- 1School of Medicine and School of Life Sciences, Shanghai University, Shanghai, China
- 2Department of Neurology and Central Laboratory, Putuo Hospital, Shanghai University of Traditional Chinese Medicine, Shanghai, China
Brain disorders, including stroke, Alzheimer's disease, depression, and chronic pain, are difficult to effectively treat. These major brain disorders have high incidence and mortality rates in the general population, and seriously affect not only the patient's quality of life, but also increases the burden of social medical care. Aerobic physical exercise is considered an effective adjuvant therapy for preventing and treating major brain disorders. Although the underlying regulatory mechanisms are still unknown, systemic processes may be involved. Here, this review aimed to reveal that aerobic physical exercise improved depression and several brain functions, including cognitive functions, and provided chronic pain relief. We concluded that aerobic physical exercise helps to maintain the regulatory mechanisms of brain homeostasis through anti-inflammatory mechanisms and enhanced synaptic plasticity and inhibition of hippocampal atrophy and neuronal apoptosis. In addition, we also discussed the cross-system mechanisms of aerobic exercise in regulating imbalances in brain function, such as the “bone-brain axis.” Furthermore, our findings provide a scientific basis for the clinical application of aerobic physical exercise in the fight against brain disorders.
Introduction
Physical exercise (PE) is a non-medical intervention that has been strongly validated by systematic reviews, statistical analyses, clinical examinations, and appropriate guidelines (1–3). Appropriate PE contributes to numerous physiological and psychological benefits, as well as a reduced tendency to develop chronic diseases, such as cardiovascular, cerebrovascular, and metabolic diseases (4, 5). PE can be divided into aerobic and resistance PE. The former includes running or cycling (6), which is better for cardiopulmonary health and reduces hippocampus decline (7–9). The latter improves bones and muscles by resisting external resistance through increased muscle strength (10). Therefore, in some chronic diseases, such as chronic heart failure (11), multiple sclerosis (12), and depression in older adults (13), PE may be a first-line treatment option.
The aerobic PE emphasized in this paper can be classified according to maximal oxygen uptake (VO2max) as low- (<45% VO2max), moderate- (45–64% VO2max), and high-intensity (70–85% VO2max) PE (14). The American College of Sports Medicine stated that moderate-intensity aerobic PE performed five or more days per week with complementary resistance exercises performed 2 or 3 days per week is beneficial to human health (15, 16). In addition, aerobic PE has two training methods: voluntary PE and forced PE. The former is a long-term self-development and self-sustainment therapy (17). The experimenters created voluntary animal models in an environment equipped with a running wheel (18), maze, or climbing gear (17). The latter refers to the voluntary use of mechanical assistance to achieve and maintain an ideal state of motion (19). Treadmills (20) and forced wheel-running (21) are often used in animal experiments to simulate this mode of movement. Forced PE can precisely control exercise intensity, and may be a better method to study the effects caused by different PE intensities, although cannot avoid individual differences among mice (22). A voluntary PE environment encourages mice to engage in low-intensity PE, such as free running; however, researchers are unable to control the amount of exercise performed (22). Moreover, forced PE seemed to produce more bromodeoxyuridine+ cells, although it increased anxiety-like behaviors in the animals (23). For some patients who are unable to perform voluntary PE, researchers first chose mechanically-assisted forced PE (19, 24–26).
Increasing evidence in recent years has focused on the notion that PE has positive effects on cognitive impairment, depression, and chronic pain. The first chapter of this paper confirms this fact using examples of some common neurodegenerative diseases that are associated with cognitive impairment, such as Alzheimer's disease (AD) (27) and Parkinson's Disease (PD) (28). In the case of AD, we have presented several studies that provide preclinical/clinical evidence (29, 30) supporting the recovery effect of aerobic PE on cognitive impairment. Next, we focused on depression. Depression is a common mood disorder that affects ~300 million people worldwide and was likely worsened in recent years because of the coronavirus disease 2019 pandemic, which exacerbated chronic stress related to work and school (31–33). Currently, the approach to managing depression is singular, and antidepressant drugs are typically used; however, this kind of drug treatment is ineffective for some patients who have a poor response and are likely to experience pharmacological side effects (34). The beneficial effects of aerobic PE on depression are clear (2, 35). Finally, we summarized the intervention effects of aerobic PE on chronic pain. Chronic pain is a serious threat to the health of the elderly, and has serious adverse effects on their physical, psychological, and social functions and increases the incidence of other complications in this population (36). There is an abundance of evidence that aerobic PE is a viable treatment option for chronic pain (37, 38), not only to improve the pain symptoms, but also to alleviate comorbidities such as sleep disturbances and poor memory (39, 40).
Chronic brain diseases may potentially share underlying pathophysiological mechanisms. In the second part of this paper, we discuss neuroinflammation (41–43), synaptic plasticity (43, 44), hippocampal volume, and neuronal apoptosis, which are associated with the pathological occurrence and development of common brain diseases, and clarify their relationships with aerobic PE. A genome-wide association study showed a high degree of genetic overlap between several mental disorders and pointed out that different mental disorders are not separate diseases but different overlapping phenotypes of the same clinical spectrum (35). Therefore, the use of the appropriate exercise types and intensities to intervene in a variety of brain diseases provides certain theory basis.
The brain has always been regarded as the “commander” of various organs, whereas bones have always been regarded as the “protector” and “supporter” of the human body. There seems to be no relationship, although recent studies have shown bilateral functional dependence between the two (45, 46). It is well-established that the brain influences bone regeneration and homeostasis through “efferent nerves” (47–49), and evidence is increasing that bones interfere with brain homeostasis through “afferent nerves” (50, 51). Bones are the main operators of exercise, and the beneficial effects of aerobic PE on bones have been proven (52, 53). Therefore, in the third part of this paper, we discuss several bone-derived proteins that may change brain function, and link them to Piezo1, a popular mechanical ion channel. Based on the above theories, this paper proposes a hypothesis that aerobic PE interferes with brain diseases through the bone-brain axis.
Aerobic PE Improves Various Functional Modalities
Aerobic PE Improves Cognitive Function
It is well-known that cognitive function declines with age, and the positive effects of aerobic PE on this decline have been well-demonstrated in rodents (54). In animal models of neurodegenerative diseases, including AD (55) and PD (56), PE has been repeatedly shown to up-regulate adult hippocampal neurogenesis and promote cognitive improvement in the aging brain (57). Among humans, the powerful benefits of aerobic PE are reflected decisively and vividly in the elderly (58). Compared with the sedentary elderly population, older adults who engage in PE have shown significant differences in bone mineral density, muscle content, and especially cognitive function (59). For instance, magnetic resonance imaging showed increased gray and white matter volume in the anterior cingulate cortex after 6 months of aerobic PE (60 min, 3 days per week) (60). In addition, aerobic PE is also beneficial in preventing AD. Older adults who were sedentary had a 53% higher prevalence than older adults who were more active (hazard ratio = 0.477, 95% confidence interval: 0.273–0.832) (61). The large, single-blinded, multi-center study showed that 16 weeks of aerobic PE increased oxygen volume (a marker of cardiorespiratory fitness) by 13%, leading to improvements in cognitive and neuropsychiatric symptoms (62). Similarly, aerobic PE reduces the progression of PD. Studies have shown that 6 months of aerobic PE leads to increased functional connectivity of the anterior putamen with the sensorimotor cortex relative to the posterior putamen and enhanced cognitive performance (63).
From the examples above, aerobic PE requires long-term adherence to show an advantage in terms of improving cognitive function. However, there is evidence that aerobic PE interventions do not improve symptoms in all age groups, such as the 60–80-year-old population (64–66). These contradictory results can be explained by the varying optimal types and intensities of PE among different age groups (67). Therefore, aerobic PE provides a low-cost and widely available intervention for improving cognition in the elderly, especially patients with AD.
Aerobic PE Can Fight Depression
Depression is a common mental disorder that threatens the physical and mental health of people worldwide and is a major cause of rising suicide rates in the 21st century (68). Doctors mainly diagnose the symptoms of some patients according to the Diagnostic and Statistical Manual of Mental Disorders (69) and International Classification of Diseases (70). However, there is little evidence on the mechanism of aerobic PE in regulating depression. Previous studies have shown that stress increases levels of kynurenic acid in the plasma and brains of mice (71) and leads to inhibited serotonin synthesis; mice could reduce the inevitable sense of helplessness caused by stress by reducing plasma kynurenic acid through 4 weeks of wheel running (71). Compared with the trained mice, mice without wheel running showed a stronger sense of helplessness in the tail suspension, escape, sugar water preference, and forced swimming tests (72). Notably, a similar phenomenon has been observed in human studies. Trivedi et al., based on clinical studies, suggested that 12 weeks of high-intensity exercise (70–85% maximum heart rate) was beneficial in reducing depression levels according to the Hamilton Depression Scale (P < 0.001) (73, 74).
Current research proves that aerobic PE increases the proportion of gray matter volume in the brain, improves the spatial structure of white matter, and leads to greater functional connectivity in the brain regions associated with major depression (75); its therapeutic effect was similar to that of antidepressants (76). The World Health Organization and National Institute for Health and Care Excellence guidelines recommend that for patients with mild to moderate depression, moderate aerobic PE should be performed in addition to standard drug treatment.
Aerobic PE in the Treatment of Chronic Pain
Recent statistics show that chronic pain affects 1.5 billion people worldwide, and that these numbers are steadily rising (77). Chronic pain is often accompanied by spontaneously progressive symptoms, including depression, anxiety, sleep disorders, intellectual disability, and anorexia, resulting in decreased quality of life for patients (78, 79). The 2021 International Association for the Study of Pain meta-analysis of 460 patients used a quality effects model. The Physiotherapy Evidence Database (PEDro) scale showed that the combination of pain neuroscience education and 12 weeks exercise had greater short-term improvements in chronic musculoskeletal pain severity, disability, kinesiophobia, and pain catastrophizing compared with those of exercise alone (80).
