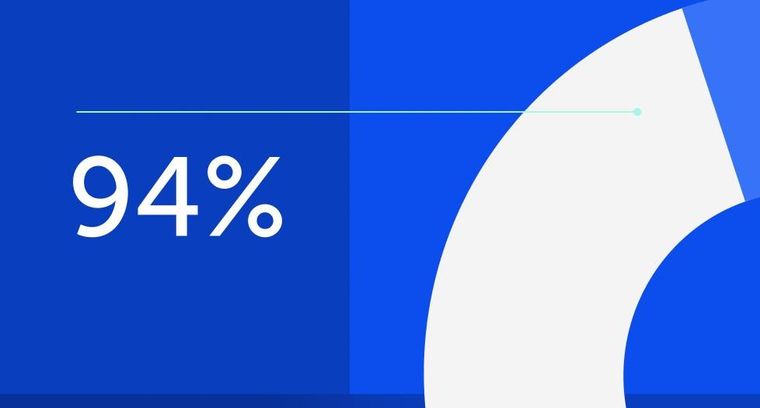
94% of researchers rate our articles as excellent or good
Learn more about the work of our research integrity team to safeguard the quality of each article we publish.
Find out more
SYSTEMATIC REVIEW article
Front. Neurol., 24 March 2023
Sec. Pediatric Neurology
Volume 14 - 2023 | https://doi.org/10.3389/fneur.2023.1092205
Introduction: The movement of fluids and solutes across the ependymal barrier, and their changes in physiologic and disease states are poorly understood. This gap in knowledge contributes strongly to treatment failures and complications in various neurological disorders.
Methods: We systematically searched and reviewed original research articles treating ependymal intercellular junctions on PubMed. Reviews, opinion papers, and abstracts were excluded. Research conducted on tissue samples, cell lines, CSF, and animal models was considered.
Results: A total of 45 novel articles treating tight, adherens and gap junctions of the ependyma were included in our review, spanning from 1960 to 2022. The findings of this review point toward a central and not yet fully characterized role of the ependymal lining ultrastructure in fluid flow interactions in the brain. In particular, tight junctions circumferentially line the apical equator of ependymal cells, changing between embryonal and adult life in several rodent models, shaping fluid and solute transit in this location. Further, adherens and gap junctions appear to have a pivotal role in several forms of congenital hydrocephalus.
Conclusions: These findings may provide an opportunity for medical management of CSF disorders, potentially allowing for tuning of CSF secretion and absorption. Beyond hydrocephalus, stroke, trauma, this information has relevance for metabolite clearance and drug delivery, with potential to affect many patients with a variety of neurological disorders. This critical look at intercellular junctions in ependyma and the surrounding interstitial spaces is meant to inspire future research on a central and rather unknown component of the CSF-brain interface.
The ependymal barrier, a simple cuboidal epithelium that lines the ventricular and aqueductal surfaces and interfaces with the brain parenchyma, is one of multiple nodes of intraventricular and extraventricular CSF circulation. For decades the ependyma has been seen as a permeable, metabolically and functionally inactive covering of the cerebral ventricles, aqueduct and central canal of the spinal cord. While the choroid plexus, according to the classic “Third Circulation” theory by Dandy and Blackfan (1, 2), has been considered as the main source of CSF production, recent microscopic and molecular studies have suggested a possible role for perivascular, lymphatic and ependymal pathways in contributing to absorption and secretion of CSF and interstitial fluid. This exchange could be even more important in disease states such as hydrocephalus, Alzheimer's disease, Normal Pressure Hydrocephalus (3), accounting for a larger proportion of fluid production. Further, this new data spurred renewed interest in the field of ependymal paracellular flow and in the changes that intercellular junctions undergo in utero and during postnatal development. The transependymal route, while accounting for only about 5–10% of total CSF reabsorption in normal states, may constitute an important route for CSF exchange in pathologic conditions. Junctional proteins such as cadherins, connexins and claudins are at the heart of its barrier function, and alterations in their characteristics, expression, integrity could affect the paracellular flow of solutes and water.
Despite growing evidence pointing toward a pivotal role for ependyma in ventricular development and CSF movement, the full extent of the involvement of intercellular junctions in trans-ependymal flow is still unclear. Further, the physiologic age-related changes in ependymal integrity and permeability have not been fully characterized. Despite recent research suggesting a role in CSF homeostasis as well as primitive neurogenesis, a thorough understanding of ependymal junctional complexes is still missing. Given the recent contributions on the role of these structures in congenital, communicating and obstructive hydrocephalus, the novel techniques used in recent years to assess flows, the discovery of alternative pathways of drainage in the brain and subarachnoid spaces, we sought to review the current state of the evidence on adherens, gap and tight junctions in the ependymal layer. The aim is to provide the reader with the most updated evidence on the role of junctions in fluid, solute flow and development of CNS-related disorders. Starting from a number of foundational studies based on electron microscopy, we integrated that knowledge with information generated with modern fluorescent imaging techniques, to provide a glimpse of the potential role of these proteins in several pathologies of the ependyma and various forms of hydrocephalus.
This review is limited to original research on the mammalian and non-mammalian ependymal system published in the past 50 years. A search of the PubMed database was conducted for the terms “gap junction,” “tight junction,” “adherens junction,” “ependyma,” as well as specific protein types “claudin,” “occludin,” “JAM,” “zonula,” “cadherin,” and “connexin.” Abstracts were reviewed, only manuscripts presenting new research were selected, and full texts were considered in the final analysis. Abstracts not associated with a published manuscript were excluded. Figure 1 and Table 1 summarize and outline the selection process used for this review. A total of 45 studies that met these criteria were identified and considered for the final manuscript. Data from each of these papers was included in the review and interpreted in the “Discussion” section. This review was conducted according to the PRISMA checklist (41).
Figure 1. Flow diagram of the systematic research and selection process used to identify the final papers in this review.
Extensive evidence has been gathered over the last decades on the composition and role of the blood-brain and blood-CSF barriers, at the capillary-astrocytic level and in the choroid plexuses (42–46). Much less is known for the CSF-brain interface at the ependymal level, despite its significant surface area and close relationship to the parenchyma and deep nuclei of the brain. The ependymal layer, despite the general belief, provides a regulated barrier to the diffusion of solutes and water between the CSF and interstitial compartments. Both regionality and age play a significant role in determining the magnitude and selectivity of this route.
The first data on the intercellular junctions at this location was gathered using electron microscopy, starting in the 1960s, when Tennyson and Pappas reported the presence of highly elaborated, thin cytoplasmic plates or finger-like projections emanating from the ependymal cells covering the aqueduct of rabbit models (4). Duckett et al. noticed that the arrangement of the germinal layer cells resembled that of other absorptive units such as the renal proximal tubules, the gallbladder and the intestine, and suggested an absorptive function for the ependyma (5, 47). Rieke et al. investigated the ependymal ultrastructure of embryonic rat cells (gestational day 14–17) via electron microscopy. Again, their observations confirmed the expression of zonula occludens and adherens in the apical third of the cell membrane, as well as membrane interdigitations (13).
A significant variability between different areas of the ventricular lining is present. While choroid plexus epithelia have fully formed apical tight junctions that constitute the blood-CSF barrier (46), circumventricular organs show hybrid characteristics. The choroid plexus is characterized by welldeveloped pentalaminar tight junctions, whereas the ependyma presents only “labile” and non-overlapping rows of morphologically distinguishable appositions that do not interfere with the intercellular movement of tracers (8). Local expression of apical claudin-3 as well as orthogonal arrays of particles have been described along the circumventricular organs, with a notable reduction in horseradish peroxidase diffusion compared to the surrounding regions (11). Similar findings were reported by Altafulla et al. in a rat model of intraventricular sucrose injections. While significant and rapid movement from the lateral ventricles across the ependymal layer was noticed, a slower rate of diffusion was present in the circumventricular organs, medullary and cerebellar tissue, superior and inferior colliculi and cerebral peduncles (48).
Other examples of self-regulating capacity come from local injection of reagents and carbohydrates. Ependymal, but not choroidal, junctional damage with phorbol ester is able to reduce the movement of biotinylated dextran by modulating protein kinase C activity (49). Kuchler et al. also showed that intraventricularly-injected mannose-containing neoglycoproteins induced the disappearance of intercellular junctions (50).
Several works have focused on age-dependent evolutions in tight and “strap” junctions (51). Cavanagh and Warren investigated the movement of endogenous vs. sheep albumin using a rodent model of CSF flow. Both proteins were detected in the brain parenchyma starting on day 16–17 of gestation, but not earlier, mimicking the changes in tight junction appearance seen in other studies with contrast tracers. Further, transcellular movement of albumin into the ependymal cells and sub-ependymal layer of the cortex was described, suggesting the existence of a specialized mechanism of uptake rather than paracellular unrestricted diffusion (9).
