- 1Clinical and Medical Affairs, LivaNova PLC (or a subsidiary), London, United Kingdom
- 2Department of Neurology, University of Alabama at Birmingham Heersink School of Medicine, Birmingham, AL, United States
- 3Department of Neurology, 4Brain, Ghent University Hospital, Ghent, Belgium
Vagus nerve stimulation (VNS) was the first device-based therapy for epilepsy, having launched in 1994 in Europe and 1997 in the United States. Since then, significant advances in the understanding of the mechanism of action of VNS and the central neurocircuitry that VNS modulates have impacted how the therapy is practically implemented. However, there has been little change to VNS stimulation parameters since the late 1990s. Short bursts of high frequency stimulation have been of increasing interest to other neuromodulation targets e.g., the spine, and these high frequency bursts elicit unique effects in the central nervous system, especially when applied to the vagus nerve. In the current study, we describe a protocol design that is aimed to assess the impact of high frequency bursts of stimulation, called “Microburst VNS”, in subjects with refractory focal and generalized epilepsies treated with this novel stimulation pattern in addition to standard anti-seizure medications. This protocol also employed an investigational, fMRI-guided titration protocol that permits personalized dosing of Microburst VNS among the treated population depending on the thalamic blood-oxygen-level-dependent signal. The study was registered on clinicaltrials.gov (NCT03446664). The first subject was enrolled in 2018 and the final results are expected in 2023.
1. Introduction
Device-based therapies for epilepsy aim to leverage intrinsic circuits to either interrupt or suppress epileptic activity. Two invasive, cranial procedures exist that are currently approved as adjunctive therapies to lessen the frequency of seizures in patients in whom multiple trials of anti-seizure medications (ASMs) have failed: responsive neurostimulation (RNS) and deep-brain stimulation (DBS). While DBS is an open loop device approved for the treatment of focal onset epilepsy with anterior nucleus of the thalamus as the therapy target (1), some researchers have implanted the stimulation electrodes in other thalamic nuclei e.g., centro-median nucleus (2). RNS, a closed-loop system, is currently FDA-approved for the treatment of focal onset epilepsy (3). However, similar to DBS, this system has also been implanted in patients with generalized epilepsies, including idiopathic generalized epilepsies (4) and Lennox-Gastaut Syndrome (LGS) (5), with specific trials for both indications registered with clinicaltrials.gov. Both approaches directly target brain structures with electrical energy. These approaches have been demonstrated to reduce seizure frequency by over 50% in more than 40% of patients in the first year of therapy with additional improvements observed over time. However, they carry the risk of rare but potentially severe adverse events due to the invasiveness of the implantation procedure (1, 3, 6, 7). VNS is considered a less invasive, peripheral approach to change epileptic networks, and it has been previously demonstrated to modulate epilepsy-associated brain structures (8).
The first VNS Therapy™ System received approval for the adjunctive treatment of medically refractory epilepsy in 1994 in Europe and in 1997 in the United States and consists of an implantable pulse generator (IPG) that supplies intermittent electrical stimulation to the left vagus nerve. The specific mechanism of action by which the VNS Therapy reduces seizure frequency is not precisely understood, because the physiological effects of VNS are documented as multifaceted (8). Modulation of vagus nerve firing rates has been shown to subsequently modulate central nervous system activity with this central modulation being required for the anti-seizure effect of VNS in epilepsy (9, 10).
In the early 2000s, an experimental VNS stimulation paradigm was developed that consists of high-frequency bursts of stimulation, herein called “Microburst VNS” (μVNS) (Figure 1). While the mechanism of traditional VNS was believed to be mediated by the nuclei closer to the brainstem, such as the nucleus of the tractus solitarius (NTS) and the nucleus of the locus coeruleus (LC), existing evidence suggests that μVNS can be employed to modulate other brain areas, including the thalamus (11, 12).
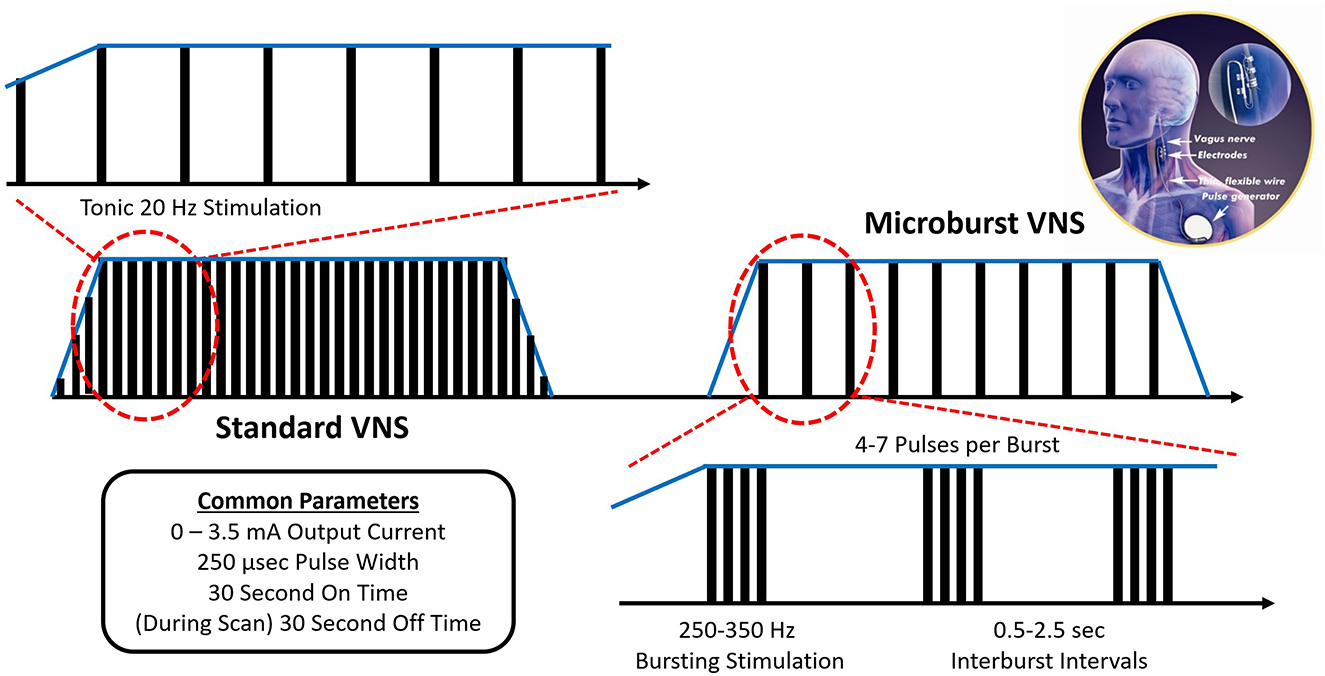
Figure 1. Microburst VNS consists of short bursts of pulses separated by brief off-times called interburst intervals (IBIs). The μVNS waveform incorporates 7 stimulation parameters with a range of available settings (Table 3). On compatible pulse generators, μVNS can be selected as a stimulation setting for the normal mode, the magnet mode, or the autostim mode, which can be set to different levels to deliver VNS (traditional or μVNS) at a regular cadence or based on a specific triggering event. In its current embodiment, μVNS can be delivered from standard VNS Therapy leads and implantable pulse generators with form factors similar to existing VNS devices.
