- 1Department of Neurology, The Second Affiliated Hospital, The Second Clinical Medical College, Fujian Medical University, Quanzhou, China
- 2Prenatal Diagnosis Center, Quanzhou Women's and Children's Hospital, Quanzhou, China
- 3Department of Neurosurgery, The Second Affiliated Hospital, The Second Clinical Medical College, Fujian Medical University, Quanzhou, China
- 4Centre of Neurological and Metabolic Research, The Second Affiliated Hospital of Fujian Medical University, Quanzhou, China
- 5Diabetes and Metabolism Division, Garvan Institute of Medical Research, Darlinghurst, NSW, Australia
Parkinson's disease (PD) has become the second largest neurodegenerative disease after Alzheimer's disease, and its incidence is increasing year by year. Traditional dopamine replacement therapy and deep brain stimulation can only alleviate the clinical symptoms of patients with PD but cannot cure the disease. In recent years, stem cell therapy has been used to treat neurodegenerative diseases. Many studies have shown that stem cell transplantation has a therapeutic effect on PD. Here, we review recent studies indicating that exosomes derived from mesenchymal stem cells also have the potential to treat PD in animal models, but the exact mechanism remains unclear. This article reviews the mechanisms through which exosomes are involved in intercellular information exchange, promote neuroprotection and freely cross the blood-brain barrier in the treatment of PD. The increase in the incidence of PD and the decline in the quality of life of patients with advanced PD have placed a heavy burden on patients, families and society. Therefore, innovative therapies for PD are urgently needed. Herein, we discuss the mechanisms underlying the effects of exosomes in PD, to provide new insights into the treatment of PD. The main purpose of this article is to explore the therapeutic potential of exosomes derived from mesenchymal stem cells and future research directions for this degenerative disease.
Introduction
Parkinson's disease (PD) mainly affects older people, with a prevalence of 1–2% (1, 2). Patients with PD have clinical manifestations of motor symptoms, such as bradykinesia, resting tremor, postural and gait disorders, as well as non-motor symptoms, such as depression, sleep disorders, dementia and peripheral injuries. The onset of PD is very difficult to detect, and patients with PD are often not identified in early stages. The early symptoms of PD are atypical, and can easily be missed or misdiagnosed. Moreover, patients with advanced PD cannot care for themselves, and their quality of life is severely affected, thus posing a heavy burden on patients and their families (3).
Currently, various methods are used to treat PD, most commonly medication and surgery. Drugs such as pharmacological formulations of dopamine substitutes are beneficial in early stages of the disease, but long-term use decreases efficacy and can even cause serious adverse reactions (4). Deep brain stimulation (DBS), another treatment for PD, is increasingly used worldwide. Studies have shown that music, dancing and physical exercise can both improve the symptoms of patients with PD and decrease PD risk (5, 6). However, these treatment methods only relieve the symptoms of PD but cannot completely prevent the degeneration process. Therefore, innovative therapies, such as inducing neuroprotection of surviving dopamine neurons and reestablishing dopamine balance, are urgently needed.
The pathological features of PD are mainly characterized by dopaminergic neuron loss in the substantia nigra (SN) (7). Consequently, cell replacement therapy may be a straightforward and potentially curative treatment. Mesenchymal stem cells (MSCs) are a type of stem cells with multiple differentiation potential. Under certain conditions, MSCs can differentiate into neurons. Because of their self-differentiation ability, and the paracrine effects of secretion of growth factors and cytokines, MSCs may have therapeutic potential (8, 9). Exosomes, an important paracrine factor of MSCs, have also been reported to be a potential treatment for central nervous system diseases.
Therefore, the purpose of this review is to explore the therapeutic potential of exosomes derived from MSCs in PD and their future development prospects for treating degenerative diseases.
Pathophysiology of PD
The etiology of PD
The etiology of PD remains unclear but is believed to involve the interaction of aging, genetics and environmental factors. The pathology is associated with a decrease in dopaminergic neurons, a decrease in neurons in the SN and other brain structures, and the formation of Lewy bodies. The diagnosis of PD is mainly based on medical history and clinical manifestations, and no specific diagnostic test is available. Auxiliary imaging studies such as positron emission tomography and magnetic resonance imaging are helpful for the clinical diagnosis of PD (1, 2). Genes are an important factor in PD. Studies have indicated that the prevalence of PD among the first-degree relatives of patients with PD is two to three times higher than that in the general population (10). The primary risk factor among external factors is aging.
The incidence of PD increases exponentially with age. Environmental exposure is another risk factor. A meta-analysis has shown that exposure to pesticides, head injury, rural living, taking beta-blockers, engaging in agriculture activities and drinking well water can increase the risk of PD, whereas alcohol consumption, smoking, drinking coffee, and using non-steroidal anti-inflammatory drugs or calcium channel blockers can decrease the risk of PD (11). A study has also shown that twins with PD tend to smoke less than twins without PD (12). Therefore, people who smoke may have lower risk of PD. In short, PD is generally believed to be caused by a variety of factors.
The genetics of PD
A series of major gene mutations are known to cause autosomal dominant and recessive PD. For example, α-Synuclein (SNCA), parkin 2 (PARK2), PTEN-induced putative kinase 1 (PINK1), park7, Leucine-rich repeat kinase 2 (LRRK2), bone marrow stromal cell antigen 1 (BST1), and microtubule-associated protein tau (MAPT) may cause familial PD. Other genetic defects at other loci may be susceptibility sites for sporadic PD (13). Mutations in the LRRK2 and Parkin genes are considered the most common causes of dominant and recessive PD, respectively (14).
Previous studies have shown that mutations in the glycocerebral glycosidase 1 (GBA1) gene are the most susceptible risk factor for PD. In addition, the GBA1 mutation rate in patients with PD is higher than that in healthy people (15). GBA1 gene mutation leads to abnormal folding of glucocerebrosidase (GCase), thus affecting the endoplasmic reticulum (ER), lysosomes and mitochondria (13). Abnormally folded glucocerebrosidase in the ER enhances the ubiquitin-proteasome system and ER stress. The ER stress in turn triggers the unfolded protein response as well as ER degradation, thereby increasing cell apoptosis. The presence of misfolded GCase in lysosomes, coupled with a decrease in wild-type GCase levels, results in SNCA degradation through delayed chaperone-mediated autophagy, and leads to the accumulation and aggregation of SNCA. Simultaneously, lysosome dysfunction leads to decreased autophagosome clearance and to the accumulation of cell debris. GBA1 mutation promotes the formation of free radicals, and decreases the production of ATP, oxygen consumption and the membrane potential (15), thereby perturbing normal mitochondrial function. In addition, GBA1 heterozygous mutation alters the lysosomal enzyme that converts glycosylceramide to ceramide and also increases the risk of PD (16).
Lewy bodies
The pathological features of PD are progressive loss of dopaminergic neurons in the substantia nigra pars compacta and the deposition of Lewy bodies (13). Both pathological features of PD extensively involve other parts of the central nervous system and surrounding tissues (17–19). Oral administration of levodopa, the precursor of dopamine, can relieve most of the symptoms. Lewy bodies, a characteristic marker of PD brain tissue, are composed of misfolded neuron inclusions formed by aggregates of SNCA. Lewy bodies are composed of a dense core in the center, surrounded by fine filamentous fainting. The formation of Lewy bodies may be associated with mitochondrial defects (20). The SNCA gene, located on chromosome 4q21, encodes a protein that plays an important role in the pathophysiology of PD and was the first PD autosomal dominant gene discovered (21). Animal model studies and human cadaver studies have shown clear cellular and molecular changes in PD brain tissues, such as subtle changes in specific gene expression, neuroinflammation, oxidative stress, mitochondrial dysfunction, apoptosis, autophagy and glial cell-derived neurotrophic factor deficiency. Imbalances in non-coding RNAs and effects of genomic variation are also associated with the pathogenesis of PD (22). Furthermore, the interactions between glial cells and miRNAs play crucial roles in the occurrence and development of disease (22, 23).
