- 1Department of Health Science and Technology, Center for Neuroplasticity and Pain, Faculty of Medicine, Aalborg University, Aalborg, Denmark
- 2Space Medicine Team, European Astronaut Centre, Cologne, Germany
- 3KBR GmbH, Cologne, Germany
- 4Centre of Human and Applied Physiological Sciences, King's College London, London, United Kingdom
Human movement is optimized to Earth's gravity and based on highly complex interactions between sensory and neuro-muscular systems. Yet, humans are able to adapt—at least partially—to extreme environments upon and beyond Earth's surface. With upcoming Lunar Gateway and Artemis missions, it is crucial to increase our understanding of the impact of hypogravity—i.e., reduced vertical loading—on physiological and sensory-motor performances to improve countermeasure programs, and define crewmember's readiness to perform mission critical tasks. Several methodologies designed to reduce vertical loading are used to simulate hypogravity on Earth, including body weight support (BWS) devices. Countering gravity and offloading the human body is also used in various rehabilitation scenarios to improve motor recovery in neurological and orthopedic impairments. Thus, BWS-devices have the potential of advancing theory and practice of both space exploration and terrestrial rehabilitation by improving our understanding of physiological and sensory-motor adaptations to reduced vertical loading and sensory input. However, lack of standardization of BWS-related research protocols and reporting hinders the exchange of key findings and new advancements in both areas. The aim of this introduction paper is to review the role of BWS in understanding human movement in simulated hypogravity and the use of BWS in terrestrial rehabilitation, and to identify relevant research areas contributing to the optimization of human spaceflight and terrestrial rehabilitation. One of the main aims of this research topic is to facilitate standardization of hypogravity-related research protocols and outcome reporting, aimed at optimizing knowledge transfer between space research and BWS-related rehabilitation sciences.
1. Introduction
Human bi-pedal locomotion, upright postural control and movement have adapted for performance in Earth's gravity (1g) based on complex interactions between the sensory and neuro-muscular systems (1, 2). Adaptation is extremely dynamic as humans are able to (at least partially) adapt to novel sensory and functional conditions due to impairment, such as with transtibial amputation (3) or stroke (4), in addition to environmental changes, such as load carrying (5) or walking on uneven surfaces (6). Human movement has also been shown to adapt to microgravity (μg), although such changes may present issues post-flight as modulation of sensori-motor (7, 8) and upright postural control (9) can persist for a number of days following the return to Earth's gravity (10, 11).
Whilst images of Apollo astronauts “hopping” across the Lunar surface are in the public consciousness, little is known about the transition from 1 g or μg to hypogravity (e.g., Moon: 0.16 g, Mars: 0.38 g) (11, 12). This is critical as the upcoming Lunar Gateway (a crewed space station orbiting the Moon) and Artemis missions (missions designed to land humans on the Moon) will mean that crewmembers will transition to Lunar gravity, potentially after prolonged exposure to μg (13). Even with limited exposure to μg (~3 days), it is reported that across the Apollo 11–17 missions, during a total of 78 h of Extra Vehicular Activities (EVAs), 23 falls, and 11 “near” falls were observed (14). The causes of Lunar instability are unknown, although novel and dynamic factors within the hypogravity environment presumably include the Lunar surface characteristics, the EVA suit (including the Portable Life Support System; PLSS) and the challenge of controlling the Center of Mass (CoM) with respect to the base of support (Center of Pressure) (15). Such factors are compounded by vestibular adaptations (16) leading to impairment of gross (i.e., postural control and locomotion) motor control, in addition to motion sickness and spatial disorientation (14). As a result, the risk of injury and/or fatality has been considered to be high on the Lunar surface (14, 17).
The Artemis missions may involve sustained (and potentially repeated) exposure to μg that may extend to many weeks/months (13). As a result, physiological adaptations associated with sustained exposure to μg may be induced, including musculoskeletal (18, 19) and cardiovascular deconditioning (20). Furthermore, changes in sensory-motor control (14) may reflect recently identified neuroplasticity (21, 22) including cortical reorganization (23). Thus, increasing the understanding of the impact of hypogravity (i.e., < 1 g; >μg) on physiological and sensory-motor function is critical to inform development of pre-flight, in-flight, and post-flight programs to facilitate mission appropriate adaptions. An improved understanding of the control of movement in hypogravity is also key to guide the development of Lunar (and Martian) EVA suit, habitat, and general operation ergonomics and both the need for, and the nature of exercise countermeasures beyond the ISS (24) that may require the definition of “required standards” in order to ensure a crewmember's readiness to perform mission critical tasks. Whilst such exercise countermeasures are yet to be defined, hypogravity hopping appears to be a prime candidate (25).
Both in preparation for and following the Apollo program, several ground-based methodologies have been used to simulate hypogravity. The vast majority of hypogravity locomotion research has employed gravity “compensation” or “offloading” systems such as vertical body weight support (BWS) (26), tilted BWS devices (27), supine suspension (28), or lower body positive pressure (LBPP) (29) although the apparent biomechanical and physiological effects of simulated hypogravity differ—suggesting methodological-specific factors (12, 30). “Offloading” has also been used as a clinical rehabilitation tool. Body weight support has been shown to enable physically or neurologically impaired individuals to start movement rehabilitation at an earlier stage following immobilization (e.g., trauma or surgery) or improve movement in patients affected by various neuro-muscular disorders, both by reducing weight-bearing, but also by providing balance support reducing both the real risk, and the fear of falling that can limit both the willingness of a patient to move (31, 32). Thus, the appropriate use of BWS has the potential to significantly contribute to the advancement of the theory and practice of human space exploration and terrestrial rehabilitation of physically and/or neurologically impaired individuals.