High frequency burst VNS, eventually labeled “Microburst” VNS, was first examined in primates in the early 2000s (11, 12). This stimulation protocol is similar to the one implemented in transcranial magnetic stimulation called intermittent theta burst stimulation (iTBS) that is known to affect long-term potentiation and induce cortical plasticity (13, 14). In the original experiments, standard VNS and high frequency bursts of VNS were used to evoke responses in the parafascicular nucleus of the thalamus, measured by simultaneous electrophysiological recordings. Only paired pulses of 1.5 mA at 400 μs (~5x the threshold charge density), delivered at 300 Hz, elicited a vagal evoked potential in the parafascicular nucleus that had not been previously detected in the mapping studies of vagal evoked potentials (11). Ito and Craig advocated that this effect could be due to paired pulse excitation or inhibition mechanics in the ascending vagus nerve circuits. Following this discovery, the experiment was replicated with multiunit discharges recorded in the parafascicular nucleus and the basal ventromedial nucleus (12). A series of studies in beagles and rats followed the initial primate work and investigated the impact of μVNS on imaging and biochemical markers in experimental epilepsy models (15–17). In beagles, standard VNS parameters were not associated with cerebral blood flow alterations, while μVNS caused significant hypoperfusion of the left frontal lobe and the right parietal lobe. Moreover, both standard VNS and μVNS were associated with a significant increase of norepinephrine release, suggesting evoked activation of the coeruleo-fugal pathways (15, 16). In rodents, both standard and μVNS increased the electrographic seizure threshold of pentylenetetrazole-kindled seizures, but decreased stimulus intensity may have contributed to microbursts not reaching a level of statistical significance (17). Most recently, and concurrently with the clinical feasibility study described herein, μVNS has returned to primate study in a naturally occurring model of genetic generalized epilepsy in baboons. In these animals, μVNS reduced the frequency of generalized tonic-clonic seizures except when the baboons received output currents of 0.25 mA for extended periods, suggesting a dose-response relationship (18). Baboons tolerated μVNS well, and this approach was not associated with cardiac or behavioral changes. However, transient regular muscle contractions could be detected during VNS on-times consistent with the 0.5-s interburst intervals that were not noted during wakefulness (18).
Vagus nerve stimulation is an MRI-conditional product, meaning that the collection of MRI images in patients with VNS therapy is safe provided certain use limitations are followed. One such restriction is the deactivation of the VNS device prior to introducing an implanted patient into an area of a strong magnetic field. The primary rationale for this particular restriction is the activation of a magnetically-sensitive component in the pulse generator, which could respond to the MRI's magnetic fields in a variety of ways.
However, investigators have demonstrated that it is possible to record the Blood Oxygen Level Dependent (BOLD) response of different brain regions to VNS, with the device being active during scanning. After the first demonstration of an MRI-compatible positioning of the device that avoids deactivation while the patient lies supine within the scanner (19) (only suitable for devices without “Magnet Mode”), an investigative team examined VNS-evoked BOLD responses in subjects receiving investigational VNS as therapy for treatment-resistant depression. In initial feasibility work, the team demonstrated that the phase lag from the onset of stimulation to the onset of the hemodynamic response was variable for each affected brain region but tended to be ~ 4–7 s with a similarly variable washout time of 15–25 s (20). The VNS-evoked BOLD response was dose dependent, with lower VNS charge densities resulting in significantly weaker BOLD responses (21, 22). BOLD response was diffuse and not always consistent between patients, but the most common areas of BOLD response were the thalamus, amygdala and insular cortex (20–23).
Following the preclinical history of microburst VNS investigations, and with some understanding of its mechanism of action and how that mechanism can be objectively studied, we designed a prospective, open-label, multicenter phase I clinical trial to investigate the potential risk-benefit profile of μVNS in humans. The study, registered as NCT03446664, examined over 12 months two cohorts of treatment-resistant epilepsy patients with focal-onset (including those with progression to bilateral tonic-clonic seizures) or primary (idiopathic/genetic) generalized-onset tonic-clonic seizures (PGTC). In addition to traditional outcome measures of epilepsy studies, an investigational fMRI protocol was executed in all subjects to offer personalized titration and measure the impact of μVNS on the thalamus.
