- Department of Neurology, The First Hospital of Jilin University, Changchun, China
Skeletal muscle disorders are mostly genetic and include several rare diseases. With disease progression, muscle fibrosis and adiposis occur, resulting in limited mobility. The long course of these diseases combined with limited treatment options affect patients both psychologically and economically, hence the development of novel treatments for neuromuscular diseases is crucial to obtain a better quality of life. As a widely used hypoglycemic drug in clinical practice, metformin not only has anti-inflammatory, autophagy-regulating, and mitochondrial biogenesis-regulating effects, but it has also been reported to improve the symptoms of neuromuscular diseases, delay hypokinesia, and regulate skeletal muscle mass. However, metformin’s specific mechanism of action in neuromuscular diseases requires further elucidation. This review summarizes the evidence showing that metformin can regulate inflammation, autophagy, and mitochondrial biogenesis through different pathways, and further explores its mechanism of action in Duchenne muscular dystrophy, statin-associated muscle disorders, and age-related sarcopenia. This review clarifies the directions of future research on therapy for neuromuscular diseases.
1. Introduction
Skeletal muscle disorders are predominantly genetic in origin, among which are a number of rare diseases. The long disease courses and limited treatment methods greatly compromise patients’ quality of life, causing a heavy psychological burden. The economic pressure on families and society is also incalculable. Duchenne muscular dystrophy (DMD) is caused by a genetic defect leading to impaired dystrophin synthesis in muscle. As the disease progresses, patients’ mobility becomes severely limited, ultimately resulting in respiratory and circulatory failure (1). Furthermore, current treatment options for age-related muscle diseases and statin-associated muscle symptoms (SAMS) are unclear. Therefore, finding new treatment targets to alleviate the development process of these neuromuscular diseases and alleviate patient pain is crucial.
Metformin, a widely used hypoglycemic drug in clinical practice, originated from the traditional European herb, Galega officinalis, which was initially used to treat thirst and frequent urination. However, the side effects owing to lactic acidosis caused by other guanidines delayed its clinical usage (2, 3). Metformin was introduced into clinical practice following research evaluating its tolerance and long-term safety (3). Metformin inhibits gluconeogenesis through different pathways to lower blood glucose (4–6). Interestingly, along with its hypoglycemic effect, metformin can also improve skeletal muscle mass and strength through different mechanisms, thereby delaying disease progression. For example, it can exert its effects via regulating oxidative stress, calcium homeostasis, the expression of inflammatory factors, and improving mitochondrial dysfunction, to enhance the quality, strength, and regenerative ability of skeletal muscles.
Several studies have verified that metformin can improve the symptoms of neuromuscular diseases. The combined treatment of L-arginine and metformin has a beneficial impact on the decline of motor function and muscle degeneration of 7- to 10-year-old boys with DMD, and can delay disease progression (7). The combination of metformin and statins can also reduce statin-induced muscle spasms and pain symptoms (8). Furthermore, another study demonstrated that combinatorial treatment with metformin and leucine could improve muscle quality during aging (9). Moreover, our study found that metformin can restore damaged autophagic flux, enhance the vitality of fibroblasts from patients with UDP-N-acetylglucosamine 2-epimerase/N-acetylmannosamine kinase (GNE) myopathy, reduce the rate of apoptosis, and thus play a protective role in GNE myopathy (10). Due to the limited treatment methods available for skeletal muscle diseases, the above mentioned positive effects of metformin for their treatment is encouraging. Therefore, we summarized the mechanism of action of metformin in neuromuscular diseases to provide a useful resource for directing future research.
2. Pharmacokinetics of metformin
Metformin is a hydrophilic compound with a positive charge at physiological pH. The oral absorption, tissue distribution, and renal excretion of metformin are dependent on drug transporters. Metformin is a substrate for many organic cation transport proteins, such as OCTN1, OCTN2, PMAT, and multidrug and toxin extrusion transporters. OCT1 is the major transporter responsible for metformin uptake in the liver (11–13), wherein metformin is absorbed within 6 h after dosing, with a 50%–60% bioavailability. Approximately 70% of the dose is absorbed from the small intestine, while the rest enters the colon and is excreted in feces. Metformin is excreted via urine in its original form, with a half-life of approximately 5 h (14). The concentration of metformin accumulated in the jejunum is 30–300 times higher than that in plasma. The side effects reported at present mainly include gastrointestinal discomfort (including abdominal distension, abdominal pain, diarrhea or stomach pain, early satiety, loss of appetite, and nausea), but these side effects usually subside after 1–2 weeks of use (15). Other studies have found that some people taking metformin develop severe vitamin B12 deficiency, especially those over 80 years of age who are prone to this condition (16). This may be because metformin interferes with the absorption of vitamin B12 in the intestine and affects the balance of intestinal microorganisms (17). People with normal renal function generally have good tolerance to this drug; however, those with severe kidney, liver, or heart diseases might suffer from lactic acidosis (18).
3. Mechanism of action of metformin
3.1. Metformin and inflammation
Previous studies have illustrated the anti-inflammatory effects of metformin across animal models of disease and in human studies (Figure 1). Among them, nuclear factor kappa-light-chain-enhancer of activated B cells (NF-κB) plays an important role in the mediation of inflammation. NF-κB is a transcription factor composed of RelA (p65), c-Rel, RelB, NF-κB1 (p50), and NF-κB2 (p52); it has a significant impact on the regulation of cell adhesion, cytokine production, apoptosis, cell growth, and barrier function (19). NF-κB mediates the activation of inflammatory bodies, and its release can act as a trigger for the inflammatory cascade response (20). Its hyperactivation is associated with inflammation. Amp-activated protein kinase (AMPK) signaling can inhibit the inflammatory response induced by the NF-κB system (21). AMPK, as a serine/threonine protein kinase, plays a key role in energy metabolism and consists of three subunits: α subunits (α1, α2), regulatory β subunits (β1, β2), and γ subunits (γ1, γ2, γ3) (22). AMPK is targeted in a variety of inflammatory disease models, and metformin can inhibit the inflammatory response through AMPK-dependent and -independent pathways.
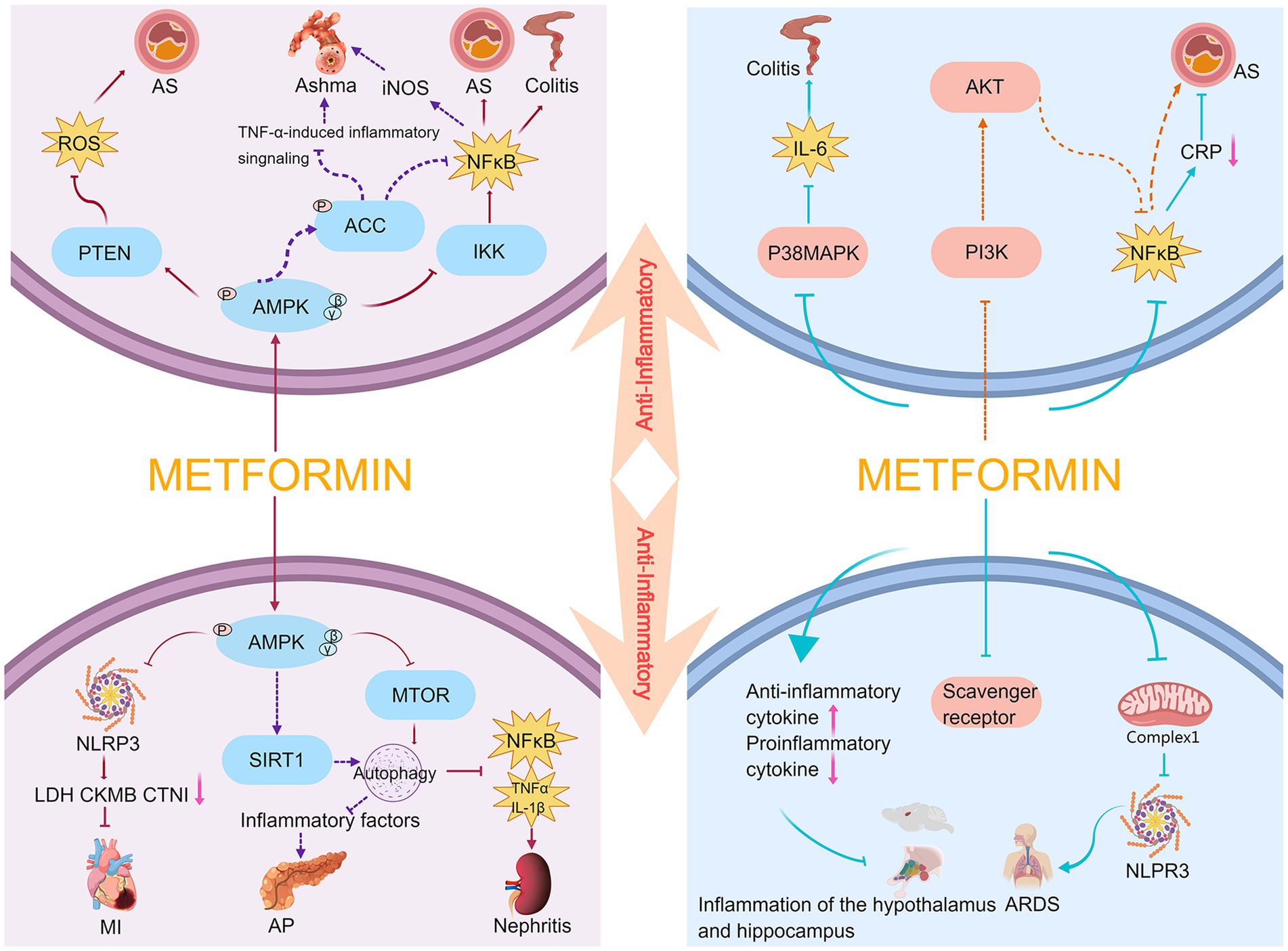
Figure 1. Anti-inflammatory mechanism of metformin. AS, atherosclerosis; AP, acute pancreatitis; MI, myocardial infarction.
Macrophages are the body’s first line of defense against external pathogens and are crucial for triggering inflammation. To trigger the body’s defense response, macrophages secrete cytokines, chemokines, and growth factors; however, overproduction of these factors causes tissue damage and chronic inflammation. It has been reported that lipopolysaccharide induces an increase in chemokines in RAW264.7 cells (a mouse macrophage cell line), and metformin can inhibit NF-κB signaling by activating AMPK to exert its anti-inflammatory effects (23). Hattori et al. found that metformin activates AMPK and inhibits IκB kinase (IKK) activity to suppress NF-κB activation, thereby reducing the progression of vasculitis or atherosclerosis (24). Koh et al. reported that metformin may attenuate intestinal inflammation by inhibiting IKK/NF-κB signaling activation through AMPK (25). Park et al. and Calixto et al. also concluded that metformin significantly inhibits eosinophil inflammation and improves airway remodeling. These studies showed that metformin increases the levels of phosphorylated AMPK and its downstream target acetyl CoA carboxylase (ACC), and inhibits tumor necrosis factor-α (TNF-α)-induced inflammatory signaling and NF-κB-mediated inducible nitric oxide synthase expression in the lung tissue of obese mice (26, 27). Sun et al. found that metformin can regulate the autophagy and subsequent downregulation of inflammatory cytokines (including interleukin (IL)-1β and TNF-α), NF-κB expression, and ROS-induced apoptosis in diabetic/dyslipidemic (db/db) mice via the AMPK/mTOR signaling pathway to attenuate the renal inflammatory response and ameliorate abnormal renal pathology (28). Phosphatase and tensin homolog (PTEN) is a lipid and protein phosphatase whose attenuation promotes the inflammatory response. Kim et al. recently reported that metformin attenuates vasculitis and delays atherosclerosis development by negatively regulating ROS production through the AMPK/PTEN pathway (22).
