- 1Department of Otolaryngology Head and Neck Surgery, Johns Hopkins University School of Medicine, Baltimore, MD, United States
- 2Division of Physical Therapy, Department of Physical Medicine and Rehabilitation School of Medicine, Emory University, Atlanta, GA, United States
- 3Physical Therapy Department, University of Pittsburgh, Pittsburgh, PA, United States
- 4Department of Otolaryngology—Head and Neck Surgery, Ohio State University Wexner Medical Center, Columbus, OH, United States
- 5School of Health and Rehabilitation Sciences, Ohio State University, Columbus, OH, United States
- 6Department of Otolaryngology, University of Rochester, Rochester, NY, United States
- 7Physical Therapy Department, University of Rochester, Rochester, NY, United States
- 8Department of Neuroscience, University of Rochester, Rochester, NY, United States
Not all dizziness presents as vertigo, suggesting other perceptual symptoms for individuals with vestibular disease. These non-specific perceptual complaints of dizziness have led to a recent resurgence in literature examining vestibular perceptual testing with the aim to enhance clinical diagnostics and therapeutics. Recent evidence supports incorporating rehabilitation methods to retrain vestibular perception. This review describes the current field of vestibular perceptual testing from scientific laboratory techniques that may not be clinic friendly to some low-tech options that may be more clinic friendly. Limitations are highlighted suggesting directions for additional research.
1. Introduction
Self-motion perception, the conscious awareness of active or passive motion/orientation of one’s body in space, is influenced by multiple factors in health and disease, including the state of peripheral vestibular reflexes (1), central postural control and spatial orientation systems (2, 3), attentional networks (4), emotional states (5), and behavioral responses to perceived postural threats (6). Individuals with persistent complaints of vertigo/disequilibrium experience abnormal vestibular self-motion perception and spatial orientation (7–12). Subjective experiences and reported symptoms are often incongruent with the results of routine diagnostic tests of vestibular function and vary across individuals with similar diagnoses (13–20). This can be particularly troublesome for individuals with dizziness who present with normal vestibular function testing which can be the case for vestibular migraine, mal de debarquement syndrome, and persistent perceptual postural dizziness (21–23). It is interesting to note that several methods of vestibular perceptual testing are able to distinguish healthy individuals from individuals with vestibular disease (8, 24–29). Performance on some vestibular perception tests correlates with balance ability (30–32), which suggests clinical utility (33). However, the role of vestibular perceptual testing in the diagnosis and treatment of individuals with dizziness remains undefined, possibly in part due to the specialized equipment (34) or time involved in testing (35). In this review, we synthesize the existing literature describing several different forms of vestibular perceptual testing that range in application from the laboratory setting to clinical use.
We expand on the traditionally narrow definition of vestibular perception (movement detection or movement discrimination) to include spatial orientation, spatial navigation, and spatial cognition as vestibular signals are known to contribute to more global spatial perception\cognition and navigation (1, 36–44). In the first section, we briefly review the anatomical pathways contributing to vestibular perceptual abilities as well as review the historical and state of the art approaches to vestibular self-motion perception. In the second section, we review approaches to vestibular spatial orientation and verticality. In the third section, we review approaches to vestibular spatial navigation. In the fourth section, we review global perspectives on vestibular cognition. We conclude the review with the authors’ perspectives on clinical value and clinical utility as well as some potential recommendations for the future.
2. Vestibular perception: anatomy to laboratory testing
Vestibular perceptual thresholds are a laboratory-based technique used to measure an individual’s sensitivity to passive self-motion cues (33, 45). This is accomplished by measuring the ability to perceive a passive whole-body motion stimulus in the absence of visual feedback (e.g., blindfolded or in a light tight room) and with tactile and auditory cues minimized (e.g., noise cancelling headphones and padded seats). Although the specific test procedures and statistical approach used to estimate a threshold will ultimately determine the exact interpretation of the value reported, in general, an individual with a lower vestibular threshold should, on average, be able to reliably perceive smaller motion stimuli. A person’s “vestibular threshold” therefore serves as a behavioral measure of the sensitivity of the vestibular system to a specific vestibular motion stimulus (e.g., tilt, rotation, or translation). In this review we will briefly cover the methodology used to measure vestibular thresholds, and then provide an overview of normal and pathologic responses captured by vestibular thresholds. Interested readers are referred to prior, more comprehensive reviews that cover the experimental methods and statistical approach to estimating vestibular thresholds (33, 45–48).
The most common method for measuring vestibular thresholds is a direction recognition task (DRT) (45). In a DRT, an individual is passively moved and then asked to judge the direction of the motion stimulus (e.g., left vs. right or up vs. down). Although this is commonly done using a two-alternative forced choice task (e.g., deciding between a left and right yaw rotation), recent approaches have successfully used up to 12 alternative choices (6 motion planes and 2 directions for each) (35, 49). An adaptive staircase procedure — decreasing the size of the motion stimulus after a number of consecutive correct responses and increasing the size of the stimulus after an incorrect response (50) — is also typically used to efficiently sample stimulus values around an individual’s threshold (45). The vestibular threshold is then determined from these binary responses by taking either (a) the average of the terminal staircase reversals [e.g., averaging the stimuli at the step up (after an incorrect response) and the step down (after a number of correct responses) (7, 51, 52) or (b) by fitting the data to a psychometric function (30, 47, 53–56). Fitting the data to a psychometric function holds the advantage of using all of the participant’s responses, rather than only the final number of reversals, and also yields a threshold value with a clear physiological interpretation based upon signal detection theory (45, 57, 58). The methods described by Merfeld and colleagues (45, 46, 48) involve fitting the binary subject responses (e.g., right versus left) and stimulus magnitudes to a Gaussian cumulative distribution function (CDF) to estimate the 1σ (i.e., “one sigma”) threshold. This parameter corresponds to the stimulus magnitude that would on average be perceived accurately on ~84.1% of trials. Therefore, if a human observer has a yaw vestibular threshold of 0.5°/s, assuming zero bias, this individual should on average be able to accurately perceive the direction (e.g., right vs. left) of a 0.5°/s yaw rotation on 84.1% of trials. For an individual that instead has a threshold of 5°/s — putatively resulting from a bilateral vestibular lesion — the probability of correctly sensing the direction of the same 0.5°/s motion stimulus is nearly the same as the flip of a coin (~53%).
Based upon principles of signal detection theory, the 1σ parameter is equivalent to the width of the CDF, and as a result, the threshold parameter is proportional to the noise in an individual’s perceptual estimate of the motion stimulus [(see 45, 59, 60) for additional details on vestibular noise]. Vestibular thresholds can therefore describe either “sensory noise,” or “sensory precision,” with the two terms being inversely related to the threshold parameter — higher thresholds indicating increased noise and lower precision (59). Regardless of whether the threshold is interpreted as a measure of “sensitivity” or a measure of “sensory precision/noise,” the definitions are harmonious. The threshold parameter in actuality reflects the ratio between signal strength and sensory noise (i.e., the signal to noise ratio). As such, a lower signal to noise ratio, as represented by a higher threshold, will make the relevant motion stimulus harder to separate from internal sensory noise resulting in both (a) the need for a larger motion stimulus to permit an accurate perception of motion (lower sensitivity) and (b) greater variability in the subjective experience of the motion (imprecision or increased noise) [(see 59) for additional reading on this topic]. It is also worth mentioning that from these methods, a “bias” parameter that describes the mean of the CDF (i.e., the displacement of the CDF along the abscissa) is also estimated (45). However, in comparison to thresholds, vestibular bias has yet to be studied in-depth in patient populations, and as a result we focus the present review only on the threshold parameter.
Throughout this section of the review, the term “vestibular threshold” will also be more precisely specified based upon the motion trajectory (translation, rotation, or tilt), plane of motion (e.g., fore-aft, interaural, superior–inferior) and the frequency of the motion stimulus; since single cycles of sinusoidal acceleration are most often used as the motion stimulus, the stimulus frequency describes the inverse of the motion duration (e.g., 1 Hz = 1 s per motion, 0.2 Hz = 5 s per motion). The vestibular system is multi-modal, with five sensors within each labyrinth that collectively allow us to sense and response to rotations (via the semicircular canals), translations (via the otolith organs), and tilts (via a combination of canal and otolith inputs) of the head (61). The relative excitability of afferent neurons within the different end-organs is determined primarily by the type of motion (rotation versus translation) and frequency of the motion stimulus (61). As such, by carefully selecting the plane of motion and the stimulus frequency (30, 53, 56, 62), vestibular thresholds can preferentially quantify motion perception with predominant contributions from the different end-organ pairs, see Figure 1 for an example. Table 1 summarizes several motion profiles commonly used to assay vestibular perceptual thresholds with predominant contributions from the various end-organs. This list is not all inclusive, but is instead based upon a recent series of empirical studies (53, 56, 62–64).
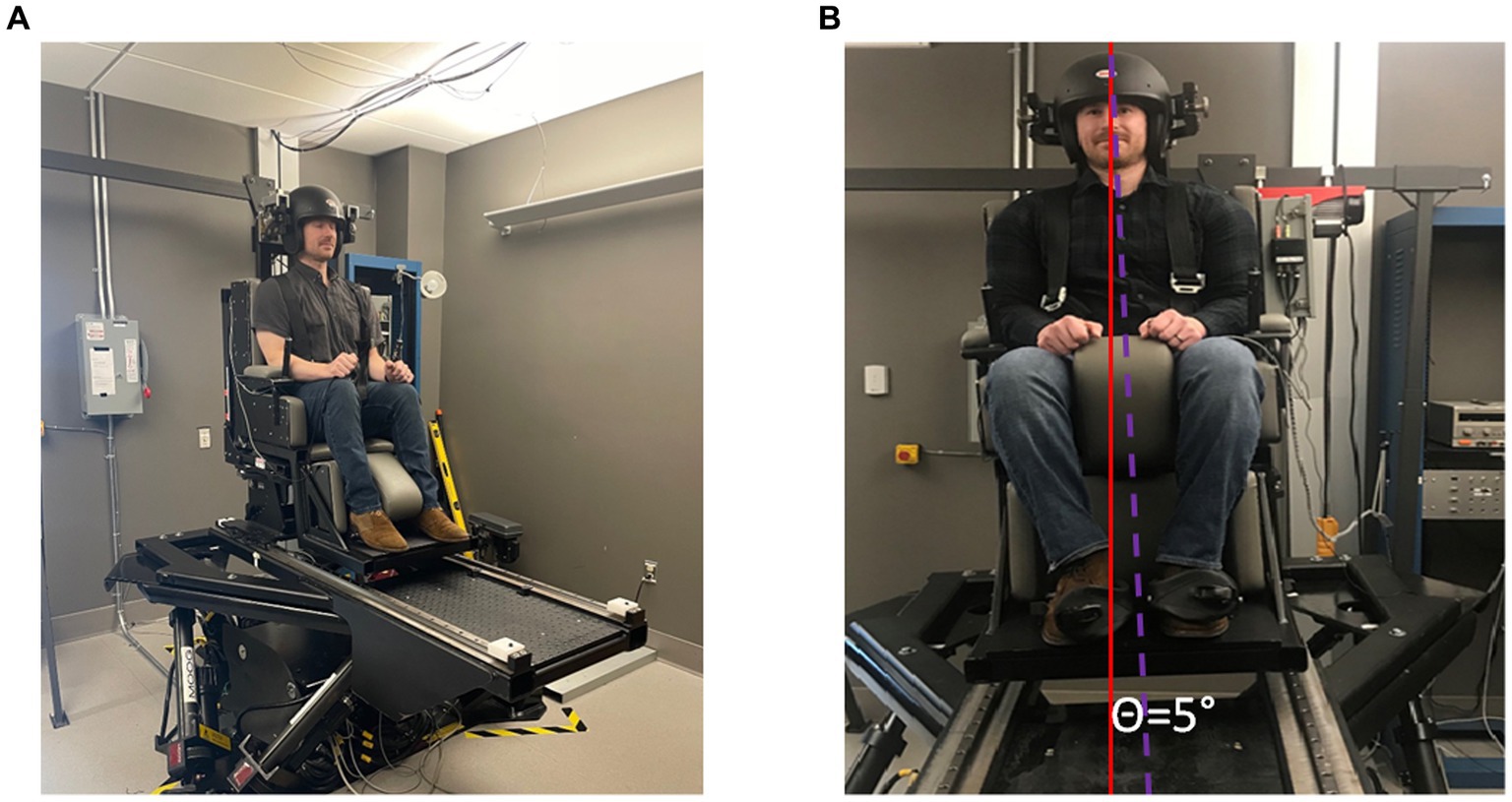
Figure 1. (A) Exemplar image of a subject sitting in a chair mounted to a hexapod base participating in an experiment to quantify roll-tilt threshold. Typically, this experiment would be conducted in the dark to emphasize vestibular signals of inertial motion. Subjects respond with a button press to indicate whether they tilted to the right or the left. (B) In this example the subject is tilted 5° to the right. The solid red line indicates earth vertical and the dashed purple line indicates body orientation after a head centered roll-tilt to the right.
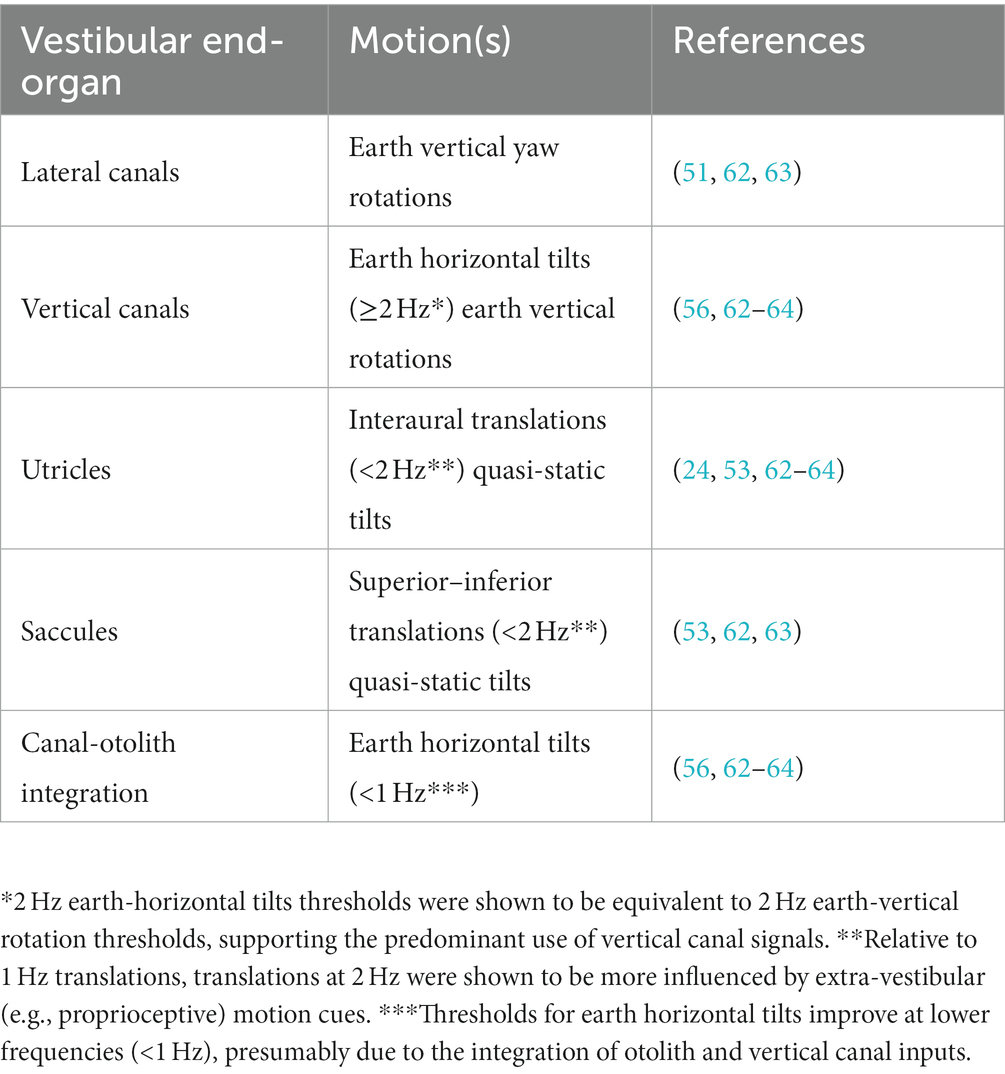
Table 1. Common motion profiles used to measure vestibular perception with predominant contributions from the different end organs.
2.1. Normative data and age effects
Bermúdez Rey et al. measured vestibular thresholds in 105 asymptomatic adults between 18 and 80 years of age (30). Direction recognition thresholds were measured for 1 Hz yaw rotations (lateral canal), 1 Hz interaural translations (utricles), 1 Hz superior–inferior translations (saccules), and roll tilt at 2 frequencies (1 Hz – vertical canals, 0.5 Hz – canal-otolith integration). A linear increase in each of the thresholds was observed beginning at approximately age 40 (30). The strongest effect seen was for superior–inferior thresholds, with an observed increase of 83% per decade (Table 1). However, by comparison yaw rotation thresholds were relatively spared, showing only a weak association with age (15% increase per decade). Although the study by Bermúdez Rey et al. is the largest to date, additional studies of smaller sample sizes similarly support (1) an age effect on vestibular translation thresholds, specifically interaural translation and superior–inferior translation thresholds (29, 54, 65), as well as (2) a weak association between age and yaw rotation thresholds (54, 66, 67). Only a single study (N = 28) showed a lack of an association between interaural threshold and age (68), however the study by Kingma and colleagues showed an effect of age on fore-aft translation thresholds and the study only included adults up to age 60, potentially reducing the strength of the age effect on interaural translation thresholds. This is consistent with epidemiology reports indicating reduced otolith function in the 7th and 8th decade (69).
