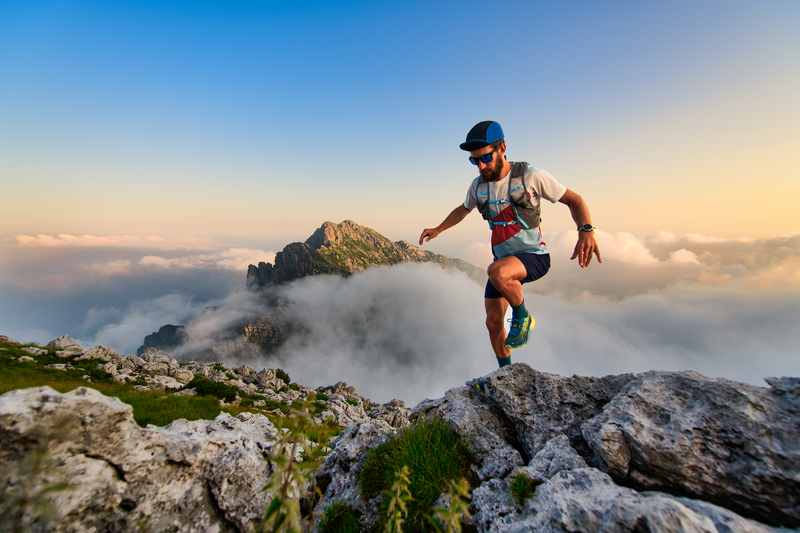
95% of researchers rate our articles as excellent or good
Learn more about the work of our research integrity team to safeguard the quality of each article we publish.
Find out more
REVIEW article
Front. Neurol. , 09 August 2022
Sec. Experimental Therapeutics
Volume 13 - 2022 | https://doi.org/10.3389/fneur.2022.909264
This article is part of the Research Topic Deep Brain Stimulation for Neuropsychiatric Disorders: Current Status and Perspectives View all 9 articles
Early research into neural correlates of obsessive compulsive disorder (OCD) has focused on individual components, several network-based models have emerged from more recent data on dysfunction within brain networks, including the the lateral orbitofrontal cortex (lOFC)-ventromedial caudate, limbic, salience, and default mode networks. Moreover, the interplay between multiple brain networks has been increasingly recognized. As the understanding of the neural circuitry underlying the pathophysiology of OCD continues to evolve, so will too our ability to specifically target these networks using invasive and noninvasive methods. This review discusses the rationale for and theory behind neuromodulation in the treatment of OCD.
Obsessive-compulsive disorder (OCD) is a disabling psychiatric condition that affects ~2% of the population. The disorder is characterized by the presence of obsessions, compulsions, or both, which are time consuming (>1 h/day) and cause significant distress and impairment in important areas of functioning (1). Obsessions are persistent, unwanted thoughts or images that cause distress and anxiety, and compulsions are repetitive behaviors or mental rituals that are aimed to neutralize the distress and anxiety. First line treatments for OCD are cognitive behavioral therapy (CBT) with exposure and response prevention (ERP) and selective serotonin reuptake inhibitors (SSRIs). Additional pharmacotherapy includes the tricyclic antidepressant (TCA) clomipramine as well as augmentation with neuroleptics. Even among those treated with these evidence-based psycho- and pharmacotherapies, approximately one third of patients have refractory disease with continued disabling symptoms. Given this ongoing and significant disease burden, optimized and novel therapies are needed. Toward this end, various neuromodulation approaches, such as transcranial magnetic stimulation (TMS), transcranial direct current stimulation (tDCS), transcranial alternating current stimulation (tACS), and deep brain stimulation (DBS), have shown efficacy in improving OCD symptom burden in this otherwise treatment-refractory population. This review discusses the rationale for and theory behind neuromodulation in the treatment of OCD.
It is important to underscore that all novel neuromodulatory treatments must be firmly rooted in hypotheses relating to the neurobiological underpinnings of OCD. While early research into OCD focused largely on psychological mechanisms, the development and evolution of modern neuroimaging techniques has advanced our understanding of its neurophysiological underpinnings. Structural techniques such as voxel-based morphometry, surface-based cortical thickness, and tractography allow for the study of gray matter volume and integrity of white matter tracts. Functional imaging techniques such as positron emission topography (PET) and functional magnetic resonance imaging (fMRI) provide indirect measurements of brain activity in regions of interest and functional connectivity across nodes within and between brain networks. Direct methods of measuring brain activity include single unit recording, local field potentials, electrocorticography, and electroencephalography, the latter also being able to measure connectivity between cortical regions in particular frequency bands. Combined, these techniques have contributed greatly to our understanding of circuit dysfunction in OCD.
Current theories of OCD pathophysiology posit dysfunction in several distinct, yet overlapping brain circuits that involve connections from cortex to basal ganglia to thalamus and reciprocally back to cortex, known as cortico-striato-thalamo-cortical loops (CSTC) (2, 3). A number of distinct networks within this pathway were described by Alexander et al. (4). based on anatomophysiological findings and have subsequently been validated based on functional connectivity maps (4–6). However, the number of distinct networks has varied among descriptions, and the degree of topographical segregation between circuits remains an area of active research. Several CSTC circuits have been implicated in OCD based on clinical symptom clusters (i.e., obsessions about contamination compared to obsessions about symmetry), structural and functional neuroimaging, results from animal models, and patient response to treatment. Here we provide an overview of this evidence as it pertains to CSTC dysfunction in OCD.
One of the earliest circuits implicated in OCD is the lateral orbitofrontal-ventromedial caudate pathway. As described by Alexander et al. (4), neurons in the lateral orbitofrontal cortex (lOFC) project to the ventromedial caudate, dorsomedial globus pallidus internus (GPi), and ventral anterior thalamus before projecting back to the lOFC (4). The role of the lOFC in behavior adaption and reversal learning is well-established (7–9). It has been postulated that dysfunction within the lOFC-ventromedial caudate circuits underlie persistence of habit-driven over goal-directed behavior (10). Similarly, the caudate is known to be involved in learning and goal-directed behavior, including a critical role in habit formation, and is thought to be hyperactive in OCD (11). These hypotheses are supported by studies showing increased gray matter volume, metabolism/activation (as measured by PET and fMRI), and functional connectivity in the lOFC, caudate and lOFC-striatal pathway, correlated with symptoms (12–15). Work from animal studies has demonstrated increased single-unit activity in the IOFC in pharmacological models of OCD, and OCD-like symptoms in mice after optogenetic stimulation of OFC-ventromedial striatum (16, 17). Interestingly, successful pharmacological or psychological treatment of OCD leads to normalization of OFC and caudate nucleus activity, again supporting a hypothesis that these structures are involved in the pathogenesis of OCD symptoms (18).
The limbic network is principally involved in emotion, reward-motivated behavior, learning, and memory. The structures considered part of the limbic network have been updated over time as novel evidence suggests roles for various brain regions (19). Generally accepted components include the hippocampus, entorhinal cortex, fornix, mammillary bodies, anterior nucleus of the thalamus (ANT), anterior cingulate cortex (ACC), medial prefrontal cortex, amygdala, septal nuclei, nucleus accumbens (NAcc), and hypothalamus (20, 21). In terms of a CSTC loop, the ventral striatum receives inputs from the medial OFC (mOFC), ventromedial prefrontal cortex (vmPFC) and the dorsal anterior cingulate cortex (dACC), and in turn projects to the ventral pallidum and mediodorsal nucleus of the thalamus (Figure 1). These structures are heavily regulated by dopaminergic input, which serves as an expectation/outcome error signaling mechanism to facilitate learning and memory. Dysregulation of the limbic system has been implicated in a number of models of OCD pathogenesis, including increased classical conditioning of compulsions; reduced modulation of a frontal-based habit system by hippocampal-based memory; and a deficit of implicit learning with compensation by explicit learning (22–24). Indeed, patients with OCD show reduced task-based reward learning, correlated with reduced activity in, and connectivity between, limbic structures such as the NAcc, ventral putamen, vmPFC, OFC, hippocampus, and amygdala (15, 24–27). During episodes of increased symptomatology there is hyperactivation in limbic structures (28, 29). There is a discrepancy in resting state functional connectivity between medicated and unmedicated OCD patients, with increased connectivity found in medicated OCD patients, and reduced connectivity, both within the limbic system and in other networks, in unmedicated patients (30, 31). While the latter may reflect deficits in reward processing and learning, there may be measurable changes in network connectivity due to medication. Further supporting this hypothesis, serotonin-selective reuptake inhibitors (SSRIs) have been shown to increase frontostriatal connectivity in patients with depression and pediatric patients with OCD (32, 33).
Figure 1. A functional circuit diagram depicting relevant afferent and efferent connectivity in the cortico-striatal-cortical and orbitofrontal circuits, implicated in the pathogenesis of obsessive compulsive disorder (OCD).
Termed the “task-negative” network, the default mode network is involved in internal mentation. In early studies using PET, decreased activity of the medial prefrontal cortex, lateral parietal cortex, posterior cingulate cortex (PCC), and precuneus was observed during externally orientated tasks with subsequent increased activity at rest, defining this network (34). However, a number of other structures, such as the medial temporal lobe, have been implicated in internal mentation, and it has been proposed that the default mode network may be comprised of multiple related networks (35). The default mode network has been the object of study in a number of neurocognitive and psychiatric conditions such as major depressive disorder and Alzheimer's disease. In OCD, dysfunction in the default mode network is thought to underlie increased self-referential attention to persistent thoughts. Consistent with this, individuals with OCD have been found to have increased task-based functional connectivity in the default mode network (36). Decreased PCC activation and increased PCC-vmPFC functional connectivity were found in OCD patients during reward processing (37). This finding corroborates evidence of reward processing deficits and limbic network dysfunction, while also suggesting a shift of attention away from external rewards toward internal thoughts through an inability to deactivate the default mode network. While findings vary between studies, meta-analysis of resting-state functional connectivity data has shown aberrant connectivity within the default mode network, and hypoconnectivity with the frontoparietal and salience networks, suggesting an abnormal balance between self-referential and task-related attention (38). After SSRI therapy, a normalization of default mode network hypoconnectivity with other “task-positive” networks, e.g., the dorsal attention network, has been demonstrated (39).
Although involved in a wide range of functions, the salience network has been particularly implicated in attention switching, response, and response inhibition. Specifically, it has been postulated to regulate the transition between the task-based frontoparietal executive control network and task-negative default mode network. Also referred to as the cinguloopercular, cinguloinsular or ventral attention network, the salience network consists of two principal structures, the dACC and anterior insula, in addition to a CSTC loop containing the dlPFC, dorsolateral caudate, and mediodorsal nucleus of the thalamus (4, 5, 40). Modulation of activity in these areas has been shown during tasks involving salient stimuli, error awareness, and uncertainty (41–43). Consistent with the role of the salience network and its components, it has been proposed that dysfunction of this network can lead to aberrant processing of stimuli, attentional control, physiological reactions, and motor response (44). In patients with OCD, there is increased activity in the anterior insula, dorsal caudate, and dACC in task-based protocols, with this activity positively correlating with symptoms (32, 45–47). In rodent models of OCD, lesioning of the anterior insula decreases compulsivity (48). Furthermore, meta-analysis of resting-state functional connectivity in OCD has demonstrated hypoconnectivity not only within the salience network, but between the salience network, default mode network, and frontoparietal networks (38). Consistent with the interplay between these three networks, in OCD there is thought to be aberrant processing of salient stimuli and an inability to shift attention away from internal obsessions mediated by the default mode network toward goal-directed behavior mediated by the frontoparietal network (44).
