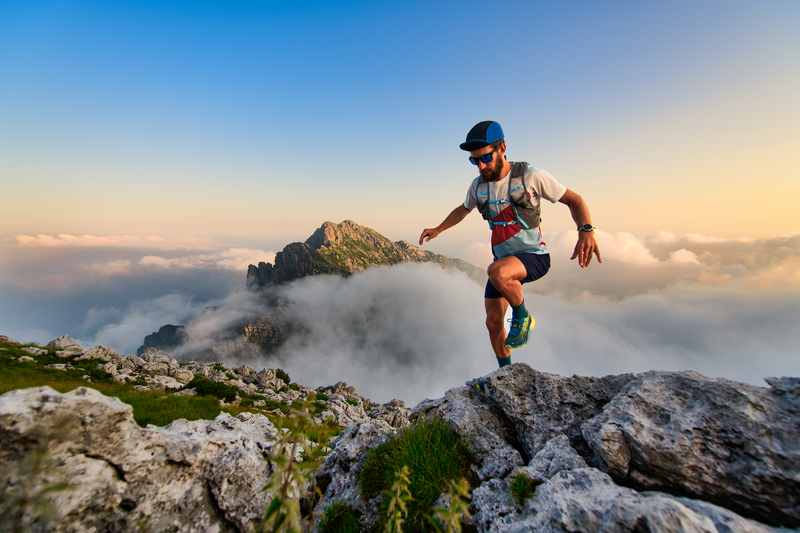
95% of researchers rate our articles as excellent or good
Learn more about the work of our research integrity team to safeguard the quality of each article we publish.
Find out more
REVIEW article
Front. Neurol. , 11 April 2022
Sec. Epilepsy
Volume 13 - 2022 | https://doi.org/10.3389/fneur.2022.836292
This article is part of the Research Topic Epilepsy in Older Adults: From Physiopathology to Improvements in Diagnosis and Management View all 9 articles
The observation that a pathophysiological link might exist between Alzheimer's disease (AD) and epilepsy dates back to the identification of the first cases of the pathology itself and is now strongly supported by an ever-increasing mountain of literature. An overwhelming majority of data suggests not only a higher prevalence of epilepsy in Alzheimer's disease compared to healthy aging, but also that AD patients with a comorbid epileptic syndrome, even subclinical, have a steeper cognitive decline. Moreover, clinical and preclinical investigations have revealed a marked sleep-related increase in the frequency of epileptic activities. This characteristic might provide clues to the pathophysiological pathways underlying this comorbidity. Furthermore, the preferential sleep-related occurrence of epileptic events opens up the possibility that they might hasten cognitive decline by interfering with the delicately orchestrated synchrony of oscillatory activities implicated in sleep-related memory consolidation. Therefore, we scrutinized the literature for mechanisms that might promote sleep-related epileptic activity in AD and, possibly dementia onset in epilepsy, and we also aimed to determine to what degree and through which processes such events might alter the progression of AD. Finally, we discuss the implications for patient care and try to identify a common basis for methodological considerations for future research and clinical practice.
In 1911, five years after presenting the first patient with the pathology that would later be named after him, Alois Alzheimer described a second case, a patient known in medical history as Johann F. Besides the progressive loss of cognitive functions and autonomy, the 56-year-old man experienced several epileptic seizures toward the later stages of the pathology (1, 2). This case, together with the detection of amyloid plaque-like structures called sclerotic plaques of neuroglia during the autopsy of an elderly epileptic patient in 1892, provided the first clues for a link between AD and epilepsy (3) [cited by Cipriani et al. (4)].
During the following century, the potential pathophysiological pathways of AD were meticulously investigated in a race to discover a treatment as the number of cases exploded, reaching ~33–38 million patients worldwide. However, the investigation of the AD-epilepsy axis failed to become what we would call today a “hot topic” in research. Sparse publications appeared every now and then, mainly advocating a link between familial forms of AD (FAD) and epilepsy. Patients with these rare forms, which account for ~1% of all AD cases and are due to mutations on the APP, PSEN1 or PSEN2 genes, were shown to have a high seizure incidence (5–11). As for sporadic AD, for quite a while, this comorbidity was defined as a mere marker of the severe stages of the disease (12, 13). A turn in these trends started with the seminal paper by Amatniek and colleagues in 2006 (14) which described an increase in seizure incidence among sporadic AD patients as of the earliest stages of the disease and which considered it a potential part of the natural history of AD (14). A smaller longitudinal study published the same year by Lozsadi and Larner echoed these results and suggested the existence of potentially shared pathogenetic processes between AD and epilepsy (15). Concomitantly, a series of studies in mouse models of AD (harboring mutations on the genes implicated in FAD) demonstrated the role of the AD-related protein-aggregates, namely Aβ plaques and neurofibrillary tangles [NFTs, (16)], in the observed neuronal hyperexcitability and spontaneous epileptic or epileptiform activity (17–20). (Note that for the remainder of this review, we will use Epileptic Activity/EA to refer to epileptic/epileptiform events). This brought about the introduction of the network dysfunction perspective in AD (21) and an explosion of publications on the link between AD and epilepsy in preclinical and clinical research.
The main objective of this review is to organize the available information fragments from these publications on the potential underlying causes and clinical consequences of the AD-epilepsy comorbidity and its apparent link to the sleep-wake cycle. Our secondary aim is to find ways to identify the pieces of the puzzle of the AD-epilepsy connection that are still missing by adapting current clinical practice and research methods. Therefore, we will first scrutinize the literature on pathophysiological processes to see how significant alterations in AD might trigger epileptic events. We will then attempt to distill coherence from the seemingly discordant clinical results on the characteristics of epileptic activity in AD patients. In the third and fourth parts, we will attempt to understand the possible consequences of these aberrant brain activities that occur during sleep on the progression of cognitive deficits in AD. Finally, we will examine how these pieces of information could be integrated into current clinical practice and research methodology that could increase coherence and comparability across studies.
Research over the past decade has made it abundantly clear that almost all mouse models used in AD research present aberrant network hypersynchrony and hyperexcitability quite early during the disease. These anomalous activities manifest as EA mostly during sleep or periods of inactivity in several models (Figures 1A,B), an aspect that, as we will see, seems to be similar in patients (Figures 1C,D). [For a more exhaustive list, see Kazim et al. (27)]. With the help of these models, significant advances have been made in elucidating the mechanistic pathways related to the aberrant network hyperactivity which have also been extensively reviewed recently (27–34). This review will only focus on the major pathways and the pathophysiological mechanisms related to clinical findings on the subject.
Figure 1. Epileptiform spikes in AD patients and the Tg2576 mouse model. (A) Representative examples of EA in Tg2576 mice (Power 1401 mk-II, CED, Cambridge, UK). Reproduced from (22) with permission (B) Distribution of epileptiform events in Tg2576 mice over the sleep-wake cycle in 6-week-old (n = 10, blue) and 6-month-old (n = 7, red) animals. Reproduced and adjusted from (23) with permission. (C) Longitudinal view of an epileptiform discharge during a vEEG examination, recorded from an AD patient at the University Hospital of Toulouse (Natus, Pleasanton, CA, USA). Calibration bar: 100 μV, 500ms. (D) Distribution of epileptiform discharges in AD patients in the studies reporting IED prevalence with awake and sleep-related data (24–26). For (24), data is pooled from AD patients with and without known epilepsy. Note that REM frequency was not reported for (25) and NREM stages were pooled together to account for differences in reporting methods.
AD is well known for the diffuse presence of senile plaques in the brain. These deposits are composed of Aβ peptide aggregates resulting from the activation of the amyloidogenic proteolytic cleavage process of the APP protein by the β1 (BACE-1) and γ-secretases which leads to a particularly aggregation-prone form, Aβ1−42 [see (35) for an illustration]. Under physiological conditions, this pathway is only secondary to the non-amyloidogenic pathway which depends on cleavage by the α- and γ-secretases and leads to less aggregation-prone forms of Aβ. However, there seems to be slanting toward the BACE-1-dependent pathways in AD, resulting in the well-known extracellular aggregates first described by Alois Alzheimer (36), and translated by Stelzmann and colleagues in 1995 (37). Throughout the literature, the excitatory impact of Aβ as one of the main stimuli of neural hyperexcitability in AD is a surprisingly coherent finding. At non-pathological Aβ levels, Aβ production and neuronal hyperactivity seem to be part of a self-regulating feedback loop (38). However, in AD, this loop becomes a vicious cycle. In fact, increasing the Aβ load by blocking its degradation leads to over-excitation in the hippocampus (39), while elevated or decreased neuronal activity leads to increased or decreased Aβ aggregates, respectively (40–43). These findings are further supported by Busche and colleagues (17, 20). They showed that (I) hyperactive neurons cluster around Amyloid plaque and that (II) in the absence of plaque during the earliest stages of AD, even soluble forms of Aβ can drive neuronal hyperactivity in the hippocampus of APP/PS1 mice. The results obtained by Reyes-Marin and Nuñez (44) point in the same direction revealing a strong correlation between plaque load and epileptic events in the same model. Finally, Aβ clearance seems to decrease neuronal hyperexcitability (45), although some results are contradictory on this subject (46).
Various candidate pathways through which Aβ drives neuronal hyperexcitability have been suggested, including differential impacts of Aβ peptides on neural activity regulation depending on their state of oligomerization [(47), see (48) for a review on further hypotheses]). This could result in a steep Aβ peptide accumulation curve and, later on, amyloid plaques. Accumulating Aβ may then drive neuronal hyperexcitability through the dysregulation of calcium homeostasis due to a high plaque load (49, 50), which would be left unchecked due to the early deterioration of several types of interneurons.
The above-mentioned dysfunctional interneurons are mainly parvalbumin-positive basket cells (PVBC) implicated in the synchronization of the activity of neuronal populations. Moreover, their dysfunction is strongly linked to cognitive impairment [for a summary of PVBC in AD, see Cattaud et al. (51)]. These interneurons seem to be dysfunctional in patients and hAPPJ20 mice due to decreased levels of the Nav1.1 subunit of voltage-gated sodium channels (52, 53). Interestingly, restoring the Nav1.1 subunit rescues both aberrant network activities and the memory-impaired phenotype of the hAAPJ20 model (52). Moreover, in the Tg2576 model (overexpressing a double mutant form of human APP695), Cattaud and colleagues recently demonstrated an early disruption of the perineuronal nets (PNN) surrounding the PVBC (51). PNNs not only protect cells from oxidative stress but they also play an essential role in stabilizing existing synapses, and through that function, memories (54, 55). Interestingly, restoring PVBC in the APP/PS1 model abolishes neuronal hyperexcitability and even improves cognitive function (56), which indicates that the observed PPN/PVBC-related deficits contribute to neuronal hyperexcitability in AD. Other populations of interneurons have also been described as suffering massive damage relatively early in APP/PS1 mice. This seems to be attenuated by the transplantation of embryonic interneuron progenitors that suppress the hyperexcitable phenotype (57). Nonetheless, the interneuron dysfunction observed in AD models seems to add insult to injury as it removes the brakes that could stop the runaway train of Aβ-induced hyperexcitability.
In addition, the second major pathophysiological hallmark of AD after Aβ deposits, the intracellular aggregations called neurofibrillary tangles (NFT), also assist and even contribute to the hypersynchronous phenotype (21). NFT aggregations, which are thought to appear downstream to Aβ aggregation, are made of deposits of the hyperphosphorylated version of the microtubule-associated protein Tau (pTau). This hyperphosphorylated form leads to a loss of protein function and an increased probability of aggregation described by Mokhtar et al. (58). While many aspects of the pathways through which pTau or its endogenous form exerts a neurotoxic effect is still under investigation, the disentangling of their role in the epileptic phenotype associated with AD has started. For example, the reduction of total tau levels relieves neuronal hyperexcitability in mouse models of AD (19, 59). Furthermore, a recent longitudinal study in AD patients found that a risk of seizure was associated with higher total CSF tau levels (60), but not with higher pTau levels alone. In AD mouse models, the effect of tau on epileptogenesis seems to be driven by (I) the tau-dependent depletion of Kv4.2 potassium channels on dendrites (61) and (II) the interaction between endogenous tau, Fyn and PSD95. This latter interaction seems to be involved in the excitotoxic effect of Aβ as it increases the number of post-synaptic glutamate receptors and renders neurons more receptive to excitatory inputs (62). However, phosphorylating tau near to the microtubule domain renders this interaction impossible, leading to NMDA receptor endocytosis, which induces short-term neuroprotective suppression of hyperexcitable networks but comes with a long-term potential to induce network hypoactivity (63). Furthermore, Tau-hyperphosphorylation seems to be accelerated in a hippocampus subfield-specific manner after status epilepticus and during epilepsy (64). At the same time, neural activity and epilepsy-related accelaration of tau pathology has recently been demonstrated (65, 66). These results are coherent with the observations of Mondragón-Rodrígez and colleagues (63), who suggest that tau phosphorylation in the early stages of AD might be a neuroprotective mechanism against Aβ-related hyperexcitability to suppress neuronal hyperexcitability. However, in light of the recent results provided by Busche et al. (67), the respective roles of soluble and aggregated forms of pTau and the different ways through which endogenous Tau can increase or decrease neuronal activity in the presence of high Aβ loads needs further investigation.
Furthermore, the accumulation of all the aggregates and the subsequent aggravation of the epileptic phenotype are linked to one of the earliest clinical signs of AD: declining sleep quality.
Firstly, the progression of AD parallels a more and more fragmented sleep with extended awake periods during the night, increasing sleep latency and shortened total sleep time. The duration of REM sleep decreases, probably due to early atrophy of the brain areas in charge of cholinergic and noradrenergic transmissions that are essential for REM sleep integrity (68, 69). These disturbed sleep patterns may also contribute to the deposition of both Aβ and pTau and aggravate network hyperexcitability. In fact, Aβ load, as measured in CSF samples, fluctuates diurnally and increases during wakefulness (70) and even after a single night of sleep deprivation (71). In response, plaque accumulation was shown to aggravate sleep fragmentation in a drosophila AD model, while enhancing sleep duration led to decreased deposits (72). Interestingly, the same authors described a net excitatory effect of sleep loss and Aβ deposition leading to neuronal hyperexcitability that was responsive to ASM treatment (Levetiracetam, LEV) and that coincidentally prolonged the animals' lifespan as well. The disruptive effect of Aβ on sleep integrity and related memory consolidation has already been described (73), and in light of previous evidence on the subject, a bidirectional link between Aβ deposition and sleep fragmentation was proposed by Ju et al. (74). This link is such that low sleep efficiency turned out to be a good predictor of Aβ deposition rates over several years even for rather long durations (mean follow-up of 3.7 years ± 2.4) in healthy elderly adults (75). Another sleep-related marker, the decrease in Non-REM (NREM) slow-wave activity, was linked to Aβ deposition (73), and a similar or even stronger relationship was observed for tau deposits (76). More importantly, tau also seems to follow a sleep-wake cycle-dependent accumulation similar to Aβ (77).
This daily fluctuation is strongly linked to one of the crucial mechanisms by which the brain can eliminate excess or potentially toxic metabolites, including soluble Aβ and Tau: the glymphatic system. This relatively newly discovered clearance pathway (78, 79) is a highly organized fluid transport mechanism that accommodates an influx of subarachnoid CSF into the brain interstitium via intracerebral arterial perivascular spaces. Due to anterograde flow toward the venous equivalent of perivascular and perineuronal spaces that ends in the meningeal lymphatic drainage system, this mechanism can eliminate unwanted metabolites [Figure 2, see Rasmussen et al. (80)]. As our understanding of this system grows, it is becoming clear that dysfunctions in this pathway can cause rapid deposition of both Aβ and tau (81). Correct functioning of this pathway is highly dependent on water transport through aquaporin-4 (AQP4) water channels at astrocyte endfeet that line both the arterial and venous end of the perivascular spaces. These channels ensure low-resistance flow between perivascular spaces and brain interstitium (78), and several pathways by which these channels become dysfunctional in AD were recently uncovered (82).
