- 1Department of Rehabilitation Medicine, The First People’s Hospital of Yunnan Province, Kunming, China
- 2Department of Neurosurgery, Mengzi People’s Hospital, Mengzi, China
- 3Department of Rehabilitation Medicine, Dongchuan District People’s Hospital, Kunming, China
- 4Department of Pulmonary and Critical Care Medicine, Kunming Municipal Hospital of Traditional Chinese Medicine, Kunming, China
- 5Department of Emergency Trauma Surgery, The First People’s Hospital of Yunnan Province, Kunming, China
- 6Key Laboratory of Traditional Chinese Medicine for Prevention and Treatment of Neuropsychiatric Diseases, Yunnan University of Chinese Medicine, Kunming, China
Traumatic or non-traumatic spinal cord injury (SCI) can lead to severe disability and complications. The incidence of SCI is high, and the rehabilitation cycle is long, which increases the economic burden on patients and the health care system. However, there is no practical method of SCI treatment. Recently, transcranial magnetic stimulation (TMS), a non-invasive brain stimulation technique, has been shown to induce changes in plasticity in specific areas of the brain by regulating the activity of neurons in the stimulation site and its functionally connected networks. TMS is a new potential method for the rehabilitation of SCI and its complications. In addition, TMS can detect the activity of neural circuits in the central nervous system and supplement the physiological evaluation of SCI severity. This review describes the pathophysiology of SCI as well as the basic principles and classification of TMS. We mainly focused on the latest research progress of TMS in the physiological evaluation of SCI as well as the treatment of motor dysfunction, neuropathic pain, spasticity, neurogenic bladder, respiratory dysfunction, and other complications. This review provides new ideas and future directions for SCI assessment and treatment.
1. Introduction
Spinal cord injury (SCI) has traumatic (e.g., car accidents and falls) and non-traumatic (e.g., infections and tumors) causes. Due to the limited repair ability of the central nervous system, SCI can lead to serious sensory, motor, and physical dysfunction below the injured segment. Moreover, SCI may cause neuropathic pain (NP), spasticity, neurogenic bladder, respiratory dysfunction, and other complications, which seriously affect the quality of life and life expectancy of patients (1).
With the growth of the global population, the prevalence and incidence of SCI remain high. In 2019, 900,000 incident cases, 20.6 million prevalent cases, and 6.2 million annual deaths related to SCI were registered (2). In China, the incidence of SCI ranges from 14.6 to 60.6 persons per million; furthermore, SCI incidence and health burden increase with time and age (3, 4). Expensive and complex medical support is needed after SCI. The lifetime financial burden of patients with SCI ranges from $1.5 million to $3 million (5). Meanwhile, the average annual health care cost incurred by patients with SCI in the United States is as high as $676,000 (6), which places a heavy economic burden on patients and the health care system. Therefore, SCI treatment and cost reduction remain a complex, global public health problem.
Protecting the nervous system and promoting neuron repair and regeneration are two primary directions for SCI therapy. In the early stage of SCI, the International Association of Neurorestoratology guidelines recommend surgical removal of fluid or tissue causing spinal cord compression, combined with the use of drugs to reduce inflammation and protect neurons (7). Subacute treatment mainly involves multi-disciplinary rehabilitation, with an extensive use of various treatments to improve neuroplasticity. Nevertheless, the efficacy of these treatments remains unclear.
In recent years, many scholars have found that neuromodulation methods, such as epidural spinal cord stimulation (8), transcutaneous spinal cord stimulation (tSCS) (9, 10), transcutaneous spinal direct current stimulation (tsDCS) (11), transcranial direct current stimulation (tDCS) (12), brain-computer interface-triggered functional electrical stimulation therapy (13), and transcranial magnetic stimulation (TMS) (14), can improve neuroplasticity, thereby potentially treating SCI. Epidural spinal cord stimulation and tsDCS can activate spinal neurons in specific segments and regulate spinal neural circuits (15). However, epidural spinal cord stimulation and tsDCS require surgical electrode implantation, and most patients do not accept invasive methods. In contrast, tSCS is a non-invasive stimulation technique. When the electrode is placed on the skin, it can stimulate spinal cord circuits and promote patient motor reflex response (9, 10). However, tSCS may be inaccurate in targeting specific areas of the spinal cord. Similarly, tDCS can stimulate neurons, but its spatial accuracy is limited, and thus connecting the stimulation with specific brain regions is challenging. In addition, brain-computer interface-triggered functional electrical stimulation therapy is complex, involves a high cost for research and development, and requires a high professional knowledge for operators; hence, its use in clinical practice is limited (16). TMS has the advantages of non-invasiveness, simple operation, and low cost compared with other neuromodulation methods. It can transmit a magnetic field through the scalp and skull using specific parameters to regulate neuronal activity in specific brain regions (17). Currently, TMS has become a primary means of non-invasive neuromodulation in patients with SCI.
Many studies have reported that TMS can improve synaptic plasticity and has a broad prospect in SCI treatment. TMS has been widely used in evaluating SCI and treating motor dysfunction, NP, spasticity, neurogenic bladder, respiratory dysfunction, and other SCI complications (Figure 1). In this article, we discuss the latest applications of TMS in SCI management. We used the PubMed database for a selective literature search of papers published between January 2000 and January 2023. Our key search terms were “spinal cord injury,” “SCI,” “transcranial magnetic stimulation,” “TMS,” “assessment,” “evaluation,” “motor dysfunction,” “neuropathic pain,” “spasticity,” “neurogenic bladder,” and “respiratory dysfunction.” Moreover, all the selected studies were written in English.
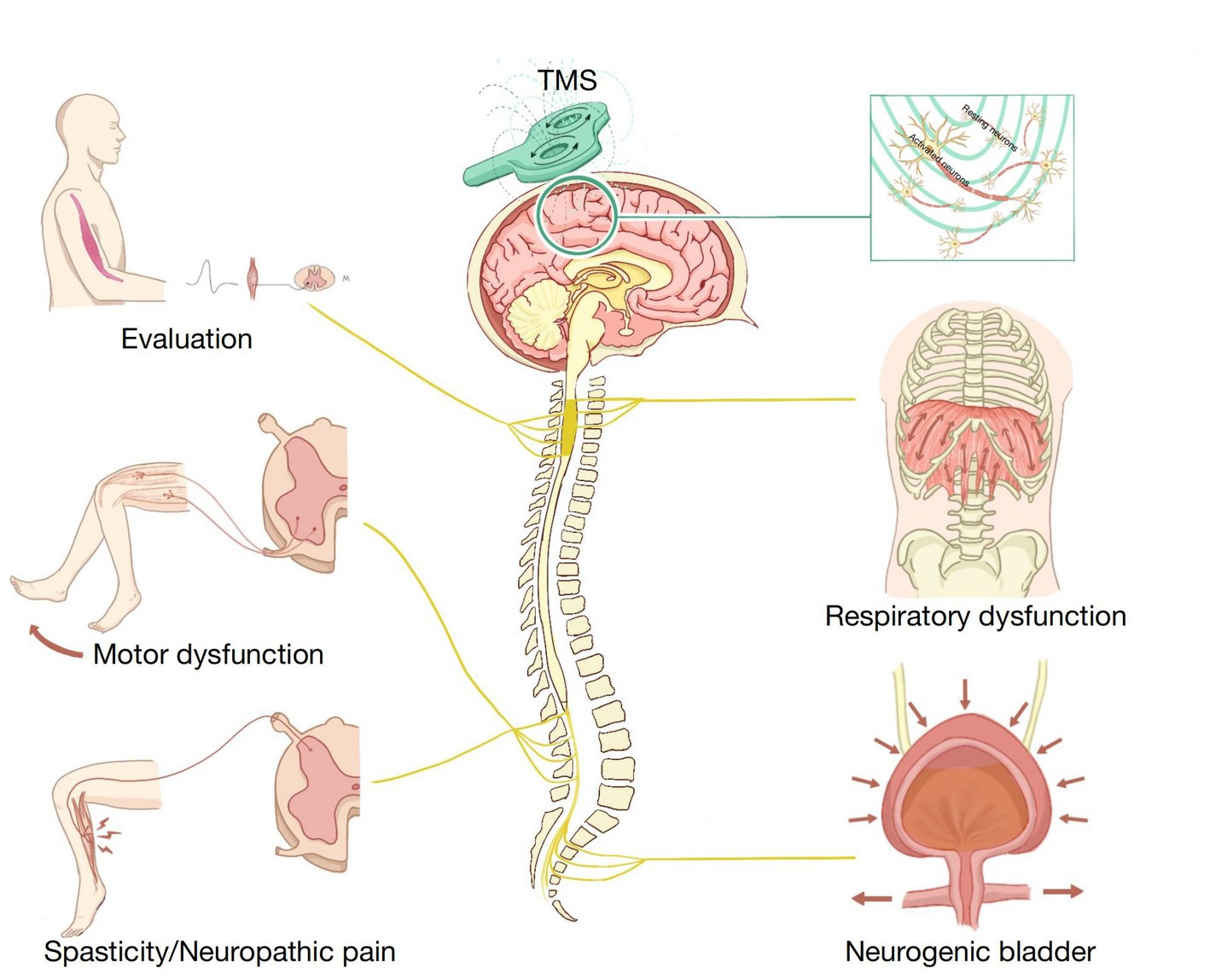
Figure 1. Application of transcranial magnetic stimulation (TMS) in spinal cord injury (SCI) rehabilitation.