Fossan et al. determined that extracellular penetration of several millimeters from the ependyma is present in older sheep fetuses and adults. Partial limitation of brain parenchyma diffusion was described in younger fetuses, with a steady increase in barrier permeability with age of both radioactive ([3H]inulin, [14C]sucrose, [125I]albumin) and fluorescent (horseradish peroxidase) markers (6). Møllgård et al. studied gap and tight junctions in a sheep model of the ventricular zone germinal matrix. With freeze-fracture electron microscopy they were able to describe not only spirals of zonulae adherens junctions, but also large gap junctions extending from the ventricular pole along the lateral cell membrane (7).
Mori and Kazanis demonstrated that neuroepithelial cells of the neural tube are connected by gap, adherens and tight junctions until embryonic day 12 (E12), but that tight junctions regress and disappear from E12 onward (52, 53). A genetic or cellular insult can therefore affect neural and ependymal lining integrity if delivered at this point in the neural tube development (54).
Age-related changes in ependymal permeability are also suggested by the pathophysiology of several hydrocephalus models. These confirmed that junctional proteins are involved in ependymal stability, and that alterations in brain and ventricular development result from their mutation. The hyh and other models characterized by cell-cell junctional defects have shown abnormal flow of CSF, VZ disruption and eventually development of hydrocephalus (37, 38, 55, 56) (Table 2).
In recent years, with the advent of fluorescent microscopy, novel dextran-based tracers and mRNA sequencing, the expression profile of intercellular junctions has finally started to become clearer. Whish et al. (14) provided the first evidence of age-related changes in protein and gene expression during the transition from the embryonic ventricular zone to the adult ependyma. Interestingly, a significant limitation to the passage of larger tracers was noticed early in ependymal development, possibly part of a defensive mechanism that protects the developing brain tissue from high CSF protein concentrations (14). Some suggest that the barrier function plays an important role in restricting access to the subependymal brain tissue during fetal life (10, 59–65), protecting the brain from high protein concentrations that would cause developmental impairment and tissue damage (66). Others hypothesize that the high colloidal pressure functions as a tool to drive water into the ventricles (66–68), creating a hydrostatic pressure gradient necessary for cortical gyral growth (Figure 2).
Figure 2. Overview of membrane, trafficking, junctional, cytoplasmic structural proteins involved in intercellular junctions and pathological processes of the ependyma. Failure of one of these components can induce ependymal denudation and hydrocephalus in murine models. A dedicated legend is provided with the figure.
Tight junctions are a central component of non-permeable epithelia, allowing cells to create a physical barrier between two compartments. Also called zonula occludens, these junctions are found in epithelial and endothelial cells and regulate the passage of cells, solutes and water. Usually located in the apical portion of the cell membrane, tight junctions structurally occlude the intercellular space between adjacent cells, creating an impermeable belt that spans the entire epithelium (69). Single- and four-pass transmembrane proteins, such as claudins, occludins and JAMs (junctional adhesion molecule), constitute the bulk of this class of proteins (69). Despite the evidence of belt-like apical junctional complexes in ependymal cells that was reported by several groups starting in the 1960s (4, 7), the significant permeability of this cellular layer to inulin, sucrose, albumin and horseradish peroxidase suggested a limited and morphologically incomplete expression of these junctions at the ependymal level (6). Recent studies shed light on several components of this class of proteins, suggesting a significant degree of plasticity during the first weeks of life and a prominent role in shaping membrane permeability to solutes and water.
The presence of a developmentally-regulated CSF–brain barrier was first hypothesized in the 1960s, when “strap junctions” were identified between neuroepithelial cells in early stages of development via electron microscopy. This function may play an important role in restricting access of proteins, present in high concentration in fetal CSF, to the brain parenchyma during neurodevelopment. A study examining the cellular distribution of native and foreign albumins injected into fetal and neonatal ventricles of rats further supported this hypothesis (9).
Recently, this interface has been the object of several studies with lipid insoluble markers that used a wide range of molecular weights, in conjunction with the rebirth of CSF circulation studies and discovery of the glymphatic system. In a recent paper, Whish et al. found that the barrier is permeable only to smaller molecules during early embryonic life and that the diffusional restraint is removed progressively during fetal and post-natal life (14). Specifically, the diffusion of molecules >3 kDa was limited at E17 and E19 but resumed at P0. Similar results were noticed for tracers with a weight >10 kDa. No significant difference in CSF protein concentrations was found between fetal and P0 animals, while a lower concentration was measured starting from P2. RNA sequencing was also performed during fetal and adult age, showing a switch from Claudin-5 expression to Claudin-11 between fetal and adult animals. These molecular changes may explain the higher permeability of the ependymal layer in adult animal models, providing important information for future studies in intercellular permeability. Most importantly, this evidence confirmed the existence of a molecular change at the ependymal level.
Other structures formed by multiple intramembrane particles such as aquaporin-4 and other junctional proteins are the orthogonal arrays of particles (OAP). OAPs are usually found in the glia limitans, but have also been described in the ependymal layer and in striated muscle, kidney, and stomach (70, 71). Mack et al. suggested the existence of a transitional zone between the ventricular ependyma and choroid plexus where the density of OAP is low and tight junctions are well developed. OAPs are usually prevalent in the ependymal layer, while tight junctions predominate in the choroid plexus (11). Gotow and Hashimoto also found one or two relatively continuous strands of tight junctions with interruptions in the apical portion of ependymocytes in the rat subcommissural organ. HRP infusions left parts of the intercellular spaces between membrane fusions of tight junctions unstained. Nonetheless, the intercellular space below the junction is usually stained by the enzyme, testifying dye movement across the ependymal lining (12).
Other members of the claudin superfamily such as claudin-1, - 2, - 3 were described in detail in the lateral and fourth ventricle choroid plexus, playing a role in fluid and ion secretion. Interestingly, these proteins appear to also line the choroid and ependymal epithelia, as well as the circumventricular organs, probably contributing to trans-ependymal flow of CSF and ions (22).
Occludin, the first transmembrane protein localized at the TJ, shares the tetraspan structure with cytoplasmic N- and C-termini with claudins. Initially thought to contribute barrier function, as well as cellular orientation, paracellular flow and lipid segregation (72), its role has been expanded in recent years to intracellular signaling via the PI3K pathway (73). Further, it is expressed in the brain vascular epithelium and has recently been connected with a congenital form of simplified gyration and polymicrogyria (15, 74).
Interestingly, upon development of parasitic ventricular infection, ependymal occludin expression appears to be reduced, allowing for increased leukocyte and microbial transmigration, as well as infection spread (23). A recent study also highlighted the changes in TJ expression secondary to variations in estrogen levels in female rodents. Kang et al. suggested the presence of occludin in murine ependyma and showed a significant decrease in expression in ovariectomized mice. Further, 17β estradiol was noticed to up-regulate occludin mRNA levels in these animals (21).
Junctional adhesion molecules (JAMs) are part of the immunoglobulin superfamily involved in a number of cellular processes, from tight junction assembly, to angiogenesis, leukocyte transmigration, platelet activation (75). Their function might be related to structural or barrier integrity, and recent evidence points toward higher expression of Jam-2 and Jam-3 in adult compared to embryonic rodents (14). A novel mutation in the Jam-3 gene was recently identified in a consanguineous family (15). Subependymal calcifications and hydrocephalus, as well as hemorrhagic destruction of the brain parenchyma were reported, suggesting a possible role in ependymal stability (15).
MPDZ is a scaffold protein of the apical complex responsible for junctional stability and frequently bound to other scaffolding proteins such as DAPLE (16, 76). Interestingly, these proteins are involved in non-syndromic forms of congenital hydrocephalus. Specifically, disruption of TJs secondary to abnormal cell-cell adhesion by MPDZ (also named MUPP1) has recently been reported in several fetuses with congenital hydrocephalus. The mechanism by which MDPZ alters CSF dynamics is likely secondary to uncontrolled production of fluid and ependymal denudation with obstruction of the aqueduct and subarachnoid spaces (17).
Feldner et al. (18) further investigated the role of MDPZ in CSF ependymal dynamics by generating mouse models with global MDPZ gene deletion. These animals showed inactivation of Nestin-positive cells, with development of hydrocephalus in the postnatal period. Despite showing morphologically normal ependymal cells, MDPZ-deficient animals had lower expression of the interacting planar cell polarity protein Pals1 and progressive loss of barrier integrity. Further, ependymal denudation was observed, together with reactive astrogliosis and aqueductal stenosis, suggesting a role for tight junctions in ependymal structural integrity (18). MRI studies have also confirmed contrast percolation in mice carrying a MDPZ loss-of-function mutation. CSF proteomic analysis of normal and MDPZ null hydrocephalic mice showed a 53-fold increase in protein concentration. This evidence suggested higher choroidal and ependymal transcytosis of fluid and solutes, explaining the development of hydrocephalus following MDPZ loss-of-function (19).