Figure 2. Degeneration of the glymphatic clearance system during healthy aging and Alzheimer's disease. White band represents time passing over the course of aging turning into neural pathology, including loss of AQP4 channels and their eventual loss of polarity on astrocyte endfeet. This leads to a loss of K+ homeostasis, which can already induce neuronal hyperexcitability, increasing A-beta production, further aggravating the process. On the other hand, progressive sleep loss also decreases glymphatic function, which amplifies all the other pathological processes mentioned as well.
First, these channels show an age-dependent decline even in wild-type mice (83), leading to a decrease by 40% of intraparenchymally injected Aβ-clearance efficiency. This is consistent with the findings that ~40–80% of all proteins and soluble metabolites are cleared via this mechanism (78). Importantly, it would appear that the glymphatic system is 90% more active during sleep than during waking hours (79). Given the considerable disruption of sleep and decreased total sleep time from the earliest stages of AD, it is plausible that the decrease induced in glymphatic clearance may further aggravate Aβ and tau accumulation (84). Moreover, fragmented sleep might do more harm than just decrease the “ON-time” of glymphatic clearance: Using two-photon in vivo imaging in non-transgenic mice, Liu et al. (85) showed that sleep deprivation leads to a mislocalization of AQP-4 channels that might render glymphatic clearance impaired. It is fascinating to note that in a transgenic model of AD (Tg-ArcSwe), Yang et al. (86) demonstrated an Aβ deposition-dependent mislocalization of AQP-4 channels on perivascular astrocytes even before the discovery of the glymphatic system. Importantly, they also drew attention to an earlier paper by Eid and colleagues (87), who found a dysfunction in perivascular AQP-4 channels in resected hippocampal sections from epileptic patients undergoing surgery. At that time, having no information about the existence of the glymphatic system, they hypothesized that such impairments may not only disturb water homeostasis but could also reduce K+ clearance, a function in which these channels were already known to be implicated. This K+ clearance deficit is yet another pathway that further spirals the hyperexcitable phenotype of AD mouse models (Figure 2). Moreover, a reactive state can also induce loss of polarity in astrocytes, as has been shown to occur near amyloid-β plaque in a transgenic FAD model (APP/PS1) (88). Deleting AQP-4 channels from the same model led to aggravated Aβ deposition both in the cortex and the hippocampus and resulted in an increased spatial memory deficits (89). In another “cross-transgenic” FAD model devoid of AQP-4 channels (5xFAD/AQP4 KO mice), an increase of ~6-fold in EA frequency was observed compared to 5xFAD mice at 10 months of age (90). Many questions remain about the glymphatic system and its relationship with AD (84, 88). Nevertheless, the possibility that yet another vicious cycle that amplifies amyloid deposition and epileptic susceptibility might exist seems plausible. In fact, age-related functional impairment of the glymphatic system may lead to increased plaque deposition. Plaque or Aβ1−42 oligomers could, in turn, create deposits in the vicinity of perivascular astrocytes, leading to a reactive glial state or the mislocalization of AQP-4 channels on astrocytes, further diminishing the process of glymphatic clearance (86, 88). This could lead not only to further acceleration of plaque deposition but also to a loss of K+ homeostasis, increasing the risk of epileptic activities, which, once again, leads to an increase in Aβ (and tau) load (Figure 2). Finally, the respiratory cycle also modulates glymphatic clearance. Inspiration is notably a strong driving force for the process in humans by its modulation on hydrostatic pressure which facilitates CSF flow toward the interstitial spaces of the brain (91). Importantly, breath-holding suppresses this flow and potentially decreases clearance, a scenario that may happen quiet often in patients with sleep apnea, which is a frequent comorbidity in AD patients (92). This is supported by the finding that the severity of obstructive sleep apnea seems to contribute to the build-up of amyloid deposition even in healthy older adults (93, 94).
Finally, it is of note that the glymphatic system, just as many physiological processes including the sleep-wake cycle, has been suggested to be under circadian control (95). Circadian rhythms are maintained via a complex transcriptional machinery that are present in most cells in the human body and are modulated via the suprachiasmatic nucleus of the hypothalamus that is thought to serve as a “master body clock” (96). Interestingly, both clinical and preclinical research have suggested a dysfunction in the circadian rhythms in AD that might happen even before the onset of the clinical symptoms of the pathology. Albeit the mechanistic pathways of the circadian clocks are outside of the scope of this review, this angle needs further investigation as mending this dysfunction that is upstream of several pathways by which AD might progress and by which EA might appear could have therapeutic implications (96).
As mentioned earlier, many other mechanisms have been suggested, such as the APO-ε4 allele, (97–99), and an early reduction in the level of either the mitochondrial protein deacetylase sirtuin 3 (100) or reelin [(101, 102), reviewed by (103)].
All these converging and synergistic pathways introduced in this paragraph could contribute to forming a dysfunctional network in which the excitation/inhibition balance has been considerably slanted toward hyperexcitability, which makes the occurrence of epileptic events or even seizures highly probable. Neuronal hyperactivity during such events might in turn further aggravate the situation by reorganizing functional networks (18, 104, 105) and generating substantial amounts of Aβ. Since the important pathological pathways that lead to AD can be triggered decades before the onset of cognitive symptoms, these processes can slowly but steadily reorganize and destroy existing functional circuits. This also applies to sporadic forms of AD, where epilepsy might not be as clear-cut a symptom as in the case of FAD, yet silent reorganization might occur during the asymptomatic period of plaque and NFT accumulation. This could lead to aberrant network activities, even before cognitive symptoms appear (25).
The suggestion of a disease-accelerating synergistic phenomenon between AD and epilepsy also begs the question of the possible bidirectionality of the link between the two pathologies (48, 106). Clinical research has suggested that age-related senile plaque deposition is more significant among TLE patients than healthy older adults. Moreover, an enhancing effect of the epileptic syndrome on plaque formation and amyloidosis has been suggested. This is based on observations from resected cerebral tissue and CSF AD-biomarker measurements on epileptic patients, as well as observations from genetic and neurotoxic AD mouse models (107–109). A similar increase to that of amyloid burden was found for pTau in post-surgery brain resections collected from TLE patients with focal drug-resistant epilepsy (65). Moreover, a very recent review by Tombini et al. (34) showed that TLE and AD patients share many pathophysiological associations starting with the presence of hallmark depositions of AD (Aβ plaques and NFTs, or their soluble forms), a similarity that is more robust for late-onset TLE cases (110).
However, does the current clinical research confirm (I) the vicious cycle of Aβ, NFTs and EA promoting a fast accumulation of aggregates and a consequent fast progression of AD, and/or (II) the bidirectional relationship between AD and epilepsy?
First of all, it is essential to note that the prevalence of subclinical epileptic activities (Table 1) and epileptic seizures (Table 2) in AD patients is highly variable across studies, reaching 64% (133) in some cases while barely hitting 2% in others (124). This is probably due to the extreme variability in data collection methods (134), an issue we will discuss in detail in the last paragraph. However, several coherent findings emerge from the apparent discordance that can guide our understanding of this peculiar link between AD and epilepsy, and we will first focus on these points.
Table 1. Main inclusion criteria and results of clinical studies exploring the prevalence of epileptic activities in AD patients.
Table 2. Main inclusion criteria and results of clinical studies that explore the prevalence of seizures in AD patients.
The first of these coherent findings is that AD-related EA is primarily subclinical and, therefore, hard to detect. This might explain why this comorbidity has been long overlooked. On one hand, these EA include subclinical epileptic spikes without a behavioral output and which are, as we shall see, difficult to detect by conventional non-invasive methods (135). On the other hand, focal seizures with temporary loss of contact in AD patients might be interpreted as a symptom of AD instead of silent epileptic seizures (26, 112). Therefore, there is a high chance that aberrant network activities go unnoticed and untreated for extended periods.
The second point of cross-study consensus is that the progression of AD in patients with a co-occurring epileptic syndrome or epileptiform activities is faster (25, 117, 130, 136). Moreover, AD patients with EA seem to have worse results on cognitive tests than patients without such aberrant network activities (26, 120, 121, 137). This correlation does not seem to be merely related to a longer duration of AD or more severe stages of the disease. First, Vossel et al. (25) demonstrated that even if patients with and without EA did not differ at baseline in their mini-mental state examination (MMSE) scores, a follow-up over several years showed a steeper cognitive decline for patients with EA. Second, Vöglein and colleagues (130) recently showed that even after adjusting for age and disease duration, the MMSE scores were still unexpectedly low for AD patients with underlying epileptic activity. Moreover, they found that a history of seizures had a significant negative impact on disease severity (as measured by the Clinical Dementia Rating Scale–Sum of Boxes/CDR-SB). Finally, a recent study of Horvath and colleagues described a 1.5 times faster decline of global cognitive performances of AD patients with EA compared to patients without epileptic abnormalities on their EEGs (136).
Another coherent finding is that AD patients with comorbid epileptic activities experience an earlier age of onset of cognitive symptoms (14, 24, 60, 112, 119, 121, 130, 138). This, as we will detail further below, is indicative of a synergistic accelerating of AD- and epilepsy-related factors on cognitive decline which could lead to a faster progression and an earlier age of onset instead of the decade-long process of insidious plaque and NFT deposition.
The localization of EA also seems to be a relatively coherent cross-study finding. Similarly to AD-independent mesial-temporal lobe epilepsies (mTLE), these aberrant network activities in AD are predominant in the temporal lobes and more often detected on the left hemisphere by EEG (24, 25). However, concerning the study of Lam and colleagues (24), it is of note that while left temporal subclinical EA were indeed highly predominant in AD patients with no history of epilepsy, patients with a history of seizures were more prone to have right and left temporal interictal discharges as well.
Last but not least, one of the cornerstones of this review is the strong relationship between the sleep-wake cycle and EA, since sleep-related increase in EA is one of the most consistent findings across both clinical and preclinical literature (24–26) (Figures 1C,D). This sleep-related increase in EA implies that they could plausibly interfere with sleep-related memory consolidation, one of the major potential consequences of AD-related epilepsy which we will examine in the next paragraph.
An increase in EA during sleep in AD patients is not surprising as such a sleep-related increase in the occurrence of epileptic events has already been shown in non-AD epileptic patients (139). However, in epileptic patients, EA seems to fall during periods of NREM sleep and is extremely rare in REM sleep (139). In FAD mice (hAPPJ20 or Tg2576), this is not entirely the case. While EA is still predominant during inactive periods (52) such as sleep, their frequency shows a drastic increase during REM sleep compared to NREMS (23, 140), pinning down a visible discrepancy between animal and human results (140). This pattern is undoubtedly puzzling, as REM sleep is thought to be highly protective against EA due to the desynchronized EEG patterns that make the occurrence of hypersynchrony-related events rather unlikely (139).
It is of note that the predominance of EA during NREM sleep in clinical results might be partially due to difficulties in detecting REM sleep in AD patients, as notable EEG slowing specifically during REM sleep has been observed in AD, which might impede correct scoring of REM sleep (141, 142). However, currently available clinical data (albeit scarce for the moment, Figure 1C) are still more compatible with what is observed in non-AD epileptic patients, with EA predominantly occurring during NREM sleep (Table 2). Consequently, while we look at the potentially detrimental impact of EA on memory consolidation in the next paragraph, we mainly focus on NREM sleep and less on REM sleep.
Throughout the past fifty years, an impressive panoply of theories have been proposed and refined concerning the role of sleep in memory consolidation and this was extensively reviewed (143–145). A theory of a memory consolidation mechanism was first postulated by Marr in the early seventies (146, 147). He suggested that the hippocampus might only serve as a temporal store for memories, while remote memory formation requires the reorganization of such stored information at the cortical level. Reactivation (or replay) of patterns of previous waking activities during “offline” periods was later suggested as a mechanism for such reorganization (148, 149). Sleep provides ideal slots for such offline consolidation as no incoming input is present, which allows the system to shift from “encoding” mode to “consolidation” mode. Such reorganization would also enable the mnesic trace to become gradually independent of the hippocampus, despite the fact that the initial encoding is dependent on both distributed cortical modules and the integrative function of the hippocampus which links the distributed aspects of the trace together. The idea of such a temporal gradient in the hippocampus-dependence of memories has been backed by many studies on anterograde amnesia which describe a temporal gradient for hippocampal-dependence of more or less remote mnesic traces. However, according to the description by Frankland and Bontempi (150), the episodic component of mnesic traces seems to escape such dissociation. This led to the multiple trace theory, according to which contextual and spatial information of a given mnesic trace remain dependent on the hippocampus despite the fact that the semantic component transfers to “cortical only” storage (151). Once again, this is coherent with AD, where episodic memory decline and a loss of hippocampal integrity are amongst the first signs of the disease. These observations converged giving rise to the currently accepted active system consolidation theory (152, 153). This framework is still based on the theory of systemic consolidation allowing remote semantic memories to claim their independence from the hippocampus and stipulates that this takes place with the help of repeated reactivation or replay of time-compressed activation patterns of previous wakeful periods (154).
Replay is thought to occur during NREM sleep and, to a lesser extent, during different wakeful behaviors (155). During these states, the synchronized activity of several oscillators enables the transmission of these traces from the hippocampus to the cortical networks. While this allows for stabilization and transfer of the mnesic traces to cortico-cortical centers during NREM sleep (systemic consolidation), REM sleep might be implicated in more local stabilizing processes (synaptic plasticity) (152, 156, 157). This role of REM sleep is also consistent with previous hypotheses which suggest a “local synaptic organizer” or “integrator” function for this specific sleep stage (158–160).
As for the role of NREM sleep-related consolidation, three hierarchically organized, nested oscillatory patterns seem to be essential (Figure 3) (152, 162): cortical slow oscillations, thalamo-cortical spindles and hippocampal sharp-wave ripples. But what makes these oscillations so crucial for memory consolidation?
Figure 3. Temporal control of sleep-related oscillations and their disruption in case of interneuronal dysfunction. In physiological conditions (A), Parvalbumin-positive basket cells (PVCB) are the main regulator of the firing patterns of pyramidal cells (PYR) in the hippocampus, leading to the generation and control of sharp-wave ripples (SW-R) nested in the trough of sleep spindles, themselves time-locked to the ascending phase of neocortical slow oscillations. This enables the transfer of correct information packets to the “long-term memory stores” from the temporary hippocampal reserve. In case of PVBC dysfunction (B), other interneurons (CCK cells = Cholecystokinin interneurons, A-A cells = Axono-axonic cells) try to compensate for the diminished inhibitory tone. Nonetheless, they are insufficient due to their more distal synapses on PYR cells. This leads to uncontrolled excitation and epileptic events that may induce spindles (161) which leads to the consolidation of nonsense information.
Cortical slow oscillations (SO) are ~0.75 Hz oscillations whose function in memory consolidation is suggested by the observed positive correlation between learning and the SO amplitude during the ensuing rest period (163). Moreover, inducing SOs via transcranial magnetic stimulation increases the consolidation of hippocampus-dependent memories (163, 164). The other role of these large-amplitude waves is the synchronization of neuronal activity by the creation of fluctuation between hyperpolarizing “down-states,” characterized by attenuated neuronal firing, and depolarizing “up-states” during which firing activity reaches levels observed during wakefulness (157, 165). Most notably, SOs also coordinate the occurrence of thalamo-cortical spindles and sharp-wave ripples (166) whose role in memory consolidation seems to be vital. However, in AD, the quantity of SOs decreases in an Aβ1−42 load-dependent manner (73).