2. Pathophysiological mechanism of SCI
Understanding the pathophysiological changes that occur during SCI is the basis for making a treatment plan. SCI can be divided into two stages: primary and secondary SCI. Primary SCI involves spinal cord compression as well as shear, tear, or traction injury caused by spinal fracture, dislocation, and other factors, which can lead to local vascular damage, nerve parenchyma injury, ion imbalance, and glial membrane destruction (18). With further changes in physiological and biochemical reactions in the tissue, the spinal cord transitions into a stage of secondary injury.
Secondary SCI can be divided into acute, subacute, and chronic injury phases. In the acute phase, a large amount of calcium ions in the extracellular fluid flow into the cell, which leads to neuronal excitotoxicity, increases the concentration of reactive oxygen species and glutamate, and causes more severe damage to neurons and glial cells (18, 19). Moreover, the increase in cell membrane permeability causes inflammatory cells such as macrophages and microglia to infiltrate the injured site and release interleukin-6, interleukin-1β, and tumor necrosis factor-α to aggravate neuronal inflammatory response (20). Furthermore, spinal cord vascular injury leads to vascular ischemia, hypotension, and hypo-perfusion, resulting in cell death and tissue destruction (21). If the acute secondary injury is not effectively controlled, the body enters the subacute phase. Spinal cord changes during this period are characterized by neuronal apoptosis, axonal demyelination, Wallerian degeneration, axonal remodeling, and glial scarring (18, 21). Oligodendrocytes are essential in promoting axonal proliferation and myelination (22). However, during SCI, oligodendrocyte necrosis and apoptosis occur, leading to axonal demyelination, which affects axonal function and stability (23). When astrocytes proliferate to form glial scars, the injury starts healing and gradually transitions to the chronic phase. Eventually, massive cell death by apoptosis leads to cystic cavity formation, accompanied by axonal loss and glial scar maturation (24). In summary, pathological changes differ in different stages of injury, and therefore targeted treatment programs should be designed to promote nerve protection and regeneration.
3. Basic principles and classification of TMS
TMS is a non-invasive technique that can be used to stimulate specific brain tissues and affect the activity of local neurons by transmitting magnetic pulses from copper coils. The mechanism of TMS may be related to synaptogenesis or recombination as well as activation or inhibition of the activity of target cortical neurons, which leads to long-term synaptic potentiation or inhibition, thereby inducing changes in brain plasticity (25, 26). Tang et al. (27) found that TMS can change the density, loss rate, and formation rate of dendritic spines in adult and aged mice, in addition to changing the function of synaptic connections in the brain. Other studies have reported that TMS can improve neural plasticity by up-regulating the expression of brain-derived neurotrophic factor (BDNF), tropomyosin receptor kinase B, N-methyl-D-aspartate receptor, and synaptophysin (28). Moreover, TMS maintains the normal nerve conduction function by enhancing the myelination of demyelinated neurons (29). In addition, it can somewhat increase the survival and maturation of neonatal oligodendrocytes in the mouse cerebral cortex, resulting in neuroprotection (29). Furthermore, TMS appears to have some anti-inflammatory effects. It can increase the anti-inflammatory polarization of microglia to alleviate neuroinflammation and apoptosis; further, it promotes nerve tissue regeneration to a certain extent (30).
TMS was first used in the treatment of depression and other mental disorders. Many scholars have found that the application of TMS on the prefrontal cortex can effectively improve depression scores and clinical symptoms of patients with drug-refractory depression (31, 32). TMS is safe and well-tolerated; the incidence of epilepsy in patients undergoing TMS is less than 0.01% (10, 33). Therefore, in 2008, the United States Food and Drug Administration approved the use of TMS in drug-resistant depression treatment (34). Currently, TMS is used in the treatment of nervous system diseases such as SCI (35), Alzheimer’s disease (36), Parkinson’s disease (37), multiple sclerosis (38), stroke-related disability (39), and schizophrenia (40). TMS use has achieved gratifying results in basic research and clinical trials; hence, it is a potential treatment method for nervous system diseases.
TMS can be used as single-pulse stimulation, paired-pulse stimulation, repetitive TMS (rTMS), and paired associative stimulation (PAS). The single-pulse TMS program consists of single-pulse discharges that activate contralateral muscles when stimulating the primary motor cortex (M1). This activation can be recorded by electromyographic motor evoked potentials (MEP) (41). Paired-pulse TMS can be used to evaluate the excitability of intracortical or intercortical connections by paired stimulation (42).
rTMS is the most common stimulation protocol in therapeutic research, and different therapeutic effects can be produced when different parameters are used to stimulate the cerebral cortex. Generally, an rTMS frequency ≥5 Hz can increase the excitability of the motor cortex. In contrast, low-frequency rTMS (≤1 Hz) reduces cortical excitability, and thus it is used to regulate overactivity in specific brain regions (43). PAS includes the repeated pairings of single-pulse peripheral nerve electrical stimulation and single-pulse TMS of the corresponding motor cortex, which affects the cortical motor excitability of the corticospinal pathway to the target muscle (44). The excitability of the motor cortex is related to the interval between the two stimuli. The excitability of the corticospinal pathway increases when peripheral afferent stimulation is synchronized with TMS or reaches the motor cortex before TMS. Otherwise, corticospinal pathways will be inhibited (44–46). Nowadays, PAS has been shown to induce motor cortex plasticity and excitability (47).
Different coils have different penetration depths and stimulation ranges. The stimulation intensity of the circular coil is 0 in the center and maximum at the edge of the coil (48). The figure-eight coil can concentrate the stimulus at the center. Unlike the circular coil with an extensive stimulation, the figure-eight coil provides a more focused stimulation (48). However, both circular and figure-eight coils have shallow penetration depths. These coils can only be used to stimulate brain areas 2–2.5 cm from the scalp, and hence they are mainly used for cortical stimulation. To stimulate the deep brain regions, scholars have designed double cone, H-, and HCA coils, whose penetration depths can reach 3–6 cm (49). Therefore, different coils and stimulation protocols should be used for different treatment purposes in clinical applications.
4. Application of TMS in the rehabilitation of SCI
4.1. Evaluation
Clinically, the international classification of SCI is used to evaluate patient sensory and motor functions. Nevertheless, it only subjectively reflects the degree of injury and the lack of detection of changes in neurophysiological mechanisms. TMS is a method of neurophysiological examination, which can detect the residual corticospinal cord connection and nerve recovery after SCI; hence, it is a supplement to the international classification of SCI (50).
The MEP produced by applying TMS on M1 can detect the integrity of the descending conduction pathway from the cortex to the area below the injury level in patients with SCI; hence, it can be used to clinically recognize complete SCI. Although patients with complete cervical and thoracic SCI cannot elicit muscle activity during clinical examination, MEPs can be detected in trunk muscles, some lower limb muscles, and pelvic floor muscles during TMS (51, 52). Thus, there are residual corticospinal connections below the injury level, and rehabilitation should focus on the consolidation of these residual innervations. Evaluating the respiratory muscle function of patients with complete SCI above T6 remains a complex problem in clinical practice. Welch et al. (53) found that TMS combined with electromyography can be used to effectively characterize diaphragm activation in healthy individuals. More importantly, the MEP had good reproducibility in all participants, indicating that TMS-induced MEP may effectively detect cortical diaphragmatic pathway connectivity. In addition, TMS can be used to evaluate abdominal muscle function. Patients with complete SCI above T6 demonstrate abdominal muscle activity and maximal spontaneous (or attempted) contractions during TMS, suggesting that TMS can identify the preservation function of the corticospinal pathway by detecting abdominal muscle activity in patients with SCI (54).
TMS can be used to evaluate abnormalities of the corticospinal descending pathway. MEP latency represents TMS conduction time from the motor cortex to the peripheral nerve, including central motor and peripheral nerve conduction times. An abnormal MEP latency may reflect central and peripheral nerve conduction impairment. Nakamae et al. (55) used TMS to detect the MEPs of 831 patients with spinal cord lesions; the MEPs were recorded in the abductor digiti minimi and abductor hallucis muscles. They found that most patients had abnormal MEPs. Compared with the control group, 711 patients had prolonged MEPs, and 493 patients had prolonged central motor conduction times. A previous study tracked MEP changes in thenar muscles using motor cortex TMS from 19 to 1,109 days after injury in patients with incomplete cervical SCI (56). MEP latency was prolonged throughout the follow-up period, probably due to axonal injury, demyelination, and corticospinal tract degeneration (57). Apart from the changes in MEP latency, SCI may also decrease the MEP amplitude and increase the motor threshold (58), which somewhat reflect corticospinal conduction pathway abnormality. TMS can effectively assess the integrity of the corticospinal pathway in patients with SCI. The application of TMS in SCI evaluation is shown in Table 1. Future studies should enhance the accurate identification of SCI severity using TMS, which would strengthen its application in clinical practice and enable the development of targeted rehabilitation programs.