Gabriel et al. recently used a two-interval detection task (“did you move in interval 1 or 2”) and a two-interval magnitude discrimination task (e.g., “was movement 1 or 2 larger”) to determine differences in 0.5 Hz superior–inferior translation and 0.5 Hz pitch tilt thresholds between young adult (N = 18) and older adult (N = 19) participants (70). They found that older adults demonstrated an increase in detection thresholds, but not discrimination thresholds, relative to young adults (70). This study also found that within the older adult group, superior–inferior detection thresholds, pitch detection thresholds, and pitch discrimination thresholds each showed significant correlations with quiet stance postural sway (70). Bermúdez Rey and colleagues also compared vestibular thresholds to “pass/fail” balance performance on the four conditions of the Modified Romberg Balance Test. In adults over the age 40 (N = 56), they showed that an individual’s 0.2 Hz roll tilt threshold (a test requiring the central integration of canal and otolith signals) was the strongest predictor of whether they were able to complete an “eyes closed, on foam” balance test (30, 31). This relationship between roll tilt thresholds and postural control has since been further characterized by studies showing significant correlations between roll tilt thresholds and quantitative measures of postural sway (32, 71). The specific link between roll tilt thresholds and postural control does however contrast with the findings of Gabriel et al. (70), as the study by Bermúdez Rey and colleagues did not find a significant association between balance performance and superior–inferior translation thresholds (30, 31, 72). Yet, the disparate methods used (two-interval detection and discrimination tasks versus a one-interval direction recognition task) prevents a direct comparison between the findings of the two studies.
As it represents the largest normative dataset currently available, Table 2 provides an overview of the threshold values per decade measured in the study by Bermúdez Rey et al. (30). Despite the healthy nature of the population [(see 30) for exclusionary criteria used] the thresholds measured displayed considerable variability within the cohort (30) (Figures 1–3). The source of variability in thresholds between asymptomatic adults may be indicative of subclinical changes to the vestibular system that cause an increases in thresholds prior to the onset of symptoms (60, 72) or may reflect the influence of confounding factors, unaccounted for in the current threshold assessment protocols. This remains an open area of investigation.
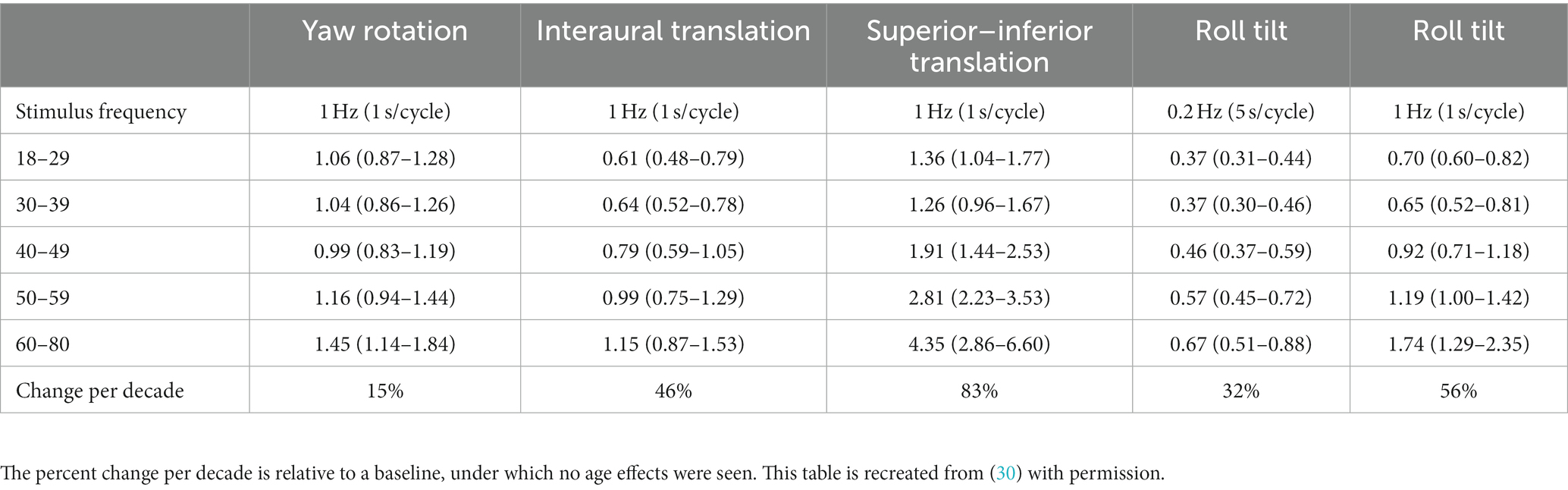
Table 2. Data presented show the geometric mean and 95% confidence intervals for each threshold measure for each age range (N = 105).
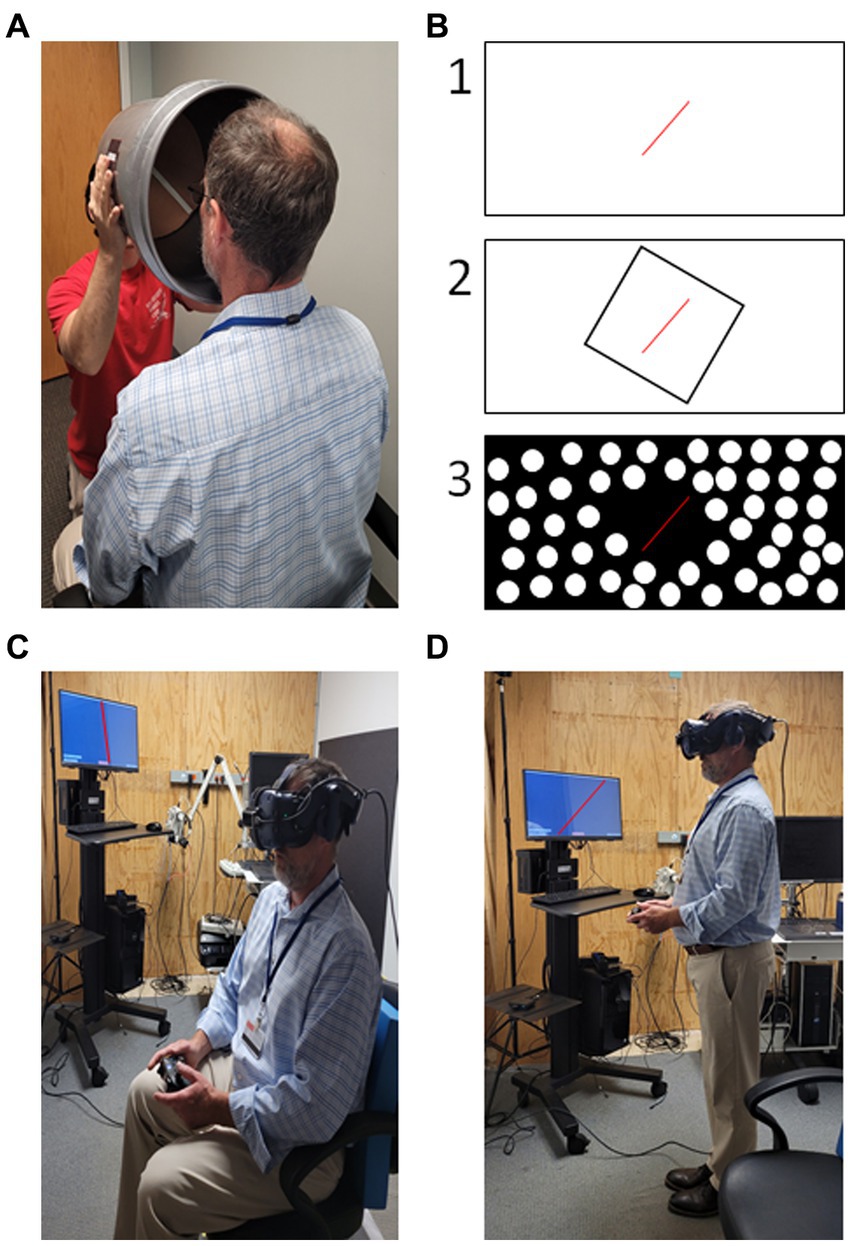
Figure 2. Examples of subjective visual vertical (SVV) testing. (A) Low-tech “bucket” method testing a seated subject (73). (B) Example styles of high-tech SVV testing with projection screens or using virtual reality, (1) tilted line on blank background; (2) “Rod and Frame” - tilted line presented in tilted square; (3) dynamic SVV – background of dots rotating clockwise or counter-clockwise with a tilted line in the center. (C) SVV performed in virtual reality while seated. (D) SVV performed in virtual reality while standing. Patient task in all scenarios is to indicate when the visible line is oriented with vertical either verbally or by button press. Photos in (C,D) digitally altered to improve visibility of the tilted red line presented on the computer monitor.
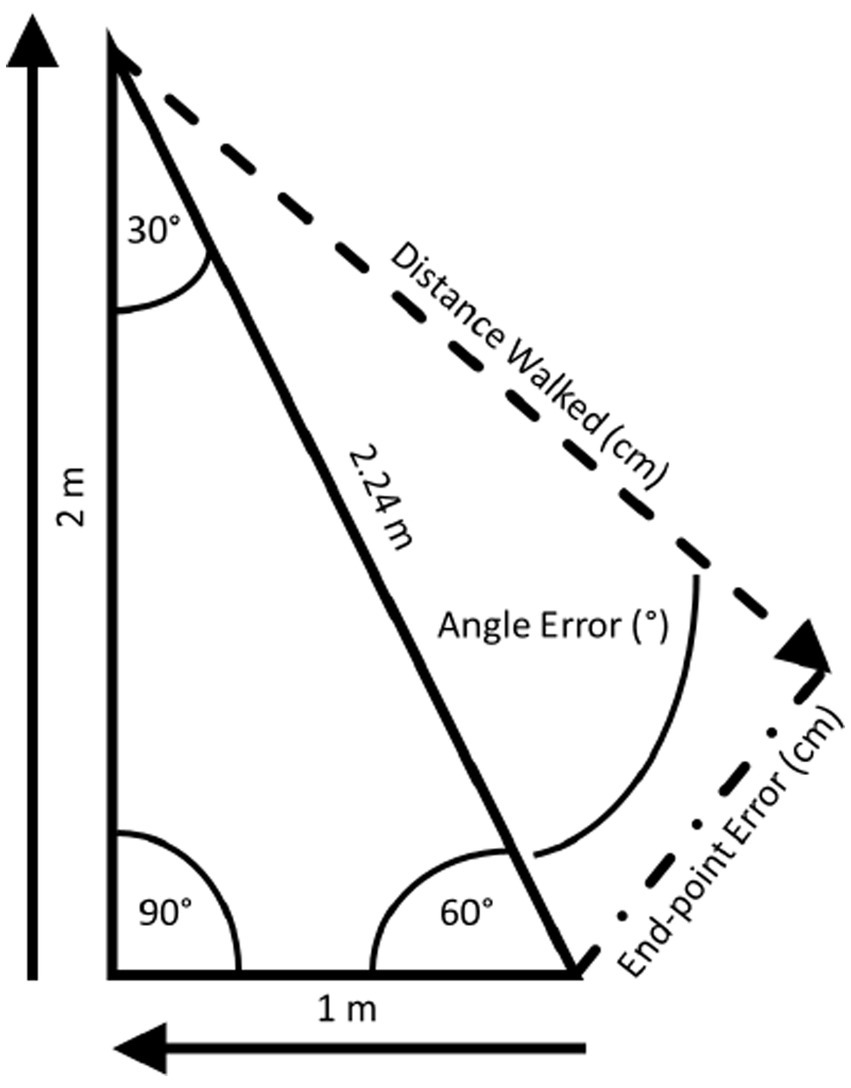
Figure 3. The triangle completion test (TCT): the TCT (74) is conducted using right triangles, the sides of which are distinctly marked on the floor. Participants don a blindfold and noise-attenuating headphones and, then, are instructed to visualize the triangle to be walked. Participants are passively guided along one leg, a 90° turn, and then the second leg. Once the examiner lets go, participants are instructed to “turn and complete the triangle”.
2.2. Vestibular hypofunction
Several studies have characterized the influence of peripheral vestibular hypofunction on vestibular thresholds. Due to the time required to complete a standard staircase procedure (i.e., to 50 to 200 trials over 8 to 30 min) (47), Cutfield et al. developed an abbreviated ‘time to response’ threshold task for the assessment of patients in the acute stages of vestibular neuritis (VN) (75). In contrast to the two-alternative forced choice task described above, individuals are asked to report the perceived direction of motion as quickly as possible, with the threshold being defined by the latency between the motion onset and a button press. Cutfield et al. (75) showed that patients with acute unilateral vestibular hypofunction (UVH) due to VN (N = 12) showed a significant elevation in yaw rotation thresholds, as indicated by a prolonged duration between motion onset and subject response. In a follow-up study, Cousins et al. similarly showed that individuals with acute UVH (N = 25) due to VN showed an elevation of vestibular thresholds relative to healthy controls (N = 30) within the first 1–5 days from symptom onset (76). Rotation thresholds were increased for both ipsilesional and contralesional rotations but showed a greater elevation toward the lesioned ear. Even after a period of presumed vestibular compensation, participants showed a consistent elevation in thresholds at 10 weeks from the time of lesion (76). Recently, Madhani et al. used a two-alternative forced choice task to measure yaw rotation thresholds in a group of 8 participants diagnosed with neurofibromatosis (NF-2) related schwannomatosis (N = 5 bilateral and N = 3 unilateral) and 38 participants with sporadic unilateral vestibular schwannomas (SWN) (77). They found that 1 Hz yaw rotation thresholds were elevated for both groups relative to healthy controls (N = 23) (77). In two of the patients with bilateral NF2-SWN, perceptual thresholds were also improved following treatment with chemotherapy (bevacizumab) (77). Since the healthy labyrinth provides a bidirectional response to yaw rotations secondary to an inhibitory response from the contralateral side, approaches such as those utilized by Roditi and Crane (54) that use independent staircases for right and left rotations may be of benefit in the assessment of vestibular hypofunction.
Prior to discussing the literature describing the impact of bilateral vestibular lesions on vestibular thresholds, a distinction must first be made between studies that include “bilateral vestibular hypofunction” (BVH) and studies that have intentionally recruited patients with a total bilateral vestibular loss due to bilateral vestibular labyrinthectomies (BVL). In BVH, the vestibular apparatus remains present, and as a result BVH is often characterized by an incomplete loss of vestibular responses bilaterally. Individuals who have instead experienced a bilateral labyrinthectomy lack a vestibular system, and as such, produce no vestibular response to motion. As a result, studies that include individuals with BVL have primarily been conducted as a means to describe vestibular contributions to motion perception (62, 63), rather than as a means to gain specific insights into a common patient population. Valko et al. found that patients with BVL (N = 3) had vestibular thresholds that were between 1.3 and 56.8 times greater than age-matched healthy controls (N = 14) with the magnitude of the difference depending upon both the specific motion direction (interaural translation, superior–inferior translation, yaw rotation, and roll tilt) and frequency (62). In a more recent study, Kobel and colleagues showed that vestibular thresholds for 2 individuals with BVL were between 2 and 35 times greater than healthy controls for a more comprehensive set of motions that included roll tilts (head rotated about an earth horizontal axis while sitting upright) and roll rotations (rotated about an earth vertical axis while supine) performed across a broad range of frequencies (0.2 to 2 Hz) (63). Together these studies highlight the dominance of vestibular inputs during vestibular thresholds assayed using a direction recognition task.
In individuals with BVH, early studies looking at motion perception were less conclusive. In the late 1990’s and early 2000’s, two studies that included four and five individuals with BVH respectively, found a lack of evidence to support a difference in translation (78) or tilt (79) perception relative to healthy adults. However later, Priesol et al. captured a more comprehensive battery of vestibular thresholds in four individuals that each had received a prior diagnosis of idiopathic BVH (26). They found that thresholds were not uniformly elevated across this sample, but instead individuals with BVH showed a selective increase in yaw rotation thresholds (assaying the lateral canals) and low frequency interaural translation thresholds (assaying the utricles) (26). Valko et al. similarly found a larger difference in translation thresholds at lower frequencies in individuals with BVL which suggests a potential increase in the contributions of non-vestibular inputs at the higher frequencies (62). Priesol et al. did not however show a significant difference between individuals with BVH and healthy controls for superior–inferior translation (assaying the saccules) or roll tilt (assaying central canal-otolith integration) thresholds. More recently, Shayman and colleagues did find a significant increase in 1 Hz yaw rotation thresholds for individuals with BVH (80), however this study included only 3 participants, and thus differences in the results of Priesol (n = 4) and Shayman (N = 3) may be a result of the small sample sizes. Since vestibular thresholds vary considerably between even healthy (asymptomatic) individuals (Table 2), the results of studies that include small sample sizes should be viewed with caution.
