- 1Department of Medicine and Surgery, Unit of Neurology, Neurophysiology, Neurobiology and Psichiatry, Università Campus Bio-Medico di Roma, Rome, Italy
- 2Fondazione Policlinico Universitario Campus Bio-Medico, Rome, Italy
- 3Istituti Clinici Scientifici Maugeri IRCCS, Neurorehabilitation Unit of Milan Institute, Milan, Italy
Ischemic stroke is characterized by a complex cascade of events starting from vessel occlusion. The term “penumbra” denotes the area of severely hypo-perfused brain tissue surrounding the ischemic core that can be potentially recovered if blood flow is reestablished. From the neurophysiological perspective, there are local alterations—reflecting the loss of function of the core and the penumbra—and widespread changes in neural networks functioning, since structural and functional connectivity is disrupted. These dynamic changes are closely related to blood flow in the affected area. However, the pathological process of stroke does not end after the acute phase, but it determines a long-term cascade of events, including changes of cortical excitability, that are quite precocious and might precede clinical evolution. Neurophysiological tools—such as Transcranial Magnetic Stimulation (TMS) or Electroencephalography (EEG)—have enough time resolution to efficiently reflect the pathological changes occurring after stroke. Even if they do not have a role in acute stroke management, EEG and TMS might be helpful for monitoring ischemia evolution—also in the sub-acute and chronic stages. The present review aims to describe the changes occurring in the infarcted area after stroke from the neurophysiological perspective, starting from the acute to the chronic phase.
Introduction
The first studies regarding cerebral perfusion in humans date back to the 1950s. Finnerty and coworkers demonstrated that when cerebral blood flow (CBF) drops below 29 mL/100 g/min, neurological impairment occurs (1). Some years later Jennet et al. showed that hemiparesis consistently occurred when relative cortical CBF was less than 30% compared with the baseline level (2) and subsequently, studies on vessel occlusion in animal models identified a critical threshold—i.e., 18 mL/100 g/min—for irreversible brain tissue damage (3). However, it is now clear that different individual factors contribute to tissue vulnerability after vessel occlusion, such as age, the brain structural reserve, inter-individual characteristics and collateral circulation. Indeed, adequate collateral blood flow limits the size of the infarct core in favor of the ischemic penumbra, i.e., the severely hypo-perfused and hypoxic brain tissue surrounding the core, that can be potentially saved if reperfusion occurs. In recent years there have been substantial advances in acute stroke management, regarding the extended time window for reperfusion therapies (4). The rationale is to select patients with large ischemic penumbra and small infarct using perfusion imaging.
However, perfusion imaging offers a “snapshot” in time of cerebral blood flow and it is not capable of capturing the evolution of stroke. MRI and CT scan provide several short-term prognostic parameters (e.g., hypoperfusion intensity ratio, Tmax, relative cerebral blood volume), reflecting the potential risk of infarction in the absence of reperfusion and, indirectly, the degree of collateral circulation (5, 6). The pathological process of stroke does not end after the acute phase, but it determines a long-term cascade of events, including changes of cortical excitability, that are quite precocious and might precede clinical evolution. These changes are difficult to detect with conventional neuroimaging, while electrophysiological techniques allow to capture the dynamic nature of stroke and thanks to their time resolution might be employed for repetitive evaluations. To this end, Electroencephalogram (EEG) or transcranial-magnetic stimulation (TMS) have been used as monitoring or prognostic tools in different stages of cerebrovascular disease.
EEG signal arises from synchronized synaptic activity in populations of cortical neurons. Studies on animal models have demonstrated that EEG actually reflects the cerebrovascular reactivity after vessel occlusion in the penumbra, while TMS might capture the reorganization of cortical circuits and the changes in functional connectivity due to plasticity mechanisms in later stages. Hence, electrophysiological techniques might be complementary to neuroimaging in the functional and structural evaluation of the brain after stroke.
In this review, we will investigate the potential employment of neurophysiological tools (e.g., EEG, TMS) to evaluate from a functional perspective how the changes in cerebrovascular reactivity influence the evolution of brain damage over time.
The pathological evolution of brain infarction
Cerebral ischemia occurs when blood flow to the brain is insufficient to meet metabolic demands. This might be focal—when a vessel supplying blood to the brain is obstructed—or global, e.g., in case of cardiac arrest. In stroke, the reduction of oxygen and glucose supply to energy-hungry brain cells—mainly neurons, but also glial cells—produces a cascade of time-dependent effects (Figure 1). The maintenance of ionic gradients and membrane potential of neurons consumes a great amount of energy, thus the reduction of CBF affects significantly brain oscillations. Neurons exhibit an individual vulnerability to hypoxia and the signal power of high frequency waves are first to be decreased at the early stage of stroke (8). If blood flow is interrupted for more than 20–60 s, synaptic dysfunction occurs, leading to neural function suppression (9). The absence of oxygen and glucose induces membrane ATPase failure and, consequently, a large Na + and Ca2+ intracellular influx with a massive neuronal depolarization. If oxygen shortage persists, the activation of calcium-dependent enzymes stimulates cell catabolism and induces cell death. In addition, glutamate release activates NMDA and AMPA receptors, exacerbating the intracellular calcium concentration, and producing excitotoxicity (10). Hypoxia damages the blood–brain barrier (BBB) and promotes the early migration of neutrophils (within 30 min) and lymphocytes (within 24 h), inducing inflammation (11, 12).
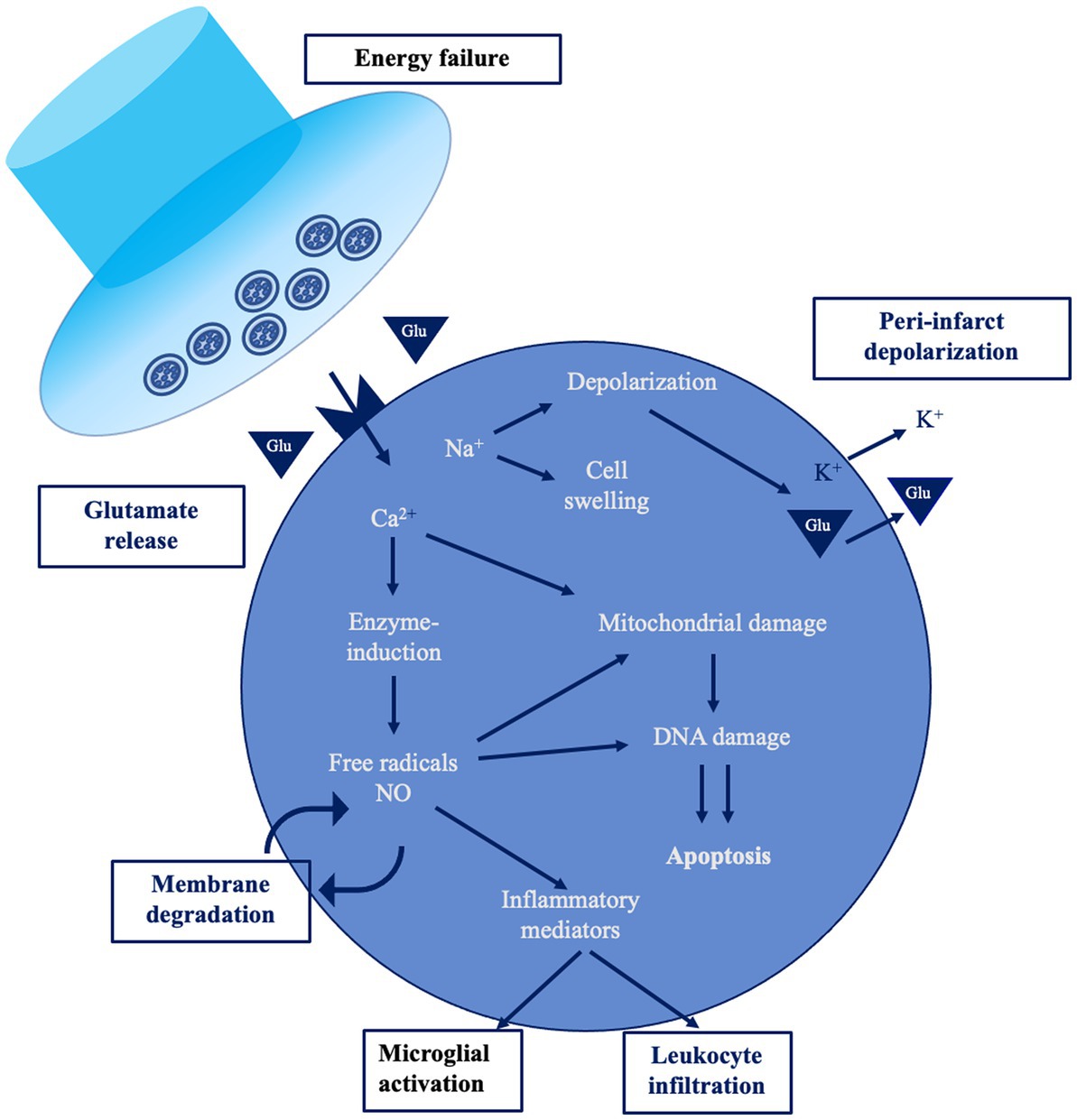
Figure 1. The ischemic cascade [modified from Endres et al. (7)]. This figure shows a simplified overview of the main pathological mechanisms occurring during acute ischemic stroke.