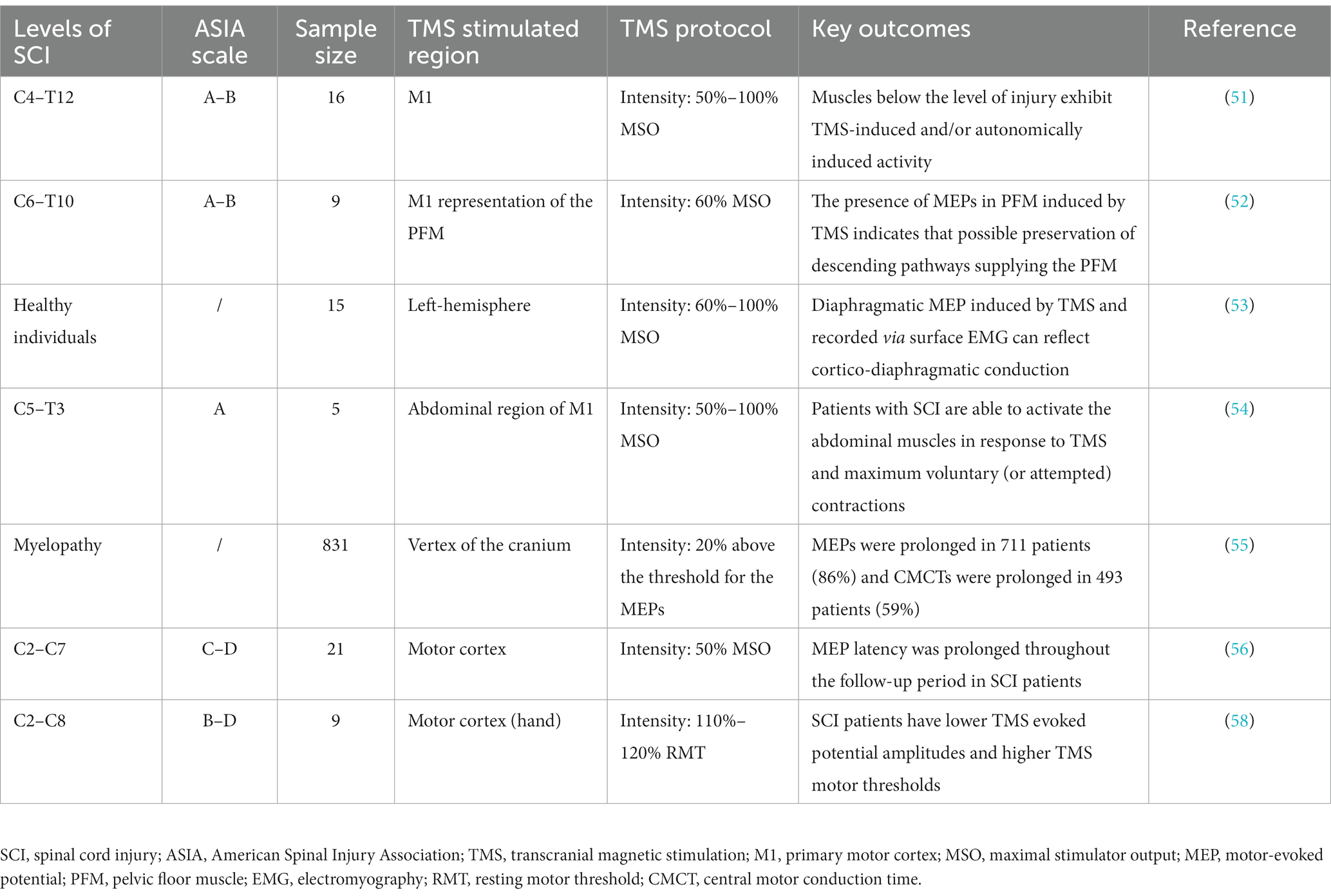
Table 1. Summary of clinical trials evaluating the efficacy of transcranial magnetic stimulation (TMS) for spinal cord injury (SCI).
4.2. Treatment
4.2.1. Motor dysfunction
Motor dysfunction causes independence loss and quality of life decline in patients with SCI. Thus, motor function recovery is the primary goal of rehabilitation. A previous study explored the efficacy of rTMS in the treatment of four patients with incomplete cervical SCI. After continuous motor cortical stimulation for 5 days, TMS significantly improved the upper limb motor function, motor score, and pinprick sensory score (59). This effect may be related to increased motor cortical excitability and decreased corticospinal tract inhibition by rTMS. In addition, rTMS improves lower extremity motor function. Benito et al. (60) found that rTMS with a frequency of 20 Hz, 1800 pulses, and 90% resting motor threshold intensity for 15 days could improve the lower limb function score and gait function of patients with SCI, and the effect could be maintained for 2 weeks after treatment. Similarly, another study reported that routine rehabilitation training combined with rTMS can improve lower limb muscle strength in patients with SCI (35). When the motor cortex of the lower extremities was exposed to rTMS at 20 Hz and 1800 pulses for 4 weeks, the maximum muscle strength of knee flexion and extension in the combined treatment group was significantly higher than that in the routine rehabilitation group. An increasing number of researchers have found the advantage of combination therapy in neural circuit regeneration and reconnection. Increasing the plasticity of the corticospinal junction may be the key to improving motor function after SCI. Wang et al. (61) compared the efficacy of rTMS combined with treadmill training with that of treadmill training only or rTMS intervention only. The motor and coordination functions of rats in the combined treatment group was significantly increased, and the curative effect was better than that of the treadmill or rTMS group. BDNF, synaptophysin, and postsynaptic density protein 95 levels in the cortex and spinal cord were significantly increased in the combined treatment group, suggesting that the combined therapy promoted the plasticity of the motor cortex and spinal cord. The effect of rTMS or treadmill training on spinal cord plasticity is limited; rTMS can only improve the plasticity of the cortex. Although treadmill training can increase the expression of BDNF, it cannot effectively induce the expression of synaptophysin and postsynaptic density protein 95.
In addition to the traditional rTMS protocols, some new stimulation protocols have proven to improve motor dysfunction after SCI. Intermittent theta burst stimulation (iTBS) is a particular scheme of TMS that can induce a long-term potentiation of the motor cortex; iTBS for 190 s can excite the cortex for 60 min (62). Marufa et al. (63) explored the effect of the iTBS protocol on rats with incomplete SCI. After 2 weeks of stimulation, the motor function score and MEP amplitude were significantly increased, and the expression of growth-associated protein 43 (GAP43) in the spinal cord was significantly up-regulated. GAP43 expression is closely related to synaptic formation. GAP43 expression up-regulation promotes innervation and neurogenesis in damaged areas (64), suggesting that TMS promotes axonal regeneration by up-regulating GAP43 expression, thereby improving the motor function of rats. Traditional rTMS at 20 Hz combined with tsDCS (rTMS-20 Hz/tsDCS) can be used to treat brain and spinal cord lesions simultaneously and is advantageous in neuroplasticity improvement. A study compared the efficacy of rTMS-20 Hz/tsDCS and rTMS-iTBS/tsDCS in patients with chronic SCI and found that both stimulation protocols significantly improved MEP latency, MEP amplitude, and lower limb muscle strength score (65). Some recent studies have reported that nerve root magnetic stimulation can improve motor function, enhance nerve conduction, and promote the recovery of synaptic ultrastructure in the sensorimotor cortex of SCI rats (66). Based on the gratifying results of animal experiments, other studies explored the effect of combined magnetic stimulation of the nerve root and cortex on the lower limb motor function of patients with SCI (67). Table 2 shows the results of clinical trials on the efficacy of rTMS in patients with post-SCI motor dysfunction.
4.2.2. NP
NP is a common complication after SCI. A meta-analysis showed that approximately 53% of patients with SCI develop NP (77). Although drugs such as gabapentin and pregabalin can somewhat relieve NP, only 30%–50% of patients experience pain alleviation (78). The latest evidence-based guidelines suggest that rTMS can relieve pain to some extent, and its analgesic effect is grade A (clearly effective) (79). rTMS may be a new alternative treatment for NP. Previous studies have found that rTMS on M1 can reduce NP in patients with SCI, and a significant analgesic effect is obtained within 48 h after the first treatment (68). Furthermore, Sun et al. (69) found that high-frequency rTMS on M1 can enhance the analgesic effect of conventional rehabilitation and drug therapy on NP. As shown by functional near-infrared spectroscopy, it was also observed that rTMS suppressed M1 and premotor cortex activation, which may account for pain relief. In addition, rTMS can relieve acute NP. A study showed that rTMS at 10 Hz in the hand region of the motor cortex could reduce acute NP in the early stage of SCI, improve MEP-related parameters, and regulate the secretion of BDNF and nerve growth factor (70). Moreover, the analgesic effect of rTMS on the abovementioned parameters can last for 2 to 3 weeks. In addition to M1, the prefrontal cortex may also be an effective target for NP treatment after SCI. Nardone et al. (71) stimulated the prefrontal cortex of patients with SCI using 10 Hz rTMS. NP was significantly alleviated after ten courses of treatment; the mechanism of pain relief may be related to the activation of the anterior cingulate gyrus and pain control circuits as well as the release of endogenous opioids during rTMS (12, 80). However, current research findings cannot explain the best target of rTMS for NP treatment, and a comparative study on the effects of M1 and prefrontal cortex stimulation is in progress (73).