Considering the above, the aims of this paper are to: (1) Review the role of BWS in understanding human movement in simulated hypogravity; (2) Review the use of BWS in terrestrial rehabilitation; (3) Identify research areas which may contribute to the optimization of human spaceflight operations and terrestrial rehabilitation. Ultimately, all three aims facilitate a standardization of hypogravity research protocols and outcome reporting to optimize knowledge transfer between studies of both space research and BWS-related rehabilitation sciences.
2. Use of BWS in simulated hypogravity research
Simulating Lunar gravity is, in theory, fairly simple in that it requires the “unloading” of five-sixths of Earth's gravitational forces acting on the human body. Hewes and Spady (33), in preparation for the Apollo program, developed a “Lunar Landing Walking Simulator” (Figure 1) based on the assumption that: (1) if body segments are constrained to move freely in only parallel planes, subjects should be able to perform tasks in a quasi-normal manner in the direction of the planes; and (2) movement of a body or object in an inclined plane with negligible friction is only controlled by the gravitational component of the plane. The Lunar Landing Walking Simulator required individuals to be suspended via suspension cables whilst being inclined on their side at 9.5° from the horizontal and standing on a similarly inclined walkway (33, 34). Thus, whilst individuals were free to perform any form of locomotion along the runway (sagittal plane), lateral or rotating movements were not possible. Despite this limitation, the Lunar Landing Walking Simulator provided to be practical and invaluable to prepare the Apollo crews for their Lunar surface activities by predicting that “hopping” would be an efficient locomotory strategy on the Lunar surface, and via estimating the metabolic cost of locomotion in Lunar gravity to determine the oxygen requirements of the Apollo PLSS for Lunar surface EVAs (33). However, the demise of the Apollo program led to the de-commissioning of the Lunar Landing Walking Simulator, which was complex to maintain, including the outdoor walkway and a dolly system mounted to a neighboring building. Subsequently, technological advances have resulted in a variety of hypogravity locomotion simulators being developed: ranging from “simple” (e.g., vertical BWS devices) to highly complex (e.g., 3D robotic BWS systems), each with specific advantages and limitations (12, 30).
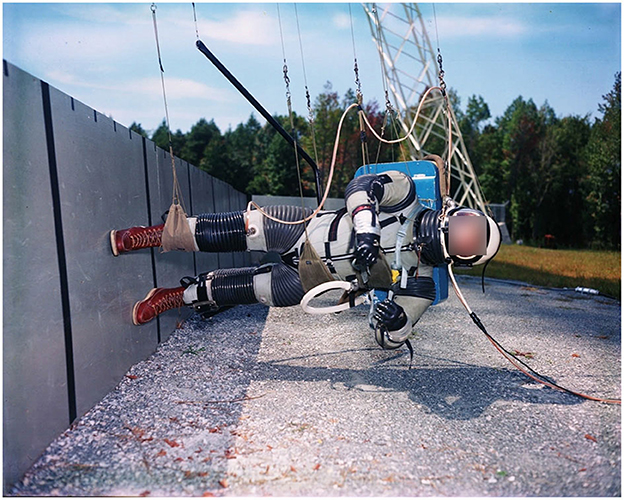
Figure 1. “Lunar Landing Walking Simulator” at NASA Langley Research Center (1965): Enabling researchers to study the ability to walk, run and perform other tasks required during Lunar exploration. Photo courtesy of NASA.
Hypogravity simulators reduce the force(s) acting on the body's CoM, affecting in particular the lower limbs, and significantly decreasing kinetic parameters (e.g., peak vertical ground reaction forces—vGRFs) which are critical for the generation of repetitive locomotor output (35, 36). Interestingly, even though peak vGRFs at simulated 0.05 g are 1/20th of those in 1 g and tend to be applied only through the forefoot rather than following the classic heel-to-toe transfer, the kinematics of the ankle, knee and hip joints remain remarkably similar, resulting in a preserved foot trajectory (shape and variability). In contrast, in the absence of contact forces during air-stepping at 100% BWS, inter-stride variability is significantly increased (12, 37). Thus, preservation of accurate lower limb kinematics and foot trajectory control appears possible in a wide range of gravity levels, including 0.05 g, as long as contact forces provide temporal signals that modulate the central pattern generators (CPGs) activity (36, 37).
However, with reduced external forces acting on the body, walking velocity, cadence and stance phase duration progressively decline and lower the rate of force development. Reductions of gravity-related mechanical load, reduce total external work (to move the body) and internal work (to move body segments) requirements, thereby reducing metabolic cost and thus cardiopulmonary demand [Figure 2; (30)]. Reductions of vGRF also reduce lower limb net joint moments and power during the stance phase (38). Reduced joint power also suggests attenuation of muscle “work” or activation, and indeed, BWS appears to result in—non-linear—decreases in knee extensor and ankle plantarflexion EMG activity during stance. Yet, knee flexor activity tended to increase during the stance phase, along with ankle dorsiflexors during the swing phase (38, 39).