Zonula Occludens-1 (ZO-1) is part of a family of three structurally related proteins, ZO-1, ZO-2 and ZO-3 that mediate protein-protein interactions downstream of tight and adherens junction complexes. Specifically, ZO-1 is responsible for binding claudins and JAMs, as well as the adherens junction proteins afadin and α-catenin, connecting these structures to the actin cytoskeleton (77–83). Petrov et al. (20) investigated the role of this protein in murine circumventricular organs using immunofluorescence techniques, showing a punctate pattern of ZO-1 distribution, suggestive of discontinuous tight junctions at the circumventricular and ependymal level. Interestingly, continuous ZO-1 distribution was observed in the areas adjacent to the organum vasculosum of the laminae terminalis and the subcommissural organ, probably a sign of unbroken tight and/or adherens junctions in these regions (20).
Cadherins are the major Ca2+-dependent families of junctional proteins present in the brain, and a central component of the ependymal tight junctions. First described in the 1980s in ultrastructural studies of the ependymal cell, their position and role have been the object of several studies in animal models and human tissue. Interestingly, both N- and E- cadherins co-exist at the ependymal level, mediating similar functions at the ependymal cell border and playing a fundamental role for structural integrity. Further, they appeared to be responsible for ependymal denudation and hydrocephalus when mutated. Several junction-associated proteins and downstream mediators, such as β-catenins, p120 and paxillins were also stained on the cytoplasmic surface. Phosphotyrosine immunoreactivity points toward the presence of several other proteins involved in downstream signaling, suggesting a role for adherens junctions in determining cell orientation (84). Rieke et al. described adherens junctions as a distinct intercellular space between membranes with dense thickening of the cytoplasmic surface, spanning a significant area on apposed cells (13). Importantly, cadherins appear to play a central role for ependymal structural stability and cell integrity, as suggested by several studies in congenital hydrocephalus models.
Oliver et al. (24) showed that blocking N-cadherin function with specific peptides that target the histidine-alanine-valine extracellular homophilic interaction domain can cause disruption of the zonula adherens and abnormal intracellular accumulation and sequestration of N-cadherin. This precedes ependymal apoptosis and disruption, with final generalized denudation. Significant reduction in the extension of adherens junctions and development of asymmetrical distribution was noticed after inhibition with HAV-peptide. Interestingly, ependymal cells start undergoing apoptosis before denudation, with ~80% of cells positive on TUNEL test after 24 h from treatment with HAV-peptide. These changes take place in absence of hydrocephalus, increased ICPs or stretch forces, and suggest that cadherins and adherens junctions not only have a structural function, but also play a role in cell survival and apoptosis when the intercellular contacts weaken (24).
The inhibition of N-cadherin in chick embryos induces the formation of ependymal rosettes and periventricular abnormalities (25, 26, 84). Human studies in control and spine bifida aperta (SBA) embryos found that loss of N-cadherin leads to ependymal denudation, formation of pseudorosettes and macrophage invasion. Further, a concomitant loss of the gap junction protein connexin 43 was noted in some of these samples (27), and while other junctional proteins were noted, small rosettes did not express N-cadherin (27). Sival et al. (27) and Guerra et al. (28) hypothesized that the abnormal subcellular localization of N-cadherin and connexin 43 may be explained by altered intracellular trafficking, with intracytoplasmic buildup of both proteins. This would result in a defect in cell-to-cell adhesion, ependymal denudation and aqueductal obstruction with ventriculomegaly (27, 28).
E-cadherin is another central component of Ca2+-mediated cell-to-cell adhesion, and plays a role in ependymal development and integrity by regulating neural stem cell proliferation (85). Several intracellular pathways are involved in mediating E-cadherin expression. Among others, Numb-mediated signaling appears to be involved in cell border E-cadherin localization during ependymal maturation, and its disruption leads to epithelial damage via Notch-mediated signaling. VEGF seems to also play a role in ventriculomegaly, as shown by several studies (86–88). Interestingly, part of this effect might be due to the reduction in E-cadherin expression and ependymal denudation induced by VEGF administration. These findings are reversed with anti-VEGF antibody use (89).
Several studies have suggested a role for intracellular vesicular trafficking in determining junctional integrity. Failure of cadherin delivery to the cell surface could therefore explain ependymal denudation and hydrocephalus in rodent models.
Dlg5, a protein involved in t-SNARE-dependent movement of vesicles to the cell membrane, is thought to affect delivery of cadherin-catenin adhesion complexes and cellular orientation. Further, this protein appears to play a central role in epithelial tube maintenance in kidney and brain development. With loss of cell polarity, hydrocephalus and renal cysts may develop, as shown in Gld5-/- mice by Nechiporuk et al. (29).
αSNAP is another protein involved in membrane fusion events, thought to be responsible for the hydrocephalus with hop gait (hyh) phenotype. Mice homozygous for this deletion develop hydrocephalus with 100% penetrance, as well as ependymal damage and denudation (56). Point mutations in αSNAP appear to be sufficient to induce ventricular denudation and hydrocephalus in this model (38). Further, animals carrying mutations in SNAP-receptor (SNARE) mediated vesicle fusion system exhibit defects in apical protein localization, as well as errors in cell fate determination in neuroepithelial cells, with consequent cortical neuronal depletion (90). Chae et al. (90) described the role of αSnap mutation in hyh mice. This trafficking intracellular protein is suspected to be responsible for the movement of N-cadherin to the cell membrane. An alteration in this process leads to N-cadherin failure with collapse of the membrane and ependymal integrity.
Jimenez et al. (30) have shown that ependymal denudation precedes and triggers the development of obstructive hydrocephalus in a hyh mutant mouse model. Two phases are noted in these animals. First, ependymal cells detach from the ventricular wall, and a communicating form of hydrocephalus is seen. In a second phase, aqueductal obstruction ensues, usually post-partum and after the ependymal lining has been cleared. This led the authors to hypothesize a role for a bulk flow mechanisms at the brain interstitium-CSF interface, potentiated by ependymal denudation secondary to failure of adherens junctions (30). Paez et al. (31) demonstrated that different degrees of ependymal denudation are present in the brain, with the cingular and frontal cortices being the most affected regions. Noticeably, these alterations developed at stages when hydrocephalus was not yet evident, indicating that abnormal cortical development and hydrocephalus are concomitant and explained by a similar mechanism (31). Evidence suggests that astrocytes can replenish the denuded areas, taking on some ependymal traits such as aquaporin and connexin 43 expression, re-establishing a number of lost functions at the brain-CSF interphase (32).
IIIG9, a protein restricted to ependymal cells located in the apical cytoplasm and cilia and associated to cadherin/β-catenin-positive junctions, was also studied and characterized in relation to hydrocephalus in rodents. Baeza et al. showed ependymal denudation and balloon-like morphology of ependymal cells, as well as loss of cell polarity, after IIIG9 (PPP1R32) deletion (33).
Other proteins associated with adherens junction formation and thought to have a role in VZ disruption, hydrocephalus and brain dysplasia are Lgl1 (34), atypical protein kinase C-lambda (aPKCλ) (35) and non-muscle myosin II-B (NMII-B) (36).
Gap junctions are considered as channels that structurally interconnect ependymal elements, as well as waterways to exchange signaling molecules between neighboring cells (91–93). Connexin 43 (Cx43) in particular is thought to interact with several intracellular cascades including kinases, phosphatases, membrane receptors, cell signaling and scaffolding proteins (91). Recent evidence has shown that gap and tight junction protein expression is regulated by connexins, and co-immunoprecipitation is frequently observed (94–98). Connexins can form hemichannels that appear to be involved in cellular regulation, as well as Ca2+ mediated toxicity, cell proliferation and response to injury. Recent evidence has shown their involvement in several neurodegenerative pathologies such as Parkinson's disease, glioma, cerebral ischemia, spinal cord injury and chronic pain (99).
A close relationship has been found between gap and adherens junction formation in epithelia throughout the body (100–106). N-cadherin and Cx43 in particular appear to co-localize to the cell membrane, and abnormalities in one usually reflect in alterations in the other's behavior and functionality (104, 107).
While initial evidence suggested that the role of gap junctions was primarily that of facilitating ciliary movement, synchronizing their beating and therefore CSF movement, recent evidence hypothesized a broader role for these connecting junctions. Cx43 helps integrating the ependymal layer with the underlying glia, possibly regulating water and ion transport across these layers and into the interstitium and circulation (58). The abundance of gap junctions in the ventricular ependyma, glia limitans and surrounding neurons suggests the existence of a functional panglial syncytium that regulates brain-CSF interactions across broad regions of the neuraxis (58).
Gap junctions may play a major role in synchronizing the beating of ependymal cilia (108–110) and directing CSF flow in the lateral ventricle and down the cerebral aqueduct (111–113) in several mammalian and non-mammalian models of CSF circulation. Further, dysfunctional cilia have been associated with communicating ventriculomegaly, despite a thorough understanding of the underlying pathophysiological mechanism is still lacking (112).
Bouille et al. first described the existence of gap junctions in the ependymal layer in long term primary cultures derived from fetal mouse or rat (40). Different cell types were injected with Lucifer Yellow CH and analyzed with freeze-fracture. The capacity to transfer dye was very different based on cell types. Direct communication via gap junctions was observed among hypothalamic ependymocytes, plexal and non-plexal ependymocytes from the diencephalic roof. On the other hand, in astrocytes found in the same primary cultures, no GJIC was observed in spite of the expression of welldifferentiated gap junctions (40). This suggests a prominent functional role for gap junctions in the ependymal layer, a role that is frequently lost in hydrocephalic states (32).