In addition to the stimulation targets, other studies compared the efficacy of deep rTMS using H- and figure-eight coils on NP. The results showed that M1 stimulation using the H-coil significantly relieved lower extremity NP, and the visual analog scale score decreased significantly after 1 h of stimulation. In contrast, stimulation using the figure-eight coil did not improve pain (72). Moreover, the frequency, intensity, and number of treatments affected the efficacy of rTMS. Table 2 presents the findings of clinical trials on the effects of rTMS on NP after SCI. However, the current stimulation parameters vary differently with rTMS, and the times for different stimuli to produce curative effects are uneven. Further research is required to evaluate the effective stimulation parameters as well as the short- and long-term curative effects of rTMS on NP.
4.2.3. Spasticity
The prevalence of spasticity after SCI is as high as 65%, and nearly 35% of patients need intervention (81). Medications such as baclofen are commonly used to manage spasticity. However, the long-term use of baclofen can produce side effects such as sedation, lethargy, ataxia, and decreased muscle activity, with limited efficacy in spasticity improvement (82). Previous studies have shown that high-frequency rTMS can reduce spasticity in patients with multiple sclerosis or stroke (83, 84); hence, rTMS may be a new method of relieving spasticity. Kumru et al. (74) stimulated the M1 of patients with SCI using 20 Hz rTMS. After 5 days of intervention, rTMS significantly improved the lower extremity spasticity of 15 patients. Therefore, the use of rTMS to improve spasticity after SCI is safe and feasible. Similarly, in the iTBS protocol, rTMS reduces the modified Ashworth scale score (an SCI assessment tool for spasticity) and the H/M amplitude ratio of the soleus H reflex in patients with SCI, thereby effectively relieving spasticity (71, 75). Notably, rTMS can maintain the effect of this treatment protocol for 1 week after the end of treatment. RTMS can reduce spasticity and create more conditions for the rehabilitation of patients with SCI, which is beneficial for muscle function consolidation and functional recovery promotion.
In addition, combination therapy is effective in improving spasticity. A recent study found that rTMS combined with treadmill training reduced hyperreflexia, improved motor function, and increased the expressions of K+-Cl− cotransporter 2 (KCC2) and glutamic acid decarboxylase 67 (GAD67) in SCI rats (85). The up-regulation of KCC2 expression in the membrane of motor neurons reduces post-SCI spasticity (86). GAD67 expression is strongly correlated with GABA levels in spinal cord inhibitory synapses, and GABA expression inhibits neuronal overexcitation (87). Increasing KCC2 and GAD67 expressions can increase the inhibitory input to motor neurons and rebalance the excitability of motor neurons, thereby improving motor function. In addition to the known molecular mechanisms, some scholars have explored the possible physiological mechanisms by which rTMS relieves spasticity. Nardone et al. (76) evaluated the mutual synaptic inhibition of type Ia motor neurons of the soleus muscle in patients with SCI and concluded that rTMS may reduce segmental spinal cord excitation by enhancing the descending projection between the motor cortex and inhibitory spinal cord neuronal circuit, thereby reducing leg spasticity. This effect reverses the loss of mutual inhibition after SCI and improves the cerebral control of the spinal cord. Table 2 shows the findings of clinical trials investigating the effect of rTMS on spasticity after SCI.
4.2.4. Neurogenic bladder
The sacral voiding center (S2–S4), pontine micturition center, and cerebral cortex are responsible for promoting and inhibiting micturition and maintaining the urinary function. Generally, sympathetic nerves (T10–L2) provide inhibitory input to the bladder, leading to bladder filling. Moreover, bladder emptying is caused by excitatory inputs supplied by parasympathetic nerves (S2–S4) (88). When SCI occurs, communication between the brain and the spinal nerves that control the bladder may be interrupted, resulting in bladder and urethral sphincter dysfunction, leading to the development of a neurogenic bladder (88). Approximately 70% of patients with SCI have neurogenic bladder (89). Neurogenic bladder causes urinary incontinence or retention. It may also be complicated by urinary tract infection as well as kidney and bladder stones, which seriously affect patient quality of life (90). Therefore, improving bladder function significantly improves the quality of life of patients with SCI. rTMS has been shown to regulate the activities of pelvic floor and bladder muscles, and hence rTMS may be an adjuvant therapy for bladder function improvement (91). Jang et al. (92) also found that the left anterior cingulate gyrus is directly related to micturition initiation and coordination. Activating the left anterior cingulate gyrus using rTMS may enhance the functional recovery of patients with bladder sphincter and reflex disorders. Another study explored the efficacy of 1 Hz rTMS of the bilateral dorsolateral prefrontal cortex on bladder pain syndrome treatment (93). At the end of the rTMS regimen, suprapubic pain disappeared completely, and the micturition frequency decreased by 60%–80%, indicating that rTMS can potentially improve bladder function. Moreover, a previous study compared the effects of high- and low-frequency rTMS of the supplementary motor area of the brain on pelvic floor muscle activity and reported that high-frequency rTMS can inhibit pelvic floor muscle activity, and thus may be used to relieve pelvic floor pain (94). In contrast, low-frequency rTMS can increase pelvic floor muscle activity, which may be an effective method of improving urinary incontinence. The two schemes are advantageous in neurogenic bladder management; hence, the appropriate scheme should be selected based on the patient’s condition. Similarly, Brusa et al. (95) reported that low-frequency rTMS of the pelvic floor motor cortex can increase bladder capacity and sensation during the first filling period and reduce bladder overactivity. The mechanism of bladder function improvement may be related to the reverse regulation effect of rTMS on the descending pathway of the corticospinal tract projecting to the detrusor muscle. Nevertheless, a previous study reported that 5 Hz, high-frequency rTMS of the primary motor cortex with a threshold intensity of 100% significantly improved bladder dysfunction during micturition (96). This suggests that high-frequency rTMS may improve detrusor contraction and/or urethral sphincter relaxation by enhancing the excitability of the corticospinal tract. Although previous studies have reported that rTMS can potentially affect the improvement of bladder function in patients with multiple sclerosis-related bladder disease, Parkinson’s disease-related bladder disease, bladder pain syndrome, and other related diseases, the effect of rTMS on post-SCI neurogenic bladder treatment remains unclear (97). Further studies are required to clarify this effect.
4.2.5. Respiratory dysfunction
Patients with high cervical SCI usually develop apparent respiratory muscle paralysis, accompanied by cough reflex and mucociliary clearance disorders, which can cause complications such as pneumonia and respiratory failure in severe cases (98, 99). Therefore, managing post-SCI respiratory dysfunction remains a complex clinical problem. Previous studies have found that magnetic stimulation activates the diaphragm and regulates diaphragmatic excitability by activating the remaining phrenic nerve circuit after cervical SCI (100). Michel-Flutot et al. (101) confirmed that a single high-frequency (10 Hz) rTMS could persistently increase the excitability of the phrenic neural network in anesthetized rats. Moreover, inhibitory rTMS on the supplementary motor area was found to suppress the excitability of the corticospinal pathway to the diaphragm in healthy individuals. Furthermore, high-frequency rTMS can reduce hyperventilation and change the respiratory pattern during inspiratory threshold loading (102, 103). Therefore, rTMS may be a new way to improve respiratory dysfunction after SCI. Michel-Flutot et al. (104) investigated the effects of rTMS on respiratory function at 7 days, 1 month, and 2 months of treatment in rats with C2 spinal cord hemisection. The results showed that regardless of the intervention time, rTMS had no significant effect on the diaphragm activity of the injured side. However, rTMS increased the diaphragm activity of the normal side at 1 month and strengthened the existing cross-phrenic nerve pathway at 2 months, which increased the diaphragm activity of the injured side during asphyxia. This effect may be due to the increased expression of GAP43-positive fibers that enhance the respiratory descending fibers in the ventrolateral funiculus, which in turn induces the plasticity of phrenic nerve cells. Additionally, the results suggest that rTMS alone may not be sufficient to stimulate significant diaphragmatic function recovery. It should be used in combination with other treatments to enhance its efficacy. A randomized controlled trial compared the effects of rTMS combined with respiratory training and respiratory training alone on pulmonary function in patients with ischemic stroke. Combined therapy significantly improved the pulmonary function of patients with ischemic stroke, and the effect was significantly better than that of patients in the respiratory training group (105). Therefore, rTMS seems to be an auxiliary method of respiratory function improvement. However, these results should be carefully considered due to the limited number of related studies. A large number of studies are needed to confirm the efficacy of rTMS in respiratory dysfunction management.
5. Conclusion and prospects
SCI causes severe sensorimotor dysfunction by damaging the corticospinal system, often resulting in lifelong disability. In addition, SCI causes an imbalance between cerebral cortical excitability and inhibition (106). Regulating the imbalance between excitability and inhibition of cortical and corticospinal tract networks may be the focus of SCI treatment. A previous study reported that dual tDCS inhibition the unaffected lateral hemispheres of patients with stroke through cathodic stimulation, thereby reducing its inhibition on the affected lateral hemispheres (107). Subsequently, the affected hemispheres were activated by anodic stimulation, which increased their MEPs, thereby improving the balance of cerebral excitability and inhibition at the central level. TMS can also affect cortical excitability and inhibition at the central level. On the one hand, TMS can improve the motor function of patients with SCI by increasing corticospinal tract excitability. On the other hand, TMS can inhibit abnormal cortical excitability to relieve spasticity and NP. Therefore, the selection of excitatory and inhibitory parameters should be determined according to the specific conditions of the patients and the specific time after the injury.