In two larger studies of adults with BVH (N > 30), van Stiphout et al. and Agrawal et al. found more global changes in vestibular thresholds (49, 65). Van Stiphout and colleagues measured thresholds for 3 planes of translation (fore-aft, interaural, and superior–inferior) and 3 planes of rotation/tilt (yaw rotation, pitch tilt, and roll tilt) in 37 adults with BVH and 34 healthy controls. In contrast to a standard two-alternative forced choice task, a 12 alternative choice task was used that required the participants to select both the direction (e.g., right vs. left, up vs. down) and type of motion (rotation vs. translation) experienced. The data were then analyzed within two subgroups, a middle-aged group aged 40–59 (N = 18 with BVH) and an older adult group aged 60–79 (N = 19 with BVH). With the exception of superior–inferior translation thresholds in the older adult group and roll tilt thresholds in the middle-aged group, all other thresholds measured were significantly elevated relative to age-matched healthy controls. Of note, superior–inferior translation thresholds are markedly increased in asymptomatic older adults (30) (Table 2), and thus, this may explain the lack of an effect of BVH of superior–inferior thresholds specifically in the older adult group. An additional study by Agrawal and colleagues found significant increases in interaural, fore-aft, and superior–inferior thresholds in individuals with BVH (N = 33) relative to healthy controls (N = 42) (65).
The principal take away from these studies is that vestibular thresholds are capable of detecting specific changes in vestibular self-motion perception in individuals with peripheral vestibular hypofunction. Since vestibular hypofunction may include varying levels of damage to the different branches of the vestibular nerve or to the individual vestibular end-organs, a characteristic change in a specific motion paradigm should not be assumed. Instead, these data highlight the need for future studies to characterize a comprehensive battery of threshold measures to fully capture the impact of UVH and BVH on the vestibular system.
2.3. Vestibular migraine and Meniere’s disease
Over the past decade, vestibular thresholds have been studied as a potential objective measure to help with the diagnosis of vestibular migraine (VM). Lewis et al. showed that individuals with VM (N = 8) demonstrated significantly lower 0.1 Hz roll tilt thresholds compared to both healthy controls (N = 8) and individuals with non-vertiginous migraine (N = 8) (7). Alternative vestibular thresholds that relied primarily upon either the semicircular canals (1 Hz dynamic roll tilt, 0.1 Hz supine roll rotation, and 1 Hz supine roll rotation) or the otoliths (quasi-static roll tilt) in isolation did not differ between patients with VM and the other groups. In a follow-up study, King et al. showed that individuals with VM (N = 12) displayed low to mid frequency (0.05 to 0.2 Hz) roll tilt thresholds that were significantly lower compared to individuals with non-vertiginous migraine (N = 12), healthy controls (N = 12), and individuals diagnosed with Meniere’s disease (MD; N = 8; only 0.2 Hz roll tilt thresholds were measured in this group) (24). Similar to the earlier study, thresholds for higher frequencies of roll tilt (≥1 Hz) as well interaural translations (0.2, 0.3, and 0.5 Hz) and supine roll rotations (0.2 and 0.5 Hz) were not significantly different between the individuals with VM and either the healthy controls or non-vertiginous migraineurs.
Due to the overlapping clinical presentations of VM and MD, additional studies have also attempted to use vestibular thresholds to differentiate between these two patient groups. Bremova et al. found that patients with MD (N = 27) demonstrated higher 1 Hz interaural, fore-aft, and superior–inferior translation thresholds compared to individuals with VM (N = 20); only superior–inferior and fore-aft thresholds were elevated relative to the healthy control group (N = 34) (29). Consistent with the findings of Lewis et al. (7) and King et al. (24), individuals with VM did not display differences in translation thresholds relative to healthy controls (29). Using a “time to response” task, Bednarczuk et al. did however find that patients with VM (N = 15) showed a longer response latency during earth vertical yaw rotations when compared to healthy controls (N = 15), as well as compared to individuals with non-vertiginous migraine (N = 15) (81). A group with benign paroxysmal positional vertigo (BPPV, N = 15) was also included and showed similar yaw rotation thresholds as the VM group (81). It is worth highlighting that this timed response task differs substantially from the forced choice direction recognition task used in the prior studies of individuals with VM.
From these data it can be concluded that vestibular thresholds in individuals with MD are consistent with a loss of sensitivity to self-motion cues, analogous to the effect of vestibular hypofunction. Conversely, individuals with VM show a higher sensitivity to low to mid frequency roll tilts (i.e., lower thresholds). Since the perception of a low to mid frequency roll tilt stimulus requires that the brain perform computations that combine angular velocity signals from the vertical semicircular canals with gravitoinertial acceleration signals from the otolith organs [(see 82–85) for greater details], these findings in individuals with VM have been posited to reflect abnormal central canal-otolith integration (7, 8, 24).
2.4. Traumatic brain injury
A traumatic brain injury (TBI) can be readily diagnosed by correlating symptom onset with a traumatic event. However, recently considerable attention has been given to further classifying individuals into concussion subtypes (e.g., vestibular, headache, cognitive) to enable early access to targeted medical and rehabilitative services (86, 87). Vestibular dysfunction following TBI is primarily determined by the subjective report of symptoms elicited by specific head motions (88); physiologic vestibular metrics that capture vestibular impairments have been more elusive (89). A recent study found that vestibular perceptual thresholds may aide in the identification TBI related vestibular impairment, referred to as “vestibular agnosia” (44). Seemungal and colleagues showed that in the acute phase after a TBI (N = 37), yaw rotation thresholds were significantly elevated relative to age-matched healthy controls (N = 37) (44). Individuals with elevated vestibular thresholds also showed an increase in postural sway (44), increasing the likelihood that the changes observed represented a change in vestibular function. However, the isolated assessment of yaw rotation thresholds restricts these data to describing only the influence of TBI on the processing of lateral canal signals, and the broader impact of TBI on the remaining vestibular modalities requires further study.
2.5. Benefits of vestibular thresholds
Vestibular thresholds are not currently available as a clinical assessment for individuals with suspected vestibular pathology. Nevertheless, thresholds hold several notable advantages over the currently available vestibular clinical assessments. One of the primary advantages to vestibular thresholds is the ability to quantify different vestibular modalities using a single experimental methodology. While vestibular thresholds are not free of confounding influences (e.g., attention, fatigue) these factors should similarly influence the perception of different motion paradigms (tilt, translation, or rotation thresholds) and as such, non-vestibular factors should not exert a disparate influence on translation versus rotation thresholds. By comparison, standard clinical metrics assess the semicircular canals by quantifying the vestibulo-ocular reflex (e.g., calorics, video head impulse testing) and the otolith organs by measuring vestibular evoked myogenic potentials (VEMPs). The marked differences in experimental methodologies (oculomotor versus electromyographic responses) introduces the potential for confounding factors that may have disparate influences on the specific canal or otolith assessments. The second fundamental advantage is that vestibular thresholds are the most direct method for specifically measuring vestibular sensation in human participants. Alternative vestibular assessments require that sensory dysfunction be inferred from changes in the sensorimotor response observed (e.g., increased sway, decreased slow phase eye velocity, absent myogenic potentials), and as a result, these measures represent changes in sensory function alongside a sensorimotor transformation and the integrity of the motor limb of the response.
2.6. Limitations of vestibular thresholds
Vestibular thresholds possess several limitations that may serve as a barrier to their eventual clinical implementation. There is currently an absence of standardized methodologies for measuring and analyzing thresholds. As a result, data collected in different laboratories should be compared with caution. An additional barrier to the use of thresholds as a diagnostic measure is the variability in thresholds within samples of healthy, asymptomatic adults. Since thresholds can vary widely in healthy adult populations (30), identifying cut-off values for vestibular pathology is challenging. It is however likely that this variability is a result of both natural variance within the population as well as variability inherent to the threshold assessment paradigms currently used. The standardization of methods, and the identification of “normative” data and cut-off values for vestibular pathology are critical steps needed to develop vestibular thresholds as a viable clinical assessment tool. Finally, the assessment of thresholds currently requires specialized equipment and software that are not at the present time available in clinical settings. It is however worth highlighting that the cost of a 6-degree-of-freedom motion platform is comparable to the cost of the computerized dynamic posturography platforms that are commonly found in many audiologic and physical therapy clinics. As such, if the clinical utility of vestibular thresholds can be established, this final limitation is unlikely to pose a substantial challenge to the clinical implementation of vestibular thresholds.
3. Vestibular perception: spatial orientation and verticality
Moving beyond detection and direction discrimination of self-movement, we next focus on vestibular contributions to spatial perception. Spatial orientation can be conceptualized as the ongoing estimate of the three-dimensional spatial relationship between self and the surrounding world which necessarily implies sensory integration (90–93), and one’s ability to update the spatial reference frame of self-relative to the world after moving within the world (94–97). This process depends on successful and accurate integration of egocentric (vision, vestibular, somatosensation) self-motion cues and allocentric spatial representations, such as landmarks (2, 98, 99). Not surprisingly, spatial orientation involves distributed higher cognitive processing (43, 100–104), and can be adversely impacted by cognitive impairment, stress, and mental health disorders (5, 105–107). For the purpose of this review, we will restrict this topic to vestibular contributions to spatial orientation: the otoliths and semicircular canals (37, 39, 95, 108–110). We also distinguish spatial orientation (rotation only) from directional linear translations (heading) that may be navigation specific. Spatial navigation will be discussed in the next section.
Rotational vestibular signals directly contribute to three-dimensional compass mapping of space (111, 112). Specifically semicircular canal inputs provide input to head direction cells via thalamic pathways (39, 113, 114), thought to directly contribute to our ability to recognize which direction we are currently facing. This head in space directional information combined with the inertial signal from motion allows for recognition of which direction we were previously facing as well as updating to the new spatial orientation (1, 28, 101, 108). Similar to frequency dependent non-linearity observed with the vestibulo-ocular reflex, spatial orientation is worse at low frequencies (99). Importantly, spatial updating depends on knowledge of results of the movement based on integrating sensory feedback or cognitive knowledge (108), and greater reliance on cognitive representations of space is needed when individuals are asked to delay their response rather than immediately respond (115). Rotational spatial orientation can be conceptualized based on the response question: “where\what am I currently facing” (1, 28, 108) versus “where\what was I facing” (99, 116). Responses vary by metrics from reporting degrees of rotation, analog clock positions, or allocentric world fixed objects as proxies for rotation.
3.1. Normative data and age effects
For small low frequency rotations (~16°) reorienting errors (“where were you facing”) in healthy subjects are biased in the direction of physical rotation (exaggerating displacement) but normalized at higher frequency rotations (99). For larger rotations, average errors in healthy adults are relatively small ~25° or less (1, 28, 108). There is conflicting information regarding the potential for an aging effect on spatial orientation updating. Jáuregui-Renaud initially found no relationship between age and spatial orientation updating ability (28). However, using a similar paradigm, we demonstrated an aging effect such that older individuals made larger errors than younger individuals independent of vestibular function (108). In a study that involved self-controlled rotation back to the origin following a rotation in the dark, Zachou and Bronstein identified an age-related decline in accuracy for the return to origin, but not for the more complex complete the circle condition (97). Importantly, this style of spatial orientation testing (rotational trajectory matching) may involve different cognitive processes and has the potential for increased sensory noise associated with aging to negatively impact accuracy on the spatial estimate for both the initial rotation and the return rotation (59). Taken together, these findings suggest that increased age has a negative influence on spatial orientation for relatively simple spatial orientation tasks, which may reflect the reduction in vestibular function associated with loss of hair cells and afferent neurons known to occur with aging (69, 117, 118). The lack of an aging effect for more complex tasks may indicate greater resilience to aging effects associated with higher cognitive processes that estimate space or time (119–121).
3.1.1. Vestibular hypofunction
Individuals with BVH demonstrate larger spatial errors during rotational testing compared to healthy individuals, tending towards underestimation for large amplitude spatial rotation tests (1, 28). Interestingly, individuals with UVH perform with similar spatial accuracy compared with healthy individuals, regardless of presence of dizziness. Intact vestibular function is linked to the ability to represent external space as well as accurate body spatial schema (122–124). This may explain why individuals with unilateral vestibular loss perform at levels similar to healthy individuals. The existing spatial orientation tests are performed at peak velocities below inhibitory cut-off, allowing afferent rotational signals from the intact ear to provide sufficient self-motion signals for spatial integration (125). In this context, any residual function should be sufficient to perform supra-threshold spatial orientation tasks with relative accuracy.
3.2. Verticality perception
The related concept of verticality (gravitational) perception can be evaluated using several different frameworks. Arguably the most common clinical method of verticality perception is subjective visual vertical (SVV) (126–129); although, subjective visual horizontal testing can also be examined (73, 130, 131). Visual vertical/horizontal perception involves orienting or judging a line with respect to the perceived direction of gravity (or orthogonal to gravity in the case of horizontal estimates) and implies integration of vision and otolithic function (127, 129, 130, 132). These methods are often applied as a solitary line in an otherwise featureless environment (133), but can also be presented with disorienting visual cues such as a tilted frame or rotating disc to evaluate the degree of visual dependence (134–137).
SVV has been found to be a valid and reliable perceptual test and is relatively easy to apply clinically (138–140). Unfortunately, abnormal percepts of verticality are not restricted to vestibular end-organ dysfunction (73, 131, 141, 142), indicating a lack of specificity from a diagnostic perspective. Recently, SVV was suggested to provide prognostic information related to resolution of BPPV (143), and within 6 months of VN errors in SVV has normalized (144).
SVV is most commonly examined in sitting, but can also be performed in standing, see Figure 2 for examples (145). Interestingly, verticality perception is also influenced by both static and dynamic body positioning suggesting integration beyond visual and vestibular contributions (25, 92, 132, 146–151). Importantly, SVV is a distinct construct from subjective body vertical (141). Differences in methodology such as reorienting the line versus indicating the direction of tilt (two-alternative forced choice) as well as whether the subject/patient performs the reorientation actively or halts an observed reorientation make it difficult to compare results across studies and are each prone to specific biases (152). Despite variations in test performance, SVV has excellent validity and reliability (133), thus making SVV testing very clinic appropriate.
3.2.1. Normative data and age effects
The normative values for SVV are less than 2° of error (153–155), and in some studies there is a suggestion that monocular viewing enhances test correlation with disease state (156). Although aging leads to reduced otolith function as assessed by VEMPs, there does not seem to be a consistent adverse effect of aging on static SVV accuracy (135, 155). In contrast, older adults make larger errors when tested with a dynamic rotating visual background during dynamic SVV testing (135), implying greater deficits in multisensory perceptual integration with age.
3.2.2. Vestibular hypofunction
Individuals with BVH have demonstrated larger visual biases with rod and frame testing and overall greater variability in response accuracy with head on body tilted positions compared to healthy individuals (157). Individuals with bilateral hypofunction, but preserved utricular function based on ocular VEMPs have lower variability and overall higher accuracy for SVV (158) than individuals with unilateral vestibular hypofunction.
3.3. Traumatic brain injury
Individuals with TBI often experience dizziness, not necessarily related to vestibular pathology (89, 159–161). It is unclear whether individuals with concussion\TBI experience spatial disorientation as a component of their dizziness, the existing knowledge is more related to navigation and cognitive challenges (12). Brainstem and cortex lesions are associated with larger errors on SVV testing, implicating central pathways are involved in processing these signals (142, 156). Approximately 40% of individuals with non-specific dizziness after a mild TBI demonstrate abnormally large errors on SVV testing (162). It is unclear whether subjective visual vertical abnormalities after TBI are due to peripheral, central or mixed causes reducing diagnostic specificity.
3.4. Implications of spatial and verticality testing in rehabilitation
Clinicians interested in including assessments of spatial orientation need to recognize the difference between (1) spatial orientation and (2) spatial updating, and select a test designed to evaluate the desired function. Spatial updating may provide a further window into vestibular cognition, but importantly should also involve progressive improvement due to the inclusion of “ground truth” knowledge of results provided after each movement via verbal feedback or multisensory integration (163–165). This would be characterized by reduction in errors over testing repetitions. For spatial orientation/updating tasks, rotational speed will be important as slower rotation velocities (lower frequencies) will lead to biased and more variable responses. Unlike the expensive equipment necessary for threshold testing, spatial orientation/updating examination can use a barber/salon style chair with a footrest, which may allow for greater clinical uptake. Importantly, spatial performance for individuals with VM and MD is unknown, and whether spatial orientation testing will have added clinical value remains to be determined.
Clinical testing for SVV can be implemented with the low tech “bucket test” (133), which provides widely available access. Virtual reality systems incorporating SVV testing have also become more affordable and widely available (166–169). This increased access combined with high reliability and validity makes testing visual vertical perception an ideal starting place for the clinician that seeks to implement vestibular perceptual testing.