From the neurophysiological standpoint, the ischemic area is electrically silent, while the release of calcium and excitatory neurotransmitters generates a peri-infarct depolarization (PID) propagating across the surrounding area. This is not just a stroke epiphenomenon, but also induces calcium accumulation favoring delayed secondary pathology and neuronal death (13). Neurons in the penumbra are functionally impaired, so that they are “freezed”—i.e., electrically silent due to membrane potential imbalance—but anatomically preserved, as first described by Astrup and colleagues in the early ‘80s (14). Inflammation and apoptosis represent the main mechanism of damage within the ischemic penumbra in case of prolonged blood flow restriction, together with the production of detrimental oxygen-reactive species, ionic imbalance, protease activation, and DNA disruption (15).
In the acute phase, arterial collateral remodeling provides a compensatory supply since the sudden distal drop of pressure due to vessel occlusion generates an increase in flow within collateral circuits, namely “collateral recruitment” (16). If this mechanism is effective, the damage is limited.
In the subacute stage, the role of excitotoxicity and oxidative stress decreases while glial activation and neuroinflammation become more important (15). After 2 weeks, an immature glial scar begins to seal and compartmentalize the area of infarction from the surrounding parenchyma. About 7 weeks after, the process of gliosis culminates into a mature glial scar, defining the chronic phase of ischemic stroke (17).
For the scope of the present review, in agreement with the Stroke Roundtable Consortium, we designated the time after stroke as “acute” within the first 7 days, “subacute” within 6 months and “chronic” after 6 months (18). However, we have to acknowledge that a major limitation of literature regarding neurophysiology and stroke is the lack of a standard definition of stroke timepoints and the great heterogeneity of papers in this regard.
Neurophysiological tools to monitor stroke evolution
Neural oscillations allow short- and long-term communication among neurons in the brain. At cortical level, they can be observed as large-scale oscillations in EEG signal. However, human networks have been studied in-vivo by other tools such as functional magnetic resonance imaging (fMRI) or noninvasive brain stimulation (NIBS) techniques (e.g., TMS) (19).
EEG is a non-invasive tool that has been frequently applied for stroke diagnosis and prognostication (20–22). EEG reflects extracellular currents resulting from excitatory and inhibitory post synaptic currents of cortical pyramidal cells. Quantitative EEG (qEEG) measures have been used as a more standardized approach to predict outcomes in ischemic stroke (23, 24), and qEEG metrics include the frequency spectrum analysis and topographic mapping. Other parameters can be derived from the EEG power spectrum. For instance, ratios of absolute power of different frequency bands, such as ratio of delta/alpha power, have shown to have a significant correlation with the clinical status—thus allowing a better categorization of stroke severity—and are also considered reliable prognostic values.
NIBS techniques explore functional alterations due to stroke on cortex excitability and on plasticity propensity (25). Besides, NIBS might be applied to evaluate connectivity. TMS is a non-invasive and painless technique which, when applied over the primary motor cortex (M1), generates a descending volley in the corticospinal pathway, and elicits a motor evoked potential (MEP) in the target muscles of the contralateral limb (26). Over the last 30 years, TMS has been widely used to study the underlying pathophysiology of various disorders, optimizing single-pulse, paired-pulse and repetitive stimulation protocols (27). TMS has been widely used in the acute phase after stroke to investigate changes in neural circuits and to provide information about cortical excitability, the cortical reorganization phenomena and to predict functional recovery.
A recent evolution of TMS technology allows to record the output of magnetic stimulation directly at the scalp using EEG. TMS-EEG has been used on stroke patients to probe cortical structural integrity and brain connectivity. Indeed, TMS-EEG elicits the so-called TMS evoked potentials (TEPs), characterized by positive and negative waveforms, that are indirect measures of functional integrity of cortical structures (28) (Table 1).
Neurophysiological changes during the acute phase
The first hours after ischemic stroke are of paramount importance in terms of survival and long terms functional prognosis. Given the critical relevance of this phase, a lot of effort has been put to identify the best diagnostic and prognostic tools and to better understand the neuronal changes occurring in acute stroke. From the neurophysiological perspective, after vessel occlusion there are local changes—reflecting the loss of function in the infarcted area—but also widespread changes in neural networks, since structural and functional connectivity is disrupted (29). Changes in neural networks activity accurately reflect the blood flow in the affected area. These changes are highly dynamic and might indicate the improvement or the worsening of perfusion of brain tissue. Prompt restoring of blood flow in viable tissue stops the ischemic pathological cascade, improving local and global functional connectivity (30, 31).
EEG studies
Immediately after vessel occlusion, high-amplitude slow activity—in the delta frequency band (1–3 Hz)—appears in the involved brain regions (22, 32–34) (Figure 2). At intermediate level of ischemia—i.e., penumbra tissue—EEG changes might be less dramatic, including attenuation of beta activity and alpha slowing (21). In a single report of an animal model of ischemia, a significant increase of alpha band power during vessel occlusion has been found, immediately followed by the marked increase of delta power (35). Delta activity is the hallmark of cerebral dysfunction and has been reliably correlated with lesion location on neuroimaging (22). In fact, it is particularly evident on fronto-temporo-central electrodes after middle cerebral artery stroke (36).