The above-mentioned thalamo-cortical spindles are waxing-and-waning oscillations of 0.5–3 s between ~10–16 Hz. Spindles occur on the depolarized up-state of SOs (167), although the literature usually differentiates further between centroparietal fast (13–15 Hz) spindles, indeed phase-locked to the ascending phase of SOs and frontal slow (10–12 Hz) spindles that occur on later (~200 ms) phases of SOs reviewed by (168, 169). The timing of SO-spindle coupling have been reported to be related to hippocampus-dependent memory processes (170), while the density and duration of spindles during post-learning sleep has been continuously shown to be positively correlated with better performances on declarative or episodic memory-related tasks (171–174). However, during aging, their density and duration decrease, their frequency slightly increases (175–177), and their precise phase-locking on SOs is disturbed (178). These changes are even more marked in AD and MCI (179), which seems to be especially true for fast spindles (180), potentially further impacting memory consolidation efficiency (181, 182). Spindles are hypothesized to be essential to consolidation as they provide the network with a time window without interference from external stimuli (183, 184) during which information can travel from the hippocampus to the cortex.
The transfer of information itself relies on sharp-wave ripples (SW-Rs) that are physiological transients of 50–150 ms, with the ripple component in the 140–200 Hz frequency range (185). They arise from the hippocampus, with the sharp-wave component originating from the CA3 and the ripple being generated at the level of the pyramidal cells of the CA1 (185). Recent results also point toward the involvement of the CA2 sub-region as an initiator of the SW-R complex and as a potential origin of SW-Rs arising following learning tasks involving social memory (186, 187). Place-cell recordings in rodents (188) showed that time-compressed replay of past waking behaviors occurs during SW-R bursts that are nested in the troughs of spindles (189–191). SW-Rs are therefore regarded as the ideal “packages” of information transfer between the hippocampus and the neocortex (149, 166, 190, 192). Their role in memory consolidation has been suggested by experiments whereby ripple activity is selectively suppressed during post-training rest periods in rodents (193, 194) which led to poor post-rest recall performances. Moreover, as is the case with spindles, a marked increase in ripple activity was observed following learning in both rodents and humans. As we will see in the next paragraph, they are also altered throughout AD progression.
The finely tuned oscillatory activity between SOs, spindles and SW-Rs can be seen as the meticulously synchronized playing of the many members of an orchestra. However, as with complex symphonies, at such high levels of precision there is always an increased probability for errors that can quickly derail into cacophony. This same risk is highly inherent in consolidation-related oscillations where a minor discrepancy suffices to push the system into a pathological state. In fact, even before the theories of memory consolidation, it was suggested that the mechanisms of remote memory formation and those of epileptic activities share many similarities (195, 196). Based on the well-known Hebbian principle (neurons that “fire together, wire together”), this early suggestion evoked a “hijacking” of learning mechanisms, be it learning or epileptic discharges. However, more recent results incited Halász and colleagues (197) to suggest that epilepsy (even simple EAs) is a derailment of plasticity-related mechanisms rather than an external force that sometimes profit from a pre-existing mechanism to wreak havoc in the brain. This risk is all the more increased for SW-Rs which are the most synchronous physiological oscillations that occur in all mammalian species (155, 185), as ~10–18% of hippocampal neurons, as well as neurons of the surrounding regions fire during SW-Rs (145). SW-R initiation is thought to be a result of the coincidental firing of pyramidal neurons, which generates a massive increase in the activity of the recurrent CA3 network, which leads to the SW-R [see (198) for more detail]. However, instead of unsupervised excitation, this activity is regulated by synchronized perisomatic inhibition by PVBCs which would be essential for maintaining the behavior of physiological SW-Rs [see (196, 199)]. However, when EAs are present, for example due to a dysfunctional transmission from PVBCs to pyramidal cells, there is a marked decrease in SW-Rs (161, 200). Remarkably, during such EAs, all other interneuron types step up their firing rates in response to the fallout of the perisomatic inhibition by PVBCs. Nevertheless, their influence on pyramidal neurons via dendritic synapses is not sufficient to suppress the induction of EAs (Figure 3) (196). What these results suggest altogether is that when PVBCs can respond to the synchronized excitatory activity of pyramidal cells, physiological transients (SW-Rs) are present, but in the absence of sufficient perisomatic inhibition, the result is a pathological SW-R, most often in the form of EA. This under-inhibition might be achieved either by dysfunctional PVBCs or by an abnormally rapid recruiting of pyramidal cells due to neuronal hyperexcitability with which PVBCs cannot keep up. As we have seen, both of these conditions are present in the AD brain and could explain the sleep-related nature of epileptic abnormalities. These results seem to converge harmoniously with the recent results provided by Caccavano et al. (201), who demonstrated in the 5xFAD transgenic mouse model of FAD that PVBCs receive unusually low levels of excitatory input during SW-Rs, which results in a reduction of almost 50% in their firing rate during SW-Rs. This hypoactivity of the PVBCs leaves the pyramidal cells with an enhanced excitation/inhibition ratio that is highly prone to EA. Even in in vitro mouse brain slices that only partially reproduce the network interactions, this gives rise to abnormal SW-Rs with decreased length, increased frequency and wider amplitudes. Interestingly, Jones et al. (202) described a predictor function of early SW-R activity decrease on subsequent spatial memory deficits in a knock-in rodent model (apoE4-KI) of AD. At the same time, two other teams observed decreased SW-R frequency and SO-SWR and SO-spindle coupling in two transgenic models of AD [(203) for 3xTg-AD and (204) for TgF344-AD].
Based on these findings, decreased SW-Rs would lead to less efficient memory consolidation. However, EA-transformed SW-Rs can inflict even more harm than detailed above. Notably, in a rat model of TLE, Gelinas et al. (161) provided further support for the SW-R-to-EA transformation and showed that EAs are associated with memory consolidation deficits. Moreover, they also found that EAs can lead to abnormal induction of spindles, especially during wakefulness but also during REM sleep, even though spindles never occur during this state in physiological conditions. Given that spindles are the safest highway of communication between the hippocampus and the neocortex, information could travel even for the abnormally induced spindles. However, as suggested by Buzsáki (185), these spindles might not carry learning-related traces which would lead to the consolidation of potentially useless or even scribbled cacophony arising from the EAs that elicited them (Figure 3). This conclusion is supported by Bower and colleagues (205), who, in an intracranial EEG (iEEG) experiment on mTLE patients, showed that neuronal assemblies activated during a seizure benefit from the most remarkable learning-associated plastic changes during the post-ictal sleep period. This suggests a potential detouring of sleep-related memory consolidation mechanisms by EA. Picking up on this thread, another team using iEEG recordings also described dysfunctions in memory consolidation stemming from sleep-related hippocampal ictal or interictal epileptic activities in epileptic patients (206). They recently completed this work by suggesting a model that explains the accelerated long-term forgetting often experienced by patients with epileptic activities during sleep (207).
But sleep-related EA may not only inflict harm on the brain by derailed oscillatory patterns but also by disrupting sleep itself which fulfills many other essential functions besides memory consolidation. We have already noted that EAs are predominant during REMS in rodent models of AD. Therefore, EAs, or their induced spindles, which interrupt REMS, could increase network hyperexcitability by disrupting downscaling functions generally attributed to REMS. In fact, although the role of REMS in memory consolidation is still disputed, findings point to a role in local, synaptic plasticity-related processes, including an impact on the expression of immediate early genes related to consolidation (ASH framework), and a general downscaling of synapses leading to the elimination of weak synapses (Synaptic Homeostasis Hypothesis). Downscaling of synapses is essential since the sum of synaptic weights in the brain should maintain a quasi-constant value to avoid hyperexcitability (145). The probability that REMS is implicated in such processes is all the more increased since evidence shows that firing rates decrease brain-wide after the short periods of REMS (159). Therefore, the interruption of theta oscillations during REMS by EAs or induced spindles might lead to an incomplete reduction of synaptic weights and elevated baseline neuronal activity, further aggravating pre-existing activity. This self-aggravating cycle between sleep and epilepsy could add yet another vicious cycle to the path between epilepsy and AD (Figure 4). Finally, as we mentioned earlier (see paragraph 1.4), we should not forget that even without EA, sleep structure in itself is already impaired early in the course of AD (208), which might further aggravate both memory consolidation and the epileptic phenotype.
Figure 4. The bidirectional road between Alzheimer's disease and epilepsy. Note that the mechanistic pathways contain several vicious cycles, meaning that no matter at which level the initial imbalance is on the road, the self-amplifying potential of the system could lead to the onset of one (or possibly both) pathologies.
We have seen the various pathways through which epileptic activity might aggravate the progression of AD (summarized in Figure 4). The concerning number of vicious cycles in this system seems to justify considering an amelioration of epilepsy-detection in AD patients and the treatment options of these aberrant network activities.
As it has been emphasized throughout this review, the prevalence of EA in AD is highly variable across studies, which we suspect is the result of methodological choices. In fact, several points of divergence create a snowball effect of inconsistencies in the results.
One such point is the diagnostic criteria of Alzheimer's disease. While most papers follow NINCDS-ADRDA or IWG-2 criteria, some authors base the diagnosis on MRI markers (26), or do not differentiate between AD and other types of dementia (116, 118, 129, 209, 210). Finally, retrospective or prospective population-based studies or projects using datasets from large memory clinics or databases cannot verify the diagnostic method for all patients in the cohort (122, 123, 127, 128, 131, 132, 211). Using only imagery and neuropsychological data for diagnosis might inflate the percentage of “AD” patients with epilepsy, given that patterns of cortical atrophy and cognitive symptoms can be highly overlapping in epilepsy and AD (212) or aMCI from which the conversion rate to AD is exceptionally high (110). On the other hand, while approximately two-thirds of all dementias are due to AD, mixed cohorts might also impact the observed prevalence of epilepsy and dementia due to AD.
Another important consideration is study type. Many previous publications consisted of retrospective cohort studies, which, besides having an inherent risk of including a relatively high percentage of misdiagnosed dementia patients, are often carried out by a meticulous sweep of patients' medical history [e.g., (123)]. Even when the problem of misdiagnosis is averted by the availability of post-mortem pathology reports confirming AD [as in (126)], using medical reports to infer the presence of epilepsy brings in several biasing factors. First of all, although convulsive seizures might be observed by caregivers, they might not be reported by the patients themselves, who might not recall such events. Secondly, while in some cases EEG results are available in the medical history, they are primarily standard 20–30-min EEGs without periods of sleep (25, 26). In fact, we have discussed at large that subclinical network anomalies or silent seizures in AD occur mainly during sleep (24–26). Accordingly, Horvath et al. (213) have shown that a 1-h EEG recorded between 0:00 and 8:00 (potentially including periods of sleep) is 16.5 times more sensitive in detecting epileptiform events than a recording of the same length during the day (from 8:00 to 16:00, mainly containing wakeful sections). Moreover, recording length strongly correlates with EEG sensitivity. Therefore, there is no comparability between results obtained with 30-min recordings and those obtained after eight or even 24-h recordings.
Another critical point is the question of inclusion and exclusion criteria. While it is now well established that EA can occur at any stage of the disease, even before symptom onset (25, 112), its prevalence still shows strong correlations with disease severity. Therefore, the form of AD or the inclusion threshold for disease severity (measured by MMSE, CDR, ACE or any other commonly used neuropsychological evaluation) could also influence results. This also applies when deciding whether or not to include patients with a known history of epilepsy (or even with ASM treatment). In such cases, separating the results according to pre-existing epilepsy, as done in the recent article by Lam et al. (24), could be a solution to avoid bias. However, other neurological antecedents (e.g., stroke, tumors, previous head trauma, etc.) and pharmaceutical treatments that suggest underlying psychiatric or neurologic comorbidities (such as antipsychotics, antihistamines, benzodiazepines, and of course, ASMs) should be considered in exclusion criteria (24). Moreover, a large proportion of AD patients are prescribed antidepressant medication, even in the early stages, and including these patients presents another issue. While such medication might not have a substantial effect on seizure susceptibility (214), it strongly impacts the sleep cycle, especially REM sleep and sleep fragmentation (215). As mentioned earlier, EA in AD is strongly linked to sleep. Therefore, including patients who are on medications that significantly modify the sleep-wake cycle has a high probability of interfering with EA detection.
Another critical question is whether, technically speaking, EA in AD can be diagnosed. First of all, for obvious medical and ethical reasons, EEG is recorded using scalp electrodes in the absence of drug-resistant epilepsy. However, as it was demonstrated in a study using implanted Foramen Ovale (FO) electrodes in two AD patients, ~95% of the spikes captured by iEEG are not detected on simultaneous scalp EEG recordings (135). Furthermore, this finding was also echoed by a case-study using FO electrodes in a fronto-temporal dementia patient, indicative of the epileptic phenotype being a shared feature of several neurodegenerative diseases (216). Moreover, even with surface electrodes, most of the EA are captured at the level of the temporal lobe (24, 25, 135). However, they are often only detected by temporo-basal electrodes, which are not included in the electrode set used in most setups. Finally, other methods such as MEG have the potential to rank between iEEG and surface electrodes, but MEG has only been applied in two studies on this topic so far with quiet discordant results (25, 113), and given the scarcity of studies having access to MEGs, this incoherence is not likely to be remediated in the near future. These issues already present a bias toward high underestimation of EA in AD. However, the more pressing problems are the detection method and sensitivity. In fact, the majority of authors used manual detection by experts, with some papers relying on one expert (111), or two neurophysiologists (26) or epileptologists (119) or a combination of these specializations (25). Most, but not all experts are blinded to the condition of the participants (AD or control) while analyzing the data, which could introduce further bias. Another problem that probably prevents EA detection, even for automatic detection, is the modified characteristics of brain activity in AD patients. In fact, their EEGs show more significant slowing than is seen during healthy aging (141, 142), which is most prominent during REM sleep (217). Therefore, these EEGs show a significant amount of slowing-related activities, which makes the classification of some pathological or unusual but physiological activities rather tricky.
Finally, other issues regarding the scoring of EEG activity are epileptic transients of sleep or sporadic sleep spikes (SSS) and temporal intermittent rhythmic delta activity (TIRDA) which have already been linked to mTLE, but which have not yet been established as pathological events. However, as Lam et al. (24) pointed out, a surprising increase in SSS is seen in AD patients with underlying EA. Defining when these events should be re-evaluated would be of interest to improve the characterization of EA as opposed to benign EEG patterns. It is all the more important since the boundary where EEG patterns are considered as subclinical or interictal activities, is already blurred. The most common definition that authors give for spikes is “sharp waveforms 20 to 200 ms, clearly distinct from ongoing background activity, with an associated subsequent slow-wave” (25, 26), but this is not unified across studies. In fact, the prevalence dropped from 42 to 48% respectively in the Vossel and Horváth studies to as little as ~6% in the study by Brunetti et al. (113), who defined EA based on stricter morphologic characteristics. However, contrary to preclinical studies (218, 219), there are no recommendations or guidelines for EA detection in AD patients, considering the many abnormalities and specificities already present on EEGs. Such recommendations would greatly facilitate the diagnosis of epilepsy or subclinical EA in AD patients. In their article, Sen and colleagues (220) have already made a step toward formulating such guidelines for neuropsychological data collection. They suggest standardized, culture-independent, short batteries of tests, with baseline evaluation and longitudinal tracking. Based on snippets from existing studies and an ongoing clinical study (ClinicalTrials identification: NCT03923569), we suggest some methodology-unifying considerations concerning the measurement and detection of epileptic events in AD in Textbox 1 in the hope of amplifying data on mechanisms that have already been suggested but which have still not been replicated.