3.5. Limitations of spatial and verticality perceptual testing
Currently there is no universal agreement for which style of spatial orientation question holds the most clinical relevance (“where am I” vs. “where was I”), nor is there an idealized response variable, or method for capturing that information (verbal report vs. rotating a dial). Further, the lack of discrimination between unilateral and bilateral vestibular hypofunction in the existing literature may relate to motion profiles below the threshold for semicircular canal inhibitory cut-off (~100°/s), which would allow the intact side to accurately detect motion direction and inertial qualities necessary to derive spatial orientation. The existing knowledge on accuracy ratings for health subjects may reflect testing interval bias (smallest intervals ~30 to 45°). To date, there is no published information regarding test–retest reliability or detectable change values. Due to the variation in application of spatial orientation across studies, clinicians should use and interpret these tests with caution. SVV testing has high reliability and validity; although, the spectrum of disease states that often have abnormally large errors is not restricted to peripheral vestibular disease (156, 170), reducing vestibular specificity. Methods for determining average error after SVV testing are also not consistent across studies with some papers recommending absolute rather than signed errors (171). Additional research is needed to optimize clinical utility for spatial and verticality perception prior to broad clinical adoption.
4. Vestibular perception: spatial navigation
Next, we turn our attention to spatial navigation, a complex sensorimotor skill that is required for accurate locomotion within the local (small-scale) and global (large-scale) environments. Egocentric (person-centered) and allocentric (world-centered) orientation processes must be integrated for accurate navigation. This section focuses on relevant data from vestibular-impaired adults who performed real-world spatial navigation tasks. Studies involving only healthy adults, sensory manipulations (e.g., galvanic stimulation), or in which solely virtual navigation was utilized are not included as our emphasis is on findings readily applicable to clinical practice.
Persons with peripheral (40, 74, 172–177), central (178–180), and age-related (181) vestibular dysfunction have impaired spatial navigation. Accuracy in spatial navigation is assessed using path integration tasks, during which a person moves along a specified path or towards a previously viewed target (102). Both linear (40, 172, 175, 176) and geometrically-shaped (182–184) paths have been utilized in prior studies in vestibular-impaired adults. Standardized tests that measure aspects of spatial navigation ability include the Triangle Completion Test (TCT) (182) and the Gait Disorientation Test (GDT) (185). Sense of direction is also commonly measured in studies of path integration, one such measure is the Santa Barbara Sense of Direction Scale (SBSODS) (186).
The TCT (182) is conducted using right triangles, the sides of which are distinctly marked on the floor (Figure 3). Before donning a blindfold and noise-attenuating headphones, participants are instructed to visualize the triangle to be walked. For each triangle, participants are passively guided along one leg, a 90° turn, and then the second leg. Once the examiner lets go, participants are instructed to “turn and complete the triangle” by walking its hypotenuse. The linear distance walked, linear end-point error (distance between where the participant stopped and the origin), and angle error (absolute value of the angular error with respect to the ideal rotation angle) are recorded. Recently, concerns about the reliability of the TCT have been raised by McLaren and colleagues (187) who found that the test–retest reliability for the distance walked was moderate but it was poor for end-point error and angle error.
The recently-developed GDT is a less complex navigation task that has excellent test–retest reliability. During the GDT, the participant is timed while walking 6.1 M with their eyes open and again with their eyes closed. Markings on the floor are used to facilitate timing (Figure 4). The result is the difference in time needed to walk that distance with eyes closed versus with eyes open. Although it has not yet been used as an outcome measure, the GDT result is proposed to be a composite measure of several determinants of spatial navigation (e.g., walking speed, spatial memory, and cognitive-perceptual processes) (185).
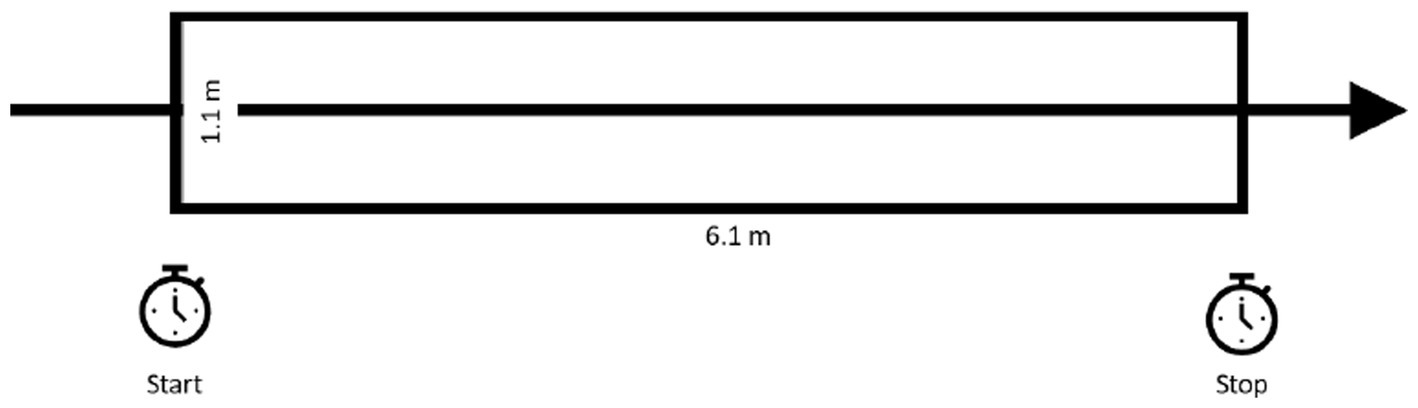
Figure 4. The gait disorientation test (GDT): during the GDT, the participant is timed while walking 6.1 M with their eyes open and again with their eyes closed. The GDT result is the difference in time needed to walk that distance with eyes closed versus with eyes open. GDT scores ≥4.5 s differentiate vestibular-impaired form healthy adults. Figure adapted from Grove et al. (185).
The SBSODS (186) is a 15-item questionnaire that consists of statements such as, “I have a very good sense of direction”; “I very easily get lost in a new city”; and “I have trouble understanding directions.” Each item is answered using a seven-point Likert scale, and negative statements are reverse-scored. A lower average score (range = 0–7 points) indicates worse self-reported sense of direction. Gandhi and colleagues (188) showed that reduced bilateral saccular function predicted lower scores on the SBSODS in a cohort of community-dwelling healthy older adults. Thus, vestibular afference appears to contribute to one’s perceived sense of direction ability.
4.1. Linear small-scale spatial navigation
In one of the earliest studies of the effects of vestibular loss on path integration, Glasauer et al. (189) studied goal-directed linear walking in healthy (N = 10) and bilaterally vestibular-impaired (N = 7) adults. Participants were tasked with walking straight ahead towards a target anchored to the floor 4 M ahead of them. Walking trials were performed at varying velocities and with either eyes open or while blindfolded. No significant between-group differences were found for distance walked errors; however, vestibular-impaired adults had significantly larger end-point errors. Vestibular-impaired adults also demonstrated significantly greater path curvature during blindfolded walking and significantly slower gait velocity compared with healthy adults. Despite the fact that vestibular-impaired participants were less stable and had larger end-point errors compared with healthy adults, the authors concluded that, because vestibular-impaired adults could perform the blindfolded task, the vestibular system was not necessary for active path integration. Vestibular afference appears to contribute to directional accuracy more so than distance accuracy.
Subsequently, Cohen (172) examined the performance of healthy adults (N = 24), individuals with vestibular SWN (N = 31), and individuals with chronic (≥3 months duration) peripheral vestibular impairments (N = 55) on a straight course. Those with vestibular SWN were tested pre-operatively and at weeks one and three post-operatively. Participants walked 7.62 M, once with eyes open and three times with eyes closed. The time needed to complete each trial, the distance walked before veering, and the angle of veering were recorded for each participant. Compared with healthy controls, SWN patients were significantly impaired on all measures. SWN patients also demonstrated a larger angle of veering and longer duration of trials compared to controls. Pre-operatively, SWN patients were significantly impaired compared to controls for the distance walked before veering. Compared with the pre-operative status, SWN patients were more impaired 1 week post-operatively for the angle of veering. Partial recovery of path integration ability was evident at week three post-operatively as individuals with SWN walked further before veering, veered less, and required less time to complete the task compared to week one post-operatively. These findings conflict with Glassauer et al. (189); however, differences in the length of the course may explain this discrepancy. Importantly, Cohen’s results suggest that central vestibular compensation partially mitigates path integration deficits following vestibular deafferentation.
4.2. Complex small-scale spatial navigation
Peruch et al. (40) evaluated spatial performance in unilaterally vestibular-impaired (N = 8) and healthy adults (N = 6) who were assessed on visual and non-visual navigation tasks. Performance was assessed at four time points before and at 1 week, 1 month, and 3 months after impaired participants underwent unilateral vestibular neurotomy. Healthy adults were assessed at similar time intervals. Participants first explored an environment in which four locations were marked by different objects. Then, participants attempted to navigate to those locations by reproducing the routes that were traversed during exploration, by reversing routes, or by taking shortcuts (making spatial inferences). Finally, participants performed identical navigation tasks in virtual reality while seated in front of a large projection screen. Navigation in the virtual environment was controlled by the participant using a joystick. Prior to surgery, vestibular-impaired participants had lower angle errors during real-world navigation when reproducing previously traversed routes compared with healthy controls. The authors postulated that patients were using compensatory strategies that relied on somatosensory afference prior to surgery to learn and reproduce routes. However, since no between-group differences were found for navigating with shortcuts prior to surgery, compensatory mechanisms were insufficient for higher-level spatial processing. Angle errors were also larger for patients compared to controls during virtual navigation, which suggests that vestibular afference must be integrated with visual cues for accurate navigation. Angle errors in real-world and virtual navigation increased after surgery for patients; however, between-group differences were seen only at 1 week post-operatively. Thus, in agreement with Cohen (172), central vestibular compensatory mechanisms may result in rapid restoration of spatial navigation ability following vestibular deafferentation.
Glasauer et al. (94) investigated the effects of vestibular dysfunction on complex path integration ability in healthy (N = 7) and vestibular-impaired (N = 5) adults. Vestibular-impaired participants had chronic (several months duration) symptoms and were assessed after the completion of vestibular rehabilitation. After viewing the course, participants walked a right triangular path (each leg length = 3 M). The task was completed in both the clockwise and counter-clockwise directions three times while blindfolded, then once with eyes open. Three-dimensional trajectories in 6° of freedom were collected from head-worn markers. The distance walked was similar to the required distance in both groups and there were no between-group differences. Additionally, there were no significant between-group differences in the arrival error at the first corner; however, there were significant between-group differences for the end-point error. Larger end-point errors in vestibular-impaired participants were attributed to angle errors. The authors postulated accuracy in path integration depends on proprioceptive and motor efference information related to turning corners and is enhanced by sensory input regarding angular velocity from the vestibular system. Interestingly, unilaterally and bilaterally vestibular-impaired participants had increased angular errors, and unilaterally involved participants had larger errors when turning towards their uninvolved side. The reason for this is not immediately clear, but larger variability in the lead time between the predictive head turn in advance of the turn may have been a factor for individuals with vestibular-impairments. Additionally, differences in walking and head turning velocities between the smaller first turn and the larger second turn may have influenced performance.
Péruch et al. (184) assessed path integration in unilaterally vestibular-impaired adults (N = 7). Participants were assessed 1 day prior, as well as 1 week and 1 month after unilateral vestibular neurectomy. Healthy, age-matched controls were also assessed at the same intervals. All participants were tasked with either (1) reproducing a right triangular path (leg length = 3 M) that had been walked with the examiner, (2) completing the reverse of the route walked with the examiner, or (3) taking a shortcut back to the origin. These tasks were performed in a real-world environment (while blindfolded) and in virtual reality (head-mounted display). Absolute and signed turn error, as well as absolute and signed distance error were measured. Unilateral vestibular deafferentation impaired the orientation component of spatial navigation, and the extent of these impairments differed depending on task complexity and on which sensory cues are available for interpretation. Performance on tasks with greater complexity significantly differed between healthy and vestibular-impaired adults. When performing more complex spatial navigation tasks, vestibular-impaired participants also showed greater distance errors than healthy controls. From these data, one can conclude that task complexity and which sensory inputs are available during performance are likely relevant for training path integration.
Guidetti et al. (174) assessed whether spatial navigation deficits persisted in adults with well-compensated UVH. In this study, vestibular-impaired (N = 50) and healthy (N = 50) adults were tasked with visually memorizing three different routes marked on the floor (a triangle, a circle, and a square), and, then, to walk these routes while blindfolded and again with vision. The time to complete each task was recorded. Additionally, participants completed a test of short-term visual–spatial memory and neuropsychological testing. Even well-compensated adults with vestibular-impairment required more time to complete the tasks with eyes closed compared with controls; however, there were no within-group differences in completion time for clockwise and counter-clockwise navigation. Vestibular-impaired participants also had worse visual–spatial ability and higher levels of anxiety and depression, which may have influenced spatial navigation performance.
Xie et al. (181) reported on the effects of age-related vestibular decline on spatial navigation ability. A total of 48 adults participated, including young healthy controls (N = 9), older healthy controls (N = 15), and older adults with dizziness (N = 24). All participants were tested for vestibular function using cervical VEMPs and the video head impulse test, they also completed the TCT. Results showed a step-wise increase in end-point errors from young controls to older controls to older participants with dizziness. Additionally, both control groups had smaller angle errors than the group with dizziness. End-point and angular errors were increased for participants (control or dizzy) with abnormal otolith and semicircular canal function. Thus, even sub-clinical changes in vestibular function may affect spatial navigation.
4.3. Complex large-scale navigation
Schoberl et al. (190) used a novel paradigm to assess real-world spatial navigation performance in adults with either complete or incomplete bilateral vestibular paresis and healthy matched controls. All participants performed standardized navigation tasks in an outpatient clinic. After a familiarization phase, the examiner cued participants to find specific landmarks without guidance and in particular sequences that required participants to retrace a familiar route, traverse a new route, or to take shortcuts. Ten minutes were allowed for the completion of 15 routes; if a participant could not locate a specific landmark, the examiner gave instructions to find the next item. The primary outcome was the error rate, defined as not being able to find, passing by, or ignoring a specific item. Gait velocity, saccades, and visual fixations were also recorded using a mobile eye tracking device. Adults with BVH\L performed worse than controls when navigating new routes, but there were no between-group differences in errors when retracing familiar routes. The number of errors was associated with the extent of vestibular loss. Additionally, compared to controls, adults with BVH\L had greater fluctuations in gait speed, spent less time at intersections, and utilized shortcuts less often. Further, adults with BVH\L had fewer gaze fixations and made horizontal head movements less often while traversing new routes compared with controls. Thus, persons with BVH\L may have more difficulty navigating and may report more disorientation in unfamiliar environments compared with familiar environments.
Dordevic et al. (191) studied cognition, spatial skills, and path integration in adults with unilateral or bilateral vestibular hypofunction (N = 15) and healthy matched controls (N = 15). All participants completed clinical vestibular tests of balance, the video head impulse test, caloric tests, the TCT, and whole-body rotational memory. Tests of visuo-constructive, spatial, attention, and concentration abilities were also administered using paper and pencil. Additionally, participants underwent imaging for structural brain analyses. Adults with UVH\BVH performed worse than controls on all clinical balance tests, whether these were conducted with eyes open or closed. Compared with controls, the impaired participants made greater distance errors on the TCT when passively transported in a wheelchair, and demonstrated worse rotational memory. However, there were no significant between-group differences in visuo-spatial, general cognitive abilities, and whole-brain or region-of-interest gray matter volumes. Although these results suggest that visuo-spatial and general cognitive abilities are preserved in persons with impaired vestibular cognition, a recent systematic review (192) provides summary evidence of the effects of vestibular loss on cognitive function.
Biju et al. (193) investigated the effects of vestibular deficits on route-based and place-based navigation in real-world and virtual environments. Adults with unilateral or bilateral vestibular hypofunction (N = 20) and matched controls (N = 20) navigated two routes in two environments, an outpatient clinic and virtual reality on a laptop computer. Prior to traversing the real-world routes, participants were familiarized with the route and specific landmarks, placed in a wheelchair and blindfolded, rotated around several times to disorient them, and, then, passively transported to the start via a random route. In the route-based task, participants were instructed to reproduce the route traversed during familiarization. During the place-based task, participants were asked to navigate to the landmark at the end of the route using the shortest path possible. Participants also completed a judgement of relative direction task in the real-world and on the laptop, as well as the SBSODS. Vestibular-impaired participants had higher path length ratios (required versus actual path) than controls. Path ratios were higher in the virtual compared to the real environment. Additionally, the place-based task resulted in higher path ratios compared with the route-based task. Regarding judgements of relative direction, there were no significant between-group differences; however, errors were greater in the virtual compared to the real environment. Although there was significant correlation between performance in real and virtual environments for controls, no such associations were found for vestibular-impaired participants. Additionally, no between-group differences were found for self-reported sense of direction. Together, these findings suggest that vestibular afference contributes to both route-based and place-based spatial navigation. Additionally, the authors postulated that, although healthy adults likely used the same strategies to navigate in real and virtual environments, vestibular-impaired participants employed different navigational strategies.