The core mechanism of aerobic PE in chronic pain is to inhibit local and systemic inflammation and prevent central sensitization (81–83). On the one hand, prolonged sitting leads to an imbalance in the proportion of cytokines in local and systemic circulation, resulting in a hyperinflammatory state that contributes to the onset and maintenance of chronic pain (84). Aerobic PE can reduce systemic inflammation and presence of proinflammatory cytokines and up regulate anti-inflammatory cytokines, allowing the neuroimmune signals in the central nervous system to be normalized. Chronic pain can be reduced, or hyperalgesia can be prevented and reversed (85, 86). On the other hand, in healthy people, aerobic PE releases endogenous opioids and acts as a pain reliever, an effect called “exercise-induced analgesia” (86, 87). Unfortunately, this mechanism does not apply to all patients with chronic diseases, as exercise-induced analgesia may be insensitive or even missing in some chronic pain diseases, such as fibromyalgia and chronic fatigue syndrome (88, 89). Furthermore, chronic pain is caused by genetics (90), stress, or sedentary (91) imbalances in central neurotransmitters such as serotonin, dopamine, and norepinephrine, whereas PE triggers a stress response in the neuroendocrine system, thereby changing the balance of these neurotransmitters (92).
Aerobic PE Maintains Brain Homeostasis Through Regulatory Mechanisms
Some studies have shown that exercise can reduce symptoms in people with brain damage (93–95). Unfortunately, while the benefits of exercise on brain and cognitive function are well-known, the mechanisms behind it not always been clear. Various chronic brain diseases have the same potential mechanisms. Here, we summarized several mechanisms of aerobic PE in alleviating brain diseases, including anti-inflammatory mechanisms, synaptic plasticity, hippocampal volume, and the apoptosis pathway of hippocampal neurons.
Effect of Aerobic PE on Brain Inflammation Through Anti-inflammatory Mechanisms
Microglia is the monitor and regulator of neuroinflammation (96). When the body endures a pathological injury, the microglia can be activated by pro-inflammatory factors (97) to mediate downstream signaling pathways that trigger inflammatory reactions and aggravate inflammation (98), or inhibited by anti-inflammatory factors that restore the body to homeostasis (99–101). Exercise can regulate microglial activity and inhibit neuroinflammation by increasing anti-inflammatory factors (102, 103). There are numerous examples that support this idea, such as animal studies that tested interleukin-6 (IL-6) (103, 104), interleukin-10 (IL-10) (105, 106), and CD200-CD200R (107, 108) levels before and after exercise; clinical trial data suggest the same thing (109, 110).
In addition to the anti-inflammatory factors mentioned above, Prof. Tony Wyss-Coray and his team found that the blood of mice produced the protein clusterin after one month of running on the wheel, which inhibited brain inflammation and promoted a large increase in the number of neurons and other cells, thereby improving cognitive impairment (111).
Effects of Aerobic PE on Synaptic Plasticity Through Neurotrophic Factors
Synaptic plasticity refers to the activity-dependent change in neuronal connection strength (112). Long-term potentiation is a persistent, activity-dependent increase in synaptic strength that occurs in response to repeated synaptic stimuli and is considered a common cellular manifestation of learning and memory. Some studies have confirmed that rats and mice undergoing running programs showed increased long-term potentiation at synapses in the hippocampus (68, 113), however, this has rarely been reported in clinical trials.
Aerobic exercise enhances synaptic plasticity in a variety of ways (114–116). For example, increased neurotrophic factors such as brain-derived neurotrophic factor (BDNF) and insulin-like growth factor 1 (IGF-1) induced by exercise training play an important role in promoting synaptic plasticity. BDNF can induce neurogenesis in the dentate gyrus of the hippocampus (117) and increase synaptic plasticity through calcium and calmodulin dependent kinase-mitogen-activated protein kinase activation mediated by tropomyosin receptor kinase B and N-methyl-D-aspartate receptors, followed by cAMP response element-binding protein activation (117). However, when tropomyosin receptor kinase B of BDNF was blocked during exercise, cognitive performance was impaired, and synaptic proteins in the hippocampus were reduced (118). In sedentary rats, upregulation of BDNF had a positive effect on the hippocampus (118). Therefore, BDNF plays an important role in synaptic plasticity (119). Similarly, intra hippocampal injection of IGF-1 functional blockers in mice demonstrated that IGF-1 signaling plays an important role in the effect of exercise on hippocampal dependent learning and plasticity (120).
Aerobic PE Intervenes in Brain Diseases by Preventing Hippocampal Atrophy
Hippocampal volume is a vital indicator of brain health that decreases with aging and neurological diseases, such as severe depression (121) and schizophrenia (122). The mechanisms involve a variety of molecular or cellular structures (123). Animal and clinical studies have consistently demonstrated that PE can effectively alleviate hippocampal atrophy (124–126). Moreover, a detailed study showed that in people with cognitive and mental health disorders (including younger and older people), proper aerobic PE for more than 6 months had a positive effect on hippocampal volume even in elderly people who are vulnerable to hippocampal atrophy (124). Participants returned to baseline levels after 6 weeks of inactivity, indicating that long-term aerobic PE is important for maintaining exercise-induced changes in hippocampal volume (127). In addition, although BDNF expression induced by aerobic PE is positively correlated with changes in hippocampal volume, there is no convincing evidence of the relationship between them (128, 129).
Aerobic PE Intervenes in Brain Diseases by Inhibiting Hippocampal Neuronal Apoptosis
Aerobic PE has two effects on hippocampal neuronal apoptosis. First, moderate exercise slows down hippocampal neuron injury caused by stress and inhibits neuronal apoptosis. Some studies have shown that the exocrine body derived from circulating endothelial progenitor cells can protect endothelial cells from hypoxia, and that moderate aerobic PE can enhance its function (130). C57BL/6 mice received moderate treadmill exercise (10 m/min) for 4 weeks following middle cerebral artery occlusion stroke. Compared with the control group, the apoptosis rate of trained mice decreased by 40% (131). In a D-galactose-induced aging rat model, swimming reportedly reduced Fas- and mitochondrial-dependent apoptotic pathways, significantly inhibited inflammatory signal activity, and also enhanced hippocampal survival pathways. Therefore, swimming can reduce the brain apoptosis and inflammatory signal activity induced by aging (132).
Excessive exercise can lead to cell damage and pathological apoptosis in multiple tissues and organs of the body. Besides skeletal muscle and cardiomyocytes, excessive exercise-induced injury and apoptosis were also found in hepatocytes, renal tubular cells, and lymphocytes in non-exercise systems (133). Currently, the research on nerve cell injury and apoptosis caused by excessive exercise is still in its infancy, mainly in the hippocampus. It can be observed that excessive exercise, like hunger, trauma, and other stressors, is a type of stress for the human body. Although moderate exercise causes benign stress that is beneficial to the human body, excessive exercise can lead to excessive stress, which causes the arrangement of hippocampal neurons to become loose and disordered with reduced communication and nuclear pyknosis, resulting in multi-system damage (134, 135) that can even lead to overtraining syndrome (136).
The Molecular Mechanism of Aerobic PE Regulating the Brain Through the Bone-Brain Axis
Bone and skeletal muscle are the two major organs mainly affected by exercise in the body; bones are regarded as scaffolds that support and protect various organs in the body (137). Muscles transmit and receive the mechanical force caused by exercise and are therefore also considered an endocrine organ (138). Piezo1 in the Piezo family is a mechanically activated ion channel (139) that acts as a mechanical sensor in osteoblasts and osteocytes. It is beneficial to the formation of bone trabeculae in the process of endochondral ossification and reportedly increased the bone thickness of mice (140, 141) and mediated the Piezo1/Yes-associated protein1 (YAP1)-collagen pathway to indirectly regulate the bone resorption activity of osteoclasts, thus affecting bone metabolism (141). Moreover, mechanical unloading can inhibit the expression of piezo and slow down osteoblast and bone formation. A previous study found that Piezo1 promoted the expression of Wnt1 in osteocytes by activating YAP1 and transcriptional coactivator with PDZ-binding motif (TAZ) (142). Piezo1 activates the Wnt1 signal pathway in osteocytes and leads to increased bone formation and decreased bone resorption (142). Recently, Sasaki et al. found that Piezo1, a mechanically-sensitive ion channel, mediated the phosphorylation of protein kinase B in osteocytes and down-regulated the expression of sclerosing proteins (143). Because sclerostin leads to bone mass loss, Piezo1 in osteocytes inhibits the expression of sclerosis proteins and promotes osteoblast formation by activating the protein kinase B signal pathway. Bone tissue regulates brain function mainly through osteoblast secretion of a variety of proteins (Table 1), including osteocalcin (OCN), lipid delivery protein-2 (LCN2), and osteopontin (OPN); cells such as bone-derived mesenchymal stem cells, hematopoietic stem cells, and microglia-like cells are also provided (144).
OCN
OCN, also known as bone γ-carboxyl glutamate protein, is uniquely secreted by osteoblasts (145). It plays an important role in the regulation of bone calcium metabolism and is a new biochemical marker in the study of bone metabolism, which has important value in the diagnosis of osteoporosis syndrome (146) and other diseases such as abnormal calcium metabolism (144, 147). In the peripheral nervous system, OCN binds to G protein-coupled receptor family C group 6 (Gprc6a), and regulates hormone levels, including insulin and testosterone, to promote skeletal muscle adaptation to PE. In the brain, OCN binds to G protein-coupled receptor 158 (Gpr158) and can cross the blood-brain barrier (BBB) to regulate transcription factors in the ventral tegmental area (VTA), dorsal raphe nucleus, middle raphe nucleus, and hippocampal CA3 neurons, thereby increasing the release of serotonin, dopamine, and norepinephrine, inhibiting the release of γ-aminobutyric acid (148, 149), and reducing depression and anxiety-like behaviors. Gpr158 was the first OCN receptor found in the brain. It is present in the somatosensory, motor, and auditory areas of the cortex, as well as in the piriform cortex, hippocampus, post splenic area, and ventral tegmental area. Significant decreases in OCN levels in elderly mice with cognitive impairment and patients with depression have been reported (150), although injection of exogenous OCN can reverse these defects (148).