Currently, rehabilitation has focused on reducing complications, improving the dysfunction, and ameliorating the quality of life of patients with SCI. As a non-invasive tool that might influence cortical excitability, TMS has demonstrated some curative effects on SCI and its complications, such as motor dysfunction, NP, spasticity, neurogenic bladder, and respiratory dysfunction. TMS may be a new method for SCI evaluation and treatment. Different TMS treatment schemes can alleviate various complications at the same time (35, 108). Furthermore, TMS can be used as an adjuvant treatment to enhance the efficacy of the initial treatment (69, 109). However, TMS is mostly applied in SCI management via basic research and experimental clinical studies; more research is required to promote its clinical application. In addition, the TMS regimens used in most studies are different; hence, the appropriate stimulation targets and parameters remain unclear. Notably, the effects of TMS on neuroplasticity in the central nervous system require further clarification, as the exact mechanisms remain unelucidated. Therefore, more large-scale, multicenter randomized controlled trials should be conducted to determine the efficacy and safety of TMS. Moreover, the mechanism of TMS on SCI rehabilitation should be continuously explored. Some non-invasive brain stimulation techniques such as tDCS have been proven to relieve spasticity and NP caused by stroke, multiple sclerosis, SCI, and other neurological diseases; furthermore, these techniques somewhat improve patient motor function (110, 111). Scholars should carefully consider these results and design more clinical trials to compare the efficacies of TMS and tDCS in SCI management.
Author contributions
All authors listed have made a substantial, direct, and intellectual contribution to the work and approved it for publication.
Funding
This work was supported by the National Natural Science Foundation of China (82160442, 31960178, and 82160923); Applied Basic Research Programs of Science and Technology Commission Foundation of Yunnan Province (2019FA007), and Kunming Medical University Joint Special Project (2019FE001(-300)).
Acknowledgments
The authors would like to thank Qi Sun for providing the figure in this article. The authors thank LetPub (https://www.letpub.com) for language assistance during manuscript preparation.
Conflict of interest
The authors declare that the research was conducted in the absence of any commercial or financial relationships that could be construed as a potential conflict of interest.
Publisher’s note
All claims expressed in this article are solely those of the authors and do not necessarily represent those of their affiliated organizations, or those of the publisher, the editors and the reviewers. Any product that may be evaluated in this article, or claim that may be made by its manufacturer, is not guaranteed or endorsed by the publisher.
References
1. Guest, J, Datta, N, Jimsheleishvili, G, and Gater, DR. Pathophysiology, classification and comorbidities after traumatic spinal cord injury. J Pers Med. (2022) 12:1126. doi: 10.3390/jpm12071126
2. Ding, W, Hu, S, Wang, P, Kang, H, Peng, R, Dong, Y, et al. Spinal cord injury: the global incidence, prevalence, and disability from the global burden of disease study 2019. Spine. (2022) 47:1532–40. doi: 10.1097/BRS.0000000000004417
3. Chen, C, Qiao, X, Liu, W, Fekete, C, and Reinhardt, JD. Epidemiology of spinal cord injury in China: a systematic review of the Chinese and English literature. Spinal Cord. (2022) 60:1050–61. doi: 10.1038/s41393-022-00826-6
4. Hu, S, Wang, P, Dong, Y, and Li, F. Incidence, prevalence and disability of spinal cord injury in China from 1990 to 2019: a systematic analysis of the global burden of disease study 2019. Eur Spine J. (2022) 32:590–600. doi: 10.1007/s00586-022-07441-2
5. Krueger, H, Noonan, VK, Trenaman, LM, Joshi, P, and Rivers, CS. The economic burden of traumatic spinal cord injury in Canada. Chronic Dis Inj Can. (2013) 33:113–22. doi: 10.24095/hpcdp.33.3.01
6. Venkatesh, K, Ghosh, SK, Mullick, M, Manivasagam, G, and Sen, D. Spinal cord injury: pathophysiology, treatment strategies, associated challenges, and future implications. Cell Tissue Res. (2019) 377:125–51. doi: 10.1007/s00441-019-03039-1
7. Huang, H, Young, W, Skaper, S, Chen, L, Moviglia, G, Saberi, H, et al. Clinical Neurorestorative therapeutic guidelines for spinal cord injury (IANR/CANR version 2019). J Orthop Translat. (2020) 20:14–24. doi: 10.1016/j.jot.2019.10.006
8. Choi, EH, Gattas, S, Brown, NJ, Hong, JD, Limbo, JN, Chan, AY, et al. Epidural electrical stimulation for spinal cord injury. Neural Regen Res. (2021) 16:2367–75. doi: 10.4103/1673-5374.313017
9. Taylor, C, McHugh, C, Mockler, D, Minogue, C, Reilly, RB, and Fleming, N. Transcutaneous spinal cord stimulation and motor responses in individuals with spinal cord injury: a methodological review. PLoS One. (2021) 16:e0260166. doi: 10.1371/journal.pone.0260166
10. Taylor, JJ, Newberger, NG, Stern, AP, Phillips, A, Feifel, D, Betensky, RA, et al. Seizure risk with repetitive TMS: survey results from over a half-million treatment sessions. Brain Stimul. (2021) 14:965–73. doi: 10.1016/j.brs.2021.05.012
11. Cogiamanian, F, Ardolino, G, Vergari, M, Ferrucci, R, Ciocca, M, Scelzo, E, et al. Transcutaneous spinal direct current stimulation. Front Psychiatry. (2012) 3:63. doi: 10.3389/fpsyt.2012.00063
12. Li, C, Jirachaipitak, S, Wrigley, P, Xu, H, and Euasobhon, P. Transcranial direct current stimulation for spinal cord injury-associated neuropathic pain. Korean J Pain. (2021) 34:156–64. doi: 10.3344/kjp.2021.34.2.156
13. Jovanovic, LI, Kapadia, N, Zivanovic, V, Rademeyer, HJ, Alavinia, M, McGillivray, C, et al. Brain-computer interface-triggered functional electrical stimulation therapy for rehabilitation of reaching and grasping after spinal cord injury: a feasibility study. Spinal Cord Ser Cases. (2021) 7:24. doi: 10.1038/s41394-020-00380-4
14. Wincek, A, Huber, J, Leszczyńska, K, Fortuna, W, Okurowski, S, Chmielak, K, et al. The long-term effect of treatment using the transcranial magnetic stimulation rTMS in patients after incomplete cervical or thoracic spinal cord injury. J Clin Med. (2021) 10:2975. doi: 10.3390/jcm10132975
15. Fava de Lima, F, Silva, CR, and Kohn, AF. Transcutaneous spinal direct current stimulation (tsDCS) does not affect postural sway of young and healthy subjects during quiet upright standing. PLoS One. (2022) 17:e0267718. doi: 10.1371/journal.pone.0267718
16. James, ND, McMahon, SB, Field-Fote, EC, and Bradbury, EJ. Neuromodulation in the restoration of function after spinal cord injury. Lancet Neurol. (2018) 17:905–17. doi: 10.1016/s1474-4422(18)30287-4
17. Di Lazzaro, V, Pilato, F, Dileone, M, Profice, P, Oliviero, A, Mazzone, P, et al. The physiological basis of the effects of intermittent theta burst stimulation of the human motor cortex. J Physiol. (2008) 586:3871–9. doi: 10.1113/jphysiol.2008.152736
18. Anjum, A, Yazid, MD, Fauzi Daud, M, Idris, J, Ng, AMH, Selvi Naicker, A, et al. Spinal cord injury: pathophysiology, multimolecular interactions, and underlying recovery mechanisms. Int J Mol Sci. (2020) 21:7533. doi: 10.3390/ijms21207533
19. Zhang, Y, Al Mamun, A, Yuan, Y, Lu, Q, Xiong, J, Yang, S, et al. Acute spinal cord injury: pathophysiology and pharmacological intervention (review). Mol Med Rep. (2021) 23:417. doi: 10.3892/mmr.2021.12056
20. Hellenbrand, DJ, Quinn, CM, Piper, ZJ, Morehouse, CN, Fixel, JA, and Hanna, AS. Inflammation after spinal cord injury: a review of the critical timeline of signaling cues and cellular infiltration. J Neuroinflammation. (2021) 18:284. doi: 10.1186/s12974-021-02337-2
21. Alizadeh, A, Dyck, SM, and Karimi-Abdolrezaee, S. Traumatic spinal cord injury: an overview of pathophysiology, models and acute injury mechanisms. Front Neurol. (2019) 10:282. doi: 10.3389/fneur.2019.00282