Long before advanced imaging studies and animal studies helped to elucidate the neural circuitry involved in OCD, clinicians have sought to provide brain-based treatments for this disorder. Early strategies implementing prefrontal lobotomies and leukotomies showed some promise in alleviating symptoms; however, the side effects were significant (49). With the dawn of stereotactic surgery, more specific lesioning strategies such as cingulotomies and anterior capsulotomies showed more promising results with more tolerable side effects (50). As more effective pharmacotherapies became available with even fewer adverse effects than the more precise surgical techniques, the interest in surgical management waned.
Around this same time, electroconvulsive therapy (ECT) also emerged as a less invasive strategy in the treatment of a variety of psychologic disorders. The results for ECT in OCD have thus far been inconsistent with some studies showing benefit while others showed no improvement, but this strategy identified a clear role of applying external fields to the brain in order to influence neural networks (51). The lessons learned from early surgical lesioning and applying electric fields to the brain were combined with emerging work in understanding the neurologic aberrations in OCD, laying the groundwork for more specific neuromodulatory strategies like transcranial magnetic stimulation (TMS), transcranial direct current stimulation (tDCS), transcranial alternating current stimulation (tACS), and deep brain stimulation (DBS) (Table 1, Figure 2).
Figure 2. Targets for both transcranial magnetic stimulation (TMS, highlighted in green to the left) and deep brain stimulation (DBS, highlighted in blue to the right) implicated in the treatment of obsessive compulsive disorder. TMS targets include the supplementary motor area (SMA), dorsolateral prefrontal cortex (dlPFC), and orbitofrontal cortex (OFC). DBS targets include the anterior limb of the internal capsule (ALIC), basal nucleus of the stria terminalis (BNST), nucleus accumbens (NAcc), subthalamic nucleus (STN), medial forebrain bundle (MFB), and inferior thalamic peduncle (ITP). The anterior cingulate cortex (aCC) is a target of both TMS and DBS.
TMS is a non-invasive neuromodulation technique that has shown efficacy in the treatment of a variety of neurologic and psychiatric conditions and has FDA-approval for treatment-refractory major depressive disorder, anxiety associated with depression, treatment-refractory OCD, smoking cessation, and migraine with aura (52). Mechanistically, a brief but strong magnetic field is generated by transiently passing electricity through wire windings of various configurations contained within the stimulation coil, which is placed against the head. This magnetic field passes through the scalp and skull to induce an electric field within the underlying brain region, causing depolarization of neurons. This process is repeated at varying frequencies, and in this way, TMS can alter electrical activity within the targeted brain region.
A variety of stimulation protocols have been studied, though they generally fall into three groups: high frequency, low frequency, and theta burst. High frequency stimulation (pulse repetition rates between 5 and 20 Hz) increases cortical excitability while low frequency stimulation (1 Hz) tends to decrease cortical excitability (52–54). Similarly, it is hypothesized that high frequency TMS influences behavioral change through long-term potentiation (LTP) while low frequency TMS initiates long-term depression (LTD) (55, 56). Through these mechanisms, the effects of stimulation on cortical excitability persist beyond the duration of the treatment. A recently introduced stimulation pattern known as theta burst stimulation (TBS), first described in human motor cortex by Huang et al. (57), delivers pulses in a theta frequency band (3 pulses at 50 Hz repeated at 5 Hz) to mimic endogenous brain rhythms. TBS can also induce either excitatory effects—if delivered intermittently (intermittent TBS, iTBS)—or inhibitory effects—if delivered continuously (continuous TBS, cTBS)—and allows for shorter treatment times and lower treatment intensities, which are generally better tolerated by patients.
As discussed previously, there are abnormalities in signaling in multiple anatomic structures and neural networks in OCD, and these pathways provide a variety of targets for TMS. There have been a number of randomized control trials that have investigated the effectiveness of TMS in treating OCD by targeting the DLPFC (58–70), the SMA (71–77), the OFC (78, 79), and the mPFC (80, 81). However, there is notable heterogeneity in these studies in terms of number of participants, stimulation frequency, number of treatments, length of follow-up, and efficacy in reducing OCD symptom burden. Several recent systematic reviews and meta-analyses have been conducted to assess the overall evidence for the use of TMS in treating OCD (82–87). The meta-analysis by Liang et al. found that targeting the right DLPFC with low frequency stimulation had the greatest effect size, while low frequency stimulation of the SMA and high frequency stimulation of the DLPFC were also more effective than sham (86). Notably, the authors find the overall quality of this evidence to be very low to low, based on the GRADE framework. These results align with recent treatment guidelines which suggest “possible efficacy of low frequency rTMS of the right DLPFC” (52). The meta-analysis by Perera et al., which included 26 total studies, demonstrated that targeting of the bilateral DLPFC with either high or low frequency stimulation has the greatest effect size (87). While these meta-analyses and treatment guidelines generally indicate the DLPFC as the preferred target for rTMS in OCD, in a large sham-controlled randomized trial, Carmi et al. (81) found significant improvement in OCD symptoms as measured by the Yale-Brown Obsessive Compulsive Scale (Y-BOCS) with high frequency, deep rTMS of the mPFC (81, 88). Based on the results of this trial, the FDA has approved TMS for treatment-refractory OCD.
Several questions have arisen from these prior studies. Perhaps most prominent is which cortical region should be targeted for an individual patient, given the heterogeneity of OCD symptoms, neural circuit dysfunction, degree of symptom burden, and presence of co-morbid psychiatric disorders (89). Imaging studies have shown that distinct neural circuits are differentially activated in patients with different OCD symptoms (90). One approach that may improve treatment outcomes is the selection of the cortical stimulation target based on personalized symptom profile, neuroanatomy, and/or circuit dysfunction. Similar approaches have shown promise for improving treatment outcomes in TMS for depression and DBS for OCD (91–93).
Another important question in the administration of TMS is whether brain state during stimulation has implications for treatment outcome. Few studies have examined this question and most current clinical use of TMS is independent of brain state, with patients permitted to engage in any variety of tasks during treatment (watching movies, listening to music, utilizing cell phones, etc.). Broadly, state-based TMS has been examined in a variety of conditions and generally been shown to be effective: in bulimia (94) food cues were presented prior to stimulation; in PTSD (95) trauma cues were presented prior to stimulation; in nicotine use disorder (96) smoking cues were presented prior to stimulation; and in depression (97) stimulation was coupled with CBT. Within OCD, two RCT's have addressed state-dependence by utilizing symptom-provocation immediately prior to TMS treatment; both studies and found that HF mPFC stimulation coupled with provocation led to an improvement in OCD symptom burden (80, 81). Overall, this is an important avenue of future research and various stimulation locations in OCD may show effectiveness if coupled with brain circuit activation prior to or during stimulation.
Taken together, there is evidence from a number of studies that TMS applied to a variety of cortical sites, either with or without symptom-provocation, can improve OCD symptom burden. Future studies should examine such outstanding questions as: (1) Might different cortical stimulation targets improve specific OCD symptoms (e.g., harm-based vs. non harm-based obsessions); (2) does underlying patient-specific circuit dysfunction impact efficacy of cortical stimulation targets; and (3) does concurrent brain state (governed by symptom-provocation or engagement in psychotherapy) impact TMS efficacy. Other future studies utilizing TMS in OCD should seek to gain a better understanding of the pathophysiologic mechanisms in order to design more specific neuromodulatory strategies. A good example of how this may be accomplished comes from the study of Alzheimer's Disease (AD). Di Lorenzo and colleagues investigated spike timing dependent cortico-cortical plasticity (STDP) in AD patients by applying timed stimulation to the posterior parietal cortex then the primary motor cortex or vice versa and studying motor-evoked potentials (98). With this study, they were able to determine that STDP was inhibited in AD patients when compared to healthy controls. This study highlights the role of TMS as a tool to aid in our understanding of networks and the pathophysiologic mechanisms underlying disease. Similar experiments with TMS could be applied in the study of OCD.
tDCS is another non-invasive neuromodulation strategy that has been studied in the treatment of OCD. In delivering tDCS, a weak direct current is conducted across the brain between two electrodes (99). Cathodal stimulation is thought to be inhibitory while anodal stimulation is thought to have excitatory effects (99). Typical stimulation parameters used in the literature are 1–2 mA delivered for 20–30 min, and patients typically undergo repeat treatments for 10–20 treatments. Numerous studies have investigated the effects of tDCS in the treatment of refractory OCD. The targets selected were the same as those used for TMS, including pre-SMA/SMA, mPFC, DLPFC, and OFC (84).
There have been several randomized control trials that have targeted the pre-SMA/SMA (100–102), the mPFC (103, 104), OFC (105). D'Urso et al. (100) found that cathodal but not anodal stimulation of the bilateral pre-SMA elicited improvement in OCD symptoms, while Gowda et al. (101) showed anodal stimulation resulted in improvement when compared to sham. Todder et al. found that tDCS to the mPFC showed a decrease in obsession-induced anxiety (103). In another study looking into tDCS to the mPFC, Adams et al. showed that anodal stimulation showed significant improvement in therapeutic safety learning. Interestingly using functional imaging, they showed that functional connectivity increased between the frontal pole and the middle and superior frontal gyri, while they found that the functional connectivity between the insula and the basal ganglia decreased as did the functional connectivity between the DMN and salience networks (104). Bation et al. showed that left anodal stimulation of the left OFC had short term effects but failed to show significant improvement during a 3 month follow up (105). Overall, these studies all showed the feasibility and efficacy of tDCS in targeting multiple brain regions. Larger randomized control trials are needed to define the optimal target and treatment parameters, but tDCS is an efficacious, non-invasive neuromodulatory modality that extends the armamentarium of treatment for refractory OCD.
Another modality that has shown some promise in the treatment of refractory OCD is tACS. tACS uses sine wave stimulation at the desired frequency range. tACS protocols employ much less stimulation intensity than tDCS (0.6 mA vs. 2 mA) (106). There have been few studies into using tACS for the treatment of OCD. In a small case series, Klimke et al. showed that tACS to the bilateral DLPFC was effective in improving OCD symptoms in 6 out of 7 patients with an average decrease in the Y-BOCS score by 52% (107). In a recent study by Grover et al. using high definition tACS to the mOFC, they found that patient-specific beta-gamma modulation influences reward- but not punishment-based learing and behaviors when compared to sham, and these protocols were found to decrease obsessive-compulsive symptoms in patients with sub-clinical obsessive compulsive symptomatology (108). The early results in tACS for OCD have shown feasibility and promise, but more work is needed to optimize this modality.
The 1930s saw the expansion of psychosurgery with prefrontal leucotomies and, after the development of the stereotactic frame in the 1940s, more targeted lesioning, including capsulotomy, cingulotomy, and subcaudate tractotomy came into favor. These destructive techniques aim to downregulate activity in individual structures or neural pathways that have been implicated in the neurocircuitry of OCD. While anterior capsulotomy and cingulotomy are still used today, ablative techniques have largely been replaced by neuromodulation, including deep brain stimulation (DBS) and vagus nerve stimulation (VNS). Unlike ablative procedures, neuromodulation is reversible and modifiable. As the only Food and Drug Administration (FDA)-approved form of invasive neuromodulation for OCD, DBS utilizes pulsed electricity at a specified pulse width, voltage, and frequency to modulate the activity of deep target structures (109–111).
As the pathogenesis of OCD becomes better understood as being due to dysfunctional brain circuitry, the regions targeted with DBS have evolved to specifically influence the neural pathways comprising these networks (112). The most common targets of DBS for OCD include the anterior limb of the internal capsule (ALIC), nucleus accumbens (NAcc), bed nucleus of the stria terminalis (BNST), subthalamic nucleus (STN), and inferior thalamic peduncle (ITP). Treatment response is most frequently evaluated with the percent decrease of Y-BOCS, with secondary outcome measures including the Hamilton Depression Rating Scale (HAM-D) and (Hamilton Anxiety Ranting Scale (HAM-A) scores (113, 114). When using Y-BOCS to evaluate treatment response, a cutoff of 35% decrease of symptoms is typically used to classify subjects as responders vs. non-responders (115).