LCN2
LCN2 is another hormone known as a neutrophil gelatinase-associated lipid carrier protein, which is a secretory glycoprotein (151). LCN2 was previously considered to be a lipid-derived factor (152), however the expression profile showed that the expression of LCN2 in bone was 10 times higher than that in adipose or other tissues; therefore, it is also a bone-derived factor (50). Similar to OCN, LCN2 acts directly on β cells to promote their proliferation as well as insulin secretion (153). Recently, researchers at Columbia University Medical Center found that LCN2 proteins secreted by osteocytes not only induce insulin secretion, but also cross the BBB and activate the anorexigenic (appetite-suppressing) pathway by binding to melanocortin 4 receptors in the hypothalamus, thereby controlling body weight, fat content, and insulin sensitivity (50). LCN2 also enhances neuronal motor and inflammatory responses by activating Janus kinase 2-activator of transcription-3 crosstalk (154) and nuclear factor kappa B pathways (155) to up-regulate the expression of chemokine (C-X-C motif) ligand 10 (156). LCN2 levels in brain tissue and astrocyte cultures of rats with ischemic stroke and astrocytes treated with standardized hypoxia were reportedly significantly increased, while BBB permeability, neurological impairment, cerebral infarction, and neutrophil infiltration were decreased in LCN2-deficient rats (157, 158). Further studies found that LCN2 promotes neuroinflammation by activating neutrophil infiltration, microglia, and astrocytes and inducing proinflammatory cytokines and chemokines (159–161). These results suggest that LCN2, neuropathology, and PE are inextricably linked.
OPN
OPN is a secretory stromal cell protein found in bones (162). It was subsequently proven to be expressed in epithelial lining, skeletal muscle, and breast and brain tissue (163, 164). OPN plays an important role in tissue remodeling, immunomodulation, and biomineralization by binding to diverse receptors, such as integrins and CD44 (165–167). In bone tissue, OPN can anchor osteoclasts to the bone mineral matrix to promote bone resorption (167–169); therefore, patients with high serum OPN concentrations have low bone mineral densities, whereas patients with low serum OPN concentrations have high bone mineral densities (170). In the brain, OPN forms different fragments after protease cleavage. These fragments can bind to different receptors (CD44 and integrin) to activate P42/44 mitogen-activated protein kinase and phosphoinositide 3 kinase pathways that have a neuroprotective function (171). OPN can also activate c-Jun N-terminal kinase and extracellular regulated protein kinase pathways to cause neuroinflammation by up-regulating proinflammatory gene expression (172, 173). In addition, OPN can act as a pro-inflammatory cytokine to recruit inflammatory cells to the lesion site and cause nervous system disease (174, 175). Therefore, OPN is an important factor in regulating bone mass and triggering neuroinflammation, and it is particularly crucial to explore the secretion mechanisms of OPN regulated by aerobic PE.
Conclusion
Human and animal studies have shown that aerobic PE has significant effects on many aspects of brain function, including preventing and improving cognitive function, depression, and chronic pain. In this paper (Figure 1), we discussed four regulatory mechanisms of aerobic PE in the intervention of neurological diseases, including anti-inflammatory pathways related to microglia, promotion of hippocampal synaptic plasticity through a variety of neurotrophic factors, and prevention of hippocampal atrophy and neuronal apoptosis. Furthermore, we have introduced a novel mechanism of bone-brain axis regulation (Figure 2), although its research is still in its infancy.
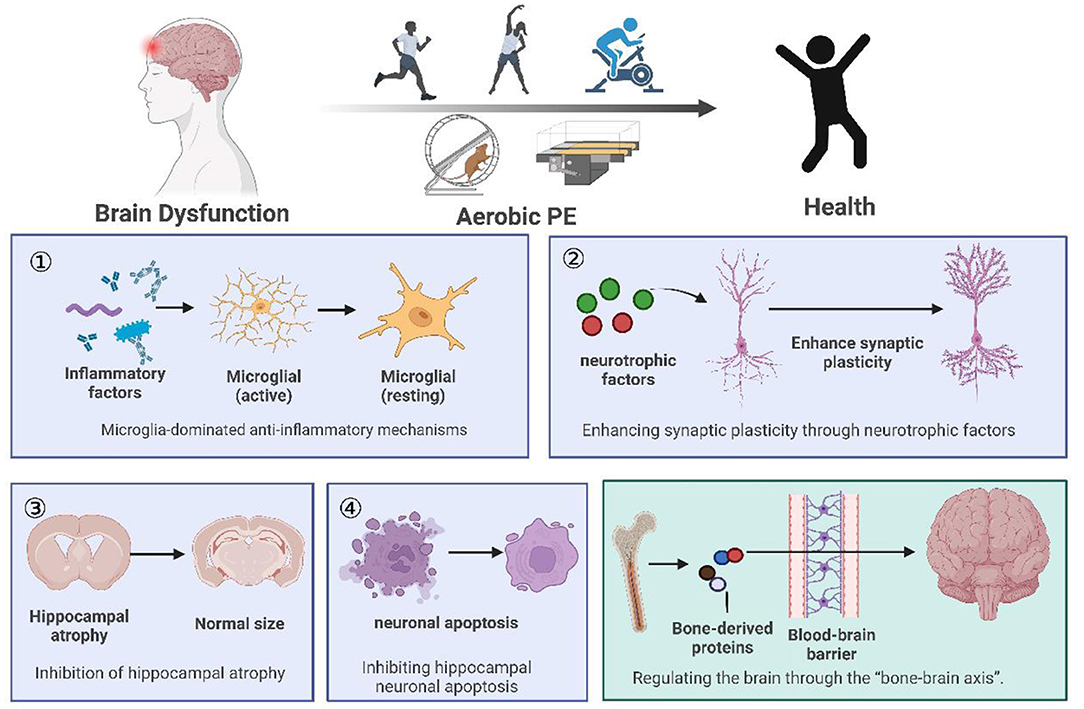
Figure 1. Summary of the review. (1) We listed animal and clinical experiments on the intervention of aerobic PE in brain dysfunction, and concluded that aerobic PE can improve cognition, fight depression, and relieve chronic pain. (2) Aerobic PE interferes with brain disorder through four common mechanisms: anti-inflammatory mechanisms, synaptic plasticity, hippocampal atrophy, and hippocampal neuronal apoptosis. (3) We propose the hypothesis that aerobic PE interferes with brain disease through the bone-brain axis.
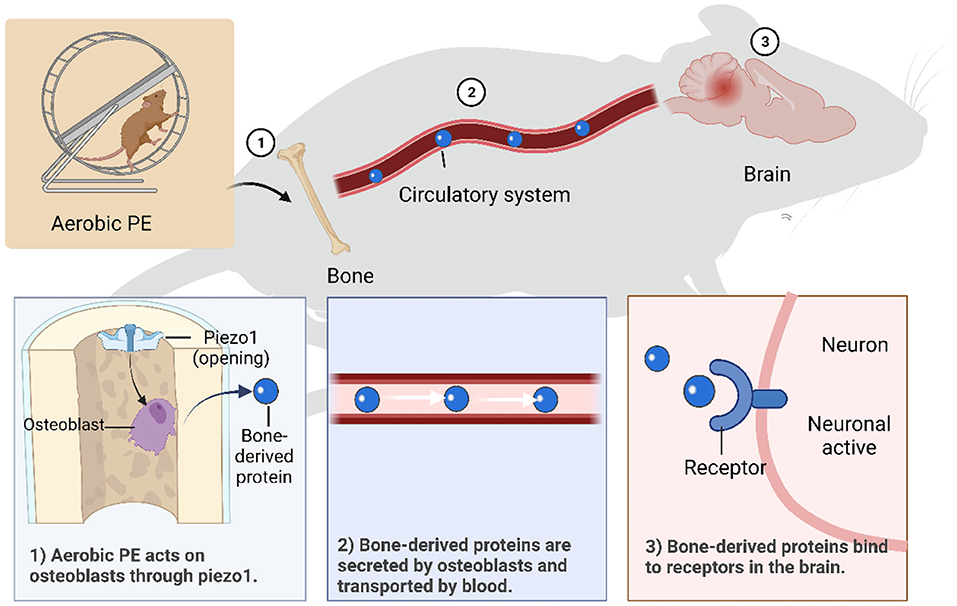
Figure 2. Mechanisms of aerobic PE intervening in brain dysfunction through bone-brain axis. (1) Piezo1 is activated by aerobic PE, which promotes osteoblast growth and secretion of bone- derived proteins. (2) Bone-derived proteins are transported in blood and can cross the blood-brain barrier. (3) Bone-derived proteins bind to specific receptors on neurons to function.
An appropriate amount of aerobic PE activates beneficial mechanisms in the body. Effective aerobic PE causes tissues to release IL-1α to activate ion channel Piezo1 (176) and promotes osteoblast formation through integrin β1 and integrin-focal adhesion kinase pathways (177). As previously mentioned, bone tissue regulates inflammation mainly by secreting a variety of factors related to neuroinflammation, including OCN (147) and OPN (170), and promotes subdivided secretion of cytokines, such as bone-derived mesenchymal stem, hematopoietic stem, and microglia-like cells (178). Therefore, regulatory factors from bone can pass through the BBB and regulate transcription factors in neurons in various regions of the brain, thereby increasing the release of related hormones and reducing the occurrence of neuroinflammation. We can conclude that aerobic PE activates Piezo1 through skeletal muscle pressure, promotes osteoblasts to secrete bone-derived proteins, and interferes with related nerve inflammation through the bone-brain axis. However, exercise intensity, time, and frequency are critical to the effects of exercise; painful exercise can aggravate nerve inflammation. Furthermore, acute high-intensity exercise with higher than normal duration or without physical adaptation level induces oxidative stress (179) and muscle injury (180).
In conclusion, it is necessary to establish different exercise intensities, times, frequencies, and even exercise methods for different backgrounds on an individual basis. Although the study carried a large workload, it can have a profound impact as a non-medical intervention.