4.4. Spatial navigation training
Cohen and Kimball (194) were the first to assess whether vestibular rehabilitation leads to improvements in spatial navigation. These authors assessed path integration in adults with chronic peripheral vestibulopathies (N = 53) who completed 4 weeks of vestibular habituation exercises. In this study, the path integration task was the same as used by Cohen (172), described above. Performance was assessed pre-and post-rehabilitation. Gait velocity increased and the angle of veering decreased over time. Natural recovery is an unlikely confounder since all participants had reported brief episodes of vertigo elicited by head movement for at least 2 months prior to enrollment. The authors concluded that performing exercises that create visual-vestibular interaction leads to reduced spatial disorientation. Thus, one may not need to practice path integration tasks to achieve improvements in this domain.
Ishikawa and Zhou (195) assessed whether spatial navigation could be improved in a group of adults with self-reported poor sense of direction (N = 40) based on the SBSODS (186). Participants were assigned to perform path integration training with knowledge of results feedback, with (Group 1) or without (Group 2) allocentric training. The performance of these two groups was compared to that of a cohort of adults with average self-reported sense of direction. A test of mental rotation was also administered. All participants completed six weekly sessions in which they were tasked with actively walking one triangular, one quadratic, and one crossing path while blindfolded. Prior to traversing each path twice, participants who received allocentric training oriented to cardinal directions (e.g., north). Once they thought they had reached the origin, participants’ perceived location and orientation was recorded; then, participants received feedback about their accuracy. During each study visit, all participants in both groups also traversed one of six real-world routes in a residential district in Tokyo, Japan that were 450 M long and incorporated five turns and four easily recognizable landmarks. After completing the real-world route, participants were assessed for their accuracy in estimating the orientation of one landmark relative to another and the distance between sets of landmarks. The groups did not differ in mental rotation or path integration at the outset. Allocentric training improved the accuracy of direction estimates, and feedback alone led to improved accuracy in straight-line distance estimates and sketching route maps. Thus, translation between egocentric and allocentric representations is difficult and may not be readily trainable.
4.5. Implications for rehabilitation
The works reviewed herein lead to several conclusions. Interventions aimed at reducing misperceptions (non-specific dizziness/vertigo), like habituation, gaze stability training, and balance/gait activities (196), may lead to improvements in spatial navigation without task-specific training of path integration for individuals with vestibular hypofunction. Navigation training will likely produce task and/or environment specific effects; thus, the type of training should be individualized to address each patient’s specific deficits. Baseline cognitive ability and emotional state may influence navigational rehabilitation outcomes. The optimal training parameters for interventions aimed at improving path integration skills are not yet known; however, intensive training appears to facilitate robust improvements immediately post-training. Practicing path integration using routes of varying levels of difficulty and incorporating varying numbers of turns and path crossings may be a useful intervention, particularly if feedback regarding allocentric orientation is provided.
5. Vestibular perception: vestibular cognition
The domains of cognitive performance are not independent of each other. Within each domain there are often subdomains which refer to component cognitive abilities or processes. To fully understand a patient’s clinical presentation related to vestibular perception it is critical to consider the distinct contribution of all cognitive abilities or impairments. One challenge in synthesizing the literature related to the interplay between vestibular and cognitive function is the variability in the assessment methods used across investigations. Another challenge is discerning if multiple cognitive abilities are contributing to the cognitive performance and/or if the specific assessment method is truly measuring the domain that it was intended to assess.
It is important for clinicians to understand which domain of cognition is impaired because it could help determine specific interventions to include within the plan of care and/or determine how best to modify the delivery of vestibular interventions to optimize outcomes for their patients. Unfortunately, the best cognitive assessment methods for vestibular scientists and clinicians to use to measure each of the component cognitive abilities/processes is yet to be determined. Despite the discordance in assessment methods used, there is considerable evidence from human studies suggesting that multiple domains and subdomains of cognition (including: attention; executive function; memory; and visual spatial ability) are associated with vestibular disorders (102).
The findings related to cognitive processes that have been observed in investigations of common vestibular diagnoses including UVH, BVH, VM, and MD will be illustrated below. The specific assessment method for each finding will be indicated within parentheses following the cognitive ability/impairment. While navigation is considered a visual spatial ability, it was discussed separately in the previous section.
5.1. Unilateral vestibular hypofunction
Compared to healthy controls, some studies show that people with UVH display impaired attention, memory, and visual spatial ability. However, there are conflicting findings which could be due in part to the choice of assessment methods used and/or the specific component processes being assessed. A 2022 study reported that compared patients with healthy controls, people with UVH do not differ significantly in working memory abilities (digit span test), executive function (Stroop Task), or attention (Attention Network Test and Flanker Test) (197).
Attention impairments were however reported in adults with UVH (n = 15) using a dual-task paradigm that measured concurrent postural demands and cognitive tasks (reaction time) (198). Redfern et al. found that during concurrent balance or vestibular tasks people with UVH displayed a delayed response and decreased accuracy for the cognitive task when the postural or vestibular challenge was added. Another study also found that people with UVH (N = 14) also displayed decreased cognitive performance during dual-task gait challenges compared to healthy controls (199). Both investigations yielded similar conclusions suggesting that the brain prioritizes attentional resources to maintain balance and motor tasks at the expense of other cognitive tasks.
Visuospatial ability impairments (visual scanning reaction time test and processing speed tasks) have been reported in people with UVH (N = 16) when compared to healthy controls (200). A 2020 investigation of people with acute UVH (N = 21) also reported impaired visuospatial ability (Benton’s Judgment of Line Orientation test), attention and psychomotor speed (verbal and non-verbal cancellation tests), and short-term memory and mental manipulation (backward digit span) when compared to healthy controls (201).
Conversely, two other studies found no differences in visuospatial cognitive processes. Spatial perception (SVV including rod and frame conditions) (124), spatial memory also did not differ and the hippocampal volume did not significantly differ in people with UVH (202). Another study of people with acute UVH (N = 28) showed that visuo-spatial ability (line bi-section task, Albert’s test, Bell’s test, and a figure copying task) was not clinically impaired (203).
5.2. Bilateral vestibular hypofunction
Compared to healthy controls, people with BVH have been shown to have impaired attention, executive function, memory, and visual spatial ability. It is appreciated that cognitive impairments are typically more pronounced in BVH compared to UVH (200).
A recent 2022 study reported clinically meaningful lower scores for global cognition (RBANS-H total scale) in people with BVH (N = 34) compared to healthy controls (N = 34) (204). Those authors reported that the subdomains of memory, visuospatial cognition, and attention on the RBANS-H assessment were impaired in BVH but there were no differences observed with language or delayed memory subdomains (204). Attention impairment has also been demonstrated using a walking and cognitive dual task paradigm, whereby both walking and cognitive performance was degraded for people with BVH (N = 12) compared to healthy controls (N = 12) (205).
Another investigation revealed that people with BVH (N = 18) had impaired short-term memory (Theory of Visual Attention), executive function (Backward Corsi Block Tapping test), processing speed (Stroop Color-Word test), and visuospatial abilities (visual scanning reaction time) compared to people with UVH (N = 16) and healthy controls (N = 17) (200).
Significant spatial memory deficits (computerized virtual water Morris maze task) associated with bilateral hippocampal atrophy have been observed in people with BVH (173, 177, 206). While there was not a difference between healthy controls and people with UVH, investigators of another study reported that people with BVH have worse spatial imagery (mental body transformations) (124).
5.3. Vestibular migraine and Meniere’s disease
There is conflicting evidence regarding cognitive impairment in people with MD. A 2023 investigation of cognition using the Montreal Cognitive Assessment (MoCA) scale people with MD (N = 30) and healthy controls (N = 17) found significantly lower memory (MoCA memory sub score) and overall cognition (MoCA total score) in the people with MD (207). Conversely, another 2023 study concluded that neither MD (N = 19) or VM (N = 19) have significant differences in cognition compared to healthy controls (208). The domains of cognition assessed in the study of people with VM and MD included: global mental status (Mini Mental State Examination); working memory and attention (Stroop test); spatial cognition (Benton’s Judgement of Line Orientation test); and working memory, visual processing, visuospatial skills, attention, and processing speed (Trail Making Test) (208). The lack of differences could be due to the episodic nature of these diagnoses and confounding accompanying symptoms that are associated with VM and MD and/or the choice of the assessment method used to measure cognition.
5.4. Implications for rehabilitation
Notably, there are many factors that can impact cognition in people with vestibular disorders including acuity of the vestibular diagnosis, age, anxiety, depression, hearing, medications, sleep hygiene. Additionally, poorer self-reported sense of direction has been shown to be significantly associated with vestibular impairment (188). Regardless of the cause of the interplay between cognitive and vestibular function seen in common vestibular diagnoses which has been proposed in the literature (209, 210), appreciating the common co-occurrence of cognitive and vestibular impairments could help clinicians develop therapeutic strategies to facilitate recovery.
The impact of cognitive impairment on vestibular rehabilitation outcomes is not well understood. Although cognitive impairment may not alter the physiology of vestibular compensation, it could impact recovery if it interferes with a person’s ability to comply with exercises and recall what he/she has previously learned in therapy. Micarelli et al. suggested worse long-term function in people with cognitive impairment (211). A modification for the delivery of vestibular rehabilitation that is in alignment with clinical practice guidelines has been published that emphasizes motor learning abilities for people with cognitive impairment (212). Utilizing modified vestibular therapy interventions is currently being investigated in people with Alzheimer’s disease (213).
Future work should aim to determine the best clinically feasible assessment methods to screen and assess cognition for people with vestibular disorders. It is proposed that working memory should be assessed in people with peripheral vestibular disorders (214). An important clinically relevant conclusion from a 2023 dual task investigation during functional gait performance in people with chronic vestibular disorders reiterated the importance of measuring cognition in people with vestibular disorders to ensure the inclusion of appropriate interventions (215). Identifying the specific cognitive abilities/processes that are impaired will allow development of targeted interventions to optimize outcomes for people with vestibular disorders.
6. Summary and conclusions
As of this writing, few studies have examined the clinical application of vestibular perception in any form despite the extensive literature demonstrating disease state discrimination. Threshold testing in current form has limited clinical availability primarily due to the equipment and space requirement. Despite this limitation, roll thresholds are associated with postural control and future development of more clinic friendly perceptual assessments is needed. Spatial orientation and navigation testing may have greater clinical utility, but current testing methods have either unclear or low test–retest reliability. Screening for neurocognitive function and other forms of vestibular function has a place in vestibular rehabilitation, but additional research to determine clinical validity and reliability is needed to expand integration beyond limited use for initial clinical screening. Additional work is also needed to characterize the exercise interventions that optimally improve perceptual deficits for individuals with vestibular disease.
Ethics statement
Written informed consent was obtained from the individual(s) for the publication of any identifiable images or data included in this article.
Author contributions
CG: Funding acquisition, Methodology, Writing – original draft, Writing – review & editing. BK: Funding acquisition, Methodology, Writing – original draft, Writing – review & editing. AW: Funding acquisition, Methodology, Writing – original draft, Writing – review & editing. EA: Conceptualization, Funding acquisition, Methodology, Supervision, Writing – original draft, Writing – review & editing.
Funding
The author(s) declare financial support was received for the research, authorship, and/or publication of this article. EA was supported in part by the National Institutes of Health (NIDCD K23 DC018303). AW was supported in part by the National Institutes of Health (NIDCD K99 DC020759). BK was supported in part by the National Institutes of Health (NIDCD K23 DC020215-01). CG was supported by the Department of Defense, Congressionally Directed Medical Research Programs (W8lXWH-l7-CTRR-CTA).
Conflict of interest
The authors declare that the research was conducted in the absence of any commercial or financial relationships that could be construed as a potential conflict of interest.
Publisher’s note
All claims expressed in this article are solely those of the authors and do not necessarily represent those of their affiliated organizations, or those of the publisher, the editors and the reviewers. Any product that may be evaluated in this article, or claim that may be made by its manufacturer, is not guaranteed or endorsed by the publisher.
References
1. Cohen, BS, Provasi, J, Leboucher, P, and Israël, I. Effects of vestibular disorders on vestibular reflex and imagery. Exp Brain Res. (2017) 235:2181–8. doi: 10.1007/s00221-017-4959-7
2. Miller, AMP, Mau, W, and Smith, DM. Retrosplenial cortical representations of space and future goal locations develop with learning. Curr Biol. (2019) 29:2083–2090.e4. doi: 10.1016/j.cub.2019.05.034