The anterior limb of the internal capsule (ALIC) connects subcortical nuclei with the prefrontal cortex (116). It has been implicated in numerous psychiatric disorders and is targeted with lesioning surgery via anterior capsulotomy as well as modulation by DBS. The therapeutic mechanism of these modalities is likely through interrupting the fibers traversing the ALIC and thus decreasing overactivity of the thalamocortical circuits thought to underlie OCD pathophysiology (117). The first study of DBS of the ALIC by Nuttin et al. was performed based on known targets of capsulotomy (118). In this non-blinded interventional cohort study, four patients received bilateral stimulation of the ALIC in locations per prior ablative protocols. Three of the four patients improved. Since then, there have been several studies demonstrating the effectiveness of ALIC stimulation for OCD (119–122).
A recent study of 70 patients by Denys et al. demonstrated effectiveness of ventral ALIC (vALIC) stimulation for OCD (121). This non-blinded cohort study showed an average Y-BOCS decrease of 40% with 62% of subjects being classified as responders. Within the responder group there was a 62% decrease of OCD symptoms as measured by Y-BOCS. The authors found that Y-BOCS decreased initially with standardized stimulation, then continued to decrease over the following year. The improvement in symptom reduction over time may have been due to optimization and personalization of stimulation parameters. The subjects' HAM-A and HAM-D scores decreased by 55 and 54%, respectively. The reduction in HAM-A and HAM-D scores was stable throughout the study, that is, the scores did not continue to decrease over the following year. The study was limited to 1 year and thus did not evaluate long-term results of ALIC DBS for OCD.
Within the ALIC, there have been investigations of whether individual fiber bundles could be targeted for optimal benefit. Indeed, Liebrand et al. found that targeted stimulation of the ALIC in closer proximity to the medial forebrain bundle (MFB) (rather than the anterior thalamic radiation), had a significant therapeutic benefit (123). Other groups have described methods to delineate and target individual tracts and predict treatment response, and the topic is still in debate, which will be discussed further (124–127). Additionally, several groups have noted clinical signs during awake lead placement and programming which are associated with better outcomes and may reflect proximity to specific fiber bundles. The most common of these is the “mirth response,” in which patients are noted to smile and laugh during lead placement and stimulation (128, 129).
Despite good response and remission rates of ALIC stimulation, its clinical usefulness is somewhat constrained by the high stimulation intensity required for beneficial results (84, 119). Most clinical studies show effectiveness with ALIC stimulation only after extensive programming and with amplitudes of 5–10 V. Compared to DBS for movement disorders (Parkinson's disease, essential tremor, dystonia), amplitudes at this intensity necessitate frequent replacement of the implantable-pulse-generator.
Adverse effects of ALIC stimulation include hypomania (including restlessness, agitation, and impulsivity). Denys et al. found that these symptoms were often correlated with stimulation changes and were typically transient (121). Other adverse effects include headaches, anxiety, and rarely, seizures (122). Additionally, some patients report transient stimulation-induced facial muscle contraction (119).
The nucleus accumbens (NAcc), known to play a significant role in reward behavior, is located in the basal forebrain ventral to the ALIC and rostral to the anterior commissure. The NAcc makes up the ventral striatum with the olfactory tubercle. The ventral capsule ventral striatum (VC/VS) consists of the ventral portion of the anterior limb of the internal capsule as well as the adjacent ventral striatum. Its use as a DBS target was based on the hypothesis that OCD is due to a dysfunctional reward pathway in the context of successful anterior capsulotomy results for the treatment of OCD (24, 130). DBS targeting the NAcc reduces excessive connectivity between the NAcc and the prefrontal cortex, as measured by EEG and fMRI (110).
Distinct regions in the NAcc may play separate functional roles, as they receive input from different areas in the brain. The medial aspect and the shell of the NAcc receive input from the rostral part of the anterior cingulate cortex (131). This innervation occurs through multiple white matter tracts, including the amygdalofugal tract between the NAcc and anterior SCG. This tract originates from the basolateral and central nuclei of the amygdala before passing under the lentiform nucleus. It then runs medially alongside and underneath the anterior commissure and internal capsule before dividing into ascending and descending branches. The ascending branch of the amygdalofugal tract courses through the NAcc, then to the anterior SCG, before terminating in the septal nuclei.
There have been several successful trials of DBS of the NAcc and VC/VS for the treatment of OCD (120, 132–134). Sturm et al. performed unilateral and bilateral high-frequency stimulation of the NAcc in four patients (132). In this study, DBS electrodes were placed at the junction of the ventral internal capsule (VIC) with the NAcc. Their group found that bilateral stimulation did not show more OCD symptom reduction compared to unilateral stimulation of the right NAcc in the first patient, so further patients were treated with unilateral stimulation of the right NAcc. They noted a significant reduction in OCD symptoms in three out of four patients studied.
Denys et al. performed double-blinded bilateral NAcc stimulation in sixteen patients (133). In this study, bilateral electrodes were placed in the NAcc following the angle of the ALIC. They found that Y-BOCS decreased by 52% after stimulation. Nine out of sixteen patients were considered responders, with a mean improvement in obsessive-compulsive symptoms of 72% within the group. Additionally, depressive and anxiety scores decreased by 55 and 57%, respectively, as measured by HAM-D and HAM-A scores. Of note, stimulation-induced elevated mood was seen in all patients in this study, even those who did not respond in terms of their OCD symptoms. In a multi-site clinical trial of 26 patients, Greenberg et al. also found significant reduction in co-morbid depression and anxiety after VC/VS stimulation (134). In this study, there was a 40 and 53% decrease in HAM-D and HAM-A scores, respectively.
There are several potential adverse effects and complications of NAcc and VC/VS stimulation, including mood disturbances (134). Mood elevation is most often seen with increased stimulation, with some subjects progressing to hypomania. Mood depression has been seen when stimulation is decreased or turned off. In patients with co-morbid depression, abrupt cessation of stimulation can lead to return to pre-DBS levels of depression. Additionally, while anxiety is often improved with stimulation, there have been reports of transiently increased anxiety.
The subthalamic nucleus (STN) is a common target for neuromodulation in Parkinson's Disease as it plays a role in both behavioral as well as motor control. The topographic localization of emotional vs. motor control has been demonstrated through stimulation of sub-regions of the STN, with the anteromedial portion being specifically implicated in emotional control (135). Because the STN is widely utilized as a target in deep brain stimulation for Parkinson's disease, its stimulation was somewhat incidentally found to have therapeutic effects for OCD management in patients undergoing DBS with co-morbid OCD and Parkinson's disease (136).
Mallet et al. examined bilateral stimulation of the anteromedial subthalamic nucleus (137). In this randomized, double-blinded study of eight patients, six out of the eight patients responded to STN stimulation as measuring by Y-BOCS improvement, with an average Y-BOCS decrease of 37% after the stimulation period vs. 7% after the sham period. Notably, this study found no improvement of depression or anxiety symptoms.
Tyagi et al. compared DBS of the STN with DBS of the VC/VS in a randomized, double-blinded study (138). They found that stimulation of the STN provided similar control of OCD symptoms with Y-BOC decreased 45% in STN compared to 53% in VC/VS. The two targets differed in secondary effects, however. STN stimulation was associated with improved cognitive flexibility as measured by the Cambridge Neuropsychological Test Automated Battery Intra-Extra Dimensional Set-Shift (IED) task, and VC/VS stimulation was not. VC/VS stimulation, on the other hand, demonstrated improved mood symptoms compared to STN stimulation, as measured by the Montgomery-Asberg Depression Rating Scale (MADRS).
One specific drawback of STN stimulation is the comparatively long duration of treatment required to reach the full effect, which can be on the order of years rather than weeks or months for other targets (139). This was outlined in case study by Wojtecki et al. in which full results were not seen until 3 years of stimulation. Adverse motor effects of STN-DBS for OCD can include hyperkinetic movement disorders such as choreiform dyskinesias.
The bed nucleus of the stria terminalis (BNST) is located in the basal forebrain caudal to the anterior commissure and inferomedial to the ALIC (140). It connects to the greater limbic circuitry via the stria terminalis, which wraps around the deep brain structures to connect the BNST to the amygdala. It has been implicated in numerous physiologic processes such as fear, anxiety, and goal-directed behavior. Its use as a target for stimulation was proposed after observing the effects of ALIC stimulation (141).
The first study of BNST stimulation for OCD, a long-term clinical trial of 24 patients published by Luyten et al. showed a significant improvement in Y-BOCS, HAM-D, and HAM-A scores (141). Additionally, this study demonstrated significantly greater effect size when compared with ALIC stimulation. In a further longitudinal study of the same patient cohort, Raymaekers et al. found long-term sustained effects of DBS of the BNST for OCD and co-morbid depression and anxiety (142). A randomized, double-blind, sham-controlled study of bilateral stimulation of the BNST was recently completed by Mosley et al. (143). This study saw a significant reduction of Y-BOCS during the blinded phase, with a mean difference of 4.9 points (15%) comparing on vs. sham. After the blinded phase, the open-label portion of the study saw further improvement at the 1-year mark, with a mean reduction of 17.4 points (47% improvement compared to pre-DBS). The discrepancy between blinded and open-label results is multi-factorial but likely due to a relatively short blinded portion, which took place prior to the open-label portion, with extensive programming optimization taking place during the open-label portion.
Adverse effects of BNST stimulation are generally similar to those of other DBS targets in the basal forebrain, e.g., mood disturbances and agitation. Mosely et al. found two serious adverse events related to DBS device placement, but there were no serious psychiatric events in any patients. However, Luyten et al. noted several subjects with post-implantation seizures leading to study discontinuation (141). These seizures were not confirmed with EEG. The mechanism by which stimulation of the BNST may lead to seizures remains unclear.
The inferior thalamic peduncle is a white matter pathway that travels bidirectionally from the thalamus to the orbitofrontal cortex and connects to the reticular activating system (144, 145). Its use as a target for stimulation for OCD was proposed after considering its prior role in subcaudate tractotomy for OCD control where ITP fibers were lesioned (146).
Studies of ITP stimulation for OCD control have shown significant benefit (144, 145). A preliminary clinical study of five patients by Jimenez et al. showed improved Y-BOCs of 51% (from 35 to 17.8), with these results sustained at 1-year follow up. A phase 1 open-label pilot study of five subjects by Lee et al. demonstrated a good safety profile with average Y-BOCS improvement of 52% across five patients. This clinical improvement was correlated with improvement of metabolic dysfunction in other implicated brain areas, including the right caudate and putamen, right SMA, right cingulum, bilateral motor areas, left temporal pole and left OFC, suggesting extensive metabolic changes associated with ITP stimulation. This study also noted a trend toward co-morbid depression improvement (p = 0.07) but was limited by small sample size.
Like ALIC DBS, ITP stimulation requires high stimulation amplitudes, necessitating frequent battery changes. Additionally, further study is needed to determine whether ITP stimulation improves co-morbid depression and anxiety in OCD patients.
Obtaining a more accurate understanding of the functional networks governing OCD pathophysiology is crucial to identify the optimal targets. Connectomic analyses have sought to define the functional networks and predict treatment response by targeting components of these networks. Balderman et al. found that the connectivity between DBS electrodes and medial and lateral prefrontal cortices strongly predicted outcomes (124, 127). Another connectomic analysis by Li et al. sought to define a network that could explain why targeting the ALIC and the STN both alleviated OCD symptoms, and they found strong connectivity in a bundle that connects the dorsal anterior cingulate and ventrolateral prefrontal cortices to the anteromedial STN, indicating the involvement in this tract (125). Building upon this work, Bouwens van der Vlis et al. sought to find a unifying connectomic target for DBS by correlating outcomes data with tractography data in patients undergoing VC/VS DBS, and they found that the tract that was associated with best outcomes was a subpart of the ALIC that connects the prefrontal cortex with the STN and mediodorsal nucleus of the thalamus (147). In contrast to the other studies, Widge et al. used patient-specific connectomics and statistical modeling in an attempt to predict treatment response, but they found that no statistical model could predict response (148). There is a clear need for more studies into defining the functional networks in OCD and using this information to inform patient-specific targeting.