22. Duncan, GJ, Simkins, TJ, and Emery, B. Neuron-oligodendrocyte interactions in the structure and integrity of axons. Front Cell Dev Biol. (2021) 9:653101. doi: 10.3389/fcell.2021.653101
23. Almad, A, Sahinkaya, FR, and McTigue, DM. Oligodendrocyte fate after spinal cord injury. Neurotherapeutics. (2011) 8:262–73. doi: 10.1007/s13311-011-0033-5
24. Tran, AP, Warren, PM, and Silver, J. The biology of regeneration failure and success after spinal cord injury. Physiol Rev. (2018) 98:881–917. doi: 10.1152/physrev.00017.2017
25. Klomjai, W, Katz, R, and Lackmy-Vallee, A. Basic principles of transcranial magnetic stimulation (TMS) and repetitive TMS (rTMS). Ann Phys Rehabil Med. (2015) 58:208–13. doi: 10.1016/j.rehab.2015.05.005
26. Li, Y, Chen, K, Wang, J, Lu, H, Li, X, Yang, L, et al. Research progress on transcranial magnetic stimulation for post-stroke dysphagia. Front Behav Neurosci. (2022) 16:995614. doi: 10.3389/fnbeh.2022.995614
27. Tang, AD, Bennett, W, Bindoff, AD, Bolland, S, Collins, J, Langley, RC, et al. Subthreshold repetitive transcranial magnetic stimulation drives structural synaptic plasticity in the young and aged motor cortex. Brain Stimul. (2021) 14:1498–507. doi: 10.1016/j.brs.2021.10.001
28. Qian, FF, He, YH, Du, XH, Lu, HX, He, RH, and Fan, JZ. Repetitive transcranial magnetic stimulation promotes neurological functional recovery in rats with traumatic brain injury by upregulating synaptic plasticity-related proteins. Neural Regen Res. (2023) 18:368–74. doi: 10.4103/1673-5374.346548
29. Cullen, CL, Senesi, M, Tang, AD, Clutterbuck, MT, Auderset, L, O’Rourke, ME, et al. Low-intensity transcranial magnetic stimulation promotes the survival and maturation of newborn oligodendrocytes in the adult mouse brain. Glia. (2019) 67:1462–77. doi: 10.1002/glia.23620
30. Luo, J, Feng, Y, Li, M, Yin, M, Qin, F, and Hu, X. Repetitive transcranial magnetic stimulation improves neurological function and promotes the anti-inflammatory polarization of microglia in ischemic rats. Front Cell Neurosci. (2022) 16:878345. doi: 10.3389/fncel.2022.878345
31. Adu, MK, Shalaby, R, Chue, P, and Agyapong, VIO. Repetitive transcranial magnetic stimulation for the treatment of resistant depression: a scoping review. Behav Sci. (2022) 12. doi: 10.3390/bs12060195
32. Roach, AE, Hines, C, Stafford, J, and Mooney, S. Repetitive transcranial magnetic stimulation for treatment-resistant depression in active-duty service members improves depressive symptoms. J ECT. (2020) 36:279–84. doi: 10.1097/YCT.0000000000000680
33. Rossi, S, Antal, A, Bestmann, S, Bikson, M, Brewer, C, Brockmoller, J, et al. Safety and recommendations for TMS use in healthy subjects and patient populations, with updates on training, ethical and regulatory issues: expert guidelines. Clin Neurophysiol. (2021) 132:269–306. doi: 10.1016/j.clinph.2020.10.003
34. Cosmo, C, Zandvakili, A, Petrosino, NJ, Berlow, YA, and Philip, NS. Repetitive transcranial magnetic stimulation for treatment-resistant depression: recent critical advances in patient care. Curr Treat Options Psychiatry. (2021) 8:47–63. doi: 10.1007/s40501-021-00238-y
35. Krogh, S, Aagaard, P, Jonsson, AB, Figlewski, K, and Kasch, H. Effects of repetitive transcranial magnetic stimulation on recovery in lower limb muscle strength and gait function following spinal cord injury: a randomized controlled trial. Spinal Cord. (2022) 60:135–41. doi: 10.1038/s41393-021-00703-8
36. Wei, Z, Fu, J, Liang, H, Liu, M, Ye, X, and Zhong, P. The therapeutic efficacy of transcranial magnetic stimulation in managing Alzheimer’s disease: a systemic review and meta-analysis. Front Aging Neurosci. (2022) 14:980998. doi: 10.3389/fnagi.2022.980998
37. Wu, Y, Cao, XB, Zeng, WQ, Zhai, H, Zhang, XQ, Yang, XM, et al. Transcranial magnetic stimulation alleviates levodopa-induced dyskinesia in Parkinson’s disease and the related mechanisms: a mini-review. Front Neurol. (2021) 12:758345. doi: 10.3389/fneur.2021.758345
38. Zhou, X, Li, K, Chen, S, Zhou, W, Li, J, Huang, Q, et al. Clinical application of transcranial magnetic stimulation in multiple sclerosis. Front Immunol. (2022) 13:902658. doi: 10.3389/fimmu.2022.902658
39. He, Y, Li, K, Chen, Q, Yin, J, and Bai, D. Repetitive transcranial magnetic stimulation on motor recovery for patients with stroke: a PRISMA compliant systematic review and meta-analysis. Am J Phys Med Rehabil. (2020) 99:99–108. doi: 10.1097/PHM.0000000000001277
40. Lorentzen, R, Nguyen, TD, McGirr, A, Hieronymus, F, and Østergaard, SD. The efficacy of transcranial magnetic stimulation (TMS) for negative symptoms in schizophrenia: a systematic review and meta-analysis. Schizophrenia. (2022) 8:35. doi: 10.1038/s41537-022-00248-6
41. Eldaief, MC, Press, DZ, and Pascual-Leone, A. Transcranial magnetic stimulation in neurology: a review of established and prospective applications. Neurol Clin Pract. (2013) 3:519–26. doi: 10.1212/01.CPJ.0000436213.11132.8e
42. Torii, T, Sato, A, Iwahashi, M, and Iramina, K. Using repetitive paired-pulse transcranial magnetic stimulation for evaluation motor cortex excitability. AIP Adv. (2019) 9:125224. doi: 10.1063/1.5129299
43. Wang, J, Deng, XP, Wu, YY, Li, XL, Feng, ZJ, Wang, HX, et al. High-frequency rTMS of the motor cortex modulates cerebellar and widespread activity as revealed by SVM. Front Neurosci. (2020) 14:186. doi: 10.3389/fnins.2020.00186
44. Alder, G, Signal, N, Olsen, S, and Taylor, D. A systematic review of paired associative stimulation (PAS) to modulate lower limb corticomotor excitability: implications for stimulation parameter selection and experimental design. Front Neurosci. (2019) 13:895. doi: 10.3389/fnins.2019.00895
45. Jannati, A, Oberman, LM, Rotenberg, A, and Pascual-Leone, A. Assessing the mechanisms of brain plasticity by transcranial magnetic stimulation. Neuropsychopharmacology. (2023) 48:191–208. doi: 10.1038/s41386-022-01453-8
46. Wolters, A, Sandbrink, F, Schlottmann, A, Kunesch, E, Stefan, K, Cohen, LG, et al. A temporally asymmetric Hebbian rule governing plasticity in the human motor cortex. J Neurophysiol. (2003) 89:2339–45. doi: 10.1152/jn.00900.2002
47. Habekost, B, Germann, M, and Baker, SN. Plastic changes in primate motor cortex following paired peripheral nerve stimulation. J Neurophysiol. (2021) 125:458–75. doi: 10.1152/jn.00288.2020
48. Ueno, S, and Sekino, M. Figure-eight coils for magnetic stimulation: from focal stimulation to deep stimulation. Front Hum Neurosci. (2021) 15:805971. doi: 10.3389/fnhum.2021.805971
49. Lu, M, and Ueno, S. Comparison of the induced fields using different coil configurations during deep transcranial magnetic stimulation. PLoS One. (2017) 12:e0178422. doi: 10.1371/journal.pone.0178422
50. Arora, T, Desai, N, Kirshblum, S, and Chen, R. Utility of transcranial magnetic stimulation in the assessment of spinal cord injury: current status and future directions. Front Rehabil Sci. (2022) 3:1005111. doi: 10.3389/fresc.2022.1005111
51. Squair, JW, Bjerkefors, A, Inglis, JT, Lam, T, and Carpenter, MG. Cortical and vestibular stimulation reveal preserved descending motor pathways in individuals with motor-complete spinal cord injury. J Rehabil Med. (2016) 48:589–96. doi: 10.2340/16501977-2101
52. Williams, AMM, Eginyan, G, Deegan, E, Chow, M, Carpenter, MG, and Lam, T. Residual innervation of the pelvic floor muscles in people with motor-complete spinal cord injury. J Neurotrauma. (2020) 37:2320–31. doi: 10.1089/neu.2019.6908
53. Welch, JF, Argento, PJ, Mitchell, GS, and Fox, EJ. Reliability of diaphragmatic motor-evoked potentials induced by transcranial magnetic stimulation. J Appl Physiol. (2020) 129:1393–404. doi: 10.1152/japplphysiol.00486.2020