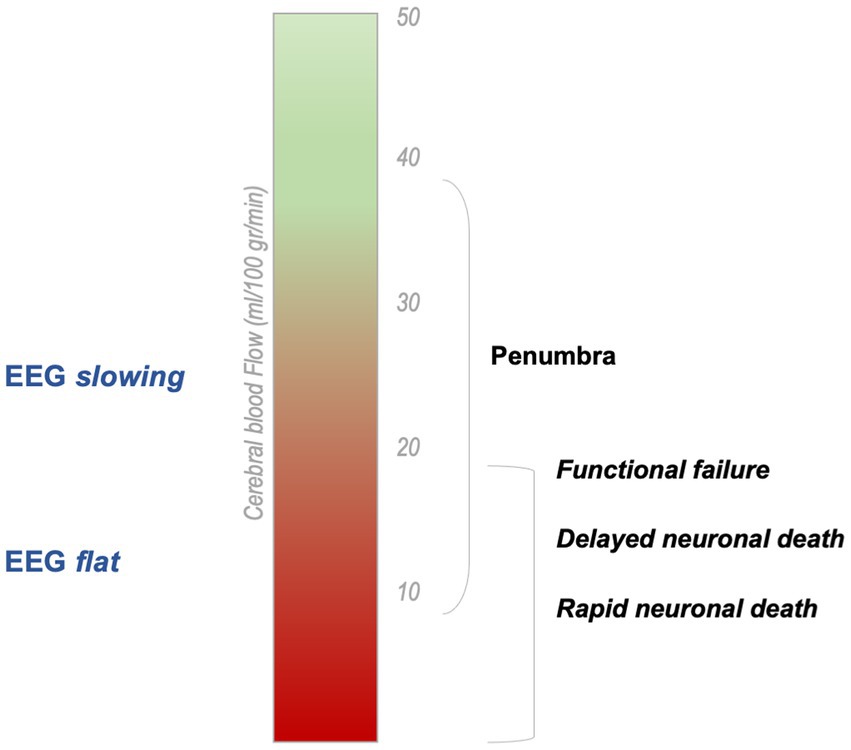
Figure 2. Cerebral blood flow related to Electroencephalographic findings in acute stroke [modified from Hofmeijer and Van Putten (9)]. In acute ischemic stroke, EEG signal changes according to CBF reduction. The infarcted area—where the damage is irreversible—is typically electrically silent on EEG.
Vascular insults produce an imbalance in the frequency activity between hemispheres since in the affected side there is a reduction of higher frequency activity and the increase of low-frequency bands. The brain asymmetry index (BSI) is a biomarker of motor functioning and recovery after stroke. Higher values of BSI—indicating a more pronounced asymmetry—are observed in acute stroke patients with a trend to normalization with spontaneous recovery (37). Most data indicate that the reestablishment of a balanced high frequency activity between motor areas predicts favorable motor recovery (28). However, the role of controlesional hemisphere is still debated and might depend upon stroke type and deficits (38).
A greater delta and theta activity within 24 h from onset, together with decreased faster activity and a greater interhemispheric asymmetry are associated with poor modified Rankin Scale (mRS) at discharge and worse prognosis (39–43). Two recent studies aimed to correlate changes of qEEG measures with long terms prognosis in acute stroke patients that underwent mechanical thrombectomy (44, 45). The results have shown that delta power 24 h after mechanical thrombectomy and the interhemispheric delta-alpha ratio are the best prognostic markers to define prognosis, even when compared with CT perfusion values (44).
Power measures—and especially delta activity—are associated with regional CBF, so that delta is negatively correlated with CBF while alpha is positively correlated (20). These measures might dynamically change, reflecting blood flow status, since reperfusion promptly induces the improvement of qEEG parameters. After blood flow restoration, there is a sudden increase of theta, alpha, and beta waves bandpower (35), while delta activity drops significantly but might persist for a while (46). Reduction of delta activity before symptoms improvement within 20 min after r-tPA administration has also been reported (46, 47). For these reasons, continuous EEG has been suggested as a monitoring tool during thrombolysis and thrombectomy.
qEEG measures include more complex indices such as delta/theta ratio (DTR), the delta/alpha ratio (DAR), and the (delta + theta)/(alpha + beta) ratio (DTABR). In an animal model of middle cerebral artery occlusion, the aperiodic spectral exponents—i.e., a putative marker of disrupted, inefficient neural communication—in peri-infarct area increased transiently and this correlated to a better recovery (48). Indeed, measures that asses the 1/f shape of the EEG spectrum have been proposed as a comprehensive way to assess the excitation/inhibition balance that is inherently expressed in EEG (49). All these parameters seem promising for monitoring stroke evolution, since their changes reflect accurately blood flow status.
Higher values of DTR, DAR and DTABR are observed during ischemia and rapidly decrease after reperfusion (32, 35). Schleiger and colleagues (50) acquired EEG continuously during thrombectomy, observing a significant reduction of DAR within several minutes of middle cerebral artery reperfusion. This change in EEG signal preceded the improvement of clinical symptoms. Authors concluded that DAR can effectively and immediately index salvage of the penumbra and might help clinicians predict the clinical outcomes in combination with the evidence of reperfusion revealed by imaging.
It has been also observed that in animal models of hypoxic brain one of the first feature to develop after vessel occlusion is a rapid reduction of EEG signal amplitude, that persist even after reperfusion occurs, probably due to a “safety mechanism” to reduce neuronal metabolism and protect cells (51). However, in the same study the reduced electrocortical brain activity after blood flow restoration was associated with lower oxygen utilization, thus suggesting the potential long-term development of brain damage (51). In this regard, neuroprotective treatments might be useful to protect tissue from delayed injury mechanism and preserve plasticity propensity in this stage (93).
To conclude, there are multiple evidence that indicate a strong relationship between EEG frequency/amplitude characteristics, CBF, and cerebral metabolism (23) in the acute phase.
TMS studies
During the hyper-acute and acute phase of stroke, TMS over the affected hemisphere often fails to elicit motor evoked potentials (MEP) (52, 53) and the persistent absence of MEP after stroke is considered a marker of poor prognosis (54). In patients with intact cortico-spinal tract and preserved MEP, the motor thresholds are typically higher (53), MEP amplitudes are smaller and cortico-motor conduction time (CMCT) is delayed (55, 56) in comparison with those recorded from the unaffected hemisphere or from healthy individuals. These findings correlate with the long-term functional outcome and predict poor motor recovery (52, 53, 57, 58), although exceptions have been reported (56, 59). Another prognostic factor is cortical silent period (CSP), that is often prolonged after stroke (60–63) and it is correlated to the development of spasticity (64, 65).
In the first hours after stroke, the balance between cortical inhibition and excitation is altered. Cortical excitability of the affected hemisphere is usually reduced in both early and chronic phases of stroke (66). Thus, in most cases, the balance between hemisphere shifts toward excitation, while activity in the local inhibitory circuits is reduced. Short-interval intracortical inhibition (SICI) (59, 67) and long-interval intracortical inhibition (LICI) (68)—markers of GABAA and GABAB activity, respectively—of the affected hemisphere are reduced in the acute phase, whereas intracortical facilitation (ICF)—indicating Glutamatergic activity—remains normal (67, 68). Also, Short-Latency Afferent Inhibition (SAI), a paired pulse TMS protocol marker of central cholinergic activity, is suppressed in the acute phase of stroke and persistent suppression might indicate a better prognosis (69). These findings suggest that there is—in most cases—a “disinhibition” of the affected hemisphere, probably to favor plasticity and functional recovery.
Data regarding controlesional hemisphere are more controversial, since most evidence confirm that cortical excitability of the unaffected hemisphere does not change during stroke (66), and only SICI has been found to be suppressed (59, 67). Data also suggest that normalization of SICI in the unaffected hemisphere seems to be associated with good recovery (59, 68). Moreover, evidence regarding hemispheric imbalance are debated, since unaffected hemisphere might contribute to stroke recovery—i.e., vicariation model of functional recovery—or might have a detrimental effect on lesioned hemisphere—i.e., competition model. In this regard, another factor should be considered, the so-called structural reserve, a term indicating the non-lesioned residual neural networks, which is a critical element in determining if the effect of the contralateral hemisphere is beneficial or detrimental (38).