The Nod-like receptor protein 3 (NLRP3) inflammasome comprises the sensor NLRP3, the adaptor protein ASC (apoptosis-associated speck-like protein containing a caspase recruitment domain), and the effector pro-caspase-1. The expression of critical components of the inflammasome and associated cytokines is upregulated by transcriptional activation, turning on the first step in the activation of the NLRP3 inflammasome (29). Recent studies have indicated that AMPK plays a key role in mediating the activation of the NLRP3 inflammasome (20, 30). Zhang et al. demonstrated that metformin exerts protective effects in a model of myocardial ischemia–reperfusion injury by reducing lactose dehydrogenase, creatine kinase MB, and cardiac troponin I through AMPK/NLRP3 and reducing myocardial inflammatory cell infiltration, myocardial collagen deposition, and the scope of myocardial infarction (31). Sirtuin 1 (SIRT1) exists in the nucleus and cytoplasm, and has nicotinamide adenine dinucleotide-dependent protein deacetylase activity (32). It has regulatory roles in various physiological functions, such as the cell cycle, cell metabolism, autophagy, mitochondrial function, oxidative stress, DNA repair, and inflammation. SIRT1 plays an important role when the body is under hypoxic or stressful conditions (33); further, it can be positively regulated by AMPK (34). In experimental acute pancreatitis models, both in vivo and in vitro experiments demonstrated that metformin restored impaired autophagy by upregulating SIRT1 expression mainly through AMPK activation, thereby reducing the release of inflammatory factors (35).
Overall, metformin can exert protective effects in atherosclerotic thrombotic disease (22, 24), intestinal inflammation (25), cardiovascular disease (31), asthma (26, 27), acute pancreatitis (35), and diabetic nephropathy (28) upon acting on the AMPK/NF-κB, AMPK/PTEN, AMPK/NLRP3, and AMPK/SIRT1 pathways. Metformin also inhibits inflammatory responses through AMPK-independent pathways. It inhibits the phosphorylation and nuclear translocation of the NF-κB subunit, p65, and inhibits the degradation of its inhibitory protein, IκB, resulting in NF-κB being isolated in the cytoplasm and unable to translocate to the nucleus to participate in the induction of inflammatory responses (36). In an atherosclerotic rabbit model, Li et al. demonstrated that metformin inhibits IκB phosphorylation and NF-κB activation, reduces serum C-reactive protein levels, and exerts anti-vascular inflammatory effects (37). Isoda et al. found that metformin ameliorates vasculitis by blocking NF-κB pro-inflammatory signaling through the PI3-K/Akt signaling pathway (38). Another study found that metformin inhibits the activation of the NLRP3 inflammasome and attenuates acute respiratory distress syndrome by targeting the electron transport chain complex 1 independent of the AMPK or NF-kB pathways (39).
In addition to the mechanisms described above, studies have reported that macrophages express several types of scavenger receptors which play an essential role in the inflammatory response. The interaction between scavenger receptors and oxidized low-density lipoprotein enhances NF-κB activity and produces pro-inflammatory cytokine production. Hyun et al. found that metformin downregulates the expression of scavenger receptors, CD36 and SR-A, to attenuate the inflammatory response in type 2 diabetic mice (40). Furthermore, Di Fusco et al. used metformin to treat mice with colitis and found that intestinal inflammation in mice was alleviated and accompanied by the activation of AMPK. However, neither activation nor the inhibition of AMPK expression affected the therapeutic effect of metformin on inflammation. Therefore, by exploring different pathways of metformin’s control on inflammation, they concluded that metformin could downregulate the activation of p38 MAP kinase in these mice through an AMPK-independent pathway and inhibit IL-6 expression (41).
Furthermore, cytokines are classified as either pro-inflammatory or anti-inflammatory. Pro-inflammatory cytokines, including IL-1, IL-6, IL-8, IL-12, TNF-α, and interferon, are secreted by Th1 cells, CD4+ T cells, macrophages, and dendritic cells. The key pro-inflammatory cytokines are IL-1, IL-6, and TNF-α. Anti-inflammatory cytokines include IL-4, IL-10, IL-11, IL-13, the IL-1 receptor antagonist, and TGF-β. The dynamic balance between pro- and anti-inflammatory cytokines plays a pivotal role in regulating inflammation (42). Additionally, Hammad et al. found that metformin decreased the relative mRNA expression of the pro-inflammatory factors IL-1β and IL-6, and increased the relative mRNA expression of the anti-inflammatory factor IL-10 in the hypothalamus and hippocampus, thereby decreasing inflammation in a rat model of oxandrolone-induced depressive-like behavior (43).
In summary, metformin can delay the progression of diseases such as vasculitis (37, 38), acute respiratory distress syndrome (39), type 2 diabetes-induced inflammatory response (40), colitis (41), and central nervous system inflammation (43) by blocking NF-κB inflammatory signaling, inhibiting NLRP3 inflammasome activation, suppressing the expression of scavenger receptors, and downregulating the expression of pro-inflammatory factors.
3.2. Metformin and autophagy
Autophagy, which was first proposed by the Belgian scientist, Christian de Duve, is one of the most investigated topics in cell biology. Also known as programmed cell death type II, the autophagic process is ubiquitous in nature, and is widely recognized as a cytoprotective mechanism (44). There are three main types of autophagy based on modes of cellular material transport to lysosomes (45, 46): (i) macroautophagy, (ii) microautophagy, and (iii) chaperone-mediated autophagy. We usually refer to macroautophagy as autophagy. The process of autophagy is divided into three stages: the formation of phagophores or isolation membranes, autophagosomes, and autolysosomes (47–49).
The formation of an autophagosome is a hallmark of autophagy initiation. Unc-51-like kinase 1 (ULK1), a molecule with serine/threonine kinase activity, is important in the formation of autophagosomes. When ULK1 is phosphorylated or ubiquitinated, it exerts an autophagy-inducing effect. During autophagy, Beclin-1 is involved in autophagosome membrane formation and material transport. ULK1 can phosphorylate Beclin-1 and VPS34; the activation of these targets is important for autophagy initiation. During autophagosome formation, microtubule-associated protein 1A/1B-light chain 3 (LC3) is processed into the soluble form, LC3-I. Then, LC3-I binds to phosphatidylethanolamine (PE) on the surface of the autophagosome membrane, becoming membrane bound LC3-II. Protein Sequestosome 1 (P62/SQSTM1) is expressed in a variety of cells and tissues, which can connect LC3 and its ubiquitination substrate as it integrates into an autophagosome and is degraded by autophagic lysosomes (50, 51). Autophagy is a dynamic process, and analyzing the expression of LC3 and P62 proteins can help us understand whether the flux of the autophagy is obstructed or not.
Under physiological conditions, autophagy remains low to maintain homeostasis. Hypoxia, nutrient deficiency, exposure to ROS, pathogen invasion, organelle damage, and excessive accumulation of abnormal proteins enhance autophagic activity (52). The process of autophagy involves several known and unknown signaling pathways. Metformin plays a vital role in regulating autophagy in many diseases (Figure 2), including polycystic ovary syndrome (PCOS) (53), tumors (54), cognitive disorders (55, 56), osteoarthritis (57), non-alcoholic fatty liver (58), hepatic steatosis (59), diabetic nephropathy (60), diabetic cardiomyopathy (61), and abdominal aortic aneurysm (62).
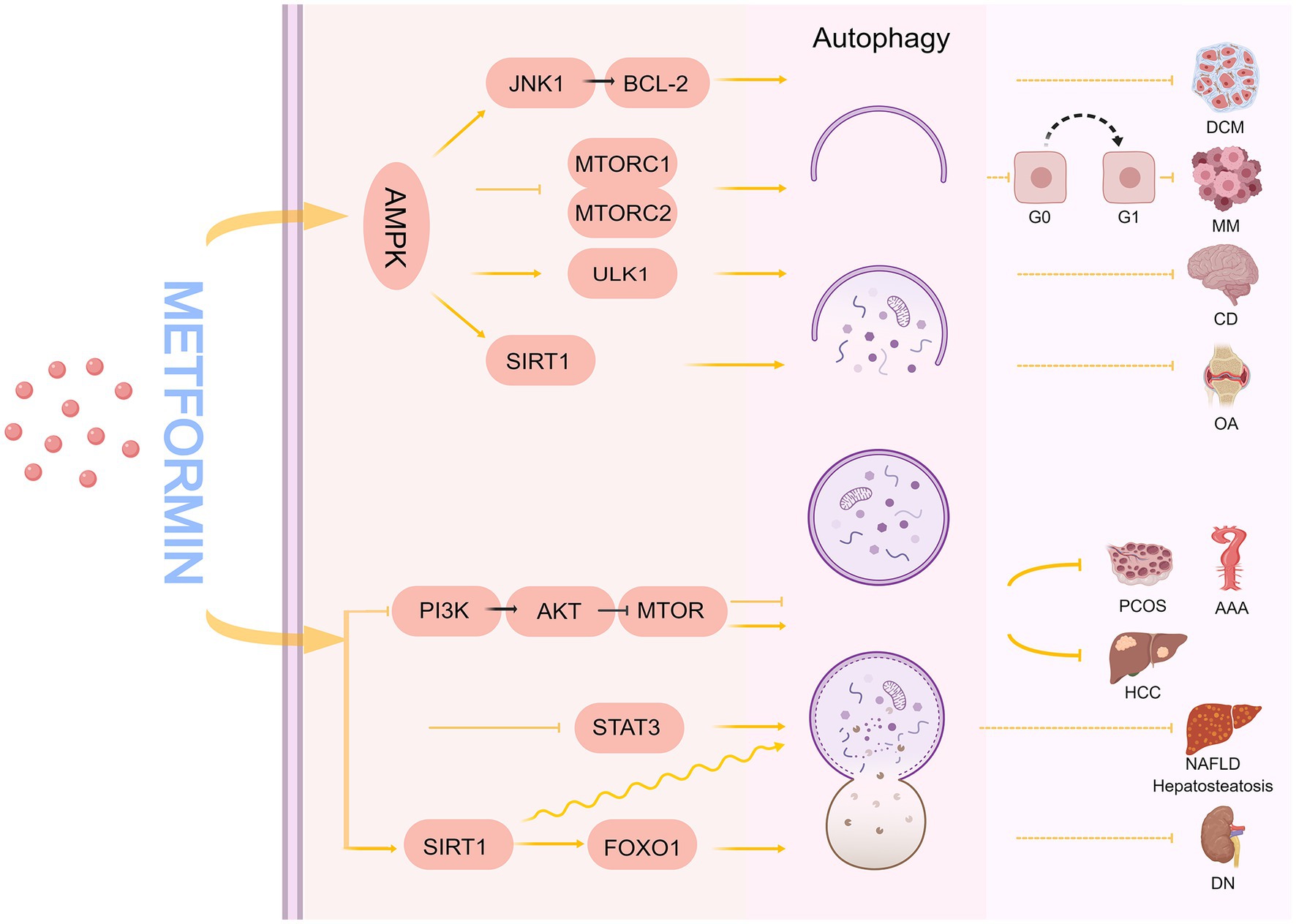
Figure 2. The mechanism of metformin regulating autophagy. DCM, diabetic cardiomyopathy; MM, multiple myeloma; CD, cognitive disorder; OA, osteoarthritis; HCC, hepatocellular carcinoma; PCOS, polycystic ovary syndrome; AAA, abdominal aortic aneurysm; NAFLD, non-alcoholic fatty liver disease; DN, diabetic nephropathy.
Many studies have demonstrated that metformin can regulate the autophagic process by relying on AMPK pathways, such as AMPK/mTOR, AMPK/ULK1, AMPK/SIRT1, and AMPK/JNK1/BCL-2. mTOR is an atypical serine/threonine protein kinase that plays a gateway role in autophagy (63); when mTOR is stimulated by nutrient, energy, and stress signals, it can promote or inhibit mTORC1/mTORC2 complex formation, which in turn regulates autophagy. In cancer, autophagy can act as a tumor suppressor (64, 65) or promoter (66) in different contexts and stages of cancer development. Wang et al. showed that metformin, as a potential specific dual mTORC1/2 inhibitor, downregulates p-mTOR (Se-2448), p-P70 S6K, p-4 EBP1, and p-AKT (Ser 473) expression through AMPK activation-mediated mTORC1/mTORC2 inhibition, induces autophagy, and causes cell cycle arrest in the G0/G1 phase of myeloma cells, which ultimately inhibits the growth of myeloma cells (54).