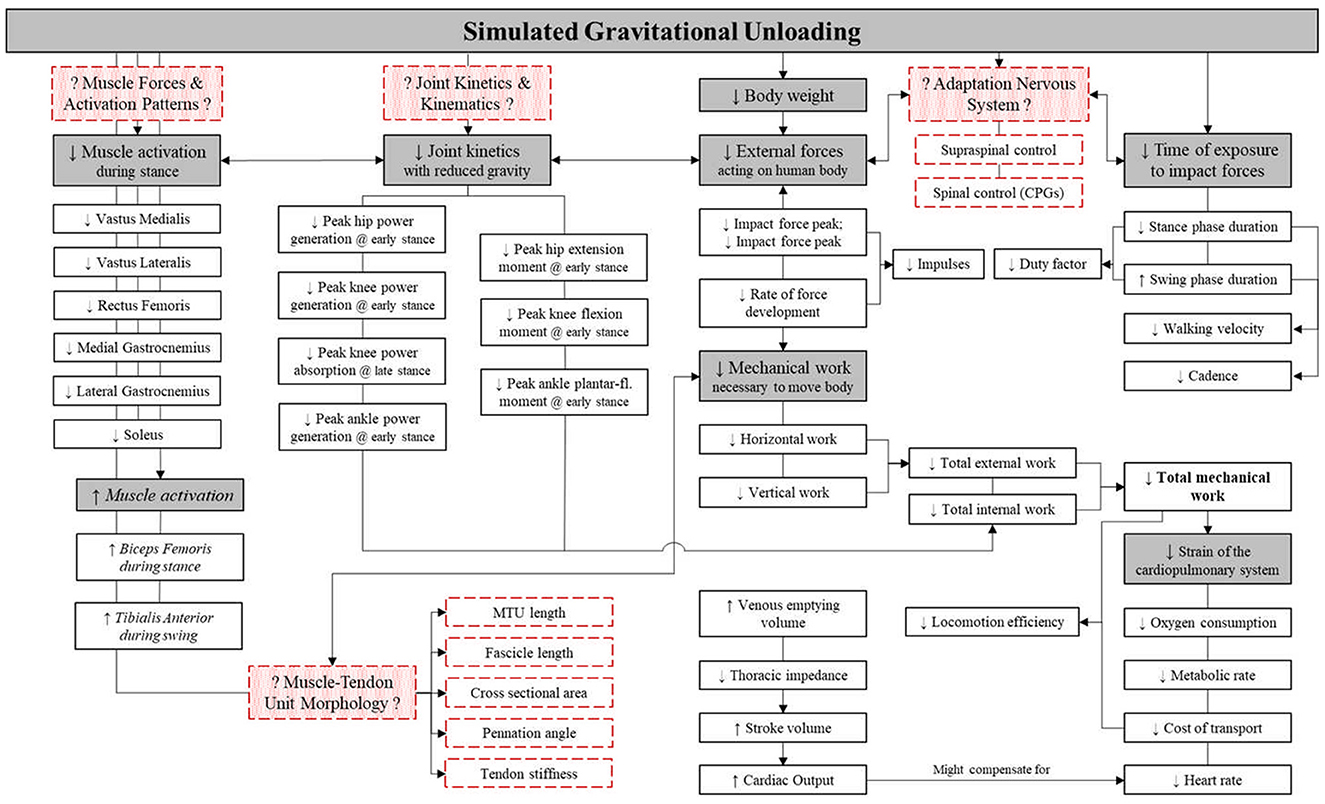
Figure 2. Schematic of the apparent effect of body weight support (BWS) upon lower limb muscle activation, whole body kinetics and kinematics and cardiorespiratory outcomes. Red boxes with dashed outline represent gaps in current knowledge to be filled. Gray boxes represent main physiological factors, with white boxes representing the underlying outcome parameters. Italics, trends; ↓, decrease; ↑, increase; CPG, central pattern generator; MTU, muscle-tendon unit. Figure adapted from Richter et al. (30).
However, muscle forces, joint angular velocities and joint torques, which are key to indicate internal work, have been understudied in hypogravity biomechanical research (30). Additionally, increasing the body of evidence of hypogravity research may also aid in the design of EVA suits, e.g., suit joint performance and behavior and PLSS oxygen requirements. Furthermore, outcomes related to the generation and transmission of forces across the muscle-tendon unit, such as muscle-tendon unit length, fascicle length, cross sectional area and pennation angles are critical to understand the internal kinetics of locomotion (40–42) and in particular strain, and strain rates that have been identified to be key in regulating musculoskeletal integrity (43). Such data is fundamental for modeling internal forces, to determine related de-conditioning risk of the musculoskeletal system, and to devise appropriate training and countermeasure programs, should they be needed. In addition, although modulation of activity of the primary sensory-motor areas has been reported to precede the loading and unloading of the lower limbs (44, 45), it is still unclear how reduction of external forces relates to involvement of supraspinal structures in the control of postural and lower limb musculature (46). Moreover, sensory feedback has been noted to be critical for the modulation and adaptation of CPG-generated motor output to environmental constraints, yet the role of primary afferent inputs in the control of bipedal locomotion is still only partially understood (47).