TMS-EEG is a more reliable measure of cortical reactivity compared with conventional TMS, since it is not influenced by distal components of the nervous system. Indeed, in patients with brainstem injuries MEPs could not be elicitable while TEPs are usually present (28). There are few interesting reports on the modifications of TEPs in acute stroke patients, because this technique requires long and careful recording sessions. Available data suggests that TEPs from lesioned hemisphere are less complex, more local and with a longer latency (70), and the absence of N100 or higher amplitude and delayed P30 are associated with poor functional recovery (59, 71). In a case report by Russo et al., the sleep-like slow-wave TMS-EEG response of acute stroke seems to partially recover with longitudinal follow up, along with clinical improvement (72).
Takin together, this evidence shows that local changes in connectivity and intracortical disinhibition of the affected hemisphere might be helpful for the recruitment of remaining motor output in the acute stage, and subsequently favor synaptic plasticity to promote recovery.
Neurophysiological changes during the subacute phase
During the subacute phase of stroke, the ischemic lesion becomes better defined, while in the brain tissue surrounding ischemic area, hypoperfusion might variably persist (94). During acute and subacute phase of stroke, the neuroradiological findings might not correlate with the clinical impairment, because of the dynamic changes of the hypoperfused area and the extent of the surrounding edema. As time passes, other mechanisms, such as neuroplasticity or the brain structural reserve, might influence the clinical presentation. Indeed, since neurons are organized in networks, clinical manifestations do not depend just on the loss of function of the lesioned area, but on the global impairment of medium and large-scale circuits. Focal brain lesions may functionally impair remote regions, a phenomenon known as “diaschisis,” in which the excitability and metabolism of the remote regions, including the hemisphere contralateral to the stroke side, are reduced (95, 96).
Finally, neuroinflammatory mechanisms and vasogenic edema due to tight junction disruption might influence lesion consolidation and thus neurological impairment.
The quantification of EEG and TMS changes in this stage is of critical importance for defining the extent of brain damage after stroke.
EEG studies
EEG and qEEG measures might be employed in this stage as prognostic tools. Slower frequency might persist on the electrodes overlying the lesioned area even after the acute stage and the magnitude of this activity depends on infarct volume (22). In one study about subacute stage, even after reperfusion, whole EEG power was lower than that of the control group, and also the DTR, DAR, and DTABR indices remained relatively high (35). This probably indicates the persistence of the ischemic stunning of the brain and the disruption of neural networks in stroke later stages.
In subacute middle cerebral artery stroke, the reduction of the asymmetry in high frequency activity between affected and unaffected hemispheres was associated with better motor performances over time (73). The presence of higher BSI value in the subacute phase is a strong indicator of poor prognosis, in particular if delta band power is present in the contralateral hemisphere (74), while more balanced high-frequency activity between hemispheres indicate a better functional prognosis.
Then, the persistence of slow activity and hemispheric asymmetry is a marker of greater damage post-stroke and of poor prognosis.
TMS studies
In the subacute phase of stroke, the recruitment of spared neural networks became progressively more important in compensating the clinical impairment. Indeed, cerebral reorganization is critical for functional recovery.
Even in this phase, the absence of MEP from lesioned hemisphere—either in case of cortical or subcortical location—is related to poor functional prognosis (61). After infarction, the clinical recovery is associated with increased excitability, while the persistence of reduced cortical excitability in the affected hemisphere—as observed by increased resting MT or reduced SICI—is negative correlated with recovery (66). Stinear and Byblow proposed a simple diagnostic algorithm to predict functional recovery at 6 months on the basis of various measures collected during the first 7–10 days after stroke (75). In this algorithm the absence of MEP response, with significant clinical impairment, likely predicts poor functional prognosis.
A single study regarding subacute stroke patients reported the abnormal decreased of SICI of both hemispheres, with abnormal IHI from affected to unaffected hemisphere and preserved IHI the other way around (97). This finding was associated with excellent recovery of motor function and was interpreted as an adaptive process supporting recovery.
Following stroke, there is the expansion of the cortical map—i.e., the number of sites from where a MEP is elicited—indicating a progressive spatial recruitment of perilesional neurons. Indeed, this effect becomes more evident in patients undergoing rehabilitation, where training stimulate plasticity processes (76). Of note, the alteration of cortical excitability and inter-hemispheric communication might persist in cases of suboptimal recovery. Casula and colleagues reported a remarkable interhemispheric imbalance in stroke patients within 6 months from onset as evaluated by TMS-EEG. Also, they found that better recovery of hand strength was associated with a more stable interhemispheric balance (77).
These results indicate that remodeling of neural circuits underlying neuroplasticity and the reestablishment of interhemispheric balance are essential for recovery.
Neurophysiological changes during the chronic phase
The natural evolution of vascular insult in the chronic phase is the formation of a glial scar. In this stage the reorganization of neural networks keeps on promoting functional recovery, even if this effect becomes weaker as time passes. However, this neural circuits remodeling might also have detrimental effects. For instance, the disruption of communication among neural networks might consolidate, constituting a putative mechanism of cognitive deficits after stroke (8).
In chronic stage, perfusion imaging shows the persistence of hypoperfusion in the area surrounding ischemic core (94). A study from Walenski et al. did not find any significant changes over time of tissue perfusion, even in patients that underwent successful rehabilitation (98). Also, hypoperfusion of areas close to the ischemic lesion was shown to correlate with the clinical status of aphasic patients (99). These data suggest that alterations of post-ischemic perfusion tend to persist in perilesional areas and cerebrovascular reactivity does not always seem to sensibly improve over time. Rather, it is the remodeling process that promote recovery.
EEG studies
EEG is an helpful instrument for the longitudinal observation of stroke (28). The alterations in the slow band, usually found in the EEG of stroke patients, were consistently shown to improve from the sub-acute to the chronic phase in patients with good recovery (22, 78). Also changes in the 1/f properties of the EEG spectrum are sensitive to stroke’s evolution from sub-acute to chronic (79). These findings were also associated with various degrees of clinical correlation (79, 80).
As already said, patients with higher interhemispheric imbalance in the acute stage have usually a worse prognosis. The persistence of higher values of BSI in the subacute and chronic stage is still a biomarker of poor functional recovery, especially for what concerns the motor system (81, 82).
Finally, EEG connectivity appears to be locally impaired in chronic stroke, with significant modifications in connectivity from the subacute to the chronic stage (83). In the chronic phase, the reduction of the beta band (12.5–30.0 Hz) oscillatory activity in the motor cortex is an index of motor impairment (84).
TMS studies
Studies regarding cortical excitability in chronic stroke confirm the findings discussed for acute and subacute stage. In particular, the presence of a motor-evoked potential within the first 2 weeks after stroke seems to have a strong predictive value on recovery (85) and the presence of MEP was shown to predict the residual potential for functional improvements, even several months after stroke (86). For these reasons, the presence of MEP in chronic stroke patients seems to be a promising marker to tailor the best rehabilitative approach (75). However, the presence of lower limb MEP did not seem to predict more specific features, such as walking speed (87, 88).
Some degree of disinhibition might persist even >6 months after stroke, without being modified by short-term training (89). Once again, this could be beneficial since it favors structural and functional remodeling but it might also indicate a persistent alteration of cortical circuitry.
TMS-EEG can increase the signal-to-noise ratio of EEG using magnetic perturbation. There are interesting reports on the modifications of TEPs in chronic stroke patients (70, 71, 90), despite the long recording sessions required. TMS-EEG modifications in peri-ischemic areas shows a peculiar modification of the evoked potentials, that compared with the contralateral hemisphere, loses fast complex oscillations and takes the shape of a bistable slow wave (91). In this regard, a higher amplitude of the P30 TEP component in chronic stroke patients has been reported (92). Generally speaking, data indicate that higher cortical reactivity and more complex evoked oscillatory activity in the lesioned hemisphere predicts better functional prognosis (28).