54. Bjerkefors, A, Squair, JW, Chua, R, Lam, T, Chen, Z, and Carpenter, MG. Assessment of abdominal muscle function in individuals with motor-complete spinal cord injury above T6 in response to transcranial magnetic stimulation. J Rehabil Med. (2015) 47:138–46. doi: 10.2340/16501977-1901
55. Nakamae, T, Tanaka, N, Nakanishi, K, Fujimoto, Y, Sasaki, H, Kamei, N, et al. Quantitative assessment of myelopathy patients using motor evoked potentials produced by transcranial magnetic stimulation. Eur Spine J. (2010) 19:685–90. doi: 10.1007/s00586-009-1246-8
56. Smith, HC, Savic, G, Frankel, HL, Ellaway, PH, Maskill, DW, Jamous, MA, et al. Corticospinal function studied over time following incomplete spinal cord injury. Spinal Cord. (2000) 38:292–300. doi: 10.1038/sj.sc.3100994
57. Oudega, M, and Perez, MA. Corticospinal reorganization after spinal cord injury. J Physiol. (2012) 590:3647–63. doi: 10.1113/jphysiol.2012.233189
58. Sfreddo, HJ, Wecht, JR, Alsalman, OA, Wu, YK, and Harel, NY. Duration and reliability of the silent period in individuals with spinal cord injury. Spinal Cord. (2021) 59:885–93. doi: 10.1038/s41393-021-00649-x
59. Belci, M, Catley, M, Husain, M, Frankel, HL, and Davey, NJ. Magnetic brain stimulation can improve clinical outcome in incomplete spinal cord injured patients. Spinal Cord. (2004) 42:417–9. doi: 10.1038/sj.sc.3101613
60. Benito, J, Kumru, H, Murillo, N, Costa, U, Medina, J, Tormos, JM, et al. Motor and gait improvement in patients with incomplete spinal cord injury induced by high-frequency repetitive transcranial magnetic stimulation. Top Spinal Cord Inj Rehabil. (2012) 18:106–12. doi: 10.1310/sci1802-106
61. Wang, P, Yin, R, Wang, S, Zhou, T, Zhang, Y, Xiao, M, et al. Effects of repetitive transcranial magnetic stimulation (rTMS) and treadmill training on recovery of motor function in a rat model of partial spinal cord injury. Med Sci Monit. (2021) 27:e931601. doi: 10.12659/msm.931601
62. Wischnewski, M, and Schutter, DJ. Efficacy and time course of theta burst stimulation in healthy humans. Brain Stimul. (2015) 8:685–92. doi: 10.1016/j.brs.2015.03.004
63. Marufa, SA, Hsieh, TH, Liou, JC, Chen, HY, and Peng, CW. Neuromodulatory effects of repetitive transcranial magnetic stimulation on neural plasticity and motor functions in rats with an incomplete spinal cord injury: a preliminary study. PLoS One. (2021) 16:e0252965. doi: 10.1371/journal.pone.0252965
64. Chung, D, Shum, A, and Caraveo, G. GAP-43 and BASP1 in axon regeneration: implications for the treatment of neurodegenerative diseases. Front Cell Dev Biol. (2020) 8:567537. doi: 10.3389/fcell.2020.567537
65. Adeel, M, Lai, CH, Lin, BS, Chan, WP, Liou, JC, Wu, CW, et al. Effects of paired stimulation with specific waveforms on cortical and spinal plasticity in subjects with a chronic spinal cord injury. J Formos Med Assoc. (2022) 121:2044–56. doi: 10.1016/j.jfma.2022.02.016
66. Zheng, Y, Zhao, D, Xue, DD, Mao, YR, Cao, LY, Zhang, Y, et al. Nerve root magnetic stimulation improves locomotor function following spinal cord injury with electrophysiological improvements and cortical synaptic reconstruction. Neural Regen Res. (2022) 17:2036–42. doi: 10.4103/1673-5374.335161
67. Sun, TT, Zhu, GY, Zheng, Y, Mao, YR, Hu, QL, Song, GM, et al. Effects of paired associative magnetic stimulation between nerve root and cortex on motor function of lower limbs after spinal cord injury: study protocol for a randomized controlled trial. Neural Regen Res. (2022) 17:2459–64. doi: 10.4103/1673-5374.339012
68. Jetté, F, Côté, I, Meziane, HB, and Mercier, C. Effect of single-session repetitive transcranial magnetic stimulation applied over the hand versus leg motor area on pain after spinal cord injury. Neurorehabil Neural Repair. (2013) 27:636–43. doi: 10.1177/1545968313484810
69. Sun, X, Long, H, Zhao, C, Duan, Q, Zhu, H, Chen, C, et al. Analgesia-enhancing effects of repetitive transcranial magnetic stimulation on neuropathic pain after spinal cord injury: an fNIRS study. Restor Neurol Neurosci. (2019) 37:497–507. doi: 10.3233/RNN-190934
70. Zhao, CG, Sun, W, Ju, F, Wang, H, Sun, XL, Mou, X, et al. Analgesic effects of directed repetitive transcranial magnetic stimulation in acute neuropathic pain after spinal cord injury. Pain Med. (2020) 21:1216–23. doi: 10.1093/pm/pnz290
71. Nardone, R, Höller, Y, Langthaler, PB, Lochner, P, Golaszewski, S, Schwenker, K, et al. rTMS of the prefrontal cortex has analgesic effects on neuropathic pain in subjects with spinal cord injury. Spinal Cord. (2017) 55:20–5. doi: 10.1038/sc.2016.87
72. Shimizu, T, Hosomi, K, Maruo, T, Goto, Y, Yokoe, M, Kageyama, Y, et al. Efficacy of deep rTMS for neuropathic pain in the lower limb: a randomized, double-blind crossover trial of an H-coil and figure-8 coil. J Neurosurg. (2017) 127:1172–80. doi: 10.3171/2016.9.JNS16815
73. Huang, M, Luo, X, Zhang, C, Xie, YJ, Wang, L, Wan, T, et al. Effects of repeated transcranial magnetic stimulation in the dorsolateral prefrontal cortex versus motor cortex in patients with neuropathic pain after spinal cord injury: a study protocol. BMJ Open. (2022) 12:e053476. doi: 10.1136/bmjopen-2021-053476
74. Kumru, H, Murillo, N, Samso, JV, Valls-Sole, J, Edwards, D, Pelayo, R, et al. Reduction of spasticity with repetitive transcranial magnetic stimulation in patients with spinal cord injury. Neurorehabil Neural Repair. (2010) 24:435–41. doi: 10.1177/1545968309356095
75. Nardone, R, Langthaler, PB, Orioli, A, Holler, P, Holler, Y, Frey, VN, et al. Effects of intermittent theta burst stimulation on spasticity after spinal cord injury. Restor Neurol Neurosci. (2017) 35:287–94. doi: 10.3233/RNN-160701
76. Nardone, R, Holler, Y, Thomschewski, A, Brigo, F, Orioli, A, Holler, P, et al. rTMS modulates reciprocal inhibition in patients with traumatic spinal cord injury. Spinal Cord. (2014) 52:831–5. doi: 10.1038/sc.2014.136
77. Burke, D, Fullen, BM, Stokes, D, and Lennon, O. Neuropathic pain prevalence following spinal cord injury: a systematic review and meta-analysis. Eur J Pain. (2017) 21:29–44. doi: 10.1002/ejp.905
78. Finnerup, NB, Otto, M, McQuay, HJ, Jensen, TS, and Sindrup, SH. Algorithm for neuropathic pain treatment: an evidence based proposal. Pain. (2005) 118:289–305. doi: 10.1016/j.pain.2005.08.013
79. Lefaucheur, JP, Aleman, A, Baeken, C, Benninger, DH, Brunelin, J, Di Lazzaro, V, et al. Evidence-based guidelines on the therapeutic use of repetitive transcranial magnetic stimulation (rTMS): an update (2014–2018). Clin Neurophysiol. (2020) 131:474–528. doi: 10.1016/j.clinph.2019.11.002
80. Li, L, Huang, H, Yu, Y, Jia, Y, Liu, Z, Shi, X, et al. Non-invasive brain stimulation for neuropathic pain after spinal cord injury: a systematic review and network meta-analysis. Front Neurosci. (2021) 15:800560. doi: 10.3389/fnins.2021.800560
81. Holtz, KA, Lipson, R, Noonan, VK, Kwon, BK, and Mills, PB. Prevalence and effect of problematic spasticity after traumatic spinal cord injury. Arch Phys Med Rehabil. (2017) 98:1132–8. doi: 10.1016/j.apmr.2016.09.124
82. Billington, ZJ, Henke, AM, and Gater, DR Jr. Spasticity management after spinal cord injury: the here and now. J Pers Med. (2022) 12:808. doi: 10.3390/jpm12050808
83. Mally, J, and Dinya, E. Recovery of motor disability and spasticity in post-stroke after repetitive transcranial magnetic stimulation (rTMS). Brain Res Bull. (2008) 76:388–95. doi: 10.1016/j.brainresbull.2007.11.019
84. Mori, F, Codeca, C, Kusayanagi, H, Monteleone, F, Boffa, L, Rimano, A, et al. Effects of intermittent theta burst stimulation on spasticity in patients with multiple sclerosis. Eur J Neurol. (2010) 17:295–300. doi: 10.1111/j.1468-1331.2009.02806.x
85. Wang, S, Wang, P, Yin, R, Xiao, M, Zhang, Y, Reinhardt, JD, et al. Combination of repetitive transcranial magnetic stimulation and treadmill training reduces hyperreflexia by rebalancing motoneuron excitability in rats after spinal cord contusion. Neurosci Lett. (2022) 775:136536. doi: 10.1016/j.neulet.2022.136536