Roales-Bujan et al. (32) demonstrated that wild-type and hyh-mutant mouse ependymal cells express connexin 43. This protein is usually located in supranuclear granules and slender patches in the lateral plasma membrane, connecting nearby cells. Further, apical localization of connexins was confirmed in this study, showing a belt-like distribution in the top third of normal ependyma, and significant disruption of this layout in hyh animals. As mentioned in a previous section, this was coupled with an overall decrease in N-cadherin expression and failure of intracellular transport machinery. HRP percolation into the surrounding brain parenchyma was comparable between the two groups, but its overall magnitude appeared to be somewhat controlled and regulated by the ependymal layer and the glial substitutes. Electron microscopy studies of the ependymal interdigitations showed the presence of adherens and gap junctions, while tight junctions were missing. Intraventricular injections of lanthanum penetrated through the extracellular space, filling the intercellular space of the neuropile and reached the intercellular space up to the tight junctions joining endothelial cells. Finally, after ependymal denudation, it was noticed that the reactive astrocytic layer contained Cx43 granules throughout the cytoplasm, especially in older astrocytic layers (P30 mice compared to P6) (32).
Connexin-26 was recently found to be associated with resumption of ependymal proliferation in the spinal central canal after spinal cord injury and reduction of connexin hemichannel activity. These findings were reversed by connexin inhibition (39).
Growing evidence suggests a prominent role for disruption of the ventricular (VZ) and subventricular (SVZ) zones during embryonic life in hydrocephalus development. Cells in the VZ and SVZ give origin to most cells of the mammalian brain, including ependymal cells. These cells are part of the pseudostratified neuroepithelium that constitutes the VZ, a transient embryonic layer that contains multipotent radial glia/stem cells. Neural stem cells (NSCs) start differentiating during the fourth through the 22nd week of gestation (114), and are bathed by primordial, protein- and solute-rich CSF during early development. Recent works have focused on the junctional changes that ependymocytes undergo during the switch from early embryonic to neonatal and adult life. Until embryonic day 12 (E12) these cells are connected by gap, adherens and tight junctions (14, 52, 53). From E12 onward, tight junctions are missing or limited to the apical segments of ependymocytes, and cell-to-cell adhesion relies mainly on gap and adherens junctions. Further, a change in claudin expression, and therefore tight junction composition, was reported by several groups. These changes affect not only the movement of interstitial fluid to and from the brain parenchyma, but also the passage of solutes such as proteins and ions across these compartments.
CSF dynamics have been studied with intraventricular, subarachnoid or lumbar injection of fluorescent and radiographic dyes for a long time. In the 1950s, studies carried out using radioactive water showed that at least part of the CSF secretion was not related to the choroid plexus (115–117). Using a rabbit model Pollay and Curl concluded that almost 30% of the total CSF secretion may come from the ependymal layer (118), while Cserr et al. showed by injecting tracers into the parenchyma that the ependymal contribution is ~1% (119).
However, in the last decade studies were able to optimize the use of fluorescent dyes for in vivo tracing of solute movement. Whish et al. (14) provided the first evidence of limited membrane permeability during early development, reporting an extensive change in junctional protein expression in peri- and post-natal stages and an overall increase in transependymal flow with age.
This work also suggests that ependymal permeability may be at the basis of several pathological states. Variations in CSF secretion or reabsorption can affect ventricular volume and brain function, as well as the parenchymal concentration of proteins and electrolytes. By highlighting the role of specific proteins such as Claudin-11 and−5, this group provided preliminary evidence that junctional complexes, previously thought to play a minor role in ependymal permeability, can profoundly affect CSF dynamics. Further, mRNA sequencing data was able to show that the evolution in junctional expression is paired with a direct alteration in the size of tracers able to diffuse across this barrier. This suggests that the ependyma may be a central part of the CSF secretion/absorption equation in certain phases of our lives. It also suggests that by changing the type of junctional claudin, the ependymal layer could function as a route for fluid and substance clearance.
Hypothetically, these findings suggest that paracellular junctional complexes could be modulated in order to increase or decrease the transependymal flow of water, solutes and medications. Importantly, this may constitute an additional avenue for treatment of hydrocephalus and other conditions affecting the CNS. By inhibiting certain isoforms such as claudin-5 or -11, it might be possible to impair their barrier function, prompting a more sustained centrifugal movement of water toward the interstitial compartment. This would build an additional pathway of reabsorption that is common to other mammals, but not particularly well developed in humans. On the other hand, inhibition of claudin-2, a paracellular channel for water and Na+ ions, could bolster fluid retention in the ventricular compartment. Finally, this lining can function as a potential route for passage of intraventricular chemo- or biological therapies. Drugs could be administered locally, and by in vivo modification of tight and adherens junctions clinicians could promote or limit the movement between these compartments.
Another important implication of intercellular junctional complexes lays in its role in ependymal denudation and obstructive hydrocephalus. Several rodent hydrocephalus models converge on this type of tissue damage, despite different mutations leading to the same effect. Most of these mutations involve genes responsible for the formation of gap and adherens junctions, or intracellular trafficking of their components. Among others, Chae, Batiz and Ferland focused on the intracellular movement of E-cadherin and β-catenin in hyh-dependent hydrocephalus (38, 54, 90), while Nechiporuk et al. suggested that Dlg5 knockout determines a deficiency in membrane assembly of cadherin-catenin complexes, leading to loss of ependymal cell polarity (29). Similarly, Rasin et al. (120) showed that Numb and NumbI inactivation disrupts intracellular transport of certain components of adherens junctions (120). Loss of adherens junction integrity was also described by Imai et al. after knockout of aPCKl in a mouse model, with derangement of ependymal cytoarchitecture (35). Klezovitch reported the onset of ventriculomegaly in animals with homozygous deletion of Lgl-1. This protein has been implicated in maintenance of cell polarity in Drosophila and mammalian cultures, and it is frequently mutated in epithelial malignancies, with loss of cell polarity and formation of pseudorosettes. Similarly, hyperproliferation and loss of polarity is seen in neuroependymal cells, with consequent development of hydrocephalus (34). Finally, Ma et al. reported ependymal collapse and aqueductal obstruction after deletion of Nonmuscle Myosin II-B in mice (36). Interestingly, increasing the local expression of this protein restored ependymal structure and function, improving CSF drainage (36).
Other studies, not directly connected to the brain ependyma, also suggest a remarkable interconnection between these proteins. ZO-1, a tight and adherens junction-associated element that links to the actin cytoskeleton, is thought to interact with Cx43 in forming gap junction plaques (121). Specifically, a failure of ZO-1 assembly results in their combined collapse. Interestingly, lack of cadherin assembly also impairs claudin and occludin formation (122).
Overall, emerging evidence suggests a central function for ependymal tight, adherens and gap junctions, and a profound interconnection that only a few years ago could not even be hypothesized. These studies show that mutations in several structural and non-structural genes result in ependymal collapse and denudation. An error in one of these proteins will likely lead to failure of the intercellular junctional system.
Another important change shared by several hydrocephalus models is the development of significant ventriculomegaly before the onset of ependymal denudation. While structural failure of the intercellular junctional proteins was thought to be a necessary element for development of obstructive hydrocephalus, the presence of a communicating form suggests that the ependymal layer is involved in CSF distribution and reabsorption in different ways. It not only preserves patency of the cerebral ventricles and aqueducts, but also allows the passage of fluids and solutes between the brain and CSF spaces.
Given the observation that the underlying glia attempts to re-form a layer similar to the native ependyma, one would think that physical ependymal disruption alters the balance of fluids and solutes across this interface. We hypothesize that three separate mechanisms are implicated in this form of hydrocephalus in mouse models and humans: (1) cerebral aqueduct and subarachnoid space obstruction caused by ependymal that takes place around day 12 of embryonic life might impair CSF movement via the paravascular route and dural granulations; (2) abnormal function or absence of multiciliated ependymocytes might curtail the flow of CSF along the ventricles, aqueduct, subarachnoid spaces; (3) given the contribution of the ependyma to fluid transfer with the brain interstitium and its evolution during embryonal life, we hypothesize that ependymal loss may result in abnormal movement of CSF and water from the brain parenchyma to the cerebral ventricles.
It is known that embryonal CSF has a significant concentration of proteins and solutes, and that ependymal tight junctions are expressed at high levels. Any damage to the neuroependyma could therefore result in interstitial fluid transfer toward the ventricles by means of osmosis, explaining certain forms of transient communicating hydrocephalus seen in models of embryonal junctional derangement.
In conclusion, (Figure 3, Stage 2) in these models a two-stage mechanism could be at the basis of the switch from communicating to obstructive hydrocephalus. The first form ensues when osmotic centripetal movement of fluids from the interstitium takes place, while obstructive hydrocephalus is a mere consequence of the final collapse of ependymal walls with obstruction of the cerebral aqueduct and Pacchioni's granulations (Figure 3, Stage 3).