Treatment of PD
The clinical manifestations of PD can be divided into motor symptoms and non-motor symptoms. Both can affect patients' ability to work and conduct daily life activities. Therefore, drug treatment aims to improve symptoms, avoid or decrease adverse reactions, and improve work ability and quality of life. Early diagnosis and treatment are advocated. Currently, the treatments for PD include pharmacological and non-pharmacological treatments. The former mainly consists of levodopa preparations, dopamine agonists, monoamine oxidase-B (MAO-B) inhibitors, anticholinergic drugs and amantadine. The latter mainly includes DBS and exercise therapy (24). As the availability of treatment methods increases, the management of patients with PD must be individualized (25).
Drug therapy
Levodopa, the precursor of dopamine, is the most commonly used drug in clinical practice. It can further supplement the loss of dopamine and can improve patients' daily lives and movement; however, it cannot improve mental function. Dopamine agonists alleviate the symptoms of PD by enhancing the activity of dopamine receptors, but they may cause abnormal reward regulation-related behaviors, nausea, orthostatic hypotension, dizziness, drowsiness, hallucinations and edema. MAO-B inhibits monoamine oxidase, thereby inhibiting the degradation of dopamine into dihydroxyphenylacetic acid and prolonging the effect of dopamine. It is often used as an adjuvant drug for patients with a weakened levodopa response. Anticholinergic drugs function by blocking postsynaptic acetylcholine receptors. Adverse effects of anticholinergic medications may include mental confusion, mydriasis with decreased vision, dry eyes, dry mouth, constipation and urinary retention. These drugs are contraindicated for patients with acute glaucoma and should be used for patients with peptic ulcers. The pharmacological mechanism of amantadine is unknown; however, thus drug can induce edema and hallucination (26). Pharmacotherapy can improve patients' symptoms to some extent but cannot change the progressive course of PD.
DBS
In 2002, DBS was approved by the US Food and Drug Administration for the treatment of PD and is commonly used in advanced PD. This surgery enables persistent microcurrent stimulation of specific sites in the deep brain via electrodes implanted into the brain, to eliminate or inhibit potential pathological neural activity. Two common targets are the subthalamic nucleus and the globus pallidus interna. This surgery can effectively improve the symptoms of patients with PD, such as bradykinesia, tremor, rigidity, and conversion pulsation. However, whether it is effective in the treatment of gait disorders, speech and cognitive dysfunction has not been determined. Cerebral hemorrhage venous thrombosis, phlebitis, pneumonia, urinary tract infections and pulmonary embolism are the common complications (27, 28). As with drug therapy, DBS can neither completely treat PD nor prevent the progression of the neurodegenerative process of PD (29).
The roles of MSCs in PD treatment
MSCs have a high capacity for self-propagation and can differentiate into neural precursor cells. Therefore, these cells are considered an ideal source to replace lost cells in degenerative diseases such as PD (30). Early studies have shown that the expression of dopaminergic markers such as tyrosine hydroxylase (TH), dopamine transporter (DAT) and dopamine D2 receptor in the substantia nigra pars compacta and the functional release of dopamine in the striatum are greater in PD rats than controls. Moreover, PD rats showed behavioral improvements. Therefore, researchers have hypothesized that MSC transplantation might prevent the progressive death of neurons and subsequently restore the function of dopamine neurons, thus leading to improvement in early behavior (31). However, few clinical studies have examined the treatment of PD with MSCs. In a study of seven patients with PD, motor function has been found to improve after injection of MSCs (32). Overall, after transplantation of MSCs, the course of PD could be modified, thereby ameliorating both motor and non-motor symptoms of PD (33, 34).
MSCs for PD
MSCs
MSCs are stem cells with self-renewal ability and multiple differentiation potential. The standards for MSCs established by the International Society for Cell Therapy are as follows: MSCs are stem cells ① that adhere to plastic ②have the potential to differentiate into osteoblasts, chondrocytes and adipocytes ③ and are characterized by CD105, CD73, and CD90 positivity, and CD45, CD34, CD14 CD11b, CD19, and human leukocyte antigen-DR (HLA-DR) negativity (35, 36).
The origin of MSCs
MSCs have many sources and can be extracted from bone marrow, umbilical cord blood and adipocytes (37, 38). Many studies have shown that MSCs can also be extracted from the endometrium and menstrual blood (39) (Figure 1). Moreover, MSCs have been demonstrated to be useful in the treatment of many diseases, such as acute kidney injury (40), and wounds (41). MSCs are useful for not only PD but also other diseases. Therefore, MSCs are a promising treatment and have been more widely studied in recent years.
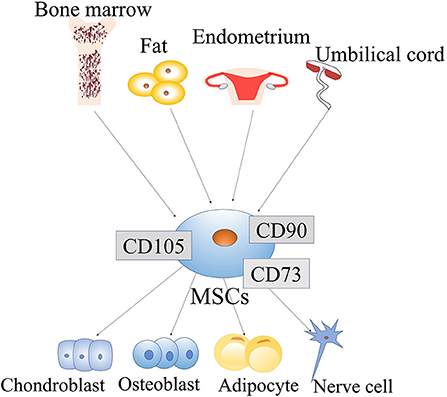
Figure 1. Origin and differentiation of mesenchymal stem cells (MSCs): MSCs come from a wide range of sources, such as the bone marrow, fat, endometrium, and umbilical cord. MSCs have the potential for self-renewal and multi-lineage differentiation. They can differentiate into various lineage of tissues, include chondroblasts, osteoblasts, adipocytes, and neuronal cells. MSCs are easily obtainable from a wide range of sources, and their ability to differentiate multi-directionally allows them to be applied in a variety of fields.
Differentiation of MSCs
Increasing evidence indicates that MSCs can transdifferentiate into certain cell lineages. In adults, MSCs are generally considered to have mesodermal origin (42) and therefore can differentiate into mesodermal lineages, such as myocytes, chondrocytes, osteocytes, adipocytes, tenocytes and stromal fibroblasts. They are also able to differentiate into endodermal and ectodermal lineages, such as hepatocytes, neurons and glia (43). Epigenetic changes can mediate the MSC phenotypic transition. For example, exposure of bone marrow stromal cells (BMSCs) to epigenetic modulators and neural induction mediators can lead to their neuronal differentiation. Under the action of adiposity-inducing factor, the morphology of these cells undergoes similar changes (44). Therefore, epigenetic regulators can regulate the differentiation potential of MSCs into multiple cell types (Figure 1).
Current research findings on MSCs in PD treatment
In recent years, researchers have increasingly paid attention to PD. Recent research has shown that in a mouse model of PD, knockdown of the RNA binding protein polypyrimidine tract-binding protein (PTB) in astrocytes can directly transform them into functional neurons and consequently effectively improve PD-related dyskinesia. More importantly, the use of antisense oligonucleotides that inhibit PTB has achieved similar therapeutic effects (45), thus providing a major breakthrough in the treatment of PD. Furthermore, various studies have shown that MSCs have potential in the treatment of PD. New evidence showing that stem cell-based cell transplantation instead of dysfunctional dopaminergic neurons in the treatment of PD has attracted widespread attention (46). In that study, transplantation of MSCs into PD animal model increased dopaminergic neurons in the animal brain and led to improvements in behavior; however, whether MSCs can be directly transformed into dopaminergic neurons remains to be confirmed (46). Another study has transplanted green fluorescent protein–labeled MSCs into a PD rat model and shown significant improvements in animal behavior; moreover, green fluorescent protein–labeled tyrosine hydroxylase positive cells were found in the brain in the rat model. These results suggest that MSCs are likely to replace missing dopaminergic neurons and have potential in the treatment of PD (47).
Early application of drug therapy for PD can effectively improve motor symptoms in patients. DBS is a surgical operation aimed at improving the motor symptoms and quality of life of patients with PD (48). However, both treatments can only improve short-term symptoms, whereas long-term use may have some adverse effects (49). Because current treatment methods cannot alter the underlying pathology, they cannot cure PD. The purpose of MSC therapy is to replace degenerative dopaminergic neurons, thus targeting the pathogenesis of PD. Autologous MSC transplantation is harmless to recipients. MSC transplantation can regenerate and recover nerves in damaged tissue, thus bringing hope for the treatment of PD (30). Current experimental evidence shows that MSCs derived from bone marrow, fat and cord blood significantly improve the symptoms of PD (50).
