliated organizations, or those of the publisher, the editors and the reviewers. Any product that may be evaluated in this article, or claim that may be made by its manufacturer, is not guaranteed or endorsed by the publisher.
Supplementary material
The Supplementary Material for this article can be found online at: https://www.frontiersin.org/articles/10.3389/fneur.2023.1286919/full#supplementary-material
Abbreviations
ABVN, auricular branch of the vagus nerve; α1, detrended fluctuation analysis self-similarity parameter that represents short-term fluctuations; α2, detrended fluctuation analysis self-similarity parameter that represents long-term fluctuations; ES, executive speed; HR, heart rate; HRV, heart rate variability; HF, high frequency; LPd, high parasympathetic delta; iVNS, invasive vagus nerve stimulation; LF, low frequency; LPd, low parasympathetic delta; MAIA, multidimensional assessment of interoceptive awareness; 1CRRT, one choice releasing reaction time; PNS, parasympathetic nervous system; PSS, perceived stress scale; pNN50, percentage of adjacent NN intervals that differ from each other by more than 50 ms; RT, reaction time; RD, reaction to difficulty; RRT, releasing reaction time task; RMSSD, root mean square of successive difference; SampEn, sample entropy; SRT, simple reaction time; SDNN, standard deviation of the normal-to-normal RR interval; SD1, standard deviation of instantaneous short-term RR variability; SD2, standard deviation of continuous long-term RR variability; RR, successive variation of the intervals between two heartbeats; taVNS, transcutaneous vagus nerve stimulation; 2CRRT, two simultaneous choices, releasing reaction time; VN, vagus nerve; VNS, vagus nerve stimulation.
References
1. Le Roy B, Aufauvre-Poupon C, Ferragu A, Vannier A, Martin-Krumm C, Trousselard M. Cardiac biosignal in confined nuclear submarine patrol: heart rate variability as a marker of adaptation. Acta Astronaut. (2023) 203:469–82. doi: 10.1016/j.actaastro.2022.12.014
2. Lefranc B, Aufauvre-Poupon C, Martin-Krumm C, Trousselard M. Cardiac biosignal and adaptation in confined nuclear submarine patrol. World academy of science, engineering and technology, open science index 174. Int J Med Health Sci. (2021). 15:190–201.
3. Yuan H, Silberstein SD. Vagus nerve and vagus nerve stimulation, a comprehensive review: part I. Headache. (2016) 56:71–8. doi: 10.1111/head.12647
4. Yuan H, Silberstein SD. Vagus nerve and vagus nerve stimulation, a comprehensive review: part II. Headache. (2016) 56:259–66. doi: 10.1111/head.12650
5. Yuan H, Silberstein SD. Vagus nerve and vagus nerve stimulation, a comprehensive review: part III. Headache. (2016) 56:479–90. doi: 10.1111/head.12649
6. Krahl SE. Vagus nerve stimulation for epilepsy: a review of the peripheral mechanisms. Surg Neurol Int. (2012) 3:S47–52. doi: 10.4103/2152-7806.91610
7. Yuan H, Silberstein SD. Vagus nerve stimulation and headache. Headache. (2017) 57:29–33. doi: 10.1111/head.12721
8. Kong J, Fang J, Park J, Li S, Rong P. Treating depression with transcutaneous auricular Vagus nerve stimulation: state of the art and future perspectives. Front Psychiatry. (2018) 9:20. doi: 10.3389/fpsyt.2018.00020
9. Butt MF, Albusoda A, Farmer AD, Aziz Q. The anatomical basis for transcutaneous auricular vagus nerve stimulation. J Anat. (2020) 236:588–611. doi: 10.1111/joa.13122
10. Peuker ET, Filler TJ. The nerve supply of the human auricle. Clin Anat. (2002) 15:35–7. doi: 10.1002/ca.1089