3. Use of BWS in terrestrial rehabilitation
The application of BWS in terrestrial rehabilitation was initially studied as an intervention to promote locomotion in spinal cord injury (SCI) patients (48). The core rationale for this approach (based on work in cordotomized cats that revealed marked locomotor improvements after a few weeks of BWS treadmill walking) was to stimulate spinal CPGs by generating cyclic locomotor patterns with reduced tonic postural contractions due to body weight support (49). Progressive reductions in BWS (starting from 60% BWS or greater) during locomotor training with incomplete SCI were employed as locomotor performance improved (50). However, whilst BWS training appears not to be superior to traditional physiotherapy and over-ground training in randomized clinical trials (51), those with the greatest impairment may particularly benefit (50, 52), presumably due to being able to perform locomotor activity without having to bear 100% body weight without risk of falling (52). More recently, BWS have been evaluated as a rehabilitation strategy to improve gait, posture and balance in patients with other neurological disorders, including stroke (53), Parkinson's disease (54), multiple sclerosis (55), and cerebral palsy (56). Whilst few well-designed, randomized clinical trials exist, BWS treadmill training is becoming increasingly popular in neurological rehabilitation in attempts to promote activity-dependent neuroplasticity. Locomotion requires the integration of descending cortical and subcortical control with CPG activity in the spinal cord along with modulatory afferent proprioceptive, mechanoreceptive and cutaneous stimuli (57).
BWS treadmill training facilitates a greater number of steps and thus task-specific stimuli within a training session. To date, there are some promising data using 30% BWS showing walking speed and endurance improvements in multiple sclerosis (55), and improvement of walking performance in Parkinson's disease (54). In contrast, BWS locomotor training (minimum 10% BWS) was not shown to be superior to progressive physiotherapist-guided home exercise in stroke patients (58) or children with cerebral palsy (56). Thus, definition of appropriate BWS-use in neurological rehabilitation remains a subject of debate (54, 59). In part this is due to a paucity of knowledge of BWS optimization (e.g., BWS methodology, BWS %, frequencies, durations, training intensities) with respect to pathological conditions (nature and severity of neurological damage) and patient-specific characteristics [e.g., age, sex, anthropometrics (including leg length)] (51, 53).
In recent years, BWS applications have been expanded to non-neurological disorders, including lower extremity injuries (60), back pain (61), and cardiac rehabilitation (29, 62). By reducing the % of body weight of such patients, locomotor training can be progressively increased to promote lower muscle strength, motor coordination, and cardiorespiratory function in respect to functionality (63). For instance, following lower limb surgery, a period of restricted weight-bearing is advised to allow surgical site healing, but attempts to ameliorate post-operative inactivity-induced results in muscular atrophy, joint stiffness, and increased thrombosis risk (64). Body weight supported walking by reducing internal joint kinetics, and muscle-tendon strain, and strain rates upon the skeletal system with minimal alteration of gait kinematics, may potentially enhance recovery following lower limb surgery or recovery of knee osteoarthritis patients, but optimal strategies remain to be determined (65). Regular 40% BWS walking has been shown to be a safe, user-friendly mode of exercise that can be used in the management of day-to-day joint symptoms associated with knee osteoarthritis (66). Furthermore, preliminary evidence suggests that BWS walking, starting from approximal 30% BWS and gradually reducing support, can promote the cardiovascular fitness, promoting autonomic regulation in patients with reduced mobility (62, 67). Finally, 40% BWS treadmill gait training promoted overground walking speed, even in healthy, older individuals (68) without increasing energy cost (69). In summary, whilst the use of BWS devices has shown potential in several rehabilitation settings, standardized randomized clinical trials are necessary to define optimal strategies (12, 70).
4. Bridging the gap between simulated hypogravity research and terrestrial rehabilitation
The use of BWS devices to reduce mechanical loading, thus mimicking exposure to a reduced gravitational loading—e.g., Lunar (0.16 g; 84% BWS) or Martian (0.38 g; 62% BWS) gravity—, is an invaluable tool for modeling adaptations crewmembers may experience during future surface exploration missions. However, the underlying biomechanical and neurophysiological mechanisms to these adaptations, as well as appropriate exercise countermeasures to counter and/or prevent maladaptation need to be investigated further. Importantly, the resulting findings also aid in enhancing terrestrial rehabilitation strategies in patients with various neuro-muscular and orthopedic disorders who may benefit from BWS locomotion training.
Due to a growing availability and great diversity in BWS devices, there is also a great variety of methodologies and conditions in which studies are performed. For example, the type of body weight unloading being used and related accuracy in mimicking the biomechanical and physiological effects of hypogravity, the amount of body weight unloading and corresponding simulated (hypo-)gravity level, or the mode of locomotion under investigation. In addition, the abundance of outcomes characterizing human movement complicates the comparison of results over different studies and drawing of general conclusions. Therefore, to ensure high quality and basic comparability between future studies, standardization of conditions used in BWS-related research, as well as determining a standard set of outcome measures to be used in future studies—as done for bed rest studies (71)—seems appropriate. Doing so enables greater scientific advancements, while also increasing the efficiency and added value of the scientific community's investment by ensuring a minimum set of standardized data are being reported by each study.
Thus, this Research Topic seeks to cover research areas aiding in the standardization and improvement of hypogravity-related research and training protocols, and reporting of data/outcomes. Relevant research areas include, but are not limited to:
• Improving the general understanding of biomechanical (e.g., spatiotemporal parameters, kinematics, kinetics) and neurophysiological adaptations (e.g., neuro-muscular activation, muscle-tendon unit behavior) related to BWS during different modes of locomotion (e.g., loping, skipping, running), movement (e.g., hopping, jumping) and % of body weight unloading;
• Improving our understanding of the association between BWS-induced reductions of external loading and changes in internal forces (e.g., forces and moments experienced at the joint and muscle);
• Improving our understanding of the interaction between supraspinal (e.g., corticomotor excitability) and spinal (e.g., CPGs) mechanisms during BWS locomotion.