As is the case of all AD-related issues, AD-related neuronal hyperactivity should be treated as soon as possible, especially since AD patients with EA seem to benefit from such treatment, as we will see below. However, the detection of subclinical epileptic activity is subject to high methodological variations, not only in research but also in clinical practice. This variability in methodology might be one of the reasons why this issue is not yet profoundly nested in current clinical practice. As we have emphasized throughout this review, EA is mainly subclinical in AD (221) and often goes unnoticed by the patient and the caregiver(s). Hence, even if periods of loss of contact or stereotypical behaviors/automatisms are present during ictal or interictal activities, they might be considered part of the disease progression rather than an epilepsy-related event. To address this issue, questionnaires or interviews targeting these symptoms could be applied preferentially in the presence of caregivers [an example of such a questionnaire, elaborated from previous observations (26, 221–223) is provided in Supplementary Material 1].
In any event, unless overt signs of epilepsy are present, EEG, especially of longer duration including periods of sleep, is rarely requested for AD patients. An exception applies to patients with suspected sleep apnea who undergo a polysomnographic examination coupled with EEG. In fact, until portable, easily applicable, useable-at-home technologies that measure brain activity during the night become widely available, more systematic screening for epileptiform activities in AD is unlikely to take place. Although FO electrodes are especially well-positioned to capture EA from anterior temporal and mesial-temporal structures, they are not feasible for the entire AD population due to the invasive nature of the procedure. Video-EEG also seems to be a relatively reliable method. However, most healthcare systems across the globe are probably unprepared to screen the entire AD population. The study by Vossel (25) indicated that MEG might be a highly sensitive non-invasive detector of EA, especially if it can be backed by computational approaches that enable the detection of signatures of EA even in the absence of large amplitude deflections (33, 224). However, MEG also requires expensive, specific and, more often than not, inaccessible equipment for EA screening.
On the plus side, although methodological differences led to highly variable incidence rates across previous studies (see Table 1), the different inclusion criteria helped to narrow down the patient group that is at higher risk for AD and EA comorbidity and for whom a vEEG screening would be the most important. For sporadic cases, this includes patients with an earlier age of onset (<65 years), APOE4 allele(s), higher educational levels or a very apparent discrepancy between cognitive symptoms and the degree of brain atrophy during the early stages (indicative of a high cognitive reserve). In FAD, given the extremely high prevalence of epilepsy (11) and the relatively low number of patients affected by this condition (1% of all AD cases), systematic screening by v-EEG at cognitive decline onset should be conducted. Detecting and treating EA before seizures with high levels of network reorganization potential might help to slow down cognitive decline.
Another crucial diagnostic consideration is that the link between AD and epilepsy is increasingly considered to be a bidirectional road (106, 225). It has been shown that while AD patients have an increased risk of developing epilepsy, epilepsy patients also present a 2-3.6-fold risk of developing dementia compared to the non-epileptic population (106, 129). Unfortunately, in current clinical practice, just as AD-related silent EA often goes unnoticed, there is also a risk that instead of being diagnosed as AD, the cognitive complaints of epilepsy patients might be mislabeled as Epileptic Amnestic Syndrome, a less severe condition with good responsiveness to ASMs (221, 226). This could lead to suboptimal treatment plans for dementia. But even if a diagnosis of AD is not justified, an altered path of cognitive decline in epileptic patients compared to healthy older adults has been observed throughout the literature, as was summarized by Breuer and colleagues (2016) (227) in favor of accelerated cognitive aging for people living with epilepsy. According to their review, while a younger age of epilepsy onset seems to be a risk factor for such an increase in cognitive decline, it was emphasized that LOEU patients are no exception. As they underscored, the brain of older adults tackles several problems at a time, such as elevated levels of inflammatory brain response, comorbidities that affect cognition (such as AD), the use of several medications with often interacting effects, and, notably, a potentially decreased cognitive reserve. This decrease means that they cannot compensate for epilepsy-induced cognitive problems like younger epileptic patients, which leads to an even more accelerated cognitive decline (227). This is consistent with the finding that AD patients with epilepsy generally have higher educational levels (25, 26). In fact, patients with high cognitive reserves can compensate even for comorbid AD and (subclinical) epilepsy for longer periods, and therefore present only mild symptoms even in more advanced stages of AD in terms of biomarkers. However, once the macro- and microstructural damages reach a level where compensation is no longer possible, the disease seems to progress at an excessive speed, as is the case for AD patients with EA (Figure 5) (25, 130, 137). However, this conclusion might beg the question of what happens to AD patients with EA with more modest cognitive reserve. It might be that the accelerating effect of the comorbid pathologies without the shield of cognitive reserve is such that polysomnographic examination might not be possible in some cases even shortly after diagnosis due to agitation.
Figure 5. Proposed model of biomarker-and cognitive decline progression over the course of AD. High cognitive reserves compensate on a functional level for marked Amyloid and Tau aggregation and atrophy may lead to delayed diagnosis. This might explain why EA is most often detected in highly educated participants who are (seemingly) in the mild stages of the disease. However, once compensation is no longer possible and EA becomes more frequent or probable, the cognitive decline might be steeper, which is compatible with the fast progression noted in AD patients with E/EA. Figure adapted from (33) with permission. The gray dashed line represents the approximate time of diagnosis.
To conclude on the bidirectionality of the link between AD and epilepsy, we would like to address a notion from recent work by Sen and colleagues (220). They noted that it could not be ascertained whether epilepsy simply facilitates dementia by lowering brain reserves or whether it produces it. According to our interpretation, both suggestions are valid: (sub)clinical epilepsy, by continuous insults to neuronal circuits and through all the harmful effects we reviewed, leads to an increase in “AD-inducing” substances (especially if clearance mechanisms are impaired) and, through the reorganization of neuronal networks, reduces brain reserve that compensates for dementia-related cognitive loss. In any event, screening of epilepsy patients for AD biomarkers, especially those at higher risk for dementia, should be systematic to enable earlier detection which might ensure a more efficient, partially preventive (228), partially medical treatment. This applies to patients at risk for AD, including those with LOEU, patients with cognitive complaints, or a family history of dementia. The importance of this screening came to light through a recent publication (106) which found abnormally decreased CSF Aβ1-42 levels in LOEU patients, with a hazard ratio of 3.4 for progressing to AD. That is why the medical recommendations that summarize the most important notions for clinical practice from this paragraph (Textbox 2) include both pathologies. This seems all the more justified since epileptic auras have also been described as preceding MCI onset by 4–7 years, leading to the suggestion of an “epileptic variant” of AD (222). Of note, cognitive functions (measured by MMSE scores) remained relatively stable at the one-year follow-up for almost all participants, suggesting a potentially positive impact of ASMs in preventing a disease course that is noted to be generally worse for the AD-epilepsy comorbidity (222).
Despite the widespread harmful effects of EA, it is quite probable that its attenuation in AD patients is not the star that sashays on the red carpet and dazzles the world with an all-in-one cure to AD. However, silencing network hyperactivity might lead to slower disease progression, a decrease in disease burden and should be considered as part of the standard medical treatment plan for AD patients. In 2007, it was estimated that delaying AD onset by as little as a year could lead to 9.2 million fewer cases in 2050 (229). A one-year delay could have a substantial impact on people living with AD and could also have major socioeconomic impacts, given that the global cost of dementia was estimated at US $818 billion per year in 2015 (230), most of which can be attributed to AD.
We have just explored whether neuronal hyperactivity might accelerate disease onset and progression. If this is the case, treatment in AD patients at the earliest possible moment might delay disease progression. A rather impressive quantity of literature is now available on treatment options for AD-related epilepsy and was recently reviewed at length by two separate research groups (33, 231). These results accentuate the positive effects of Levetiracetam (LEV) both in rodent models and in AD patients. In fact, LEV led to improved memory performance in aged rats in the study by Koh et al. (232). Interestingly, the treatment only benefited older but not younger animals, suggesting that this ASM treats excess neuronal activity without inducing potentially detrimental decreases in baseline activity. This preclinical result was also replicated in a recent clinical study with AD patients in mild-moderate stages of the disease (MMSE > 18) in which a marked improvement in executive functions (measured by the Stroop test) and in navigation capacities (as shown by results on a virtual route learning test) were noted after only 4 weeks of treatment with low doses of LEV (125 mg/day) (114). Importantly, beneficial effects in the above-mentioned tests were only observed for patients with EA, further suggesting that LEV targets only excess neuronal activity and that this excess activity impairs cognitive functions. Among the other clinical studies on ASM treatment in AD-related EA, that of Cumbo and Ligori (233) should be noted, where the impact of long-term use of several ASMs (LEV, phenobarbital (PB) and lamotrigine (LTG) in AD patients with concomitant epilepsy was examined (n = 38, 28 and 29 respectively in the three treatment arms). They found similar responder rates between the three ASMs (71% responded to LEV, 59% to LTG and 64% to PB) which were also comparable to the rate noted in epilepsy unrelated to AD (~66%). However, while LEV had relatively few side effects and slightly improved cognitive functions, PB negatively impacted cognitive functions. On the other hand, LTG was associated with a slight decline in cognitive functions but with an improvement in mood. Given the encouraging results with LEV, it is not surprising that most clinical trials tested this medication rather than other ASMs. For example, another study of chronic treatment with LEV in cases of severe AD with late-onset seizure found that 72% of the patients were seizure-free after the one-year follow-up, only 8% were non-responsive, while 16% had undesirable side effects (234). In addition, in a study with a small sample size of 7 AD patients, Musaeus et al. (235) described normalized EEG activity after a single dose of 7.5 mg/kg (~500 mg) of LEV but not after 2.5 mg/kg. This normalization was manifested by decreased coherence in slower and increased coherence in faster bands. In two other studies with aMCI patients, Bakker et al. (236, 237) showed that a 2-week treatment with lower doses of LEV (most notably 125 mg) lead to decreased hippocampal hyperactivity (as measured by fMRI signals in the CA3 and the entorhinal cortex). Although this relatively short-term treatment did not seem to affect long-term memory performances, it did improve pattern separation, which is an important component of episodic memory. Furthermore, it appears that ASMs (including LEV) do elicit such a positive effect on cognition even with chronic treatment in LOEU patients without AD (238), especially in patients diagnosed with focal seizures. Although the amount of randomized case-control studies remains too low for further conclusions (231), preclinical results of antiepileptic treatments in AD are promising [reviewed by (27), and (239)]. They suggest decreases in excess neuronal activity (61, 240), improved cognitive functions (240–242), reduced amyloid plaque load in some cases (242), or a rescue from the depletion of neuronal stem cells due to network hyperactivity [(241), see (27) for a review]. LEV also seems to prevent tau-dependent depletion of the potassium channels of the Kv4.2 type which were mentioned earlier as being implicated in neuronal hyperexcitability and further aggravate the above-mentioned vicious cycles (61). Finally, LEV restores impaired LTP function in the perforant path toward dentate granule cell synapses and normalizes levels of NPY, Fos and calbindin proteins, all of which are related to excess neuronal activity (240). Moreover, many potential pathways for treatment are under investigation, and have been reviewed recently (33).
It is important to note that the data that is currently available mainly describes the effect of LEV although other, less explored molecules should also be tested, especially given the circadian variations of EA activity in AD patients. In fact, since EA are predominant during sleep, a posology including a bedtime dose of ASM might be the most effective way to tackle these anomalies. Moreover, limiting the strongest effect of the ASMs to sleep intervals could also prevent most of their side effects during wakefulness. On the other hand, the half-life of LEV is rather short, and even residual impacts are improbable after an entire night. Consequently, taking it before sleep could lead to an overspill of EA during wakefulness. This might be a rationale for testing other available treatments with a longer half-life. However, until more data are available on the subject, LEV seems to be the most effective treatment of EA for AD patients of all the rarely tested ASMs in this field.
Finally, sleep-related EA might only be one of the ways through which sleep (and related consolidation processes) is altered in AD. We have seen that fragmented sleep could affect amyloid and Tau clearance from the brain by impeding glymphatic functions, and it has been shown that altered sleep quality is linked to Tau and Amyloid deposition alike (76, 243). Attempts at remediating fragmented sleep (due to apnea, confusional arousals, restless leg syndrome, REM sleep behavior disorder or other causes) should be made as early as complaints concerning sleep quality are reported by the patient or by their caregiver(s), especially since the literature on the close relationship between sleep quality and cognitive decline is growing daily. A morning dose of donepezil has been suggested to counteract REM sleep decline and REM-sleep related EEG slowing. However, other pharmacological treatments are still under investigation for AD (208). Non-pharmaceutical treatments such as bright-light therapy show encouraging results and could be considered, along with psycho-educational measures and behavioral changes aimed at improving sleep (daily physical activity, less time spent in bed during the day, more time spent outside in natural light) (208).
In this paper, we reviewed how the often behaviorally masked epileptic phenotypes in AD (or possibly, vice versa) might be unmasked and aggravated during sleep. Sleep is a crossroads where AD-related proteins can propagate unsupervised and when epileptic activities are more likely to happen through the occurrence of highly synchronized consolidation-related neuronal activities in under-inhibited networks. We also aimed to draw attention to the risk that such activities might cause cognitive decline, in particular, difficulties with memory consolidation and further insult to the already fragile hippocampal formation by chronic reorganization of neuronal circuits. We emphasized the importance, or rather, the absolute necessity of early screening during medical care, for both epileptic events in AD and AD biomarkers in epilepsy, to enable early medical intervention and to prevent the escalation of neuronal and generalized brain damage. Given that such screening is impossible in the entire AD and epileptic population, we filtered homogenous results from previous findings to characterize patients at risk. Finally, we briefly summarized the available knowledge on the relationship between AD and epilepsy, which has grown incredibly since the first observations of Blocq and Marinesco (3) and is still growing daily. However, it will be challenging to arrive at clinically relevant conclusions from coherent results without unified methodologies across research teams. As others have noted before us, such coherence will only be possible by comparing cross-study samples, or through joint data acquisition in large-scale collaborations.
ABS contributed to the conceptualization of the review, collected references, and drafted the manuscript. BC, LD, EB, JC, JP, and LV contributed to the conceptualization of the review and provided feedback. JC and BC organized references. FG and JC devised the supplementary questionnaire and provided feedback. LV and LD supervised the project. All authors contributed to the article and approved the submitted version.
This research was supported by the French organizations Fondation Alzheimer (Project EREMAD), France Alzheimer and the Agence Régionale de Santé (ARS).
The authors declare that the research was conducted in the absence of any commercial or financial relationships that could be construed as a potential conflict of interest.
All claims expressed in this article are solely those of the authors and do not necessarily represent those of their affiliated organizations, or those of the publisher, the editors and the reviewers. Any product that may be evaluated in this article, or claim that may be made by its manufacturer, is not guaranteed or endorsed by the publisher.
We thank Laure Verret, Ph.D., for her helpful comments on the manuscript.