Author Contributions
YJia, YY, LZ, XC, CY, YJi, and JT drafted the manuscript and revised it critically for intellectual content. YZ drew the table. All authors read and approved the final version of the manuscript before submission.
Funding
This work was supported by grants from National Key Research and Development Program (No. 2020YFA0803800), National Natural Science Foundation of China (Nos. 31771191, 82074162, and 81903995), Young Elite Scientists Sponsorship Program by CACM (No. CACM-2019-QNRC2-C10), Project for Capacity Promotion of Putuo District Clinical Special Disease (No. 2019tszb02), Science, Technology Innovation Project of Putuo District Health System (Nos. ptkwws201902, ptkwws201908, and ptkwws202107), and the One Hundred Talents Project of Putuo Hospital, Shanghai University of Traditional Chinese Medicine (No. 2022LH002).
Conflict of Interest
The authors declare that the research was conducted in the absence of any commercial or financial relationships that could be construed as a potential conflict of interest.
Publisher's Note
All claims expressed in this article are solely those of the authors and do not necessarily represent those of their affiliated organizations, or those of the publisher, the editors and the reviewers. Any product that may be evaluated in this article, or claim that may be made by its manufacturer, is not guaranteed or endorsed by the publisher.
References
1. Jaworska N, Courtright AK, De Somma E, MacQueen GM, MacMaster FP. Aerobic exercise in depressed youth: a feasibility and clinical outcomes pilot. Early Interv Psychiatry. (2019) 13:128–32. doi: 10.1111/eip.12537
2. Morres ID, Hatzigeorgiadis A, Stathi A, Comoutos N, Arpin-Cribbie C, Krommidas C, et al. Aerobic exercise for adult patients with major depressive disorder in mental health services: a systematic review and meta-analysis. Depress Anxiety. (2019) 36:39–53. doi: 10.1002/da.22842
3. Belvederi Murri M, Ekkekakis P, Magagnoli M, Zampogna D, Cattedra S, Capobianco L, et al. Physical exercise in major depression: reducing the mortality gap while improving clinical outcomes. Front Psychiatry. (2018) 9:762. doi: 10.3389/fpsyt.2018.00762
4. Warburton DE, Nicol CW, Bredin SS. Health benefits of physical activity: the evidence. CMAJ. (2006) 174:801–9. doi: 10.1503/cmaj.051351
5. Mandolesi L, Polverino A, Montuori S, Foti F, Ferraioli G, Sorrentino P, et al. Effects of physical exercise on cognitive functioning and wellbeing: biological and psychological benefits. Front Psychol. (2018) 9:509. doi: 10.3389/fpsyg.2018.00509
6. Guiney H, Machado L. Benefits of regular aerobic exercise for executive functioning in healthy populations. Psychon Bull Rev. (2013) 20:73–86. doi: 10.3758/s13423-012-0345-4
7. Crimi E, Ignarro LJ, Cacciatore F, Napoli C. Mechanisms by which exercise training benefits patients with heart failure. Nat Rev Cardiol. (2009) 6:292–300. doi: 10.1038/nrcardio.2009.8
8. Aghjayan SL, Lesnovskaya A, Esteban-Cornejo I, Peven JC, Stillman CM, Erickson KI. Aerobic exercise, cardiorespiratory fitness, and the human hippocampus. Hippocampus. (2021) 31:817–44. doi: 10.1002/hipo.23337
9. Ruilian L, Honglin Q, Jun X, Jianxin L, Qingyun B, Yilin C, et al. HS-mediated aerobic exercise antagonizes the hippocampal inflammatory response in CUMS-depressed mice. J Affect Disord. (2021) 283:410–9. doi: 10.1016/j.jad.2021.02.005
10. Hashida R, Kawaguchi T, Bekki M, Omoto M, Matsuse H, Nago T, et al. Aerobic vs. resistance exercise in non-alcoholic fatty liver disease: a systematic review. J Hepatol. (2017) 66:142–52. doi: 10.1016/j.jhep.2016.08.023
11. Cattadori G, Segurini C, Picozzi A, Padeletti L, Anzà C. Exercise and heart failure: an update. ESC Heart Fail. (2018) 5:222–32. doi: 10.1002/ehf2.12225
12. Reynolds ER, Ashbaugh AD, Hockenberry BJ, McGrew CA. Multiple sclerosis and exercise: a literature review. Curr Sports Med Rep. (2018) 17:31–5. doi: 10.1249/JSR.0000000000000446
13. López-Torres Hidalgo J. Effectiveness of physical exercise in the treatment of depression in older adults as an alternative to antidepressant drugs in primary care. BMC Psychiatry. (2019) 19:21. doi: 10.1186/s12888-018-1982-6
14. Boutcher YN, Boutcher SH. Exercise intensity and hypertension: what's new? J Hum Hypertens. (2017) 31:157–64. doi: 10.1038/jhh.2016.62
15. Garber CE, Blissmer B, Deschenes MR, Franklin BA, Lamonte MJ, Lee I-M., et al. Quantity and quality of exercise for developing and maintaining cardiorespiratory, musculoskeletal, and neuromotor fitness in apparently healthy adults: guidance for prescribing exercise. Med Sci Sports Exerc. (2011) 43:1334–59. doi: 10.1249/MSS.0b013e318213fefb
16. Hills AP, Byrne NM, Ramage AJ. Submaximal markers of exercise intensity. J Sports Sci. (1998) 16(Suppl):S71–6. doi: 10.1080/026404198366696
17. Carroll ME. Voluntary exercise as a treatment for incubated and expanded drug craving leading to relapse to addiction: animal models. Pharmacol Biochem Behav. (2021) 208:173210. doi: 10.1016/j.pbb.2021.173210
18. Lapveteläinen T, Tiihonen A, Koskela P, Nevalainen T, Lindblom J, Király K, et al. Training a large number of laboratory mice using running wheels and analyzing running behavior by use of a computer-assisted system. Lab Anim Sci. (1997) 47:172–9.
19. Miner DG, Aron A, DiSalvo E. Therapeutic effects of forced exercise cycling in individuals with Parkinson's disease. J Neurol Sci. (2020) 410:116677. doi: 10.1016/j.jns.2020.116677
20. Kemi OJ, Loennechen JP, Wisløff U, Ellingsen Ø. Intensity-controlled treadmill running in mice: cardiac and skeletal muscle hypertrophy. J Appl Physiol. (2002) 93:1301–9. doi: 10.1152/japplphysiol.00231.2002
21. Kim TK, Kim JE, Park JY, Lee JE, Choi J, Kim H, et al. Antidepressant effects of exercise are produced via suppression of hypocretin/orexin and melanin-concentrating hormone in the basolateral amygdala. Neurobiol Dis. (2015) 79:59–69. doi: 10.1016/j.nbd.2015.04.004
22. Guo S, Huang Y, Zhang Y, Huang H, Hong S, Liu T. Impacts of exercise interventions on different diseases and organ functions in mice. J Sport Health Sci. (2020) 9:53–73. doi: 10.1016/j.jshs.2019.07.004
23. Leasure JL, Jones M. Forced and voluntary exercise differentially affect brain and behavior. Neuroscience. (2008) 156:456–65. doi: 10.1016/j.neuroscience.2008.07.041
24. Linder SM, Rosenfeldt AB, Davidson S, Zimmerman N, Penko A, Lee J, et al. Forced, not voluntary, aerobic exercise enhances motor recovery in persons with chronic stroke. Neurorehabil Neural Repair. (2019) 33:681–90. doi: 10.1177/1545968319862557
25. Rosenfeldt AB, Linder SM, Davidson S, Clark C, Zimmerman NM, Lee JJ, et al. Combined aerobic exercise and task practice improve health-related quality of life poststroke: a preliminary analysis. Arch Phys Med Rehabil. (2019) 100:923–30. doi: 10.1016/j.apmr.2018.11.011
26. Iqbal M, Rahman MS, Zafar S, Chen XL, Liu JX, Liu Y. Systematic review and meta-analysis of the efficacy of different exercise programs in pilocarpine induced status epilepticus models. Epilepsy Behav. (2017) 73:256–67. doi: 10.1016/j.yebeh.2017.06.007
27. Iqbal K, Grundke-Iqbal I. Alzheimer's disease, a multifactorial disorder seeking multitherapies. Alzheimers Dement. (2010) 6:420–4. doi: 10.1016/j.jalz.2010.04.006
28. Xu X, Fu Z, Le W. Exercise and Parkinson's disease. Int Rev Neurobiol. (2019) 147:45–74. doi: 10.1016/bs.irn.2019.06.003
29. De la Rosa A, Olaso-Gonzalez G, Arc-Chagnaud C, Millan F, Salvador-Pascual A, García-Lucerga C, et al. Physical exercise in the prevention and treatment of Alzheimer's disease. J Sport Health Sci. (2020) 9:394–404. doi: 10.1016/j.jshs.2020.01.004
30. Cass SP. Alzheimer's disease and exercise: a literature review. Curr Sports Med Rep. (2017) 16:19–22. doi: 10.1249/JSR.0000000000000332
31. Stanton R, To QG, Khalesi S, Williams SL, Alley SJ, Thwaite TL, et al. Depression, anxiety and stress during COVID-19: associations with changes in physical activity, sleep, tobacco and alcohol use in australian adults. Int J Environ Res Public Health. (2020) 17:4065. doi: 10.3390/ijerph17114065
32. Dubey S, Biswas P, Ghosh R, Chatterjee S, Dubey MJ, Chatterjee S, et al. Psychosocial impact of COVID-19. Diabetes Metab Syndr. (2020) 14:779–88. doi: 10.1016/j.dsx.2020.05.035
33. Salari N, Hosseinian-Far A, Jalali R, Vaisi-Raygani A, Rasoulpoor S, Mohammadi M, et al. Prevalence of stress, anxiety, depression among the general population during the COVID-19 pandemic: a systematic review and meta-analysis. Global Health. (2020) 16:57. doi: 10.1186/s12992-020-00589-w
34. Penn E, Tracy DK. The drugs don't work? antidepressants and the current and future pharmacological management of depression. Ther Adv Psychopharmacol. (2012) 2:179–88. doi: 10.1177/2045125312445469
35. Kvam S, Kleppe CL, Nordhus IH, Hovland A. Exercise as a treatment for depression: a meta-analysis. J Affect Disord. (2016) 202:67–86. doi: 10.1016/j.jad.2016.03.063
36. Anderson KO, Green CR, Payne R. Racial and ethnic disparities in pain: causes and consequences of unequal care. J Pain. (2009) 10:1187–204. doi: 10.1016/j.jpain.2009.10.002
37. Borisovskaya A, Chmelik E, Karnik A. Exercise and chronic pain. In: Xiao J, editor. Physical Exercise for Human Health. Singapore: Springer Singapore (2020).