3. Kerr, B, Condon, SM, and McDonald, LA. Cognitive spatial processing and the regulation of posture. J Exp Psychol Hum Percept Perform. (1985) 11:617–22. doi: 10.1037/0096-1523.11.5.617
4. Zanchi, S, Cuturi, LF, Sandini, G, and Gori, M. How much I moved: robust biases in self-rotation perception. Atten Percept Psychophys. (2022) 84:2670–83. doi: 10.3758/s13414-022-02589-x
5. Alcantara-Thome, M, Miguel-Puga, JA, and Jauregui-Renaud, K. Anxiety and motion sickness susceptibility may influence the ability to update orientation in the horizontal plane of healthy subjects. Front Integr Neurosci. (2021) 15:742100. doi: 10.3389/fnint.2021.742100
6. Zaback, M, Reiter, ER, Adkin, AL, and Carpenter, MG. Initial experience of balance assessment introduces ‘first trial’ effects on emotional state and postural control. Gait Posture. (2021) 88:116–21. doi: 10.1016/j.gaitpost.2021.05.013
7. Lewis, RF, Priesol, AJ, Nicoucar, K, Lim, K, and Merfeld, D. Dynamic tilt thresholds are reduced in vestibular migraine. J Vestib Res. (2011) 21:323–30. doi: 10.3233/VES-2011-0422
8. Wang, J, and Lewis, RF. Abnormal tilt perception during centrifugation in patients with vestibular migraine. J Assoc Res Otolaryngol JARO. (2016) 17:253–8. doi: 10.1007/s10162-016-0559-7
9. Dai, M, Cohen, B, Smouha, E, and Cho, C. Readaptation of the Vestibulo-ocular reflex relieves the mal De Debarquement syndrome. Front Neurol. (2014) 5:124. doi: 10.3389/fneur.2014.00124
10. Miller, MA, and Crane, BT. Roll vection in migraine and controls using inertial nulling and certainty estimate techniques. PLoS One. (2017) 12:e0171332–2. doi: 10.1371/journal.pone.0171332
11. Panichi, R, Faralli, M, Bruni, R, Kiriakarely, A, Occhigrossi, C, Ferraresi, A, et al. Asymmetric vestibular stimulation reveals persistent disruption of motion perception in unilateral vestibular lesions. J Neurophysiol. (2017) 118:2819–32. doi: 10.1152/jn.00674.2016
12. Catena, RD, van Donkelaar, P, Halterman, CI, and Chou, L-S. Spatial orientation of attention and obstacle avoidance following concussion. Exp Brain Res. (2009) 194:67–77. doi: 10.1007/s00221-008-1669-1
13. Bhansali, SA, Stockwell, CW, and Bojrab, DI. Oscilopsia in patients with loss of vestibular function. Otolaryngol Head Neck Surg. (1993) 109:120–5. doi: 10.1177/019459989310900122
14. Anson, ER, Kiemel, T, Carey, JP, and Jeka, JJ. Eye movements are correctly timed during walking despite bilateral vestibular hypofunction. J Assoc Res Otolaryngol. (2017) 18:589–600. doi: 10.1007/s10162-017-0626-8
15. Guinand, N, Pijnenburg, M, Janssen, M, and Kingma, H. Visual acuity while walking and oscillopsia severity in healthy subjects and patients with unilateral and bilateral vestibular function loss. Arch Otolaryngol Head Neck Surg. (2012) 138:301–6. doi: 10.1001/archoto.2012.4
16. Grunfeld, EA, Morland, AB, Bronstein, AM, Gresty, MA, and Bronstein, CAM. Adaptation to oscillopsia a psychophysical and questionnaire investigation. Brain. (2000) 123:277–90. doi: 10.1093/brain/123.2.277
17. Stewart, VM, Mendis, MD, and Low Choy, N. A systematic review of patient-reported measures associated with vestibular dysfunction. Laryngoscope. (2018) 128:971–81. doi: 10.1002/lary.26641
18. Jacobson, GP, and Newman, CW. The development of the dizziness handicap inventory. Arch Otolaryngol Head Neck Surg. (1990) 116:424–7. doi: 10.1001/archotol.1990.01870040046011
19. McCaslin, DL, Jacobson, GP, Grantham, SL, Piker, EG, and Verghese, S. The influence of unilateral saccular impairment on functional balance performance and self-report dizziness. J Am Acad Audiol. (2011) 22:542–9. doi: 10.3766/jaaa.22.8.6
20. Jacobson, GP, McCaslin, DL, Piker, EG, Gruenwald, J, Grantham, S, and Tegel, L. Insensitivity of the Romberg test of standing balance on firm and compliant support surfaces to the results of caloric and VEMP tests. Ear Hear. (2011) 32:e1–5. doi: 10.1097/AUD.0b013e31822802bb
21. Staab, JP, Eckhardt-Henn, A, Horii, A, Jacob, R, Strupp, M, Brandt, T, et al. Diagnostic criteria for persistent postural-perceptual dizziness (PPPD): consensus document of the committee for the classification of vestibular disorders of the Bárány society. J Vestib Res. (2017) 27:191–208. doi: 10.3233/VES-170622
22. Lempert, T, Olesen, J, Furman, J, Waterston, J, Seemungal, B, Carey, J, et al. Vestibular migraine: diagnostic criteria. J Vestib Res Equilib Orientat. (2012) 22:167–72. doi: 10.3233/VES-2012-0453
23. Cha, YH, Baloh, RW, Cho, C, Magnusson, M, Song, JJ, Strupp, M, et al. Mal de débarquement syndrome diagnostic criteria: consensus document of the classification Committee of the Bárány Society. J Vestib Res. (2020) 30:285–93. doi: 10.3233/VES-200714
24. King, S, Priesol, AJ, Davidi, SE, Merfeld, DM, Ehtemam, F, and Lewis, RF. Self-motion perception is sensitized in vestibular migraine: pathophysiologic and clinical implications. Sci Rep. (2019) 9:–14323. doi: 10.1038/s41598-019-50803-y
25. Winnick, AA, Wang, C-H, Ko, Y-H, and Chang, T-P. Subjective visual vertical imprecision during lateral head tilt in patients with chronic dizziness. Exp Brain Res. (2021) 240:199–206. doi: 10.1007/S00221-021-06247-W
26. Priesol, AJ, Valko, Y, Merfeld, DM, and Lewis, RF. Motion perception in patients with idiopathic bilateral vestibular hypofunction. Otolaryngol Head Neck Surg. (2014) 150:1040–2. doi: 10.1177/0194599814526557
27. Wurthmann, S, Holle, D, Obermann, M, Roesner, M, Nsaka, M, Scheffler, A, et al. Reduced vestibular perception thresholds in persistent postural-perceptual dizziness – a cross-sectional study. BMC Neurol. (2021) 21:394. doi: 10.1186/s12883-021-02417-z
28. Jáuregui-Renaud, K, Sang, FYP, Gresty, MA, Green, DA, and Bronstein, AM. Depersonalisation/derealisation symptoms and updating orientation in patients with vestibular disease. J Neurol Neurosurg Psychiatry. (2008) 79:276–83. doi: 10.1136/jnnp.2007.122119
29. Bremova, T, Caushaj, A, Ertl, M, Strobl, R, Böttcher, N, Strupp, M, et al. Comparison of linear motion perception thresholds in vestibular migraine and Menière’s disease. Eur Arch Otorhinolaryngol. (2016) 273:2931–9. doi: 10.1007/s00405-015-3835-y
30. Bermúdez Rey, MC, Clark, TK, Wang, W, Leeder, T, Bian, Y, and Merfeld, DM. Vestibular perceptual thresholds increase above the age of 40. Front Neurol. (2016) 7:162. doi: 10.3389/fneur.2016.00162
31. Karmali, F, Bermúdez Rey, MC, Clark, TK, Wang, W, and Merfeld, DM. Multivariate analyses of balance test performance, vestibular thresholds, and age. Front Neurol. (2017) 8:578. doi: 10.3389/fneur.2017.00578
32. Wagner, AR, Kobel, MJ, and Merfeld, DM. Impact of canal-otolith integration on postural control. Front Integr Neurosci. (2021) 15:773008. doi: 10.3389/fnint.2021.773008
33. Kobel, MJ, Wagner, AR, Merfeld, DM, and Mattingly, JK. Vestibular thresholds: a review of advances and challenges in clinical applications. Front Neurol. (2021) 12:643634. doi: 10.3389/fneur.2021.643634
34. Starkov, D, Strupp, M, Pleshkov, M, Kingma, H, and van de Berg, R. Diagnosing vestibular hypofunction: an update. J Neurol. (2021) 268:377–85. doi: 10.1007/s00415-020-10139-4
35. Dupuits, B, Pleshkov, M, Lucieer, F, Guinand, N, Pérez Fornos, A, Guyot, JP, et al. A new and faster test to assess vestibular perception. Front Neurol. (2019) 10:707. doi: 10.3389/fneur.2019.00707
36. Mackrous, I, Carriot, J, Cullen, KE, and Chacron, MJ. Neural variability determines coding strategies for natural self-motion in macaque monkeys. elife. (2020) 9:e57484. doi: 10.7554/eLife.57484
37. Yoder, RM, and Taube, JS. Head direction cell activity in mice: robust directional signal depends on intact otolith organs. J Neurosci. (2009) 29:1061–76. doi: 10.1523/JNEUROSCI.1679-08.2009
38. Harvey, RE, Rutan, SA, Willey, GR, Siegel, JJ, Clark, BJ, and Yoder, RM. Linear self-motion cues support the spatial distribution and stability of hippocampal place cells. Curr Biol. (2018) 28:1803–1810.e5. doi: 10.1016/j.cub.2018.04.034
39. Muir, GM, Brown, JE, Carey, JP, Hirvonen, TP, Della Santina, CC, Minor, LB, et al. Disruption of the head direction cell signal after occlusion of the semicircular canals in the freely moving chinchilla. J Neurosci. (2009) 29:14521–33. doi: 10.1523/JNEUROSCI.3450-09.2009
40. Peruch, P, Borel, L, Gaunet, F, Thinus-Blanc, G, Magnan, J, and Lacour, M. Spatial performance of unilateral vestibular defective patients in nonvisual versus visual navigation. J Vestib Res Equilib Orientat. (1999) 9:37–47.
41. Borel, L, Lopez, C, Péruch, P, and Lacour, M. Vestibular syndrome: a change in internal spatial representation. Clin Neurophysiol. (2008) 38:375–89. doi: 10.1016/j.neucli.2008.09.002
42. Borel, L, Harlay, F, Lopez, C, Magnan, J, Chays, A, and Lacour, M. Walking performance of vestibular-defective patients before and after unilateral vestibular neurotomy. Behav Brain Res. (2004) 150:191–200. doi: 10.1016/S0166-4328(03)00257-2
43. Smith, PF. The vestibular system and cognition. Curr Opin Neurol. (2017) 30:84–9. doi: 10.1097/WCO.0000000000000403
44. Calzolari, E, Chepisheva, M, Smith, RM, Mahmud, M, Hellyer, PJ, Tahtis, V, et al. Vestibular agnosia in traumatic brain injury and its link to imbalance. Brain J Neurol. (2021) 144:128–43. doi: 10.1093/brain/awaa386
45. Merfeld, DM. Signal detection theory and vestibular thresholds: I. basic theory and practical considerations. Exp Brain Res. (2011) 210:389–405. doi: 10.1007/s00221-011-2557-7
46. Lim, K, and Merfeld, DM. Signal detection theory and vestibular perception: II. Fitting perceptual thresholds as a function of frequency. Exp Brain Res. (2012) 222:303–20. doi: 10.1007/s00221-012-3217-2
47. Karmali, F, Chaudhuri, SE, Yi, Y, and Merfeld, DM. Determining thresholds using adaptive procedures and psychometric fits: evaluating efficiency using theory, simulations, and human experiments. Exp Brain Res. (2016) 234:773–89. doi: 10.1007/s00221-015-4501-8
48. Chaudhuri, SE, and Merfeld, DM. Signal detection theory and vestibular perception: III. Estimating unbiased fit parameters for psychometric functions. Exp Brain Res. (2013) 225:133–46. doi: 10.1007/s00221-012-3354-7
49. van Stiphout, L, Lucieer, F, Pleshkov, M, Van Rompaey, V, Widdershoven, J, Guinand, N, et al. Bilateral vestibulopathy decreases self-motion perception. J Neurol. (2021) 269:5216–28. doi: 10.1007/s00415-021-10695-3
50. Leek, MR. Adaptive procedures in psychophysical research. Percept Psychophys. (2001) 63:1279–92. doi: 10.3758/BF03194543
51. Grabherr, L, Nicoucar, K, Mast, FW, and Merfeld, DM. Vestibular thresholds for yaw rotation about an earth-vertical axis as a function of frequency. Exp Brain Res. (2008) 186:677–81. doi: 10.1007/s00221-008-1350-8
52. Lee, TL, Shayman, CS, Oh, Y, Peterka, RJ, and Hullar, TE. Reliability of vestibular perceptual threshold testing about the yaw Axis. Ear Hear. (2020) 41:1772–4. doi: 10.1097/AUD.0000000000000859
53. Kobel, MJ, Wagner, AR, and Merfeld, DM. Impact of gravity on the perception of linear motion. J Neurophysiol. (2021) 126:875–87. doi: 10.1152/jn.00274.2021
54. Roditi, RE, and Crane, BT. Directional asymmetries and age effects in human self-motion perception. J Assoc Res Otolaryngol. (2012) 13:381–401. doi: 10.1007/s10162-012-0318-3
55. Keywan, A, Dietrich, H, and Wuehr, M. Subliminal passive motion stimulation improves vestibular perception. Neuroscience. (2020) 441:1–7. doi: 10.1016/j.neuroscience.2020.05.053
56. Wagner, AR, Kobel, MJ, and Merfeld, DM. Impacts of rotation Axis and frequency on vestibular perceptual thresholds. Multisens Res. (2022) 35:259–87. doi: 10.1163/22134808-bja10069
57. Green, DM, and Swets, JA. Signal Detection Theory and Psychophysics. Oxford, England: John Wiley (1966).
58. Macmillan, NA, and Creelman, CD. Detection Theory: A User’s Guide. 2nd ed. Mahwah, N.J: Lawrence Erlbaum Associates (2005).
59. Diaz-Artiles, A, and Karmali, F. Vestibular precision at the level of perception, eye movements, posture, and neurons. Neuroscience. (2021) 468:282–320. doi: 10.1016/j.neuroscience.2021.05.028
60. Wagner, AR, Chaudhari, AMW, and Merfeld, DM. Might vestibular noise cause subclinical balance impairment and falls? Int J Phys Med Rehabil. (2021) 9:4.
61. Goldberg, JM, Wilson, VJ, Cullen, KE, Angelaki, DE, Broussard, DM, Buttner-Ennever, J, et al. The Vestibular System: A Sixth Sense. Oxford: Oxford University Press (2012).
62. Valko, Y, Lewis, RF, Priesol, AJ, and Merfeld, DM. Vestibular labyrinth contributions to human whole-body motion discrimination. J Neurosci. (2012) 32:13537–42. doi: 10.1523/JNEUROSCI.2157-12.2012
63. Kobel, MJ, Wagner, AR, and Merfeld, DM. Evaluating vestibular contributions to rotation and tilt perception. Exp Brain Res. (2023) 241:1873–85. doi: 10.1007/s00221-023-06650-5
64. Lim, K, Karmali, F, Nicoucar, K, and Merfeld, DM. Perceptual precision of passive body tilt is consistent with statistically optimal cue integration. J Neurophysiol. (2017) 117:2037–52. doi: 10.1152/jn.00073.2016
65. Agrawal, Y, Bremova, T, Kremmyda, O, Strupp, M, and MacNeilage, PR. Clinical testing of otolith function: perceptual thresholds and myogenic potentials. J Assoc Res Otolaryngol. (2013) 14:905–15. doi: 10.1007/s10162-013-0416-x
66. Chang, N-YN, Hiss, MM, Sanders, MC, Olomu, OU, MacNeilage, PR, Uchanski, RM, et al. Vestibular perception and the Vestibulo-ocular reflex in Young and older adults. Ear Hear. (2014) 35:565–70. doi: 10.1097/AUD.0000000000000052
67. Seemungal, BM, Gunaratne, IA, Fleming, IO, Gresty, MA, and Bronstein, AM. Perceptual and nystagmic thresholds of vestibular function in yaw. J Vestib Res Equilib Orientat. (2004) 14:461–6.
68. Kingma, H. Thresholds for perception of direction of linear acceleration as a possible evaluation of the otolith function. BMC Ear Nose Throat Disord. (2005) 5:5. doi: 10.1186/1472-6815-5-5
69. Li, C, Layman, AJ, Carey, JP, and Agrawal, Y. Epidemiology of vestibular evoked myogenic potentials: data from the Baltimore longitudinal study of aging. Clin Neurophysiol. (2015) 126:2207–15. doi: 10.1016/j.clinph.2015.01.008
70. Gabriel, GA, Harris, LR, Gnanasegaram, JJ, Cushing, SL, Gordon, KA, Haycock, BC, et al. Age-related changes to vestibular heave and pitch perception and associations with postural control. Sci Rep. (2022) 12:6426. doi: 10.1038/s41598-022-09807-4
71. Wagner, AR (2023) Uncovering Vestibular Contributions to Age-Related Imbalance. PhD diss., The Ohio State University
72. Beylergil, SB, Karmali, F, Wang, W, Bermúdez Rey, MC, and Merfeld, DM. Vestibular roll tilt thresholds partially mediate age-related effects on balance. Prog Brain Res. (2019) 248:249–67. doi: 10.1016/bs.pbr.2019.04.019
73. Ashish, G, Augustine, AM, Tyagi, AK, Lepcha, A, and Balraj, A. Subjective visual vertical and horizontal in vestibular migraine. J Int Adv Otol. (2017) 13:254–8. doi: 10.5152/iao.2017.4056
74. Paquet, N, Kulkarni, K, Fung, J, and Watt, D. Spatial navigation after surgical resection of an acoustic neuroma: pilot study. J Otolaryngol. (2003) 32:180–4. doi: 10.2310/7070.2003.40428
75. Cutfield, NJ, Cousins, S, Seemungal, BM, Gresty, MA, and Bronstein, AM. Vestibular perceptual thresholds to angular rotation in acute unilateral vestibular paresis and with galvanic stimulation. Ann N Y Acad Sci. (2011) 1233:256–62. doi: 10.1111/j.1749-6632.2011.06159.x
76. Cousins, S, Kaski, D, Cutfield, N, Seemungal, B, Golding, JF, Gresty, M, et al. Vestibular perception following acute unilateral vestibular lesions. PLoS One. (2013) 8:e61862. doi: 10.1371/journal.pone.0061862
77. Madhani, AS, King, S, Zhu, J, Karmali, F, Welling, DB, Cai, W, et al. Vestibular dysfunction in neurofibromatosis type 2-related schwannomatosis. Brain Commun. (2023) 5:fcad089. doi: 10.1093/braincomms/fcad089
78. Gianna, C, Heimbrand, S, and Gresty, M. Thresholds for detection of motion direction during passive lateral whole-body acceleration in normal subjects and patients with bilateral loss of labyrinthine function. Brain Res Bull. (1996) 40:443–9. doi: 10.1016/0361-9230(96)00140-2
79. Bringoux, L, Schmerber, S, Nougier, V, Dumas, G, Barraud, PA, and Raphel, C. Perception of slow pitch and roll body tilts in bilateral labyrinthine-defective subjects. Neuropsychologia. (2002) 40:367–72. doi: 10.1016/S0028-3932(01)00103-8