The Supplementary Material for this article can be found online at: https://www.frontiersin.org/articles/10.3389/fneur.2022.836292/full#supplementary-material
Aβ, Amyloid-β peptide; ACE, Addenbrooke's Cognitive Examination; AD, Alzheimer's disease; APP, Amyloid Precursor Protein; AQP-4, Aquaporin-4; ASM, Antiseizure medication; (a)MCI, (amnestic) Mild Cognitive Impairment; BZD, Benzodiazepines; CDR-SB, Clinical Dementia Rating Scale–Sum of Boxes; CSF, Cerebrospinal Fluid; EOAD, Early-onset Alzheimer's disease; EA, Epileptic/Epileptiform activity; FAD, Familial Alzheimer's disease; IED, Interictal epileptic discharge; iEEG, intracranial EEG; LTG, Lamotrigine; LEV, Levetiracetam; LOAD, Late-onset Alzheimer's disease; LOEU, Late-onset epilepsy of unknown origin; MMSE, Mini-mental State Examination (Folstein Version); (m)TLE, (Mesial) Temporal Lobe Epilepsy; NFT, Neurofibrillary tangle; PH, Phenytoin; (N)REMS, (Non)-Rapid-eye movement sleep; PSEN, Presenilin; PSG, Polysomnography; PVBC, Parvalbumin positive basket cells; SSS, sporadic sleep spikes; SWD, Sharp-wave discharge; SW-R, Sharp-wave ripple; SO, Slow oscillation; v-EEG, video-electroencephalography.
1. Alzheimer A. über eigenartige Krankheitsfälle des späteren Alters. Z Für Gesamte Neurol Psychiatr. (1911) 4:356. doi: 10.1007/BF02866241
2. Möller HJ, Graeber MB. The case described by Alois Alzheimer in 1911. Eur Arch Psychiatry Clin Neurosci. (1998) 248:111–22. doi: 10.1007/s004060050027
3. Blocq P, Marinesco G. Sur les lésions et la pathogénie de l'épilepsie dite essentielle. Seimane Médical. (1892) 12:445–6.
4. Cipriani G, Dolciotti C, Picchi L, Bonuccelli U. Alzheimer and his disease: a brief history. Neurol Sci. (2011) 32:275–9. doi: 10.1007/s10072-010-0454-7
5. Cabrejo L, Guyant-Maréchal L, Laquerrière A, Vercelletto M, De La Fournière F, Thomas-Antérion C, et al. Phenotype associated with APP duplication in five families. Brain. (2006) 129:2966–76. doi: 10.1093/brain/awl237
6. Furuya H, Yasuda M, Terasawa K, Tanaka K, Murai H, Kira J, et al. A novel mutation (L250V) in the presenilin 1 gene in a Japanese familial Alzheimer's disease with myoclonus and generalized convulsion. J Neurol Sci. (2003) 209:75–7. doi: 10.1016/S0022-510X(02)00466-5
7. Larner AJ. Presenilin-1 mutation Alzheimer's disease: a genetic epilepsy syndrome? Epilepsy Behav. (2011) 21:20–2. doi: 10.1016/j.yebeh.2011.03.022
8. Shrimpton AE, Schelper RL, Linke RP, Hardy J, Crook R, Dickson DW, et al. A presenilin 1 mutation (L420R) in a family with early onset Alzheimer disease, seizures and cotton wool plaques, but not spastic paraparesis. Neuropathology. (2007) 27:228–32. doi: 10.1111/j.1440-1789.2007.00766.x
9. Snider BJ, Norton J, Coats MA, Chakraverty S, Hou CE, Jervis R, et al. Novel Presenilin 1 Mutation (S170F) Causing Alzheimer Disease With Lewy Bodies in the Third Decade of Life. Arch Neurol. (2005) 62:1821–30. doi: 10.1001/archneur.62.12.1821
10. Velez-Pardo C, Arellano JI, Cardona-Gomez P, Del Rio MJ, Lopera F, et al. CA1 Hippocampal Neuronal Loss in Familial Alzheimer's Disease Presenilin-1 E280A Mutation Is Related to Epilepsy. Epilepsia. (2004) 45:751–6. doi: 10.1111/j.0013-9580.2004.55403.x
11. Zarea A, Charbonnier C, Rovelet-Lecrux A, Nicolas G, Rousseau S, Borden A, et al. Seizures in dominantly inherited Alzheimer disease. Neurology. (2016) 87:912–9. doi: 10.1212/WNL.0000000000003048
12. Romanelli MF, Morris JC, Ashkin K, Coben LA. Advanced Alzheimer's Disease Is a Risk Factor for Late-Onset Seizures. Arch Neurol. (1990) 47:847–50. doi: 10.1001/archneur.1990.00530080029006
13. Mendez MF, Catanzaro P, Doss RC, Arguello R, Frey WH. Seizures in Alzheimer's Disease: Clinicopathologic Study. J Geriatr Psychiatry Neurol. (1994) 7:230–3. doi: 10.1177/089198879400700407
14. Amatniek JC, Hauser WA, DelCastillo-Castaneda C, Jacobs DM, Marder K, Bell K, et al. Incidence and Predictors of Seizures in Patients with Alzheimer's Disease. Epilepsia. (2006) 47:867–72. doi: 10.1111/j.1528-1167.2006.00554.x
15. Lozsadi DA, Larner AJ. Prevalence and Causes of Seizures at the Time of Diagnosis of Probable Alzheimer's Disease. Dement Geriatr Cogn Disord. (2006) 22:121–4. doi: 10.1159/000093664
16. Selkoe DJ. The molecular pathology of Alzheimer's disease. Neuron. (1991) 6:487–98. doi: 10.1016/0896-6273(91)90052-2
17. Busche MA, Eichhoff G, Adelsberger H, Abramowski D, Wiederhold KH, Haass C, et al. Clusters of Hyperactive Neurons Near Amyloid Plaques in a Mouse Model of Alzheimer's Disease. Science. (2008) 321:1686–9. doi: 10.1126/science.1162844
18. Palop JJ, Chin J, Roberson ED, Wang J, Thwin MT, Bien-Ly N, et al. Aberrant Excitatory Neuronal Activity and Compensatory Remodeling of Inhibitory Hippocampal Circuits in Mouse Models of Alzheimer's Disease. Neuron. (2007) 55:697–711. doi: 10.1016/j.neuron.2007.07.025
19. Roberson ED, Scearce-Levie K, Palop JJ, Yan F, Cheng IH, Wu T., et al. Reducing Endogenous Tau Ameliorates Amyloid b–Induced Deficits in an Alzheimer's Disease Mouse Model. Science. (2007) 316:6. doi: 10.1126/science.1141736
20. Busche MA, Chen X, Henning HA, Reichwald J, Staufenbiel M, Sakmann B, et al. Critical role of soluble amyloid-β for early hippocampal hyperactivity in a mouse model of Alzheimer's disease. Proc Natl Acad Sci. (2012) 109:8740–5. doi: 10.1073/pnas.1206171109
21. Palop JJ, Chin J, Mucke L. A network dysfunction perspective on neurodegenerative diseases. Nature. (2006) 443:768–73. doi: 10.1038/nature05289
22. Bezzina C. Early Onset of Hypersynchronous Network Activity and Expression of a Marker of Chronic Seizures in the Tg2576 Mouse Model of Alzheimer's Disease. PLoS ONE. (2015) 10:e0119910. doi: 10.1371/journal.pone.0119910
23. Kam K, Duffy ÁM, Moretto J, LaFrancois JJ, Scharfman HE. Interictal spikes during sleep are an early defect in the Tg2576 mouse model of β-amyloid neuropathology. Sci Rep. (2016) 6:20119. doi: 10.1038/srep20119
24. Lam AD, Sarkis RA, Pellerin KR, Jing J, Dworetzky BA, Hoch DB, et al. Association of epileptiform abnormalities and seizures in Alzheimer disease. Neurology. (2020) 95:e2259–70. doi: 10.1212/WNL.0000000000010612
25. Vossel KA, Ranasinghe KG, Beagle AJ, Mizuiri D, Honma SM, Dowling AF, et al. Incidence and impact of subclinical epileptiform activity in Alzheimer's disease. Ann Neurol. (2016) 80:858–70. doi: 10.1002/ana.24794
26. Horváth A, Szucs A, Hidasi Z, Csukly G, Barcs G, Kamondi A., et al. Prevalence, semiology, and risk factors of epilepsy in Alzheimer's disease: an ambulatory EEG study. J Alzheimers Dis. (2018) 63:1045–54. doi: 10.3233/JAD-170925
27. Kazim SF, Seo JH, Bianchi R, Larson CS, Sharma A, Wong RK, et al. Neuronal network excitability in Alzheimer's disease: the puzzle of similar versus divergent roles of amyloid β and tau. eNeuro. (2021) 8.
28. Ambrad Giovannetti E, Fuhrmann M. Unsupervised excitation: GABAergic dysfunctions in Alzheimer's disease. Brain Res. (2019) 1707:216–26. doi: 10.1016/j.brainres.2018.11.042
29. Rey C, Cattaud V, Rampon C, Verret L. What's New on Alzheimer's Disease? In: Insights From AD Mouse Models. Biomedical Gerontoscience. (2019). doi: 10.1016/b978-0-12-801238-3.11400-x.hal-03058577
30. Vico Varela E, Etter G, Williams S. Excitatory-inhibitory imbalance in Alzheimer's disease and therapeutic significance. Neurobiol Dis. (2019) 127:605–15. doi: 10.1016/j.nbd.2019.04.010
31. Busche MA, Hyman BT. Synergy between amyloid-β and tau in Alzheimer's disease. Nat Neurosci. (2020) 23:1183–93. doi: 10.1038/s41593-020-0687-6
32. Giorgi FS, Saccaro LF, Busceti CL, Biagioni F, Fornai F. Epilepsy and Alzheimer's Disease: Potential mechanisms for an association. Brain Res Bull. (2020) 160:107–20. doi: 10.1016/j.brainresbull.2020.04.009
33. Toniolo S, Sen A, Husain M. Modulation of Brain Hyperexcitability: Potential New Therapeutic Approaches in Alzheimer's Disease. Int J Mol Sci. (2020) 21:9318. doi: 10.3390/ijms21239318
34. Tombini M, Assenza G, Ricci L, Lanzone J, Boscarino M, Vico C, et al. Temporal Lobe Epilepsy and Alzheimer's Disease: From Preclinical to Clinical Evidence of a Strong Association. J Alzheimers Dis Rep. (2021) 5:243–61. doi: 10.3233/ADR-200286
35. Querfurth HW, LaFerla FM. Alzheimer's disease. N Engl J Med. (2010) 362:329–44. doi: 10.1056/NEJMra0909142
36. Alzheimer A. Uber einen eigenartigen schweren Er Krankungsprozeb der Hirnrinde. Neurol Cent. (1906) 2:1129–36.
37. Stelzmann RA, Norman Schnitzlein H, Reed Murtagh F. An english translation of alzheimer's 1907 paper, Über eine eigenartige erkankung der hirnrinde? Clin Anat. (1995) 8:429–31. doi: 10.1002/ca.980080612
38. Kamenetz F, Tomita T, Hsieh H, Seabrook G, Borchelt D, Iwatsubo T, et al. APP Processing and Synaptic Function. Neuron. (2003) 37:925–37. doi: 10.1016/S0896-6273(03)00124-7
39. Abramov E, Dolev I, Fogel H, Ciccotosto GD, Ruff E, Slutsky I. Amyloid-β as a positive endogenous regulator of release probability at hippocampal synapses. Nat Neurosci. (2009) 12:1567–76. doi: 10.1038/nn.2433
40. Bero AW, Yan P, Roh JH, Cirrito JR, Stewart FR, Raichle ME, et al. Neuronal activity regulates the regional vulnerability to amyloid-β deposition. Nat Neurosci. (2011) 14:750–6. doi: 10.1038/nn.2801
41. Leal SL, Landau SM, Bell RK, Jagust WJ. Hippocampal activation is associated with longitudinal amyloid accumulation and cognitive decline. Elife. (2017) 6:e22978. doi: 10.7554/eLife.22978
42. Minkeviciene R, Rheims S, Dobszay MB, Zilberter M, Hartikainen J, Fulop L, et al. Amyloid-β-Induced Neuronal Hyperexcitability Triggers Progressive Epilepsy. J Neurosci. (2009) 29:3453–62. doi: 10.1523/JNEUROSCI.5215-08.2009
43. Yuan P, Grutzendler J. Attenuation of β-Amyloid Deposition Neurotoxicity by Chemogenetic Modulation of Neural Activity. J Neurosci. (2016) 36:632–41. doi: 10.1523/JNEUROSCI.2531-15.2016
44. Reyes-Marin KE, Nuñez A. Seizure susceptibility in the APP/PS1 mouse model of Alzheimer's disease and relationship with amyloid β plaques. Brain Res. (2017) 1677:93–100. doi: 10.1016/j.brainres.2017.09.026
45. Kazim SF, Chuang SC, Zhao W, Wong RKS, Bianchi R, Iqbal K. Early-Onset Network Hyperexcitability in Presymptomatic Alzheimer's Disease Transgenic Mice Is Suppressed by Passive Immunization with Anti-Human APP/Aβ Antibody and by mGluR5 Blockade. Front Aging Neurosci. (2017) 9:71. doi: 10.3389/fnagi.2017.00071
46. Busche MA, Grienberger C, Keskin AD, Song B, Neumann U, Staufenbiel M, et al. Decreased amyloid-β and increased neuronal hyperactivity by immunotherapy in Alzheimer's models. Nat Neurosci. (2015) 18:1725–7. doi: 10.1038/nn.4163
47. Orbán G, Völgyi K, Juhász G, Penke B, Kékesi KA, Kardos J, et al. Different electrophysiological actions of 24- and 72-hour aggregated amyloid-beta oligomers on hippocampal field population spike in both anesthetized and awake rats. Brain Res. (2010) 1354:227–35. doi: 10.1016/j.brainres.2010.07.061
48. Liguori C, Spanetta M, Romoli M, Placidi F, Nardi Cesarini E, Mercuri NB, et al. Sleep disorders and late-onset epilepsy of unknown origin: Understanding new trajectories to brain amyloidopathy. Mech Ageing Dev. (2021) 194:111434. doi: 10.1016/j.mad.2021.111434
49. Lerdkrai C, Asavapanumas N, Brawek B, Kovalchuk Y, Mojtahedi NM, del Moral MO, et al. Intracellular Ca2+ stores control in vivo neuronal hyperactivity in a mouse model of Alzheimer's disease. Proc Natl Acad Sci. (2018) 115:E1279–88. doi: 10.1073/pnas.1714409115
50. Sun JL, Stokoe SA, Roberts JP, Sathler MF, Nip KA, Shou J, et al. Co-activation of selective nicotinic acetylcholine receptors is required to reverse beta amyloid–induced Ca2+ hyperexcitation. Neurobiol Aging. (2019) 84:166–77. doi: 10.1016/j.neurobiolaging.2019.09.005
51. Cattaud V, Bezzina C, Rey CC, Lejards C, Dahan L, Verret L. Early disruption of parvalbumin expression and perineuronal nets in the hippocampus of the Tg2576 mouse model of Alzheimer's disease can be rescued by enriched environment. Neurobiol Aging. (2018) 72:147–58. doi: 10.1016/j.neurobiolaging.2018.08.024
52. Verret L, Mann EO, Hang GB, Barth AMI, Cobos I, Ho K., et al. Inhibitory Interneuron Deficit Links Altered Network Activity and Cognitive Dysfunction in Alzheimer Model. Cell. (2012) 149:708–21. doi: 10.1016/j.cell.2012.02.046
53. Corbett BF, Leiser SC, Ling HP, Nagy R, Breysse N, Zhang X, et al. Sodium channel cleavage is associated with aberrant neuronal activity and cognitive deficits in a mouse model of Alzheimer's disease. J Neurosci. (2013) 33:7020–6. doi: 10.1523/JNEUROSCI.2325-12.2013