38. Ambrose KR, Golightly YM. Physical exercise as non-pharmacological treatment of chronic pain: why and when. Best Pract Res Clin Rheumatol. (2015) 29:120–30. doi: 10.1016/j.berh.2015.04.022
39. Clauw DJ. Diagnosing and treating chronic musculoskeletal pain based on the underlying mechanism(s). Best Pract Res Clin Rheumatol. (2015) 29:6–19. doi: 10.1016/j.berh.2015.04.024
40. Mansfield KE, Sim J, Jordan JL, Jordan KP. A systematic review and meta-analysis of the prevalence of chronic widespread pain in the general population. Pain. (2016) 157:55–64. doi: 10.1097/j.pain.0000000000000314
41. Heneka MT, Carson MJ, El Khoury J, Landreth GE, Brosseron F, Feinstein DL, et al. Neuroinflammation in Alzheimer's disease. Lancet Neurol. (2015) 14:388–405. doi: 10.1016/S1474-4422(15)70016-5
42. Hirsch EC, Hunot S. Neuroinflammation in Parkinson's disease: a target for neuroprotection? Lancet Neurol. (2009) 8:382–97. doi: 10.1016/S1474-4422(09)70062-6
43. Lotharius J, Brundin P. Pathogenesis of Parkinson's disease: dopamine, vesicles and alpha-synuclein. Nat Rev Neurosci. (2002) 3:932–42. doi: 10.1038/nrn983
44. López-Figueroa AL, Norton CS, López-Figueroa MO, Armellini-Dodel D, Burke S, Akil H, et al. Serotonin 5-HT1A, 5-HT1B, and 5-HT2A receptor mRNA expression in subjects with major depression, bipolar disorder, and schizophrenia. Biol Psychiatry. (2004) 55:225–33. doi: 10.1016/j.biopsych.2003.09.017
45. Chamouni A, Schreiweis C, Oury F. Bone, brain and beyond. Rev Endocr Metab Disord. (2015) 16:99–113. doi: 10.1007/s11154-015-9312-5
46. Rousseaud A, Moriceau S, Ramos-Brossier M, Oury F. Bone-brain crosstalk and potential associated diseases. Horm Mol Biol Clin Investig. (2016) 28:69–83. doi: 10.1515/hmbci-2016-0030
47. Gualano B, Rawson ES, Candow DG, Chilibeck PD. Creatine supplementation in the aging population: effects on skeletal muscle, bone and brain. Amino Acids. (2016) 48:1793–805. doi: 10.1007/s00726-016-2239-7
48. Xia W, Xie J, Cai Z, Liu X, Wen J, Cui ZK, et al. Damaged brain accelerates bone healing by releasing small extracellular vesicles that target osteoprogenitors. Nat Commun. (2021) 12:6043. doi: 10.1038/s41467-021-26302-y
49. Idelevich A, Baron R. Brain to bone: what is the contribution of the brain to skeletal homeostasis? Bone. (2018) 115:31–42. doi: 10.1016/j.bone.2018.05.018
50. Mosialou I, Shikhel S, Liu JM, Maurizi A, Luo N, He Z, et al. MC4R-dependent suppression of appetite by bone-derived lipocalin 2. Nature. (2017) 543:385–90. doi: 10.1038/nature21697
51. Frame G, Bretland KA, Dengler-Crish CM. Mechanistic complexities of bone loss in Alzheimer's disease: a review. Connect Tissue Res. (2020) 61:4–18. doi: 10.1080/03008207.2019.1624734
52. Benedetti MG, Furlini G, Zati A, Letizia Mauro G. The effectiveness of physical exercise on bone density in osteoporotic patients. Biomed Res Int. (2018) 2018:4840531. doi: 10.1155/2018/4840531
53. Tong X, Chen X, Zhang S, Huang M, Shen X, Xu J, et al. The effect of exercise on the prevention of osteoporosis and bone angiogenesis. Biomed Res Int. (2019) 2019:8171897. doi: 10.1155/2019/8171897
54. van Praag H, Shubert T, Zhao C, Gage FH. Exercise enhances learning and hippocampal neurogenesis in aged mice. J Neurosci. (2005) 25:8680–5. doi: 10.1523/JNEUROSCI.1731-05.2005
55. Nichol K, Deeny SP, Seif J, Camaclang K, Cotman CW. Exercise improves cognition and hippocampal plasticity in APOE epsilon4 mice. Alzheimers Dement. (2009) 5:287–94. doi: 10.1016/j.jalz.2009.02.006
56. Tillerson JL, Caudle WM, Reverón ME, Miller GW. Exercise induces behavioral recovery and attenuates neurochemical deficits in rodent models of Parkinson's disease. Neuroscience. (2003) 119:899–911. doi: 10.1016/S0306-4522(03)00096-4
57. Yau SY, Gil-Mohapel J, Christie BR, So KF. Physical exercise-induced adult neurogenesis: a good strategy to prevent cognitive decline in neurodegenerative diseases? Biomed Res Int. (2014) 2014:403120. doi: 10.1155/2014/403120
58. Galloza J, Castillo B, Micheo W. Benefits of exercise in the older population. Phys Med Rehabil Clin N Am. (2017) 28:659–69. doi: 10.1016/j.pmr.2017.06.001
59. Mora JC, Valencia WM. Exercise and older adults. Clin Geriatr Med. (2018) 34:145–62. doi: 10.1016/j.cger.2017.08.007
60. Colcombe SJ, Erickson KI, Scalf PE, Kim JS, Prakash R, McAuley E, et al. Aerobic exercise training increases brain volume in aging humans. J Gerontol A Biol. (2006) 61:1166–70. doi: 10.1093/gerona/61.11.1166
61. Buchman AS, Boyle PA, Yu L, Shah RC, Wilson RS, Bennett DA. Total daily physical activity and the risk of AD and cognitive decline in older adults. Neurology. (2012) 78:1323–9. doi: 10.1212/WNL.0b013e3182535d35
62. Sobol NA, Dall CH, Høgh P, Hoffmann K, Frederiksen KS, Vogel A, et al. Change in fitness and the relation to change in cognition and neuropsychiatric symptoms after aerobic exercise in patients with mild Alzheimer's disease. J Alzheimer's Dis. (2018) 65:137–45. doi: 10.3233/JAD-180253
63. Johansson ME, Cameron IGM, Van der Kolk NM, de Vries NM, Klimars E, Toni I, et al. Aerobic exercise alters brain function and structure in parkinson's disease: a randomized controlled trial. Ann Neurol. (2022) 91:203–16. doi: 10.1002/ana.26291
64. Blumenthal JA, Emery CF, Madden DJ, Schniebolk S, Walsh-Riddle M, George LK, et al. Long-term effects of exercise on psychological functioning in older men and women. J Gerontol. (1991) 46:P352–61. doi: 10.1093/geronj/46.6.P352
65. Hill RD, Storandt M, Malley M. The impact of long-term exercise training on psychological function in older adults. J Gerontol. (1993) 48:P12–7. doi: 10.1093/geronj/48.1.P12
66. Panton LB, Graves JE, Pollock ML, Hagberg JM, Chen W. Effect of aerobic and resistance training on fractionated reaction time and speed of movement. J Gerontol. (1990) 45:M26–31. doi: 10.1093/geronj/45.1.M26
67. Morris JK, Vidoni ED, Johnson DK, Van Sciver A, Mahnken JD, Honea RA, et al. Aerobic exercise for Alzheimer's disease: a randomized controlled pilot trial. PLoS ONE. (2017) 12:e0170547. doi: 10.1371/journal.pone.0170547
68. O'Callaghan RM, Ohle R, Kelly AM. The effects of forced exercise on hippocampal plasticity in the rat: a comparison of LTP, spatial- and non-spatial learning. Behav Brain Res. (2007) 176:362–6. doi: 10.1016/j.bbr.2006.10.018
70. Jessen F, Frölich L. [ICD-11: neurocognitive disorders]. Fortschr Neurol Psychiatr. (2018) 86:172–7. doi: 10.1055/s-0044-101607
71. Su CH, Chuang HC, Hong CJ. Physical exercise prevents mice from L-Kynurenine-induced depression-like behavior. Asian J Psychiatr. (2020) 48:101894. doi: 10.1016/j.ajp.2019.101894
72. Quak J, Doornbos B, Roest AM, Duivis HE, Vogelzangs N, Nolen WA, et al. Does tryptophan degradation along the kynurenine pathway mediate the association between pro-inflammatory immune activity and depressive symptoms? Psychoneuroendocrinology. (2014) 45:202–10. doi: 10.1016/j.psyneuen.2014.03.013
73. Hanssen H, Minghetti A, Faude O, Schmidt-Trucksäss A, Zahner L, Beck J, et al. Effects of endurance exercise modalities on arterial stiffness in patients suffering from unipolar depression: a randomized controlled trial. Front Psychiatry. (2017) 8:311. doi: 10.3389/fpsyt.2017.00311
74. Trivedi MH, Greer TL, Church TS, Carmody TJ, Grannemann BD, Galper DI, et al. Exercise as an augmentation treatment for nonremitted major depressive disorder: a randomized, parallel dose comparison. J Clin Psychiatry. (2011) 72:677–84. doi: 10.4088/JCP.10m06743
75. Repple J, Opel N. [Sport and physical exercise in unipolar depression : Prevention, therapy, and neurobiological mechanisms of action]. Nervenarzt. (2021) 92:507–14. doi: 10.1007/s00115-021-01113-0
76. Helgadóttir B, Hallgren M, Ekblom Ö, Forsell Y. Training fast or slow? Exercise for depression: a randomized controlled trial. Prev Med. (2016) 91:123–31. doi: 10.1016/j.ypmed.2016.08.011