The mechanism through which MSCs play a beneficial role in PD
The mechanism of MSCs in the treatment of PD
The mechanism of MSCs in the treatment of PD has not been well-elucidated. The current research findings are as follows. First, MSCs secrete neurotrophic growth factors, including glial cell-derived neurotrophic factor (GDNF), vascular endothelial growth factor and brain-derived neurotrophic factor (51, 52), which are further secreted under specific culture conditions (53). Neurotrophic growth factor is necessary for the growth and development of neurons. Some neurotrophic factors support cell survival and protect against neuronal degeneration (54). In many preclinical models of neurological diseases, including amyotrophic lateral sclerosis, PD, and multiple system atrophy transgenic animal models, neurotrophic factors have been found to increase the survival rate of neurons (23, 55). The same mechanism is also believed to be involved in the treatment of PD. Second, MSCs are weak in immunogenicity and exert immunosuppressive effects through various mechanisms, such as inhibiting T cells and B cells, dendritic cells, natural killer cells and neutrophils (3). Therefore, they may confer potential benefits in the treatment of allograft rejection and suppression of autoimmune diseases. Moreover, MSCs have anti-apoptosis and anti-inflammatory effects, and can improve the prognosis of PD. They also protect against SNCA-induced degeneration of dopaminergic neurons, as confirmed in a neurotoxin-induced PD model (56, 57). In recent years, studies have attributed the therapeutic effects of MSCs to paracrine effectors. Notably, the isolation process of MSCs is simple, and the cells have high expansion capacity, high biological safety, low ethical challenges and low tumorigenic risk (58–60). In summary, the benefits of MSCs in treating PD include their availability, low immunogenicity multiple differentiation capability, and secretion of neurotrophic growth factors and paracrine factors.
Clinical trials
Currently, MSCs in the treatment of PD have been examined in 12 clinical trials. Five clinical trials are in the recruitment stage, and two clinical trials are no longer available (Table 1). The two discontinued trials involved eight or 12 injections with cord blood MSCs; patients were followed up to observe changes in activities of daily living and quality of life, to evaluate the efficacy of the drugs. The reasons for discontinuation remain unknown. In the five clinical trials in recruitment, MSCs and placebo experiments, autologous MSCs and allogeneic MSC transplantation experiments have been performed to compare their efficacy. However, no results have been reported. The number of clinical trials is small, and therefore the application of MSCs in the treatment of PD requires further research.
Adverse effects of mesenchymal stem cells in PD
MSCs have been used to treat many diseases because of their differentiation potential, but several problems remain. For example, MSCs cannot effectively cross the blood-brain barrier without the help of osmotic agents. Moreover, MSC have only a low survival rate in hosts after transplantation (61). In addition, allogeneic MSCs can cause transplant rejection, thus causing harm to patients. Therefore, many researchers have used different approaches to solve these problems.
Researchers have found that exosomes derived from MSCs can freely cross the blood-brain barrier, thus decreasing transplant rejection and effectively ameliorating PD symptoms. Therefore, exosomes may be a better treatment for PD with fewer side effects.
MSC derived exosomes in PD
Application of MSC derived exosomes in PD
In recent years, with the development of MSCs in the treatment of PD, many researchers have attributed the positive effects of MSCs in the treatment of PD to exosomes. Exosomes are extracellular vesicles released by MSCs. MSCs are regulated by the extracellular vesicles (EVs) that they secrete (50, 51). EVs are involved in the exchange of materials between cells (62).
EVs are released by a variety of eukaryotic cells, and contain many substances including RNA, DNA and major nutrients. EVs play important roles in intercellular communication (63). In recent years, EVs have gradually been applied in tumor prevention. EVs are selectively delivered to target organs/tumors after loading with therapeutic agents (e.g., drugs). Cell-free therapy is another treatment option. However, EVs have several limitations. For example, tumor-derived EVs promote angiogenesis and consequently promote tumor growth, and also play a role in tumor development (64–66). In addition, the expression of exosome related proteins and genes increases after metformin treatment of glioblastoma multiforme (GBM) cells, and the growth of cancer cells is inhibited. Thus, activation of the exosome secretion pathway plays an important role in cancer treatment (67). Feghhi et al. have found that exosomes promote angiogenesis (68).
According to differences in size, density, surface markers and sources, EVs can be further subdivided into apoptotic bodies, cellular microparticles and exosomes (69). Apoptotic bodies are the EVs with the largest diameter formed by MSCs in apoptosis. Cellular microparticles are formed by budding of the MSC cell membrane (70). Exosomes, the most typical EVs, were first discovered in mature sheep reticulocytes in 1983 (35). Exosomes are a subtype of vesicles that can be released by various cells in all living systems (52, 53). In addition, studies increasingly indicate that exosomes derived from MSCs can effectively improve PD symptoms. Experimental models of PD treated with exosomes secreted by MSCs have shown that exosome treatment can avoid the adverse effects caused by MSC transplantation alone, such as poor differentiation (71). Moreover, studies have shown that injection of exosomes secreted by MSCs into the substantia nigra and striatum partially reverse motor and histological symptoms in a 6-OHDA PD rat model (72). In another study in a 6-OHDA rat model of PD, injection of human bone marrow stromal cells (hBMSC) derived exosomes, compared with hBMSC self-transplantation, has been found to be more effective in rescuing dopaminergic neurons and inducing higher levels of neuronal differentiation (73) (Table 2). MSC derived exosomes can transfer miRNAs to neuronal cells. MiR-133b is expressed in midbrain dopaminergic neurons and is a type of miRNA that regulates the production of tyrosine hydroxylase. Exosomes with high miR-133b content can promote the growth of neurites (74). Researchers have discovered that patients with PD lack miR-133b (75). Exosomes that are released by genetically modified macrophages contain antioxidants, catalase and GDNF, which can effectively improve the symptoms of PD (76–78). Exosomes derived from MSCs have been studied in a variety of PD models and have therapeutic potential in the treatment of PD. Therefore, exosomes are considered a promising treatment for PD.
The concept and structure of exosomes
Exosomes are single membrane vesicles with the same topological structure as cells (79). The early endosomal membrane buds inward to form exosomal vesicles, which mature into multivesicular bodies, which in turn fuse with the cell membrane and are released into the extracellular space as exosomes (80–82). Multivesicular bodies may also degrade exosomes by binding lysosomes and circulating biomolecules to the plasma membrane (83). Both ESCRT-dependent and ESCRT independent mechanisms are involved in the production of exosomes. However, the interaction between these two mechanisms is unclear (84). The released exosomes exert their effects on receptor cells through endocytosis, ligand-receptor binding or direct binding (85, 86). Exosomes can be secreted by all cells in the body (87), such as adipocytes (88), and hypothalamic stem cells (89). Exosomes contain a variety of molecules, such as immune components, hormones, sugars, steroids, RNA, microRNAs, lipids and nucleic acid polymers (35, 90) (Figure 2). Exosomes can affect gene expression and protein biological activity via receptor cells through the messenger RNAs and proteins that they carry (91). For example, a study of colon cancer with KRAS gene mutation has shown that miR-10b is selectively increased in KRAS wild-type-derived exosomes, whereas miR-100 is increased in KRAS mutant-derived exosomes. This study has indicated that the KRAS gene may control the expression of miRNA by exosomes (92). Furthermore, exosomes from different sources carry different proteins and lipids, and many exosomes carry specific proteins. For example, myelin protein is specifically expressed in the exosomes secreted by oligodendrocytes (93). Some proteins, such as CD63, CD89, CD81, CD9 and CD82, and the heat shock proteins Heat Shock Protein 70 (HSP70) and Heat Shock Protein 90 (HSP90) (94), are specifically expressed in all exosomes and are called exosome-related-proteins, thereby providing a theoretical basis for the extraction and identification of exosomes. According to ExoCarta (Home-Exosome database), ~8,000 proteins and 194 lipids are known to be associated with exosomes (95). No evidence has indicated that exosomes affect gene expression related to neurodegenerative diseases. Moreover, exosomes from different sources may have different functions. For example, exosomes from antigen-presenting cells can express major histocompatibility complex class I and class II molecules on the cell surface, which help activate CD4+ and CD8+ T cells and induce specific immune responses. In another example, exosomes containing prostaglandins secreted by platelets have been suggested be involved in the inflammatory response (96, 97). As described above, exosomes are taken up by receptor cells through endocytosis, receptor ligand interaction or fusion with the cell membrane. These processes rely on the interactions between proteins on the surface of exosomes and their receptor cells. Exosomes containing Tetraspanin 8 (TSPAN8) and integrin α-4 have been shown to be easily detected by CD54+ pancreatic cells (98).