Vagus nerve stimulation (VNS) is an alternative modality of brain stimulation with FDA approval for treatment-resistant epilepsy and treatment-resistant depression (TRD). VNS typically involves placement of electrodes around the cervical portion of the left vagus nerve with stimulation delivered in an intermittent but chronic manner (149, 150). Through stimulation of this cranial nerve, VNS indirectly modulates the activity of brain networks and has the potential to induce changes in cortical connectivity and excitability (151). The use of VNS in psychiatry has largely been confined to TRD and its efficacy in other psychiatric disorders have been less widely studied. To our knowledge, only one pilot study investigated its potential impact on OCD (152). In this study, seven patients with a diagnosis of treatment-resistant OCD received VNS for a period of 12 weeks. Three of these patients responded acutely during the 12-week period, and two patients demonstrated continued reduction in disease severity for 4 years after implantation. These results warrant further investigation of the efficacy of VNS as an adjunctive treatment for OCD in a larger sample size of participants.
As discussed in this review, there are multiple promising modalities to provide neuromodulation for the treatment of refractory OCD both non-invasively and invasively. DBS is of particular interest because it works to directly modulate the known networks continuously over longer timescales. One of the major limitations in DBS for OCD relates to the quality of evidence. In 2014, the Congress of Neurological Surgeons (CNS) and the American Society for Stereotactic and Function Neurosurgeons (ASSFN) released guidelines for DBS in OCD, and they found that there was only Level I evidence to support bilateral STN DBS over medical management in treatment-refractory OCD (153). The quality of evidence for other targets was level II, and there was inconclusive evidence to inform a best target. Despite considerable research in the field, there were no major updates to the 2020 CNS guidelines as there was no new Level I evidence (154). Similarly, the World Society for Stereotactic and Functional Neurosurgeons (WSSFN) published consensus guidelines on DBS for OCD (155). From their analysis of the literature, they determined that DBS to “ventral anterior capsule region (including bed nucleus of stria terminalis and nucleus accumbens) remains investigational. It represents an emerging, but not yet established therapy.” The guidelines from the two major societies of stereotactic and functional neurosurgeons indicate that there is a clear need for more, high quality evidence to better define targets and optimal parameters for DBS for OCD.
Another limitation in DBS for OCD is that many of the patients who would be candidates for DBS are not getting surgery. In 2009, the FDA granted a Humanitarian Device Exemption (HDE) for DBS to the ALIC in hopes of increasing access to this therapy. Unexpectedly, since the HDE was granted, the number of patients receiving DBS implantation has decreased (156). In a thoughtful analysis into the decline in DBS for OCD, Pinckard-Dover et al. identified the contributing factors (156). They found that at their own institution, there was approximately a 50% decline in DBS for OCD when comparing before and after the HDE was granted. They found that the main reason for this decline was the failure of private insurance companies to provide financial coverage for the procedure. Before the HDE was granted, the cost of the devices was largely covered in the NIH budgets for clinical trials, but afterwards, researchers expected that insurance companies would pick up these costs. This was not the case, and enrollment for clinical trials dwindled as a result. The future of providing this efficacious treatment to patients and the future of continued research will require more buy-in from private insurance companies.
Perhaps the principal issue that remains with respect to DBS is in defining the optimal targets. The targeted areas comprise overlapping regions, leads traverse multiple brain regions, and there is some variability in terms of the coordinates used in the literature. In one of the landmark studies, Greenberg et al. discussed targeting the VC/VS, and they found that better results were achieved with progressively more posterior target (134). This is thought to be because the distal contacts were in the NAcc or BNST. In their systemic review of DBS targets, Raviv et al. they found that there was considerable overlap in the striatal regions (ALIC, NAcc, VC/VS) with some studies using the same coordinates to describe distinct structures while some studies did not even report coordinates (157). They suggest a more careful anatomic consideration, consistency in reporting coordinates, and a change in nomenclature to “strial” vs. STN/pallidal/ITP. They posit that in order to improve OCD DBS outcomes, we must gain a better understanding of the subanatomy of the strial region and use patient-specific connectomic analysis to define the best individualized targets for the individual patient. To this end, in their extensive review of the relevant anatomy of the VC/VS and NAcc, Park et al. found that the caudal part of the NAcc passing through the IC-AC junction may be an effective DBS target for better symptom control (131).
Although the purpose of this article was to highlight neuromodulation, surgical lesioning strategies should be mentioned as well as there have been technological advances that have made this a safer and more precise strategy with comparable outcomes to DBS (158). Cingulotomies and capsulotomies are well-established techniques that have been improved upon over the past several decades, and multiple innovations such as stereotactic navigation, image-guidance, stereotactic radiosurgery, laser interstitial thermal therapy (LITT), and now MR-guided focused ultrasound (MRgFUS) have made lesioning a more viable option (159). Hageman et al. performed a meta-analysis to compare the outcomes for lesioning when compared to DBS for OCD (158). They found that there were equivalent responses and effect sizes. Both DBS and surgical lesioning come with the risk of hemorrhage, infection, suicidality, impulsivity, hypomania, and sleep changes. DBS had a statistically significant increased incidence of impulsivity when compared to ablation. Modern lesioning procedures do have some advantages over invasive neuromodulatory strategies (160). Potential advantages of DBS over lesioning relate to the reversibility of the procedure, and with lesioning, any targeting errors could lead to permanent off-target effects. Although there is no cost-effectiveness analysis to compare DBS vs. lesioning, the cost of the device, the visits to optimize parameters, and generator replacements certainly makes DBS more expensive and cumbersome than lesioning. Cost effectiveness analyses were performed in two international studies (161, 162), but there is a clear need to analyze the costs of DBS in the US. Other disadvantages of DBS relative to ablation is surgical incisions or modifiable device may become an object of obsession (163).
Overall, our opinion is that neuromodulatory strategies in the treatment of OCD are better than lesioning as new technologies will lead to more adaptable, patient-specific outcomes, and they do not involve permanent lesioning of critical brain structures.
Despite some of the limitations in DBS for OCD, it remains a promising strategy in the treatment of refractory OCD, and it continues to be an active area in research. Perhaps the most promising strategy to improve outcomes for a variety of pathologies is with closed-loop stimulation or adaptive stimulation (164). This would allow more adaptable, patient-specific, and symptom-appropriate stimulation. There are several clinical trials ongoing with the goal of demonstrating the efficacy of adaptive stimulation (NCT02773082, NCT03457675, NCT04281134, NCT04806516). Other active studies are looking provide more support for DBS to strial targets, such as ALIC/NAcc (NCT04967560), VIC and NAcc (NCT04228744), and VC/VS (NCT04217408). These ongoing clinical trials will hopefully help to define an optimal target and provide a groundwork for adaptive DBS.
In summary, while early research into neural correlates of OCD has focused on individual components, several network-based models have emerged from more recent data on dysfunction within brain networks, including the lOFC-ventromedial caudate, limbic, salience, and default mode networks. Moreover, the interplay between multiple brain networks has been increasingly recognized. The implications of this for neuromodulation therapies in OCD is a shift more holistically toward modulation of networks. As our understanding of the neural circuitry underlying the pathophysiology of OCD continues to evolve, so will too our ability to specifically target these networks using invasive and non-invasive methods.
AK created the manuscript and contributed to the DBS section. JC and JL contributed to the networks and TMS sections. AF and XM provided resources, guidance, and reviewed the manuscript. WC, MP, and KB contributed figures and edits to the manuscript. BV and SM provided useful feedback and edits to the manuscript. DL oversaw the project and outlined the review. All authors contributed to the article and approved the submitted version.
The authors declare that the research was conducted in the absence of any commercial or financial relationships that could be construed as a potential conflict of interest.
All claims expressed in this article are solely those of the authors and do not necessarily represent those of their affiliated organizations, or those of the publisher, the editors and the reviewers. Any product that may be evaluated in this article, or claim that may be made by its manufacturer, is not guaranteed or endorsed by the publisher.
1. American Psychiatric A. Diagnostic and Statistical Manual of Mental Disorders. Arlington, VA: American Psychiatric Association. (2013).