77. Steglitz J, Buscemi J, Ferguson MJ. The future of pain research, education, and treatment: a summary of the IOM report “Relieving pain in America: a blueprint for transforming prevention, care, education, and research”. Transl Behav Med. (2012) 2:6–8. doi: 10.1007/s13142-012-0110-2
78. Zhuo M. Descending facilitation. Mol Pain. (2017) 13:1744806917699212. doi: 10.1177/1744806917699212
79. Finnerup NB, Kuner R, Jensen TS. Neuropathic pain: from mechanisms to treatment. Physiol Rev. (2021) 101:259–301. doi: 10.1152/physrev.00045.2019
80. Siddall B, Ram A, Jones MD, Booth J, Perriman D, Summers SJ. Short-term impact of combining pain neuroscience education with exercise for chronic musculoskeletal pain: a systematic review and meta-analysis. Pain. (2022) 163:e20–30. doi: 10.1097/j.pain.0000000000002308
81. Nijs J, Lluch Girbés E, Lundberg M, Malfliet A, Sterling M. Exercise therapy for chronic musculoskeletal pain: innovation by altering pain memories. Man Ther. (2015) 20:216–20. doi: 10.1016/j.math.2014.07.004
82. Paley CA, Johnson MI. Physical activity to reduce systemic inflammation associated with chronic pain and obesity: a narrative review. Clin J Pain. (2016) 32:365–70. doi: 10.1097/AJP.0000000000000258
83. Moseley GL, Butler DS. Fifteen years of explaining pain: the past, present, and future. J Pain. (2015) 16:807–13. doi: 10.1016/j.jpain.2015.05.005
84. Sluka KA, O'Donnell JM, Danielson J, Rasmussen LA. Regular physical activity prevents development of chronic pain and activation of central neurons. J Appl Physiol. (2013) 114:725–33. doi: 10.1152/japplphysiol.01317.2012
85. Cooper MA, Kluding PM, Wright DE. Emerging relationships between exercise, sensory nerves, and neuropathic pain. Front Neurosci. (2016) 10:372. doi: 10.3389/fnins.2016.00372
86. Sluka KA, Frey-Law L, Hoeger Bement M. Exercise-induced pain and analgesia? Underlying mechanisms and clinical translation. Pain. (2018) 159(Suppl. 1):S91–7. doi: 10.1097/j.pain.0000000000001235
87. Naugle KM, Fillingim RB, Riley JL 3rd. A meta-analytic review of the hypoalgesic effects of exercise. J Pain. (2012) 13:1139–50. doi: 10.1016/j.jpain.2012.09.006
88. Nijs J, Kosek E, Van Oosterwijck J, Meeus M. Dysfunctional endogenous analgesia during exercise in patients with chronic pain: to exercise or not to exercise? Pain Physician. (2012) 15(3 Suppl):ES205–13. doi: 10.36076/ppj.2012/15/ES205
89. Daenen L, Varkey E, Kellmann M, Nijs J. Exercise, not to exercise, or how to exercise in patients with chronic pain? applying science to practice. Clin J Pain. (2015) 31:108–14. doi: 10.1097/AJP.0000000000000099
90. Crofford LJ. Chronic pain: where the body meets the brain. Trans Am Clin Climatol Assoc. (2015) 126:167–83.
91. Tajerian M, Clark JD. Nonpharmacological Interventions in targeting pain-related brain plasticity. Neural Plast. (2017) 2017:2038573. doi: 10.1155/2017/2038573
92. Geneen LJ, Moore RA, Clarke C, Martin D, Colvin LA, Smith BH. Physical activity and exercise for chronic pain in adults: an overview of cochrane reviews. Cochrane Database Syst Rev. (2017) 1:Cd011279. doi: 10.1002/14651858.CD011279.pub2
93. Toscano CVA, Barros L, Lima AB, Nunes T, Carvalho HM, Gaspar JM. Neuroinflammation in autism spectrum disorders: exercise as a “pharmacological” tool. Neurosci Biobehav Rev. (2021) 129:63–74. doi: 10.1016/j.neubiorev.2021.07.023
94. Xie Y, Wu Z, Sun L, Zhou L, Wang G, Xiao L, et al. The effects and mechanisms of exercise on the treatment of depression. Front Psychiatry. (2021) 12:705559. doi: 10.3389/fpsyt.2021.705559
95. Lee B, Shin M, Park Y, Won SY, Cho KS. Physical exercise-induced myokines in neurodegenerative diseases. Int J Mol Sci. (2021) 22:5795. doi: 10.3390/ijms22115795
96. Perry VH, Teeling J. Microglia and macrophages of the central nervous system: the contribution of microglia priming and systemic inflammation to chronic neurodegeneration. Semin Immunopathol. (2013) 35:601–12. doi: 10.1007/s00281-013-0382-8
97. Kim EJ, Kwon KJ, Park JY, Lee SH, Moon CH, Baik EJ. Neuroprotective effects of prostaglandin E2 or cAMP against microglial and neuronal free radical mediated toxicity associated with inflammation. J Neurosci Res. (2002) 70:97–107. doi: 10.1002/jnr.10373
98. Zhang S, Wang XJ, Tian LP, Pan J, Lu GQ, Zhang YJ, et al. CD200-CD200R dysfunction exacerbates microglial activation and dopaminergic neurodegeneration in a rat model of Parkinson's disease. J Neuroinflammation. (2011) 8:154. doi: 10.1186/1742-2094-8-154
99. Mota BC, Kelly ÁM. Exercise alters LPS-induced glial activation in the mouse brain. Neuronal Signal. (2020) 4:NS20200003. doi: 10.1042/NS20200003
100. Perry VH, Nicoll JA, Holmes C. Microglia in neurodegenerative disease. Nat Rev Neurol. (2010) 6:193–201. doi: 10.1038/nrneurol.2010.17
101. Shrikant P, Weber E, Jilling T, Benveniste EN. Intercellular adhesion molecule-1 gene expression by glial cells. Differential mechanisms of inhibition by IL-10 and IL-6. J Immunol. (1995) 155:1489–501.
102. Bobinski F, Teixeira JM, Sluka KA, Santos ARS. Interleukin-4 mediates the analgesia produced by low-intensity exercise in mice with neuropathic pain. Pain. (2018) 159:437–50. doi: 10.1097/j.pain.0000000000001109
103. Calegari L, Nunes RB, Mozzaquattro BB, Rossato DD, Dal Lago P. Exercise training improves the IL-10/TNF-α cytokine balance in the gastrocnemius of rats with heart failure. Braz J Phys Ther. (2018) 22:154–60. doi: 10.1016/j.bjpt.2017.09.004
104. Pedersen BK, Akerström TC, Nielsen AR, Fischer CP. Role of myokines in exercise and metabolism. J Appl Physiol. (2007) 103:1093–8. doi: 10.1152/japplphysiol.00080.2007
105. Szot P, Franklin A, Figlewicz DP, Beuca TP, Bullock K, Hansen K, et al. Multiple lipopolysaccharide (LPS) injections alter interleukin 6 (IL-6), IL-7, IL-10 and IL-6 and IL-7 receptor mRNA in CNS and spleen. Neuroscience. (2017) 355:9–21. doi: 10.1016/j.neuroscience.2017.04.028
106. Zhao J, Bi W, Xiao S, Lan X, Cheng X, Zhang J, et al. Neuroinflammation induced by lipopolysaccharide causes cognitive impairment in mice. Sci Rep. (2019) 9:5790. doi: 10.1038/s41598-019-42286-8
107. Biber K, Neumann H, Inoue K, Boddeke HW. Neuronal 'On' and 'Off' signals control microglia. Trends Neurosci. (2007) 30:596–602. doi: 10.1016/j.tins.2007.08.007
108. Sung YH, Kim SC, Hong HP, Park CY, Shin MS, Kim CJ, et al. Treadmill exercise ameliorates dopaminergic neuronal loss through suppressing microglial activation in Parkinson's disease mice. Life Sci. (2012) 91:1309–16. doi: 10.1016/j.lfs.2012.10.003
109. Jensen CS, Bahl JM, Østergaard LB, Høgh P, Wermuth L, Heslegrave A, et al. Exercise as a potential modulator of inflammation in patients with Alzheimer's disease measured in cerebrospinal fluid and plasma. Exp Gerontol. (2019) 121:91–8. doi: 10.1016/j.exger.2019.04.003
110. Abd El-Kader SM, Al-Jiffri OH. Aerobic exercise modulates cytokine profile and sleep quality in elderly. Afr Health Sci. (2019) 19:2198–207. doi: 10.4314/ahs.v19i2.45
111. De Miguel Z, Khoury N, Betley MJ, Lehallier B, Willoughby D, Olsson N, et al. Exercise plasma boosts memory and dampens brain inflammation via clusterin. Nature. (2021) 600:494–9. doi: 10.1038/s41586-021-04183-x
112. Magee JC, Grienberger C. Synaptic plasticity forms and functions. Annu Rev Neurosci. (2020) 43:95–117. doi: 10.1146/annurev-neuro-090919-022842
113. van Praag H, Christie BR, Sejnowski TJ, Gage FH. Running enhances neurogenesis, learning, and long-term potentiation in mice. Proc Natl Acad Sci U S A. (1999) 96:13427–31. doi: 10.1073/pnas.96.23.13427
114. Ding Y, Li J, Clark J, Diaz FG, Rafols JA. Synaptic plasticity in thalamic nuclei enhanced by motor skill training in rat with transient middle cerebral artery occlusion. Neurol Res. (2003) 25:189–94. doi: 10.1179/016164103101201184
115. Xing Y, Bai Y. A review of exercise-induced neuroplasticity in ischemic stroke: pathology and mechanisms. Mol Neurobiol. (2020) 57:4218–31. doi: 10.1007/s12035-020-02021-1