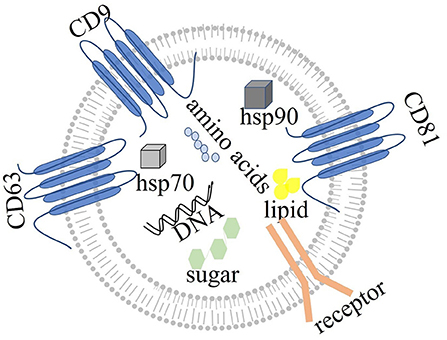
Figure 2. The structure of exosomes: exosomes, with single membranes, are rich in amino acids, sugar, lipids, and nucleic acids. Exosomes also express specific proteins, such as tetraspanin (CD63, CD81, and CD9), Heat Shock Protein 70 (hsp70), and Heat Shock Protein 90 (hsp90). Exosomes act by binding other cells through membrane receptors.
Mechanisms through which exosomes derived from MSCs may have a beneficial effect on PD
According to clinical research reports, 98% of potentially effective drugs for the treatment of central nervous system diseases have failed in clinical trials because they cannot cross the blood-brain barrier (99). In general, the diameter of exosomes is only 30–150 nm (100, 101), which is sufficiently small to allow them to freely cross the blood-brain barrier and reach the central nervous system. In fact, some studies have demonstrated that exosomes have small diameters, low immunogenicity and long circulating half-lives, and consequently can be used as therapeutic signals or drug delivery carriers (102). For example, catalase is a promising treatment enzyme for PD, but the enzyme nanoparticles cannot cross the blood-brain barrier. In contrast, catalase administered by exosomes can reach the target neurons in a Parkinson's mouse model and accumulate in target cells (103). A recent study has discovered that in idiopathic PD, autophosphorylated ser (P)−1292 LRRK2 levels in urinary exosomes are elevated. Moreover, Ser (P)-1292 LRRK2 levels are higher in patients with PD with poorer cognition and are correlated with poor performance. Exosomes result in biochemical changes in LRRK2 in idiopathic PD (104). Exosomes can transfer protein, RNA and lipid components from one cell to another, and thus play an important role in intercellular communication. In addition, exosomes can communicate between cells under various physiological and pathological conditions, and therefore may play an important role in the treatment of PD (105–109). Frühbeis has reported that exosomes can mediate the interactions between neurons and oligodendrocytes. With the activation of neuronal pressure signal transduction, oligodendrocytes release exosomes, which are absorbed by neurons through endocytosis, and subsequently transfer protective proteins and mRNA to axons, thereby promoting neuroprotection (110, 111) (Figure 3). Furthermore, exosomes mediate neuron development, nerve regeneration and synaptic plasticity. Exosomes transfer regulatory elements to sites of nerve injury, thus aiding in protein synthesis and tissue regeneration (112). Some researchers have found that under pathological conditions, microglia are activated and transformed into antigen presenting cells by secreting exosomes. Under the stimulation with ATP and the activation of sphingomyelinase, the plasma membranes of microglia and astrocytes release the pro-inflammatory cytokine IL-β (113, 114). Therefore, exosomes may contribute to the antigen presentation of immune cells, participate in cell signal transduction, and have anti-inflammatory or pro-inflammatory characteristics (115, 116). A recent study has indicated that the motor symptoms and dopamine neurons in the substantia nigra striatum are upregulated after exosome treatment in PD mice, owing to the autophagy induced by exosomes (117).
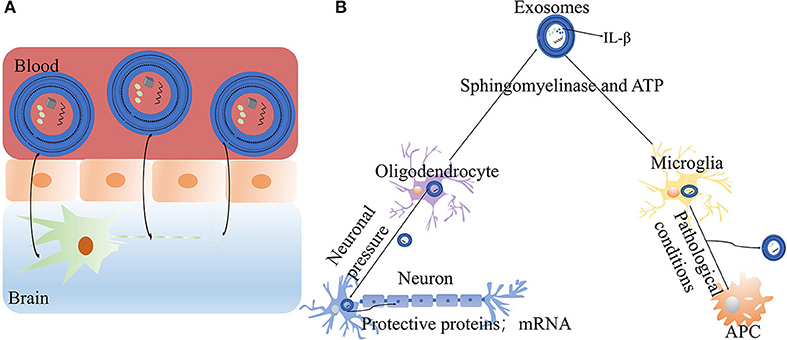
Figure 3. The mechanisms of exosomes in PD: (A) Exosomes can freely cross the blood-brain barrier, reach the central nervous system and exert therapeutic effects. (B) The roles of exosomes in neurons and cells: exosomes released by oligodendrocytes promote neuroprotection (ATP, adenosine-triphosphate; IL-β, Interleukin-1β).
However, anti-inflammatory drugs have been suggested to decrease the risk of PD, thus indicating that inflammation may promote the underlying PD process. Exosomes are believed to function in this way (118). All the above mechanisms may be involved in the treatment of PD by exosomes.
Prospects of exosomes in the treatment of PD
In 1817, James Parkinson's described PD in an article entitled “Tremor Paralysis.” Since then, understanding of PD has made great progress (119, 120). In recent years, increasing attention has been paid to PD, thereby improving understanding of PD. In the past 30 years, with the development of medical technology, the overall condition of patients with PD has significantly improved (121). Although studies increasingly involve research on PD, no satisfactory treatment has been found.
Exosomes have been demonstrated to be important in the development and treatment of many diseases. For example, the level of CD8 in exosomes from patients with chronic hepatitis C is higher than that in healthy people, and appears to be associated with inflammatory activity and fibrosis (122); Exogenous mir-145–5p delivered by exosomes can effectively inhibit the development of pancreatic ductal adenocarcinoma (123). As new carriers, exosomes have been used to provide nucleic acids for the treatment of cancer (124). Therefore, exosomes are useful in the treatment of many diseases. Only a few clinical trials have examined exosomes in the treatment of PD; therefore, more studies are needed. Compared with MSC injection therapy, exosomes are safer and easier to control. Exosomes are natural nanoparticle biological carriers with stable properties and good membrane permeability, which can cross the blood-brain barrier (125). Moreover, exosomes can recognize specific cells, thus resulting in better curative effects and fewer off-target effects than other biological carriers (98). Exosomes are easy to obtain and can be separated from the blood, urine, saliva, amniotic fluid, malignant ascites, bronchoalveolar lavage fluid, synovial fluid and breast milk (97). Therefore, exosome-based drug therapy may be a potential treatment for many diseases.
Conclusion and future prospects
This review highlighted the important roles of MSC-derived exosomes in the treatment of PD. The precise mechanism of MSC-derived exosomes involved in in PD treatment remains unknown but may involve crossing of the blood-brain barrier, intercellular communication, neuroprotection and anti-inflammatory effects. Crossing the blood-brain barrier and intercellular communication may be especially important in PD treatment. The neuroprotective and anti-inflammatory effects still require further research.
Although MSC-derived exosome therapy is a promising modality for PD, several problems require further attention and exploration: (1) MSC-derived exosomes have demonstrated great potential and availability for treating PD in animal models. However, research in large animal models and humans is lacking and remains to be conducted. In addition, randomized controlled trials should be performed to confirm the therapeutic effects of exosomes. (2) Exosomes can be secreted by all cells and have a wide range of sources. What types of cells should be extracted for research? Olfactory ensheathing cells, a special type glial cells, are the best materials for nerve repair and can release exosomes. Therefore, these cells may be a good choice.
Author contributions
S-fL, J-lZ, and L-yL are responsible for writing the manuscript. M-mL, L-cY, and X-rC are responsible for data collection. C-nC and SL are mainly responsible for reviewing and revising the article. All authors contributed to the article and approved the submitted version.
Funding
This work was supported by grants from the Natural Science Foundation of Fujian Province of China (Grant number 2019J01164), the Scientific Foundation of Quanzhou City for High Level Talents (Grant number 2019C075R) from C-nC, the Foundation of Science and Technology Bureau of Quanzhou (Grant number 2020CT003) from SL, and the Fujian Provincial Health Technology Project (2019-1-54).
Acknowledgments
We thank International Science Editing for editing this manuscript.
Conflict of interest
The authors declare that the research was conducted in the absence of any commercial or financial relationships that could be construed as a potential conflict of interest.