11. Kraus T, Kiess O, Hösl K, Terekhin P, Kornhuber J, Forster C. CNS BOLD fMRI effects of sham-controlled transcutaneous electrical nerve stimulation in the left outer auditory canal - a pilot study. Brain Stimul. (2013) 6:798–804. doi: 10.1016/j.brs.2013.01.011
12. Frangos E, Ellrich J, Komisaruk BR. Non-invasive access to the vagus nerve central projections via electrical stimulation of the external ear: fMRI evidence in humans. Brain Stimul. (2015) 8:624–36. doi: 10.1016/j.brs.2014.11.018
13. Yakunina N, Kim SS, Nam EC. Optimization of transcutaneous vagus nerve stimulation using functional MRI. Neuromodulation. (2017) 20:290–300. doi: 10.1111/ner.12541
14. Badran BW, Dowdle LT, Mithoefer OJ, LaBate NT, Coatsworth J, Brown JC, et al. Neurophysiologic effects of transcutaneous auricular vagus nerve stimulation (taVNS) via electrical stimulation of the tragus: a concurrent taVNS/fMRI study and review. Brain Stimul. (2018) 11:492–500. doi: 10.1016/j.brs.2017.12.009
15. Badran BW, Yu AB, Adair D, Mappin G, DeVries WH, Jenkins DD, et al. Laboratory administration of transcutaneous auricular vagus nerve stimulation (taVNS): technique, targeting, and considerations. J Vis Exp. (2019). doi: 10.3791/58984
16. Ridgewell C, Heaton KJ, Hildebrandt A, Couse J, Leeder T, Neumeier WH. The effects of transcutaneous auricular vagal nerve stimulation on cognition in healthy individuals: a meta-analysis. Neuropsychology. (2021) 35:352–65. doi: 10.1037/neu0000735
17. Wang L, Wang Y, Wang Y, Wang F, Zhang J, Li S, et al. Transcutaneous auricular vagus nerve stimulators: a review of past, present, and future devices. Expert Rev Med Devices. (2022) 19:43–61. doi: 10.1080/17434440.2022.2020095
18. Kaniusas E, Kampusch S, Tittgemeyer M, Panetsos F, Gines RF, Papa M, et al. Current directions in the auricular vagus nerve stimulation i - a physiological perspective. Front Neurosci. (2019) 13:854. doi: 10.3389/fnins.2019.00854
19. D'Agostini M, Burger AM, Franssen M, Claes N, Weymar M, von Leupoldt A, et al. Effects of transcutaneous auricular vagus nerve stimulation on reversal learning, tonic pupil size, salivary alpha-amylase, and cortisol. Psychophysiology. (2021) 58:e13885. doi: 10.1111/psyp.13885
20. Ferrer MH, Severin R, Lefort H, Jacob S, Trousselard M. [Improving parasympathetic functioning to contain the inflammatory response of COVID-19 infection]. Soins. (2020) 65:63–5. doi: 10.1016/S0038-0814(20)30248-6
21. Dedoncker J, Vanderhasselt MA, Ottaviani C, Slavich GM. Mental health during the COVID-19 pandemic and beyond: the importance of the vagus nerve for biopsychosocial resilience. Neurosci Biobehav Rev. (2021) 125:1–10. doi: 10.1016/j.neubiorev.2021.02.010
22. Kaniusas E, Szeles JC, Kampusch S, Alfageme-Lopez N, Yucuma-Conde D, Li X, et al. Non-invasive auricular vagus nerve stimulation as a potential treatment for Covid19-originated acute respiratory distress syndrome. Front Physiol. (2020) 11:890. doi: 10.3389/fphys.2020.00890
23. Luo M, Qu X, Li S, Zhao J, Zhao Y, Jiao Y, et al. Transcutaneous vagus nerve stimulation for primary insomnia and affective disorder: a report of 35 cases. Zhongguo Zhen Jiu. (2017) 37:269–73. doi: 10.13703/j.0255-2930.2017.03.013
24. Jiao Y, Guo X, Luo M, Li S, Liu A, Zhao Y, et al. Effect of transcutaneous vagus nerve stimulation at auricular concha for insomnia: a randomized clinical trial. Evid Based Complement Alternat Med. (2020) 6049891. doi: 10.1155/2020/2536573