Figure 3. Progression of ependymal failure and development of hydrocephalus. (1) normal ependyma with intact junctions, solute/CSF interchange is maintained. (2) junctional damage with increased transependymal flow toward the ventricles represented by yellow arrows and water particles penetrating into the ependymal layer. This could be caused by an osmotic gradient secondary to high CSF protein concentration. A form of communicating hydrocephalus may develop at this stage. (3) failure and collapse of the ependymal layer, with denudation of the ventricular wall and aqueductal/subarachnoid occlusion. This mechanism could lead to obstructive hydrocephalus. Green arrows represent ependymocytes detaching from the ependymal basement membrane and causing ventricular denudation. Trans-ependymal flow of water and solutes is at this point uncontrolled, leading to additional ventricular enlargement, further ependymal distension and damage.
The ependymal lining, one of the main interfaces between the brain and CSF systems, has long been considered an inert and permeable layer of epithelial cells with almost no barrier function. However, recent studies suggested that this interface undergoes several changes during in utero and postnatal life, mainly secondary to variable expression of different paracellular junctional proteins. By harnessing the characteristics of these complexes, it may be possible to understand several forms of congenital communicating and obstructive hydrocephalus. Further, differential expression of junctional proteins could lead to selective modulation of the ependymal permeability, allowing for its use as an avenue for CSF redistribution, as well as a local site of drug administration.
The original contributions presented in the study are included in the article/supplementary material, further inquiries can be directed to the corresponding author.
RS and JS designed the study together. RS wrote the first draft of the manuscript and prepared the figures. JS contributed to and edited the final draft. Both authors approved the manuscript.
The authors declare that the research was conducted in the absence of any commercial or financial relationships that could be construed as a potential conflict of interest.
All claims expressed in this article are solely those of the authors and do not necessarily represent those of their affiliated organizations, or those of the publisher, the editors and the reviewers. Any product that may be evaluated in this article, or claim that may be made by its manufacturer, is not guaranteed or endorsed by the publisher.
1. Dandy WE, Blackfan KD. An experimental and clinical study of internal hydrocephalus. J Am Med Assoc. (1913) 61:2216–7. doi: 10.1001/jama.1913.04350260014006
2. Dandy WE, Blackfan KD. Internal hydrocephalus: second paper. Am J Dis Child. (1917) 14:424–43. doi: 10.1001/archpedi.1917.01910120029002
3. Reeves BC, Karimy JK, Kundishora AJ, Mestre H, Cerci HM, Matouk C, et al. Glymphatic system impairment in Alzheimer's disease and idiopathic normal pressure hydrocephalus. Trends Mol Med. (2020) 26:285–95. doi: 10.1016/j.molmed.2019.11.008
4. Tennyson VM, Pappas GD. An electron microscope study of ependymal cells of the fetal, early postnatal and adult rabbit. Zeitschrift für Zellforschung und mikroskopische Anatomie. (1962) 56:595–618. doi: 10.1007/BF00540584
5. Duckett S. The germinal layer of the growing human brain during early fetal life. Anat Rec. (1968) 161:231–45. doi: 10.1002/ar.1091610208
6. Fossan G, Cavanagh M, Evans C, Malinowska D, Møllgård K, Reynolds M, et al. CSF-brain permeability in the immature sheep fetus: a CSF-brain barrier. Development Brain Res. (1985) 18:113–24. doi: 10.1016/0165-3806(85)90255-X
7. Møllgård K, Balslev Y, Lauritzen B, Saunders NR. Cell junctions and membrane specializations in the ventricular zone (germinal matrix) of the developing sheep brain: a CSF-brain barrier. J Neurocytol. (1987) 16:433–44. doi: 10.1007/BF01668498
8. Brightman M, Reese T. Junctions between intimately apposed cell membranes in the vertebrate brain. J Cell Biol. (1969) 40:648–77. doi: 10.1083/jcb.40.3.648
9. Cavanagh M, Warren A. The distribution of native albumin and foreign albumin injected into lateral ventricles of prenatal and neonatal rat forebrains. Anat Embryol. (1985) 172:345–51. doi: 10.1007/BF00318983
10. Dziegielewska K, Evans C, Lai P, Lorscheider F, Malinowska D, Møllgrd K, et al. Proteins in cerebrospinal fluid and plasma of fetal rats during development. Dev Biol. (1981) 83:193–200. doi: 10.1016/S0012-1606(81)80024-3
11. Mack A, Neuhaus J, Wolburg H. Relationship between orthogonal arrays of particles and tight junctions as demonstrated in cells of the ventricular wall of the rat brain. Cell Tissue Res. (1987) 248:619–25. doi: 10.1007/BF00216492
12. Gotow T, Hashimoto PH. Intercellular junctions between specialized ependymal cells in the subcommissural organ of the rat. J Neurocytol. (1982) 11:363–79. doi: 10.1007/BF01257983
13. Rieke G, Wynder H, Jordan F, Thomas W. Ultrastructure of ependymal cells in primary cultures of cerebral cortex. J Neurosci Res. (1987) 18:484–92. doi: 10.1002/jnr.490180316
14. Whish S, Dziegielewska KM, Møllgård K, Noor NM, Liddelow SA, Habgood MD, et al. The inner CSF–brain barrier: developmentally controlled access to the brain via intercellular junctions. Front Neurosci. (2015) 9:16. doi: 10.3389/fnins.2015.00016
15. Mochida GH, Ganesh VS, Felie JM, Gleason D, Hill RS, Clapham KR, et al. A homozygous mutation in the tight-junction protein JAM3 causes hemorrhagic destruction of the brain, subependymal calcification, and congenital cataracts. Am J Hum Genetics. (2010) 87:882–9. doi: 10.1016/j.ajhg.2010.10.026
16. Drielsma A, Jalas C, Simonis N, Désir J, Simanovsky N, Pirson I, et al. Two novel CCDC88C mutations confirm the role of DAPLE in autosomal recessive congenital hydrocephalus. J Med Genet. (2012) 49:708–12. doi: 10.1136/jmedgenet-2012-101190
17. Saugier-Veber P, Marguet F, Lecoquierre F, Adle-Biassette H, Guimiot F, Cipriani S, et al. Hydrocephalus due to multiple ependymal malformations is caused by mutations in the MPDZ gene. Acta Neuropathologica Commun. (2017) 5:1–12. doi: 10.1186/s40478-017-0438-4
18. Feldner A, Adam MG, Tetzlaff F, Moll I, Komljenovic D, Sahm F, et al. Loss of Mpdz impairs ependymal cell integrity leading to perinatal-onset hydrocephalus in mice. EMBO Mol Med. (2017) 9:890–905. doi: 10.15252/emmm.201606430
19. Yang J, Simonneau C, Kilker R, Oakley L, Byrne MD, Nichtova Z, et al. Murine MPDZ-linked hydrocephalus is caused by hyperpermeability of the choroid plexus. EMBO Mol Med. (2019) 11:e9540. doi: 10.15252/emmm.201809540
20. Petrov T, Howarth AG, Krukoff TL, Stevenson BR. Distribution of the tight junction-associated protein ZO-1 in circumventricular organs of the CNS. Mol Brain Res. (1994) 21:235–46. doi: 10.1016/0169-328X(94)90254-2
21. Kang HS, Ahn HS, Kang HJ, Gye MC. Effect of estrogen on the expression of occludin in ovariectomized mouse brain. Neurosci Lett. (2006) 402:30–4. doi: 10.1016/j.neulet.2006.03.052
22. Steinemann A, Galm I, Chip S, Nitsch C, Maly IP. Claudin-1,-2 and-3 are selectively expressed in the epithelia of the choroid plexus of the mouse from early development and into adulthood while claudin-5 is restricted to endothelial cells. Front Neuroanat. (2016) 10:16. doi: 10.3389/fnana.2016.00016
23. Alvarez JI, Teale JM. Differential changes in junctional complex proteins suggest the ependymal lining as the main source of leukocyte infiltration into ventricles in murine neurocysticercosis. J Neuroimmunol. (2007) 187:102–13. doi: 10.1016/j.jneuroim.2007.05.005
24. Oliver C, González CA, Alvial G, Flores CA, Rodríguez EM, Bátiz LF. Disruption of CDH2/N-cadherin-based adherens junctions leads to apoptosis of ependymal cells and denudation of brain ventricular walls. J Neuropathol Experiment Neurol. (2013) 72:846–60. doi: 10.1097/NEN.0b013e3182a2d5fe