Publisher's note
All claims expressed in this article are solely those of the authors and do not necessarily represent those of their affiliated organizations, or those of the publisher, the editors and the reviewers. Any product that may be evaluated in this article, or claim that may be made by its manufacturer, is not guaranteed or endorsed by the publisher.
References
1. Massano J, Bhatia KP. Clinical approach to Parkinson's disease: features, diagnosis, and principles of management. Cold Spring Harb Perspect Med. (2012) 2:a008870. doi: 10.1101/cshperspect.a008870
2. Gao LL, Wu T. The study of brain functional connectivity in Parkinson's disease. Transl Neurodegener. (2016) 5:18. doi: 10.1186/s40035-016-0066-0
3. Berrío Sánchez J, Cucarian Hurtado J, Barcos Nunes R, de Oliveira AA. Mesenchymal stem cell transplantation and aerobic exercise for Parkinson's disease: therapeutic assets beyond the motor domain. Rev Neurosci. (2019) 30:165–78. doi: 10.1515/revneuro-2018-0011
4. Asahina M, Vichayanrat E, Low DA, Iodice V, Mathias CJ. Autonomic dysfunction in parkinsonian disorders: assessment and pathophysiology. J Neurol Neurosurg Psychiatry. (2013) 84:674–80. doi: 10.1136/jnnp-2012-303135
5. Pereira A, Marinho V, Gupta D, Magalhães F, Ayres C, Teixeira S. Music therapy and dance as gait rehabilitation in patients with Parkinson disease: a review of evidence. J Geriatr Psychiatry Neurol. (2019) 32:49–56. doi: 10.1177/0891988718819858
6. Xu X, Fu Z, Le W. Exercise and Parkinson's disease. Int Rev Neurobiol. (2019) 147:45–74. doi: 10.1016/bs.irn.2019.06.003
7. Dickson DW. Neuropathology of Parkinson disease. Parkinsonism Relat Disord. (2018) 46(Suppl.1):S30–30S33. doi: 10.1016/j.parkreldis.2017.07.033
8. Lo Furno D, Mannino G, Giuffrida R. Functional role of mesenchymal stem cells in the treatment of chronic neurodegenerative diseases. J Cell Physiol. (2018) 233:3982–99. doi: 10.1002/jcp.26192
9. Joo HS, Suh JH, Lee HJ, Bang ES, Lee JM. Current knowledge and future perspectives on mesenchymal stem cell-derived exosomes as a new therapeutic agent. Int J Mol Sci. (2020) 21:30727. doi: 10.3390/ijms21030727
10. Tysnes OB, Storstein A. Epidemiology of Parkinson's disease. J Neural Transm. (2017) 124:901–5. doi: 10.1007/s00702-017-1686-y
11. Kalia LV, Lang AE. Parkinson's disease. Lancet. (2015) 386:896–912. doi: 10.1016/S0140-6736(14)61393-3
12. Ritz B, Lee PC, Lassen CF, Arah OA. Parkinson disease and smoking revisited: ease of quitting is an early sign of the disease. Neurology. (2014) 83:1396–402. doi: 10.1212/WNL.0000000000000879
13. Cacabelos R. Parkinson's disease: from pathogenesis to pharmacogenomics. Int J Mol Sci. (2017) 18:30551. doi: 10.3390/ijms18030551
14. Corti O, Lesage S, Brice A. What genetics tells us about the causes and mechanisms of Parkinson's disease. Physiol Rev. (2011) 91:1161–218. doi: 10.1152/physrev.00022.2010
15. Migdalska-Richards A, Schapira AH. The relationship between glucocerebrosidase mutations and Parkinson disease. J Neurochem. (2016) 139(Suppl.1):77–90. doi: 10.1111/jnc.13385
16. Ferrazza R, Cogo S, Melrose H, Bubacco L, Greggio E, Guella G, et al. LRRK2 deficiency impacts ceramide metabolism in brain. Biochem Biophys Res Commun. (2016) 478:1141–6. doi: 10.1016/j.bbrc.2016.08.082
17. Naughton C, O'Toole D, Kirik D, Dowd E. Interaction between subclinical doses of the Parkinson's disease associated gene, α-synuclein, and the pesticide, rotenone, precipitates motor dysfunction and nigrostriatal neurodegeneration in rats. Behav Brain Res. (2017) 316:160–8. doi: 10.1016/j.bbr.2016.08.056
18. Ritz BR, Paul KC, Bronstein JM. Of pesticides and men: a California story of genes and environment in Parkinson's disease. Curr Environ Health Rep. (2016) 3:40–52. doi: 10.1007/s40572-016-0083-2
19. Rokad D, Ghaisas S, Harischandra DS, Jin H, Anantharam V, Kanthasamy A, et al. Role of neurotoxicants and traumatic brain injury in α-synuclein protein misfolding and aggregation. Brain Res Bull. (2017) 133:60–70. doi: 10.1016/j.brainresbull.2016.12.003
20. Cao Z, Wu Y, Liu G, Jiang Y, Wang X, Wang Z, et al. α-Synuclein in salivary extracellular vesicles as a potential biomarker of Parkinson's disease. Neurosci Lett. (2019) 696:114–20. doi: 10.1016/j.neulet.2018.12.030
21. Cheng L, Wang L, Li NN, Yu WJ, Sun XY, Li JY, et al. rs356182 variant increases risk of sporadic Parkinson's disease in ethnic Chinese. J Neurol Sci. (2016) 368:231–4. doi: 10.1016/j.jns.2016.07.032
22. Rezaei O, Nateghinia S, Estiar MA, Taheri M, Ghafouri-Fard S. Assessment of the role of non-coding RNAs in the pathophysiology of Parkinson's disease. Eur J Pharmacol. (2021) 896:173914. doi: 10.1016/j.ejphar.2021.173914
23. Zaman V, Shields DC, Shams R, Drasites KP, Matzelle D, Haque A, et al. Cellular and molecular pathophysiology in the progression of Parkinson's disease. Metab Brain Dis. (2021) 689:5. doi: 10.1007/s11011-021-00689-5
24. Armstrong MJ, Okun MS. Diagnosis and treatment of Parkinson disease: a review. J Am Med Assoc. (2020) 323:548–60. doi: 10.1001/jama.2019.22360
25. Fox SH, Katzenschlager R, Lim SY, Barton B, de Bie R, Seppi K, et al. International Parkinson and movement disorder society evidence-based medicine review: update on treatments for the motor symptoms of Parkinson's disease. Mov Disord. (2018) 33:1248–66. doi: 10.1002/mds.27372
26. Hermanowicz N. Drug therapy for Parkinson's disease. Semin Neurol. (2007) 27:97–105. doi: 10.1055/s-2007-971177
27. McIntyre CC, Hahn PJ. Network perspectives on the mechanisms of deep brain stimulation. Neurobiol Dis. (2010) 38:329–37. doi: 10.1016/j.nbd.2009.09.022
28. Lee DJ, Lozano CS, Dallapiazza RF, Lozano AM. Current and future directions of deep brain stimulation for neurological and psychiatric disorders. J Neurosurg. (2019) 131:333–42. doi: 10.3171/2019.4.JNS181761
29. Malek N. Deep brain stimulation in Parkinson's disease. Neurol India. (2019) 67:968–78. doi: 10.4103/0028-3886.266268
30. Venkatesh K, Sen D. Mesenchymal stem cells as a source of dopaminergic neurons: a potential cell based therapy for Parkinson's disease. Curr Stem Cell Res Ther. (2017) 12:326–47. doi: 10.2174/1574888X12666161114122059
31. Bouchez G, Sensebé L, Vourch P, Garreau L, Bodard S, Rico A, et al. Partial recovery of dopaminergic pathway after graft of adult mesenchymal stem cells in a rat model of Parkinson's disease. Neurochem Int. (2008) 52:1332–42. doi: 10.1016/j.neuint.2008.02.003
32. Andrzejewska A, Dabrowska S, Lukomska B, Janowski M. Mesenchymal stem cells for neurological disorders. Adv Sci. (2021) 8:2002944. doi: 10.1002/advs.202002944