Autophagy regulates neuronal homeostasis in the central nervous system (67, 68). Defective autophagy may be a key driver of neurodegeneration, ultimately leading to neurocognitive impairment (69, 70). Li et al. found that 1.5% isoflurane caused impaired autophagy in the hippocampus of aged rats in a time-dependent manner, affecting spatial learning and memory (71). Mounting evidence indicates that autophagy upregulation has neuroprotective effects (55, 69). Zhu et al. found that 20-month-old mice (analogous to elderly humans) developed spatial learning and memory deficits with reduced autophagic activity when exposed to 3% sevoflurane for 2 h, and that autophagy deficits may have led to reduced microtubule-associated protein 2 (MAP2) and postsynaptic density protein 95 (PSD95) levels, as well as reduced synaptic density. Interestingly, another study found that metformin increased phosphorylation of AMPK (Thr172), which in turn caused increased phosphorylation of ULK1 (Ser317 and Ser777) and upregulated autophagic activity to protect against sevoflurane-induced synaptic decline and neurocognitive deficits, suggesting that metformin is useful in preventing or treating anesthesia-induced cognitive deficits in mice (55, 56).
SIRT1, a nicotinamide adenine dinucleotide-dependent deacetylase, is a key regulator of energy metabolism that significantly affects metabolism, inflammation, and autophagy regulation (72–74). It has been demonstrated that metformin can promote chondrocyte autophagy and reduce chondrocyte apoptosis through the AMPKα2/SIRT1 pathway to alleviate osteoarthritis, and the protective effect of metformin is reversed by silencing AMPKα2 or blocking SIRT1 expression (57). Furthermore, Beclin-1 binds to Vps34 to form a PI3K-Beclin-1 complex involved in the formation of the autophagic pre-membrane, and a growing body of data supports the hypothesis that Bcl-2 inhibits autophagy membrane generation by binding Beclin-1. Consequently, interference with Bcl-2 may result in the dissociation of Bcl 2/Beclin1, which will lead to increased cellular autophagy (75). He et al. found that metformin can activate AMPK to stimulate the JNK1-BCL2 signaling pathway, cause Bcl-2 and Beclin-1 dissociation, restore autophagy, and alleviate diabetic cardiomyopathy (61).
In addition to the AMPK pathway mentioned above, metformin can also regulate autophagy through AMPK-independent pathways. Among them, the PI3K/AKT/mTOR signaling pathway is associated with various functions such as cell proliferation, differentiation, metabolism, apoptosis, and autophagy (76, 77). Sun et al. found that aloin and metformin alone or in combination can induce apoptosis and autophagy, inhibit the growth and invasion of hepatocellular carcinoma, and enhance anti-tumor effects through the PI3K/AKT/mTOR pathway (78). The PI3K/AKT/mTOR signaling pathway can regulate autophagy in both directions (79, 80). One study demonstrated that in an angiotensin II (Ang-II)-induced ApoE−/− mouse abdominal aortic aneurysm model, metformin inhibited PI3K/AKT/mTOR pathway activation, and reduced Ang-II-induced autophagy-related protein levels to inhibit aortic dissection, thus, preserving aortic elastin structure and reducing collagen loss and aortic cell apoptosis (62). In PCOS, oxidative stress levels are significantly elevated, leading to the excessive activation of autophagy, while metformin reduces the excessive autophagy in ovarian granulosa cells through PI3K/AKT/mTOR targeting to ameliorate PCOS symptoms (53). In addition, metformin inhibits STAT3 mRNA and protein expression, activates autophagy, and reduces the production of inflammatory cytokines in non-alcoholic steatohepatitis disease models (58). Evidence also supports that metformin can regulate autophagy through an AMPK-independent pathway. For example, Song et al. found that SIRT1 and autophagy were significantly downregulated in the livers of ob/ob (obese) mice, and in a hepatocyte model induced by oleic acid and high glucose, metformin increased SIRT1 expression and induced autophagy through an AMPK-independent pathway to reduce hepatosteatosis in ob/ob mice (59). Xu et al. constructed a diabetic rat model through a high-fat diet combined with an intraperitoneal injection of streptozotocin. After 8 weeks of intraperitoneal metformin injections, they found that the glomerular structure and renal function of diabetic rats were significantly improved, and the primary mechanism was that metformin regulated the SIRT1/FOXO1 autophagic signaling axis to induce renal autophagy (60).
Vacuolar protein sorting 15 (VPS15), which is one of the components of the phosphatidylinositol 3-kinase (P13K) complex, contributes to the generation of autophagosomes and endosomes. In patients with Danon disease and glycogen storage disease type II, it was found that VPS15 expression is elevated (81), indicating autophagy disorders (81, 82). Nascimbeni et al. considered that the effects of early use of short to long term Enzyme Replacement Therapy can help restore autophagic flux, enhance skeletal muscle mass, and enhance acid α-glucosidase enzyme activity (83). Therefore, it is speculated that metformin may be helpful in restoring autophagic flux in this disease. However, further research is needed to confirm these findings.
3.3. Metformin promotes mitochondrial biogenesis
Mitochondrial biogenesis is the intracellular synthesis of new healthy mitochondria, which can be activated by different signals under stressful conditions (84, 85). Mitochondrial biogenesis is related to cell division, oxidative stimulation, increased cellular energy demand, and exercise training. Some diseases and other factors can also affect mitochondrial biogenesis, including the pathogenesis of Huntington’s, Alzheimer’s, and Parkinson’s disease (86, 87).
AMPK is considered a key regulator in initiating mitochondrial biogenesis (88). Cells can regulate mitochondrial biogenesis through Peroxisome proliferator-activated receptor-γ co-activator-1α (PGC-1α), estrogen receptors, and transcription factors. PGC-1α is a major regulator of mitochondrial biogenesis. AMPK stimulates PGC-1α phosphorylation, which triggers the expression of transcription factors involved in mitochondrial biogenesis (nuclear respiratory factor (NRF)-1 and NRF-2) and mitochondrial transcription factor A (TFAM) (89, 90), thereby promoting mitochondrial DNA replication and transcription (Figure 3). The levels of PGC-1α, NRF-1, NRF-2, and TFAM proteins were reduced in a mouse model of peritoneal fibrosis associated with peritoneal dialysis, indicating significant inhibition of mitochondrial biosynthesis. Metformin may partially act on the AMPK/PGC-1α pathway to increase mitochondrial DNA content, improve mitochondrial morphology, and enhance mitochondrial biosynthesis to reduce peritoneal dialysis related peritoneal fibrosis in mice (91).
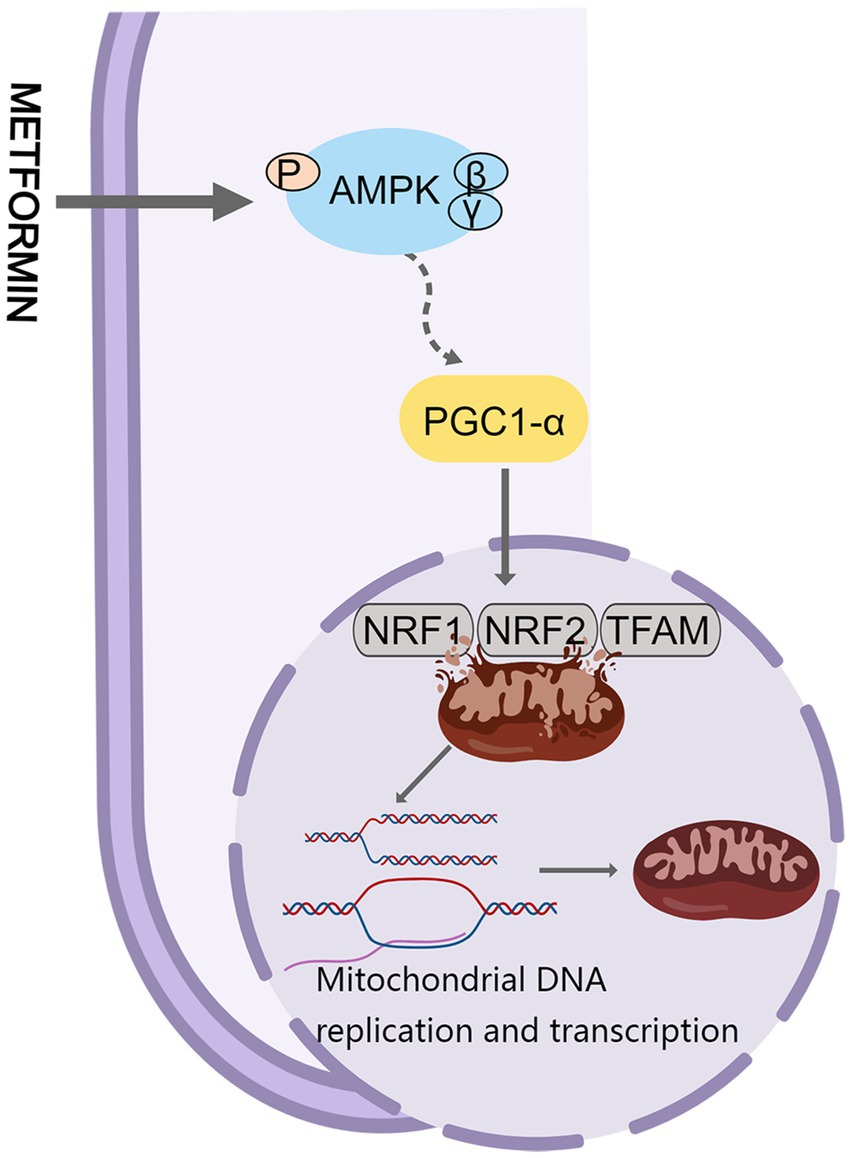
Figure 3. Metformin and mitochondrial biogenesis. PGC-1α, Peroxisome proliferator-activated receptor-γ co-activator-1α.
In the central nervous system, neuronal survival is compromised when mitochondria are damaged (92). The function or expression of PGC-1α is impaired to different degrees in degenerative diseases. Remarkably, overexpression of PGC-1α reduces mitochondrial damage and improves biogenesis (93). Khang et al. found that metformin exerts neuroprotective effects against Parkinson’s disease by reversing the reduction of Parkin in the substantia nigra and the accumulation of parkin-interacting substrate, and by downregulating the expression of PGC-1α in db/db mice and mice fed a high-fat diet (94). Metformin markedly improves aging-induced cardiomyocyte dysfunction (95). Gundewar et al. found that administration of 125 mg/kg metformin to a mouse model of heart failure resulted in AMPK activation, increased PGC-1α expression, and increased phosphorylation of endothelial nitric oxide synthase, which in turn improved mitochondrial function and significantly reduced ventricular dysfunction and improved survival (96). To sum up, metformin may have therapeutic potential in treating peritoneal fibrosis, neurodegenerative diseases, and cardiovascular diseases by modulating the quality of the mitochondria.
4. Metformin and skeletal muscle disorders
4.1. DMD
4.1.1. Clinical manifestations of DMD
Mutations in the Dystrophin gene result in dystrophinopathies, such as DMD, Becker muscular dystrophy, and others. Approximately 75% of Dystrophin gene mutations are intragene deletions (65%) or duplications (10%), with the remaining 25% being nucleotide variants (97). One of the most severe phenotypes is DMD, an X-linked recessive neuromuscular disorder. About 1 in 5,000 people suffer from DMD (97). Muscle fiber cells rely on dystrophin to maintain their functionality by linking the intracellular cytoskeleton with the transmembrane components of the dystrophin-glycoprotein complex (98). In the absence of dystrophin, the cytoskeleton and sarcolemma fail to connect properly, resulting in damage during muscle contraction, leading to chronic inflammation which impairs muscle fiber myogenesis and regeneration (99, 100). In DMD, delayed milestones in motor development during early childhood at the age of 4–5 years, typical Gowers syndrome, limited mobility, and pseudohypertrophy of calf muscles can occur (101). As the disease progresses, abnormal posture and gait may develop (102). Myocardial involvement develops in severe cases. Over time, it may even progress into dilated cardiomyopathy (1). Ultimately, death occurs by 20–30 years of age owing to respiratory and circulatory failure (103). Glucocorticoid therapy would significantly delay the decline of muscle strength and function, however, side effects such as obesity, cushingoid facies, and osteoporosis should be considered. In addition, deflazacort, Debio-025, and vamorolone can also improve the symptoms of DMD to varying degrees. Angiotensin-converting enzyme inhibitors, angiotensin receptor blockers, and β-blockers are the first choice for patients with cardiac involvement. The gene therapy strategies for DMD include gene transfer, exon reframing, exon skipping, exon reconstruction, and CRISPR gene editing. The latter three as personalized treatment options are beneficial for only a small number of patients (97).