86. Bilchak, JN, Yeakle, K, Caron, G, Malloy, D, and Cote, MP. Enhancing KCC2 activity decreases hyperreflexia and spasticity after chronic spinal cord injury. Exp Neurol. (2021) 338:113605. doi: 10.1016/j.expneurol.2021.113605
87. Lau, CG, and Murthy, VN. Activity-dependent regulation of inhibition via GAD67. J Neurosci. (2012) 32:8521–31. doi: 10.1523/JNEUROSCI.1245-12.2012
88. Perez, NE, Godbole, NP, Amin, K, Syan, R, and Gater, DR Jr. Neurogenic bladder physiology, pathogenesis, and management after spinal cord injury. J Pers Med. (2022) 12:968. doi: 10.3390/jpm12060968
89. Alsulihem, A, and Corcos, J. Evaluation, treatment, and surveillance of neurogenic detrusor overactivity in spinal cord injury patients. Neurol.: Neuroimmunol. (2019) 6:13. doi: 10.20517/2347-8659.2019.007
90. Best, KL, Ethans, K, Craven, BC, Noreau, L, and Hitzig, SL. Identifying and classifying quality of life tools for neurogenic bladder function after spinal cord injury: a systematic review. J Spinal Cord Med. (2017) 40:505–29. doi: 10.1080/10790268.2016.1226700
91. Nardone, R, Versace, V, Sebastianelli, L, Brigo, F, Golaszewski, S, Christova, M, et al. Transcranial magnetic stimulation and bladder function: a systematic review. Clin Neurophysiol. (2019) 130:2032–7. doi: 10.1016/j.clinph.2019.08.020
92. Jang, Y, Tran, K, Shi, Z, Christof, K, Choksi, D, Salazar, BH, et al. Predictors for outcomes of noninvasive, individualized transcranial magnetic neuromodulation in multiple sclerosis women with neurogenic voiding dysfunction. Continence. (2022) 4:100517. doi: 10.1016/j.cont.2022.100517
93. Nizard, J, Esnault, J, Bouche, B, Suarez Moreno, A, Lefaucheur, JP, and Nguyen, JP. Long-term relief of painful bladder syndrome by high-intensity, low-frequency repetitive transcranial magnetic stimulation of the right and left dorsolateral prefrontal cortices. Front Neurosci. (2018) 12:925. doi: 10.3389/fnins.2018.00925
94. Yani, MS, Fenske, SJ, Rodriguez, LV, and Kutch, JJ. Motor cortical neuromodulation of pelvic floor muscle tone: potential implications for the treatment of urologic conditions. Neurourol Urodyn. (2019) 38:1517–23. doi: 10.1002/nau.24014
95. Brusa, L, Finazzi Agro, E, Petta, F, Sciobica, F, Torriero, S, Lo Gerfo, E, et al. Effects of inhibitory rTMS on bladder function in Parkinson’s disease patients. Mov Disord. (2009) 24:445–7. doi: 10.1002/mds.22434
96. Centonze, D, Petta, F, Versace, V, Rossi, S, Torelli, F, Prosperetti, C, et al. Effects of motor cortex rTMS on lower urinary tract dysfunction in multiple sclerosis. Mult Scler. (2007) 13:269–71. doi: 10.1177/1352458506070729
97. Xu, L, Fu, C, Zhang, Q, Xiong, F, Peng, L, Liang, Z, et al. Efficacy of biofeedback, repetitive transcranial magnetic stimulation and pelvic floor muscle training for female neurogenic bladder dysfunction after spinal cord injury: a study protocol for a randomised controlled trial. BMJ Open. (2020) 10:e034582. doi: 10.1136/bmjopen-2019-034582
98. Berlowitz, DJ, Wadsworth, B, and Ross, J. Respiratory problems and management in people with spinal cord injury. Breathe. (2016) 12:328–40. doi: 10.1183/20734735.012616
99. Locke, KC, Randelman, ML, Hoh, DJ, Zholudeva, LV, and Lane, MA. Respiratory plasticity following spinal cord injury: perspectives from mouse to man. Neural Regen Res. (2022) 17:2141–8. doi: 10.4103/1673-5374.335839
100. Lee, KZ, Liou, LM, Vinit, S, and Ren, MY. Rostral-caudal effect of cervical magnetic stimulation on the diaphragm motor evoked potential after cervical spinal cord contusion in the rat. J Neurotrauma. (2022) 39:683–700. doi: 10.1089/neu.2021.0403
101. Michel-Flutot, P, Zholudeva, LV, Randelman, ML, Deramaudt, TB, Mansart, A, Alvarez, JC, et al. High frequency repetitive transcranial magnetic stimulation promotes long lasting phrenic motoneuron excitability via GABAergic networks. Respir Physiol Neurobiol. (2021) 292:103704. doi: 10.1016/j.resp.2021.103704
102. Laviolette, L, Nierat, MC, Hudson, AL, Raux, M, Allard, E, and Similowski, T. The supplementary motor area exerts a tonic excitatory influence on corticospinal projections to phrenic motoneurons in awake humans. PLoS One. (2013) 8:e62258. doi: 10.1371/journal.pone.0062258
103. Nierat, MC, Hudson, AL, Chaskalovic, J, Similowski, T, and Laviolette, L. Repetitive transcranial magnetic stimulation over the supplementary motor area modifies breathing pattern in response to inspiratory loading in normal humans. Front Physiol. (2015) 6:273. doi: 10.3389/fphys.2015.00273
104. Michel-Flutot, P, Jesus, I, Vanhee, V, Bourcier, CH, Emam, L, Ouguerroudj, A, et al. Effects of chronic high-frequency rTMS protocol on respiratory neuroplasticity following C2 spinal cord hemisection in rats. Biology. (2022) 11:473. doi: 10.3390/biology11030473
105. Cao, H, Chen, X, Ren, X, Chen, Z, Liu, C, Ni, J, et al. Repetitive transcranial magnetic stimulation combined with respiratory muscle training for pulmonary rehabilitation after ischemic stroke-a randomized, case-control study. Front Aging Neurosci. (2022) 14:1006696. doi: 10.3389/fnagi.2022.1006696
106. Benedetti, B, Weidenhammer, A, Reisinger, M, and Couillard-Despres, S. Spinal cord injury and loss of cortical inhibition. Int J Mol Sci. (2022) 23:5622. doi: 10.3390/ijms23105622
107. Morone, G, Capone, F, Iosa, M, Cruciani, A, Paolucci, M, Martino Cinnera, A, et al. May dual transcranial direct current stimulation enhance the efficacy of robot-assisted therapy for promoting upper limb recovery in chronic stroke? Neurorehabil Neural Repair. (2022) 36:800–9. doi: 10.1177/15459683221138743
108. Hou, J, Nelson, R, Nissim, N, Parmer, R, Thompson, FJ, and Bose, P. Effect of combined treadmill training and magnetic stimulation on spasticity and gait impairments after cervical spinal cord injury. J Neurotrauma. (2014) 31:1088–106. doi: 10.1089/neu.2013.3096
109. Yamanaka, H, Takata, Y, Nakagawa, H, Isosaka-Yamanaka, T, Yamashita, T, and Takada, M. An enhanced therapeutic effect of repetitive transcranial magnetic stimulation combined with antibody treatment in a primate model of spinal cord injury. PLoS One. (2021) 16:e0252023. doi: 10.1371/journal.pone.0252023
110. Palm, U, Chalah, MA, Padberg, F, Al-Ani, T, Abdellaoui, M, Sorel, M, et al. Effects of transcranial random noise stimulation (tRNS) on affect, pain and attention in multiple sclerosis. Restor Neurol Neurosci. (2016) 34:189–99. doi: 10.3233/rnn-150557
Keywords: spinal cord injury, transcranial magnetic stimulation, rehabilitation, neuroplasticity, non-invasive brain stimulation
Citation: Wang Y, Dong T, Li X, Zhao H, Yang L, Xu R, Fu Y, Li L, Gai X and Qin D (2023) Research progress on the application of transcranial magnetic stimulation in spinal cord injury rehabilitation: a narrative review. Front. Neurol. 14:1219590. doi: 10.3389/fneur.2023.1219590
Edited by:
Mariella Pazzaglia, Sapienza University of Rome, ItalyReviewed by:
Francesco Motolese, Campus Bio-Medico University, ItalyAbdul Hanif Khan Yusof Khan, Putra Malaysia University, Malaysia
Abdulhameed Tomeh, Universiti Putra Malaysia, Malaysia
Elias Shaaya, Rhode Island Hospital, United States
Copyright © 2023 Wang, Dong, Li, Zhao, Yang, Xu, Fu, Li, Gai and Qin. This is an open-access article distributed under the terms of the Creative Commons Attribution License (CC BY). The use, distribution or reproduction in other forums is permitted, provided the original author(s) and the copyright owner(s) are credited and that the original publication in this journal is cited, in accordance with accepted academic practice. No use, distribution or reproduction is permitted which does not comply with these terms.
*Correspondence: Li Li, a2h5eV9sbEAxNjMuY29t; Xuesong Gai, MTU4MjUyNTUyODhAMTM5LmNvbQ==; Dongdong Qin, cWluZG9uZzEwOEAxNjMuY29t
†These authors have contributed equally to this work