33. Boika A, Aleinikava N, Chyzhyk V, Zafranskaya M, Nizheharodava D, Ponomarev V. Mesenchymal stem cells in Parkinson's disease: motor and nonmotor symptoms in the early posttransplant period. Surg Neurol Int. (2020) 11:380. doi: 10.25259/SNI_233_2020
34. Gugliandolo A, Bramanti P, Mazzon E. Mesenchymal stem cell therapy in Parkinson's disease animal models. Curr Res Transl Med. (2017) 65:51–60. doi: 10.1016/j.retram.2016.10.007
35. Vilaça-Faria H, Salgado AJ, Teixeira FG. Mesenchymal stem cells-derived exosomes: a new possible therapeutic strategy for Parkinson's disease. Cells. (2019) 8:20118. doi: 10.3390/cells8020118
36. Teixeira FG, Carvalho MM, Sousa N, Salgado AJ. Mesenchymal stem cells secretome: a new paradigm for central nervous system regeneration. Cell Mol Life Sci. (2013) 70:3871–82. doi: 10.1007/s00018-013-1290-8
37. Galipeau J, Sensébé L. Mesenchymal stromal cells: clinical challenges and therapeutic opportunities. Cell Stem Cell. (2018) 22:824–33. doi: 10.1016/j.stem.2018.05.004
38. Chen Y, Shen J, Ke K, Gu X. Clinical potential and current progress of mesenchymal stem cells for Parkinson's disease: a systematic review. Neurol Sci. (2020) 41:1051–61. doi: 10.1007/s10072-020-04240-9
39. Ding DC, Shyu WC, Lin SZ. Mesenchymal stem cells. Cell Transplant. (2011) 20:5–14. doi: 10.3727/096368910X
40. Zilberman-Itskovich S, Efrati S. Mesenchymal stromal cell uses for acute kidney injury-current available data and future perspectives: a mini-review. Front Immunol. (2020) 11:1369. doi: 10.3389/fimmu.2020.01369
41. Zomer HD, Jeremias T, Ratner B, Trentin AG. Mesenchymal stromal cells from dermal and adipose tissues induce macrophage polarization to a pro-repair phenotype and improve skin wound healing. Cytotherapy. (2020) 22:247–60. doi: 10.1016/j.jcyt.2020.02.003
42. Uccelli A, Moretta L, Pistoia V. Mesenchymal stem cells in health and disease. Nat Rev Immunol. (2008) 8:726–36. doi: 10.1038/nri2395
43. Oliveira JT, Mostacada K, de Lima S, Martinez AM. Bone marrow mesenchymal stem cell transplantation for improving nerve regeneration. Int Rev Neurobiol. (2013) 108:59–77. doi: 10.1016/B978-0-12-410499-0.00003-4
44. Mortada I, Mortada R. Epigenetic changes in mesenchymal stem cells differentiation. Eur J Med Genet. (2018) 61:114–8. doi: 10.1016/j.ejmg.2017.10.015
45. Qian H, Kang X, Hu J, Zhang D, Liang Z, Meng F, et al. Reversing a model of Parkinson's disease with in situ converted nigral neurons. Nature. (2020) 582:550–6. doi: 10.1038/s41586-020-2388-4
46. Kim HW, Lee HS, Kang JM, Bae SH, Kim C, Lee SH, et al. Dual effects of human placenta-derived neural cells on neuroprotection and the inhibition of neuroinflammation in a rodent model of Parkinson's disease. Cell Transplant. (2018) 27:814–30. doi: 10.1177/0963689718766324
47. Mostafavi H, Ghassemifard L, Rostami A, Alipour M, Nadri S. Trabecular meshwork mesenchymal stem cell transplantation improve motor symptoms of parkinsonian rat model. Biologicals. (2019) 61:61–7. doi: 10.1016/j.biologicals.2019.06.006
48. Elkouzi A, Vedam-Mai V, Eisinger RS, Okun MS. Emerging therapies in Parkinson disease - repurposed drugs and new approaches. Nat Rev Neurol. (2019) 15:204–23. doi: 10.1038/s41582-019-0155-7
49. Samii A, Nutt JG, Ransom BR. Parkinson's disease. Lancet. (2004) 363:1783–93. doi: 10.1016/S0140-6736(04)16305-8
50. Chang YH, Wu KC, Harn HJ, Lin SZ, Ding DC. Exosomes and stem cells in degenerative disease diagnosis and therapy. Cell Transplant. (2018) 27:349–63. doi: 10.1177/0963689717723636
51. Baglio SR, Pegtel DM, Baldini N. Mesenchymal stem cell secreted vesicles provide novel opportunities in (stem) cell-free therapy. Front Physiol. (2012) 3:359. doi: 10.3389/fphys.2012.00359
52. Lai CP, Breakefield XO. Role of exosomes/microvesicles in the nervous system and use in emerging therapies. Front Physiol. (2012) 3:228. doi: 10.3389/fphys.2012.00228
53. György B, Hung ME, Breakefield XO, Leonard JN. Therapeutic applications of extracellular vesicles: clinical promise and open questions. Annu Rev Pharmacol Toxicol. (2015) 55:439–64. doi: 10.1146/annurev-pharmtox-010814-124630
54. Wang J, Hu WW, Jiang Z, Feng MJ. Advances in treatment of neurodegenerative diseases: perspectives for combination of stem cells with neurotrophic factors. World J Stem Cells. (2020) 12:323–38. doi: 10.4252/wjsc.v12.i5.323
55. Phinney DG, Pittenger MF. Concise review: MSC-derived exosomes for cell-free therapy. Stem Cells. (2017) 35:851–8. doi: 10.1002/stem.2575
56. Oh SH, Lee SC, Kim DY, Kim HN, Shin JY, Ye BS, et al. Mesenchymal stem cells stabilize axonal transports for autophagic clearance of α-synuclein in Parkinsonian models. Stem Cells. (2017) 35:1934–47. doi: 10.1002/stem.2650
57. Liu Z, Cheung HH. Stem cell-based therapies for Parkinson disease. Int J Mol Sci. (2020) 21:218060. doi: 10.3390/ijms21218060
58. Venkataramana NK, Kumar SK, Balaraju S, Radhakrishnan RC, Bansal A, Dixit A, et al. Open-labeled study of unilateral autologous bone-marrow-derived mesenchymal stem cell transplantation in Parkinson's disease. Transl Res. (2010) 155:62–70. doi: 10.1016/j.trsl.2009.07.006
59. Xiong N, Cao X, Zhang Z, Huang J, Chen C, Zhang Z, et al. Long-term efficacy and safety of human umbilical cord mesenchymal stromal cells in rotenone-induced hemiparkinsonian rats. Biol Blood Marrow Transplant. (2010) 16:1519–29. doi: 10.1016/j.bbmt.2010.06.004
60. Zhou Y, Yamamoto Y, Xiao Z, Ochiya T. The immunomodulatory functions of mesenchymal stromal/stem cells mediated via paracrine activity. J Clin Med. (2019) 8:71025. doi: 10.3390/jcm8071025
61. Staff NP, Jones DT, Singer W. Mesenchymal stromal cell therapies for neurodegenerative diseases. Mayo Clin Proc. (2019) 94:892–905. doi: 10.1016/j.mayocp.2019.01.001
63. Hassanpour M, Rezaie J, Nouri M, Panahi Y. The role of extracellular vesicles in COVID-19 virus infection. Infect Genet Evol. (2020) 85:104422. doi: 10.1016/j.meegid.2020.104422
64. Rezaie J, Ahmadi M, Ravanbakhsh R, Mojarad B, Mahbubfam S, Shaban SA, et al. Tumor-derived extracellular vesicles: the metastatic organotropism drivers. Life Sci. (2022) 289:120216. doi: 10.1016/j.lfs.2021.120216
65. Vahabi A, Rezaie J, Hassanpour M, Panahi Y, Nemati M, Rasmi Y, et al. Tumor cells-derived exosomal CircRNAs: novel cancer drivers, molecular mechanisms, and clinical opportunities. Biochem Pharmacol. (2022) 200:115038. doi: 10.1016/j.bcp.2022.115038
66. Rezaie J, Akbari A, Rahbarghazi R. Inhibition of extracellular vesicle biogenesis in tumor cells: a possible way to reduce tumorigenesis. Cell Biochem Funct. (2022) 40:248–62. doi: 10.1002/cbf.3695