2. Goodman WK, Storch EA, Sheth SA. Harmonizing the neurobiology and treatment of obsessive-compulsive disorder. Am J Psychiatry. (2021) 178:17–29. doi: 10.1176/appi.ajp.2020.20111601
3. Shephard E, Stern ER, Van Den Heuvel OA, Costa DLC, Batistuzzo MC, Godoy PBG, et al. Toward a neurocircuit-based taxonomy to guide treatment of obsessive-compulsive disorder. Mol Psychiatry. (2021) 26:4583–604. doi: 10.1038/s41380-020-01007-8
4. Alexander GE, Delong MR, Strick PL. Parallel organization of functionally segregated circuits linking basal ganglia and cortex. Annu Rev Neurosci. (1986) 9:357–81. doi: 10.1146/annurev.ne.09.030186.002041
5. Yeo BT, Krienen FM, Sepulcre J, Sabuncu MR, Lashkari D, Hollinshead M, et al. The organization of the human cerebral cortex estimated by intrinsic functional connectivity. J Neurophysiol. (2011) 106:1125–65. doi: 10.1152/jn.00338.2011
6. Choi EY, Yeo BT, Buckner RL. The organization of the human striatum estimated by intrinsic functional connectivity. J Neurophysiol. (2012) 108:2242–63. doi: 10.1152/jn.00270.2012
7. Hampshire A, Chaudhry AM, Owen AM, Roberts AC. Dissociable roles for lateral orbitofrontal cortex and lateral prefrontal cortex during preference driven reversal learning. Neuroimage. (2012) 59:4102–12. doi: 10.1016/j.neuroimage.2011.10.072
8. Dalton GL, Wang NY, Phillips AG, Floresco SB. Multifaceted contributions by different regions of the orbitofrontal and medial prefrontal cortex to probabilistic reversal learning. J Neurosci. (2016) 36:1996–2006. doi: 10.1523/JNEUROSCI.3366-15.2016
9. Panayi MC, Killcross S. (2018) Functional heterogeneity within the rodent lateral orbitofrontal cortex dissociates outcome devaluation and reversal learning deficits. Elife 7:e37357. doi: 10.7554/eLife.37357.014
10. Gremel CM, Costa RM. Orbitofrontal and striatal circuits dynamically encode the shift between goal-directed and habitual actions. Nat Commun. (2013) 4:2264. doi: 10.1038/ncomms3264
11. Gillan CM, Apergis-Schoute AM, Morein-Zamir S, Urcelay GP, Sule A, Fineberg NA, et al. Functional neuroimaging of avoidance habits in obsessive-compulsive disorder. Am J Psychiatry. (2015) 172:284–293. doi: 10.1176/appi.ajp.2014.14040525
12. Rauch SL, Wedig MM, Wright CI, Martis B, Mcmullin KG, Shin LM, et al. Functional magnetic resonance imaging study of regional brain activation during implicit sequence learning in obsessive-compulsive disorder. Biol Psychiatry. (2007) 61:330–336. doi: 10.1016/j.biopsych.2005.12.012
13. Ursu S, Carter CS. An initial investigation of the orbitofrontal cortex hyperactivity in obsessive-compulsive disorder: exaggerated representations of anticipated aversive events? Neuropsychologia. (2009) 47:2145–2148. doi: 10.1016/j.neuropsychologia.2009.03.018
14. Rotge JY, Langbour N, Guehl D, Bioulac B, Jaafari N, Allard M, et al. Gray matter alterations in obsessive-compulsive disorder: an anatomic likelihood estimation meta-analysis. Neuropsychopharmacology. (2010) 35:686–691. doi: 10.1038/npp.2009.175
15. Jung WH, Kang DH, Kim E, Shin KS, Jang JH, Kwon JS. Abnormal corticostriatal-limbic functional connectivity in obsessive-compulsive disorder during reward processing and resting-state. Neuroimage Clin. (2013) 3:27–38. doi: 10.1016/j.nicl.2013.06.013
16. Ahmari SE, Spellman T, Douglass NL, Kheirbek MA, Simpson HB, Deisseroth K, et al. Repeated cortico-striatal stimulation generates persistent OCD-like behavior. Science. (2013) 340:1234–1239. doi: 10.1126/science.1234733
17. Lei H, Lai J, Sun X, Xu Q, Feng G. Lateral orbitofrontal dysfunction in the Sapap3 knockout mouse model of obsessive-compulsive disorder. J Psychiatry Neurosci. (2019) 44:120–131. doi: 10.1503/jpn.180032
18. Van Der Straten AL, Denys D, Van Wingen. G. A. Impact of treatment on resting cerebral blood flow and metabolism in obsessive compulsive disorder: a meta-analysis. Sci Rep. (2017) 7:17464. doi: 10.1038/s41598-017-17593-7
19. Catani M, Dell'acqua F, Thiebaut De Schotten M. A revised limbic system model for memory, emotion and behaviour. Neurosci Biobehav Rev. (2013) 37:1724–1737. doi: 10.1016/j.neubiorev.2013.07.001
20. Papez JW. A proposed mechanism of emotion. Arch Neurol Psychiatry. (1937) 38:725–43. doi: 10.1001/archneurpsyc.1937.02260220069003
21. Maclean PD. The limbic system and its hippocampal formation; studies in animals and their possible application to man. J Neurosurg. (1954) 11:29–44. doi: 10.3171/jns.1954.11.1.0029
22. Pitman RK. Animal models of compulsive behavior. Biological Psychiatry. (1989) 26:189–198. doi: 10.1016/0006-3223(89)90022-X
23. Rauch SL, Jenike MA, Alpert NM, Baer L, Breiter HC, Savage CR, et al. Regional cerebral blood flow measured during symptom provocation in obsessive-compulsive disorder using oxygen 15-labeled carbon dioxide and positron emission tomography. Arch Gen Psychiatry. (1994) 51:62–70. doi: 10.1001/archpsyc.1994.03950010062008
24. Figee M, Vink M, De Geus F, Vulink N, Veltman DJ, Westenberg H, et al. Dysfunctional reward circuitry in obsessive-compulsive disorder. Biol Psychiatry. (2011) 69:867–874. doi: 10.1016/j.biopsych.2010.12.003
25. Kaufmann C, Beucke JC, Preusse F, Endrass T, Schlagenhauf F, Heinz A, et al. Medial prefrontal brain activation to anticipated reward and loss in obsessive-compulsive disorder. Neuroimage Clin. (2013) 2:212–220. doi: 10.1016/j.nicl.2013.01.005
26. Marsh R, Tau GZ, Wang Z, Huo Y, Liu G, Hao X, et al. Reward-based spatial learning in unmedicated adults with obsessive-compulsive disorder. Am J Psychiatry. (2015) 172:383–392. doi: 10.1176/appi.ajp.2014.13121700
27. Alves-Pinto A, Rus OG, Reess TJ, Wohlschlager A, Wagner G, Berberich G, et al. Altered reward-related effective connectivity in obsessive-compulsive disorder: an fMRI study. J Psychiatry Neurosci. (2019) 44:395–406. doi: 10.1503/jpn.180195
28. Simon D, Kaufmann C, Musch K, Kischkel E, Kathmann N. Fronto-striato-limbic hyperactivation in obsessive-compulsive disorder during individually tailored symptom provocation. Psychophysiology. (2010) 47:728–738. doi: 10.1111/j.1469-8986.2010.00980.x
29. Thorsen AL, Hagland P, Radua J, Mataix-Cols D, Kvale G, Hansen B, et al. Emotional Processing in Obsessive-Compulsive Disorder: A Systematic Review and Meta-analysis of 25 Functional Neuroimaging Studies. Biol Psychiatry Cogn Neurosci Neuroimaging. (2018) 3:563–571. doi: 10.1016/j.bpsc.2018.01.009
30. Gottlich M, Kramer UM, Kordon A, Hohagen F, Zurowski B. Decreased limbic and increased fronto-parietal connectivity in unmedicated patients with obsessive-compulsive disorder. Hum Brain Mapp. (2014) 35:5617–5632. doi: 10.1002/hbm.22574
31. Posner J, Marsh R, Maia TV, Peterson BS, Gruber A, Simpson HB. Reduced functional connectivity within the limbic cortico-striato-thalamo-cortical loop in unmedicated adults with obsessive-compulsive disorder. Hum Brain Mapp. (2014) 35:2852–2860. doi: 10.1002/hbm.22371
32. Heller AS, Johnstone T, Light SN, Peterson MJ, Kolden GG, Kalin NH, et al. Relationships between changes in sustained fronto-striatal connectivity and positive affect in major depression resulting from antidepressant treatment. Am J Psychiatry. (2013) 170:197–206. doi: 10.1176/appi.ajp.2012.12010014
33. Bernstein GA, Cullen KR, Harris EC, Conelea CA, Zagoloff AD, Carstedt PA, et al. Sertraline effects on striatal resting-state functional connectivity in youth with obsessive-compulsive disorder: a pilot study. J Am Acad Child Adolesc Psychiatry. (2019) 58:486–495. doi: 10.1016/j.jaac.2018.07.897
34. Mazoyer B, Zago L, Mellet E, Bricogne S, Etard O, Houdé O, et al. Cortical networks for working memory and executive functions sustain the conscious resting state in man. Brain Research Bulletin. (2001) 54:287–98. doi: 10.1016/S0361-9230(00)00437-8
35. Buckner RL, Dinicola LM. The brain's default network: updated anatomy, physiology and evolving insights. Nat Rev Neurosci. (2019) 20:593–608. doi: 10.1038/s41583-019-0212-7
36. Goncalves OF, Soares JM, Carvalho S, Leite J, Ganho-Avila A, Fernandes-Goncalves A, et al. Patterns of default mode network deactivation in obsessive compulsive disorder. Sci Rep. (2017) 7:44468. doi: 10.1038/srep44468
37. Koch K, Reess TJ, Rus OG, Gursel DA, Wagner G, Berberich G, et al. Increased default mode network connectivity in obsessive-compulsive disorder during reward processing. Front Psychiatry. (2018) 9:254. doi: 10.3389/fpsyt.2018.00254
38. Gursel DA, Avram M, Sorg C, Brandl F, Koch K. Frontoparietal areas link impairments of large-scale intrinsic brain networks with aberrant fronto-striatal interactions in OCD: a meta-analysis of resting-state functional connectivity. Neurosci Biobehav Rev. (2018) 87:151–160. doi: 10.1016/j.neubiorev.2018.01.016
39. Kim M, Jung WH, Shim G, Kwon JS. The effects of selective serotonin reuptake inhibitors on brain functional networks during goal-directed planning in obsessive-compulsive disorder. Sci Rep. (2020) 10:20619. doi: 10.1038/s41598-020-77814-4
40. Peters SK, Dunlop K, Downar J. Cortico-striatal-thalamic loop circuits of the salience network: a central pathway in psychiatric disease and treatment. Front Syst Neurosci. (2016) 10:104. doi: 10.3389/fnsys.2016.00104
41. Harsay HA, Spaan M, Wijnen JG, Ridderinkhof KR. Error awareness and salience processing in the oddball task: shared neural mechanisms. Front Hum Neurosci. (2012) 6:246. doi: 10.3389/fnhum.2012.00246
42. Lamichhane B, Adhikari BM, Dhamala M. Salience network activity in perceptual decisions. Brain Connect. (2016) 6:558–71. doi: 10.1089/brain.2015.0392
43. Davis B, Hasson U. Predictability of what or where reduces brain activity, but a bottleneck occurs when both are predictable. Neuroimage. (2018) 167:224–36. doi: 10.1016/j.neuroimage.2016.06.001
44. Menon V. Large-scale brain networks and psychopathology: a unifying triple network model. Trends Cogn Sci. (2011) 15:483–506. doi: 10.1016/j.tics.2011.08.003
45. Breiter HC, Rauch SL, Kwong KK, Baker JR, Weisskoff RM, Kennedy DN, et al. Functional magnetic resonance imaging of symptom provocation in obsessive-compulsive disorder. Arch Gen Psychiatry. (1996) 53:595–606. doi: 10.1001/archpsyc.1996.01830070041008
46. Hansen ES, Hasselbalch S, Law I, Bolwig TG. The caudate nucleus in obsessive-compulsive disorder. Reduced metabolism following treatment with paroxetine: a PET study. Int J Neuropsychopharmacol. (2002) 5:1–10. doi: 10.1017/S1461145701002681
47. Fitzgerald KD, Welsh RC, Gehring WJ, Abelson JL, Himle JA, Liberzon I, et al. Error-related hyperactivity of the anterior cingulate cortex in obsessive-compulsive disorder. Biol Psychiatry. (2005) 57:287–94. doi: 10.1016/j.biopsych.2004.10.038
48. Belin-Rauscent A, Daniel ML, Puaud M, Jupp B, Sawiak S, Howett D, et al. From impulses to maladaptive actions: the insula is a neurobiological gate for the development of compulsive behavior. Mol Psychiatry. (2016) 21:491–9. doi: 10.1038/mp.2015.140
49. Rasmussen SA, Goodman WK. The prefrontal cortex and neurosurgical treatment for intractable OCD. Neuropsychopharmacology. (2022) 47:349–60. doi: 10.1038/s41386-021-01149-5
50. Brotis AG, Kapsalaki EZ, Paterakis K, Smith JR, Fountas KN. Historic evolution of open cingulectomy and stereotactic cingulotomy in the management of medically intractable psychiatric disorders, pain and drug addiction. Stereotact Funct Neurosurg. (2009) 87:271–91. doi: 10.1159/000226669
51. Fontenelle LF, Coutinho ES, Lins-Martins NM, Fitzgerald PB, Fujiwara H, Yücel M. Electroconvulsive therapy for obsessive-compulsive disorder: a systematic review. J Clin Psychiatry. (2015) 76:949–57. doi: 10.4088/JCP.14r09129
52. Lefaucheur JP, Aleman A, Baeken C, Benninger DH, Brunelin J, Di Lazzaro V, et al. Evidence-based guidelines on the therapeutic use of repetitive transcranial magnetic stimulation (rTMS): An update (2014-2018). Clin Neurophysiol. (2020) 131:474–528. doi: 10.1016/j.clinph.2019.11.002
53. Pascual-Leone A, Valls-Sol, é J, Wassermann EM, Hallett M. Responses to rapid-rate transcranial magnetic stimulation of the human motor cortex. Brain. (1994) 117, 847–58. doi: 10.1093/brain/117.4.847
54. Chen R, Classen J, Gerloff C, Celnik P, Wassermann EM, Hallett M, et al. Depression of motor cortex excitability by low-frequency transcranial magnetic stimulation. Neurology. (1997) 48:1398–1403. doi: 10.1212/WNL.48.5.1398
55. Vlachos A, Müller-Dahlhaus F, Rosskopp J, Lenz M, Ziemann U, Deller T. Repetitive magnetic stimulation induces functional and structural plasticity of excitatory postsynapses in mouse organotypic hippocampal slice cultures. J Neurosci. (2012) 32:17514–17523. doi: 10.1523/JNEUROSCI.0409-12.2012
56. Lenz M, Galanis C, Müller-Dahlhaus F, Opitz A, Wierenga CJ, Szabó G, et al. Repetitive magnetic stimulation induces plasticity of inhibitory synapses. Nat Commun. (2016) 7:10020. doi: 10.1038/ncomms10020
57. Huang YZ, Edwards MJ, Rounis E, Bhatia KP, Rothwell JC. Theta burst stimulation of the human motor cortex. Neuron. (2005) 45:201–6. doi: 10.1016/j.neuron.2004.12.033
58. Alonso P, Pujol J, Cardoner N, Benlloch L, Deus J, Menchón JM, et al. Right prefrontal repetitive transcranial magnetic stimulation in obsessive-compulsive disorder: a double-blind, placebo-controlled study. Am J Psychiatry. (2001) 158:1143–5. doi: 10.1176/appi.ajp.158.7.1143
59. Prasko J, Pasková B, Záleský R, Novák T, Kopecek M, Bares M, et al. The effect of repetitive transcranial magnetic stimulation (rTMS) on symptoms in obsessive compulsive disorder. A randomized, double blind, sham controlled study. Neuro Endocrinol Lett. (2006) 27:327–32.