2. Methods and analysis
This prospective, non-randomized, interventional, open-label phase I clinical trial was designed to collect data on up to 40 subjects (20 PGTC and 20 focal onset) implanted with an investigational μVNS delivering therapy over 12 months of follow-up (Figure 2). The study was registered on clinicaltrials.gov (NCT03446664) and approved by the Institutional Review Boards and Ethics Committees of all study sites. All research procedures were conducted in accordance with the ethical principles of informed consent and the Declaration of Helsinki. All participants received care in academic hospitals from epileptologists trained in the use of the VNS Therapy System (Table 1).
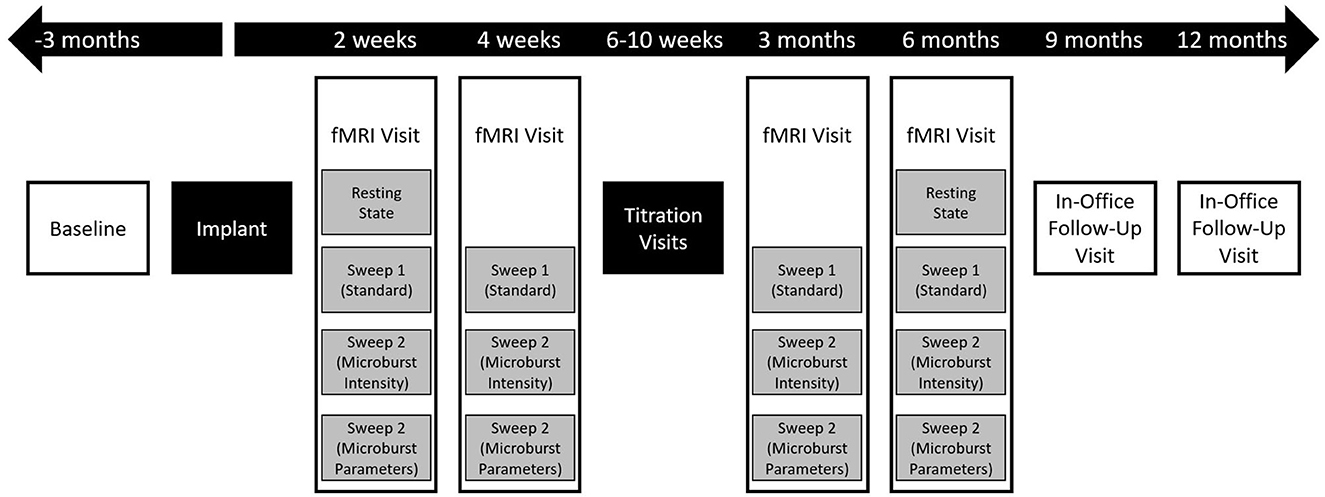
Figure 2. Flow chart of overall study events. After screening and consent, enrolled patients underwent a 3-month prospective baseline period followed by implantation. All subjects were then followed for up to 12 months, with the first 6 months including an intensive, imaging-guided titration program and the last 6 months including telephone and in-office visits. Clinical outcome measures were collected at all boxes shown in white, though reports of adverse events could be collected at any time, including outside of study visits. During fMRI visits (Figure 3), study outcome measures were collected sporadically during rest periods between scans to minimize the impact of the duration of the visit on the subject's schedule.
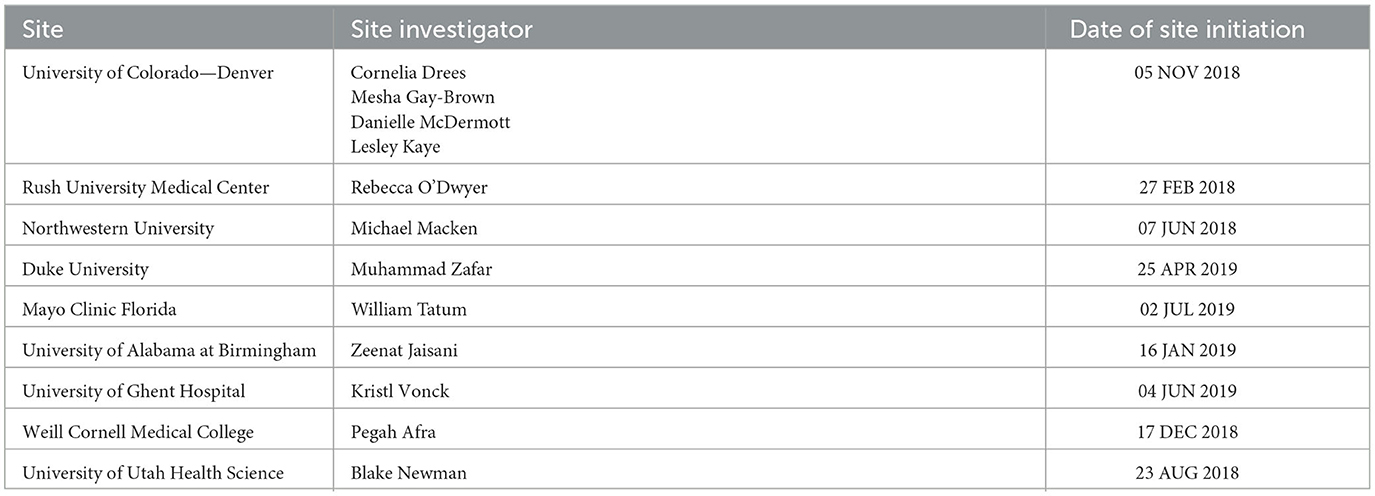
Table 1. Microburst study sites, site investigators, and date of site authorized to start recruitment.