67. Soraya H, Sani NA, Jabbari N, Rezaie J. Metformin increases exosome biogenesis and secretion in U87 MG human glioblastoma cells: a possible mechanism of therapeutic resistance. Arch Med Res. (2021) 52:151–62. doi: 10.1016/j.arcmed.2020.10.007
68. Maryam F, Jafar R, Ali A, Nasrollah J, Hessam J, Farzad S, Sławomir S. Effect of multi-functional polyhydroxylated polyhedral oligomeric silsesquioxane (POSS) nanoparticles on the angiogenesis and exosome biogenesis in human umbilical vein endothelial cells (HUVECs). Mater Design. (2021) 197:109227. doi: 10.1016/j.matdes.2020.109227
69. Beer L, Mildner M, Ankersmit HJ. Cell secretome based drug substances in regenerative medicine: when regulatory affairs meet basic science. Ann Transl Med. (2017) 5:170. doi: 10.21037/atm.2017.03.50
70. Cha JM, Shin EK, Sung JH, Moon GJ, Kim EH, Cho YH, et al. Efficient scalable production of therapeutic microvesicles derived from human mesenchymal stem cells. Sci Rep. (2018) 8:1171. doi: 10.1038/s41598-018-19211-6
71. de Angelo M, Cimini A, Castelli V. Insights into the effects of mesenchymal stem cell-derived secretome in Parkinson's disease. Int J Mol Sci. (2020) 21:155241. doi: 10.3390/ijms21155241
72. Teixeira FG, Carvalho MM, Panchalingam KM, Rodrigues AJ, Mendes-Pinheiro B, Anjo S, et al. Impact of the secretome of human mesenchymal stem cells on brain structure and animal behavior in a rat model of Parkinson's disease. Stem Cells Transl Med. (2017) 6:634–46. doi: 10.5966/sctm.2016-0071
73. Mendes-Pinheiro B, Anjo SI, Manadas B, Da Silva JD, Marote A, Behie LA, et al. Bone marrow mesenchymal stem cells' secretome exerts neuroprotective effects in a Parkinson's disease rat model. Front Bioeng Biotechnol. (2019) 7:294. doi: 10.3389/fbioe.2019.00294
74. Xin H, Li Y, Buller B, Katakowski M, Zhang Y, Wang X, et al. Exosome-mediated transfer of miR-133b from multipotent mesenchymal stromal cells to neural cells contributes to neurite outgrowth. Stem Cells. (2012) 30:1556–64. doi: 10.1002/stem.1129
75. Kim J, Inoue K, Ishii J, Vanti WB, Voronov SV, Murchison E, et al. MicroRNA feedback circuit in midbrain dopamine neurons. Science. (2007) 317:1220–4. doi: 10.1126/science.1140481
76. Haney MJ, Zhao Y, Harrison EB, Mahajan V, Ahmed S, He Z, et al. Specific transfection of inflamed brain by macrophages: a new therapeutic strategy for neurodegenerative diseases. PLoS ONE. (2013) 8:e61852. doi: 10.1371/journal.pone.0061852
77. Zhao Y, Haney MJ, Gupta R, Bohnsack JP, He Z, Kabanov AV, et al. GDNF-transfected macrophages produce potent neuroprotective effects in Parkinson's disease mouse model. PLoS ONE. (2014) 9:e106867. doi: 10.1371/journal.pone.0106867
78. Batrakova EV, Kim MS. Using exosomes, naturally-equipped nanocarriers, for drug delivery. J Control Release. (2015) 219:396–405. doi: 10.1016/j.jconrel.2015.07.030
79. Pegtel DM, Gould SJ. Exosomes. Annu Rev Biochem. (2019) 88:487–514. doi: 10.1146/annurev-biochem-013118-111902
80. Fauré J, Lachenal G, Court M, Hirrlinger J, Chatellard-Causse C, Blot B, et al. Exosomes are released by cultured cortical neurones. Mol Cell Neurosci. (2006) 31:642–8. doi: 10.1016/j.mcn.2005.12.003
81. Doyle LM, Wang MZ. Overview of extracellular vesicles, their origin, composition, purpose, and methods for exosome isolation and analysis. Cells. (2019) 8:70727. doi: 10.3390/cells8070727
82. Yuan L, Li JY. Exosomes in Parkinson's disease: current perspectives and future challenges. ACS Chem Neurosci. (2019) 10:964–72. doi: 10.1021/acschemneuro.8b00469
83. Jafari R, Rahbarghazi R, Ahmadi M, Hassanpour M, Rezaie J. Hypoxic exosomes orchestrate tumorigenesis: molecular mechanisms and therapeutic implications. J Transl Med. (2020) 18:474. doi: 10.1186/s12967-020-02662-9
84. Hassanpour M, Rezabakhsh A, Rezaie J, Nouri M, Rahbarghazi R. Exosomal cargos modulate autophagy in recipient cells via different signaling pathways. Cell Biosci. (2020) 10:92. doi: 10.1186/s13578-020-00455-7
85. Fitzner D, Schnaars M, van Rossum D, Krishnamoorthy G, Dibaj P, Bakhti M, et al. Selective transfer of exosomes from oligodendrocytes to microglia by macropinocytosis. J Cell Sci. (2011) 124:447–58. doi: 10.1242/jcs.074088
86. Kahroba H, Hejazi MS, Samadi N. Exosomes: from carcinogenesis and metastasis to diagnosis and treatment of gastric cancer. Cell Mol Life Sci. (2019) 76:1747–58. doi: 10.1007/s00018-019-03035-2
87. Porro C, Panaro MA, Lofrumento DD, Hasalla E, Trotta T. The multiple roles of exosomes in Parkinson's disease: an overview. Immunopharmacol Immunotoxicol. (2019) 41:469–76. doi: 10.1080/08923973.2019.1650371
88. Thomou T, Mori MA, Dreyfuss JM, Konishi M, Sakaguchi M, Wolfrum C, et al. Adipose-derived circulating miRNAs regulate gene expression in other tissues. Nature. (2017) 542:450–5. doi: 10.1038/nature21365
89. Zhang Y, Kim MS, Jia B, Yan J, Zuniga-Hertz JP, Han C, et al. Hypothalamic stem cells control ageing speed partly through exosomal miRNAs. Nature. (2017) 548:52–7. doi: 10.1038/nature23282
90. Beeraka NM, Doreswamy SH, Sadhu SP, Srinivasan A, Pragada RR, Madhunapantula SV, et al. The role of exosomes in stemness and neurodegenerative diseases-chemoresistant-cancer therapeutics and phytochemicals. Int J Mol Sci. (2020) 21:186818. doi: 10.3390/ijms21186818
91. Chistiakov DA, Chistiakov AA. α-Synuclein-carrying extracellular vesicles in Parkinson's disease: deadly transmitters. Acta Neurol Belg. (2017) 117:43–51. doi: 10.1007/s13760-016-0679-1
92. Cha DJ, Franklin JL, Dou Y, Liu Q, Higginbotham JN, Demory Beckler M, et al. KRAS-dependent sorting of miRNA to exosomes. Elife. (2015) 4:e07197. doi: 10.7554/eLife.07197.027
93. Krämer-Albers EM, Bretz N, Tenzer S, Winterstein C, Möbius W, Berger H, et al. Oligodendrocytes secrete exosomes containing major myelin and stress-protective proteins: trophic support for axons. Proteomics Clin Appl. (2007) 1:1446–61. doi: 10.1002/prca.200700522
94. Simons M, Raposo G. Exosomes–vesicular carriers for intercellular communication. Curr Opin Cell Biol. (2009) 21:575–81. doi: 10.1016/j.ceb.2009.03.007
95. Vlassov AV, Magdaleno S, Setterquist R, Conrad R. Exosomes: current knowledge of their composition, biological functions, and diagnostic and therapeutic potentials. Biochim Biophys Acta. (2012) 1820:940–8. doi: 10.1016/j.bbagen.2012.03.017
96. Ha D, Yang N, Nadithe V. Exosomes as therapeutic drug carriers and delivery vehicles across biological membranes: current perspectives and future challenges. Acta Pharm Sin B. (2016) 6:287–96. doi: 10.1016/j.apsb.2016.02.001
97. De Toro J, Herschlik L, Waldner C, Mongini C. Emerging roles of exosomes in normal and pathological conditions: new insights for diagnosis and therapeutic applications. Front Immunol. (2015) 6:203. doi: 10.3389/fimmu.2015.00203