25. Yoon H–K. Therapeutic application of transcutaneous auricular vagus nerve stimulation in primary insomnia. Chronobiol Med. (2019) 1:51–4. doi: 10.33069/cim.2019.0011
26. Zhao B, Bi Y, Li L, Zhang J, Hong Y, Zhang L, et al. the instant spontaneous neuronal activity modulation of transcutaneous auricular vagus nerve stimulation on patients with primary insomnia. Front Neurosci. (2020) 14:205. doi: 10.3389/fnins.2020.00205
27. Hjortskov N, Rissén D, Blangsted AK, Fallentin N, Lundberg U, Søgaard K. The effect of mental stress on heart rate variability and blood pressure during computer work. Eur J Appl Physiol. (2004) 92:84–9. doi: 10.1007/s00421-004-1055-z
28. Taelman J, Vandeput S, Spaepen A, Van Huffel S. Influence of mental stress on heart rate and heart rate variability. In: 4th European Conference of the International Federation for Medical and Biological Engineering, eds J. Sloten, P. Verdonck, M. Nyssen, and J. Haueisen (Antwerp: Springer Berlin Heidelberg) (2009). p. 1366–9. doi: 10.1007/978-3-540-89208-3_324
29. Thayer JF, Ahs F, Fredrikson M, Sollers JJ 3rd, Wager TD. A meta-analysis of heart rate variability and neuroimaging studies: implications for heart rate variability as a marker of stress and health. Neurosci Biobehav Rev. (2012) 36:747–56. doi: 10.1016/j.neubiorev.2011.11.009
30. Brosschot JF, Verkuil B, Thayer JF. The default response to uncertainty and the importance of perceived safety in anxiety and stress: an evolution-theoretical perspective. J Anxiety Disord. (2016) 41:22–34. doi: 10.1016/j.janxdis.2016.04.012
31. Brosschot JF, Verkuil B, Thayer JF. Exposed to events that never happen: generalized unsafety, the default stress response, and prolonged autonomic activity. Neurosci Biobehav Rev. (2017) 74:287–96. doi: 10.1016/j.neubiorev.2016.07.019
32. Brosschot JF, Verkuil B, Thayer JF. Generalized unsafety theory of stress: unsafe environments and conditions, and the default stress response. Int J Environ Res Public Health. (2018) 15:464. doi: 10.3390/ijerph15030464
33. Dell'Acqua C, Dal Bò E, Benvenuti SM, Palomba D. Reduced heart rate variability is associated with vulnerability to depression. J Affect Disord Rep. (2020) 1:100006. doi: 10.1016/j.jadr.2020.100006
34. Kim HG, Cheon EJ, Bai DS, Lee YH, Koo BH. Stress and heart rate variability: a meta-analysis and review of the literature. Psychiatry Investig. (2018) 15:235–45. doi: 10.30773/pi.2017.08.17
35. Porges SW. Vagal tone: a physiologic marker of stress vulnerability. Pediatrics. (1992) 90:498–504. doi: 10.1542/peds.90.3.498
36. Burger AM, D'Agostini M, Verkuil B, Van Diest I. Moving beyond belief: a narrative review of potential biomarkers for transcutaneous vagus nerve stimulation. Psychophysiol. (2020). 57:e13571. doi: 10.1111/psyp.13571
37. Chapleau MW, Sabharwal R. Methods of assessing vagus nerve activity and reflexes. Heart Fail Rev. (2011) 16:109–27. doi: 10.1007/s10741-010-9174-6
38. Laborde S, Mosley E, Thayer JF. Heart rate variability and cardiac vagal tone in psychophysiological research - recommendations for experiment planning, data analysis, and data reporting. Front Psychol. (2017) 8:213. doi: 10.3389/fpsyg.2017.00213
39. Suarez S, Eynard B, Granon S. A Dissociation of attention, executive function and reaction to difficulty: development of the mindpulse test, a novel digital neuropsychological test for precise quantification of perceptual-motor decision-making processes. Front Neurosci. (2021) 15:650219. doi: 10.3389/fnins.2021.650219