Conclusion
Neurophysiological tools might still play a role in the evaluation of stroke, even in the “Imaging is brain” era (100). Ischemic stroke is a dynamic process, in which a cascade of events takes place after vessel occlusion. Neurophysiological tools are of great value for capturing these changes over time thanks to the excellent time resolution, allowing the longitudinal evaluation till the chronic phase. However, the methodological heterogeneity of the literature has limited the diffusion of techniques such as EEG or TMS in daily practice.
Neurophysiology and neuroimaging should be then considered as complementary tools exploring the same event from two different perspectives. This regards also the viability of penumbra brain tissue during stroke and, indirectly, the blood flow status. More studies are warranted for getting a better insight into stroke pathophysiology. This is of critical importance for developing tailored rehabilitation approach.
Author contributions
FM, JL, and FP contributed to the conception. FM, JL, MR, AT, FS, and AC drafted the paper. FC, VL, and FP revised it critically for important intellectual content. All authors contributed to the article and approved the submitted version.
Conflict of interest
The authors declare that the research was conducted in the absence of any commercial or financial relationships that could be construed as a potential conflict of interest.
The reviewer AM declared a past co-authorship with the author FP to the handling editor.
Publisher’s note
All claims expressed in this article are solely those of the authors and do not necessarily represent those of their affiliated organizations, or those of the publisher, the editors and the reviewers. Any product that may be evaluated in this article, or claim that may be made by its manufacturer, is not guaranteed or endorsed by the publisher.
References
1. Finnerty, FA, Witkin, L, and Fazekas, JF. Cerebral hemodynamics during cerebral ischemia induced by acute hypotension. J Clin Invest. (1954) 33:1227–32. doi: 10.1172/JCI102997
2. Jennett, WB, Harper, AM, and Gillespie, FC. Measurement of regional cerebral blood-flow during carotid ligation. Lancet Lond Engl. (1966) 2:1162–3.
3. Jones, TH, Morawetz, RB, Crowell, RM, Marcoux, FW, FitzGibbon, SJ, DeGirolami, U, et al. Thresholds of focal cerebral ischemia in awake monkeys. J Neurosurg. (1981) 54:773–82. doi: 10.3171/jns.1981.54.6.0773
5. Nagakane, Y, Christensen, S, Ogata, T, Churilov, L, Ma, H, Parsons, MW, et al. Moving beyond a single perfusion threshold to define penumbra. Stroke. (2012) 43:1548–55. doi: 10.1161/STROKEAHA.111.643932
6. Arenillas, JF, Cortijo, E, García-Bermejo, P, Levy, EI, Jahan, R, Liebeskind, D, et al. Relative cerebral blood volume is associated with collateral status and infarct growth in stroke patients in SWIFT PRIME. J Cereb Blood Flow Metab. (2018) 38:1839–47. doi: 10.1177/0271678X17740293
7. Endres, M, Dirnagl, U, and Moskowitz, MA. The ischemic cascade and mediators of ischemic injury. Handb Clin Neurol. (2009) 92:31–41. doi: 10.1016/S0072-9752(08)01902-7
8. Sato, Y, Schmitt, O, Ip, Z, Rabiller, G, Omodaka, S, Tominaga, T, et al. Pathological changes of brain oscillations following ischemic stroke. J Cereb Blood Flow Metab. (2022) 42:1753–76. doi: 10.1177/0271678X221105677
9. Hofmeijer, J, and van Putten, MJAM. Ischemic cerebral damage: an appraisal of synaptic failure. Stroke. (2012) 43:607–15. doi: 10.1161/STROKEAHA.111.632943
10. Chamorro, Á, Dirnagl, U, Urra, X, and Planas, AM. Neuroprotection in acute stroke: targeting excitotoxicity, oxidative and nitrosative stress, and inflammation. Lancet Neurol. (2016) 15:869–81. doi: 10.1016/S1474-4422(16)00114-9
11. Bernardo-Castro, S, Sousa, JA, Brás, A, Cecília, C, Rodrigues, B, Almendra, L, et al. Pathophysiology of blood–brain barrier permeability throughout the different stages of ischemic stroke and its implication on Hemorrhagic transformation and recovery. Front Neurol. (2020) 11:594672. doi: 10.3389/fneur.2020.594672
12. Huang, J, Upadhyay, UM, and Tamargo, RJ. Inflammation in stroke and focal cerebral ischemia. Surg Neurol. (2006) 66:232–45. doi: 10.1016/j.surneu.2005.12.028
13. Fabricius, M, Fuhr, S, Bhatia, R, Boutelle, M, Hashemi, P, Strong, AJ, et al. Cortical spreading depression and peri-infarct depolarization in acutely injured human cerebral cortex. Brain J Neurol. (2006) 129:778–90. doi: 10.1093/brain/awh716
14. Astrup, J, Siesjö, BK, and Symon, L. Thresholds in cerebral ischemia—the ischemic penumbra. Stroke. (1981) 12:723–5. doi: 10.1161/01.STR.12.6.723
15. Doyle, KP, Simon, RP, and Stenzel-Poore, MP. Mechanisms of ischemic brain damage. Neuropharmacology. (2008) 55:310–8. doi: 10.1016/j.neuropharm.2008.01.005
16. Malhotra, K, and Liebeskind, DS. Collaterals in ischemic stroke. Brain Hemorrhages. (2020) 1:6–12. doi: 10.1016/j.hest.2019.12.003
17. Doyle, KP. Unraveling the pathophysiology of chronic stroke lesions could yield treatments for stroke-related dementia. Future Neurol. (2016) 11:1–4. doi: 10.2217/fnl.15.47
18. Bernhardt, J, Hayward, KS, Kwakkel, G, Ward, NS, Wolf, SL, Borschmann, K, et al. Agreed definitions and a shared vision for new standards in stroke recovery research: the stroke recovery and rehabilitation roundtable taskforce. Neurorehabil Neural Repair. (2017) 31:793–9. doi: 10.1177/1545968317732668
19. Hallett, M, de Haan, W, Deco, G, Dengler, R, Di Iorio, R, Gallea, C, et al. Human brain connectivity: clinical applications for clinical neurophysiology. Clin Neurophysiol. (2020) 131:1621–51. doi: 10.1016/j.clinph.2020.03.031
20. Tolonen, U, and Sulg, IA. Comparison of quantitative EEG parameters from four different analysis techniques in evaluation of relationships between EEG and CBF in brain infarction. Electroencephalogr Clin Neurophysiol. (1981) 51:177–85. doi: 10.1016/0013-4694(81)90007-9
21. Jordan, KG. Emergency EEG and continuous EEG monitoring in acute ischemic stroke. J Clin Neurophysiol. (2004) 21:341–52.
22. Finnigan, S, and van Putten, MJAM. EEG in ischaemic stroke: quantitative EEG can uniquely inform (sub-)acute prognoses and clinical management. Clin Neurophysiol. (2013) 124:10–9. doi: 10.1016/j.clinph.2012.07.003
23. Finnigan, SP, Rose, SE, Walsh, M, Griffin, M, Janke, AL, McMahon, KL, et al. Correlation of quantitative EEG in acute ischemic stroke with 30-day NIHSS score. Stroke. (2004) 35:899–903. doi: 10.1161/01.STR.0000122622.73916.d2
24. Sheorajpanday, RVA, Nagels, G, Weeren, AJTM, and De Deyn, PP. Quantitative EEG in ischemic stroke: correlation with infarct volume and functional status in posterior circulation and lacunar syndromes. Clin Neurophysiol. (2011) 122:884–90. doi: 10.1016/j.clinph.2010.08.020
25. Motolese, F, Capone, F, and Di Lazzaro, V. New tools for shaping plasticity to enhance recovery after stroke. In: Ghilardi B Quartarone, Handbook of clinical neurology, 184 (3rd series) Neuroplasticity: From Bench to Bedside. Elsevier B.V, (2022).