98. Rana S, Yue S, Stadel D, Zöller M. Toward tailored exosomes: the exosomal tetraspanin web contributes to target cell selection. Int J Biochem Cell Biol. (2012) 44:1574–84. doi: 10.1016/j.biocel.2012.06.018
99. Pardridge WM. Drug transport across the blood-brain barrier. J Cereb Blood Flow Metab. (2012) 32:1959–72. doi: 10.1038/jcbfm.2012.126
100. Kalluri R, LeBleu VS. The biology, function, and biomedical applications of exosomes. Science. (2020) 367:aau6977. doi: 10.1126/science.aau6977
101. Ahmadi M, Rezaie J. Ageing and mesenchymal stem cells derived exosomes: molecular insight and challenges. Cell Biochem Funct. (2021) 39:60–6. doi: 10.1002/cbf.3602
102. Kalani A, Tyagi A, Tyagi N. Exosomes: mediators of neurodegeneration, neuroprotection and therapeutics. Mol Neurobiol. (2014) 49:590–600. doi: 10.1007/s12035-013-8544-1
103. Haney MJ, Klyachko NL, Zhao Y, Gupta R, Plotnikova EG, He Z, et al. Exosomes as drug delivery vehicles for Parkinson's disease therapy. J Control Release. (2015) 207:18–30. doi: 10.1016/j.jconrel.2015.03.033
104. Fraser KB, Rawlins AB, Clark RG, Alcalay RN, Standaert DG, Liu N, et al. Ser(P)-1292 LRRK2 in urinary exosomes is elevated in idiopathic Parkinson's disease. Mov Disord. (2016) 31:1543–50. doi: 10.1002/mds.26686
105. Lotvall J, Valadi H. Cell to cell signalling via exosomes through esRNA. Cell Adh Migr. (2007) 1:156–8. doi: 10.4161/cam.1.3.5114
106. Smalheiser NR. Exosomal transfer of proteins and RNAs at synapses in the nervous system. Biol Direct. (2007) 2:35. doi: 10.1186/1745-6150-2-35
107. Record M, Subra C, Silvente-Poirot S, Poirot M. Exosomes as intercellular signalosomes and pharmacological effectors. Biochem Pharmacol. (2011) 81:1171–82. doi: 10.1016/j.bcp.2011.02.011
108. Lin J, Li J, Huang B, Liu J, Chen X, Chen XM, et al. Exosomes: novel biomarkers for clinical diagnosis. ScientificWorldJournal. (2015) 2015:657086. doi: 10.1155/2015/657086
109. Sarko DK, McKinney CE. Exosomes: origins and therapeutic potential for neurodegenerative disease. Front Neurosci. (2017) 11:82. doi: 10.3389/fnins.2017.00082
110. Frühbeis C, Fröhlich D, Kuo WP, Amphornrat J, Thilemann S, Saab AS, et al. Neurotransmitter-triggered transfer of exosomes mediates oligodendrocyte-neuron communication. PLoS Biol. (2013) 11:e1001604. doi: 10.1371/journal.pbio.1001604
111. Frühbeis C, Fröhlich D, Kuo WP, Krämer-Albers EM. Extracellular vesicles as mediators of neuron-glia communication. Front Cell Neurosci. (2013) 7:182. doi: 10.3389/fncel.2013.00182
112. de Rivero Vaccari JP, Brand F, Adamczak S, Lee SW, Perez-Barcena J, Wang MY, et al. Exosome-mediated inflammasome signaling after central nervous system injury. J Neurochem. (2016) 136(Suppl.1):39–48. doi: 10.1111/jnc.13036
113. Bianco F, Perrotta C, Novellino L, Francolini M, Riganti L, Menna E, et al. Acid sphingomyelinase activity triggers microparticle release from glial cells. EMBO J. (2009) 28:1043–54. doi: 10.1038/emboj.2009.45
114. Kettenmann H, Hanisch UK, Noda M, Verkhratsky A. Physiology of microglia. Physiol Rev. (2011) 91:461–553. doi: 10.1152/physrev.00011.2010
115. Iero M, Valenti R, Huber V, Filipazzi P, Parmiani G, Fais S, et al. Tumour-released exosomes and their implications in cancer immunity. Cell Death Differ. (2008) 15:80–8. doi: 10.1038/sj.cdd.4402237
116. Théry C, Ostrowski M, Segura E. Membrane vesicles as conveyors of immune responses. Nat Rev Immunol. (2009) 9:581–93. doi: 10.1038/nri2567
117. Chen HX, Liang FC, Gu P, Xu BL, Xu HJ, Wang WT, et al. Exosomes derived from mesenchymal stem cells repair a Parkinson's disease model by inducing autophagy. Cell Death Dis. (2020) 11:288. doi: 10.1038/s41419-020-2473-5
118. De Virgilio A, Greco A, Fabbrini G, Inghilleri M, Rizzo MI, Gallo A, et al. Parkinson's disease: autoimmunity and neuroinflammation. Autoimmun Rev. (2016) 15:1005–11. doi: 10.1016/j.autrev.2016.07.022
119. Jagadeesan AJ, Murugesan R, Vimala Devi S, Meera M, Madhumala G, Vishwanathan Padmaja M, et al. Current trends in etiology, prognosis and therapeutic aspects of Parkinson's disease: a review. Acta Biomed. (2017)88:249–62.
120. Reich SG, Savitt JM. Parkinson's disease. Med Clin North Am. (2019) 103:337–50. doi: 10.1016/j.mcna.2018.10.014
121. Obeso JA, Stamelou M, Goetz CG, Poewe W, Lang AE, Weintraub D, et al. Past, present, and future of Parkinson's disease: a special essay on the 200th Anniversary of the Shaking Palsy. Mov Disord. (2017) 32:1264–310. doi: 10.1002/mds.27115
122. Welker MW, Reichert D, Susser S, Sarrazin C, Martinez Y, Herrmann E, et al. Soluble serum CD81 is elevated in patients with chronic hepatitis C and correlates with alanine aminotransferase serum activity. PLoS ONE. (2012) 7:e30796. doi: 10.1371/journal.pone.0030796
123. Ding Y, Cao F, Sun H, Wang Y, Liu S, Wu Y, et al. Exosomes derived from human umbilical cord mesenchymal stromal cells deliver exogenous miR-145-5p to inhibit pancreatic ductal adenocarcinoma progression. Cancer Lett. (2019) 442:351–61. doi: 10.1016/j.canlet.2018.10.039
124. Sahebi R, Langari H, Fathinezhad Z, Bahari Sani Z, Avan A, Ghayour Mobarhan M, et al. Exosomes: new insights into cancer mechanisms. J Cell Biochem. (2020) 121:7–16. doi: 10.1002/jcb.29120
Keywords: mesenchymal stem cells, exosomes, Parkinson's disease, treatment, miRNA
Citation: Liu S-f, Li L-y, Zhuang J-l, Li M-m, Ye L-c, Chen X-r, Lin S and Chen C-n (2022) Update on the application of mesenchymal stem cell-derived exosomes in the treatment of Parkinson's disease: A systematic review. Front. Neurol. 13:950715. doi: 10.3389/fneur.2022.950715
Received: 23 May 2022; Accepted: 07 July 2022;
Published: 03 October 2022.
Edited by:
Hailiang Tang, Fudan University, ChinaReviewed by:
Jafar Rezaie, Urmia University of Medical Sciences, IranMercedes Azucena Hernandez Sapiens, CONACYT Centro de Investigación y Asistencia en Tecnología y Diseño del Estado de Jalisco (CIATEJ), Mexico
Copyright © 2022 Liu, Li, Zhuang, Li, Ye, Chen, Lin and Chen. This is an open-access article distributed under the terms of the Creative Commons Attribution License (CC BY). The use, distribution or reproduction in other forums is permitted, provided the original author(s) and the copyright owner(s) are credited and that the original publication in this journal is cited, in accordance with accepted academic practice. No use, distribution or reproduction is permitted which does not comply with these terms.
*Correspondence: Chun-nuan Chen, Y2hlbmNodW5udWFuMTk4M0BhbGl5dW4uY29t; Shu Lin, c2h1bGluMTk1NkAxMjYuY29t
†These authors have contributed equally to this work and share first authorship