25. Hatta K, Takagi S, Fujisawa H, Takeichi M. Spatial and temporal expression pattern of N-cadherin cell adhesion molecules correlated with morphogenetic processes of chicken embryos. Dev Biol. (1987) 120:215–27. doi: 10.1016/0012-1606(87)90119-9
26. Gänzler-Odenthal SI, Redies C. Blocking N-cadherin function disrupts the epithelial structure of differentiating neural tissue in the embryonic chicken brain. J Neurosci. (1998) 18:5415–25. doi: 10.1523/JNEUROSCI.18-14-05415.1998
27. Sival DA, Guerra M, den Dunnen WFA, Bátiz LF, Alvial G, Castañeyra-Perdomo A, et al. Neuroependymal denudation is in progress in full-term human foetal spina bifida aperta. Brain Pathol. (2011) 21:163–79. doi: 10.1111/j.1750-3639.2010.00432.x
28. Guerra M, Sival D, Jimenez A, Dominguez Pinos MD, den Dunnen W, Bátiz LF, et al. Defects in cell-cell junctions lead to neuroepithelial/ependymal denudation in the telencephalon of human hydrocephalic foetuses. Cerebrospinal Fluid Res. (2010). 7:1. doi: 10.1186/1743-8454-7-S1-S56
29. Nechiporuk T, Fernandez TE, Vasioukhin V. Failure of epithelial tube maintenance causes hydrocephalus and renal cysts in Dlg5–/– mice. Dev Cell. (2007) 13:338–50. doi: 10.1016/j.devcel.2007.07.017
30. Jiménez A, Tomé M, Páez P, Wagner C, Rodríguez S, Fernández-Llebrez P, et al. A programmed ependymal denudation precedes congenital hydrocephalus in the hyh mutant mouse. J Neuropathol Experiment Neurol. (2001) 60:1105–19. doi: 10.1093/jnen/60.11.1105
31. Páez P, Bátiz L-F, Roales-Buján R, Rodríguez-Pérez L-M, Rodríguez S, Jiménez AJ, et al. Patterned neuropathologic events occurring in hyh congenital hydrocephalic mutant mice. J Neuropathol Experiment Neurol. (2007) 66:1082–92. doi: 10.1097/nen.0b013e31815c1952
32. Roales-Buján R, Páez P, Guerra M, Rodríguez S, Vío K, Ho-Plagaro A, et al. Astrocytes acquire morphological and functional characteristics of ependymal cells following disruption of ependyma in hydrocephalus. Acta Neuropathol. (2012) 124:531–46. doi: 10.1007/s00401-012-0992-6
33. Baeza V, Cifuentes M, Martínez F, Ramírez E, Nualart F, Ferrada L, et al. IIIG9 inhibition in adult ependymal cells changes adherens junctions structure and induces cellular detachment. Sci Rep. (2021) 11:1–16. doi: 10.1038/s41598-021-97948-3
34. Klezovitch O, Fernandez TE, Tapscott SJ, Vasioukhin V. Loss of cell polarity causes severe brain dysplasia in Lgl1 knockout mice. Genes Dev. (2004) 18:559–71. doi: 10.1101/gad.1178004
35. Imai F, Hirai S-i, Akimoto K, Koyama H, Miyata T, Ogawa M, et al. Inactivation of aPKCλ results in the loss of adherens junctions in neuroepithelial cells without affecting neurogenesis in mouse neocortex. Genes Dev. (2006) 6:2389. doi: 10.1242/dev.02389
36. Ma X, Bao J, Adelstein RS. Loss of cell adhesion causes hydrocephalus in nonmuscle myosin II-B–ablated and mutated mice. Mol Biol Cell. (2007) 18:2305–12. doi: 10.1091/mbc.e07-01-0073
37. Tullio AN, Bridgman PC, Tresser NJ, Chan CC, Conti MA, Adelstein RS, et al. Structural abnormalities develop in the brain after ablation of the gene encoding nonmuscle myosin II-B heavy chain. J Comparat Neurol. (2001) 433:62–74. doi: 10.1002/cne.1125
38. Bátiz LF, Páez P, Jiménez AJ, Rodríguez S, Wagner C, Pérez-Fígares JM, et al. Heterogeneous expression of hydrocephalic phenotype in the hyh mice carrying a point mutation in α-SNAP. Neurobiol Dis. (2006) 23:152–68. doi: 10.1016/j.nbd.2006.02.009
39. Fabbiani G, Reali C, Valentín-Kahan A, Rehermann MI, Fagetti J, Falco MV, et al. Connexin signaling is involved in the reactivation of a latent stem cell niche after spinal cord injury. J Neurosci. (2020) 40:2246–58. doi: 10.1523/JNEUROSCI.2056-19.2020
40. Bouillé C, Mesnil M, Barriere H, Gabrion J. Gap junctional intercellular communication between cultured ependymal cells, revealed by lucifer yellow CH transfer and freeze-fracture. Glia. (1991) 4:25–36. doi: 10.1002/glia.440040104
41. Page MJ, McKenzie JE, Bossuyt PM, Boutron I, Hoffmann TC, Mulrow CD, et al. The PRISMA 2020 statement: an updated guideline for reporting systematic reviews. Syst Rev. (2021) 10:1–11. doi: 10.1186/s13643-021-01626-4
42. Engelhardt B, Liebner S. Novel insights into the development and maintenance of the blood–brain barrier. Cell Tissue Res. (2014) 355:687–99. doi: 10.1007/s00441-014-1811-2
43. Daneman R, Prat A. The blood–brain barrier. Cold Spring Harb Perspect Biol. (2015) 7:a020412. doi: 10.1101/cshperspect.a020412
44. Arvanitis CD, Ferraro GB, Jain RK. The blood–brain barrier and blood–tumour barrier in brain tumours and metastases. Nat Rev Cancer. (2020) 20:26–41. doi: 10.1038/s41568-019-0205-x
45. Profaci CP, Munji RN, Pulido RS, Daneman R. The blood–brain barrier in health and disease: Important unanswered questions. J Experiment Med. (2020) 217:62. doi: 10.1084/jem.20190062
46. Solár P, Zamani A, Kubíčková L, Dubový P, Joukal M. Choroid plexus and the blood–cerebrospinal fluid barrier in disease. Fluids Barriers CNS. (2020) 17:1–29. doi: 10.1186/s12987-020-00196-2
47. Levitt P, Cooper ML, Rakic P. Coexistence of neuronal and glial precursor cells in the cerebral ventricular zone of the fetal monkey: an ultrastructural immunoperoxidase analysis. J Neurosci. (1981) 1:27–39. doi: 10.1523/JNEUROSCI.01-01-00027.1981
48. Altafulla J, Bordes S, Jenkins S, Litvack Z, Iwanaga J, Loukas M, et al. The basal subarachnoid cisterns: surgical and anatomical considerations. World Neurosurg. (2019) 129:190–9. doi: 10.1016/j.wneu.2019.05.087
49. Lippoldt A, Jansson A, Kniesel U, Andbjer B, Andersson A, Wolburg H, et al. Phorbol ester induced changes in tight and adherens junctions in the choroid plexus epithelium and in the ependyma. Brain Res. (2000) 854:197–206. doi: 10.1016/S0006-8993(99)02355-0
50. Kuchler S, Graff M-N, Gobaille S, Vincendon G, Roche A-C, Delaunoy J-P, et al. Mannose dependent tightening of the rat ependymal cell barrier. In vivo and in vitro study using neoglycoproteins. Neurochemistr Int. (1994) 24:43–55. doi: 10.1016/0197-0186(94)90128-7
51. Møllgård K, Saunders N. The development of the human blood-brain and blood-CSF barriers. Neuropathol Appl Neurobiol. (1986) 12:337–58. doi: 10.1111/j.1365-2990.1986.tb00146.x
52. Mori T, Buffo A, Götz M. The novel roles of glial cells revisited: the contribution of radial glia and astrocytes to neurogenesis. Curr Top Dev Biol. (2005) 69:67–99. doi: 10.1016/S0070-2153(05)69004-7
53. Kazanis I, Lathia J, Moss L. The neural stem cell microenvironment. StemBook [Internet]. (2008).
54. Ferland RJ, Batiz LF, Neal J, Lian G, Bundock E, Lu J, et al. Disruption of neural progenitors along the ventricular and subventricular zones in periventricular heterotopia. Hum Mol Genet. (2009) 18:497–516. doi: 10.1093/hmg/ddn377
55. Kamiguchi H, Hlavin ML, Lemmon V. Role of L1 in neural development: what the knockouts tell us. Mol Cell Neurosci. (1998) 12:48–55. doi: 10.1006/mcne.1998.0702
56. Bátiz LF, Roales-Bujan R, Rodríguez-Pérez LM, Matas IM, Páez P, Roque M, et al. A simple PCR-based genotyping method for M105I mutation of alpha-SNAP enhances the study of early pathological changes in hyh phenotype. Mol Cell Probes. (2009) 23:281–90. doi: 10.1016/j.mcp.2009.07.002
57. Söhl G, Odermatt B, Maxeiner S, Degen J, Willecke K. New insights into the expression and function of neural connexins with transgenic mouse mutants. Brain Res Rev. (2004) 47:245–59. doi: 10.1016/j.brainresrev.2004.05.006
58. Rash JE, Duffy HS, Dudek FE, Bilhartz BL, Whalen LR, Yasumura T. Grid-mapped freeze-fracture analysis of gap junctions in gray and white matter of adult rat central nervous system, with evidence for a “panglial syncytium” that is not coupled to neurons. J Comparat Neurol. (1997) 388:265–92.