80. Shayman, CS, Seo, J-H, Oh, Y, Lewis, RF, Peterka, RJ, and Hullar, TE. Relationship between vestibular sensitivity and multisensory temporal integration. J Neurophysiol. (2018) 120:1572–7. doi: 10.1152/jn.00379.2018
81. Bednarczuk, NF, Bonsu, A, Ortega, MC, Fluri, AS, Chan, J, Rust, H, et al. Abnormal visuo-vestibular interactions in vestibular migraine: a cross sectional study. Brain J Neurol. (2019) 142:606–16. doi: 10.1093/brain/awy355
82. Angelaki, DE, Merfeld, DM, and Hess, BJ. Low-frequency otolith and semicircular canal interactions after canal inactivation. Exp Brain Res. (2000) 132:539–49. doi: 10.1007/s002210000364
83. Merfeld, DM, and Zupan, LH. Neural processing of gravitoinertial cues in humans. III. Modeling tilt and translation responses. J Neurophysiol. (2002) 87:819–33. doi: 10.1152/jn.00485.2001
84. Angelaki, DE, and Yakusheva, TA. How vestibular neurons solve the tilt/translation ambiguity: comparison of brainstem, cerebellum, and thalamus. Ann N Y Acad Sci. (2009) 1164:19–28. doi: 10.1111/j.1749-6632.2009.03939.x
85. Angelaki, DE, Shaikh, AG, Green, AM, and Dickman, JD. Neurons compute internal models of the physical laws of motion. Nature. (2004) 430:560–4. doi: 10.1038/nature02754
86. Lumba-Brown, A, Teramoto, M, Bloom, OJ, Brody, D, Chesnutt, J, Clugston, JR, et al. Concussion guidelines step 2: evidence for subtype classification. Neurosurgery. (2020) 86:2–13. doi: 10.1093/neuros/nyz332
87. Kontos, AP, Sufrinko, A, Sandel, N, Emami, K, and Collins, MW. Sport-related concussion clinical profiles: clinical characteristics, targeted treatments, and preliminary evidence. Curr Sports Med Rep. (2019) 18:82–92. doi: 10.1249/JSR.0000000000000573
88. Mucha, A, Collins, MW, Elbin, RJ, Furman, JM, Troutman-Enseki, C, DeWolf, RM, et al. A brief vestibular/ocular motor screening (VOMS) assessment to evaluate concussions: preliminary findings. Am J Sports Med. (2014) 42:2479–86. doi: 10.1177/0363546514543775
89. Alkathiry, AA, Kontos, AP, Furman, JM, Whitney, SL, Anson, ER, and Sparto, PJ. Vestibulo-ocular reflex function in adolescents with sport-related concussion: preliminary results. Sports Health. (2019) 11:479–85. doi: 10.1177/1941738119865262
90. Chen, X, DeAngelis, GC, and Angelaki, DE. Diverse spatial reference frames of vestibular signals in parietal cortex. Neuron. (2013) 80:1310–21. doi: 10.1016/j.neuron.2013.09.006
91. Chen, A, de Angelis, GC, and Angelaki, DE. Convergence of vestibular and visual self-motion signals in an area of the posterior sylvian fissure. J Neurosci. (2011) 31:11617–27. doi: 10.1523/JNEUROSCI.1266-11.2011
92. Alberts, BBGT, Selen, LPJ, Bertolini, G, Straumann, D, Medendorp, WP, and Tarnutzer, AA. Dissociating vestibular and somatosensory contributions to spatial orientation. J Neurophysiol. (2016) 116:30–40. doi: 10.1152/jn.00056.2016
93. Pfeiffer, C, Serino, A, and Blanke, O. The vestibular system: a spatial reference for bodily self-consciousness. Front Integr Neurosci. (2014) 8:31. doi: 10.3389/fnint.2014.00031
94. Glasauer, S, Amorim, M-A, Viaud-Delmon, I, and Berthoz, A. Differential effects of labyrinthine dysfunction on distance and direction during blindfolded walking of a triangular path. Exp Brain Res. (2002) 145:489–97. doi: 10.1007/s00221-002-1146-1
95. Shinder, ME, and Taube, JS. Differentiating ascending vestibular pathways to the cortex involved in spatial cognition. J Vestib Res Equilib Orientat. (2010) 20:3–23. doi: 10.3233/VES-2010-0344
96. He, Q, McNamara, TP, and Kelly, JW. Reference frames in spatial updating when body-based cues are absent. Mem Cogn. (2018) 46:32–42. doi: 10.3758/s13421-017-0743-y
97. Zachou, A, and Bronstein, AM. Vestibulo-spatial navigation: pathways and sense of direction. J Neurophysiol. (2023) 129:672–84. doi: 10.1152/jn.00422.2022
98. Mou, W, McNamara, TP, Valiquette, CM, and Rump, B. Allocentric and egocentric updating of spatial memories. J Exp Psychol Learn Mem Cogn. (2004) 30:142–57. doi: 10.1037/0278-7393.30.1.142
99. Anagnostou, E, Skarlatou, V, Mergner, T, and Anastasopoulos, D. Idiothetic signal processing and spatial orientation in patients with unilateral hippocampal sclerosis. J Neurophysiol. (2018) 120:1256–63. doi: 10.1152/jn.00016.2018
100. Gu, Y, and DeAngelis, GC. Angelaki DE (2007) a functional link between area MSTd and heading perception based on vestibular signals. Nat Neurosci. (2007) 10:1038–47. doi: 10.1038/nn1935
101. Blouin, J, Pialasse, JP, Mouchnino, L, and Simoneau, M. On the dynamics of spatial updating. Front Neurosci. (2022) 16:122–2. doi: 10.3389/fnins.2022.780027
102. Bigelow, RT, and Agrawal, Y. Vestibular involvement in cognition: visuospatial ability, attention, executive function, and memory. J Vestib Res Equilib Orientat. (2015) 25:73–89. doi: 10.3233/VES-150544
103. Taube, JS. The head direction signal: origins and sensory-motor integration. Annu Rev Neurosci. (2007) 30:181–207. doi: 10.1146/annurev.neuro.29.051605.112854
104. Lin, CT, Chiu, TC, and Gramann, K. EEG correlates of spatial orientation in the human retrosplenial complex. NeuroImage. (2015) 120:123–32. doi: 10.1016/j.neuroimage.2015.07.009
105. Da, CRQM, Pompeu, JE, De, VLAP, and Brucki, SMD. Spatial orientation tasks show moderate to high accuracy for the diagnosis of mild cognitive impairment: a systematic literature review. Arq Neuropsiquiatr. (2020) 78:713–23. doi: 10.1590/0004-282X20200043
106. da Costa, RQM, Pompeu, JE, Moretto, E, Silva, JM, Dos Santos, MD, Nitrini, R, et al. Two immersive virtual reality tasks for the assessment of spatial orientation in older adults with and without cognitive impairment: concurrent validity, group comparison, and accuracy results. J Int Neuropsychol Soc JINS. (2022) 28:460–72. doi: 10.1017/S1355617721000655
107. Brown, TI, Gagnon, SA, and Wagner, AD. Stress disrupts human hippocampal-prefrontal function during prospective spatial navigation and hinders flexible behavior. Curr Biol. (2020) 30:1821–1833.e8. doi: 10.1016/j.cub.2020.03.006
108. Anson, E, Ehrenburg, MR, Simonsick, EM, and Agrawal, Y. Association between vestibular function and rotational spatial orientation perception in older adults. J Vestib Res Equilib Orientat. (2021) 31:469–78. doi: 10.3233/VES-201582
109. Maxwell, R, von Kirschbaum, C, Jerin, C, Lehnen, N, Krause, E, and Gürkov, R. Effect of spatial orientation of the horizontal Semicircular Canal on the Vestibulo-ocular reflex. Otol Neurotol. (2016) 38:239–43. doi: 10.1097/MAO.0000000000001291
110. Zupan, LH, Merfeld, DM, and Darlot, C. Using sensory weighting to model the influence of canal, otolith and visual cues on spatial orientation and eye movements. Biol Cybern. (2002) 86:209–30. doi: 10.1007/s00422-001-0290-1
111. Laurens, J, and Angelaki, DE. The brain compass: a perspective on how self-motion updates the head direction cell attractor. Neuron. (2018) 97:275–89. doi: 10.1016/j.neuron.2017.12.020
112. Dieterich, M, and Brandt, T. Global orientation in space and the lateralization of brain functions. Curr Opin Neurol. (2018) 31:96–104. doi: 10.1097/WCO.0000000000000516
113. Valerio, S, and Taube, JS. Head direction cell activity is absent in mice without the horizontal semicircular canals. J Neurosci. (2016) 36:741–54. doi: 10.1523/JNEUROSCI.3790-14.2016
114. Arleo, A, Dejean, C, Allegraud, P, Khamassi, M, Zugaro, MB, and Wiener, SI. Optic flow stimuli update Anterodorsal thalamus head direction neuronal activity in rats. J Neurosci. (2013) 33:16790–5. doi: 10.1523/JNEUROSCI.2698-13.2013
115. Blouin, J, Saradjian, AH, Pialasse, JP, Manson, GA, Mouchnino, L, and Simoneau, M. Two neural circuits to point towards home position after passive body displacements. Front Neural Circuits. (2019) 13:70. doi: 10.3389/fncir.2019.00070
116. Israël, I, Fetter, M, and Koenig, E. Vestibular perception of passive whole-body rotation about horizontal and vertical axes in humans: goal-directed vestibulo-ocular reflex and vestibular memory-contingent saccades. Exp Brain Res. (1993) 96:335–46. doi: 10.1007/BF00227113
117. Paige, GD. Senescence of human visual-vestibular interactions. 1. Vestibulo-ocular reflex and adaptive plasticity with aging. J Vestib Res Equilib Orientat. (1992) 2:133–51.
118. Li, C, Layman, AJ, Geary, R, Anson, E, Carey, JP, Ferrucci, L, et al. Epidemiology of vestibulo-ocular reflex function: data from the Baltimore longitudinal study of aging. Otol Neurotol. (2015) 36:267–72. doi: 10.1097/MAO.0000000000000610
119. Aaen-Stockdale, C, Hotchkiss, J, Heron, J, and Whitaker, D. Perceived time is spatial frequency dependent. Vis Res. (2011) 51:1232–8. doi: 10.1016/j.visres.2011.03.019
120. Huberle, E, and Brugger, P. Altered time judgements highlight common mechanisms of time and space perception. Cogn Neuropsychol. (2018) 35:458–70. doi: 10.1080/02643294.2018.1549027
121. Choi, JY, Kwon, EJ, Lee, JY, Song, JM, Kim, HJ, and Kim, JS. Vestibular perception in time and space during whole-body rotation in humans. Cerebellum. (2021) 20:509–17. doi: 10.1007/s12311-020-01229-0
122. Borel, L, Honoré, J, Bachelard-Serra, M, Lavieille, JP, and Saj, A. Representation of body orientation in vestibular-defective patients before and after unilateral vestibular loss. Front Syst Neurosci. (2021) 15:117–7. doi: 10.3389/fnsys.2021.733684
123. Péruch, P, Lopez, C, Redon-Zouiteni, C, Escoffier, G, Zeitoun, A, Sanjuan, M, et al. Vestibular information is necessary for maintaining metric properties of representational space: evidence from mental imagery. Neuropsychologia. (2011) 49:3136–44. doi: 10.1016/j.neuropsychologia.2011.07.026
124. Grabherr, L, Cuffel, C, Guyot, J-P, and Mast, FW. Mental transformation abilities in patients with unilateral and bilateral vestibular loss. Exp Brain Res. (2011) 209:205–14. doi: 10.1007/s00221-011-2535-0
125. Minor, LB, and Lasker, DM. Tonic and phasic contributions to the pathways mediating compensation and adaptation of the Vestibulo-ocular reflex. J Vestib Res Equilib Orientat. (2009) 19:159–70. doi: 10.3233/VES-2009-0353
126. Böhmer, A, and Rickenmann, J. The subjective visual vertical as a clinical parameter of vestibular function in peripheral vestibular diseases. J Vestib Res. (1995) 5:35–45. doi: 10.3233/VES-1995-5104
127. Baier, B, Thömke, F, Wilting, J, Heinze, C, Geber, C, and Dieterich, M. A pathway in the brainstem for roll-tilt of the subjective visual vertical: evidence from a lesion-behavior mapping study. J Neurosci. (2012) 32:14854–8. doi: 10.1523/JNEUROSCI.0770-12.2012
128. Celis-Aguilar, E, Castro-Urquizo, A, and Mariscal-Castro, J. Evaluation and interpretation of the bucket test in healthy individuals. Acta Otolaryngol (Stockh). (2018) 138:458–62. doi: 10.1080/00016489.2017.1410289
129. Sun, DQ, Zuniga, MG, Davalos-Bichara, M, Carey, JP, and Agrawal, Y. Evaluation of a bedside test of utricular function - the bucket test - in older individuals. Acta Otolaryngol (Stockh). (2014) 134:382–9. doi: 10.3109/00016489.2013.867456
130. Argaet, EC, Kwok, BYC, Bradley, J, Young, AS, Nham, B, Calic, Z, et al. Subjective visual horizontal correlates better with ocular than with cervical vestibular evoked myogenic potentials. Clin Neurophysiol. (2023) 152:1–10. doi: 10.1016/j.clinph.2023.04.011
131. Min, KK, Ha, JS, Kim, MJ, Cho, CH, Cha, HE, and Lee, JH. Clinical use of subjective visual horizontal and vertical in patients of unilateral vestibular neuritis. Otol Neurotol. (2007) 28:520–5. doi: 10.1097/01.mao.0000271674.41307.f2
132. Tarnutzer, AA, Bockisch, C, Straumann, D, and Olasagasti, I. Gravity dependence of subjective visual vertical variability. J Neurophysiol. (2009) 102:1657–71. doi: 10.1152/jn.00007.2008
133. Zwergal, A, Rettinger, N, Frenzel, C, Dieterich, M, Brandt, T, and Strupp, M. A bucket of static vestibular function. Neurology. (2009) 72:1689–92. doi: 10.1212/WNL.0b013e3181a55ecf
134. Almajid, R, Tucker, C, Keshner, E, Vasudevan, E, and Wright, WG. Effects of wearing a head-mounted display during a standard clinical test of dynamic balance. Gait Posture. (2021) 85:78–83. doi: 10.1016/j.gaitpost.2021.01.020
135. Kobayashi, H, Hayashi, Y, Higashino, K, Saito, A, Kunihiro, T, Kanzaki, J, et al. Dynamic and static subjective visual vertical with aging. Auris Nasus Larynx. (2002) 29:325–8. doi: 10.1016/S0385-8146(02)00058-5
136. Zaleski-King, A, Pinto, R, Lee, G, and Brungart, D. Use of commercial virtual reality technology to assess verticality perception in static and dynamic visual backgrounds. Ear Hear. (2019) 41:125–35. doi: 10.1097/AUD.0000000000000736
137. Castro, P, Hussain, S, Mohamed, OG, Kaski, D, Arshad, Q, Bronstein, AM, et al. Visuospatial orientation: differential effects of head and body positions. Neurosci Lett. (2022) 775:136548–8. doi: 10.1016/j.neulet.2022.136548
138. Wang, C, Winnick, A, Ko, Y, Wang, Z, and Chang, T. Test-retest reliability of subjective visual vertical measurements with lateral head tilt in virtual reality goggles. Tzu Chi Med J. (2021) 33:294–300. doi: 10.4103/tcmj.tcmj_207_20
139. Michelson, PL, McCaslin, DL, Jacobson, GP, Petrak, M, English, L, and Hatton, K. Assessment of subjective visual vertical (SVV) using the bucket test and the virtual SVV system. Am J Audiol. (2018) 27:249–59. doi: 10.1044/2018_AJA-17-0019
140. Dai, T, Kurien, G, and Lin, V. Mobile phone app vs bucket test as a subjective visual vertical test: a validation study. J Otolaryngol Head Neck Surg. (2000) 49:1–3. doi: 10.1186/s40463-020-0402-3
141. Dieterich, M, and Brandt, T. Perception of verticality and vestibular disorders of balance and falls. Front Neurol. (2019) 10:172. doi: 10.3389/fneur.2019.00172
142. Brandt, T, Dieterich, M, and Danek, A. Vestibular cortex lesions affect the perception of verticality. Ann Neurol. (1994) 35:403–12. doi: 10.1002/ana.410350406
143. Saxena, S, Patel, B, Roy, R, Swami, H, Singh, SK, Goyal, S, et al. Role of subjective visual vertical in patients with posterior canal benign paroxysmal positional vertigo as a prognostic marker after canalith repositioning maneuver. J Otol. (2022) 17:111–5. doi: 10.1016/j.joto.2022.03.002
144. Batuecas-Caletrio, A, Santacruz-Ruiz, S, Muñoz-Herrera, A, Sousa, P, Otero, A, and Perez-Fernandez, N. Vestibular compensation after vestibular schwannoma surgery: normalization of the subjective visual vertical and disability. Acta Otolaryngol (Stockh). (2013) 133:475–80. doi: 10.3109/00016489.2012.757798
145. Guerraz, M, Yardley, L, Bertholon, P, Pollak, L, Rudge, P, Gresty, MA, et al. Visual vertigo: symptom assessment, spatial orientation and postural control. Brain. (2001) 124:1646–56. doi: 10.1093/brain/124.8.1646
146. Ward, BK, Bockisch, CJ, Caramia, N, Bertolini, G, and Tarnutzer, AA. Gravity dependence of the effect of optokinetic stimulation on the subjective visual vertical. J Neurophysiol. (2017) 117:1948–58. doi: 10.1152/jn.00303.2016
147. Tarnutzer, AA, Bockisch, CJ, and Straumann, D. Head roll dependent variability of subjective visual vertical and ocular counterroll. Exp Brain Res. (2009) 195:621–6. doi: 10.1007/s00221-009-1823-4
148. Schönfeld, U, and Clarke, AH. A clinical study of the subjective visual vertical during unilateral centrifugation and static tilt. Acta Otolaryngol (Stockh). (2011) 131:1040–50. doi: 10.3109/00016489.2011.584902
149. Janky, KL, and Shepard, NT. Unilateral centrifugation: Utricular assessment and protocol. Otol Neurotol. (2011) 32:116–21. doi: 10.1097/MAO.0b013e3181ff7549
150. McCarthy, J, Castro, P, Cottier, R, Buttell, J, Arshad, Q, and Kheradmand, A. Multisensory contribution in visuospatial orientation: an interaction between neck and trunk proprioception. Exp Brain Res. (2021) 2398:2501–8. doi: 10.1007/s00221-021-06146-0
151. Kheradmand, A, and Otero-Millan, J. Spatial orientation: model-based approach to multi-sensory mechanisms. Prog Brain Res. (2019) 248:209–23. doi: 10.1016/bs.pbr.2019.04.029
152. Kim, MJ, Otero-Millan, J, Tian, J, and Kheradmand, A. Measures of spatial orientation: Spatial Bias analogs in visual and haptic tasks. eNeuro. (2022) 9:ENEURO.0233-22.2022. doi: 10.1523/ENEURO.0233-22.2022
153. Akin, FW, Murnane, OD, Pearson, A, Byrd, S, and Kelly, JK. Normative data for the subjective visual vertical test during centrifugation. J Am Acad Audiol. (2011) 22:460–8. doi: 10.3766/jaaa.22.7.6
154. Friedmann, G. The influence of unilateral labyrinthectomy on orientation in space. Acta Otolaryngol (Stockh). (1971) 71:289–98.