60. Sachdev PS, Loo CK, Mitchell PB, Mcfarquhar TF, Malhi GS. Repetitive transcranial magnetic stimulation for the treatment of obsessive compulsive disorder: a double-blind controlled investigation. Psychol Med. (2007) 37:1645–9. doi: 10.1017/S0033291707001092
61. Kang JI, Kim CH, Namkoong K, Lee CI, Kim SJ. A randomized controlled study of sequentially applied repetitive transcranial magnetic stimulation in obsessive-compulsive disorder. J Clin Psychiatry. (2009) 70:1645–51. doi: 10.4088/JCP.08m04500
62. Sarkhel S, Sinha VK, Praharaj SK. Adjunctive high-frequency right prefrontal repetitive transcranial magnetic stimulation (rTMS) was not effective in obsessive-compulsive disorder but improved secondary depression. J Anxiety Disord. (2010) 24:535–9. doi: 10.1016/j.janxdis.2010.03.011
63. Mansur CG, Myczkowki ML, De Barros Cabral S, Sartorelli MOC, Bellini BB, Dias AM, et al. Placebo effect after prefrontal magnetic stimulation in the treatment of resistant obsessive-compulsive disorder: a randomized controlled trial. Int J Neuropsychopharmacol. (2011) 14:1389–97. doi: 10.1017/S1461145711000575
64. Ma ZR, Shi LJ. Repetitive transcranial magnetic stimulation (rTMS) augmentation of selective serotonin reuptake inhibitors (SSRIs) for SSRI-resistant obsessive-compulsive disorder (OCD): a meta-analysis of randomized controlled trials. Int J Clin Exp Med. (2014) 7:4897–905.
65. Haghighi M, Shayganfard M, Jahangard L, Ahmadpanah M, Bajoghli H, Pirdehghan A, et al. Repetitive Transcranial Magnetic Stimulation (rTMS) improves symptoms and reduces clinical illness in patients suffering from OCD–Results from a single-blind, randomized clinical trial with sham cross-over condition. J Psychiatr Res. (2015) 68:238–44. doi: 10.1016/j.jpsychires.2015.06.020
66. Elbeh KAM, Elserogy YMB, Khalifa HE, Ahmed MA, Hafez MH, Khedr EM. Repetitive transcranial magnetic stimulation in the treatment of obsessive-compulsive disorders: Double blind randomized clinical trial. Psychiatry Res. (2016) 238:264–9. doi: 10.1016/j.psychres.2016.02.031
67. Jahangard L, Haghighi M, Shyayganfard M, Ahmadpanah M, Sadeghi Bahmani D, Bajoghli H, et al. Repetitive transcranial magnetic stimulation improved symptoms of obsessive-compulsive disorder, but also cognitive performance: results from a randomized clinical trial with a cross-over design and sham condition. Neuropsychobiology. (2016) 73:224–32. doi: 10.1159/000446287
68. Seo HJ, Jung YE, Lim HK, Um YH, Lee CU, Chae JH. Adjunctive low-frequency repetitive transcranial magnetic stimulation over the right dorsolateral prefrontal cortex in patients with treatment-resistant obsessive-compulsive disorder: a randomized controlled trial. Clin Psychopharmacol Neurosci. (2016) 14:153–60. doi: 10.9758/cpn.2016.14.2.153
69. Shayganfard M, Jahangard L, Nazaribadie M, Haghighi M, Ahmadpanah M, Sadeghi Bahmani D, et al. repetitive transcranial magnetic stimulation improved symptoms of obsessive-compulsive disorders but not executive functions: results from a randomized clinical trial with crossover design and sham condition. Neuropsychobiology. (2016) 74:115–24. doi: 10.1159/000457128
70. Naro A, Billeri L, Cannavò A, De Luca R, Portaro S, Bramanti P, et al. Theta burst stimulation for the treatment of obsessive-compulsive disorder: a pilot study. J Neural Transm. (2019) 126:1667–77. doi: 10.1007/s00702-019-02098-6
71. Mantovani A, Simpson HB, Fallon BA, Rossi S, Lisanby SH. Randomized sham-controlled trial of repetitive transcranial magnetic stimulation in treatment-resistant obsessive-compulsive disorder. Int J Neuropsychopharmacol. (2010) 13:217–227. doi: 10.1017/S1461145709990435
72. Gomes PV, Brasil-Neto JP, Allam N, Rodrigues De Souza E. A randomized, double-blind trial of repetitive transcranial magnetic stimulation in obsessive-compulsive disorder with three-month follow-up. J Neuropsychiatry Clin Neurosci. (2012) 24:437–43. doi: 10.1176/appi.neuropsych.11100242
73. Hawken ER, Dilkov D, Kaludiev E, Simek S, Zhang F, Milev R. Transcranial magnetic stimulation of the supplementary motor area in the treatment of obsessive-compulsive disorder: a multi-site study. Int J Mol Sci. (2016) 17:420. doi: 10.3390/ijms17030420
74. Pelissolo A, Harika-Germaneau G, Rachid F, Gaudeau-Bosma C, Tanguy ML, Benadhira R, et al. Repetitive transcranial magnetic stimulation to supplementary motor area in refractory obsessive-compulsive disorder treatment: a sham-controlled trial. Int J Neuropsychopharmacol. (2016) 19:pyw025. doi: 10.1093/ijnp/pyw025
75. Arumugham SS, Vs S, Hn M, B V, Ravi M, Sharma E, et al. Augmentation effect of low-frequency repetitive transcranial magnetic stimulation over presupplementary motor area in obsessive-compulsive disorder: a randomized controlled trial. J ECT. (2018) 34:253–7. doi: 10.1097/YCT.0000000000000509
76. Harika-Germaneau G, Rachid F, Chatard A, Lafay-Chebassier C, Solinas M, Thirioux B, et al. Continuous theta burst stimulation over the supplementary motor area in refractory obsessive-compulsive disorder treatment: a randomized sham-controlled trial. Brain Stimul. (2019) 12:1565–71. doi: 10.1016/j.brs.2019.07.019
77. Zhang K, Fan X, Yuan J, Yin J, Su H, Hashimoto K, et al. Impact of serotonin transporter gene on rTMS augmentation of SSRIs for obsessive compulsive disorder. Neuropsychiatr Dis Treat. (2019) 15:1771–9. doi: 10.2147/NDT.S209319
78. Ruffini C, Locatelli M, Lucca A, Benedetti F, Insacco C, Smeraldi E. Augmentation effect of repetitive transcranial magnetic stimulation over the orbitofrontal cortex in drug-resistant obsessive-compulsive disorder patients: a controlled investigation. Prim Care Companion J Clin Psychiatry. (2009) 11:226–30. doi: 10.4088/PCC.08m00663
79. Nauczyciel C, Le Jeune F, Naudet F, Douabin S, Esquevin A, Vérin M, et al. Repetitive transcranial magnetic stimulation over the orbitofrontal cortex for obsessive-compulsive disorder: a double-blind, crossover study. Transl Psychiatry. (2014) 4:e436. doi: 10.1038/tp.2014.62
80. Carmi L, Alyagon U, Barnea-Ygael N, Zohar J, Dar R, Zangen A. Clinical and electrophysiological outcomes of deep TMS over the medial prefrontal and anterior cingulate cortices in OCD patients. Brain Stimul. (2018) 11:158–165. doi: 10.1016/j.brs.2017.09.004
81. Carmi L, Tendler A, Bystritsky A, Hollander E, Blumberger DM, Daskalakis J, et al. Efficacy and safety of deep transcranial magnetic stimulation for obsessive-compulsive disorder: a prospective multicenter randomized double-blind placebo-controlled trial. Am J Psychiatry. (2019) 176:931–8. doi: 10.1176/appi.ajp.2019.18101180
82. Rehn S, Eslick GD, Brakoulias V. A Meta-analysis of the effectiveness of different cortical targets used in repetitive transcranial magnetic stimulation (rtms) for the treatment of obsessive-compulsive disorder (OCD). Psychiatr Q. (2018) 89:645–65. doi: 10.1007/s11126-018-9566-7
83. Rapinesi C, Kotzalidis GD, Ferracuti S, Sani G, Girardi P, Del Casale. A. Brain stimulation in obsessive-compulsive disorder (OCD): a systematic review. Curr Neuropharmacol. (2019) 17:787–807. doi: 10.2174/1570159X17666190409142555
84. Acevedo N, Bosanac P, Pikoos T, Rossell S, Castle D. Therapeutic neurostimulation in obsessive-compulsive and related disorders: a systematic review. Brain Sci. (2021) 11:948. doi: 10.3390/brainsci11070948
85. Bergfeld IO, Dijkstra E, Graat I, De Koning P, Van Den Boom BJG, Arbab T, et al. Invasive and non-invasive neurostimulation for OCD. Curr Top Behav Neurosci. (2021) 49:399–436. doi: 10.1007/7854_2020_206
86. Liang K, Li H, Bu X, Li X, Cao L, Liu J, et al. Efficacy and tolerability of repetitive transcranial magnetic stimulation for the treatment of obsessive-compulsive disorder in adults: a systematic review and network meta-analysis. Transl Psychiatry. (2021) 11:332. doi: 10.1038/s41398-021-01453-0
87. Perera MPN, Mallawaarachchi S, Miljevic A, Bailey NW, Herring SE, Fitzgerald PB. Repetitive transcranial magnetic stimulation for obsessive-compulsive disorder: a meta-analysis of randomized, sham-controlled trials. Biol Psychiatry Cogn Neurosci Neuroimaging. (2021) 6:947–60. doi: 10.1016/j.bpsc.2021.03.010
88. Goodman WK, Price LH, Rasmussen SA, Mazure C, Fleischmann RL, Hill CL, et al. The yale-brown obsessive compulsive scale. I. Development, use, and reliability. Arch Gen Psychiatry. (1989) 46:1006–11. doi: 10.1001/archpsyc.1989.01810110048007
89. Stein DJ, Costa DLC, Lochner C, Miguel EC, Reddy YCJ, Shavitt RG, et al. Obsessive-compulsive disorder. In: Pittenger C, editor. Nature Reviews. Disease primers. Oxford: Oxford University Press (2019).