59. Dziegielewska K, Evans C, Malinowska D, Møllgård K, Reynolds J, Reynolds M, et al. Studies of the development of brain barrier systems to lipid insoluble molecules in fetal sheep. J Physiol. (1979) 292:207–31. doi: 10.1113/jphysiol.1979.sp012847
60. Dziegielewska K, Evans C, Malinowska D, Møllgård K, Reynolds M, Saunders N. Blood—cerebrospinal fluid transfer of plasma proteins during fetal development in the sheep. J Physiol. (1980) 300:457–65. doi: 10.1113/jphysiol.1980.sp013172
61. Dziegielewska K, Habgood M, Jones S, Reader M, Saunders N. Proteins in cerebrospinal fluid and plasma of postnatal Monodelphis domestica (grey short-tailed oposum). Comparat Biochemistr Physiol Part B: Comparat Biochemistr. (1989) 92:569–76. doi: 10.1016/0305-0491(89)90133-8
62. Dziegielewska K, Hinds L, Sarantis M, Saunders N, Tyndale-Biscoe C. Proteins in cerebrospinal fluid and plasma of the pouch young tammar wallaby (Macropus eugenii) during development. Comparat Biochemistr Physiol Part B: Comparat Biochemistr. (1986) 83:561–7. doi: 10.1016/0305-0491(86)90297-X
63. Dziegielewska KM, Saunders NR. The ins and outs of brain-barrier mechanisms. Trends Neurosci. (2002) 25:69–71. doi: 10.1016/S0166-2236(02)02068-4
64. Dziegielewska KM, Saunders NR. The development of the blood-brain barrier: proteins in fetal and neonatal CSF, their nature and origins. Handbook Hum Growth Development Biol. (1988) 1:169–91.
65. Adinolfi M. The development of the human blood-CSF-brain barrier. Dev Med Child Neurol. (1985) 27:532–7. doi: 10.1111/j.1469-8749.1985.tb04581.x
66. Stolp HB, Turnquist C, Dziegielewska KM, Saunders NR, Anthony DC, Molnar Z. Reduced ventricular proliferation in the foetal cortex following maternal inflammation in the mouse. Brain. (2011) 134:3236–48. doi: 10.1093/brain/awr237
67. Desmond ME, Jacobson AG. Embryonic brain enlargement requires cerebrospinal fluid pressure. Dev Biol. (1977) 57:188–98. doi: 10.1016/0012-1606(77)90364-5
68. Stolp HB, Liddelow SA, Sá-Pereira I, Dziegielewska KM, Saunders NR. Immune responses at brain barriers and implications for brain development and neurological function in later life. Front Integr Neurosci. (2013) 7:61. doi: 10.3389/fnint.2013.00061
69. Steed E, Balda MS, Matter K. Dynamics and functions of tight junctions. Trends Cell Biol. (2010) 20:142–9. doi: 10.1016/j.tcb.2009.12.002
70. de Bellis M, Cibelli A, Mola MG, Pisani F, Barile B, Mastrodonato M, et al. Orthogonal arrays of particle assembly are essential for normal aquaporin-4 expression level in the brain. Glia. (2021) 69:473–88. doi: 10.1002/glia.23909
71. Wolburg H, Wolburg-Buchholz K, Fallier-Becker P, Noell S, Mack AF. Structure and functions of aquaporin-4-based orthogonal arrays of particles. Int Rev Cell Mol Biol. (2011) 287:1–41. doi: 10.1016/B978-0-12-386043-9.00001-3
72. Feldman GJ, Mullin JM, Ryan MP. Occludin: structure, function and regulation. Adv Drug Deliv Rev. (2005) 57:883–917. doi: 10.1016/j.addr.2005.01.009
73. Du D, Xu F, Yu L, Zhang C, Lu X, Yuan H, et al. The tight junction protein, occludin, regulates the directional migration of epithelial cells. Dev Cell. (2010) 18:52–63. doi: 10.1016/j.devcel.2009.12.008
74. O'Driscoll MC, Daly SB, Urquhart JE, Black GC, Pilz DT, Brockmann K, et al. Recessive mutations in the gene encoding the tight junction protein occludin cause band-like calcification with simplified gyration and polymicrogyria. Am J Hum Genetics. (2010) 87:354–64. doi: 10.1016/j.ajhg.2010.07.012
75. Mandell KJ, Parkos CA. The JAM family of proteins. Adv Drug Deliv Rev. (2005) 57:857–67. doi: 10.1016/j.addr.2005.01.005
76. Marivin A, Garcia-Marcos M. DAPLE and MPDZ bind to each other and cooperate to promote apical cell constriction. Mol Biol Cell. (2019) 30:1900–10. doi: 10.1091/mbc.E19-02-0091
77. Itoh M, Furuse M, Morita K, Kubota K, Saitou M, Tsukita S. Direct binding of three tight junction-associated MAGUKs, ZO-1, ZO-2, and ZO-3, with the COOH termini of claudins. J Cell Biol. (1999) 147:1351–63. doi: 10.1083/jcb.147.6.1351
78. Ebnet K, Schulz CU. zu Brickwedde M-KM, Pendl GG, Vestweber D. Junctional adhesion molecule interacts with the PDZ domain-containing proteins AF-6 and ZO-1. J Biologic Chemistr. (2000) 275:27979–88. doi: 10.1074/jbc.M002363200
79. Fanning AS, Ma TY, Anderson JM. Isolation and functional characterization of the actin-binding region in the tight junction protein ZO-1. The FASEB J. (2002) 16:1–23. doi: 10.1096/fj.02-0121fje
80. Rohde V, Rohde I, Thiex R, Ince A, Jung A, Dückers G, et al. Fibrinolysis therapy achieved with tissue plasminogen activator and aspiration of the liquefied clot after experimental intracerebral hemorrhage: rapid reduction in hematoma volume but intensification of delayed edema formation. J Neurosurg. (2002) 97:954–62. doi: 10.3171/jns.2002.97.4.0954
81. Rajasekaran AK, Hojo M, Huima T, Rodriguez-Boulan E. Catenins and zonula occludens-1 form a complex during early stages in the assembly of tight junctions. J Cell Biol. (1996) 132:451–63. doi: 10.1083/jcb.132.3.451
82. Ooshio T, Kobayashi R, Ikeda W, Miyata M, Fukumoto Y, Matsuzawa N, et al. Involvement of the interaction of afadin with ZO-1 in the formation of tight junctions in Madin-Darby canine kidney cells. J Biol Chem. (2010) 285:5003–12. doi: 10.1074/jbc.M109.043760
83. Itoh M, Nagafuchi A, Moroi S, Tsukita S. Involvement of ZO-1 in cadherin-based cell adhesion through its direct binding to α catenin and actin filaments. J Cell Biol. (1997) 138:181–92. doi: 10.1083/jcb.138.1.181
84. Chenn A, Zhang YA, Chang BT, McConnell SK. Intrinsic polarity of mammalian neuroepithelial cells. Mol Cell Neurosci. (1998) 11:183–93. doi: 10.1006/mcne.1998.0680
85. Karpowicz P, Willaime-Morawek S, Balenci L, DeVeale B, Inoue T, van der Kooy D. E-Cadherin regulates neural stem cell self-renewal. J Neurosci. (2009) 29:3885–96. doi: 10.1523/JNEUROSCI.0037-09.2009
86. Lien W-H, Klezovitch O, Fernandez TE, Delrow J, Vasioukhin V. αE-catenin controls cerebral cortical size by regulating the hedgehog signaling pathway. Science. (2006) 311:1609–12. doi: 10.1126/science.1121449
87. Del Bigio MR, Slobodian I, Schellenberg AE, Buist RJ, Kemp-Buors TL. Magnetic resonance imaging indicators of blood-brain barrier and brain water changes in young rats with kaolin-induced hydrocephalus. Fluids Barriers CNS. (2011) 8:1–13. doi: 10.1186/2045-8118-8-22
88. Dombrowski SM, Deshpande A, Dingwall C, Leichliter A, Leibson Z, Luciano MG. Chronic hydrocephalus–induced hypoxia: Increased expression of VEGFR-2+ and blood vessel density in hippocampus. Neuroscience. (2008) 152:346–59. doi: 10.1016/j.neuroscience.2007.11.049
89. Shim JW, Sandlund J, Han CH, Hameed MQ, Connors S, Klagsbrun M, et al. VEGF, which is elevated in the CSF of patients with hydrocephalus, causes ventriculomegaly and ependymal changes in rats. Exp Neurol. (2013) 247:703–9. doi: 10.1016/j.expneurol.2013.03.011
90. Chae TH, Kim S, Marz KE, Hanson PI, Walsh CA. The hyh mutation uncovers roles for αSnap in apical protein localization and control of neural cell fate. Nat Genet. (2004) 36:264–70. doi: 10.1038/ng1302
91. Dbouk HA, Mroue RM, El-Sabban ME, Talhouk RS. Connexins: a myriad of functions extending beyond assembly of gap junction channels. Cell Commun Signal. (2009) 7:1–17. doi: 10.1186/1478-811X-7-4
92. Sáez JC, Berthoud VM, Branes MC, Martinez AD, Beyer EC. Plasma membrane channels formed by connexins: their regulation and functions. Physiol Rev. (2003) 83:1359–400. doi: 10.1152/physrev.00007.2003