40. Godefroy O, Martinaud O, Narme P, Joseph PA, Mosca C, Lhommée E, et al. Dysexecutive disorders and their diagnosis: a position paper. Cortex. (2018) 109:322–35. doi: 10.1016/j.cortex.2018.09.026
41. Pittaras E, Callebert J, Dorey R, Chennaoui M, Granon S, Rabat A. Mouse Gambling Task reveals differential effects of acute sleep debt on decision-making and associated neurochemical changes. Sleep. (2018) 41:zsy168. doi: 10.1093/sleep/zsy168
42. Cabeza L, Giustiniani J, Chabin T, Ramadan B, Joucla C, Nicolier M, et al. Modelling decision-making under uncertainty: a direct comparison study between human and mouse gambling data. Eur Neuropsychopharmacol. (2020) 31:58–68. doi: 10.1016/j.euroneuro.2019.11.005
43. Volz KG, Schubotz RI, Von Cramon DY. Decision-making and the frontal lobes. Curr Opin Neurol. (2006) 19:401–6. doi: 10.1097/01.wco.0000236621.83872.71
44. Pittaras E, Callebert J, Chennaoui M, Rabat A, Granon S. Individual behavioral and neurochemical markers of unadapted decision-making processes in healthy inbred mice. Brain Struct Funct. (2016) 221:4615–29. doi: 10.1007/s00429-016-1192-2
45. Bechara A, Damasio H, Damasio AR. Emotion, decision making and the orbitofrontal cortex. Cereb Cortex. (2000) 10:295–307. doi: 10.1093/cercor/10.3.295
46. Bechara A, Damasio AR, Damasio H, Anderson SW. Insensitivity to future consequences following damage to human prefrontal cortex. Cognition. (1994) 50:7–15. doi: 10.1016/0010-0277(94)90018-3
47. Ernst M, Paulus MP. Neurobiology of decision making: a selective review from a neurocognitive and clinical perspective. Biol Psychiatry. (2005) 58:597–604. doi: 10.1016/j.biopsych.2005.06.004
49. Rabat A, Gomez-Merino D, Roca-Paixao L, Bougard C, Van Beers P, Dispersyn G, et al. Differential kinetics in alteration and recovery of cognitive processes from a chronic sleep restriction in young healthy Men. Front Behav Neurosci. (2016) 10:95. doi: 10.3389/fnbeh.2016.00095
50. Clément GR, Boyle RD, George KA, Nelson GA, Reschke MF, Williams TJ, et al. Challenges to the central nervous system during human spaceflight missions to Mars. J Neurophysiol. (2020) 123:2037–63. doi: 10.1152/jn.00476.2019
51. Marazziti D, Arone A, Ivaldi T, Kuts K, Loganovsky K. Space missions: psychological and psychopathological issues. CNS Spectr. (2021) 27:536–40. doi: 10.1017/S1092852921000535
52. Oluwafemi FA, Abdelbaki R, Lai JC, Mora-Almanza JG, Afolayan EM. A review of astronaut mental health in manned missions: potential interventions for cognitive and mental health challenges. Life Sci Space Res. (2021) 28:26–31. doi: 10.1016/j.lssr.2020.12.002
53. Pagel JI, Choukèr A. Effects of isolation and confinement on humans-implications for manned space explorations. J Appl Physiol. (2016) 120:1449–57. doi: 10.1152/japplphysiol.00928.2015
54. Schneider S, Bubeev JA, Choukèr A, Morukov B, Johannes B, Strüder HK. Imaging of neuro-cognitive performance in extreme environments—A (p) review. Planet Space Sci. (2012) 74:135–41. doi: 10.1016/j.pss.2012.06.021
55. Shepanek M. Human behavioral research in space: quandaries for research subjects and researchers. Aviat Space Environ Med. (2005) 76:B25–30.
56. Strangman GE, Sipes W, Beven G. Human cognitive performance in spaceflight and analogue environments. Aviat Space Environ Med. (2014) 85:1033–48. doi: 10.3357/ASEM.3961.2014
57. Flynn CF. An operational approach to long-duration mission behavioral health and performance factors. Aviat Space Environ Med. (2005)0.76:B42–51.