2.1. Patient selection
Patients were recruited into a cohort based on their seizure history, baseline characteristics, and satisfaction of the inclusion criteria without meeting any of the exclusion criteria. No specific methods for patient recruitment were employed, and each site investigator was responsible for identifying appropriate patients in their practice to screen for the study. Patients recruited in the focal-onset seizure cohort had to have a clinical diagnosis of medically refractory epilepsy with focal-onset seizures, which could include seizures that secondarily progressed to bilateral tonic-clonic seizures, and had to have an average of at least three countable seizures per month during the 3-month baseline period without any seizure-free interval >30 days during the baseline period. Patients recruited into the PGTC seizure cohort had to have a clinical diagnosis of medically refractory idiopathic/genetic generalized epilepsy with generalized-onset tonic-clonic seizures, though they may also have other seizure types, and must have at least three countable seizures during the 3-month baseline period. Clinical diagnosis of PGTC seizures was required to be confirmed by historical EEG within the past 3 years by the investigator. If no historical EEG was available, a prospective EEG could be collected to verify the diagnosis by independent review.
Other inclusion criteria and exclusion criteria are described in Table 2.
2.2. Intervention
The VNS Therapy System is approved for use in epilepsy as an adjunctive treatment in reducing seizure frequency for adults and children 4 years of age or older (in Europe, all ages) with drug-resistant focal epilepsy (in Europe, also generalized epilepsy). The principal components of the system are an implantable VNS Therapy generator, a lead, and an external programming system used to change stimulation settings. The pulse generator is housed in a hermetically sealed titanium case and is powered by a single battery. Electrical signals are transmitted to the left cervical vagus nerve through the lead. The system is manufactured by LivaNova USA, Inc.
Subjects enrolled in this study received the investigational M1000C μB SenTiva™ VNS Therapy System along with a commercial, FDA approved VNS Therapy System lead, either the M302, M303, or M304. The M1000C unit was programmed to provide investigational “microburst” stimulation patterns (Figure 1). Microburst stimulation consists of short bursts of pulses separated by brief off-times called “interburst intervals” (IBI) (13). The microburst waveform can be fully described by 6 stimulation parameters with a range of available settings: Output Current, Pulse Width, Signal Frequency, Duty Cycle, Interburst Interval, and Number of Pulses (Table 3). The M1000C investigational VNS Therapy system provided all the basic functionality of previous VNS Therapy models as well as the new microburst feature under investigation.
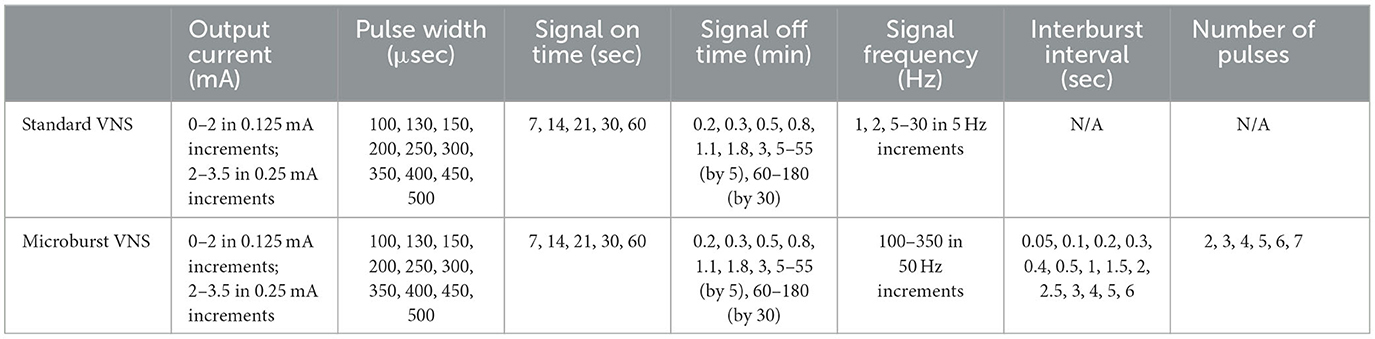
Table 3. VNS settings, microburst and standard VNS on the M3000C investigational VNS programming system.
In addition to the μVNS settings, the M1000C VNS Therapy System includes a “parameter sweep” feature that is designed to allow for the stimulation of the vagus nerve using up to 7 sets of existing parameter values (e.g., the choice of a value for each VNS parameter creates one set) over a short period of time. The parameter sets were delivered sequentially at pre-defined intervals (e.g., 5 min of parameter set 1, 5 min of parameter set 2, and so on). Simultaneously, the parameter sweep feature disengaged the functionality of the reed switch, which is an electrical component that responds to the presence of a strong magnetic field by opening or closing a circuit. Disengaging the reed switch allowed the M1000C VNS Therapy system to deliver stimulation inside the bore of a MRI scanner for investigational purposes.
All patients received an active VNS implant. There was no group with inactive or intentionally low output μVNS.
2.3. Titration strategy
A critically important element to the design of this study was the fMRI-guided titration strategy (Figure 3). Post-implant, at weeks 2, 4, 12, and 24, patients were required to return to their study site for a follow-up visit that included a personalized, BOLD-driven titration protocol. Subjects proceeded directly to an MRI scanning facility at the hospital for these visits, where they met with the site investigator, a sponsor's clinical engineer, and other MR facility personnel. First, tolerability of both standard VNS and μVNS was assessed to determine the maximum tolerability output current and pulse width for that study visit. Following the maximum tolerability determination, the subject's device was programmed using the parameter sweep function to deliver up to seven unique parameter sets over the course of the following 45–60 min (see Tables 4A, B for examples). The subject was then placed into the MRI scanner while the parameter sweep function was active. Each patient underwent a series of three fMRI scans with parameter sweeps per visit, totaling 45–60 min per scan or up to 180 min of scanning per visit day.