5. Conclusion
The use of BWS devices is a valuable tool to increase our knowledge of biomechanical, physiological, and sensori-motor adaptations to partial body unloading. As a result, it has the potential to make important contributions to the optimization of spaceflight operations as well as terrestrial rehabilitation. Yet, current scientific contributions are heterogenous as protocols and reporting of data vary widely. Establishing guidelines for standardization of hypogravity-related research would greatly improve scientific advancements in both areas.
Author contributions
ED, DG, DC, TW, and NH: conceptualization, revision, and editing. ED and NH: writing first draft. All authors have read and agreed to the published version of the manuscript. All authors contributed to the article and approved the submitted version.
Funding
This work was supported by the European Space Agency (ESA).
Conflict of interest
DG and TW were employed by KBR GmbH, Cologne, Germany.
The remaining authors declare that the research was conducted in the absence of any commercial or financial relationships that could be construed as a potential conflict of interest.
Publisher's note
All claims expressed in this article are solely those of the authors and do not necessarily represent those of their affiliated organizations, or those of the publisher, the editors and the reviewers. Any product that may be evaluated in this article, or claim that may be made by its manufacturer, is not guaranteed or endorsed by the publisher.
References
1. Watkins J. Structure and Function of the Musculoskeletal System. 2nd ed. Leeds: Human Kinetics (2010).
2. Prochazka A, Ellaway P. Sensory systems in the control of movement. Compr Physiol. (2012) 2:2615–27. doi: 10.1002/cphy.c100086
3. Darter BJ, Bastian AJ, Wolf EJ, Husson EM, Labrecque BA, Hendershot BD. Locomotor adaptability in persons with unilateral transtibial amputation. PLoS ONE. (2017) 12:e0181120. doi: 10.1371/journal.pone.0181120
4. Preston E, Ada L, Stanton R, Mahendran N, Dean CM. Prediction of independent walking in people who are nonambulatory early after stroke: a systematic review. Stroke. (2021) 52:3217–24. doi: 10.1161/STROKEAHA.120.032345
5. Walsh GS, Low DC. Military load carriage effects on the gait of military personnel: a systematic review. Appl Ergon. (2021) 93:103376. doi: 10.1016/j.apergo.2021.103376
6. Luo Y, Coppola SM, Dixon PC, Li S, Dennerlein JT, Hu B. A database of human gait performance on irregular and uneven surfaces collected by wearable sensors. Sci Data. (2020) 7:219. doi: 10.1038/s41597-020-0563-y
7. Kornilova LN, Goncharenko AM, Bodo G, Elkan K, Grigorova V, Manev A. Pathogenesis of sensory disorders in microgravity. Physiologist. (1991) 34:S36–9.
8. Lipshits MI, Gurfinkel EV, Matsakis I, Lestén F. Effects of weightlessness on sensorimotor interaction in the operator'work: proprioceptive feedbacks. Aviakosmicheskaia Ekol Meditsina Aerosp Environ Med. (1993) 27:26–30.
9. Black FO, Paloski WH, Reschke MF, Igarashi M, Guedry F, Anderson DJ. Disruption of postural readaptation by inertial stimuli following space flight. J Vestib Res Equilib Orientat. (1999) 9:369–78. doi: 10.3233/VES-1999-9506
10. Mulavara AP, Feiveson AH, Fiedler J, Cohen H, Peters BT, Miller C, et al. Locomotor function after long-duration space flight: effects and motor learning during recovery. Exp Brain Res. (2010) 202:649–59. doi: 10.1007/s00221-010-2171-0
11. White O, Clément G, Fortrat J-O, Pavy-LeTraon A, Thonnard J-L, Blanc S, et al. Towards human exploration of space: the THESEUS review series on neurophysiology research priorities. Npj Microgravity. (2016) 2:16023. doi: 10.1038/npjmgrav.2016.23
12. Lacquaniti F, Ivanenko YP, Sylos-Labini F, La Scaleia V, La Scaleia B, Willems PA, et al. Human locomotion in hypogravity: from basic research to clinical applications. Front Physiol. (2017) 8:893. doi: 10.3389/fphys.2017.00893
13. NASA. NASA's Lunar Exploration Program Overview. (2020). Available online at: https://www.nasa.gov/sites/default/files/atoms/files/artemis_plan-20200921.pdf (accessed September 06, 2022).
14. Bloomberg JJ, Reschke, MF, Clément, GR, Mulavara, AP, Taylor, LC,. Risk of Impaired Control of Spacecraft/Associated Systems Decreased Mobility Due to Vestibular/Sensorimotor Alterations Associated With Space Flight. NASA (2016). Available online at: https://humanresearchroadmap.nasa.gov/evidence/reports/SM.pdf?rnd=0.435052425337514 (accessed June 13, 2022).
15. Kubis JF, Elrod JT, Rusnak R, Barnes JE. Apollo 15 Time And Motion Study. Final Report M72-4. Houston, TX: NASA Manned Spacecraft Center (1972).
16. Carriot J, Mackrous I, Cullen KE. Challenges to the vestibular system in space: how the brain responds and adapts to microgravity. Front Neural Circuits. (2021) 15:760313. doi: 10.3389/fncir.2021.760313
17. Chappell SP, Norcross, JR, Abercromby, AF, Bekdash, OS, Benson, EA, Jarvis, SL,. Risk of Injury Compromised Performance Due to EVA Operations. NASA (2017). Available online at: https://humanresearchroadmap.nasa.gov/evidence/reports/EVA.pdf?rnd=0.228920993036088 (accessed June 13, 2022).