4.1.2. Mechanism of metformin in the treatment of DMD
A clinical study showed that combined treatment with L-arginine and metformin positively affected hypokinesia and muscle degeneration in boys aged 7–10 years with DMD (7). Ljubicic et al. demonstrated that 42 days of metformin treatment of mdx mice resulted in increased expression of utrophin A, a compensatory functional target for the lack of dystrophin (104). The neuromuscular junction (NMJ), which connects motor neurons to skeletal muscle fibers, plays a crucial role in controlling muscle contractions (1). Fragmentation of NMJs has been observed in DMD patients and mdx mouse models (105). A previous study suggested that the NMJ in mdx mice show decreased levels of muscle specific kinase (MuSK) (106); this deficiency results in abnormal NMJ morphology in mdx mice as it plays an important role in acetylcholine receptor aggregation. The reduction of MuSK in mdx mice disrupts the normal latticed network of microtubules, potentially causing disruption of the resting NMJ structure. Dong et al. discovered that metformin increased muscle strength and improved muscle membrane integrity and NMJ transmission in mdx mice (1). All these studies demonstrate that metformin is a highly potent drug for treating DMD.
AMPK is essential for the normal tilting process of macrophages, which is required for normal muscle regeneration; it further plays an invaluable role in maintaining and remodeling skeletal muscle (107). For example, in myopathies caused by mitochondrial diseases, AMPK activator (AICAR)-induced AMPK activation notably attenuated the pathology, mainly by promoting muscle regeneration (108). Apart from this, Thomase et al. found that double knockout of AMPKβ1 and AMPKβ2 in mice produced a myopathic phenotype with a marked increase in the number of myofibers in the central nucleus, an abnormal distribution of myofibers, and many opaque fibers (109). Wang et al. argue that deletion of the AMPKα1/2 subunit decreases mitochondrial density and membrane potential. Further, using metformin in primary hepatocytes prepared from AMPKα1/2-knockout mice did not improve the mitochondrial membrane potential or density, suggesting that metformin increases mitochondrial density through an AMPK-dependent pathway (110). Metformin activates AMPK primarily by inhibiting mitochondrial electron transport chain complex I and regulating AMP/ATP and/or ADP/ATP ratios, which regulates mitochondrial biogenesis and function (111). The activation of AMPK plays a crucial role in maintaining skeletal muscle function. AMPK activity and phosphorylated AMPK levels in skeletal muscle were increased by approximately 80% from baseline levels in humans taking metformin (2 g/day) for approximately 10 weeks (112).
Ca2+, the main regulator and signaling molecule in skeletal muscle, acts as a messenger in processes ranging from contraction activation to myocyte degradation. A sophisticated system of controlling Ca2+ concentration in the cytoplasm exists in skeletal muscle (113). Muscle calcium overload usually occurs in myodystrophy, at which point calpain is activated, leading to the proteolytic hydrolysis of cellular components (114). Cardiotoxins initiate muscle damage, inflammation, and myofilament destruction, similar to the pathology of myodystrophy (115). Through in vitro and in vivo experiments, Langone et al. demonstrated that metformin most likely reduces muscle necrosis by negatively regulating the inward flow of Ca2+ (114).
In DMD, due to the absence of muscular dystrophy protein, matrix metalloproteinase-9 (MMP-9) expression is upregulated, leading to the disruption of the connection between the extracellular matrix and cell membrane in skeletal muscle (116). MMP-9 is a biomarker associated with pathological progression of muscle injury and malnutrition, and increased expression of MMP-9 may be involved in muscle inflammatory responses (117). A recent study found that inhibiting MMP-9 gene expression can improve muscle function and reduce inflammation and fiber necrosis in mdx mice (118). TGF β-1 as a key fibrogenic biomarker positively correlated with the skeletal muscle pathology of mdx mice. MMP-9 is an activator of latent TGFβ-1 in skeletal muscle. Mantuano et al. found that metformin can significantly reduce TGFβ-1 levels to reduce pathological changes in mdx mice (111).
In general, the evidence supports the notion of a beneficial role of metformin in maintaining skeletal muscle function and treating DMD through the modulation of AMPK or skeletal muscle Ca2+ distribution or TGFβ-1 levels. Therefore, metformin may be a highly promising agent for the treatment of DMD.
4.2. Statin-associated myopathy
4.2.1. Clinical manifestations of statin-associated myopathy
Statins are inhibitors of hydroxymethylglutaryl-coenzyme A (HMG-CoA) reductase, the key enzyme inhibiting cholesterol biosynthesis, and are widely used in treating patients with dyslipidemia. Muscle toxicity is a rare adverse effect associated with statin therapy, and clinical symptoms are related to skeletal muscle discomfort, ranging in severity from mild muscle stiffness or pain to severe life-threatening rhabdomyolysis (119, 120). After discontinuation of medication, it can also be accompanied by persistent myalgia and elevated serum creatine kinase (CK) (121). At present, the best method to manage statin related muscle diseases is to prevent them in advance, use the lowest dose of statin drugs as possible to achieve therapeutic effects, avoid combining them with other drugs that can increase the risk of muscle disease, and guide patients to pay attention to whether muscle discomfort symptoms occur when taking medication (122). Skeletal muscle biopsy reveals myonecrosis or even rhabdomyolysis. Unfortunately, the muscle pain caused by statins has led many patients to stop or refuse to take the drug.
4.2.2. Mechanism of metformin in the treatment of statin-associated myopathy
Multiple lines of evidence indicated that SAMS pathogenesis may be due to statin-associated mitochondrial dysfunction (123–126). Increased Atrogin-1 in muscle tissue (127) causes increased skeletal muscle proteolysis. Mallinson et al. observed an increase in Atrogin-1 mRNA expression in muscles associated with mitochondrial dysfunction during patient treatment with statins (128). Type II muscle fibers with low mitochondrial content have long been considered more susceptible to the effects of statin therapy (119). An 8- to 40-fold increase in Atrogin-1 mRNA was found in different types of muscle atrophy (129), and the increase in Atrogin-1 expression preceded the decrease in muscle weight (130). FOXO3 is a crucial factor for the induction of Atrogin-1 (129). Sandri et al. showed that overexpression of PGC-1α inhibited the rapid loss of muscle mass induced by FOXO3. PCG-1α appears to exert this protective effect by repressing the transcription of FOXO3 via genes involved in muscle atrophy (131). One study found that overexpression of PGC-1α significantly ameliorated lovastatin-induced muscle damage and reduced Atrogin-1 expression in zebrafish and mouse myotubes. Therefore, it is speculated that metformin, a drug that increases the action or activity of PGC-1α, may prevent or counteract the deleterious effects of statins on muscle (127).
There are relatively few studies on metformin and SAMS, and the mechanisms by which metformin improves the progression of SAMS and the role of mitochondria in the disease still need to be further explored.
4.3. Age-related sarcopenia
4.3.1. Clinical manifestations of age-related sarcopenia
Aging is an inevitable part of life, and with advancing age, skeletal muscles experience a decrease in muscle mass, strength, and regenerative capacity (132–134). The decline in skeletal muscle mass and function caused by aging is also defined as sarcopenia.
The gradual loss of muscle mass causes a decline in both strength and function of the tissues, and reduced mobility leads to a decline in quality of life. Sarcopenia and its complications are a major threat to the health of the elderly. Starting from the age of 50, muscle mass decreases by 1–2% annually (135), the probability of developing sarcopenia is approximately 6–22% for individuals aged 60 to 70 years, and as high as 50% for individuals over 80 years of age (136). With the development of society and the pursuit of quality of life of older adults, it is urgent to overcome the damage to muscle structure and function caused by aging.
4.3.2. Mechanism of metformin in age-related sarcopenia
Among the underlying factors of age-related muscle atrophy are reduced mitochondrial function, impaired function of satellite cells, and increased expression of inflammatory factors (137–139). Mitochondria play a crucial role in maintaining normal cellular function and skeletal muscle bioenergetics. Numerous findings support the notion that mitochondrial content is age dependent. For instance: (a) aging leads to a decrease in the mitochondrial number, density, and size; (b) mitochondrial DNA and protein expression decline (140–143); and (c) mitochondrial function decline occurs (143–145). PGC-1α, a target for protecting skeletal muscle atrophy, downregulates FOXO3 to prevent muscle atrophy (131). Cannavinoa et al. found that the overexpression of PGC-1α blocks MuRF-1 and Atrogin-1 induction, and activates autophagy to exert protective muscle function, and compounds that stimulate PGC-1α expression that can be used to treat or prevent muscle atrophy (146). This may provide novel guidance for the clinical treatment of muscular dystrophy myopathy. Hasan et al. reported that the modulation of the PGC-1α/FOXO3 signaling pathway by metformin improved high-fat diet-induced myofiber atrophy and fibrosis (147). Therefore, modulating PGC-1α might be beneficial in preventing age-related muscle atrophy.
It is reported that as age increases, the loss of innervation of motor neurons and muscle fibers leads to an increase in fast switch myofibers loss, leading to a decrease in muscle mass and strength (148, 149). Tezze et al. found that the combination of metformin and galantamine (RJx-01) can prolong the lifespan, exercise ability, and beneficial effects on muscle structure and function of C. elegans. Moreover, Rjx-01 treatment effectively increased the fast glycolytic fibers in Opa1−/− mice (characterized by a generalized aging phenotype) (150). Mitochondrial dysfunction and mitochondrial quality control system disorder are two of the factors leading to sarcopenia, fragmentation or abnormal enlargement of mitochondria in aging skeletal muscles of rodents or humans, Mitochondrial dysfunction leads to increased expression of mitogenes from muscles (FGF21 and GDF15) and impaired mitochondrial activity (151). In aged mice treated with RJx-01, there was a significant improvement in the number of mitochondrial cristae, electron particle matrix, a swollen appearance, and levels of FGF21 and GDF15 compared to the untreated group (150).
Muscle stem cells, also known as satellite cells, usually remain stationary. Satellite cells are essential in the process of aging muscle disuse and recovery. As age increases, the number and function of satellite cells decrease, and the stationary state of satellite cells in aging skeletal muscles is disrupted, resulting in damage to the cell quality control system and inability to maintain organelles and protein homeostasis. A previous study showed that aging satellite cells exhibit autophagy disorders, leading to a decrease in the number and function of satellite cells. Using rapamycin to induce autophagy can prevent satellite cells from aging and restore their regenerative ability (152). Tezze et al. also found that the levels of the transcription factor Pax7 (a marker of satellite cells) increased in aged mice treated with RJx-01, which is beneficial for satellite cell renewal (150). Another study found that the dual treatment of metformin and leucine can improve muscle quality during aging, mainly by increasing the content of satellite cells and reducing the accumulation of collagen, thus increasing the contractible tissue per cross-sectional area of muscle (9).
Inflammation is also one of the causes of sarcopenia. During the aging process, pro-inflammatory cytokines increase, inducing a chronic low-grade inflammatory state in the body. Due to the increase in pro-inflammatory cytokines, muscle strength in the elderly is significantly impaired (153, 154). Tezze et al. found that RJx-01 treatment of Opa1−/− mice can reduce the expression of inflammatory factors (IL-6, IL-1α and IL-1β) in plasma and skeletal muscle (150). Another study found that metformin may play a protective role in preventing sarcopenia by activating AMPK and reducing the expression of pro-inflammatory factors (155). It has been suggested that aging leads to impaired macrophage function (156). There are two types of macrophages: pro-inflammatory type M1 and anti-inflammatory type M2. M2 macrophages prevent muscle atrophy and promote muscle recovery (157). Long et al. found that 10-weeks of metformin treatment can increase the abundance of M2 macrophages and reduce the expression of inflammatory factor genes in muscle tissue (158).
In the future, the molecular and cellular underpinnings of mitochondrial regulation, muscle satellite cells, and inflammatory regulation should be investigated to explore the mechanisms by which metformin improves age-related skeletal muscle atrophy and functional decline to prolong and improve the quality of life of older patients.