58. Kanas N, Sandal GM, Boyd JE, Gushin VI, Manzey D, North R, et al. “Psychology and culture during long-duration space missions,” in Vakoch DA, editor. On Orbit and Beyond: Psychological Perspectives on Human Spaceflight. Berlin; Heidelberg: Springer-Verlag Publishing (2013). p. 153–84. doi: 10.1007/978-3-642-30583-2_9
59. Nasrini J, Hermosillo E, Dinges DF, Moore TM, Gur RC, Basner M. Cognitive performance during confinement and sleep restriction in NASA's human exploration research analog (HERA). Front Phys. (2020) 11:394. doi: 10.3389/fphys.2020.00394
60. Palinkas LA, Suedfeld P. Psychosocial issues in isolated and confined extreme environments. Neurosci Biobehav Rev. (2021) 126:413–29. doi: 10.1016/j.neubiorev.2021.03.032
61. Burger AM, Van Diest I, van der Does W, Hysaj M, Thayer JF, Brosschot JF, et al. Transcutaneous vagus nerve stimulation and extinction of prepared fear: a conceptual non-replication. Sci Rep. (2018) 8:11471. doi: 10.1038/s41598-018-29561-w
62. Genheimer H, Andreatta M, Asan E, Pauli P. Reinstatement of contextual conditioned anxiety in virtual reality and the effects of transcutaneous vagus nerve stimulation in humans. Sci Rep. (2017) 7:17886. doi: 10.1038/s41598-017-18183-3
63. Wolf V, Kühnel A, Teckentrup V, Koenig J, Kroemer NB. Does transcutaneous auricular vagus nerve stimulation affect vagally mediated heart rate variability? a living and interactive Bayesian meta-analysis. Psychophysiology. (2021) 58:e13933. doi: 10.1111/psyp.13933
64. Redgrave J, Day D, Leung H, Laud PJ, Ali A, Lindert R, et al. Safety and tolerability of transcutaneous Vagus nerve stimulation in humans; a systematic review. Brain Stimul. (2018) 11:1225–38. doi: 10.1016/j.brs.2018.08.010
65. Thompson SL, O'Leary GH, Austelle CW, Gruber E, Kahn AT, Manett AJ, et al. A Review of parameter settings for invasive and non-invasive Vagus nerve stimulation (VNS) applied in neurological and psychiatric disorders. Front Neurol. (2021) 15:709436. doi: 10.3389/fnins.2021.709436
66. Usichenko TI, Hua K, Cummings M, Nowak A, Hahnenkamp K, Brinkhaus B, et al. Auricular stimulation for preoperative anxiety - a systematic review and meta-analysis of randomized controlled clinical trials. J Clin Anesth. (2022) 76:110581. doi: 10.1016/j.jclinane.2021.110581
67. Wang Y, Li SY, Wang D, Wu MZ, He JK, Zhang JL, et al. Transcutaneous auricular Vagus nerve stimulation: from concept to application. Neurosci Bull. (2021) 37:853–62. doi: 10.1007/s12264-020-00619-y
68. Yap J, Keatch C, Lambert E, Woods W, Stoddart PR, Kameneva T. Critical review of transcutaneous Vagus nerve stimulation: challenges for translation to clinical practice. Front Neurosci. (2020) 14:284. doi: 10.3389/fnins.2020.00284
69. Farmer AD, Strzelczyk A, Finisguerra A, Gourine AV, Gharabaghi A, Hasan A, et al. International consensus based review and recommendations for minimum reporting standards in research on transcutaneous Vagus nerve stimulation (version 2020). Front Hum Neurosci. (2021) 14:568051. doi: 10.3389/fnhum.2020.568051
70. Boujut A, Verty LV, Maltezos S, Lussier M, Mellah S, Bherer L, et al. Effects of computerized updating and inhibition training in older adults: the ACTOP three-arm randomized double-blind controlled trial. Front Neurol. (2020) 11:606873. doi: 10.3389/fneur.2020.606873
71. Brehmer Y, Westerberg H, Bäckman L. Working-memory training in younger and older adults: training gains, transfer, and maintenance. Front Hum Neurosci. (2012) 6:63. doi: 10.3389/fnhum.2012.00063
72. Chiu HL, Chu H, Tsai JC, Liu D, Chen YR, Yang HL, et al. The effect of cognitive-based training for the healthy older people: a meta-analysis of randomized controlled trials. PLoS ONE. (2017) 12:e0176742. doi: 10.1371/journal.pone.0176742
73. Lampit A, Hallock H, Valenzuela M. Computerized cognitive training in cognitively healthy older adults: a systematic review and meta-analysis of effect modifiers. PLoS Med. (2014) 11:e1001756. doi: 10.1371/journal.pmed.1001756
74. Macaulay TR, Pa J, Kutch JJ, Lane CJ, Duncan D, Yan L, et al. 12 weeks of strength training improves fluid cognition in older adults: a nonrandomized pilot trial. PLoS ONE. (2021) 16:e0255018. doi: 10.1371/journal.pone.0255018
75. Zigmond AS, Snaith RP. The hospital anxiety and depression scale. Acta Psychiatr Scand. (1983) 67:361–70. doi: 10.1111/j.1600-0447.1983.tb09716.x
76. Da Costa Silva L, Belrose C, Trousselard M, Rea B, Seery E, Verdonk C, et al. Self-reported body awareness: validation of the postural awareness scale and the multidimensional assessment of interoceptive awareness (version 2) in a non-clinical adult French-speaking sample. Front Psychol. (2022) 13:946271. doi: 10.3389/fpsyg.2022.946271
77. Lesage FX, Berjot S, Deschamps F. Psychometric properties of the French versions of the perceived stress scale. Int J Occup Med Environ Health. (2012) 25:178–84. doi: 10.2478/s13382-012-0024-8
78. Heart Heart rate variability: standards of measurement physiological interpretation and clinical use. task force of the European society of cardiology and the North American society of pacing and electrophysiology. Circulation. (1996) 93:1043–65.