18. Trappe S, Costill D, Gallagher P, Creer A, Peters JR, Evans H, et al. Exercise in space: human skeletal muscle after 6 months aboard the International Space Station. J Appl Physiol. (2009) 106:1159–68. doi: 10.1152/japplphysiol.91578.2008
19. Stavnichuk M, Mikolajewicz N, Corlett T, Morris M, Komarova SV. A systematic review and meta-analysis of bone loss in space travelers. Npj Microgravity. (2020) 6:13–13. doi: 10.1038/s41526-020-0103-2
20. Hargens AR, Richardson S. Cardiovascular adaptations, fluid shifts, and countermeasures related to space flight. Respir Physiol Neurobiol. (2009) 169:S30–3. doi: 10.1016/j.resp.2009.07.005
21. Van Ombergen A, Laureys S, Sunaert S, Tomilovskaya E, Parizel PM, Wuyts FL. Spaceflight-induced neuroplasticity in humans as measured by MRI: what do we know so far? Npj Microgravity. (2017) 3:2. doi: 10.1038/s41526-016-0010-8
22. Kramer LA, Hasan KM, Stenger MB, Sargsyan A, Laurie SS, Otto C, et al. Intracranial effects of microgravity: a prospective longitudinal MRI study. Radiology. (2020) 295:640–8. doi: 10.1148/radiol.2020191413
23. Demertzi A, Van Ombergen A, Tomilovskaya E, Jeurissen B, Pechenkova E, Di Perri C, et al. Cortical reorganization in an astronaut's brain after long-duration spaceflight. Brain Struct Funct. (2016) 221:2873–6. doi: 10.1007/s00429-015-1054-3
24. Scott JPR, Weber T, Green DA. Introduction to the frontiers research topic: optimization of exercise countermeasures for human space flight – lessons from terrestrial physiology and operational considerations. Front Physiol. (2019) 10:173. doi: 10.3389/fphys.2019.00173
25. Weber T, Green DA, Attias J, Sies W, Frechette A, Braunstein B, et al. Hopping in hypogravity—A rationale for a plyometric exercise countermeasure in planetary exploration missions. PLoS ONE. (2019) 14:e0211263. doi: 10.1371/journal.pone.0211263
26. Kram R, Domingo A, Ferris DP. Effect of reduced gravity on the preferred walk-run transition speed. J Exp Biol. (1997) 200:821–6. doi: 10.1242/jeb.200.4.821
27. Sylos-Labini F, Ivanenko YP, Cappellini G, Portone A, MacLellan MJ, Lacquaniti F. Changes of gait kinematics in different simulators of reduced gravity. J Mot Behav. (2013) 45:495–505. doi: 10.1080/00222895.2013.833080
28. De Witt JK, Perusek GP, Bentley J, Edwards WB, Gilkey KM, Lewandowski BE, et al. Kinematic and Electromyographic Evaluation of Locomotion on the Enhanced Zero-gravity Locomotion Simulator: A Comparison of External Loading Mechanisms. US National Aeronautics and Space Administration TP-2007-214764. Hanover, MD: NASA Center for AeroSpace Information (2008). p. 1–27.
29. Cutuk A, Groppo ER, Quigley EJ, White KW, Pedowitz RA, Hargens AR. Ambulation in simulated fractional gravity using lower body positive pressure: cardiovascular safety and gait analyses. J Appl Physiol. (2006) 101:771–7. doi: 10.1152/japplphysiol.00644.2005
30. Richter C, Braunstein B, Winnard A, Nasser M, Weber T. Human biomechanical and cardiopulmonary responses to partial gravity – a systematic review. Front Physiol. (2017) 8:583. doi: 10.3389/fphys.2017.00583
31. Sousa CO, Barela JA, Prado-Medeiros CL, Salvini TF, Barela AM. The use of body weight support on ground level: an alternative strategy for gait training of individuals with stroke. J NeuroEngineering Rehabil. (2009) 6:43. doi: 10.1186/1743-0003-6-43
32. Apte S, Plooij M, Vallery H. Influence of body weight unloading on human gait characteristics: a systematic review. J NeuroEngineering Rehabil. (2018) 15:53–53. doi: 10.1186/s12984-018-0380-0
33. Hewes DE, Spady AA. Evaluation of a Gravity-Simulation Technique for Studies of Man's Self-Locomotion in Lunar Environment. Technical Note D-2176 NASA Contract Rep NASA CR U S Natl Aeronaut Space Adm. Hampton, VA: Langley Research Center (1964). p. 1–34.