90. Mataix-Cols D, Wooderson S, Lawrence N, Brammer MJ, Speckens A, Phillips ML. Distinct neural correlates of washing, checking, and hoarding symptom dimensions in obsessive-compulsive disorder. Archives of General Psychiatry. (2004) 61:564–76. doi: 10.1001/archpsyc.61.6.564
91. Fitzgerald PB, Hoy K, Mcqueen S, Maller JJ, Herring S, Segrave R, et al. A randomized trial of rTMS targeted with MRI based neuro-navigation in treatment-resistant depression. Neuropsychopharmacology. (2009) 34:1255–62. doi: 10.1038/npp.2008.233
92. Barcia JA, Avecillas-Chasin JM, Nombela C, Arza R, Garcia-Albea J, Pineda-Pardo JA, et al. Personalized striatal targets for deep brain stimulation in obsessive-compulsive disorder. Brain Stimul. (2019) 12:724–734. doi: 10.1016/j.brs.2018.12.226
93. Cole EJ, Stimpson KH, Bentzley BS, Gulser M, Cherian K, Tischler C, et al. Stanford accelerated intelligent neuromodulation therapy for treatment-resistant depression. Am J Psychiatry. (2020) 177:716–26. doi: 10.1176/appi.ajp.2019.19070720
94. Van Den Eynde F, Claudino AM, Mogg A, Horrell L, Stahl D, Ribeiro W, et al. Repetitive transcranial magnetic stimulation reduces cue-induced food craving in bulimic disorders. Biol. Psychiatr. (2010) 67:793–5. doi: 10.1016/j.biopsych.2009.11.023
95. Isserles M, Shalev AY, Roth Y, Peri T, Kutz I, Zlotnick E, et al. Effectiveness of deep transcranial magnetic stimulation combined with a brief exposure procedure in post-traumatic stress disorder–a pilot study. Brain stimulation. (2013) 6:377–83. doi: 10.1016/j.brs.2012.07.008
96. Dinur-Klein L, Dannon P, Hadar A, Rosenberg O, Roth Y, Kotler M, et al. Smoking cessation induced by deep repetitive transcranial magnetic stimulation of the prefrontal and insular cortices: a prospective, randomized controlled trial. Biol Psychiatry. (2014) 76:742–9. doi: 10.1016/j.biopsych.2014.05.020
97. Donse L, Padberg F, Sack AT, Rush AJ, Arns M. Simultaneous rTMS and psychotherapy in major depressive disorder: clinical outcomes and predictors from a large naturalistic study. Brain Stimulation. (2018) 11:337–45. doi: 10.1016/j.brs.2017.11.004
98. Di Lorenzo F, Ponzo V, Motta C, Bonni S, Picazio S, Caltagirone C, et al. Impaired spike timing dependent cortico-cortical plasticity in alzheimer's disease patients. J Alzheimers Dis. (2018) 66:983–91. doi: 10.3233/JAD-180503
99. Nitsche MA, Paulus W. Excitability changes induced in the human motor cortex by weak transcranial direct current stimulation. J Physiol. (2000) 3:633–9. doi: 10.1111/j.1469-7793.2000.t01-1-00633.x
100. D'urso G, Brunoni AR, Mazzaferro MP, Anastasia A, De Bartolomeis A, Mantovani A. Transcranial direct current stimulation for obsessive-compulsive disorder: A randomized, controlled, partial crossover trial. Depress Anxiety. (2016) 33:1132–40. doi: 10.1002/da.22578
101. Gowda SM, Narayanaswamy JC, Hazari N, Bose A, Chhabra H, Balachander S, et al. Efficacy of pre-supplementary motor area transcranial direct current stimulation for treatment resistant obsessive compulsive disorder: A randomized, double blinded, sham controlled trial. Brain Stimul. (2019) 12:922–9. doi: 10.1016/j.brs.2019.02.005
102. Silva R, Brunoni AR, Goerigk S, Batistuzzo MC, Costa D, Diniz JB, et al. Efficacy and safety of transcranial direct current stimulation as an add-on treatment for obsessive-compulsive disorder: a randomized, sham-controlled trial. Neuropsychopharmacology. (2021) 46:1028–34. doi: 10.1038/s41386-020-00928-w
103. Todder D, Gershi A, Perry Z, Kaplan Z, Levine J, Avirame K. Immediate effects of transcranial direct current stimulation on obsession-induced anxiety in refractory obsessive-compulsive disorder: a pilot study. J ECT. (2018) 34:e51–7. doi: 10.1097/YCT.0000000000000473
104. Adams TG, Cisler JM, Kelmendi B, George JR, Kichuk SA, Averill CL, et al. Transcranial direct current stimulation targeting the medial prefrontal cortex modulates functional connectivity and enhances safety learning in obsessive-compulsive disorder: results from two pilot studies. Depress Anxiety. (2022) 39:37–48. doi: 10.1002/da.23212
105. Bation R, Mondino M, Le Camus F, Saoud M, Brunelin J. Transcranial direct current stimulation in patients with obsessive compulsive disorder: a randomized controlled trial. Eur Psychiatry. (2019) 62:38–44. doi: 10.1016/j.eurpsy.2019.08.011
106. Antal A, Paulus W. Transcranial alternating current stimulation (tACS). Front Hum Neurosci. (2013) 7:317. doi: 10.3389/fnhum.2013.00317
107. Klimke A, Nitsche MA, Maurer K, Voss U. Case Report: Successful Treatment of Therapy-Resistant OCD With Application of Transcranial Alternating Current Stimulation (tACS). Brain Stimul. (2016) 9:463–5. doi: 10.1016/j.brs.2016.03.005
108. Grover S, Nguyen JA, Viswanathan V, Reinhart RMG. High-frequency neuromodulation improves obsessive-compulsive behavior. Nat Med. (2021) 27:232–8. doi: 10.1038/s41591-020-01173-w
109. Berry SM, Broglio K, Bunker M, Jayewardene A, Olin B, Rush AJ. A patient-level meta-analysis of studies evaluating vagus nerve stimulation therapy for treatment-resistant depression. Med Devices. (2013) 6:17–35. doi: 10.2147/MDER.S41017
110. Figee M, Luigjes J, Smolders R, Valencia-Alfonso CE, Van Wingen G, De Kwaasteniet B, et al. Deep brain stimulation restores frontostriatal network activity in obsessive-compulsive disorder. Nat Neurosci. (2013) 16:386–387. doi: 10.1038/nn.3344
111. Alonso P, Cuadras D, Gabriels L, Denys D, Goodman W, Greenberg BD, et al. Deep brain stimulation for obsessive-compulsive disorder: a meta-analysis of treatment outcome and predictors of response. PLoS ONE. (2015) 10:e0133591. doi: 10.1371/journal.pone.0133591
112. Karas PJ, Lee S, Jimenez-Shahed J, Goodman WK, Viswanathan A, Sheth SA. Deep brain stimulation for obsessive compulsive disorder: evolution of surgical stimulation target parallels changing model of dysfunctional brain circuits. Front Neurosci. (2018) 12:998. doi: 10.3389/fnins.2018.00998
113. Hamilton M. The assessment of anxiety states by rating. Br J Med Psychol. (1959) 32:50–55. doi: 10.1111/j.2044-8341.1959.tb00467.x
114. Hamilton M. A rating scale for depression. J Neurol Neurosurg Psychiatry. (1960) 23:56–62. doi: 10.1136/jnnp.23.1.56
115. Lewin AB, De Nadai AS, Park J, Goodman WK, Murphy TK, Storch EA. Refining clinical judgment of treatment outcome in obsessive-compulsive disorder. Psychiatry Res. (2011) 185:394–401. doi: 10.1016/j.psychres.2010.08.021
116. De Koning PP, Figee M, Van Den Munckhof P, Schuurman PR, Denys D. Current status of deep brain stimulation for obsessive-compulsive disorder: a clinical review of different targets. Curr Psychiatry Rep. (2011) 13:274–82. doi: 10.1007/s11920-011-0200-8
117. Lippitz BE, Mindus P, Meyerson BA, Kihlstrom L, Lindquist C. Lesion topography and outcome after thermocapsulotomy or gamma knife capsulotomy for obsessive-compulsive disorder: relevance of the right hemisphere. Neurosurgery. (1999) 44:452–8; discussion 458–60. doi: 10.1097/00006123-199903000-00005
118. Nuttin B, Cosyns P, Demeulemeester H, Gybels J, Meyerson B. Electrical stimulation in anterior limbs of internal capsules in patients with obsessive-compulsive disorder. Lancet. (1999) 354:1526. doi: 10.1016/S0140-6736(99)02376-4
119. Nuttin BJ, Gabriels LA, Cosyns PR, Meyerson BA, Andreewitch S, Sunaert SG, et al. Long-term electrical capsular stimulation in patients with obsessive-compulsive disorder. Neurosurgery. (2003) 52:1263–72; discussion 1272–4. doi: 10.1227/01.NEU.0000064565.49299.9A
120. Goodman WK, Foote KD, Greenberg BD, Ricciuti N, Bauer R, Ward H, et al. Deep brain stimulation for intractable obsessive compulsive disorder: pilot study using a blinded, staggered-onset design. Biol Psychiatry. (2010) 67:535–42. doi: 10.1016/j.biopsych.2009.11.028
121. Denys D, Graat I, Mocking R, De Koning P, Vulink N, Figee M, et al. Efficacy of deep brain stimulation of the ventral anterior limb of the internal capsule for refractory obsessive-compulsive disorder: a clinical cohort of 70 patients. Am J Psychiatry. (2020) 177:265–71. doi: 10.1176/appi.ajp.2019.19060656
122. Menchon JM, Real E, Alonso P, Aparicio MA, Segalas C, Plans G, et al. A prospective international multi-center study on safety and efficacy of deep brain stimulation for resistant obsessive-compulsive disorder. Mol Psychiatry. (2021) 26:1234–47. doi: 10.1038/s41380-019-0562-6
123. Liebrand LC, Caan MWA, Schuurman PR, Van Den Munckhof P, Figee M, Denys D, et al. Individual white matter bundle trajectories are associated with deep brain stimulation response in obsessive-compulsive disorder. Brain Stimul. (2019) 12:353–60. doi: 10.1016/j.brs.2018.11.014
124. Baldermann JC, Melzer C, Zapf A, Kohl S, Timmermann L, Tittgemeyer M, et al. Connectivity profile predictive of effective deep brain stimulation in obsessive-compulsive disorder. Biol Psychiatry. (2019) 85:735–43. doi: 10.1016/j.biopsych.2018.12.019
125. Li N, Baldermann JC, Kibleur A, Treu S, Akram H, Elias GJB, et al. A unified connectomic target for deep brain stimulation in obsessive-compulsive disorder. Nat Commun. (2020) 11:3364. doi: 10.1038/s41467-020-16734-3
126. Baldermann JC, Schüller T, Kohl S, Voon V, Li N, Hollunder B, et al. Connectomic deep brain stimulation for obsessive-compulsive disorder. Biol Psychiatry. (2021) 90:678–88. doi: 10.1016/j.biopsych.2021.07.010
127. Li N, Hollunder B, Baldermann JC, Kibleur A, Treu S, Akram H, et al. A unified functional network target for deep brain stimulation in obsessive-compulsive disorder. Biol Psychiatry. (2021) 90:701–13. doi: 10.1016/j.biopsych.2021.04.006
128. Haq IU, Foote KD, Goodman WG, Wu SS, Sudhyadhom A, Ricciuti N, et al. Smile and laughter induction and intraoperative predictors of response to deep brain stimulation for obsessive-compulsive disorder. Neuroimage. (2011) 54(Suppl.1):S247–55. doi: 10.1016/j.neuroimage.2010.03.009
129. Goodman WK, Storch EA, Cohn JF, Sheth SA. Deep brain stimulation for intractable obsessive-compulsive disorder: progress and opportunities. Am J Psychiatry. (2020) 177:200–3. doi: 10.1176/appi.ajp.2020.20010037
130. Bingley T, Leksell L, Meyerson B, Rylander G. Long Term Results of Stereotactic Capsulotomy in Chronic Obsessive-Compulsive Neurosis. Neurosurgical Treatment in Psychiatry, Pain and Epilepsy. Baltimore: University Park Press (1977). p. 287–9.