93. Sáez JC, Retamal MA, Basilio D, Bukauskas FF, Bennett MV. Connexin-based gap junction hemichannels: gating mechanisms. Biochimica et Biophysica Acta (BBA)-Biomembranes. (2005) 1711:215–24. doi: 10.1016/j.bbamem.2005.01.014
94. Morita K, Furuse M, Fujimoto K, Tsukita S. Claudin multigene family encoding four-transmembrane domain protein components of tight junction strands. Proc Nat Acad Sci. (1999) 96:511–6. doi: 10.1073/pnas.96.2.511
95. Kojima T, Kokai Y, Chiba H, Yamamoto M, Mochizuki Y, Sawada N. Cx32 but not Cx26 is associated with tight junctions in primary cultures of rat hepatocytes. Exp Cell Res. (2001) 263:193–201. doi: 10.1006/excr.2000.5103
96. Kojima T, Spray DC, Kokai Y, Chiba H, Mochizuki Y, Sawada N. Cx32 formation and/or Cx32-mediated intercellular communication induces expression and function of tight junctions in hepatocytic cell line. Exp Cell Res. (2002) 276:40–51. doi: 10.1006/excr.2002.5511
97. Nagasawa K, Chiba H, Fujita H, Kojima T, Saito T, Endo T, et al. Possible involvement of gap junctions in the barrier function of tight junctions of brain and lung endothelial cells. J Cell Physiol. (2006) 208:123–32. doi: 10.1002/jcp.20647
98. Giepmans BN. Gap junctions and connexin-interacting proteins. Cardiovasc Res. (2004) 62:233–45. doi: 10.1016/j.cardiores.2003.12.009
99. Xing L, Yang T, Cui S, Chen G. Connexin hemichannels in astrocytes: Role in CNS disorders. Front Mol Neurosci. (2019) 12:23. doi: 10.3389/fnmol.2019.00023
100. Jongen W, Fitzgerald DJ, Asamoto M, Piccoli C, Slaga TJ, Gros D, et al. Regulation of connexin 43-mediated gap junctional intercellular communication by Ca2+ in mouse epidermal cells is controlled by E-cadherin. J Cell Biol. (1991) 114:545–55. doi: 10.1083/jcb.114.3.545
101. Fujimoto K, Nagafuchi A, Tsukita S, Kuraoka A, Ohokuma A, Shibata Y. Dynamics of connexins, E-cadherin and alpha-catenin on cell membranes during gap junction formation. J Cell Sci. (1997) 110:311–22. doi: 10.1242/jcs.110.3.311
102. Meyer RA, Laird DW, Revel J-P, Johnson RG. Inhibition of gap junction and adherens junction assembly by connexin and A-CAM antibodies. J Cell Biol. (1992) 119:179–89. doi: 10.1083/jcb.119.1.179
103. Oka C, Matsuda H, Sarai N, Noma A. Modeling the calcium gate of cardiac gap junction channel. J Physiologic Sci. (2006) 2006:0602240007. doi: 10.2170/physiolsci.R2139
104. Wei C-J, Francis R, Xu X, Lo CW. Connexin43 associated with an N-cadherin-containing multiprotein complex is required for gap junction formation in NIH3T3 cells. J Biol Chem. (2005) 280:19925–36. doi: 10.1074/jbc.M412921200
105. Laird DW. Life cycle of connexins in health and disease. Biochem J. (2006) 394:527–43. doi: 10.1042/BJ20051922
106. Derangeon M, Bozon V, Defamie N, Peineau N, Bourmeyster N, Sarrouilhe D, et al. 5-HT4 and 5-HT2 receptors antagonistically influence gap junctional coupling between rat auricular myocytes. J Mol Cell Cardiol. (2010) 48:220–9. doi: 10.1016/j.yjmcc.2009.07.005
107. Govindarajan R, Chakraborty S, Johnson KE, Falk MM, Wheelock MJ, Johnson KR, et al. Assembly of connexin43 into gap junctions is regulated differentially by E-cadherin and N-cadherin in rat liver epithelial cells. Mol Biol Cell. (2010) 21:4089–107. doi: 10.1091/mbc.e10-05-0403
108. Goodenough DA, Goliger JA, Paul DL. Connexins, connexons, and intercellular communication. Annu Rev Biochem. (1996) 65:475–502. doi: 10.1146/annurev.bi.65.070196.002355
109. Velazquez JLP, Carlen PL. Gap junctions, synchrony and seizures. Trends Neurosci. (2000) 23:68–74. doi: 10.1016/S0166-2236(99)01497-6
110. Rouach N, Avignone E, Meme W, Koulakoff A, Venance L, Blomstrand F, et al. Gap junctions and connexin expression in the normal and pathological central nervous system. Biol Cell. (2002) 94:457–75. doi: 10.1016/S0248-4900(02)00016-3
111. Banizs B, Pike MM, Millican CL, Ferguson WB, Komlosi P, Sheetz J, et al. Dysfunctional cilia lead to altered ependyma and choroid plexus function, and result in the formation of hydrocephalus. Development. (2005) 132, 5329-39. doi: 10.1242/dev.02153
112. Swiderski RE, Agassandian K, Ross JL, Bugge K, Cassell MD, Yeaman C. Structural defects in cilia of the choroid plexus, subfornical organ and ventricular ependyma are associated with ventriculomegaly. Fluids Barriers CNS. (2012) 9:1–13. doi: 10.1186/2045-8118-9-22
113. Dur A, Tang T, Viviano S, Sekuri A, Willsey H, Tagare H, et al. In Xenopus ependymal cilia drive embryonic CSF circulation and brain development independently of cardiac pulsatile forces. Fluids Barriers CNS. (2020) 17:1–22. doi: 10.1186/s12987-020-00234-z
114. Sarnat HB. Role of human fetal ependyma. Pediatr Neurol. (1992) 8:163–78. doi: 10.1016/0887-8994(92)90063-5
115. Bering JR EA. Cerebrospinal fluid production and its relationship to cerebral metabolism and cerebral blood flow. Am J Physiol Legacy Content. (1959) 197:825–8. doi: 10.1152/ajplegacy.1959.197.4.825
116. Bering EA, Sato O. Hydrocephalus: changes in formation and absorption of cerebrospinal fluid within the cerebral ventricles. J Neurosurg. (1963) 20:1050–63. doi: 10.3171/jns.1963.20.12.1050
117. Weed LH. The development of the cerebro-spinal space in pig and in man. Contributions in embryology. (1917).
118. Pollay M, Curl F. Secretion of cerebrospinal fluid by the ventricular ependyma of the rabbit. Am J Physiol Legacy Content. (1967) 213:1031–8. doi: 10.1152/ajplegacy.1967.213.4.1031
119. Cserr HF. Role of secretion and bulk flow of brain interstitial fluid in brain volume regulation. Ann N Y Acad Sci. (1988) 529:9–20. doi: 10.1111/j.1749-6632.1988.tb51415.x
120. Rašin M-R, Gazula V-R, Breunig JJ, Kwan KY, Johnson MB, Liu-Chen S, et al. Numb and Numbl are required for maintenance of cadherin-based adhesion and polarity of neural progenitors. Nat Neurosci. (2007) 10:819–27. doi: 10.1038/nn1924
121. Falk MM, Bell CL, Kells Andrews RM, Murray SA. Molecular mechanisms regulating formation, trafficking and processing of annular gap junctions. BMC Cell Biol. (2016) 17:5–27. doi: 10.1186/s12860-016-0087-7
Keywords: ependyma, tight junctions, adherens junctions, gap junctions, hydrocephalus
Citation: Serra R and Simard JM (2023) Adherens, tight, and gap junctions in ependymal cells: A systematic review of their contribution to CSF-brain barrier. Front. Neurol. 14:1092205. doi: 10.3389/fneur.2023.1092205
Received: 07 November 2022; Accepted: 27 February 2023;
Published: 24 March 2023.
Edited by:
Andrew Hale, University of Alabama at Birmingham, United StatesReviewed by:
Marek Joukal, Masaryk University, CzechiaCopyright © 2023 Serra and Simard. This is an open-access article distributed under the terms of the Creative Commons Attribution License (CC BY). The use, distribution or reproduction in other forums is permitted, provided the original author(s) and the copyright owner(s) are credited and that the original publication in this journal is cited, in accordance with accepted academic practice. No use, distribution or reproduction is permitted which does not comply with these terms.
*Correspondence: Riccardo Serra, cnNlcnJhQHNvbS51bWFyeWxhbmQuZWR1
Disclaimer: All claims expressed in this article are solely those of the authors and do not necessarily represent those of their affiliated organizations, or those of the publisher, the editors and the reviewers. Any product that may be evaluated in this article or claim that may be made by its manufacturer is not guaranteed or endorsed by the publisher.
Research integrity at Frontiers
Learn more about the work of our research integrity team to safeguard the quality of each article we publish.