79. Gomes P, Margaritoff P, Silva H. pyHRV: development and evaluation of an open-source python toolbox for heart rate variability (HRV). In: Proceedings of International Conference on Electrical, On Electrical, Electronic and Computing Engineering (Serbia: icetran). (2019), p. 822–8.
80. Carreiras C, Alves AP, Lourenço A, Canento F, Silva H, Fred A. BioSPPy: Biosignal Processing in Python. (2015). Available online at: https://github.com/PIA-Group/BioSPPy/
81. Ellrich J. Transcutaneous auricular vagus nerve stimulation. J Clin Neurophysiol. (2019) 36:437–42. doi: 10.1097/WNP.0000000000000576
82. Diamond A. Executive functions. Ann Rev Psychol. (2013) 64:135–68. doi: 10.1146/annurev-psych-113011-143750
83. Alderman BL, Olson RL. The relation of aerobic fitness to cognitive control and heart rate variability: a neurovisceral integration study. Biol Psychol. (2014) 99:26–33. doi: 10.1016/j.biopsycho.2014.02.007
84. Hansen AL, Johnsen BH, Thayer JF. Vagal influence on working memory and attention. Int J Psychophysiol. (2003) 48:263–74. doi: 10.1016/S0167-8760(03)00073-4
85. Borges U, Knops L, Laborde S, Klatt S, Raab M. Transcutaneous vagus nerve stimulation may enhance only specific aspects of the core executive functions. a randomized crossover trial. Front Neurosci. (2020) 14:523. doi: 10.3389/fnins.2020.00523
86. Thayer JF, Hansen AL, Saus-Rose E, Johnsen BH. Heart rate variability, prefrontal neural function, and cognitive performance: the neurovisceral integration perspective on self-regulation, adaptation, and health. Ann Behav Med. (2009) 37:141–53. doi: 10.1007/s12160-009-9101-z
87. Murray AR, Atkinson L, Mahadi MK, Deuchars SA, Deuchars J. The strange case of the ear and the heart: the auricular vagus nerve and its influence on cardiac control. Auton Neurosci. (2016) 199:48–53. doi: 10.1016/j.autneu.2016.06.004
88. Wu Y, Song L, Wang X, Li N, Zhan S, Rong P, et al. Transcutaneous vagus nerve stimulation could improve the effective rate on the quality of sleep in the treatment of primary insomnia: a randomized control trial. Brain Sci. (2022) 12:1296. doi: 10.3390/brainsci12101296
89. Zhang S, He JK, Meng H, Zhao B, Zhao YN, Wang Y, et al. Effects of transcutaneous auricular Vagus nerve stimulation on brain functional connectivity of medial prefrontal cortex in patients with primary insomnia. Anat Rec. (2021) 304:2426–35. doi: 10.1002/ar.24785
90. Rizzo P, Beelke M, De Carli F, Canovaro P, Nobili L, Robert A, et al. Chronic vagus nerve stimulation improves alertness and reduces rapid eye movement sleep in patients affected by refractory epilepsy. Sleep. (2003) 26:607–11. doi: 10.1093/sleep/26.5.607
91. Malow BA, Edwards J, Marzec M, Sagher O, Fromes G. Effects of vagus nerve stimulation on respiration during sleep: a pilot study. Neurology. (2000) 55:1450–4. doi: 10.1212/WNL.55.10.1450
92. Malow BA, Edwards J, Marzec M, Sagher O, Ross D, Fromes G. Vagus nerve stimulation reduces daytime sleepiness in epilepsy patients. Neurology. (2001) 57:879–84. doi: 10.1212/WNL.57.5.879
93. Van de Borne P, Nguyen H, Biston P, Linkowski P, Degaute JP. Effects of wake and sleep stages on the 24-h autonomic control of blood pressure and heart rate in recumbent men. Am J Physiol. (1994) 266:H548–54. doi: 10.1152/ajpheart.1994.266.2.H548