34. Hewes DE. Reduced-gravity simulators for studies of man's mobility in space and on the moon. Hum Fact J Hum Factors Ergon Soc. (1969) 11:419–31. doi: 10.1177/001872086901100502
35. Duysens J, Clarac F, Cruse H. Load-regulating mechanisms in gait and posture: comparative aspects. Physiol Rev. (2000) 80:83–133. doi: 10.1152/physrev.2000.80.1.83
36. Sylos-Labini F, Lacquaniti F, Ivanenko YP. Human locomotion under reduced gravity conditions: biomechanical and neurophysiological considerations. BioMed Res Int. (2014) 2014:1–12. doi: 10.1155/2014/547242
37. Ivanenko YP, Grasso R, Macellari V, Lacquaniti F. Control of foot trajectory in human locomotion: role of ground contact forces in simulated reduced gravity. J Neurophysiol. (2002) 87:3070–89. doi: 10.1152/jn.2002.87.6.3070
38. MacLean MK, Ferris DP. Human muscle activity and lower limb biomechanics of overground walking at varying levels of simulated reduced gravity and gait speeds. PLoS ONE. (2021) 16:e0253467. doi: 10.1371/journal.pone.0253467
39. Kristiansen M, Odderskær N, Kristensen DH. Effect of body weight support on muscle activation during walking on a lower body positive pressure treadmill. J Electromyogr Kinesiol. (2019) 48:9–16. doi: 10.1016/j.jelekin.2019.05.021
40. Richter C, Braunstein B, Staeudle B, Attias J, Suess A, Weber T, et al. Contractile behavior of the gastrocnemius medialis muscle during running in simulated hypogravity. Npj Microgravity. (2021) 7:32. doi: 10.1038/s41526-021-00155-7
41. Richter C, Braunstein B, Staeudle B, Attias J, Suess A, Weber T, et al. Gastrocnemius medialis contractile behavior during running differs between simulated Lunar and Martian gravities. Sci Rep. (2021) 11:22555. doi: 10.1038/s41598-021-00527-9
42. Richter C, Braunstein B, Staeudle B, Attias J, Suess A, Weber T, et al. Gastrocnemius medialis contractile behavior is preserved during 30% body weight supported gait training. Front Sports Act Living. (2021) 2. doi: 10.3389/fspor.2020.614559
43. Rittweger J. Ten years muscle-bone hypothesis: what have we learned so far?–almost a festschrift. J. Musculoskelet. Neuronal Interact. 8, 174–178.
44. Wieser M, Haefeli J, Bütler L, Jäncke L, Riener R, Koeneke S. Temporal and spatial patterns of cortical activation during assisted lower limb movement. Exp Brain Res. (2010) 203:181–91. doi: 10.1007/s00221-010-2223-5
45. Gwin JT, Gramann K, Makeig S, Ferris DP. Electrocortical activity is coupled to gait cycle phase during treadmill walking. Neuroimage. (2011) 54:1289–96. doi: 10.1016/j.neuroimage.2010.08.066
46. Jaeger L, Marchal-Crespo L, Wolf P, Luft AR, Riener R, Michels L, et al. On the modulation of brain activation during simulated weight bearing in supine gait-like stepping. Brain Topogr. (2016) 29:193–205. doi: 10.1007/s10548-015-0441-7
47. Guertin PA. Central pattern generator for locomotion: anatomical, physiological, and pathophysiological considerations. Front Neurol. (2013) 3:183. doi: 10.3389/fneur.2012.00183
48. Gardner MB, Holden MK, Leikauskas JM, Richard RL. Partial body weight support with treadmill locomotion to improve gait after incomplete spinal cord injury: a single-subject experimental design. Phys Ther. (1998) 78:361–74. doi: 10.1093/ptj/78.4.361
49. Smith JL, Smith LA, Zernicke RF, Hoy M. Locomotion in exercised and nonexercised cats cordotomized at two or twelve weeks of age. Exp Neurol. (1982) 76:393–413. doi: 10.1016/0014-4886(82)90217-5
50. Hicks AL, Adams MM, Martin Ginis K, Giangregorio L, Latimer A, Phillips SM, et al. Long-term body-weight-supported treadmill training and subsequent follow-up in persons with chronic SCI: effects on functional walking ability and measures of subjective well-being. Spinal Cord. (2005) 43:291–8. doi: 10.1038/sj.sc.3101710
51. Wessels M, Lucas C, Eriks I, de Groot S. Body weight-supported gait training for restoration of walking in people with an incomplete spinal cord injury : a systematic review. J Rehabil Med. (2010) 42:513–9. doi: 10.2340/16501977-0525
52. Field-Fote EC, Lindley SD, Sherman AL. Locomotor training approaches for individuals with spinal cord injury: a preliminary report of walking-related outcomes. J Neurol Phys Ther. (2005) 29:127–37. doi: 10.1097/01.NPT.0000282245.31158.09
53. Mehrholz J, Thomas S, Elsner B. Treadmill training and body weight support for walking after stroke. Cochr Database Syst Rev. (2017). doi: 10.1002/14651858.CD002840.pub4
54. Lorenzo-García P, Cavero-Redondo I, Torres-Costoso AI, Guzmán-Pavón MJ, Núñez de Arenas-Arroyo S, Álvarez-Bueno C. Body weight support gait training for patients with Parkinson disease: a systematic review and meta-analyses. Arch. Phys. Med. Rehabil. (2021) 102:2012–21. doi: 10.1016/j.apmr.2021.02.016
55. Swinnen E, Beckwée D, Pinte D, Meeusen R, Baeyens J-P, Kerckhofs E. Treadmill training in multiple sclerosis: can body weight support or robot assistance provide added value? A systematic review. Mult Scler Int. (2012) 2012:1–15. doi: 10.1155/2012/240274