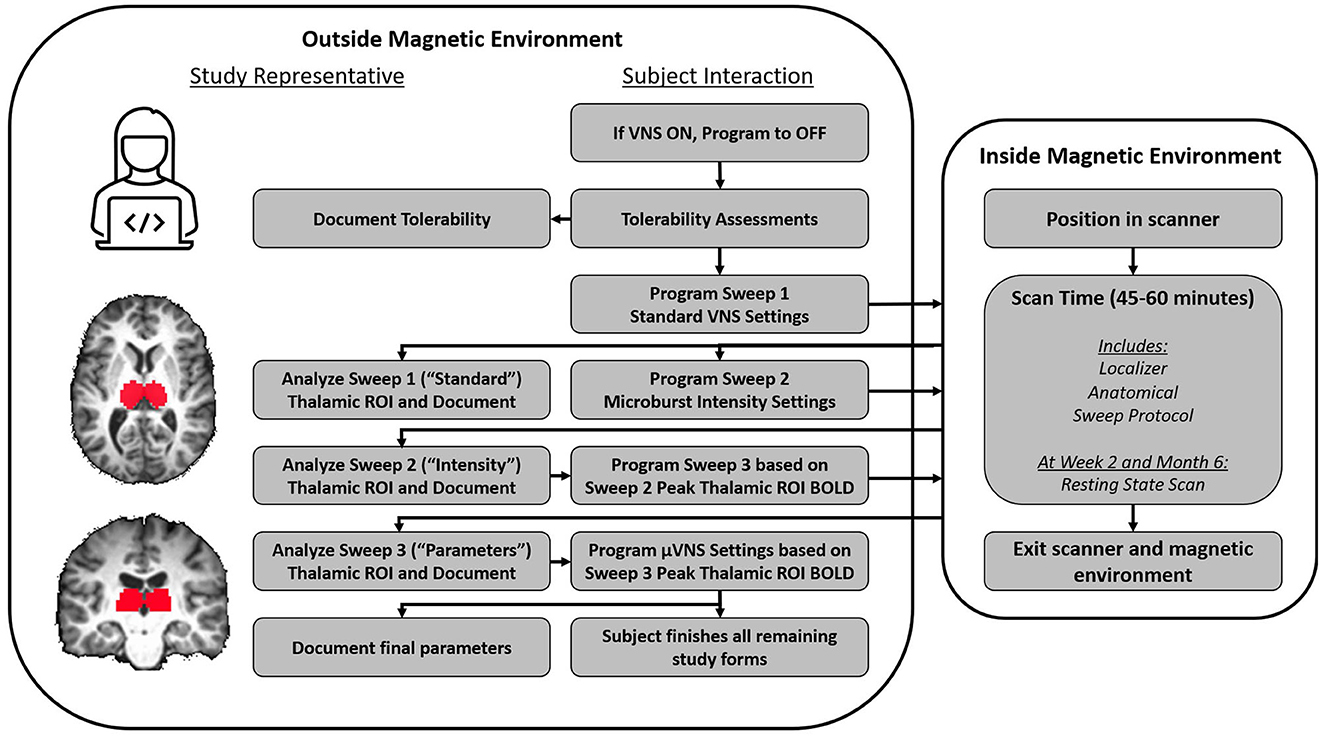
Figure 3. Flow chart of activities during an fMRI titration visit. The device was initially deactivated, and then, a tolerability protocol was followed. The purpose of the tolerability protocol was to identify VNS and μVNS intensities that evoked intolerable side effects so that side effects that induced involuntary movement (e.g., cough) could be avoided in the scanner. Based on the tolerability assessments, a maximum intensity was determined for VNS (Sweep 1) and μVNS (Sweeps 2 and 3). A study representative then programmed the Sweep 1 parameters into the device's “Parameter Sweep Mode”, which is an investigational function that allows the pulse generator to sequentially modify programming settings at a future time (e.g., in the scanner). The subject was then positioned into the scanner, and multiple functional and anatomical scans were collected. When Sweep 1 concluded, the subject was removed from the scanner and study representatives programmed Sweep 2 based on the tolerability assessment and re-admitted the subject to the scanning environment. While Sweep 2 was underway, study representatives analyzed the Sweep 1 fMRI data to identify the settings associated with the peak BOLD response in the thalamic ROI. When Sweep 2 concluded and the subject was removed from the scanner, study representatives analyzed the Sweep 2 fMRI results similar to the procedure for the Sweep 1 results; however, when programming the subject for Sweep 3, the optimal μVNS intensity identified in the Sweep 2 results was used. When Sweep 3 concluded, a final analysis was completed by study representatives to identify the μVNS parameters that the subject would leave the clinic with.
The principal objective of each 45–60 min scan was to examine the BOLD signal within a region of interest (ROI) centered over the left and right thalamus. After completion of the structural scanning protocol (structural voxel size not larger than 1 mm3 isotropic), a 30-min fMRI sequence was initiated at the same time as the first set of pre-programmed parameters from the parameter sweep (functional voxel size not larger than 4 mm3 isotropic). The parameter sweep programmed VNS settings in a 30 s ON/30 s OFF manner and switched to a new group of VNS settings every 5 min. This paradigm permitted a later off-line analysis of BOLD signal in the ON vs. OFF state for each pre-programmed group of settings. Maximal BOLD signal increases from each 45–60 min MR session within the thalamic ROI were used to identify settings for the next parameter sweep, refining the VNS programming with each scanning session. Settings for the next scan were determined by identifying settings in the preceding scan that resulted in the greatest thalamic ROI BOLD intensity and by the maximum t-value calculated from at least 2 contiguous voxels.