94. Somers VK, Dyken ME, Mark AL, Abboud FM. Sympathetic-nerve activity during sleep in normal subjects. N Engl J Med. (1993) 328:303–7. doi: 10.1056/NEJM199302043280502
95. Bräscher AK, Kleinböhl D, Hölzl R, Becker S. Differential classical conditioning of the nocebo effect: increasing heat-pain perception without verbal suggestions. Front Psychol. (2017) 8:2163. doi: 10.3389/fpsyg.2017.02163
96. Latremoliere A, Woolf CJ. Central sensitization: a generator of pain hypersensitivity by central neural plasticity. J Pain. (2009) 19:895–926. doi: 10.1016/j.jpain.2009.06.012
97. Shaffer F, Ginsberg JP. An overview of heart rate variability metrics and norms. Front Public Health. (2017) 5:258. doi: 10.3389/fpubh.2017.00258
98. Burger AM, Verkuil B, Van Diest I, Van der Does W, Thayer JF, Brosschot JF. The effects of transcutaneous vagus nerve stimulation on conditioned fear extinction in humans. Neurobiol Learn Mem. (2016) 132:49–56. doi: 10.1016/j.nlm.2016.05.007
99. Burger AM, Van der Does W, Thayer JF, Brosschot JF, Verkuil B. Transcutaneous vagus nerve stimulation reduces spontaneous but not induced negative thought intrusions in high worriers. Biol Psychol. (2019) 142:80–9. doi: 10.1016/j.biopsycho.2019.01.014
100. De Couck M, Cserjesi R, Caers R, Zijlstra WP, Widjaja D, Wolf N, et al. Effects of short and prolonged transcutaneous vagus nerve stimulation on heart rate variability in healthy subjects. Auton Neurosci. (2017) 203:88–96. doi: 10.1016/j.autneu.2016.11.003
101. Borges U, Laborde S, Raab M. Influence of transcutaneous vagus nerve stimulation on cardiac vagal activity: not different from sham stimulation and no effect of stimulation intensity. PLoS ONE. (2019) 14:e0223848. doi: 10.1371/journal.pone.0223848
102. Clancy JA, Mary DA, Witte KK, Greenwood JP, Deuchars SA, Deuchars J. Non-invasive vagus nerve stimulation in healthy humans reduces sympathetic nerve activity. Brain Stimul. (2014) 7:871–7. doi: 10.1016/j.brs.2014.07.031
103. Gurel NZ, Wittbrodt MT, Jung H, Ladd SL, Shah AJ, Vaccarino V, et al. Automatic detection of target engagement in transcutaneous cervical vagal nerve stimulation for traumatic stress triggers. IEEE J Biomed Health Inform. (2020) 24:1917–25. doi: 10.1109/JBHI.2020.2981116
104. Machetanz K, Berelidze L, Guggenberger R, Gharabaghi A. Brain-heart interaction during transcutaneous auricular vagus nerve stimulation. Front Neurosci. (2021) 15:632697. doi: 10.3389/fnins.2021.632697
105. Machetanz K, Berelidze L, Guggenberger R, Gharabaghi A. Transcutaneous auricular vagus nerve stimulation and heart rate variability: analysis of parameters and targets. Auton Neurosci. (2021) 236:102894. doi: 10.1016/j.autneu.2021.102894
106. Keute M, Machetanz K, Berelidze L, Guggenberger R, Gharabaghi A. Neuro-cardiac coupling predicts transcutaneous auricular vagus nerve stimulation effects. Brain Stimul. (2021) 14:209–16. doi: 10.1016/j.brs.2021.01.001
107. Villani V, Tsakiris M, Azevedo RT. Transcutaneous vagus nerve stimulation improves interoceptive accuracy. Neuropsychologia. (2019) 134:107201. doi: 10.1016/j.neuropsychologia.2019.107201
108. Mehling WE, Acree M, Stewart A, Silas J, Jones A. The multidimensional assessment of interoceptive awareness, version 2 (MAIA-2). PLoS ONE. (2018) 13:e0208034. doi: 10.1371/journal.pone.0208034