26. Barker, AT, Jalinous, R, and Freeston, IL. Non-invasive magnetic stimulation of human motor cortex. Lancet Lond Engl. (1985) 325:1106–7. doi: 10.1016/S0140-6736(85)92413-4
27. Burke, MJ, Fried, PJ, and Pascual-Leone, A. Transcranial magnetic stimulation: neurophysiological and clinical applications. Handb Clin Neurol. (2019) 163:73–92. doi: 10.1016/B978-0-12-804281-6.00005-7
28. Keser, Z, Buchl, SC, Seven, NA, Markota, M, Clark, HM, Jones, DT, et al. Electroencephalogram (EEG) with or without transcranial magnetic stimulation (TMS) as biomarkers for Post-stroke recovery: a narrative review. Front Neurol. (2022) 13:304. doi: 10.3389/fneur.2022.827866
29. Schlemm, E, Ingwersen, T, Königsberg, A, Boutitie, F, Ebinger, M, Endres, M, et al. Preserved structural connectivity mediates the clinical effect of thrombolysis in patients with anterior-circulation stroke. Nat Commun. (2021) 12:2590. doi: 10.1038/s41467-021-22786-w
30. Wang, Z, Luo, W, Zhou, F, Li, P, and Luo, Q. Dynamic change of collateral flow varying with distribution of regional blood flow in acute ischemic rat cortex. J Biomed Opt. (2012) 17:125001. doi: 10.1117/1.JBO.17.12.125001
31. Uniken Venema, SM, Dankbaar, JW, van der Lugt, A, Dippel, DWJ, and van der Worp, HB. Cerebral collateral circulation in the era of reperfusion therapies for acute ischemic stroke. Stroke. (2022) 53:3222–34. doi: 10.1161/STROKEAHA.121.037869
32. Finnigan, S, Wong, A, and Read, S. Defining abnormal slow EEG activity in acute ischaemic stroke: Delta/alpha ratio as an optimal QEEG index. Clin Neurophysiol. (2016) 127:1452–9. doi: 10.1016/j.clinph.2015.07.014
33. Shreve, L, Kaur, A, Vo, C, Wu, J, Cassidy, JM, Nguyen, A, et al. Electroencephalography measures are useful for identifying large acute ischemic stroke in the emergency department. J Stroke Cerebrovasc Dis. (2019) 28:2280–6. doi: 10.1016/j.jstrokecerebrovasdis.2019.05.019
34. Cohen, BA, Bravo-Fernandez, EJ, and Sances, A. Automated electroencephalographic analysis as a prognostic indicator in stroke. Med Biol Eng Comput. (1977) 15:431–7. doi: 10.1007/BF02457998
35. Ferreira, LO, Mattos, BG, Jóia de Mello, V, Martins-Filho, AJ, da Costa, ET, Yamada, ES, et al. Increased Relative Delta Bandpower and Delta indices revealed by continuous qEEG monitoring in a rat model of ischemia-reperfusion. Front Neurol. (2021) 12:645138. doi: 10.3389/fneur.2021.645138
36. Phan, TG, Gureyev, T, Nesterets, Y, Ma, H, and Thyagarajan, D. Novel application of EEG source localization in the assessment of the penumbra. Cerebrovasc Dis. (2012) 33:405–7. doi: 10.1159/000336332
37. Agius Anastasi, A, Falzon, O, Camilleri, K, Vella, M, and Muscat, R. Brain symmetry index in healthy and stroke patients for assessment and prognosis. Stroke Res Treat. (2017) 2017. doi: 10.1155/2017/8276136
38. Di Pino, G, Pellegrino, G, Assenza, G, Capone, F, Ferreri, F, Formica, D, et al. Modulation of brain plasticity in stroke: a novel model for neurorehabilitation. Nat Rev Neurol. (2014) 10:597–608. doi: 10.1038/nrneurol.2014.162
39. Cuspineda, E, Machado, C, Aubert, E, Galán, L, Llopis, F, and Avila, Y. Predicting outcome in acute stroke: a comparison between QEEG and the Canadian neurological scale. Clin EEG Electroencephalogr. (2003) 34:1–4. doi: 10.1177/155005940303400104
40. Bentes, C, Peralta, AR, Martins, H, Casimiro, C, Morgado, C, Franco, AC, et al. Seizures, electroencephalographic abnormalities, and outcome of ischemic stroke patients. Epilepsia Open. (2017) 2:441–52. doi: 10.1002/epi4.12075
41. Jiang, M, Su, Y, Liu, G, Chen, W, and Gao, D. Predicting the non-survival outcome of large hemispheric infarction patients via quantitative electroencephalography: superiority to visual electroencephalography and the Glasgow coma scale. Neurosci Lett. (2019) 706:88–92. doi: 10.1016/j.neulet.2019.05.007
42. Cuspineda, E, Machado, C, Galán, L, Aubert, E, Alvarez, MA, Llopis, F, et al. QEEG prognostic value in acute stroke. Clin EEG Neurosci. (2007) 38:155–60. doi: 10.1177/155005940703800312
43. Aminov, A, Rogers, JM, Johnstone, SJ, Middleton, S, and Wilson, PH. Acute single channel EEG predictors of cognitive function after stroke. PLoS One. (2017) 12:e0185841. doi: 10.1371/journal.pone.0185841
44. Zhang, N, Chen, F, Xie, X, Xie, Z, Hong, D, Li, J, et al. Application of quantitative EEG in acute ischemic stroke patients who underwent thrombectomy: a comparison with CT perfusion. Clin Neurophysiol. (2022) 141:24–33. doi: 10.1016/j.clinph.2022.06.007
45. Dickey, AS, Mitsias, PD, Olango, WM, Agan, MC, Roche, WP, Thomas, JR, et al. The prognostic value of quantitative EEG in patients undergoing mechanical thrombectomy for acute ischemic stroke. J Clin Neurophysiol. (2022) 39:276–82. doi: 10.1097/WNP.0000000000000769
46. Finnigan, SP, Rose, SE, and Chalk, JB. Rapid EEG changes indicate reperfusion after tissue plasminogen activator injection in acute ischaemic stroke. Clin Neurophysiol. (2006) 117:2338–9. doi: 10.1016/j.clinph.2006.06.718
47. Ajčević, M, Furlanis, G, Naccarato, M, Miladinović, A, Buoite Stella, A, Caruso, P, et al. Hyper-acute EEG alterations predict functional and morphological outcomes in thrombolysis-treated ischemic stroke: a wireless EEG study. Med Biol Eng Comput. (2021) 59:121–9. doi: 10.1007/s11517-020-02280-z
48. Biskamp, J, Isla Cainzos, S, Higgen, FL, Gerloff, C, and Magnus, T. Normalization of aperiodic electrocorticography components indicates fine motor recovery after sensory cortical stroke in mice. Stroke. (2022) 53:2945–53. doi: 10.1161/STROKEAHA.122.039335
49. Johnston, PR, McIntosh, AR, and Meltzer, JA. Spectral slowing in chronic stroke reflects abnormalities in both periodic and aperiodic neural dynamics. Rochester, NY; (2022). Available at: https://papers.ssrn.com/abstract=4224354 (Accessed 26 February 2023).