116. Triviño-Paredes J, Patten AR, Gil-Mohapel J, Christie BR. The effects of hormones and physical exercise on hippocampal structural plasticity. Front Neuroendocrinol. (2016) 41:23–43. doi: 10.1016/j.yfrne.2016.03.001
117. Cotman CW, Berchtold NC. Exercise: a behavioral intervention to enhance brain health and plasticity. Trends Neurosci. (2002) 25:295–301. doi: 10.1016/S0166-2236(02)02143-4
118. Intlekofer KA, Berchtold NC, Malvaez M, Carlos AJ, McQuown SC, Cunningham MJ, et al. Exercise and sodium butyrate transform a subthreshold learning event into long-term memory via a brain-derived neurotrophic factor-dependent mechanism. Neuropsychopharmacology. (2013) 38:2027–34. doi: 10.1038/npp.2013.104
119. Walsh JJ, Tschakovsky ME. Exercise and circulating BDNF: Mechanisms of release and implications for the design of exercise interventions. Appl Physiol Nutr Metab. (2018) 43:1095–104. doi: 10.1139/apnm-2018-0192
120. Ding Q, Vaynman S, Akhavan M, Ying Z, Gomez-Pinilla F. Insulin-like growth factor I interfaces with brain-derived neurotrophic factor-mediated synaptic plasticity to modulate aspects of exercise-induced cognitive function. Neuroscience. (2006) 140:823–33. doi: 10.1016/j.neuroscience.2006.02.084
121. Arnold SJ, Ivleva EI, Gopal TA, Reddy AP, Jeon-Slaughter H, Sacco CB, et al. Hippocampal volume is reduced in schizophrenia and schizoaffective disorder but not in psychotic bipolar I disorder demonstrated by both manual tracing and automated parcellation (FreeSurfer). Schizophr Bull. (2015) 41:233–49. doi: 10.1093/schbul/sbu009
122. Shepherd AM, Laurens KR, Matheson SL, Carr VJ, Green MJ. Systematic meta-review and quality assessment of the structural brain alterations in schizophrenia. Neurosci Biobehav Rev. (2012) 36:1342–56. doi: 10.1016/j.neubiorev.2011.12.015
123. von Bohlen und Halbach O. Involvement of BDNF in age-dependent alterations in the hippocampus. Front Aging Neurosci. (2010) 2:36. doi: 10.3389/fnagi.2010.00036
124. Wilckens KA, Stillman CM, Waiwood AM, Kang C, Leckie RL, Peven JC, et al. Exercise interventions preserve hippocampal volume: a meta-analysis. Hippocampus. (2021) 31:335–47. doi: 10.1002/hipo.23292
125. Li MY, Huang MM, Li SZ, Tao J, Zheng GH, Chen LD. The effects of aerobic exercise on the structure and function of DMN-related brain regions: a systematic review. Int J Neurosci. (2017) 127:634–49. doi: 10.1080/00207454.2016.1212855
126. Codd LN, Blackmore DG, Vukovic J, Bartlett PF. Exercise reverses learning deficits induced by hippocampal injury by promoting neurogenesis. Sci Rep. (2020) 10:19269. doi: 10.1038/s41598-020-76176-1
127. Firth J, Stubbs B, Vancampfort D, Schuch F, Lagopoulos J, Rosenbaum S, et al. Effect of aerobic exercise on hippocampal volume in humans: a systematic review and meta-analysis. Neuroimage. (2018) 166:230–8. doi: 10.1016/j.neuroimage.2017.11.007
128. Erickson KI, Miller DL, Roecklein KA. The aging hippocampus: interactions between exercise, depression, and BDNF. Neuroscientist. (2012) 18:82–97. doi: 10.1177/1073858410397054
129. Wang R, Holsinger RMD. Exercise-induced brain-derived neurotrophic factor expression: therapeutic implications for Alzheimer's dementia. Ageing Res Rev. (2018) 48:109–21. doi: 10.1016/j.arr.2018.10.002
130. Wei Y, Fang CL, Liu SJ, Yang WQ, Wei LS, Lei XJ, et al. Long-term moderate exercise enhances specific proteins that constitute neurotrophin signaling pathway: a TMT-based quantitative proteomic analysis of rat plasma. J Proteomics. (2018) 185:39–50. doi: 10.1016/j.jprot.2018.06.017
131. Zhai ZY, Feng J. Constraint-induced movement therapy enhances angiogenesis and neurogenesis after cerebral ischemia/reperfusion. Neural Regen Res. (2019) 14:1743–54. doi: 10.4103/1673-5374.257528
132. Chen WK, Tsai YL, Shibu MA, Shen CY, Chang-Lee SN, Chen RJ, et al. Exercise training augments Sirt1-signaling and attenuates cardiac inflammation in D-galactose induced-aging rats. Aging. (2018) 10:4166–74. doi: 10.18632/aging.101714
133. Ding Y, Chang C, Xie L, Chen Z, Ai H. Intense exercise can cause excessive apoptosis and synapse plasticity damage in rat hippocampus through Ca2? overload and endoplasmic reticulum stress-induced apoptosis pathway. Chin Med J. (2014) 127:3265–71. doi: 10.1097/00029330-201409200-00014
134. da Rocha AL, Pinto AP, Kohama EB, Pauli JR, de Moura LP, Cintra DE, et al. The proinflammatory effects of chronic excessive exercise. Cytokine. (2019) 119:57–61. doi: 10.1016/j.cyto.2019.02.016
135. Wang Y, Xia Z, Xie H, Dong J. Rational design and evaluation of GLP-1 derivative for treating hyperglycemia combined with overexercise-induced myocardial injury. Life Sci. (2021) 272:119030. doi: 10.1016/j.lfs.2021.119030
136. Cadegiani FA, Kater CE. Hormonal aspects of overtraining syndrome: a systematic review. BMC Sports Sci Med Rehabil. (2017) 9:14. doi: 10.1186/s13102-017-0079-8
137. Buckwalter JA, Glimcher MJ, Cooper RR, Recker R. Bone biology. II: formation, form, modeling, remodeling, and regulation of cell function. Instr Course Lect. (1996) 45:387–99.
138. Diaz-Franco MC, Franco-Diaz de.Leon R, Villafan-Bernal JR. Osteocalcin-GPRC6A: an update of its clinical and biological multi-organic interactions (Review). Mol Med Rep. (2019) 19:15–22. doi: 10.3892/mmr.2018.9627
139. Xiao B. Levering mechanically activated piezo channels for potential pharmacological intervention. Annu Rev Pharmacol Toxicol. (2020) 60:195–218. doi: 10.1146/annurev-pharmtox-010919-023703
140. Li X, Han L, Nookaew I, Mannen E, Silva MJ, Almeida M, et al. Stimulation of Piezo1 by mechanical signals promotes bone anabolism. Elife. (2019). 8:e49631. doi: 10.7554/eLife.49631
141. Wang L, You X, Lotinun S, Zhang L, Wu N, Zou W. Mechanical sensing protein PIEZO1 regulates bone homeostasis via osteoblast-osteoclast crosstalk. Nat Commun. (2020) 11:282. doi: 10.1038/s41467-019-14146-6
142. Sugimoto A, Miyazaki A, Kawarabayashi K, Shono M, Akazawa Y, Hasegawa T, et al. Piezo type mechanosensitive ion channel component 1 functions as a regulator of the cell fate determination of mesenchymal stem cells. Sci Rep. (2017) 7:17696. doi: 10.1038/s41598-017-18089-0
143. Sasaki F, Hayashi M, Mouri Y, Nakamura S, Adachi T, Nakashima T. Mechanotransduction via the piezo1-Akt pathway underlies sost suppression in osteocytes. Biochem Biophys Res Commun. (2020) 521:806–13. doi: 10.1016/j.bbrc.2019.10.174
144. Otto E, Knapstein PR, Jahn D, Appelt J, Frosch KH, Tsitsilonis S, et al. Crosstalk of brain and bone-clinical observations and their molecular bases. Int J Mol Sci. (2020) 21:4946. doi: 10.3390/ijms21144946
145. Komori T. Functions of osteocalcin in bone, pancreas, testis, and muscle. Int J Mol Sci. (2020) 21:7513. doi: 10.3390/ijms21207513
146. Ji W, Sun X. Methyl-CpG-binding protein 2 promotes osteogenic differentiation of bone marrow mesenchymal stem cells through regulating forkhead box F1/Wnt/β-Catenin axis. Bioengineered. (2022) 13:583–92. doi: 10.1080/21655979.2021.2012357
147. Mizokami A, Kawakubo-Yasukochi T, Hirata M. Osteocalcin and its endocrine functions. Biochem Pharmacol. (2017) 132:1–8. doi: 10.1016/j.bcp.2017.02.001
148. Khrimian L, Obri A, Ramos-Brossier M, Rousseaud A, Moriceau S, Nicot AS, et al. Gpr158 mediates osteocalcin's regulation of cognition. J Exp Med. (2017) 214:2859–73. doi: 10.1084/jem.20171320
149. Oury F, Khrimian L, Denny CA, Gardin A, Chamouni A, Goeden N, et al. Maternal and offspring pools of osteocalcin influence brain development and functions. Cell. (2013) 155:228–41. doi: 10.1016/j.cell.2013.08.042
150. Nakazawa K, Quirk MC, Chitwood RA, Watanabe M, Yeckel MF, Sun LD, et al. Requirement for hippocampal CA3 NMDA receptors in associative memory recall. Science. (2002) 297:211–8. doi: 10.1126/science.1071795
151. Borregaard N, Cowland JB. Neutrophil gelatinase-associated lipocalin, a siderophore-binding eukaryotic protein. Biometals. (2006) 19:211–5. doi: 10.1007/s10534-005-3251-7