5. The negative effects of metformin on skeletal muscle disorders
There are differing opinions on the role of metformin in skeletal muscle diseases (Table 1). One study found that, after administering 1,700 milligrams of metformin (N = 46) or placebo (N = 48) to patients who underwent 14 weeks of progressive resistance exercise training, metformin had a negative impact on the increase in skeletal muscle mass and strength caused by progressive resistance exercise training (159). Kang et al. believe that metformin decreases skeletal muscle mass by regulating the expression of myostatin through the AMPK-FOxO3a-HDAC6 axis (160). In another study, it was found that long-term use of low-dose metformin (0.1% w/w in diet) can prolong the lifespan of mice, but higher doses (1% w/w) may significantly shorten the lifespan due to lactic acid poisoning and renal failure (161). Campagnoli et al. found that oral metformin (1,500 mg/day) can cause a decrease of sex hormone levels in breast cancer patients without diabetes (162); however, drastic changes in hormones can easily lead to the degradation of skeletal muscle mass, increase the risk of sarcopenia, and to some extent, cause a decrease in lean mass (163). Therefore, the role of metformin in the regulation of skeletal muscle mass still needs further exploration, and hormone levels and dose dependence are issues that need further consideration.
6. Conclusion and perspectives
To summarize, although there are some controversies regarding the role of metformin in skeletal muscle diseases, numerous cell culture studies, animal studies, and clinical trials have shown that the beneficial effects of metformin on inflammation, autophagy, and mitochondrial function give us great confidence in its ability to improve skeletal muscle atrophy and delay disease progression. These influential reports further advance our understanding of the molecular mechanisms by which metformin exerts multiple biological activities. In the future, focusing on the molecular signaling mechanisms involved in the multiple activities of metformin should provide additional directions for the treatment of neuromuscular diseases.
Author contributions
RS: Conceptualization, Investigation, Visualization, Writing – original draft. JM: Conceptualization, Project administration, Supervision, Writing – review & editing.
Funding
The author(s) declare financial support was received for the research, authorship, and/or publication of this article. This work was supported by the National Natural Science Foundation of China (grant number 81974191).
Acknowledgments
The authors thank Medpeer for help in creating the figures and illustrations.
Conflict of interest
The authors declare that the research was conducted in the absence of any commercial or financial relationships that could be construed as a potential conflict of interest.
Publisher’s note
All claims expressed in this article are solely those of the authors and do not necessarily represent those of their affiliated organizations, or those of the publisher, the editors and the reviewers. Any product that may be evaluated in this article, or claim that may be made by its manufacturer, is not guaranteed or endorsed by the publisher.
References
1. Dong, X, Hui, T, Chen, J, Yu, Z, Ren, D, Zou, S, et al. Metformin increases sarcolemma integrity and ameliorates neuromuscular deficits in a murine model of Duchenne muscular dystrophy. Front Physiol. (2021) 12:642908. doi: 10.3389/fphys.2021.642908
2. Song, Y, Wu, Z, and Zhao, P. The effects of metformin in the treatment of osteoarthritis: current perspectives. Front Pharmacol. (2022) 13:952560. doi: 10.3389/fphar.2022.952560
3. Bailey, CJ. Metformin: historical overview. Diabetologia. (2017) 60:1566–76. doi: 10.1007/s00125-017-4318-z
4. Lv, Z, and Guo, Y. Metformin and its benefits for various diseases. Front Endocrinol. (2020) 11:191. doi: 10.3389/fendo.2020.00191
5. Howell, JJ, Hellberg, K, Turner, M, Talbott, G, Kolar, MJ, Ross, DS, et al. Metformin inhibits hepatic Mtorc1 Signaling via dose-dependent mechanisms involving Ampk and the Tsc complex. Cell Metab. (2017) 25:463–71. doi: 10.1016/j.cmet.2016.12.009
6. Hunter, RW, Hughey, CC, Lantier, L, Sundelin, EI, Peggie, M, Zeqiraj, E, et al. Metformin reduces liver glucose production by inhibition of Fructose-1-6-Bisphosphatase. Nat Med. (2018) 24:1395–406. doi: 10.1038/s41591-018-0159-7
7. Hafner, P, Bonati, U, Klein, A, Rubino, D, Gocheva, V, Schmidt, S, et al. Effect of combination L-citrulline and metformin treatment on motor function in patients with Duchenne muscular dystrophy: a randomized clinical trial. JAMA Netw Open. (2019) 2:e1914171. doi: 10.1001/jamanetworkopen.2019.14171
8. Carris, NW, Tsalatsanis, A, Tipparaju, SM, Cheng, F, Magness, RR, and Kumar, A. Metformin's impact on statin-associated muscle symptoms: An analysis of accord study data and research materials from the Nhlbi biologic specimen and data repository information coordinating Center. Diabetes Obes Metab. (2018) 20:1994–9. doi: 10.1111/dom.13302
9. Petrocelli, JJ, Mahmassani, ZS, Fix, DK, Montgomery, JA, Reidy, PT, McKenzie, AI, et al. Metformin and leucine increase satellite cells and collagen Remodeling during disuse and recovery in aged muscle. FASEB J. (2021) 35:e21862. doi: 10.1096/fj.202100883R
10. Zhang, T, Yin, X, Yu, X, Shang, R, Lu, L, and Miao, J. Metformin protects fibroblasts from patients with Gne myopathy by restoring autophagic flux via an Ampk/Mtor-independent pathway. Biomed Pharmacother. (2023) 164:114958. doi: 10.1016/j.biopha.2023.114958
11. Kulkarni, AS, Gubbi, S, and Barzilai, N. Benefits of metformin in attenuating the hallmarks of aging. Cell Metab. (2020) 32:15–30. doi: 10.1016/j.cmet.2020.04.001
12. Choi, YH, Lee, U, Lee, BK, and Lee, MG. Pharmacokinetic interaction between itraconazole and metformin in rats: competitive inhibition of metabolism of each drug by each other via hepatic and intestinal Cyp3a1/2. Br J Pharmacol. (2010) 161:815–29. doi: 10.1111/j.1476-5381.2010.00913.x
13. Choi, YH, and Lee, MG. Effects of enzyme inducers and inhibitors on the pharmacokinetics of metformin in rats: involvement of Cyp2c11, 2d1 and 3a1/2 for the metabolism of metformin. Br J Pharmacol. (2006) 149:424–30. doi: 10.1038/sj.bjp.0706875
14. Rena, G, Hardie, DG, and Pearson, ER. The mechanisms of action of metformin. Diabetologia. (2017) 60:1577–85. doi: 10.1007/s00125-017-4342-z
15. McCreight, LJ, Bailey, CJ, and Pearson, ER. Metformin and the gastrointestinal tract. Diabetologia. (2016) 59:426–35. doi: 10.1007/s00125-015-3844-9
16. Martin, D, Thaker, J, Shreve, M, Lamerato, L, and Budzynska, K. Assessment of vitamin B12 deficiency and B12 screening trends for patients on metformin: a retrospective cohort case review. BMJ Nutr Prev Health. (2021) 4:30–5. doi: 10.1136/bmjnph-2020-000193
17. Infante, M, Leoni, M, Caprio, M, and Fabbri, A. Long-term metformin therapy and vitamin B12 deficiency: An association to bear in mind. World J Diabetes. (2021) 12:916–31. doi: 10.4239/wjd.v12.i7.916
18. DeFronzo, R, Fleming, GA, Chen, K, and Bicsak, TA. Metformin-associated lactic acidosis: current perspectives on causes and risk. Metabolism. (2016) 65:20–9. doi: 10.1016/j.metabol.2015.10.014
19. Christian Jobin aRBS. The Iκb/Nf-Κb system: a key determinant of mucosal inflammation and protection. Am J Physiol Cell Physiol. (2000) 278:C451–62. doi: 10.1152/ajpcell.2000.278.3.C451
20. Liu, C, Yang, M, Li, L, Luo, S, Yang, J, Li, C, et al. A glimpse of inflammation and anti-inflammation therapy in diabetic kidney disease. Front Physiol. (2022) 13:909569. doi: 10.3389/fphys.2022.909569
21. Salminen, A, Hyttinen, JM, and Kaarniranta, K. Amp-activated protein kinase inhibits Nf-Kappab Signaling and inflammation: impact on Healthspan and lifespan. J Mol Med. (2011) 89:667–76. doi: 10.1007/s00109-011-0748-0
22. Kim, SA, and Choi, HC. Metformin inhibits inflammatory response via Ampk-Pten pathway in vascular smooth muscle cells. Biochem Biophys Res Commun. (2012) 425:866–72. doi: 10.1016/j.bbrc.2012.07.165
23. Ye, J, Zhu, N, Sun, R, Liao, W, Fan, S, Shi, F, et al. Metformin inhibits chemokine expression through the Ampk/Nf-Kappab Signaling pathway. J Interf Cytokine Res. (2018) 38:363–9. doi: 10.1089/jir.2018.0061
24. Hattori, Y, Suzuki, K, Hattori, S, and Kasai, K. Metformin inhibits cytokine-induced nuclear factor Kappab activation via amp-activated protein kinase activation in vascular endothelial cells. Hypertension. (2006) 47:1183–8. doi: 10.1161/01.HYP.0000221429.94591.72
25. Koh, SJ, Kim, JM, Kim, IK, Ko, SH, and Kim, JS. Anti-inflammatory mechanism of metformin and its effects in intestinal inflammation and colitis-associated colon cancer. J Gastroenterol Hepatol. (2014) 29:502–10. doi: 10.1111/jgh.12435
26. Calixto, MC, Lintomen, L, Andre, DM, Leiria, LO, Ferreira, D, Lellis-Santos, C, et al. Metformin attenuates the exacerbation of the allergic eosinophilic inflammation in high fat-diet-induced obesity in mice. PLoS One. (2013) 8:e76786. doi: 10.1371/journal.pone.0076786
27. Park, CS, Bang, BR, Kwon, HS, Moon, KA, Kim, TB, Lee, KY, et al. Metformin reduces airway inflammation and Remodeling via activation of amp-activated protein kinase. Biochem Pharmacol. (2012) 84:1660–70. doi: 10.1016/j.bcp.2012.09.025
28. Sun, T, Liu, J, Xie, C, Yang, J, Zhao, L, and Yang, J. Metformin attenuates diabetic renal injury via the Ampk-autophagy Axis. Exp Ther Med. (2021) 21:578. doi: 10.3892/etm.2021.10010
29. Dobrev, D, Heijman, J, Hiram, R, Li, N, and Nattel, S. Inflammatory signalling in atrial cardiomyocytes: a novel unifying principle in atrial fibrillation pathophysiology. Nat Rev Cardiol. (2023) 20:145–67. doi: 10.1038/s41569-022-00759-w
30. Yue, RC, Lu, SZ, Luo, Y, Wang, T, Liang, H, Zeng, J, et al. Calpain silencing alleviates myocardial ischemia-reperfusion injury through the Nlrp3/Asc/Caspase-1 Axis in mice. Life Sci. (2019) 233:116631. doi: 10.1016/j.lfs.2019.116631
31. Zhang, J, Huang, L, Shi, X, Yang, L, Hua, F, Ma, J, et al. Metformin protects against myocardial ischemia-reperfusion injury and cell Pyroptosis via Ampk/Nlrp3 inflammasome pathway. Aging (Albany NY). (2020) 12:24270–87. doi: 10.18632/aging.202143