50. Schleiger, E, Wong, A, Read, S, Coulthard, A, and Finnigan, S. Improved cerebral pathophysiology immediately following thrombectomy in acute ischaemic stroke: monitoring via quantitative EEG. Clin Neurophysiol. (2016) 127:2832–3. doi: 10.1016/j.clinph.2016.06.001
51. Ioroi, T, Peeters-Scholte, C, Post, I, Leusink, C, Groenendaal, F, and van Bel, F. Changes in cerebral haemodynamics, regional oxygen saturation and amplitude-integrated continuous EEG during hypoxia-ischaemia and reperfusion in newborn piglets. Exp Brain Res. (2002) 144:172–7. doi: 10.1007/s00221-002-1030-z
52. Pennisi, G, Rapisarda, G, Bella, R, Calabrese, V, Maertens de Noordhout, A, and Delwaide, PJ. Absence of response to early transcranial magnetic stimulation in ischemic stroke patients. Stroke. (1999) 30:2666–70. doi: 10.1161/01.STR.30.12.2666
53. Rapisarda, G, Bastings, E, de Noordhout, AM, Pennisi, G, and Delwaide, PJ. Can motor recovery in stroke patients be predicted by early transcranial magnetic stimulation? Stroke. (1996) 27:2191–6. doi: 10.1161/01.STR.27.12.2191
54. Smith, MC, and Stinear, CM. Transcranial magnetic stimulation (TMS) in stroke: ready for clinical practice? J Clin Neurosci. (2016) 31:10–4. doi: 10.1016/j.jocn.2016.01.034
55. Heald, A, Bates, D, Cartlidge, NEF, French, JM, and Miller, S. Longitudinal study of central motor conduction time following stroke: 2. Central motor conduction measured within 72 h after stroke as a predictor of functional outcome at 12 months. Brain. (1993) 116:1371–85. doi: 10.1093/brain/116.6.1371
56. Delvaux, V, Alagona, G, Gérard, P, De Pasqua, V, Pennisi, G, and de Noordhout, AM. Post-stroke reorganization of hand motor area: a 1-year prospective follow-up with focal transcranial magnetic stimulation. Clin Neurophysiol. (2003) 114:1217–25. doi: 10.1016/S1388-2457(03)00070-1
57. Escudero, JV, Sancho, J, Bautista, D, Escudero, M, and López-Trigo, J. Prognostic value of motor evoked potential obtained by transcranial magnetic brain stimulation in motor function recovery in patients with acute ischemic stroke. Stroke. (1998) 29:1854–9. doi: 10.1161/01.STR.29.9.1854
58. Stinear, CM, Byblow, WD, Ackerley, SJ, Smith, MC, Borges, VM, and Barber, PA. Proportional motor recovery after stroke. Stroke. (2017) 48:795–8. doi: 10.1161/STROKEAHA.116.016020
59. Manganotti, P, Patuzzo, S, Cortese, F, Palermo, A, Smania, N, and Fiaschi, A. Motor disinhibition in affected and unaffected hemisphere in the early period of recovery after stroke. Clin Neurophysiol. (2002) 113:936–43. doi: 10.1016/S1388-2457(02)00062-7
60. Ahonen, JP, Jehkonen, M, Dastidar, P, Molnár, G, and Häkkinen, V. Cortical silent period evoked by transcranial magnetic stimulation in ischemic stroke. Electroencephalogr Clin Neurophysiol Mot Control. (1998) 109:224–9. doi: 10.1016/S0924-980X(98)00014-9
61. Traversa, R, Cicinelli, P, Bassi, A, Rossini, PM, and Bernardi, G. Mapping of motor cortical reorganization after stroke. Stroke. (1997) 28:110–7. doi: 10.1161/01.STR.28.1.110
62. Classen, J, Schnitzler, A, Binkofski, F, Werhahn, KJ, Kim, YS, Kessler, KR, et al. The motor syndrome associated with exaggerated inhibition within the primary motor cortex of patients with hemiparetic. Brain J Neurol. (1997) 120:605–19. doi: 10.1093/brain/120.4.605
63. Cicinelli, P, Pasqualetti, P, Zaccagnini, M, Traversa, R, Oliveri, M, and Rossini, PM. Interhemispheric asymmetries of motor cortex excitability in the Postacute stroke stage. Stroke. (2003) 34:2653–8. doi: 10.1161/01.STR.0000092122.96722.72
64. Catano, A, Houa, M, and Noël, P. Magnetic transcranial stimulation: clinical interest of the silent period in acute and chronic stages of stroke. Electroencephalogr Clin Neurophysiol Mot Control. (1997) 105:290–6. doi: 10.1016/S0924-980X(97)00021-0
65. Oozumi, T, Ito, Y, Tsuji, S, and Murai, Y. Inhibitory period following motor potentials evoked by magnetic cortical stimulation. Electroencephalogr Clin Neurophysiol Potentials Sect. (1992) 85:273–9. doi: 10.1016/0168-5597(92)90116-S
66. McDonnell, MN, and Stinear, CM. TMS measures of motor cortex function after stroke: a meta-analysis. Brain Stimul. (2017) 10:721–34. doi: 10.1016/j.brs.2017.03.008
67. Liepert, J, Storch, P, Fritsch, A, and Weiller, C. Motor cortex disinhibition in acute stroke. Clin Neurophysiol. (2000) 111:671–6. doi: 10.1016/S1388-2457(99)00312-0
68. Swayne, OBC, Rothwell, JC, Ward, NS, and Greenwood, RJ. Stages of motor output reorganization after hemispheric stroke suggested by longitudinal studies of cortical physiology. Cereb Cortex. (2008) 18:1909–22. doi: 10.1093/cercor/bhm218
69. Di Lazzaro, V, Profice, P, Pilato, F, Capone, F, Ranieri, F, Florio, L, et al. The level of cortical afferent inhibition in acute stroke correlates with long-term functional recovery in humans. Stroke. (2012) 43:250–2. doi: 10.1161/STROKEAHA.111.631085
70. Tscherpel, C, Dern, S, Hensel, L, Ziemann, U, Fink, GR, and Grefkes, C. Brain responsivity provides an individual readout for motor recovery after stroke. Brain J Neurol. (2020) 143:1873–88. doi: 10.1093/brain/awaa127
71. Gray, WA, Palmer, JA, Wolf, SL, and Borich, MR. Abnormal EEG responses to TMS during the cortical silent period are associated with hand function in chronic stroke. Neurorehabil Neural Repair. (2017) 31:666–76. doi: 10.1177/1545968317712470
72. Russo, S, D’Ambrosio, S, De Giampaulis, P, Mora, G, Massimini, M, Sarasso, S, et al. Probing cortical structural integrity after focal hemorrhagic stroke: a TMS-EEG case. Clin Neurophysiol. (2022) 140:159–60. doi: 10.1016/j.clinph.2022.06.006
73. Giaquinto, S, Cobianchi, A, Macera, F, and Nolfe, G. EEG recordings in the course of recovery from stroke. Stroke. (1994) 25:2204–9. doi: 10.1161/01.STR.25.11.2204
74. Assenza, G, Zappasodi, F, Pasqualetti, P, Vernieri, F, and Tecchio, F. A contralesional EEG power increase mediated by interhemispheric disconnection provides negative prognosis in acute stroke. Restor Neurol Neurosci. (2013) 31:177–88. doi: 10.3233/RNN-120244
75. Stinear, CM, Barber, PA, Smale, PR, Coxon, JP, Fleming, MK, and Byblow, WD. Functional potential in chronic stroke patients depends on corticospinal tract integrity. Brain. (2007) 130:170–80. doi: 10.1093/brain/awl333
76. Bütefisch, CM, Kleiser, R, and Seitz, RJ. Post-lesional cerebral reorganisation: evidence from functional neuroimaging and transcranial magnetic stimulation. J Physiol-Paris. (2006) 99:437–54. doi: 10.1016/j.jphysparis.2006.03.001