155. Zakaria, MN, Salim, R, Tahir, A, Zainun, Z, and Mohd Sakeri, NS. The influences of age, gender and geometric pattern of visual image on the verticality perception: a subjective visual vertical (SVV) study among Malaysian adults. Clin Otolaryngol. (2019) 44:166–71. doi: 10.1111/coa.13255
156. Dieterich, M, and Brandt, T. Ocular torsion and tilt of subjective visual vertical are sensitive brainstem signs. Ann Neurol. (1993) 33:292–9. doi: 10.1002/ana.410330311
157. Alberts, BBGT, Selen, LPJ, Verhagen, WIM, Pennings, RJE, and Medendorp, WP. Bayesian quantification of sensory reweighting in a familial bilateral vestibular disorder (DFNA9). J Neurophysiol. (2018) 119:1209–21. doi: 10.1152/jn.00082.2017
158. Bürgin, A, Bockisch, CJ, and Tarnutzer, AA. Precision of perceived direction of gravity in partial bilateral vestibulopathy correlates with residual utricular function. Clin Neurophysiol. (2018) 129:934–45. doi: 10.1016/j.clinph.2018.02.121
159. Cochrane, GD, Christy, JB, Almutairi, A, Busettini, C, Swanson, MW, and Weise, KK. Visuo-oculomotor function and reaction times in athletes with and without concussion. Optom Vis Sci. (2019) 96:256–65. doi: 10.1097/OPX.0000000000001364
160. Heyer, GL, Young, JA, and Fischer, AN. Lightheadedness after concussion: not all dizziness is Vertigo. Clin J Sport Med. (2018) 28:272–7. doi: 10.1097/JSM.0000000000000445
161. Zhou, G, and Brodsky, JR. Objective vestibular testing of children with dizziness and balance complaints following sports-related concussions. Otolaryngol--Head Neck Surg Off J Am Acad Otolaryngol-Head Neck Surg. (2015) 152:1133–9. doi: 10.1177/0194599815576720
162. Lee, JD, Park, MK, Lee, BD, Park, JY, Lee, TK, and Sung, K-B. Otolith function in patients with head trauma. Eur Arch Otorhinolaryngol. (2011) 268:1427–30. doi: 10.1007/s00405-010-1426-5
163. Israël, I, Ventre-Dominey, J, and Denise, P. Vestibular information contributes to update retinotopic maps. Neuroreport. (1999) 10:3479–83. doi: 10.1097/00001756-199911260-00003
164. Mackrous, I, and Simoneau, M. Visuo-vestibular interaction: predicting the position of a visual target during passive body rotation. Neuroscience. (2011) 195:45–53. doi: 10.1016/j.neuroscience.2011.07.032
165. Mackrous, I, and Simoneau, M. Generalization of vestibular learning to earth-fixed targets is possible but limited when the polarity of afferent vestibular information is changed. Neuroscience. (2014) 260:12–22. doi: 10.1016/j.neuroscience.2013.12.002
166. Cheng, Y, Zhang, Y, Ma, W, Chen, Y, Zhang, Q, and Xu, M. Effect of age on virtual reality-assisted subjective visual vertical and subjective visual horizontal at different head-tilt angles. Braz J Otorhinolaryngol. (2022) 88:S139–46. doi: 10.1016/j.bjorl.2022.10.001
167. Ardıç, FN, Metin, U, and Gökcan, BE. Subjective visual vertical test with the 3D virtual reality system: effective factors and cybersickness. Acta Otolaryngol (Stockh). (2023) 143:570–5. doi: 10.1080/00016489.2023.2238769
168. Ulozienė, I, Totilienė, M, Paulauskas, A, Blažauskas, T, Marozas, V, Kaski, D, et al. Subjective visual vertical assessment with mobile virtual reality system. Med Kaunas Lith. (2017) 53:394–402. doi: 10.1016/j.medici.2018.02.002
169. Cheng, Y, Zhang, Y, Chen, F, Chen, Z, Xue, T, Zhang, Q, et al. Virtual reality for the measurement of SVV and SVH during static head tilt in healthy adults: a novel vestibular test. Acta Otolaryngol (Stockh). (2022) 142:291–7. doi: 10.1080/00016489.2022.2058082
170. Klatt, BN, Sparto, PJ, Terhorst, L, Winser, S, Heyman, R, and Whitney, SL. Relationship between subjective visual vertical and balance in individuals with multiple sclerosis. Physiother Res Int. (2019) 24:–e1757. doi: 10.1002/pri.1757
171. Funabashi, M, Santos-Pontelli, TEG, Colafêmina, JF, Pavan, TZ, Carneiro, AAO, and Takayanagui, OM. A new method to analyze the subjective visual vertical in patients with bilateral vestibular dysfunction. Clinics. (2012) 67:1127–31. doi: 10.6061/clinics/2012(10)02
172. Cohen, HS. Vestibular disorders and impaired path integration along a linear trajectory. J Vestib Res Equilib Orientat. (2000) 10:7–15. doi: 10.3233/VES-2000-10102
173. Schautzer, F, Hamilton, D, Kalla, R, Strupp, M, and Brandt, T. Spatial memory deficits in patients with chronic bilateral vestibular failure. Ann N Y Acad Sci. (2003) 1004:316–24. doi: 10.1196/annals.1303.029
174. Guidetti, G, Monzani, D, Trebbi, M, and Rovatti, V. Impaired navigation skills in patients with psychological distress and chronic peripheral vestibular hypofunction without vertigo. Acta Otorhinolaryngol. (2008) 28:21–5.
175. Cohen, HS, and Sangi-Haghpeykar, H. Walking speed and vestibular disorders in a path integration task. Gait Posture. (2011) 33:211–3. doi: 10.1016/j.gaitpost.2010.11.007
176. Arthur, JC, Kortte, KB, Shelhamer, M, and Schubert, MC. Linear path integration deficits in patients with abnormal vestibular afference. Seeing Perceiving. (2012) 25:155–78. doi: 10.1163/187847612X629928
177. Kremmyda, O, Hüfner, K, Flanagin, VL, Hamilton, DA, Linn, J, Strupp, M, et al. Beyond dizziness: virtual navigation, spatial anxiety and hippocampal volume in bilateral Vestibulopathy. Front Hum Neurosci. (2016) 10:139. doi: 10.3389/fnhum.2016.00139
178. Franke, LM, Walker, WC, Cifu, DX, Ochs, AL, and Lew, HL. Sensorintegrative dysfunction underlying vestibular disorders after traumatic brain injury: a review. J Rehabil Res Dev. (2012) 49:985–94. doi: 10.1682/JRRD.2011.12.0250
179. Teel, E, Gay, M, Johnson, B, and Slobounov, S. Determining sensitivity/specificity of virtual reality-based neuropsychological tool for detecting residual abnormalities following sport-related concussion. Neuropsychology. (2016) 30:474–83. doi: 10.1037/neu0000261
180. Beylergil, SB, Ozinga, S, Walker, MF, McIntyre, CC, and Shaikh, AG. Vestibular heading perception in Parkinson’s disease. Prog Brain Res. (2019) 249:307–19. doi: 10.1016/bs.pbr.2019.03.034
181. Xie, Y, Bigelow, RT, Frankenthaler, SF, Studenski, SA, Moffat, SD, and Agrawal, Y. Vestibular loss in older adults is associated with impaired spatial navigation: data from the triangle completion task. Front Neurol. (2017) 8:173. doi: 10.3389/fneur.2017.00173
182. Loomis, JM, Klatzky, RL, Golledge, RG, Cicinelli, JG, Pellegrino, JW, and Fry, PA. Nonvisual navigation by blind and sighted: assessment of path integration ability. J Exp Psychol Gen. (1993) 122:73–91. doi: 10.1037/0096-3445.122.1.73
183. Philbeck, JW, Klatzky, RL, Behrmann, M, Loomis, JM, and Goodridge, J. Active control of locomotion facilitates nonvisual navigation. J Exp Psychol Hum Percept Perform. (2001) 27:141–53. doi: 10.1037/0096-1523.27.1.141
184. Péruch, P, Borel, L, Magnan, J, and Lacour, M. Direction and distance deficits in path integration after unilateral vestibular loss depend on task complexity. Brain Res Cogn Brain Res. (2005) 25:862–72. doi: 10.1016/j.cogbrainres.2005.09.012
185. Grove, CR, Heiderscheit, BC, Pyle, GM, Loyd, BJ, and Whitney, SL. The gait disorientation test: a new method for screening adults with dizziness and imbalance. Arch Phys Med Rehabil. (2021) 102:582–90. doi: 10.1016/j.apmr.2020.11.010
186. Hegarty, M, Richardson, AE, Montello, DR, Lovelace, K, and Subbiah, I. Development of a self-report measure of environmental spatial ability. Intelligence. (2002) 30:425–47. doi: 10.1016/S0160-2896(02)00116-2
187. McLaren, R, Chaudhary, S, Rashid, U, Ravindran, S, and Taylor, D. Reliability of the triangle completion test in the real-world and in virtual reality. Front Hum Neurosci. (2022) 16:945953. doi: 10.3389/fnhum.2022.945953
188. Gandhi, P, Biju, K, Klatt, BN, Simonsick, E, and Agrawal, Y. Self-reported sense of direction and vestibular function in the Baltimore longitudinal study of aging (BLSA). J Assoc Res Otolaryngol JARO. (2021) 22:207–14. doi: 10.1007/s10162-020-00780-1
189. Glasauer, S, Amorim, MA, Vitte, E, and Berthoz, A. Goal-directed linear locomotion in normal and labyrinthine-defective subjects. Exp Brain Res. (1994) 98:323–35. doi: 10.1007/BF00228420
190. Schöberl, F, Pradhan, C, Grosch, M, Brendel, M, Jostes, F, Obermaier, K, et al. Bilateral vestibulopathy causes selective deficits in recombining novel routes in real space. Sci Rep. (2021) 11:2695. doi: 10.1038/s41598-021-82427-6
191. Dordevic, M, Sulzer, S, Barche, D, Dieterich, M, Arens, C, and Müller, NG. Chronic, mild Vestibulopathy leads to deficits in spatial tasks that rely on vestibular input while leaving other cognitive functions and brain volumes intact. Life Basel. (2021) 11:1369. doi: 10.3390/life11121369
192. Chari, DA, Madhani, A, Sharon, JD, and Lewis, RF. Evidence for cognitive impairment in patients with vestibular disorders. J Neurol. (2022) 269:5831–42. doi: 10.1007/s00415-022-11289-3
193. Biju, K, Wei, EX, Rebello, E, Matthews, J, He, Q, McNamara, TP, et al. Performance in real world-and virtual reality-based spatial navigation tasks in patients with vestibular dysfunction. Otol Neurotol. (2021) 42:e1524–31. doi: 10.1097/MAO.0000000000003289
194. Cohen, HS, and Kimball, KT. Improvements in path integration after vestibular rehabilitation. J Vestib Res Equilib Orientat. (2002) 12:47–51. doi: 10.3233/VES-2002-12105
195. Ishikawa, T, and Zhou, Y. Improving cognitive mapping by training for people with a poor sense of direction. Cogn Res Princ Implic. (2020) 5:39. doi: 10.1186/s41235-020-00238-1
196. Hall, CD, Herdman, SJ, Whitney, SL, Anson, ER, Carender, WJ, Hoppes, CW, et al. Vestibular rehabilitation for peripheral vestibular hypofunction: an updated clinical practice guideline from the academy of neurologic physical therapy of the American Physical Therapy Association. J Neurol Phys Ther. (2022) 46:118–77. doi: 10.1097/NPT.0000000000000382
197. Ahmad, M, Bola, L, Boutabla, A, King, S, Lewis, RF, and Chari, DA. Visuospatial cognitive dysfunction in patients with vestibular loss. Otol Neurotol. (2022) 43:E1140–7. doi: 10.1097/MAO.0000000000003696
198. Redfern, MS, Talkowski, ME, Jennings, JR, and Furman, JM. Cognitive influences in postural control of patients with unilateral vestibular loss. Gait Posture. (2004) 19:105–14. doi: 10.1016/S0966-6362(03)00032-8
199. Nascimbeni, A, Gaffuri, A, Penno, A, and Tavoni, M. Dual task interference during gait in patients with unilateral vestibular disorders. J Neuroeng Rehabil. (2010) 7:47. doi: 10.1186/1743-0003-7-47
200. Popp, P, Wulff, M, Finke, K, Rühl, M, Brandt, T, and Dieterich, M. Cognitive deficits in patients with a chronic vestibular failure. J Neurol. (2017) 264:554–63. doi: 10.1007/s00415-016-8386-7
201. Ayar, DA, Kumral, E, and Celebisoy, N. Cognitive functions in acute unilateral vestibular loss. J Neurol. (2020) 267:153–9. doi: 10.1007/s00415-020-09829-w
202. Hüfner, K, Hamilton, DA, Kalla, R, Stephan, T, Glasauer, S, Ma, J, et al. Spatial memory and hippocampal volume in humans with unilateral vestibular deafferentation. Hippocampus. (2007) 17:471–85. doi: 10.1002/hipo.20283
203. Conrad, J, Habs, M, Brandt, T, and Dieterich, M. Acute unilateral vestibular failure does not cause spatial Hemineglect. PLoS One. (2015) 10:e0135147. doi: 10.1371/journal.pone.0135147
204. Bosmans, J, Gommeren, H, Mertens, G, Cras, P, Engelborghs, S, Van Ombergen, A, et al. Associations of bilateral Vestibulopathy with cognition in older adults matched with healthy controls for hearing status. JAMA Otolaryngol Head Neck Surg. (2022) 148:731–9. doi: 10.1001/jamaoto.2022.1303
205. Bessot, N, Denise, P, Toupet, M, Van Nechel, C, and Chavoix, C. Interference between walking and a cognitive task is increased in patients with bilateral vestibular loss. Gait Posture. (2012) 36:319–21. doi: 10.1016/j.gaitpost.2012.02.021
206. Brandt, T, Schautzer, F, Hamilton, DA, Brüning, R, Markowitsch, HJ, Kalla, R, et al. Vestibular loss causes hippocampal atrophy and impaired spatial memory in humans. Brain. (2005) 128:2732–41. doi: 10.1093/brain/awh617
207. Zhong, J, Li, X, Xu, J, Chen, W, Gao, J, Lu, X, et al. Analysis of cognitive function and its related factors after treatment in Meniere’s disease. Front Neurosci. (2023) 17:1137734. doi: 10.3389/fnins.2023.1137734
208. Demirhan, MA, and Celebisoy, N. Cognitive functions in episodic vestibular disorders: Meniere’s disease and vestibular migraine. J Vestib Res Equilib Orientat. (2023) 33:63–70. doi: 10.3233/VES-220025
209. Hitier, M, Besnard, S, and Smith, PF. Vestibular pathways involved in cognition. Front Integr Neurosci. (2014) 8:59–9. doi: 10.3389/fnint.2014.00059
210. Smith, PF, and Zheng, Y. From ear to uncertainty: vestibular contributions to cognitive function. Front Integr Neurosci. (2013) 7:84–4. doi: 10.3389/fnint.2013.00084
211. Micarelli, A, Viziano, A, Micarelli, B, Augimeri, I, and Alessandrini, M. Vestibular rehabilitation in older adults with and without mild cognitive impairment: effects of virtual reality using a head-mounted display. Arch Gerontol Geriatr. (2019) 83:246–56. doi: 10.1016/j.archger.2019.05.008
212. Klatt, BN, Ries, JD, Dunlap, PM, Whitney, SL, and Agrawal, Y. Vestibular physical therapy in individuals with cognitive impairment. J Neurol Phys Ther. (2019) 43:S14–9. doi: 10.1097/NPT.0000000000000266
213. Yesantharao, LV, Rosenberg, P, Oh, E, Leoutsakos, J, Munro, CA, and Agrawal, Y. Vestibular therapy to reduce falls in people with Alzheimer’s disease: study protocol for a pilot randomized controlled trial. Pilot Feasibility Stud. (2022) 8:167. doi: 10.1186/s40814-022-01133-w
214. Schöne, CG, and Mast, FW. High-current galvanic vestibular stimulation impairs working memory span, but not other executive functions. Neuropsychologia. (2023) 188:108617. doi: 10.1016/j.neuropsychologia.2023.108617
Keywords: vestibular, perception, spatial orientation, navigation, cognition
Citation: Grove CR, Klatt BN, Wagner AR and Anson ER (2023) Vestibular perceptual testing from lab to clinic: a review. Front. Neurol. 14:1265889. doi: 10.3389/fneur.2023.1265889
Edited by:
Philippe Perrin, Université de Lorraine, FranceReviewed by:
Art Mallinson, University of British Columbia, CanadaDiego Kaski, University College London, United Kingdom
Copyright © 2023 Grove, Klatt, Wagner and Anson. This is an open-access article distributed under the terms of the Creative Commons Attribution License (CC BY). The use, distribution or reproduction in other forums is permitted, provided the original author(s) and the copyright owner(s) are credited and that the original publication in this journal is cited, in accordance with accepted academic practice. No use, distribution or reproduction is permitted which does not comply with these terms.
*Correspondence: Eric R. Anson, ZXJpY19hbnNvbkB1cm1jLnJvY2hlc3Rlci5lZHU=