152. Zhang J, Hou Y, Du XL, Chen D, Sui G, Qi Y, et al. ADORA-driven brain-sympathetic neuro-adipose connections control body weight and adipose lipid metabolism. Mol Psychiatry. (2020). doi: 10.1038/s41380-020-00908-y
153. Majeed Y, Halabi N, Madani AY, Engelke R, Bhagwat AM, Abdesselem H, et al. SIRT1 promotes lipid metabolism and mitochondrial biogenesis in adipocytes and coordinates adipogenesis by targeting key enzymatic pathways. Sci Rep. (2021) 11:8177. doi: 10.1038/s41598-021-87759-x
154. Wang X, Li X, Zuo X, Liang Z, Ding T, Li K, et al. Photobiomodulation inhibits the activation of neurotoxic microglia and astrocytes by inhibiting Lcn2/JAK2-STAT3 crosstalk after spinal cord injury in male rats. J Neuroinflammation. (2021) 18:256. doi: 10.1186/s12974-021-02312-x
155. Huang Z, Zhang Y, Li H, Zhou Y, Zhang Q, Chen R, et al. Vitamin D promotes the cisplatin sensitivity of oral squamous cell carcinoma by inhibiting LCN2-modulated NF-κB pathway activation through RPS3. Cell Death Dis. (2019) 10:936. doi: 10.1038/s41419-019-2177-x
156. Cheng Q, Ng KT, Xu A, Li CX, Liu XB, Guo DY, et al. The roles of lipocalin-2 in small-for-size fatty liver graft injury. Ann Surg. (2014) 260:1062–72. doi: 10.1097/SLA.0000000000000427
157. Zhao N, Xu X, Jiang Y, Gao J, Wang F, Xu X, et al. Lipocalin-2 may produce damaging effect after cerebral ischemia by inducing astrocytes classical activation. J Neuroinflammation. (2019) 16:168. doi: 10.1186/s12974-019-1556-7
158. Ranjbar Taklimie F, Gasterich N, Scheld M, Weiskirchen R, Beyer C, Clarner T, et al. Hypoxia induces astrocyte-derived lipocalin-2 in ischemic stroke. Int J Mol Sci. (2019) 20:1271. doi: 10.3390/ijms20061271
159. Du Y, Li W, Lin L, Lo EH, Xing C. Effects of lipocalin-2 on brain endothelial adhesion and permeability. PLoS ONE. (2019) 14:e0218965. doi: 10.1371/journal.pone.0218965
160. Zhang Y, Liu J, Yao M, Song W, Zheng Y, Xu L, et al. Sailuotong capsule prevents the cerebral ischaemia-induced neuroinflammation and impairment of recognition memory through inhibition of LCN2 expression. Oxid Med Cell Longev. (2019) 2019:8416105. doi: 10.1155/2019/8416105
161. Suk K. Lipocalin-2 as a therapeutic target for brain injury: an astrocentric perspective. Prog Neurobiol. (2016) 144:158–72. doi: 10.1016/j.pneurobio.2016.08.001
162. Franzén A, Heinegård D. Isolation and characterization of two sialoproteins present only in bone calcified matrix. Biochem J. (1985) 232:715–24. doi: 10.1042/bj2320715
163. Clemente N, Raineri D, Cappellano G, Boggio E, Favero F, Soluri MF, et al. Osteopontin bridging innate and adaptive immunity in autoimmune diseases. J Immunol Res. (2016) 2016:7675437. doi: 10.1155/2016/7675437
164. Wirestam L, Saleh M, Svensson C, Compagno M, Zachrisson H, Wetterö J, et al. Plasma osteopontin versus intima media thickness of the common carotid arteries in well-characterised patients with systemic lupus erythematosus. Lupus. (2021) 30:1244–53. doi: 10.1177/09612033211013898
165. Lin YH, Yang-Yen HF. The osteopontin-CD44 survival signal involves activation of the phosphatidylinositol 3-kinase/Akt signaling pathway. J Biol Chem. (2001) 276:46024–30. doi: 10.1074/jbc.M105132200
166. Su J, Basso D, Iyer S, Su K, Wei J, Fox MA. Paracrine role for somatostatin interneurons in the assembly of perisomatic inhibitory synapses. J Neurosci. (2020) 40:7421–35. doi: 10.1523/JNEUROSCI.0613-20.2020
167. Yokosaki Y, Tanaka K, Higashikawa F, Yamashita K, Eboshida A. Distinct structural requirements for binding of the integrins alphavbeta6, alphavbeta3, alphavbeta5, alpha5beta1 and alpha9beta1 to osteopontin. Matrix Biol. (2005) 24:418–27. doi: 10.1016/j.matbio.2005.05.005
168. Shaalan AAM, El-Sherbiny M, El-Abaseri TB, Shoaeir MZ, Abdel-Aziz TM, Mohamed MI, et al. Supplement with calcium or alendronate suppresses osteopenia due to long term rabeprazole treatment in female mice: influence on bone TRAP and osteopontin levels. Front Pharmacol. (2020) 11:583. doi: 10.3389/fphar.2020.00583
169. Depalle B, McGilvery CM, Nobakhti S, Aldegaither N, Shefelbine SJ, Porter AE. Osteopontin regulates type I collagen fibril formation in bone tissue. Acta Biomater. (2021) 120:194–202. doi: 10.1016/j.actbio.2020.04.040
170. Filardi T, Carnevale V, Massoud R, Russo C, Nieddu L, Tavaglione F, et al. High serum osteopontin levels are associated with prevalent fractures and worse lipid profile in post-menopausal women with type 2 diabetes. J Endocrinol Invest. (2019) 42:295–301. doi: 10.1007/s40618-018-0914-0
171. Meller R, Stevens SL, Minami M, Cameron JA, King S, Rosenzweig H, et al. Neuroprotection by osteopontin in stroke. J Cereb Blood Flow Metab. (2005) 25:217–25. doi: 10.1038/sj.jcbfm.9600022
172. Wang J, Huang J, Zhu M, Chen S, Chen C, Miao C, et al. Osteopontin potentiates PM-induced IL-1α and IL-1β production via the ERK/JNK signaling pathway. Ecotoxicol Environ Saf. (2019) 171:467–74. doi: 10.1016/j.ecoenv.2019.01.005
173. Cappellano G, Vecchio D, Magistrelli L, Clemente N, Raineri D, Barbero Mazzucca C, et al. The Yin-Yang of osteopontin in nervous system diseases: damage versus repair. Neural Regen Res. (2021) 16:1131–7. doi: 10.4103/1673-5374.300328
174. Cho HJ, Cho HJ, Kim HS. Osteopontin: a multifunctional protein at the crossroads of inflammation, atherosclerosis, and vascular calcification. Curr Atheroscler Rep. (2009) 11:206–13. doi: 10.1007/s11883-009-0032-8
175. Xin D, Li T, Chu X, Ke H, Liu D, Wang Z. MSCs-extracellular vesicles attenuated neuroinflammation, synapse damage and microglial phagocytosis after hypoxia-ischemia injury by preventing osteopontin expression. Pharmacol Res. (2021) 164:105322. doi: 10.1016/j.phrs.2020.105322
176. Lee W, Nims RJ, Savadipour A, Zhang Q, Leddy HA, Liu F, et al. Inflammatory signaling sensitizes Piezo1 mechanotransduction in articular chondrocytes as a pathogenic feed-forward mechanism in osteoarthritis. Proc Natl Acad Sci U S A. (2021) 118: e2001611118. doi: 10.1073/pnas.2001611118
177. Geoghegan IP, Hoey DA, McNamara LM. Integrins in osteocyte biology and mechanotransduction. Curr Osteoporos Rep. (2019) 17:195–206. doi: 10.1007/s11914-019-00520-2
178. Chen H, Shang D, Wen Y, Liang C. Bone-derived modulators that regulate brain function: emerging therapeutic targets for neurological disorders. Front Cell Dev Biol. (2021) 9:683457. doi: 10.3389/fcell.2021.683457
179. Viña J, Gomez-Cabrera MC, Lloret A, Marquez R, Miñana JB, Pallardó FV, et al. Free radicals in exhaustive physical exercise: mechanism of production, and protection by antioxidants. IUBMB Life. (2000) 50:271–7. doi: 10.1080/15216540051080994
Keywords: aerobic physical exercise, cognition, depression, chronic pain, neuroinflammation, synaptic plasticity, hippocampal atrophy, bone-brain axis
Citation: Jia Y, Yao Y, Zhuo L, Chen X, Yan C, Ji Y, Tao J and Zhu Y (2022) Aerobic Physical Exercise as a Non-medical Intervention for Brain Dysfunction: State of the Art and Beyond. Front. Neurol. 13:862078. doi: 10.3389/fneur.2022.862078
Received: 10 February 2022; Accepted: 28 March 2022;
Published: 13 May 2022.
Edited by:
Suk Yu Sonata Yau, Hong Kong Polytechnic University, Hong Kong SAR, ChinaReviewed by:
Vanessa Castelli, University of L'Aquila, ItalyJoana Gil-Mohapel, University of Victoria, Canada
Liu Hua, Wuhan Sports University, China
Copyright © 2022 Jia, Yao, Zhuo, Chen, Yan, Ji, Tao and Zhu. This is an open-access article distributed under the terms of the Creative Commons Attribution License (CC BY). The use, distribution or reproduction in other forums is permitted, provided the original author(s) and the copyright owner(s) are credited and that the original publication in this journal is cited, in accordance with accepted academic practice. No use, distribution or reproduction is permitted which does not comply with these terms.
*Correspondence: Yonghua Ji, eWhqaSYjeDAwMDQwO3N0YWZmLnNodS5lZHUuY24=; Jie Tao, amlldGFvX3B1dHVvJiN4MDAwNDA7Zm94bWFpbC5jb20=; Yudan Zhu, eXVkYW56aHVfcHV0dW8mI3gwMDA0MDtmb3htYWlsLmNvbQ==