131. Park YS, Sammartino F, Young NA, Corrigan J, Krishna V, Rezai AR. Anatomic review of the ventral capsule/ventral striatum and the nucleus accumbens to guide target selection for deep brain stimulation for obsessive-compulsive disorder. World Neurosurg. (2019) 126:1–10. doi: 10.1016/j.wneu.2019.01.254
132. Sturm V, Lenartz D, Koulousakis A, Treuer H, Herholz K, Klein JC, et al. The nucleus accumbens: a target for deep brain stimulation in obsessive-compulsive- and anxiety-disorders. J Chem Neuroanat. (2003) 26:293–9. doi: 10.1016/j.jchemneu.2003.09.003
133. Denys D, Mantione M, Figee M, Van Den Munckhof P, Koerselman F, Westenberg H, et al. Deep brain stimulation of the nucleus accumbens for treatment-refractory obsessive-compulsive disorder. Arch Gen Psychiatry. (2010) 67:1061–8. doi: 10.1001/archgenpsychiatry.2010.122
134. Greenberg BD, Gabriels LA, Malone DA Jr, Rezai AR, Friehs GM, et al. Deep brain stimulation of the ventral internal capsule/ventral striatum for obsessive-compulsive disorder: worldwide experience. Mol Psychiatry. (2010) 15:64–79. doi: 10.1038/mp.2008.55
135. Mallet L, Schupbach M, N'diaye K, Remy P, Bardinet E, Czernecki V, et al. Stimulation of subterritories of the subthalamic nucleus reveals its role in the integration of the emotional and motor aspects of behavior. Proc Natl Acad Sci USA. (2007) 104:10661–6. doi: 10.1073/pnas.0610849104
136. Fontaine D, Mattei V, Borg M, Von Langsdorff D, Magnie MN, Chanalet S, et al. Effect of subthalamic nucleus stimulation on obsessive-compulsive disorder in a patient with Parkinson disease. Case report. J Neurosurg. (2004) 100:1084–6. doi: 10.3171/jns.2004.100.6.1084
137. Mallet L, Polosan M, Jaafari N, Baup N, Welter ML, Fontaine D, et al. Subthalamic nucleus stimulation in severe obsessive-compulsive disorder. N Engl J Med. (2008) 359:2121–34. doi: 10.1056/NEJMoa0708514
138. Tyagi H, Apergis-Schoute AM, Akram H, Foltynie T, Limousin P, Drummond LM, et al. A randomized trial directly comparing ventral capsule and anteromedial subthalamic nucleus stimulation in obsessive-compulsive disorder: clinical and imaging evidence for dissociable effects. Biol Psychiatry. (2019) 85:726–34. doi: 10.1016/j.biopsych.2019.01.017
139. Wojtecki L, Elben S, Rubenach J, Hartmann C, Vesper J, Schnitzler A. Subthalamic deep brain stimulation in obsessive-compulsive disorder: first german experience and future outlook. World Neurosurg. (2016) 95:607–8. doi: 10.1016/j.wneu.2016.07.033
140. Dumont EC. What is the bed nucleus of the stria terminalis? Prog Neuropsychopharmacol Biol Psychiatry. (2009) 33:1289–90. doi: 10.1016/j.pnpbp.2009.07.006
141. Luyten L, Hendrickx S, Raymaekers S, Gabriels L, Nuttin B. Electrical stimulation in the bed nucleus of the stria terminalis alleviates severe obsessive-compulsive disorder. Mol Psychiatry. (2016) 21:1272–80. doi: 10.1038/mp.2015.124
142. Raymaekers S, Vansteelandt K, Luyten L, Bervoets C, Demyttenaere K, Gabriels L, et al. Long-term electrical stimulation of bed nucleus of stria terminalis for obsessive-compulsive disorder. Mol Psychiatry. (2017) 22:931–934. doi: 10.1038/mp.2016.124
143. Mosley PE, Windels F, Morris J, Coyne T, Marsh R, Giorni A, et al. A randomised, double-blind, sham-controlled trial of deep brain stimulation of the bed nucleus of the stria terminalis for treatment-resistant obsessive-compulsive disorder. Transl Psychiatry. (2021) 11:190. doi: 10.1038/s41398-021-01307-9
144. Jimenez-Ponce F, Velasco-Campos F, Castro-Farfan G, Nicolini H, Velasco AL, Salin-Pascual R, et al. Preliminary study in patients with obsessive-compulsive disorder treated with electrical stimulation in the inferior thalamic peduncle. Neurosurgery. (2009) 65:203–9; discussion 209. doi: 10.1227/01.NEU.0000345938.39199.90
145. Lee DJ, Dallapiazza RF, De Vloo P, Elias GJB, Fomenko A, Boutet A, et al. Inferior thalamic peduncle deep brain stimulation for treatment-refractory obsessive-compulsive disorder: a phase 1 pilot trial. Brain Stimul. (2019) 12:344–52. doi: 10.1016/j.brs.2018.11.012
146. Velasco F, Velasco M, Jimenez F, Velasco AL, Salin-Pascual R. Neurobiological background for performing surgical intervention in the inferior thalamic peduncle for treatment of major depression disorders. Neurosurgery. (2005) 57:439–48; discussion 439–48. doi: 10.1227/01.NEU.0000172172.51818.51
147. Van Der Vlis T, Ackermans L, Mulders AEP, Vrij CA, Schruers K, Temel Y, et al. Ventral capsule/ventral striatum stimulation in obsessive-compulsive disorder: toward a unified connectomic target for deep brain stimulation? Neuromodulation. (2021) 24:316–23. doi: 10.1111/ner.13339
148. Widge AS, Zhang F, Gosai A, Papadimitrou G, Wilson-Braun P, Tsintou M, et al. Patient-specific connectomic models correlate with, but do not reliably predict, outcomes in deep brain stimulation for obsessive-compulsive disorder. Neuropsychopharmacology. (2022) 47:965–72. doi: 10.1038/s41386-021-01199-9
149. Carreno FR, Frazer A. Vagal nerve stimulation for treatment-resistant depression. Neurotherapeutics. (2017) 14:716–27. doi: 10.1007/s13311-017-0537-8
150. Cimpianu CL, Strube W, Falkai P, Palm U, Hasan A. Vagus nerve stimulation in psychiatry: a systematic review of the available evidence. J Neural Transm. (2017) 124:145–58. doi: 10.1007/s00702-016-1642-2
151. Fraschini M, Demuru M, Puligheddu M, Floridia S, Polizzi L, Maleci A, et al. The re-organization of functional brain networks in pharmaco-resistant epileptic patients who respond to VNS. Neurosci Lett. (2014) 580:153–7. doi: 10.1016/j.neulet.2014.08.010
152. George MS, Ward H. E. Jr., Ninan PT, Pollack M, Nahas Z, et al. A pilot study of vagus nerve stimulation (VNS) for treatment-resistant anxiety disorders. Brain Stimul. (2008) 1:112–21. doi: 10.1016/j.brs.2008.02.001
153. Hamani C, Pilitsis J, Rughani AI, Rosenow JM, Patil PG, Slavin KS, et al. Deep brain stimulation for obsessive-compulsive disorder: systematic review and evidence-based guideline sponsored by the American Society for Stereotactic and Functional Neurosurgery and the Congress of Neurological Surgeons (CNS) and endorsed by the CNS and American Association of Neurological Surgeons. Neurosurgery. (2014) 75:327–33; quiz 333. doi: 10.1227/NEU.0000000000000499
154. Staudt MD, Pouratian N, Miller JP, Hamani C, Raviv N, Mckhann GM, et al. Congress of neurological surgeons systematic review and evidence-based guidelines for deep brain stimulations for obsessive-compulsive disorder: update of the 2014. Guidelines. Neurosurgery. (2021) 88:710–2. doi: 10.1093/neuros/nyaa596
155. Wu H, Hariz M, Visser-Vandewalle V, Zrinzo L, Coenen VA, Sheth SA, et al. Deep brain stimulation for refractory obsessive-compulsive disorder (OCD): emerging or established therapy? Mol Psychiatry. (2021) 26:60–5. doi: 10.1038/s41380-020-00933-x
156. Pinckard-Dover H, Ward H, Foote KD. The decline of deep brain stimulation for obsessive-compulsive disorder following FDA humanitarian device exemption approval. Front Surg. (2021) 8:642503. doi: 10.3389/fsurg.2021.642503
157. Raviv N, Staudt MD, Rock AK, Macdonell J, Slyer J, Pilitsis JG. A systematic review of deep brain stimulation targets for obsessive compulsive disorder. Neurosurgery. (2020) 87:1098–110. doi: 10.1093/neuros/nyaa249
158. Hageman SB, Van Rooijen G, Bergfeld IO, Schirmbeck F, De Koning P, Schuurman PR, et al. Deep brain stimulation versus ablative surgery for treatment-refractory obsessive-compulsive disorder: a meta-analysis. Acta Psychiatr Scand. (2021) 143:307–18. doi: 10.1111/acps.13276
159. Mustroph ML, Cosgrove GR, Williams ZM. The evolution of modern ablative surgery for the treatment of obsessive-compulsive and major depression disorders. Front Integr Neurosci. (2022) 16:797533. doi: 10.3389/fnint.2022.797533
160. Kumar KK, Appelboom G, Lamsam L, Caplan AL, Williams NR, Bhati MT, et al. Comparative effectiveness of neuroablation and deep brain stimulation for treatment-resistant obsessive-compulsive disorder: a meta-analytic study. J Neurol Neurosurg Psychiatry. (2019) 90:469–73. doi: 10.1136/jnnp-2018-319318
161. Moon W, Kim SN, Park S, Paek SH, Kwon JS. The cost-effectiveness of deep brain stimulation for patients with treatment-resistant obsessive-compulsive disorder. Medicine. (2017) 96:e7397. doi: 10.1097/MD.0000000000007397
162. Ooms P, Blankers M, Figee M, Bergfeld IO, Van Den Munckhof P, Schuurman PR, et al. Cost-effectiveness of deep brain stimulation versus treatment as usual for obsessive-compulsive disorder. Brain Stimul. (2017) 10:836–42. doi: 10.1016/j.brs.2017.04.120
163. Dell'osso B, Porta M, Servello D, Altamura AC. Deep Brain Stimulation device removal after scar picking behaviors in a patient with treatment-resistant obsessive compulsive disorder. Brain Stimul. (2013) 6:96–8. doi: 10.1016/j.brs.2012.01.007
Keywords: neuromodulation, obsessive compulsive disorder (OCD), deep brain stimulation (DBS), TMS, VNS
Citation: Kammen A, Cavaleri J, Lam J, Frank AC, Mason X, Choi W, Penn M, Brasfield K, Van Noppen B, Murray SB and Lee DJ (2022) Neuromodulation of OCD: A review of invasive and non-invasive methods. Front. Neurol. 13:909264. doi: 10.3389/fneur.2022.909264
Received: 31 March 2022; Accepted: 19 July 2022;
Published: 09 August 2022.
Edited by:
William Omar Contreras Lopez, Clínica FOSCAL, ColombiaReviewed by:
Francesco Di Lorenzo, Santa Lucia Foundation (IRCCS), ItalyCopyright © 2022 Kammen, Cavaleri, Lam, Frank, Mason, Choi, Penn, Brasfield, Van Noppen, Murray and Lee. This is an open-access article distributed under the terms of the Creative Commons Attribution License (CC BY). The use, distribution or reproduction in other forums is permitted, provided the original author(s) and the copyright owner(s) are credited and that the original publication in this journal is cited, in accordance with accepted academic practice. No use, distribution or reproduction is permitted which does not comply with these terms.
*Correspondence: Darrin Jason Lee, ZGFycmluamxlZUBnbWFpbC5jb20=
Disclaimer: All claims expressed in this article are solely those of the authors and do not necessarily represent those of their affiliated organizations, or those of the publisher, the editors and the reviewers. Any product that may be evaluated in this article or claim that may be made by its manufacturer is not guaranteed or endorsed by the publisher.
Research integrity at Frontiers
Learn more about the work of our research integrity team to safeguard the quality of each article we publish.