The first scan of a visit day assessed standard VNS settings, and the output current was the only parameter that varied during the fMRI session. Subjects started with a resting scan with no stimulation (VNS inactive) and then proceeded from a low-intensity stimulation to a higher intensity stimulation, as determined by the tolerability assessment from that visit day. After the scan, the subject was given a short break while the sponsor's engineer analyzed the fMRI data using a customized processing pipeline utilizing the Analysis of Functional NeuroImages (AFNI) software (24) to determine the output current associated with peak thalamic ROI BOLD signal increase. The subject was then programmed for a μVNS sweep that also assessed output current, starting from low intensities and moving to higher intensities limited by the tolerability. Patient tolerance to stimulation was assessed separately for both standard VNS and μVNS, so the output current settings were not always the same between the first and second parameter sweep protocols. After the second scan, the subject again exited the scanner and the sponsor's engineer analyzed the data. For the third scan, the patient's parameter sweep was programmed to the output current intensity of the thalamic ROI BOLD peak from the second scan. At that intensity, the other μVNS settings of IBI, number of pulses, and signal frequency were adjusted. After this scan was completed, the sponsor's engineer again analyzed the fMRI data using the custom fMRI processing pipeline. The pulse generator was programmed to the intensity (output current and pulse width) resulting from the second scan and the μVNS settings that drove peak thalamic ROI activation in the third scan. The patient left the clinic with these settings.
After the 4-week MRI visit, patients were asked to visit the clinic once every 2 weeks for titration of their output current, up to the 12-week MRI visit. While the relationship between standard VNS titration and μVNS titration is not fully understood, interim titration visits to adjust output current were performed so that patients would be more likely to achieve a dose range associated with effectiveness for standard VNS, likely between 1.5 mA and 2.25 mA at 250–500 μs (25). Output current increases during these titration visits were not aided by fMRI.
2.4. Outcome measures and data collection
The primary effectiveness endpoint was the percent change in seizure frequency per month (over a 3-month period) compared to the seizure frequency per month (over the 3-month period) calculated at baseline, at 6 months post-implant, and 12 months post-implant. The primary safety endpoint was the occurrence of stimulation-related adverse events in the first 6 months after implant and in months 6 to 12 thereafter.
The study also assessed other secondary outcome measures related to seizure severity (Seizure Severity Questionnaire; SSQ), quality of life (Quality of Life in Epilepsy scales; QOLIE-31P, QOLIE-AD-48), medication load (prescribed daily dose/defined daily dose), and suicidality (Columbia Suicide Severity Rating Scale).
During the MRI scanning days, at the 2-week and 6-month visits, resting state fMRI was also collected from each patient as an exploratory outcome. This was collected at the beginning of the scanning day, shortly after the tolerability assessment but before any other MRI procedures were performed.
Study data were collected by site investigators or their designees and were entered into a custom-built, 21 CFR Part 11 compliant electronic data capture system managed by the study sponsor for subsequent analysis.
2.5. Statistical analysis methods
This clinical study was exploratory in nature. All the inferential statistics should be considered hypothesis-generating in nature and not confirmatory. Cohorts were not powered for the purpose of confirmatory statistical testing, and the population characteristics of each cohort are not expected to be suitable for a clinically meaningful comparison. Each cohort will be analyzed separately as soon as each cohort completes the relevant recruitment and subjects reach the expected follow-up threshold. Descriptive statistics (mean, standard deviation, median, mode, range, and confidence intervals, as appropriate) will be used to describe the population outcome of within-subject changes between baseline and each follow-up visit.
We plan for an intermediate analysis at the time of all subjects completing their 6-month follow-up visit. The final analysis was conducted when all subjects completed the study at the 12-month visit.
2.6. Withdrawal of consent and study exit
Subjects were permitted to withdraw their consent for the study at any time. Withdrawal of consent could be made through not signing a study-related form, through checking a box on that form indicating the subject's intention to withdraw their consent, or by emailing a representative of the sponsor directly if the subject was not actively completing study related forms.
Study investigators were also empowered to withdraw subjects from the study if they perceived a developing or active safety concern.
3. Discussion and design limitations
The study was designed to demonstrate the safety and potential efficacy of the investigational μVNS stimulation paradigm. In addition to this primary objective, an investigational fMRI protocol was employed to guide patients to an appropriate personalized dose of the therapy. The presence of two investigational variables in this study may increase the difficulty of assigning treatment effect sizes.
There were also risks to the study outcome driven by choices made in the design of the fMRI protocol. At the time of study design, the best choice of target ROI for standard vs. microburst was not clear; hence, a decision was made to use a thalamic ROI as the target measure of VNS response with adjustments based on the peak of BOLD responses. The selection was grounded in the data from the available literature including previous VNS neuroimaging studies (20–22, 26). It was also unclear at that time whether the peak is the best measure and whether, e.g., the volume of activated tissue in the thalamus or the volume of the overall activated brain should be used instead. The VNS cycle time in the scanner also created risks, as there is little available evidence in humans to confirm the validity of the 30 s off-time for washing out VNS effects in the central nervous system. Further, it was not feasible to analyze all available options for parameters; thus, it was possible to have missed an optimal parameter. Finally, randomization of treatment settings (intensity, or other μVNS parameters) was not conducted within each scan in order to reduce the risk of side effects that would impact the imaging procedure (e.g., a participant coughing during fMRI acquisition). Regarding the risk of bias driven by patient selection, there were no indications from the literature on whether the VNS treatment targets should be different between focal epilepsies and idiopathic/genetic generalized epilepsies.
Due to the complicating factor of the investigational fMRI titration paradigm, the investigators proposed a publication plan that specifically addresses subject outcomes during the titration phase separately from the longer-term outcomes. A pair of study outcomes manuscripts will be developed to address these matters in the future. In addition, one or more manuscripts focused on the potential mechanism of μVNS and its impact of resting state functional networks will be developed using the fMRI data.
Ethics statement
The studies involving human participants were reviewed and approved by Northwestern University Biomedical IRB, Rush University Medical Center IRB, University of Utah IRB, Weill Cornell Medical College IRB, Western Institutional Review Board, Mayo Clinic Institutional Review Board, and Ethisch Comite UZ Gent. Written informed consent to participate in this study was provided by the participants' legal guardian/next of kin.