32. Guarente, L, and Franklin, H. Epstein lecture: Sirtuins, aging, and medicine. N Engl J Med. (2011) 364:2235–44. doi: 10.1056/NEJMra1100831
33. Kitada, M, Ogura, Y, Monno, I, and Koya, D. Sirtuins and type 2 diabetes: role in inflammation, oxidative stress, and mitochondrial function. Front Endocrinol. (2019) 10:187. doi: 10.3389/fendo.2019.00187
34. Tulino, R, Benjamin, AC, Jolinon, N, Smith, DL, Chini, EN, Carnemolla, A, et al. Sirt1 activity is linked to its brain region-specific phosphorylation and is impaired in Huntington's disease mice. PLoS One. (2016) 11:e0145425. doi: 10.1371/journal.pone.0145425
35. Wang, XD, Yu, WL, and Sun, Y. Activation of Ampk restored impaired autophagy and inhibited inflammation reaction by up-regulating Sirt1 in acute pancreatitis. Life Sci. (2021) 277:119435. doi: 10.1016/j.lfs.2021.119435
36. Feng, YY, Wang, Z, and Pang, H. Role of metformin in inflammation. Mol Biol Rep. (2023) 50:789–98. doi: 10.1007/s11033-022-07954-5
37. Li, SN, Wang, X, Zeng, QT, Feng, YB, Cheng, X, Mao, XB, et al. Metformin inhibits nuclear factor Kappab activation and decreases serum high-sensitivity C-reactive protein level in experimental atherogenesis of rabbits. Heart Vessel. (2009) 24:446–53. doi: 10.1007/s00380-008-1137-7
38. Isoda, K, Young, JL, Zirlik, A, MacFarlane, LA, Tsuboi, N, Gerdes, N, et al. Metformin inhibits proinflammatory responses and nuclear factor-Kappab in human Vascular Wall cells. Arterioscler Thromb Vasc Biol. (2006) 26:611–7. doi: 10.1161/01.ATV.0000201938.78044.75
39. Xian, H, Liu, Y, Rundberg Nilsson, A, Gatchalian, R, Crother, TR, Tourtellotte, WG, et al. Metformin inhibition of mitochondrial Atp and DNA synthesis abrogates Nlrp3 inflammasome activation and pulmonary inflammation. Immunity. (2021) 54:1463–77 e11. doi: 10.1016/j.immuni.2021.05.004
40. Hyun, B, Shin, S, Lee, A, Lee, S, Song, Y, Ha, NJ, et al. Metformin Down-regulates Tnf-alpha secretion via suppression of scavenger receptors in macrophages. Immune Netw. (2013) 13:123–32. doi: 10.4110/in.2013.13.4.123
41. Di Fusco, D, Dinallo, V, Monteleone, I, Laudisi, F, Marafini, I, Franze, E, et al. Metformin inhibits inflammatory signals in the gut by controlling Ampk and P38 map kinase activation. Clin Sci. (2018) 132:1155–68. doi: 10.1042/CS20180167
42. Liu, C, Chu, D, Kalantar-Zadeh, K, George, J, Young, HA, and Liu, G. Cytokines: from clinical significance to quantification. Adv Sci. (2021) 8:e2004433. doi: 10.1002/advs.202004433
43. Hammad, AM, Ibrahim, YA, Khdair, SI, Hall, FS, Alfaraj, M, Jarrar, Y, et al. Metformin reduces oxandrolone-induced depression-like behavior in rats via modulating the expression of il-1beta, il-6, il-10 and Tnf-alpha. Behav Brain Res. (2021) 414:113475. doi: 10.1016/j.bbr.2021.113475
44. Li, X, He, S, and Ma, B. Autophagy and autophagy-related proteins in cancer. Mol Cancer. (2020) 19:12. doi: 10.1186/s12943-020-1138-4
45. De Meyer, GR, Grootaert, MO, Michiels, CF, Kurdi, A, Schrijvers, DM, and Martinet, W. Autophagy in vascular disease. Circ Res. (2015) 116:468–79. doi: 10.1161/CIRCRESAHA.116.303804
46. Jia, G, and Sowers, JR. Autophagy: a housekeeper in cardiorenal metabolic health and disease. Biochim Biophys Acta. (2015) 1852:219–24. doi: 10.1016/j.bbadis.2014.06.025
47. Tooze, SA, and Yoshimori, T. The origin of the Autophagosomal membrane. Nat Cell Biol. (2010) 12:831–5. doi: 10.1038/ncb0910-831
48. Glick, D, Barth, S, and Macleod, KF. Autophagy: cellular and molecular mechanisms. J Pathol. (2010) 221:3–12. doi: 10.1002/path.2697
49. Mari, M, Tooze, SA, and Reggiori, F. The Puzzling Origin of the Autophagosomal Membrane. F1000 Biol Rep. (2011) 3:25. doi: 10.3410/B3-25
50. Cao, W, Li, J, Yang, K, and Cao, D. An overview of autophagy: mechanism, regulation and research Progress. Bull Cancer. (2021) 108:304–22. doi: 10.1016/j.bulcan.2020.11.004
51. Zhou, Y, Manghwar, H, Hu, W, and Liu, F. Degradation mechanism of autophagy-related proteins and research Progress. Int J Mol Sci. (2022) 23:7301. doi: 10.3390/ijms23137301
52. Hamano, T, Enomoto, S, Shirafuji, N, Ikawa, M, Yamamura, O, Yen, SH, et al. Autophagy and tau protein. Int J Mol Sci. (2021) 22:7475. doi: 10.3390/ijms22147475
53. Xu, B, Dai, W, Liu, L, Han, H, Zhang, J, Du, X, et al. Metformin ameliorates polycystic ovary syndrome in a rat model by decreasing excessive autophagy in ovarian granulosa cells via the Pi3k/Akt/Mtor pathway. Endocr J. (2022) 69:863–75. doi: 10.1507/endocrj.EJ21-0480
54. Wang, Y, Xu, W, Yan, Z, Zhao, W, Mi, J, Li, J, et al. Metformin induces autophagy and G0/G1 phase cell cycle arrest in myeloma by targeting the Ampk/Mtorc1 and Mtorc2 pathways. J Exp Clin Cancer Res. (2018) 37:63. doi: 10.1186/s13046-018-0731-5
55. Vijayan, V, and Verstreken, P. Autophagy in the presynaptic compartment in health and disease. J Cell Biol. (2017) 216:1895–906. doi: 10.1083/jcb.201611113
56. Jinpiao, Z, Zongze, Z, Qiuyue, Y, Peng, F, Qi, Z, Yanlin, W, et al. Metformin attenuates sevoflurane-induced neurocognitive impairment through Ampk-Ulk1-dependent autophagy in aged mice. Brain Res Bull. (2020) 157:18–25. doi: 10.1016/j.brainresbull.2020.01.018
57. Wang, C, Yao, Z, Zhang, Y, Yang, Y, Liu, J, Shi, Y, et al. Metformin mitigates cartilage degradation by activating Ampk/Sirt1-mediated autophagy in a mouse osteoarthritis model. Front Pharmacol. (2020) 11:1114. doi: 10.3389/fphar.2020.01114
58. Li, YL, Li, XQ, Wang, YD, Shen, C, and Zhao, CY. Metformin alleviates inflammatory response in non-alcoholic steatohepatitis by restraining signal transducer and activator of transcription 3-mediated autophagy inhibition in vitro and in vivo. Biochem Biophys Res Commun. (2019) 513:64–72. doi: 10.1016/j.bbrc.2019.03.077
59. Song, YM, Lee, YH, Kim, JW, Ham, DS, Kang, ES, Cha, BS, et al. Metformin alleviates Hepatosteatosis by restoring Sirt1-mediated autophagy induction via an amp-activated protein kinase-independent pathway. Autophagy. (2015) 11:46–59. doi: 10.4161/15548627.2014.984271
60. Xu, J, Liu, LQ, Xu, LL, Xing, Y, and Ye, S. Metformin alleviates renal injury in diabetic rats by inducing Sirt1/Foxo1 autophagic signal Axis. Clin Exp Pharmacol Physiol. (2020) 47:599–608. doi: 10.1111/1440-1681.13226
61. He, C, Zhu, H, Li, H, Zou, MH, and Xie, Z. Dissociation of Bcl-2-Beclin1 complex by activated Ampk enhances cardiac autophagy and protects against cardiomyocyte apoptosis in diabetes. Diabetes. (2013) 62:1270–81. doi: 10.2337/db12-0533
62. Wang, Z, Guo, J, Han, X, Xue, M, Wang, W, Mi, L, et al. Metformin represses the pathophysiology of Aaa by suppressing the activation of Pi3k/Akt/Mtor/autophagy pathway in Apoe−/− mice. Cell Biosci. (2019) 9:68. doi: 10.1186/s13578-019-0332-9
63. Laplante, M, and Sabatini, DM. Mtor Signaling in growth control and disease. Cells. (2012) 149:274–93. doi: 10.1016/j.cell.2012.03.017
64. Yang, ZJ, Chee, CE, Huang, S, and Sinicrope, FA. The role of autophagy in cancer: therapeutic implications. Mol Cancer Ther. (2011) 10:1533–41. doi: 10.1158/1535-7163.MCT-11-0047
65. Levine, B. Unraveling the role of autophagy in cancer. Autophagy. (2006) 2:65–6. doi: 10.4161/auto.2.2.2457
66. White, E. The role for autophagy in cancer. J Clin Invest. (2015) 125:42–6. doi: 10.1172/JCI73941
67. Harris, H, and Rubinsztein, DC. Control of autophagy as a therapy for neurodegenerative disease. Nat Rev Neurol. (2011) 8:108–17. doi: 10.1038/nrneurol.2011.200
68. Nixon, RA. The role of autophagy in neurodegenerative disease. Nat Med. (2013) 19:983–97. doi: 10.1038/nm.3232
69. Liang, Y, and Sigrist, S. Autophagy and Proteostasis in the control of synapse aging and disease. Curr Opin Neurobiol. (2018) 48:113–21. doi: 10.1016/j.conb.2017.12.006
70. Son, JH, Shim, JH, Kim, KH, Ha, JY, and Han, JY. Neuronal autophagy and neurodegenerative diseases. Exp Mol Med. (2012) 44:89–98. doi: 10.3858/emm.2012.44.2.031
71. Li, ZQ, Li, LX, Mo, N, Cao, YY, Kuerban, B, Liang, YX, et al. Duration-dependent regulation of autophagy by isoflurane exposure in aged rats. Neurosci Bull. (2015) 31:505–13. doi: 10.1007/s12264-015-1549-1
72. Lu, C, Zhao, H, Liu, Y, Yang, Z, Yao, H, Liu, T, et al. Novel role of the Sirt1 in endocrine and metabolic diseases. Int J Biol Sci. (2023) 19:484–501. doi: 10.7150/ijbs.78654
73. Baeken, MW. Sirtuins and their influence on autophagy. J Cell Biochem. (2023). doi: 10.1002/jcb.30377
74. Nogueiras, R, Habegger, KM, Chaudhary, N, Finan, B, Banks, AS, Dietrich, MO, et al. Sirtuin 1 and Sirtuin 3: physiological modulators of metabolism. Physiol Rev. (2012) 92:1479–514. doi: 10.1152/physrev.00022.2011
75. Feng, Y, Ke, C, Tang, Q, Dong, H, Zheng, X, Lin, W, et al. Metformin promotes autophagy and apoptosis in Esophageal squamous cell carcinoma by downregulating Stat3 Signaling. Cell Death Dis. (2014) 5:e1088. doi: 10.1038/cddis.2014.59
76. Nakagawa, A, Sullivan, KD, and Xue, D. Caspase-activated phosphoinositide binding by Cnt-1 promotes apoptosis by inhibiting the Akt pathway. Nat Struct Mol Biol. (2014) 21:1082–90. doi: 10.1038/nsmb.2915
77. Malemud, CJ. The Pi3k/Akt/Pten/Mtor pathway: a fruitful target for inducing cell death in rheumatoid arthritis? Future Med Chem. (2015) 7:1137–47. doi: 10.4155/fmc.15.55
78. Sun, R, Zhai, R, Ma, C, and Miao, W. Combination of aloin and metformin enhances the antitumor effect by inhibiting the growth and invasion and inducing apoptosis and autophagy in hepatocellular carcinoma through Pi3k/Akt/Mtor pathway. Cancer Med. (2020) 9:1141–51. doi: 10.1002/cam4.2723
79. Liu, B, Deng, X, Jiang, Q, Li, G, Zhang, J, Zhang, N, et al. Scoparone improves hepatic inflammation and autophagy in mice with nonalcoholic steatohepatitis by regulating the Ros/P38/Nrf2 Axis and Pi3k/Akt/Mtor pathway in macrophages. Biomed Pharmacother. (2020) 125:109895. doi: 10.1016/j.biopha.2020.109895
80. Sun, H, Wang, Z, and Yakisich, J. Natural Products Targeting Autophagy Via the Pi3k/Akt/Mtor Pathway as Anticancer Agents. Anticancer Agents Med Chem. (2013) 13:1048–56. doi: 10.2174/18715206113139990130