77. Casula, EP, Pellicciari, MC, Bonnì, S, Spanò, B, Ponzo, V, Salsano, I, et al. Evidence for interhemispheric imbalance in stroke patients as revealed by combining transcranial magnetic stimulation and electroencephalography. Hum Brain Mapp. (2021) 42:1343–58. doi: 10.1002/hbm.25297
78. Finnigan, SP, Walsh, M, Rose, SE, and Chalk, JB. Quantitative EEG indices of sub-acute ischaemic stroke correlate with clinical outcomes. Clin Neurophysiol. (2007) 118:2525–32. doi: 10.1016/j.clinph.2007.07.021
79. Lanzone, J, Colombo, MA, Sarasso, S, Zappasodi, F, Rosanova, M, Massimini, M, et al. EEG spectral exponent as a synthetic index for the longitudinal assessment of stroke recovery. Clin Neurophysiol. (2022) 137:92–101. doi: 10.1016/j.clinph.2022.02.022
80. Vatinno, AA, Simpson, A, Ramakrishnan, V, Bonilha, HS, Bonilha, L, and Seo, NJ. The prognostic utility of electroencephalography in stroke recovery: a systematic review and meta-analysis. Neurorehabil Neural Repair. (2022) 36:255–68. doi: 10.1177/15459683221078294
81. Saes, M, Meskers, CGM, Daffertshofer, A, de Munck, JC, Kwakkel, G, van Wegen, EEH, et al. How does upper extremity Fugl-Meyer motor score relate to resting-state EEG in chronic stroke? A power spectral density analysis. Clin Neurophysiol. (2019) 130:856–62. doi: 10.1016/j.clinph.2019.01.007
82. Cassidy, JM, Wodeyar, A, Wu, J, Kaur, K, Masuda, AK, Srinivasan, R, et al. Low-frequency oscillations are a biomarker of injury and recovery after stroke. Stroke. (2020) 51:1442–50. doi: 10.1161/STROKEAHA.120.028932
83. Pirovano, I, Mastropietro, A, Antonacci, Y, Barà, C, Guanziroli, E, Molteni, F, et al. Resting state EEG directed functional connectivity unveils changes in motor network Organization in Subacute Stroke Patients after Rehabilitation. Front Physiol. (2022) 13:591. doi: 10.3389/fphys.2022.862207
84. Graziadio, S, Tomasevic, L, Assenza, G, Tecchio, F, and Eyre, JA. The myth of the «unaffected» side after unilateral stroke: is reorganisation of the non-infarcted corticospinal system to re-establish balance the price for recovery? Exp Neurol. (2012) 238:168–75. doi: 10.1016/j.expneurol.2012.08.031
85. Bembenek, JP, Kurczych, K, Karli Nski, M, and Czlonkowska, A. The prognostic value of motor-evoked potentials in motor recovery and functional outcome after stroke—a systematic review of the literature. Funct Neurol. (2012) 27:79–84.
86. Stinear, CM, Byblow, WD, Ackerley, SJ, Smith, MC, Borges, VM, and Barber, PA. PREP2: a biomarker-based algorithm for predicting upper limb function after stroke. Ann Clin Transl Neurol. (2017) 4:811–20. doi: 10.1002/acn3.488
87. Madhavan, S, Yerrapothu, M, Williams, A, and Gradowska, A. Does the presence of a TMS-evoked MEP predict walking speeds in individuals with chronic stroke? Brain Stimul. (2017) 10:359–60. doi: 10.1016/j.brs.2017.01.058
88. Sivaramakrishnan, A, and Madhavan, S. Absence of a transcranial magnetic stimulation-induced lower limb corticomotor response does not affect walking speed in chronic stroke survivors. Stroke. (2018) 49:2004–7. doi: 10.1161/STROKEAHA.118.021718
89. Blicher, JU, Jakobsen, J, Andersen, G, and Nielsen, JF. Cortical excitability in chronic stroke and modulation by training: a TMS study. Neurorehabil Neural Repair. (2009) 23:486–93. doi: 10.1177/1545968308328730
90. Rolle, CE, Baumer, FM, Jordan, JT, Berry, K, Garcia, M, Monusko, K, et al. Mapping causal circuit dynamics in stroke using simultaneous electroencephalography and transcranial magnetic stimulation. BMC Neurol. (2021) 21:1–11. doi: 10.1186/s12883-021-02319-0
91. Sarasso, S, D’Ambrosio, S, Fecchio, M, Casarotto, S, Viganò, A, Landi, C, et al. Local sleep-like cortical reactivity in the awake brain after focal injury. Brain. (2020) 143:3672–84. doi: 10.1093/brain/awaa338
92. Hordacre, B, Ghosh, R, and Goldsworthy, MR. Ridding MC. Transcranial magnetic stimulation-EEG biomarkers of poststroke upper-limb motor function. J Stroke Cerebrovasc Dis. (2019) 28. doi: 10.1016/j.jstrokecerebrovasdis.2019.104452
93. Capone, F, Pellegrino, G, Motolese, F, Rossi, M, Musumeci, G, and Di Lazzaro, V. Extremely low frequency magnetic fields do not affect LTP-like plasticity in healthy humans. Front Hum Neurosci. (2020) 14:00014. doi: 10.3389/fnhum.2020.00014
94. Richardson, JD, Baker, JM, Morgan, PS, Rorden, C, Bonilha, L, and Fridriksson, J. Cerebral perfusion in chronic stroke: implications for lesion-symptom mapping and functional MRI. Behav Neurol. (2011) 24:117–22. doi: 10.1155/2011/380810
95. Carrera, E, and Tononi, G. Diaschisis: past, present, future. Brain J Neurol. (2014) 137:2408–22. doi: 10.1093/brain/awu101
96. Motolese, F, Pezzella, FR, Marano, M, Di Lazzaro, V, and Anticoli, S. Post-stroke hyperkinetic movement disorders: a brain network issue. Neurol Sci. (2021) 42. doi: 10.1007/s10072-020-04832-5
97. Bütefisch, CM, Weβling, M, Netz, J, Seitz, RJ, and Hömberg, V. Relationship between interhemispheric inhibition and motor cortex excitability in subacute stroke patients. Neurorehabil Neural Repair. (2008) 22:4–21. doi: 10.1177/1545968307301769
98. Walenski, M, Chen, Y, Litcofsky, KA, Caplan, D, Kiran, S, Rapp, B, et al. Perilesional perfusion in chronic stroke-induced aphasia and its response to Behavioral treatment interventions. Neurobiol Lang. (2022) 3:345–63. doi: 10.1162/nol_a_00068
99. Thompson, CK, Walenski, M, Chen, Y, Caplan, D, Kiran, S, Rapp, B, et al. Intrahemispheric perfusion in chronic stroke-induced aphasia. Neural Plast. (2017) 2017. doi: 10.1155/2017/2361691
Keywords: neurophysiology, TMS, EEG, collateral circulation, stroke, penumbra
Citation: Motolese F, Lanzone J, Todisco A, Rossi M, Santoro F, Cruciani A, Capone F, Di Lazzaro V and Pilato F (2023) The role of neurophysiological tools in the evaluation of ischemic stroke evolution: a narrative review. Front. Neurol. 14:1178408. doi: 10.3389/fneur.2023.1178408
Edited by:
Jean-Claude Baron, University of Cambridge, United KingdomReviewed by:
Luca Scarcia, Hôpital Pitié-Salpêtrière, FranceAntonio Marrazzo, Ospedale Santissima Annunziata, Italy
Copyright © 2023 Motolese, Lanzone, Todisco, Rossi, Santoro, Cruciani, Capone, Di Lazzaro and Pilato. This is an open-access article distributed under the terms of the Creative Commons Attribution License (CC BY). The use, distribution or reproduction in other forums is permitted, provided the original author(s) and the copyright owner(s) are credited and that the original publication in this journal is cited, in accordance with accepted academic practice. No use, distribution or reproduction is permitted which does not comply with these terms.
*Correspondence: Francesco Motolese, Zi5tb3RvbGVzZUBwb2xpY2xpbmljb2NhbXB1cy5pdA==