54. Kwok JCF, Dick G, Wang D, Fawcett JW. Extracellular matrix and perineuronal nets in CNS repair. Dev Neurobiol. (2011) 71:1073–89. doi: 10.1002/dneu.20974
55. Shi W, Wei X, Wang X, Du S, Liu W, Song J, et al. Perineuronal nets protect long-term memory by limiting activity-dependent inhibition from parvalbumin interneurons. Proc Natl Acad Sci. (2019) 116:27063–73. doi: 10.1073/pnas.1902680116
56. Hijazi S, Heistek TS, Scheltens P, Neumann U, Shimshek DR, Mansvelder HD., Smit AB, van Kesteren RE, et al. Early restoration of parvalbumin interneuron activity prevents memory loss and network hyperexcitability in a mouse model of Alzheimer's disease. Mol Psychiatry. (2019) 25:3380–98. doi: 10.1038/s41380-019-0483-4
57. Lu MH, Zhao XY, Xu DE, Chen JB, Ji WL, Huang ZP, et al. Transplantation of GABAergic interneuron progenitor attenuates cognitive deficits of Alzheimer's disease model mice. J. Alzheimer's Dis. (2020) 75:245–60. doi: 10.3233/JAD-200010
58. Mokhtar SH, Bakhuraysah MM, Cram DS, Petratos S. The Beta-Amyloid Protein of Alzheimer's Disease: Communication Breakdown by Modifying the Neuronal Cytoskeleton. Int J AlzheimerDis. (2013) 2013:e910502. doi: 10.1155/2013/910502
59. DeVos SL, Goncharoff DK, Chen G, Kebodeaux CS, Yamada K, Stewart FR, et al. Antisense reduction of tau in adult mice protects against seizures. J Neurosci. (2013) 33:12887–97. doi: 10.1523/JNEUROSCI.2107-13.2013
60. Tábuas-Pereira M, Durães J, Lopes J, Sales F, Bento C, Duro D, et al. Increased CSF tau is associated with a higher risk of seizures in patients with Alzheimer's disease. Epilepsy Behav. (2019) 98:207–9. doi: 10.1016/j.yebeh.2019.06.033
61. Hall AM, Throesch BT, Buckingham SC, Markwardt SJ, Peng Y, Wang Q, et al. Tau-dependent Kv4. 2 depletion and dendritic hyperexcitability in a mouse model of Alzheimer's disease. J Neurosci. (2015) 35:6221–30. doi: 10.1523/JNEUROSCI.2552-14.2015
62. Ittner LM, Ke YD, Delerue F, Bi M, Gladbach A, van Eersel J, et al. Dendritic Function of Tau Mediates Amyloid-β Toxicity in Alzheimer's Disease Mouse Models. Cell. (2010) 142:387–97. doi: 10.1016/j.cell.2010.06.036
63. Mondragón-Rodríguez S, Salgado-Burgos H, Peña-Ortega F. Circuitry and Synaptic Dysfunction in Alzheimer's Disease: A New Tau Hypothesis. Neural Plast. (2020) 2020:e2960343. doi: 10.1155/2020/2960343
64. Alves M, Kenny A, de Leo G, Beamer EH, Engel T. Tau Phosphorylation in a Mouse Model of Temporal Lobe Epilepsy. Front Aging Neurosci. (2019) 11:308. doi: 10.3389/fnagi.2019.00308
65. Tai XY, Koepp M, Duncan JS, Fox N, Thompson P, Baxendale S, et al. Hyperphosphorylated tau in patients with refractory epilepsy correlates with cognitive decline: a study of temporal lobe resections. Brain. (2016) 139:2441–55. doi: 10.1093/brain/aww187
66. Wu JW, Hussaini SA, Bastille IM, Rodriguez GA, Mrejeru A, Rilett K, et al. Neuronal activity enhances tau propagation and tau pathology in vivo. Nat Neurosci. (2016) 19:1085–92. doi: 10.1038/nn.4328
67. Busche MA, Wegmann S, Dujardin S, Commins C, Schiantarelli J, Klickstein N, et al. Tau impairs neural circuits, dominating amyloid-β effects, in Alzheimer models in vivo. Nat Neurosci. (2019) 22:57–64. doi: 10.1038/s41593-018-0289-8
68. Cummings JL, Back C. The Cholinergic Hypothesis of Neuropsychiatric Symptoms in Alzheimer's Disease. Am J Geriatr Psychiatry. (1998) 6:S64–78. doi: 10.1097/00019442-199821001-00009
69. Szot P. Common factors among Alzheimer's disease, Parkinson's disease, and epilepsy: Possible role of the noradrenergic nervous system. Epilepsia. (2012) 53:61–6. doi: 10.1111/j.1528-1167.2012.03476.x
70. Kang JE, Lim MM, Bateman RJ, Lee JJ, Smyth LP, Cirrito JR, et al. Amyloid-b Dynamics Are Regulated by Orexin and the Sleep-Wake Cycle. Science. (2009) 326:4. doi: 10.1126/science.1180962
71. Shokri-Kojori E, Wang GJ, Wiers CE, Demiral SB, Guo M, Kim SW, et al. β-Amyloid accumulation in the human brain after one night of sleep deprivation. Proc Natl Acad Sci. (2018) 115:4483–8. doi: 10.1073/pnas.1721694115
72. Tabuchi M, Lone SR, Liu S, Liu Q, Zhang J, Spira AP, et al. Sleep Interacts with Aβ to Modulate Intrinsic Neuronal Excitability. Curr Biol. (2015) 25:702–12. doi: 10.1016/j.cub.2015.01.016
73. Mander BA, Marks SM, Vogel JW, Rao V, Lu B, Saletin JM, et al. β-amyloid disrupts human NREM slow waves and related hippocampus-dependent memory consolidation. Nat Neurosci. (2015) 18:1051–7. doi: 10.1038/nn.4035
74. Ju ES, Lucey BP, Holtzman DM. Sleep and Alzheimer disease pathology—a bidirectional relationship. Nat Rev Neurol. (2014) 10:115–9. doi: 10.1038/nrneurol.2013.269
75. Winer JR, Mander BA, Kumar S, Reed M, Baker SL, Jagust WJ, et al. Sleep Disturbance Forecasts β-Amyloid Accumulation across Subsequent Years. Curr Biol. (2020) 30:4291–8.e3. doi: 10.1016/j.cub.2020.08.017
76. Lucey BP, McCullough A, Landsness EC, Toedebusch CD, McLeland JS, Zaza AM, et al. Reduced non–rapid eye movement sleep is associated with tau pathology in early Alzheimer's disease. Sci Transl Med. (2019) 11:eaau6550. doi: 10.1126/scitranslmed.aau6550
77. Holth JK, Fritschi SK, Wang C, Pedersen NP, Cirrito JR, Mahan TE, et al. The sleep-wake cycle regulates brain interstitial fluid tau in mice and CSF tau in humans. Science. (2019) 363:880–4. doi: 10.1126/science.aav2546
78. Iliff JJ, Wang M, Liao Y, Plogg BA, Peng W, Gundersen GA, et al. A Paravascular Pathway Facilitates CSF Flow Through the Brain Parenchyma and the Clearance of Interstitial Solutes, Including Amyloid β. Sci Transl Med. (2012) 4:147ra111. doi: 10.1126/scitranslmed.3003748
79. Xie L, Kang H, Xu Q, Chen MJ, Liao Y, Thiyagarajan M, et al. Sleep Drives Metabolite Clearance from the Adult Brain. Science. (2013) 342:373–7. doi: 10.1126/science.1241224
80. Rasmussen MK, Mestre H, Nedergaard M. The glymphatic pathway in neurological disorders. Lancet Neurol. (2018) 17:1016–24. doi: 10.1016/S1474-4422(18)30318-1
81. Boespflug EL, Iliff JJ. The Emerging Relationship Between Interstitial Fluid–Cerebrospinal Fluid Exchange, Amyloid-β, and Sleep. Biol Psychiatry. (2018) 83:328–36. doi: 10.1016/j.biopsych.2017.11.031
82. Silva I, Silva J, Ferreira R, Trigo D. Glymphatic system, AQP4, and their implications in Alzheimer's disease. Neurol Res Pract. (2021) 3:5. doi: 10.1186/s42466-021-00102-7
83. Kress BT, Iliff JJ, Xia M, Wang M, Wei HS, Zeppenfeld D, et al. Nedergaard M, Impairment of paravascular clearance pathways in the aging brain. Ann Neurol. (2014) 76:845–61. doi: 10.1002/ana.24271
84. Christensen J, Yamakawa GR, Shultz SR, Mychasiuk R. Is the glymphatic system the missing link between sleep impairments and neurological disorders? Examining the implications and uncertainties. Prog Neurobiol. (2021) 198:101917. doi: 10.1016/j.pneurobio.2020.101917
85. Liu D, He X, Wu D, Zhang Q, Yang C, Liang F, et al. Continuous theta burst stimulation facilitates the clearance efficiency of the glymphatic pathway in a mouse model of sleep deprivation. Neurosci Lett. (2017) 653:189–94. doi: 10.1016/j.neulet.2017.05.064
86. Yang J, Lunde LK, Nuntagij P, Oguchi T, Camassa LMA, Nilsson LNG, et al. J Alzheimers Dis. (2011) 27:711–22. doi: 10.3233/JAD-2011-110725
87. Eid T., Lee TSW, Thomas MJ, Amiry-Moghaddam M, Bjørnsen LP, Spencer DD, et al. Loss of perivascular aquaporin 4 may underlie deficient water and K+ homeostasis in the human epileptogenic hippocampus. Proc Natl Acad Sci. (2005) 102:1193–8. doi: 10.1073/pnas.0409308102
88. Peng W, Achariyar TM, Li B, Liao Y, Mestre H, Hitomi E, et al. Suppression of glymphatic fluid transport in a mouse model of Alzheimer's disease. Neurobiol Dis. (2016) 93:215–25. doi: 10.1016/j.nbd.2016.05.015
89. Xu Z, Xiao N, Chen Y, Huang H, Marshall C, Gao J, et al. Deletion of aquaporin-4 in APP/PS1 mice exacerbates brain Aβ accumulation and memory deficits. Mol Neurodegener. (2015) 10:58. doi: 10.1186/s13024-015-0056-1
90. Abe Y, Ikegawa N, Yoshida K, Muramatsu K, Hattori S, Kawai K, et al. Behavioral and electrophysiological evidence for a neuroprotective role of aquaporin-4 in the 5xFAD transgenic mice model. Acta Neuropathol Commun. (2020) 8:67. doi: 10.1186/s40478-020-00936-3
91. Dreha-Kulaczewski S, Joseph AA, Merboldt KD, Ludwig HC, Gärtner J, Frahm J. Inspiration is the major regulator of human CSF flow. J Neurosci. (2015) 35:2485–91. doi: 10.1523/JNEUROSCI.3246-14.2015
92. Bliwise DL. Sleep apnea, APOE4 Alzheimer's disease 20 years counting? J Psychosom Res. (2002) 53:539–46. doi: 10.1016/S0022-3999(02)00436-1
93. Liguori C, Mercuri NB, Nuccetelli M, Izzi F, Cordella A, Bernardini S, et al. Obstructive sleep apnea may induce orexinergic system and cerebral β-amyloid metabolism dysregulation: is it a further proof for Alzheimer's disease risk? Sleep Med. (2019) 56:171–6. doi: 10.1016/j.sleep.2019.01.003
94. Sharma RA, Varga AW, Bubu OM, Pirraglia E, Kam K, Parekh A, et al. Obstructive sleep apnea severity affects amyloid burden in cognitively normal elderly: A longitudinal study. Am J Respir Critic Care Med. (2018) 197:933–43. doi: 10.1164/rccm.201704-0704OC
95. Hablitz LM, Plá V, Giannetto M, Vinitsky HS, Stæger FF, Metcalfe T, et al. Circadian control of brain glymphatic and lymphatic fluid flow. Nat Commun. (2020) 11:4411. doi: 10.1038/s41467-020-18115-2
96. Musiek ES. Circadian clock disruption in neurodegenerative diseases: cause and effect? Front Pharmacol. (2015) 6:29. doi: 10.3389/fphar.2015.00029
97. Bookheimer SY, Strojwas MH, Cohen MS, Saunders AM, Pericak-Vance MA, Mazziotta JC, et al. N Engl J Med. (2000) 343:450–6. doi: 10.1056/NEJM200008173430701
98. Andrews-Zwilling Y, Bien-Ly N, Xu Q, Li G, Bernardo A, Yoon SY, et al. Apolipoprotein E4 causes age- and Tau-dependent impairment of GABAergic interneurons, leading to learning and memory deficits in mice. J Neurosci. (2010) 30:13707–17. doi: 10.1523/JNEUROSCI.4040-10.2010
99. Najm R, Jones EA, Huang Y. Apolipoprotein E4, inhibitory network dysfunction, Alzheimer's disease. Mol Neurodegener. (2019) 14:24. doi: 10.1186/s13024-019-0324-6
100. Aroor A, Brewster AL. Seizing the Alzheimer's Brain: A Role for Sirtuin 3 in Hyperexcitability. Epilepsy Curr. (2020) 20:224–6. doi: 10.1177/1535759720932157
101. Chin J, Massaro CM, Palop JJ, Thwin MT Yu G-Q, Bien-Ly N., et al. Reelin depletion in the entorhinal cortex of human amyloid precursor protein transgenic mice and humans with Alzheimer's disease. J Neurosci. (2007) 27:2727–33. doi: 10.1523/JNEUROSCI.3758-06.2007
102. Machado RA, Benjumea-Cuartas V, Zapata Berruecos JF, Agudelo-Flóres PM, Salazar-Peláez LM. Reelin, tau phosphorylation and psychiatric complications in patients with hippocampal sclerosis and structural abnormalities in temporal lobe epilepsy. Epilepsy Behav. (2019) 96:192–9. doi: 10.1016/j.yebeh.2019.04.052
103. Haberman RP, Branch A, Gallagher M. Targeting Neural Hyperactivity as a Treatment to Stem Progression of Late-Onset Alzheimer's Disease. Neurotherapeutics. (2017) 14:662–76. doi: 10.1007/s13311-017-0541-z
104. Krezymon A, Richetin K, Halley H, Roybon L, Lassalle JM, Francès B, et al. Modifications of Hippocampal Circuits and Early Disruption of Adult Neurogenesis in the Tg2576 Mouse Model of Alzheimer's Disease. PLoS ONE. (2013) 8:e76497. doi: 10.1371/journal.pone.0076497
105. Shapiro LA, Ribak CE, Jessberger S. Structural changes for adult-born dentate granule cells after status epilepticus. Epilepsia. (2008) 49:13–8. doi: 10.1111/j.1528-1167.2008.01633.x
106. Costa C, Romoli M, Liguori C, Farotti L, Eusebi P, Bedetti C, et al. Alzheimer's disease and late-onset epilepsy of unknown origin: two faces of beta amyloid pathology. Neurobiol Aging. (2019) 73:61–7. doi: 10.1016/j.neurobiolaging.2018.09.006
107. Costa C, Parnetti L, D'Amelio M, Tozzi A, Tantucci M, Romigi A, et al. Epilepsy, amyloid-β, and D1 dopamine receptors: a possible pathogenetic link? Neurobiol Aging. (2016) 48:161–71. doi: 10.1016/j.neurobiolaging.2016.08.025
108. Mackenzie IRA, Miller LA. Senile plaques in temporal lobe epilepsy. Acta Neuropathol (Berl). (1994) 87:504–10. doi: 10.1007/BF00294177
109. Nardi Cesarini E, Babiloni C, Salvadori N, Farotti L, Del Percio C, Pascarelli MT, et al. Late-Onset Epilepsy With Unknown Etiology: A Pilot Study on Neuropsychological Profile, Cerebrospinal Fluid Biomarkers, and Quantitative EEG Characteristics. Front Neurol. (2020) 11:199. doi: 10.3389/fneur.2020.00199