81. Nascimbeni, AC, Fanin, M, Angelini, C, and Sandri, M. Autophagy dysregulation in Danon disease. Cell Death Dis. (2017) 8:e2565. doi: 10.1038/cddis.2016.475
82. Ripolone, M, Violano, R, Ronchi, D, Mondello, S, Nascimbeni, A, Colombo, I, et al. Effects of Short-to-Long term enzyme replacement therapy (Ert) on skeletal muscle tissue in late onset Pompe disease (Lopd). Neuropathol Appl Neurobiol. (2018) 44:449–62. doi: 10.1111/nan.12414
83. Nascimbeni, AC, Fanin, M, Masiero, E, Angelini, C, and Sandri, M. The role of autophagy in the pathogenesis of glycogen storage disease type ii (Gsdii). Cell Death Differ. (2012) 19:1698–708. doi: 10.1038/cdd.2012.52
84. Zhang, C, Gao, X, Li, M, Yu, X, Huang, F, Wang, Y, et al. The role of mitochondrial quality surveillance in skin aging: focus on mitochondrial dynamics, Biogenesis and Mitophagy. Ageing Res Rev. (2023) 87:101917. doi: 10.1016/j.arr.2023.101917
85. Valero, T. Mitochondrial biogenesis: pharmacological approaches. Curr Pharm Des. (2014) 20:5507–9. doi: 10.2174/138161282035140911142118
86. Jamwal, S, Blackburn, JK, and Elsworth, JD. Ppargamma/Pgc1alpha Signaling as a potential therapeutic target for mitochondrial biogenesis in neurodegenerative disorders. Pharmacol Ther. (2021) 219:107705. doi: 10.1016/j.pharmthera.2020.107705
87. Khot, M, Sood, A, Tryphena, KP, Khan, S, Srivastava, S, Singh, SB, et al. Nlrp3 inflammasomes: a potential target to improve mitochondrial biogenesis in Parkinson's disease. Eur J Pharmacol. (2022) 934:175300. doi: 10.1016/j.ejphar.2022.175300
88. Suwa, M, Egashira, T, Nakano, H, Sasaki, H, and Kumagai, S. Metformin increases the Pgc-1alpha protein and oxidative enzyme activities possibly via Ampk phosphorylation in skeletal muscle in vivo. J Appl Phys. (2006) 101:1685–92. doi: 10.1152/japplphysiol.00255.2006
89. Wu, Z, Puigserver, P, Andersson, U, Zhang, C, Adelmant, G, Mootha, V, et al. Mechanisms controlling mitochondrial biogenesis and respiration through the thermogenic coactivator Pgc-1. Cells. (1999) 98:115–24. doi: 10.1016/S0092-8674(00)80611-X
90. Jager, S, Handschin, C, St-Pierre, J, and Spiegelman, BM. Amp-activated protein kinase (Ampk) action in skeletal muscle via direct phosphorylation of Pgc-1alpha. Proc Natl Acad Sci U S A. (2007) 104:12017–22. doi: 10.1073/pnas.0705070104
91. Wu, J, Li, J, Feng, B, Bi, Z, Zhu, G, Zhang, Y, et al. Activation of Ampk-Pgc-1alpha pathway ameliorates peritoneal dialysis related peritoneal fibrosis in mice by enhancing mitochondrial biogenesis. Ren Fail. (2022) 44:1545–57. doi: 10.1080/0886022X.2022.2126789
92. Wang, X, Su, B, Zheng, L, Perry, G, Smith, MA, and Zhu, X. The role of abnormal mitochondrial dynamics in the pathogenesis of Alzheimer's disease. J Neurochem. (2009) 109:153–9. doi: 10.1111/j.1471-4159.2009.05867.x
93. Sheng, B, Wang, X, Su, B, Lee, HG, Casadesus, G, Perry, G, et al. Impaired mitochondrial biogenesis contributes to mitochondrial dysfunction in Alzheimer's disease. J Neurochem. (2012) 120:419–29. doi: 10.1111/j.1471-4159.2011.07581.x
94. Khang, R, Park, C, and Shin, JH. Dysregulation of parkin in the substantia nigra of Db/Db and high-fat diet mice. Neuroscience. (2015) 294:182–92. doi: 10.1016/j.neuroscience.2015.03.017
95. Turdi, S, Fan, X, Li, J, Zhao, J, Huff, AF, Du, M, et al. Amp-activated protein kinase deficiency exacerbates aging-induced myocardial contractile dysfunction. Aging Cell. (2010) 9:592–606. doi: 10.1111/j.1474-9726.2010.00586.x
96. Gundewar, S, Calvert, JW, Jha, S, Toedt-Pingel, I, Ji, SY, Nunez, D, et al. Activation of amp-activated protein kinase by metformin improves left ventricular function and survival in heart failure. Circ Res. (2009) 104:403–11. doi: 10.1161/CIRCRESAHA.108.190918
97. Saad, FA, Siciliano, G, and Angelini, C. Advances in Dystrophinopathy diagnosis and therapy. Biomol Ther. (2023) 13:1319. doi: 10.3390/biom13091319
98. Deng, J, Zhang, J, Shi, K, and Liu, Z. Drug development Progress in Duchenne muscular dystrophy. Front Pharmacol. (2022) 13:950651. doi: 10.3389/fphar.2022.950651
99. Allen, DG, Whitehead, NP, and Froehner, SC. Absence of dystrophin disrupts skeletal muscle Signaling: roles of Ca2+, reactive oxygen species, and nitric oxide in the development of muscular dystrophy. Physiol Rev. (2016) 96:253–305. doi: 10.1152/physrev.00007.2015
100. Grounds, MD, Terrill, JR, Al-Mshhdani, BA, Duong, MN, Radley-Crabb, HG, and Arthur, PG. Biomarkers for Duchenne muscular dystrophy: myonecrosis, inflammation and oxidative stress. Dis Model Mech. (2020) 13:dmm043638. doi: 10.1242/dmm.043638
101. Henricson, EK, Abresch, RT, Cnaan, A, Hu, F, Duong, T, Arrieta, A, et al. The cooperative international neuromuscular research group Duchenne natural history study: glucocorticoid treatment preserves clinically meaningful functional milestones and reduces rate of disease progression as measured by manual muscle testing and Othe. Muscle Nerve. (2013) 48:55–67. doi: 10.1002/mus.23808
102. Sutherland, DH, Olshen, R, Cooper, L, Wyatt, M, Leach, J, Mubarak, S, et al. The Pathomechanics of gait in Duchenne muscular dystrophy. Dev Med Child Neurol. (2008) 23:3–22. doi: 10.1111/j.1469-8749.1981.tb08442.x
103. Whitehead, NP, Yeung, EW, Froehner, SC, and Allen, DG. Skeletal muscle Nadph oxidase is increased and triggers stretch-induced damage in the mdx mouse. PLoS One. (2010) 5:e15354. doi: 10.1371/journal.pone.0015354
104. Ljubicic, V, and Jasmin, BJ. Metformin increases peroxisome proliferator-activated receptor gamma co-activator-1alpha and utrophin a expression in dystrophic skeletal muscle. Muscle Nerve. (2015) 52:139–42. doi: 10.1002/mus.24692
105. Lovering, RM, Iyer, SR, Edwards, B, and Davies, KE. Alterations of neuromuscular junctions in Duchenne muscular dystrophy. Neurosci Lett. (2020):737. doi: 10.1016/j.neulet.2020.135304
106. Pratt, SJP, Shah, SB, Ward, CW, Kerr, JP, Stains, JP, and Lovering, RM. Recovery of altered neuromuscular junction morphology and muscle function in mdx mice after injury. Cell Mol Life Sci. (2014) 72:153–64. doi: 10.1007/s00018-014-1663-7
107. Mounier, R, Theret, M, Arnold, L, Cuvellier, S, Bultot, L, Goransson, O, et al. Ampkalpha1 regulates macrophage skewing at the time of resolution of inflammation during skeletal muscle regeneration. Cell Metab. (2013) 18:251–64. doi: 10.1016/j.cmet.2013.06.017
108. Peralta, S, Garcia, S, Yin, HY, Arguello, T, Diaz, F, and Moraes, CT. Sustained Ampk activation improves muscle function in a mitochondrial myopathy mouse model by promoting muscle Fiber regeneration. Hum Mol Genet. (2016) 25:3178–91. doi: 10.1093/hmg/ddw167
109. Thomas, MM, Wang, DC, D'Souza, DM, Krause, MP, Layne, AS, Criswell, DS, et al. Muscle-specific Ampk Beta1beta2-null mice display a myopathy due to loss of capillary density in nonpostural muscles. FASEB J. (2014) 28:2098–107. doi: 10.1096/fj.13-238972
110. Wang, Y, An, H, Liu, T, Qin, C, Sesaki, H, Guo, S, et al. Metformin improves mitochondrial respiratory activity through activation of Ampk. Cell Rep. (2019) 29:1511–23 e5. doi: 10.1016/j.celrep.2019.09.070
111. Mantuano, P, Sanarica, F, Conte, E, Morgese, MG, Capogrosso, RF, Cozzoli, A, et al. Effect of a Long-term treatment with metformin in dystrophic mdx mice: a reconsideration of its potential clinical interest in Duchenne muscular dystrophy. Biochem Pharmacol. (2018):154. doi: 10.1016/j.bcp.2018.04.022
112. Musi, N, Hirshman, MF, Nygren, J, Svanfeldt, M, Bavenholm, P, Rooyackers, O, et al. Metformin increases amp-activated protein kinase activity in skeletal muscle of subjects with type 2 diabetes. Diabetes. (2002) 51:2074–81. doi: 10.2337/diabetes.51.7.2074
113. Gissel, H. The role of Ca2+ in muscle cell damage. Ann N Y Acad Sci. (2005) 1066:166–80. doi: 10.1196/annals.1363.013
114. Langone, F, Cannata, S, Fuoco, C, Lettieri Barbato, D, Testa, S, Nardozza, AP, et al. Metformin protects skeletal muscle from cardiotoxin induced degeneration. PLoS One. (2014) 9:e114018. doi: 10.1371/journal.pone.0114018
115. Ramadasan-Nair, R, Gayathri, N, Mishra, S, Sunitha, B, Mythri, RB, Nalini, A, et al. Mitochondrial alterations and oxidative stress in an acute transient mouse model of muscle degeneration: implications for muscular dystrophy and related muscle pathologies. J Biol Chem. (2014) 289:485–509. doi: 10.1074/jbc.M113.493270
116. Matsumura, K, Zhong, D, Saito, F, Arai, K, Adachi, K, Kawai, H, et al. Proteolysis of Beta-Dystroglycan in muscular diseases. Neuromuscul Disord. (2005) 15:336–41. doi: 10.1016/j.nmd.2005.01.007
117. Fukushima, K, Nakamura, A, Ueda, H, Yuasa, K, Yoshida, K, Si, T, et al. Activation and localization of matrix Metalloproteinase-2 and -9 in the skeletal muscle of the muscular dystrophy dog (Cxmdj). BMC Musculoskelet Disord. (2007) 8:54. doi: 10.1186/1471-2474-8-54
118. Li, H, Mittal, A, Makonchuk, DY, Bhatnagar, S, and Kumar, A. Matrix Metalloproteinase-9 inhibition ameliorates pathogenesis and improves skeletal muscle regeneration in muscular dystrophy. Hum Mol Genet. (2009) 18:2584–98. doi: 10.1093/hmg/ddp191
119. Westwood, FR, Bigley, A, Randall, K, Marsden, AM, and Scott, RC. Statin-induced muscle necrosis in the rat: distribution, development, and fibre selectivity. Toxicol Pathol. (2005) 33:246–57. doi: 10.1080/01926230590908213
120. Carris, NW, Tipparaju, SM, Magness, DJ, Chapalamadugu, KC, and Magness, RR. Pleiotropic effects of metformin to rescue statin-induced muscle injury and insulin resistance: a proposed mechanism and potential clinical implications. Med Hypotheses. (2017) 107:39–44. doi: 10.1016/j.mehy.2017.07.007
121. Walravens, PA, Greene, C, and Frerman, FE. Lovastatin, Isoprenes, and myopathy. Lancet. (1989) 2:1097–8. doi: 10.1016/s0140-6736(89)91108-2
122. Pasternak, RC, Smith, SC, Bairey-Merz, CN, Grundy, SM, Cleeman, JI, and Lenfant, C. Acc/aha/Nhlbi clinical advisory on the use and safety of statins. J Am Coll Cardiol. (2002) 40:567–72. doi: 10.1016/s0735-1097(02)02030-2
123. Vaklavas, C, Chatzizisis, YS, Ziakas, A, Zamboulis, C, and Giannoglou, GD. Molecular basis of statin-associated myopathy. Atherosclerosis. (2009) 202:18–28. doi: 10.1016/j.atherosclerosis.2008.05.021