Microburst Study Group
Danielle McDermott, University of Colorado and Denver Health; Mesha Gay Brown, University of Colorado School of Medicine; Lesley Kaye, University of Colorado School of Medicine; Michael Macken, Northwestern University; William O. Tatum, Mayo Clinic Florida; Cornelia Drees, Mayo Clinic Arizona; Selim R. Benbadis, University of South Florida; Zeenat Jaisani, University of Alabama at Birmingham; Muhammad Zafar, Duke University Hospital; Kristl Vonck, Ghent Unviersity Hospital; Rebecca O'Dwyer, Rush Epilepsy Center, Rush University Medical Center, Chicago, IL; Blake Newman, Univ of Utah School of Medicine; Pegah Afra, Weill-Cornell Medicine, University of Utah School of Medicine; Jane Allendorfer, University of Alabama at Birmingham (UAB) Department of Neurology; Jerzy P. Szaflarski, University of Alabama at Birmingham (UAB) Department of Neurology and the UAB Epilepsy Center, Birmingham, AL, USA; Ryan Verner, LivaNova PLC; Kathryn Nichol, LivaNova PLC; Charles Gordon, LivaNova PLC; Jason Begnaud, LivaNova PLC; Amy Keith, LivaNova PLC; Elhum Shamshiri, LivaNova PLC; Steffen Fetzer, LivaNova PLC; Giovanni Ranuzzi, LivaNova PLC; Gaia Giannicola, LivaNova PLC; Mei Jiang, LivaNova PLC; Wim Van Grunderbeek, LivaNova PLC; Irena Bellinski, Northwestern University; Elizabeth Cunningham, Northwestern University; Ann Mertens, Ghent Unviersity Hospital; Fiona Lynn, Rush Epilepsy Center, Rush University Medical Center, Chicago, IL; and Seyhmus Aydemir, Cornell.
Author contributions
RV, JS, KV, and GG equally contributed to the initial draft of the manuscript. JA provided additional feedback during the draft revision process. The remainder of the Microburst Study Group offered critical review and feedback. All authors contributed to the article and approved the submitted version.
Funding
The Microburst Feasibility Study was sponsored by LivaNova USA, Inc., Houston, TX, United States.
Acknowledgments
The authors acknowledge the work of LivaNova staff, specifically Amy Keith, Jeffrey Way, Katherine Eggleston, Kenny Henderson, Bita Najimipour, and Jason Begnaud, who worked with external experts in the initial design of the study protocol. External experts who provided critical input during the study design process included the Microburst Study Group investigators, specifically the lead investigator Selim Benbadis, as well as Deepak Madhavan.
Conflict of interest
RV and GG are employees of LivaNova PLC or a subsidiary, and own stock and/or stock options with the sponsor of this study. KV was an investigator on the Microburst Feasibility Study. JS and JA developed the imaging protocol for this study under a consulting agreement with LivaNova USA, Inc. KV, JA, and JS have active consulting agreements with LivaNova PLC or its subsidiary businesses, related to advisory services, speaking services, and/or research activities. The Microburst Study Group consists of site investigators from each clinical study site. These investigators received some funding from LivaNova USA, Inc. to execute the Microburst Feasibility Study. No author was compensated for time spent writing this manuscript, and the content reflects the views of the authors and not LivaNova PLC or a subsidiary.
Publisher's note
All claims expressed in this article are solely those of the authors and do not necessarily represent those of their affiliated organizations, or those of the publisher, the editors and the reviewers. Any product that may be evaluated in this article, or claim that may be made by its manufacturer, is not guaranteed or endorsed by the publisher.
Supplementary material
The Supplementary Material for this article can be found online at: https://www.frontiersin.org/articles/10.3389/fneur.2023.1169161/full#supplementary-material
References
1. Salanova V, Witt T, Worth R, Henry TR, Gross RE, Nazzaro JM, et al. Long-term efficacy and safety of thalamic stimulation for drug-resistant partial epilepsy. Neurology. (2015) 84:1017–25. doi: 10.1212/WNL.0000000000001334
2. Vetkas A, Fomenko A, Germann J, Sarica C, Iorio-Morin C, Samuel N, et al. Deep brain stimulation targets in epilepsy: systematic review and meta-analysis of anterior and centromedian thalamic nuclei and hippocampus. Epilepsia. (2022) 63:513–24. doi: 10.1111/epi.17157
3. Heck CN, King-Stephens D, Massey AD, Nair DR, Jobst BC, Barkley GL, et al. Two-year seizure reduction in adults with medically intractable partial onset epilepsy treated with responsive neurostimulation: final results of the RNS System Pivotal trial. Epilepsia. (2014) 55:432–41. doi: 10.1111/epi.12534
4. Sisterson ND, Kokkinos V, Urban A, Li N, Richardson RM. Responsive neurostimulation of the thalamus improves seizure control in idiopathic generalised epilepsy: initial case series. J Neurol Neurosurg Psychiatry. (2022) 93:491–8. doi: 10.1136/jnnp-2021-327512
5. Kwon C-S, Schupper AJ, Fields MC, Marcuse LV, Vega-Talbott ML, Panov F, et al. Centromedian thalamic responsive neurostimulation for Lennox-Gastaut epilepsy and autism. Ann Clin Transl Neurol. (2020) 7:2035–40. doi: 10.1002/acn3.51173
6. Bolden L, Pati S, Szaflarski J. Neurostimulation, neuromodulation, and the treatment of epilepsies. J Epileptol. (2015) 23:45–59. doi: 10.1515/joepi-2015-0022