110. Kaestner E, Reyes A, Chen A, Rao J, Macari AC, Choi JY, et al. for the Alzheimer's Disease Neuroimaging Initiative. Atrophy and cognitive profiles in older adults with temporal lobe epilepsy are similar to mild cognitive impairment. Brain. (2021) 144:236–50. doi: 10.1093/brain/awaa397
111. Liedorp M, Stam CJ., van der Flier WM, Pijnenburg YAL, Scheltens P. Prevalence and Clinical Significance of Epileptiform EEG Discharges in a Large Memory Clinic Cohort. Dement Geriatr Cogn Disord. (2010) 29:432–7. doi: 10.1159/000278620
112. Vossel KA, Beagle AJ, Rabinovici GD, Shu H, Lee SE, Naasan G, et al. Seizures and Epileptiform Activity in the Early Stages of Alzheimer Disease. JAMA Neurol. (2013) 70:1158. doi: 10.1001/jamaneurol.2013.136
113. Brunetti V, D'Atri A, Della Marca G, Vollono C, Marra C, Vita MG, et al. Subclinical epileptiform activity during sleep in Alzheimer's disease and mild cognitive impairment. Clin Neurophysiol. (2020) 131:1011–8. doi: 10.1016/j.clinph.2020.02.015
114. Vossel K, Ranasinghe KG, Beagle AJ, La A, Ah Pook K, Castro M, et al. Effect of Levetiracetam on Cognition in Patients With Alzheimer Disease With and Without Epileptiform Activity: A Randomized Clinical Trial. JAMA Neurol. (2021). doi: 10.1001/jamaneurol.2021.3310
115. Morris JC, McKeel DW Jr, Fulling K, Torack RM, Berg L. Validation of clinical diagnostic criteria for Alzheimer's disease. Ann Neurol. (1988) 24, 17–22. doi: 10.1002/ana.410240105
116. McAreavey MJ, Ballinger BR, Fenton GW. Epileptic Seizures in Elderly Patients with Dementia. Epilepsia. (1992) 33:657–60. doi: 10.1111/j.1528-1157.1992.tb02343.x
117. Volicer L, Smith S, Volicer BJ. Effect of Seizures on Progression of Dementia of the Alzheimer Type. Dement Geriatr Cogn Disord. (1995) 6:258–63. doi: 10.1159/000106956
118. Rao SC, Dove G, Cascino GD, Petersen RC. Recurrent seizures in patients with dementia: Frequency, seizure types, treatment outcome. Epilepsy Behav. (2009) 14:118–20. doi: 10.1016/j.yebeh.2008.08.012
119. Scarmeas N, Honig LS, Choi H, Cantero J, Brandt J, Blacker D, et al. Seizures in Alzheimer Disease: Who, When, and How Common? Arch Neurol. (2009) 66:992–7. doi: 10.1001/archneurol.2009.130
120. Bernardi S, Scaldaferri N, Vanacore N, Trebbastoni A, Francia A, D'Amico A. Prencipe M, Seizures in Alzheimer's disease: a retrospective study of a cohort of outpatients. Epileptic Disord. (2010) 12:16–21. doi: 10.1684/epd.2010.0290
121. Irizarry MC, Jin S, He F, Emond JA, Raman R, Thomas RG, et al. Incidence of New-Onset Seizures in Mild to Moderate Alzheimer Disease. Arch Neurol. (2012) 69:368. doi: 10.1001/archneurol.2011.830
122. Imfeld P, Bodmer M, Schuerch M, Jick SS, Meier CR. Seizures in patients with Alzheimer's disease or vascular dementia: A population-based nested case-control analysis: Seizures in Alzheimer's and Vascular Dementia. Epilepsia. (2013) 54:700–7. doi: 10.1111/epi.12045
123. Cook M, Baker N, Lanes S, Bullock R, Wentworth C, Arrighi HM. Incidence of stroke and seizure in Alzheimer's disease dementia. Age Ageing. (2015) 44:695–9. doi: 10.1093/ageing/afv061
124. Giorgi FS, Baldacci F, Dini E, Tognoni G, Bonuccelli U. Epilepsy occurrence in patients with Alzheimer's disease: clinical experience in a tertiary dementia center. Neurol Sci. (2016) 37:645–7. doi: 10.1007/s10072-015-2442-4
125. DiFrancesco JC, Tremolizzo L, Polonia V, Giussani G, Bianchi E, Franchi C, et al. J Alzheimers Dis. (2017) 60:1267–74. doi: 10.3233/JAD-170392
126. Rauramaa T, Saxlin A, Lohvansuu K, Alafuzoff I, Pitkänen A, Soininen H. Epilepsy in neuropathologically verified Alzheimer's disease. Seizure. (2018) 58:9–12. doi: 10.1016/j.seizure.2018.03.014
127. Baker J, Libretto T, Henley W, Zeman A. The prevalence and clinical features of epileptic seizures in a memory clinic population. Seizure. (2019) 71:83–92. doi: 10.1016/j.seizure.2019.06.016
128. Lyou HJ, Seo KD, Lee JE, Pak HY, Lee JH. Association of Alzheimer's Disease with the Risk of Developing Epilepsy: a 10-Year Nationwide Cohort Study. Dement Neurocognitive Disord. (2019) 17:156–62. doi: 10.12779/dnd.2018.17.4.156
129. Stefanidou M, Beiser AS, Himali JJ, Peng TJ, Devinsky O, Seshadri S, et al. Bi-directional association between epilepsy and dementia: The Framingham Heart Study. Neurology. (2020) 95:e3241–7. doi: 10.1212/WNL.0000000000011077
130. Vöglein J, Ricard I, Noachtar S, Kukull WA, Dieterich M, Levin J, et al. Seizures in Alzheimer's disease are highly recurrent and associated with a poor disease course. J Neurol. (2020) 267:2941–8. doi: 10.1007/s00415-020-09937-7
131. Zelano J, Brigo F, Garcia-Patek S. Increased risk of epilepsy in patients registered in the Swedish Dementia Registry. Eur J Neurol. (2020) 27:129–35. doi: 10.1111/ene.14043
132. Blank LJ, Willis AW. Incidence of Epilepsy Among Medicare Beneficiaries Diagnosed with Alzheimer Dementia or Parkinson Disease. Neurology. (2019) 92.
133. Risse SC, Lampe TH, Bird TD, Nochlin D, Sumi SM, Keenan T, et al. Myoclonus, seizures, and paratonia in Alzheimer disease. Alzheimer Dis Assoc Disord. (1990) 4:217–25. doi: 10.1097/00002093-199040400-00003
134. Born HA. Seizures in Alzheimer's disease. Neuroscience. (2015) 286:251–63. doi: 10.1016/j.neuroscience.2014.11.051
135. Lam AD, Deck G, Goldman A, Eskandar EN, Noebels J, Cole AJ. Silent hippocampal seizures and spikes identified by foramen ovale electrodes in Alzheimer's disease. Nat Med. (2017) 23:678–80. doi: 10.1038/nm.4330
136. Horvath AA, Papp A, Zsuffa J, Szucs A, Luckl J, Radai F, et al. Subclinical epileptiform activity accelerates the progression of Alzheimer's disease: A long-term EEG study. Clin Neurophysiol. (2021) 132:1982–9. doi: 10.1016/j.clinph.2021.03.050
137. Haoudy S, Jonveaux T, Aron O, Puisieux S, Hopes L, Tyvaert L. Epilepsy in early onset Alzheimer's disease. Alzheimers Dement. (2020) 16. doi: 10.1002/alz.037573
138. Mendez MF, Lim GTH. Seizures in Elderly Patients with Dementia: Epidemiology and Management. Drugs Aging. (2003) 20:791–803. doi: 10.2165/00002512-200320110-00001
139. Ng M, Pavlova M. Why Are Seizures Rare in Rapid Eye Movement Sleep? Review of the Frequency of Seizures in Different Sleep Stages. Epilepsy Res Treat. (2013) 2013:1–10. doi: 10.1155/2013/932790
140. Brown R, Lam AD, Gonzalez-Sulser A, Ying A, Jones MR, Chou CC, et al. Circadian and Brain State Modulation of Network Hyperexcitability in Alzheimer's Disease. eNeuro. (2018) 5. doi: 10.1523/ENEURO.0426-17.2018
141. Montplaisir J, Petit D, Gauthier S, Gaudreau H, Décary A. Sleep disturbances and eeg slowing in alzheimer's disease. Sleep Res Online. (1998) 1:147–51.
142. Petit D, Gagnon JF, Fantini ML, Ferini-Strambi L, Montplaisir J. Sleep quantitative EEG in neurodegenerative disorders. J Psychosom Res. (2004) 56:487–96. doi: 10.1016/j.jpsychores.2004.02.001
143. Klinzing JG, Niethard N, Born J. Mechanisms of systems memory consolidation during sleep. Nat Neurosci. (2019) 22:1598–610. doi: 10.1038/s41593-019-0467-3
144. Rasch B, Born J. About Sleep's Role in Memory. Physiol Rev. (2013) 93:681–766. doi: 10.1152/physrev.00032.2012
145. Watson BO, Buzsáki G. Sleep, Memory & Brain Rhythms. Daedalus. (2015) 144:67–82. doi: 10.1162/DAED_a_00318
146. Marr D. A theory for cerebral neocortex. Proc R Soc Lond. (1970) 176:161–234. doi: 10.1098/rspb.1970.0040
147. Marr D. Simple memory: a theory for archicortex. Philocophical Trans R Soc Lond. (1971) 262:23–81. doi: 10.1098/rstb.1971.0078
148. McClelland JL, O'Reilly RC. Why There Are Complementary Learning Systems in the Hippocampus and Neocortex:InsightsFrom the Successesand Failuresof Connectionist Models of Learning and Memory. Psychol Rev. (1995) 102:419–57. doi: 10.1037/0033-295X.102.3.419
149. Wilson M, McNaughton B. Reactivation of hippocampal ensemble memories during sleep. Science. (1994) 265:676–9. doi: 10.1126/science.8036517
150. Frankland PW, Bontempi B. The organization of recent and remote memories. Nat Rev Neurosci. (2005) 6:119–30. doi: 10.1038/nrn1607
151. Nadel L, Moscovitcht M. Memory consolidation, retrograde amnesia and the hippocampal complex. Curr Opin Neurobiol. (1997) 217–227. doi: 10.1016/S0959-4388(97)80010-4
152. Diekelmann S, Born J. The memory function of sleep. Nat Rev Neurosci. (2010) 11:114–26. doi: 10.1038/nrn2762
153. Marshall L, Born J. The contribution of sleep to hippocampus-dependent memory consolidation. Trends Cogn Sci. (2007) 11:442–50. doi: 10.1016/j.tics.2007.09.001
154. Buzsáki G. Memory consolidation during sleep: a neurophysiological perspective. J Sleep Res. (1998) 7:17–23. doi: 10.1046/j.1365-2869.7.s1.3.x
155. Buzsáki G. Two-stage model of memory trace formation: A role for “noisy” brain states. Neuroscience. (1989) 31:551–70. doi: 10.1016/0306-4522(89)90423-5
156. Ribeiro S. Reverberation, storage, and postsynaptic propagation of memories during sleep. Learn Mem. (2004) 11:686–96. doi: 10.1101/lm.75604
157. Born J, Wilhelm I. System consolidation of memory during sleep. Psychol Res. (2012) 76:192–203. doi: 10.1007/s00426-011-0335-6
158. Giuditta A, Ambrosini MV, Montagnese P, Mandile P, Cotugno M, Zucconi GG, et al. The sequential hypothesis of the function of sleep. Behav Brain Res. (1995) 69:157–66. doi: 10.1016/0166-4328(95)00012-I
159. Grosmark AD, Mizuseki K, Pastalkova E, Diba K, Buzsáki G. REM Sleep Reorganizes Hippocampal Excitability. Neuron. (2012) 75:1001–7. doi: 10.1016/j.neuron.2012.08.015
160. Tononi G, Cirelli C. Sleep and the Price of Plasticity: From Synaptic and Cellular Homeostasis to Memory Consolidation and Integration. Neuron. (2014) 81:12–34. doi: 10.1016/j.neuron.2013.12.025
161. Gelinas JN, Khodagholy D, Thesen T, Devinsky O, Buzsáki G. Interictal epileptiform discharges induce hippocampal–cortical coupling in temporal lobe epilepsy. Nat Med. (2016) 22:641–8. doi: 10.1038/nm.4084
162. Dudai Y, Karni A, Born J. The Consolidation and Transformation of Memory. Neuron. (2015) 88:20–32. doi: 10.1016/j.neuron.2015.09.004
163. Mölle M, Eschenko O, Gais S, Sara SJ, Born J. The influence of learning on sleep slow oscillations and associated spindles and ripples in humans and rats. Eur J Neurosci. (2009) 29:1071–81. doi: 10.1111/j.1460-9568.2009.06654.x
164. Marshall L, Helgadóttir H, Mölle M, Born J. Boosting slow oscillations during sleep potentiates memory. Nature. (2006) 444:610–3. doi: 10.1038/nature05278
165. Steriade M. Grouping of brain rhythms in corticothalamic systems. Neuroscience. (2006) 137:1087–106. doi: 10.1016/j.neuroscience.2005.10.029
166. Sirota A, Csicsvari J, Buhl D, Buzsaki G. Communication between neocortex and hippocampus during sleep in rodents. Proc Natl Acad Sci. (2003) 100:2065–9. doi: 10.1073/pnas.0437938100
167. Clemens Z, Mölle M, Eross L, Barsi P, Halász P, Born J. Temporal coupling of parahippocampal ripples, sleep spindles and slow oscillations in humans. Brain. (2007) 130:2868–78. doi: 10.1093/brain/awm146
168. Mölle M, Bergmann TO, Marshall L, Born J. Fast and Slow Spindles during the Sleep Slow Oscillation: Disparate Coalescence and Engagement in Memory Processing. Sleep. (2011) 34:1411–21. doi: 10.5665/SLEEP.1290
169. Andrillon T, Nir Y, Staba RJ, Ferrarelli F, Cirelli C, Tononi G, et al. Sleep spindles in humans: insights from intracranial EEG and unit recordings. J Neurosci. (2011) 31:17821–34. doi: 10.1523/JNEUROSCI.2604-11.2011
170. Latchoumane FV, Ngo HVV, Born J, Shin H-S. Thalamic Spindles Promote Memory Formation during Sleep through Triple Phase-Locking of Cortical, Thalamic, Hippocampal Rhythms. Neuron. (2017) 95:424–35.e6. doi: 10.1016/j.neuron.2017.06.025
171. Clemens Z, Fabó D, Halász P. Overnight verbal memory retention correlates with the number of sleep spindles. Neuroscience. (2005) 132:529–35. doi: 10.1016/j.neuroscience.2005.01.011
172. Cox R, Hofman WF, Talamini LM. Involvement of spindles in memory consolidation is slow wave sleep-specific. Learn Mem. (2012) 264–267. doi: 10.1101/lm.026252.112
173. Griessenberger H, Hoedlmoser K, Heib DPJ, Lechinger J, Klimesch W, Schabus M. Consolidation of temporal order in episodic memories. Biol Psychol. (2012) 91:150–5. doi: 10.1016/j.biopsycho.2012.05.012
174. van der Helm E, Gujar N, Nishida M, Walker MP. Sleep-Dependent Facilitation of Episodic Memory Details. PLoS ONE. (2011) 6:e27421. doi: 10.1371/journal.pone.0027421