124. Wu, JS, Buettner, C, Smithline, H, Ngo, LH, and Greenman, RL. Evaluation of skeletal muscle during calf exercise by 31-phosphorus magnetic resonance spectroscopy in patients on statin medications. Muscle Nerve. (2011) 43:76–81. doi: 10.1002/mus.21847
125. Hubal, MJ, Reich, KA, De Biase, A, Bilbie, C, Clarkson, PM, Hoffman, EP, et al. Transcriptional deficits in oxidative phosphorylation with statin myopathy. Muscle Nerve. (2011) 44:393–401. doi: 10.1002/mus.22081
126. Thompson, PD, Clarkson, P, and Karas, RH. Statin-associated myopathy. JAMA. (2003) 289:1681–90. doi: 10.1001/jama.289.13.1681
127. Hanai, J, Cao, P, Tanksale, P, Imamura, S, Koshimizu, E, Zhao, J, et al. The muscle-specific ubiquitin ligase Atrogin-1/Mafbx mediates statin-induced muscle toxicity. J Clin Invest. (2007) 117:3940–51. doi: 10.1172/JCI32741
128. Mallinson, JE, Marimuthu, K, Murton, A, Selby, A, Smith, K, Constantin-Teodosiu, D, et al. Statin myalgia is not associated with reduced muscle strength, mass or protein turnover in older male volunteers, but is allied with a slowing of time to peak power output, insulin resistance and differential muscle Mrna expression. J Physiol. (2015) 593:1239–57. doi: 10.1113/jphysiol.2014.285577
129. Sandri, M, Sandri, C, Gilbert, A, Skurk, C, Calabria, E, Picard, A, et al. Foxo transcription factors induce the atrophy-related ubiquitin ligase Atrogin-1 and cause skeletal muscle atrophy. Cells. (2004) 117:399–412. doi: 10.1016/s0092-8674(04)00400-3
130. Gomes, MD, Lecker, SH, Jagoe, RT, Navon, A, and Goldberg, AL. Atrogin-1, a muscle-specific F-box protein highly expressed during muscle atrophy. Proc Natl Acad Sci U S A. (2001) 98:14440–5. doi: 10.1073/pnas.251541198
131. Sandri, M, Lin, J, Handschin, C, Yang, W, Arany, ZP, Lecker, SH, et al. Pgc-1alpha protects skeletal muscle from atrophy by suppressing Foxo3 action and atrophy-specific gene transcription. Proc Natl Acad Sci U S A. (2006) 103:16260–5. doi: 10.1073/pnas.0607795103
132. Faulkner, JA, Larkin, LM, Claflin, DR, and Brooks, SV. Age-related changes in the structure and function of skeletal muscles. Clin Exp Pharmacol Physiol. (2007) 34:1091–6. doi: 10.1111/j.1440-1681.2007.04752.x
133. Distefano, G, and Goodpaster, BH. Effects of exercise and aging on skeletal muscle. Cold Spring Harb Perspect Med. (2018) 8:a029785. doi: 10.1101/cshperspect.a029785
134. Aoyagi, Y, and Shephard, RJ. Aging and muscle function. Sports Med. (1992) 14:376–96. doi: 10.2165/00007256-199214060-00005
135. Chen, F, Xu, S, Wang, Y, Chen, F, Cao, L, Liu, T, et al. Risk factors for sarcopenia in the elderly with type 2 diabetes mellitus and the effect of metformin. J Diabetes Res. (2020): 2020, 1–10. doi: 10.1155/2020/3950404
136. Dent, E, Morley, JE, Cruz-Jentoft, AJ, Arai, H, Kritchevsky, SB, Guralnik, J, et al. International clinical practice guidelines for sarcopenia (Icfsr): screening, diagnosis and management. J Nutr Health Aging. (2018) 22:1148–61. doi: 10.1007/s12603-018-1139-9
137. Koster, A, Ding, J, Stenholm, S, Caserotti, P, Houston, DK, Nicklas, BJ, et al. Does the amount of fat mass predict age-related loss of lean mass, muscle strength, and muscle quality in older adults? J Gerontol Ser A Biol Med Sci. (2011) 66A:888–95. doi: 10.1093/gerona/glr070
138. Alway, SE, Myers, MJ, and Mohamed, JS. Regulation of satellite cell function in sarcopenia. Front Aging Neurosci. (2014) 6:246. doi: 10.3389/fnagi.2014.00246
139. Schaap, LA, Pluijm, SM, Deeg, DJ, Harris, TB, Kritchevsky, SB, Newman, AB, et al. Higher inflammatory marker levels in older persons: associations with 5-year change in muscle mass and muscle strength. J Gerontol A Biol Sci Med Sci. (2009) 64:1183–9. doi: 10.1093/gerona/glp097
140. Lanza, IR, Short, DK, Short, KR, Raghavakaimal, S, Basu, R, Joyner, MJ, et al. Endurance exercise as a countermeasure for aging. Diabetes. (2008) 57:2933–42. doi: 10.2337/db08-0349
141. Orlander, J, Kiessling, KH, Larsson, L, Karlsson, J, and Aniansson, A. Skeletal muscle metabolism and ultrastructure in relation to age in sedentary men. Acta Physiol Scand. (1978) 104:249–61. doi: 10.1111/j.1748-1716.1978.tb06277.x
142. Conley, KE, Jubrias, SA, and Esselman, PC. Oxidative capacity and ageing in human muscle. J Physiol. (2000) 526:203–10. doi: 10.1111/j.1469-7793.2000.t01-1-00203.x
143. Short, KR, Bigelow, ML, Kahl, J, Singh, R, Coenen-Schimke, J, Raghavakaimal, S, et al. Decline in skeletal muscle mitochondrial function with aging in humans. Proc Natl Acad Sci U S A. (2005) 102:5618–23. doi: 10.1073/pnas.0501559102
144. Chabi, B, Ljubicic, V, Menzies, KJ, Huang, JH, Saleem, A, and Hood, DA. Mitochondrial function and apoptotic susceptibility in aging skeletal muscle. Aging Cell. (2008) 7:2–12. doi: 10.1111/j.1474-9726.2007.00347.x
145. Kumaran, S, Panneerselvam, KS, Shila, S, Sivarajan, K, and Panneerselvam, C. Age-associated deficit of mitochondrial oxidative phosphorylation in skeletal muscle: role of carnitine and lipoic acid. Mol Cell Biochem. (2005) 280:83–9. doi: 10.1007/s11010-005-8234-z
146. Cannavino, J, Brocca, L, Sandri, M, Bottinelli, R, and Pellegrino, MA. Pgc1-alpha over-expression prevents metabolic alterations and soleus muscle atrophy in hindlimb unloaded mice. J Physiol. (2014) 592:4575–89. doi: 10.1113/jphysiol.2014.275545
147. Hasan, MM, Shalaby, SM, El-Gendy, J, and Abdelghany, EMA. Beneficial effects of metformin on muscle atrophy induced by obesity in rats. J Cell Biochem. (2019) 120:5677–86. doi: 10.1002/jcb.27852
148. Larsson, L, Degens, H, Li, M, Salviati, L, Yi, L, Thompson, W, et al. Sarcopenia: aging-related loss of muscle mass and function. Physiol Rev. (2019) 99:427–511. doi: 10.1152/physrev.00061.2017
149. Akasaki, Y, Ouchi, N, Izumiya, Y, Bernardo, BL, LeBrasseur, NK, and Walsh, K. Glycolytic fast-twitch muscle Fiber restoration counters adverse age-related changes in body composition and metabolism. Aging Cell. (2014) 13:80–91. doi: 10.1111/acel.12153
150. Tezze, C, Amendolagine, FI, Nogara, L, Baraldo, M, Ciciliot, S, Arcidiacono, D, et al. A combination of metformin and galantamine exhibits synergistic benefits in the treatment of sarcopenia. JCI Insight. (2023) 8:e168787. doi: 10.1172/jci.insight.168787
151. Romanello, V. The interplay between mitochondrial morphology and Myomitokines in aging sarcopenia. Int J Mol Sci. (2020) 22:91. doi: 10.3390/ijms22010091
152. García-Prat, L, Martínez-Vicente, M, Perdiguero, E, Ortet, L, Rodríguez-Ubreva, J, Rebollo, E, et al. Autophagy maintains stemness by preventing senescence. Nature. (2016) 529:37–42. doi: 10.1038/nature16187
153. Dalle, S, Rossmeislova, L, and Koppo, K. The role of inflammation in age-related sarcopenia. Front Physiol. (2017):8. doi: 10.3389/fphys.2017.01045
154. Chen, H-T, Wu, H-J, Chen, Y-J, Ho, S-Y, and Chung, Y-C. Effects of 8-week kettlebell training on body composition, muscle strength, pulmonary function, and chronic low-grade inflammation in elderly women with sarcopenia. Exp Gerontol. (2018) 112:112–8. doi: 10.1016/j.exger.2018.09.015
155. Lyu, Q, Wen, Y, He, B, Zhang, X, Chen, J, Sun, Y, et al. The ameliorating effects of metformin on disarrangement ongoing in gastrocnemius muscle of sarcopenic and obese sarcopenic mice. Biochim Biophys Acta Mol Basis Dis. (2022) 1868:166508. doi: 10.1016/j.bbadis.2022.166508
156. Przybyla, B, Gurley, C, Harvey, JF, Bearden, E, Kortebein, P, Evans, WJ, et al. Aging alters macrophage properties in human skeletal muscle both at rest and in response to acute resistance exercise. Exp Gerontol. (2006) 41:320–7. doi: 10.1016/j.exger.2005.12.007
157. Dumont, N, and Frenette, J. Macrophages protect against muscle atrophy and promote muscle recovery in vivo and in vitro: a mechanism partly dependent on the insulin-like growth Factor-1 Signaling molecule. Am J Pathol. (2010) 176:2228–35. doi: 10.2353/ajpath.2010.090884
158. Long, DE, Peck, BD, Martz, JL, Tuggle, SC, Bush, HM, McGwin, G, et al. Metformin to augment strength training effective response in seniors (masters): study protocol for a randomized controlled trial. Trials. (2017) 18:192. doi: 10.1186/s13063-017-1932-5
159. Walton, RG, Dungan, CM, Long, DE, Tuggle, SC, Kosmac, K, Peck, BD, et al. Metformin blunts muscle hypertrophy in response to progressive resistance exercise training in older adults: a randomized, double-blind, placebo-controlled, Multicenter trial: the masters trial. Aging Cell. (2019) 18:e13039. doi: 10.1111/acel.13039
160. Kang, MJ, Moon, JW, Lee, JO, Kim, JH, Jung, EJ, Kim, SJ, et al. Metformin induces muscle atrophy by transcriptional regulation of myostatin via Hdac6 and Foxo3a. J Cachexia Sarcopenia Muscle. (2022) 13:605–20. doi: 10.1002/jcsm.12833
161. Martin-Montalvo, A, Mercken, EM, Mitchell, SJ, Palacios, HH, Mote, PL, Scheibye-Knudsen, M, et al. Metformin improves Healthspan and lifespan in mice. Nat Commun. (2013) 4:2192. doi: 10.1038/ncomms3192
162. Campagnoli, C, Berrino, F, Venturelli, E, Abbà, C, Biglia, N, Brucato, T, et al. Metformin decreases circulating androgen and Estrogen levels in nondiabetic women with breast cancer. Clin Breast Cancer. (2013) 13:433–8. doi: 10.1016/j.clbc.2013.08.012
Keywords: metformin, inflammation, autophagy, mitochondrial biogenesis, skeletal muscle disorders
Citation: Shang R and Miao J (2023) Mechanisms and effects of metformin on skeletal muscle disorders. Front. Neurol. 14:1275266. doi: 10.3389/fneur.2023.1275266
Edited by:
Jens Schmidt, Immanuel Klinik Rüdersdorf, GermanyReviewed by:
Paola Mantuano, University of Bari Aldo Moro, ItalyCorrado Italo Angelini, University of Padua, Italy
Copyright © 2023 Shang and Miao. This is an open-access article distributed under the terms of the Creative Commons Attribution License (CC BY). The use, distribution or reproduction in other forums is permitted, provided the original author(s) and the copyright owner(s) are credited and that the original publication in this journal is cited, in accordance with accepted academic practice. No use, distribution or reproduction is permitted which does not comply with these terms.
*Correspondence: Jing Miao, amR5eW1pYW9qaW5nQGpsdS5lZHUuY24=