109. McIntire LK, McKinley RA, Goodyear C, McIntire JP, Brown RD. Cervical transcutaneous vagal nerve stimulation (ctVNS) improves human cognitive performance under sleep deprivation stress. Commun Biol. (2021) 4:634. doi: 10.1038/s42003-021-02145-7
110. Suedfeld P. Applying positive psychology in the study of extreme environments. Hum Perf Extrem Environ. (2001) 6:21–5. doi: 10.7771/2327-2937.1020
111. Kanas N. Psychosocial value of space simulation for extended spaceflight. Adv Space Biol Med. (1997) 6:81–91. doi: 10.1016/S1569-2574(08)60078-7
112. Basner M, Savitt A, Moore TM, Port AM, McGuire S, Ecker AJ, et al. Development and validation of the cognition test battery for spaceflight. Aerosp Med Hum Perform. (2015) 86:942–52. doi: 10.3357/AMHP.4343.2015
113. Frantzidis CA, Kontana E, Karkala A, Nigdelis V, Karagianni M, Nday CM, et al. Current trends and future perspectives of space neuroscience towards preparation for interplanetary missions. Neurol India. (2019) 67:S182–7. doi: 10.4103/0028-3886.259124
114. Palinkas LA. Going to extremes: the cultural context of stress, illness and coping in Antarctica. Soc Sci Med. (1992) 35:651–64. doi: 10.1016/0277-9536(92)90004-A
115. Weybrew BB. Periods of submergence completely submerged. Project NM23 00 00, Task NM 23 02 20, Report 281, U. S. Naval Medical Research Laboratory, Conn (1957).
116. Baisden DL, Beven GE, Campbell MR, Charles JB, Dervay JP, Foster E, et al. Human health and performance for long-duration spaceflight. Aviat Space Environ Med. (2008) 79:629–35. doi: 10.3357/ASEM.2314.2008
117. Manier O, Colas M-D. La conquête de la planète Mars: enjeux médico-psychologiques et éthiques. Annales Medico Psychologiques. (2016) 174:770–3. doi: 10.1016/j.amp.2016.08.008
118. Martin-Krumm C, Lefranc B, Moelo A, Poupon C, Pontis J, Vannier A, et al. Is regular physical activity practice during a submarine patrol an efficient coping strategy? Front Psychiatry. (2021) 12:704981. doi: 10.3389/fpsyt.2021.704981
Keywords: adaptation, cognition, countermeasure, health, stress, taVNS
Citation: Le Roy B, Martin-Krumm C, Gille A, Jacob S, Vigier C, Laborde S, Claverie D, Besnard S and Trousselard M (2023) Evaluation of taVNS for extreme environments: an exploration study of health benefits and stress operationality. Front. Neurol. 14:1286919. doi: 10.3389/fneur.2023.1286919
Received: 31 August 2023; Accepted: 10 October 2023;
Published: 22 November 2023.
Edited by:
Emmanuel Sagui, Hôpital Européen Marseille, FranceReviewed by:
Mireille Michel-Cherqui, Hôpital Foch, FranceWeiming Sun, First Affiliated Hospital of Nanchang University, China
Copyright © 2023 Le Roy, Martin-Krumm, Gille, Jacob, Vigier, Laborde, Claverie, Besnard and Trousselard. This is an open-access article distributed under the terms of the Creative Commons Attribution License (CC BY). The use, distribution or reproduction in other forums is permitted, provided the original author(s) and the copyright owner(s) are credited and that the original publication in this journal is cited, in accordance with accepted academic practice. No use, distribution or reproduction is permitted which does not comply with these terms.
*Correspondence: Barbara Le Roy, YmFyYmFyYS5tLmxlLnJveSYjeDAwMDQwO2dtYWlsLmNvbQ==