56. Willoughby KL, Dodd KJ, Shields N, Foley S. Efficacy of partial body weight–supported treadmill training compared with overground walking practice for children with cerebral palsy: a randomized controlled trial. Arch Phys Med Rehabil. (2010) 91:333–9. doi: 10.1016/j.apmr.2009.10.029
57. Dunlop SA. Activity-dependent plasticity: implications for recovery after spinal cord injury. Trends Neurosci. (2008) 31:410–8. doi: 10.1016/j.tins.2008.05.004
58. Duncan PW, Sullivan KJ, Behrman AL, Azen SP, Wu SS, Nadeau SE, et al. Body-weight–supported treadmill rehabilitation after stroke. N Engl J Med. (2011) 364:2026–36. doi: 10.1056/NEJMoa1010790
59. Mehrholz J, Harvey LA, Thomas S, Elsner B. Is body-weight-supported treadmill training or robotic-assisted gait training superior to overground gait training and other forms of physiotherapy in people with spinal cord injury? A systematic review. Spinal Cord. (2017) 55:722–9. doi: 10.1038/sc.2017.31
60. Greenwood R, Ellison J, Gleeson P, Mitchell K. Reliability of pain scores during a body weight support protocol in individuals with knee osteoarthritis. Disabil Rehabil. (2021) 1–6. doi: 10.1080/09638288.2021.2017028
61. Joffe D, Watkins M, Steiner L, Pfeifer BA. Treadmill ambulation with partial body weight support for the treatment of low back and leg pain. J Orthop Sports Phys Ther. (2002) 32:202–15. doi: 10.2519/jospt.2002.32.5.202
62. MacKay-Lyons M, McDonald A, Matheson J, Eskes G, Klus M-A. Dual effects of body-weight supported treadmill training on cardiovascular fitness and walking ability early after stroke: a randomized controlled trial. Neurorehabil Neural Repair. (2013) 27:644–53. doi: 10.1177/1545968313484809
63. Webber SC, Horvey KJ, Yurach Pikaluk MT, Butcher SJ. Cardiovascular responses in older adults with total knee arthroplasty at rest and with exercise on a positive pressure treadmill. Eur J Appl Physiol. (2014) 114:653–62. doi: 10.1007/s00421-013-2798-1
64. Healy WL, Della Valle CJ, Iorio R, Berend KR, Cushner FD, Dalury DF, et al. Complications of total knee arthroplasty: standardized list and definitions of the knee society. Clin Orthop. (2013) 471:215–20. doi: 10.1007/s11999-012-2489-y
65. Peeler J, Leiter J, MacDonald P. Effect of Body Weight–Supported Exercise on Symptoms of Knee Osteoarthritis: A Follow-up Investigation. Clin. J. Sport Med. (2020) 30:e178–85. doi: 10.1097/JSM.0000000000000668
66. Peeler J, Christian M, Cooper J, Leiter J, MacDonald P. Managing knee osteoarthritis: the effects of body weight supported physical activity on joint pain, function, and thigh muscle strength. Clin J Sport Med. (2015) 25:518–23. doi: 10.1097/JSM.0000000000000173
67. Ditor DS, Kamath MV, MacDonald MJ, Bugaresti J, McCartney N, Hicks AL. Effects of body weight-supported treadmill training on heart rate variability and blood pressure variability in individuals with spinal cord injury. J Appl Physiol. (2005) 98:1519–25. doi: 10.1152/japplphysiol.01004.2004
68. Thomas EE, Vito GD, Macaluso A. Speed training with body weight unloading improves walking energy cost and maximal speed in 75- to 85-year-old healthy women. J Appl Physiol. (2007) 103:1598–603. doi: 10.1152/japplphysiol.00399.2007
69. Thomas EE, Stewart D, Mitchell S, Aiken K, Farina D, Macaluso A. Comparison of neural activation and energy cost during treadmill walking with body weight unloading between frail and healthy older women. Gait Post. (2011) 33:356–60. doi: 10.1016/j.gaitpost.2010.12.001
70. Bishnoi A, Lee R, Hu Y, Mahoney JR, Hernandez ME. Effect of treadmill training interventions on spatiotemporal gait parameters in older adults with neurological disorders: systematic review and meta-analysis of randomized controlled trials. Int J Environ Res Public Health. (2022) 19:2824. doi: 10.3390/ijerph19052824
71. Sundblad P, Orlov, O,. (eds.). Guidelines for Standardization of Bed Rest Studies in the Spaceflight Context. International Academy of Astronautics, (2014). Available online at: https://www.nasgov/sites/default/files/atoms/files/bed_rest_studies_complete.pdf (accessed July 18, 2022).
Keywords: hypogravity, body weight support, neurorehabilitation, orthopedic rehabilitation, spaceflight, exercise, reconditioning
Citation: De Martino E, Green DA, Ciampi de Andrade D, Weber T and Herssens N (2023) Human movement in simulated hypogravity—Bridging the gap between space research and terrestrial rehabilitation. Front. Neurol. 14:1062349. doi: 10.3389/fneur.2023.1062349
Received: 05 October 2022; Accepted: 18 January 2023;
Published: 06 February 2023.
Edited by:
Jean Blouin, CNRS, FranceReviewed by:
Marc-Antoine Custaud, Université d'Angers, FranceCopyright © 2023 De Martino, Green, Ciampi de Andrade, Weber and Herssens. This is an open-access article distributed under the terms of the Creative Commons Attribution License (CC BY). The use, distribution or reproduction in other forums is permitted, provided the original author(s) and the copyright owner(s) are credited and that the original publication in this journal is cited, in accordance with accepted academic practice. No use, distribution or reproduction is permitted which does not comply with these terms.
*Correspondence: Nolan Herssens, bm9sYW4uaGVyc3NlbnNAZXNhLmludA==