7. Nair DR, Laxer KD, Weber PB, Murro AM, Park YD, Barkley GL, et al. Nine-year prospective efficacy and safety of brain-responsive neurostimulation for focal epilepsy. Neurology. (2020) 95:e1244–56. doi: 10.1212/WNL.0000000000010154
8. Carron R, Roncon P, Lagarde S, Dibué M, Zanello M, Bartolomei F. Latest views on the mechanisms of action of surgically implanted cervical vagal nerve stimulation in epilepsy. Neuromodulation. (2022) 26:498–506. doi: 10.1016/j.neurom.2022.08.447
9. Krahl SE, Clark KB, Smith DC, Browning RA. Locus coeruleus lesions suppress the seizure-attenuating effects of vagus nerve stimulation. Epilepsia. (1998) 39:709–14. doi: 10.1111/j.1528-1157.1998.tb01155.x
10. Krahl SE, Senanayake SS, Handforth A. Seizure suppression by systemic epinephrine is mediated by the vagus nerve. Epilepsy Res. (2000) 38:171–5. doi: 10.1016/S0920-1211(99)00089-3
11. Ito S, Craig AD. Vagal-evoked activity in the parafascicular nucleus of the primate thalamus. J Neurophysiol. (2005) 94:2976–82. doi: 10.1152/jn.00235.2005
12. Ito S, Craig AD. Striatal projections of the vagal-responsive region of the thalamic parafascicular nucleus in macaque monkeys. J Comp Neurol. (2008) 506:301–27. doi: 10.1002/cne.21513
13. Huang YZ, Edwards MJ, Rounis E, Bhatia KP, Rothwell JC. Theta burst stimulation of the human motor cortex. Neuron. (2005) 45:201–6. doi: 10.1016/j.neuron.2004.12.033
14. Shah PP, Szaflarski JP, Allendorfer J, Hamilton RH. Induction of neuroplasticity and recovery in post-stroke aphasia by non-invasive brain stimulation. Front Hum Neurosci. (2013) 7:888. doi: 10.3389/fnhum.2013.00888
15. Martlé V, Peremans K, Raedt R, Vermeire S, Vonck K, Boon P, et al. Regional brain perfusion changes during standard and microburst vagus nerve stimulation in dogs. Epilepsy Res. (2014) 108:616–22. doi: 10.1016/j.eplepsyres.2014.02.004
16. Martlé V, Raedt R, Waelbers T, Smolders I, Vonck K, Boon P, et al. The effect of vagus nerve stimulation on CSF monoamines and the PTZ seizure threshold in dogs. Brain Stimul. (2015) 8:1–6. doi: 10.1016/j.brs.2014.07.032
17. Alexander GM, McNamara JO. (2012). Vagus nerve stimulation elevates seizure threshold in the kindling model. Epilepsia, 53, 2043-52. doi: 10.1111/j.1528-1167.2012.03646.x
18. Szabó CÁ, Salinas FS, Papanastassiou AM, Begnaud J, Ravan M, Eggleston KS, et al. High-frequency burst vagal nerve simulation therapy in a natural primate model of genetic generalized epilepsy. Epilepsy Res. (2017) 138:46–52. doi: 10.1016/j.eplepsyres.2017.10.010
19. Maniker A, Liu WC, Marks D, Moser K, Kalnin A. Positioning of vagal nerve stimulators: technical note. Surg Neurol. (2000) 53:178–81. doi: 10.1016/S0090-3019(99)00176-7
20. Bohning DE, Lomarev MP, Denslow S, Nahas Z, Shastri A, George MS. Feasibility of vagus nerve stimulation-synchronized blood oxygenation level-dependent functional MRI. Invest Radiol. (2001) 36:470–9. doi: 10.1097/00004424-200108000-00006
21. Lomarev M, Denslow S, Nahas Z, Chae JH, George MS, Bohning DE. Vagus nerve stimulation (VNS) synchronized BOLD fMRI suggests that VNS in depressed adults has frequency/dose dependent effects. J Psychiatr Res. (2002) 36:219–27. doi: 10.1016/S0022-3956(02)00013-4
22. Mu Q, Bohning DE, Nahas Z, Walker J, Anderson B, Johnson KA, et al. Acute vagus nerve stimulation using different pulse widths produces varying brain effects. Biol Psychiatry. (2004) 55:816–25. doi: 10.1016/j.biopsych.2003.12.004
23. Narayanan JT, Watts R, Haddad N, Labar DR, Li PM, Filippi CG. Cerebral activation during vagus nerve stimulation: a functional MR study. Epilepsia. (2002) 43:1509–14. doi: 10.1046/j.1528-1157.2002.16102.x
24. Cox RW. AFNI: software for analysis and visualization of functional magnetic resonance neuroimages. Comput Biomed Res. (1996) 29:162–73. doi: 10.1006/cbmr.1996.0014
25. Fahoum F, Boffini M, Kann L, Faini S, Gordon C, Tzadok M, et al. VNS parameters for clinical response in Epilepsy. Brain Stimul. (2022) 15:814–21. doi: 10.1016/j.brs.2022.05.016
Keywords: vagus nerve stimulation, drug-resistant epilepsy, focal epilepsy, generalized epilepsy, feasibility study
Citation: Verner R, Szaflarski JP, Allendorfer JB, Vonck K, Giannicola G and Microburst Study Group (2023) Modulation of the thalamus by microburst vagus nerve stimulation: a feasibility study protocol. Front. Neurol. 14:1169161. doi: 10.3389/fneur.2023.1169161
Received: 18 February 2023; Accepted: 04 May 2023;
Published: 13 June 2023.
Edited by:
Tianfu Li, Capital Medical University, ChinaReviewed by:
Kedi Xu, Zhejiang University, ChinaMichael D. Staudt, Oakland University William Beaumont School of Medicine, United States
Copyright © 2023 Verner, Szaflarski, Allendorfer, Vonck, Giannicola and Microburst Study Group. This is an open-access article distributed under the terms of the Creative Commons Attribution License (CC BY). The use, distribution or reproduction in other forums is permitted, provided the original author(s) and the copyright owner(s) are credited and that the original publication in this journal is cited, in accordance with accepted academic practice. No use, distribution or reproduction is permitted which does not comply with these terms.
*Correspondence: Ryan Verner, cnlhbi52ZXJuZXJAbGl2YW5vdmEuY29t
†These authors have contributed equally to this work