175. Crowley K, Trinder J, Kim Y, Carrington M, Colrain IM. The effects of normal aging on sleep spindle and K-complex production. Clin Neurophysiol. (2002) 113:1615–22. doi: 10.1016/S1388-2457(02)00237-7
176. Mander BA, Rao V, Lu B, Saletin JM, Ancoli-Israel S, Jagust WJ, et al. Impaired Prefrontal Sleep Spindle Regulation of Hippocampal-Dependent Learning in Older Adults. Cereb Cortex. (2014) 24:3301–9. doi: 10.1093/cercor/bht188
177. Nicolas A, Petit D, Rompré S, Montplaisir J. Sleep spindle characteristics in healthy subjects of different age groups. Clin Neurophysiol. (2001) 112:521–7. doi: 10.1016/S1388-2457(00)00556-3
178. Helfrich RF, Mander BA, Jagust WJ, Knight RT, Walker MP. Old Brains Come Uncoupled in Sleep: Slow Wave-Spindle Synchrony, Brain Atrophy, and Forgetting. Neuron. (2018) 97:221–230.e4. doi: 10.1016/j.neuron.2017.11.020
179. Liu S, Pan J, Tang K, Lei Q, He L, Meng Y, et al. Sleep spindles, K-complexes, limb movements and sleep stage proportions may be biomarkers for amnestic mild cognitive impairment and Alzheimer's disease. Sleep Breath. (2020) 24:637–51. doi: 10.1007/s11325-019-01970-9
180. Gorgoni M, Lauri G, Truglia I, Cordone S, Sarasso S, Scarpelli S, et al. Parietal Fast Sleep Spindle Density Decrease in Alzheimer's Disease and Amnesic Mild Cognitive Impairment. Neural Plast. (2016) 2016:e8376108. doi: 10.1155/2016/8376108
181. Kam K, Parekh A, Sharma RA, Andrade A, Lewin M, Castillo B, et al. Ayappa, de Leon MJ, Petkova E, Varga AW, Osorio RS, Sleep oscillation-specific associations with Alzheimer's disease CSF biomarkers: novel roles for sleep spindles and tau. Mol Neurodegener. (2019) 14:10. doi: 10.1186/s13024-019-0309-5
182. Rauchs G, Schabus M, Parapatics S, Bertran F, Clochon P, Hot P, et al. Is there a link between sleep changes and memory in Alzheimer's disease? Neuroreport. (2008) 19:1159–62. doi: 10.1097/WNR.0b013e32830867c4
183. Dang-Vu TT, Bonjean M, Schabus M, Boly M, Darsaud A, Desseilles M, et al. Interplay between spontaneous and induced brain activity during human non-rapid eye movement sleep. Proc Natl Acad Sci. (2011) 108:15438–43. doi: 10.1073/pnas.1112503108
184. Schabus MD, Dang-Vu TT, Heib DPJ, Boly M, Desseilles M, Vandewalle G, et al. The Fate of Incoming Stimuli during NREM Sleep is Determined by Spindles and the Phase of the Slow Oscillation. Front Neurol. (2012) 3:40. doi: 10.3389/fneur.2012.00040
185. Buzsáki G. Hippocampal sharp wave-ripple: A cognitive biomarker for episodic memory and planning. Hippocampus. (2015) 25:1073–188. doi: 10.1002/hipo.22488
186. Oliva A, Fernández-Ruiz A, Buzsáki G, Berényi A. Role of Hippocampal CA2 Region in Triggering Sharp-Wave Ripples. Neuron. (2016) 91:1342–55. doi: 10.1016/j.neuron.2016.08.008
187. Oliva A, Fernández-Ruiz A, Leroy F, Siegelbaum SA. Hippocampal CA2 sharp-wave ripples reactivate and promote social memory. Nature. (2020) 587:264–9. doi: 10.1038/s41586-020-2758-y
188. O'Keefe J, Dostrovsky J. (1971). The hippocampus as a spatial map: Preliminary evidence from unit activity in the freely-moving rat. Brain Res. 34:171–5. doi: 10.1016/0006-8993(71)90358-1
189. Clemens Z, Mölle M, Erss L, Jakus R, Rásonyi G, Halász P, et al. Fine tuned coupling between human parahippocampal ripples and sleep spindles. Eur J Neurosci. (2011) 33:511–20. doi: 10.1111/j.1460-9568.2010.07505.x
190. Siapas AG, Wilson MA. Coordinated Interactions between Hippocampal Ripples and Cortical Spindles during Slow-Wave Sleep. Neuron. (1998) 21:1123–8. doi: 10.1016/S0896-6273(00)80629-7
191. Staresina BP, Bergmann TO, Bonnefond M, van der Meij R, Jensen O, Deuker L, et al. Hierarchical nesting of slow oscillations, spindles and ripples in the human hippocampus during sleep. Nat Neurosci. (2015) 18:1679–86. doi: 10.1038/nn.4119
192. Chrobak JJ, Buzsaki G. Selective activation of deep layer (V-VI) retrohippocampal cortical neurons during hippocampal sharp waves in the behaving rat. J Neurosci. (1994) 14:6160–70. doi: 10.1523/JNEUROSCI.14-10-06160.1994
193. Ego-Stengel V, Wilson MA. Disruption of ripple-associated hippocampal activity during rest impairs spatial learning in the rat. Hippocampus. (2009) 20:1–11. doi: 10.1002/hipo.20707
194. Girardeau G, Benchenane K, Wiener SI, Buzsáki G, Zugaro MB. Selective suppression of hippocampal ripples impairs spatial memory. Nat Neurosci. (2009) 12:1222–3. doi: 10.1038/nn.2384
195. Goddard GV, Douglas RM. Does the Engram of Kindling Model the Engram of Normal Long Term Memory? Can J Neurol Sci. (1975) 2:385–94. doi: 10.1017/S0317167100020539
196. Gulyás AI, Freund TT. Generation of physiological and pathological high frequency oscillations: the role of perisomatic inhibition in sharp-wave ripple and interictal spike generation. Curr Opin Neurobiol. (2015) 31:26–32. doi: 10.1016/j.conb.2014.07.020
197. Halász P, Bódizs R, Ujma PP, Fabó D, Szucs A. Strong relationship between NREM sleep, epilepsy and plastic functions — A conceptual review on the neurophysiology background. Epilepsy Res. (2019) 150:95–105. doi: 10.1016/j.eplepsyres.2018.11.008
198. Hájos N, Karlócai MR, Németh B, Ulbert I, Monyer H, Szabó G, et al. Input-output features of anatomically identified CA3 neurons during hippocampal sharp wave/ripple oscillation in vitro. J Neurosci. (2013) 33:11677–91. doi: 10.1523/JNEUROSCI.5729-12.2013
199. Schlingloff D, Káli S, Freund TF, Hájos N, Gulyás AI. Mechanisms of Sharp Wave Initiation Ripple Generation. J Neurosci. (2014) 34:11385–98. doi: 10.1523/JNEUROSCI.0867-14.2014
200. Karlócai MR, Kohus Z, Káli S, Ulbert I, Szabó G, Máté Z, et al. Physiological sharp wave-ripples and interictal events in vitro: what's the difference? Brain. (2014) 137:463–85. doi: 10.1093/brain/awt348
201. Caccavano A, Bozzelli PL, Forcelli PA, Pak DT, Wu JY, Conant K. Inhibitory parvalbumin basket cell activity is selectively reduced during hippocampal sharp wave ripples in a mouse model of familial Alzheimer's disease. J Neurosci. (2020) 40:5116–36. doi: 10.1523/JNEUROSCI.0425-20.2020
202. Jones EA, Gillespie AK, Yoon SY, Frank LM, Huang Y. Early Hippocampal Sharp-Wave Ripple Deficits Predict Later Learning and Memory Impairments in an Alzheimer's Disease Mouse Model. Cell Rep. (2019) 29:2123–33.e4. doi: 10.1016/j.celrep.2019.10.056
203. Stoiljkovic M, Kelley C, Stutz B, Horvath TL, Hajós M. Altered Cortical and Hippocampal Excitability in TgF344-AD Rats Modeling Alzheimer's Disease Pathology. Cereb Cortex. (2018) 29:2716–27. doi: 10.1093/cercor/bhy140
204. Benthem SD, Skelin I, Moseley SC, Stimmell AC, Dixon JR, Melilli AS, et al. Impaired Hippocampal-Cortical Interactions during Sleep in a Mouse Model of Alzheimer's Disease. Curr Biol. (2020) 30:2588–601.e5. doi: 10.1016/j.cub.2020.04.087
205. Bower MR, Stead M, Bower RS, Kucewicz MT, Sulc V, Cimbalnik J, et al. Evidence for Consolidation of Neuronal Assemblies after Seizures in Humans. J Neurosci. (2015) 35:999–1010. doi: 10.1523/JNEUROSCI.3019-14.2015
206. Lambert I, Tramoni-Negre E, Lagarde S, Roehri N, Giusiano B, Trebuchon-Da Fonseca A, et al. Hippocampal Interictal Spikes during Sleep Impact Long-Term Memory Consolidation. Ann Neurol. (2020) 87:976–87. doi: 10.1002/ana.25744
207. Lambert I, Tramoni-Negre E, Lagarde S, Pizzo F, Trebuchon-Da Fonseca A, Bartolomei F, et al. Accelerated long-term forgetting in focal epilepsy: Do interictal spikes during sleep matter? Epilepsia. (2021) 62:563–9. doi: 10.1111/epi.16823
208. Peter-Derex L, Yammine P, Bastuji H, Croisile B. Sleep and Alzheimer's disease. Sleep Med Rev. (2015) 19:29–38. doi: 10.1016/j.smrv.2014.03.007
209. Johnson EL, Krauss GL, Kucharska-Newton A, Albert MS, Brandt J, Walker KA, et al. Dementia in late-onset epilepsy: The Atherosclerosis Risk in Communities study. Neurology. (2020) 95:e3248–56. doi: 10.1212/WNL.0000000000011080
210. Keret O, Hoang TD, Xia F, Rosen HJ, Yaffe K. Association of Late-Onset Unprovoked Seizures of Unknown Etiology With the Risk of Developing Dementia in Older Veterans. JAMA Neurol. (2020) 77:710–5. doi: 10.1001/jamaneurol.2020.0187
211. Sherzai D, Losey T, Vega S, Sherzai A. Seizures and dementia in the elderly: Nationwide Inpatient Sample 1999–2008. Epilepsy Behav. (2014) 36:53–6. doi: 10.1016/j.yebeh.2014.04.015
212. Noebels J. A perfect storm: Converging paths of epilepsy and Alzheimer's dementia intersect in the hippocampal formation: Epilepsy and Alzheimer's Disease. Epilepsia. (2011) 52:39–46. doi: 10.1111/j.1528-1167.2010.02909.x
213. Horváth A, Szucs A, Barcs G, Kamondi A. Sleep EEG Detects Epileptiform Activity in Alzheimer's Disease with High Sensitivity. J Alzheimers Dis. (2017) 56:1175–83. doi: 10.3233/JAD-160994
214. Kanner AM. Most antidepressant drugs are safe for patients with epilepsy at therapeutic doses: A review of the evidence. Epilepsy Behav. (2016) 61:282–6. doi: 10.1016/j.yebeh.2016.03.022
215. Wichniak A, Wierzbicka A, Walecka M, Jernajczyk W. Effects of Antidepressants on Sleep. Curr Psychiatry Rep. (2017) 19:63. doi: 10.1007/s11920-017-0816-4
216. Horváth A, Szűcs A, Barcs G, Fabó D, Kelemen A, Halász P, et al. Interictal epileptiform activity in the foramen ovale electrodes of a frontotemporal dementia patient. J Alzheimer's Dis Rep. (2017) 1:89–96. doi: 10.3233/ADR-170020
217. Petit D, Montplaisir J, Lorrain D, Gauthier S. Spectral analysis of the rapid eye movement sleep electroencephalogram in right and left temporal regions: a biological marker of Alzheimer's disease. Ann Neurol. (1992) 32:172–6. doi: 10.1002/ana.410320208
218. Gureviciene I, Ishchenko I, Ziyatdinova S, Jin N, Lipponen A, Gurevicius K, et al. Characterization of Epileptic Spiking Associated With Brain Amyloidosis in APP/PS1 Mice. Front Neurol. (2019) 10:1151. doi: 10.3389/fneur.2019.01151
219. Jin N, Babiloni C, Drinkenburg WH, Hajós M, Nygaard HB, Tanila H, et al. Recommendations for preclinical testing of treatments against alzheimer's disease-related epileptiform spikes in transgenic rodent models. J Alzheimers Dis. (2021) 1–16. doi: 10.3233/JAD-210209
220. Sen A, Capelli V, Husain M. Cognition and dementia in older patients with epilepsy. Brain. (2018) 141:1592–608. doi: 10.1093/brain/awy022
221. Cretin B, Philippi N, Bousiges O, Dibitonto L, Sellal F, Martin-Hunyadi C, et al. Do we know how to diagnose epilepsy early in Alzheimer's disease? Rev Neurol (Paris). (2017) 173:374–80. doi: 10.1016/j.neurol.2017.03.028
222. Cretin B, Sellal F, Philippi N, Bousiges O, Di Bitonto L, Martin-Hunyadi C, et al. Epileptic prodromal Alzheimer's disease, a retrospective study of 13 new cases: expanding the spectrum of Alzheimer's disease to an epileptic variant?. J Alzheimer's Dis. (2016) 52:1125–33. doi: 10.3233/JAD-150096
223. Vossel KA, Tartaglia MC, Nygaard HB, Zeman AZ, Miller BL. Epileptic activity in Alzheimer's disease: causes and clinical relevance. Lancet Neurol. (2017) 16:311–22. doi: 10.1016/S1474-4422(17)30044-3
224. Lam AD, Noebels J. Night Watch on the Titanic: Detecting Early Signs of Epileptogenesis in Alzheimer Disease. Epilepsy Curr. (2020) 20:369–74. doi: 10.1177/1535759720964775
225. Sen A, Romoli M. Pathological brain ageing in epilepsy and dementia: two sides of the same coin? Brain. (2021) 144:9–11. doi: 10.1093/brain/awaa441
226. Gallassi R. Epileptic Amnesic Syndrome: An Update and Further Considerations. Epilepsia. (2006) 47:103–5. doi: 10.1111/j.1528-1167.2006.00704.x
227. Breuer LEM, Boon P, Bergmans JWM, Mess WH, Besseling RMH, de Louw A, et al. Cognitive deterioration in adult epilepsy: Does accelerated cognitive ageing exist? Neurosci Biobehav Rev. (2016) 64:1–11. doi: 10.1016/j.neubiorev.2016.02.004
228. Crous-Bou M, Minguillón C, Gramunt N, Molinuevo JL. Alzheimer's disease prevention: from risk factors to early intervention. Alzheimers Res Ther. (2017) 9:71. doi: 10.1186/s13195-017-0297-z
229. Brookmeyer R, Johnson E, Ziegler-Graham K, Arrighi HM. Forecasting the global burden of Alzheimer's disease. Alzheimers Dement. (2007) 3:186–91. doi: 10.1016/j.jalz.2007.04.381
230. Wimo A, Guerchet M, Ali GC, Wu YT, Prina AM, Winblad B, et al. The worldwide costs of dementia 2015 and comparisons with 2010. Alzheimers Dement. (2017) 13:1–7. doi: 10.1016/j.jalz.2016.07.150
231. Cretin B. Treatment of Seizures in Older Patients with Dementia. Drugs Aging. (2021) 38:181–92. doi: 10.1007/s40266-020-00826-2
232. Koh MT, Haberman RP, Foti S, McCown TJ, Gallagher M. Treatment Strategies Targeting Excess Hippocampal Activity Benefit Aged Rats with Cognitive Impairment. Neuropsychopharmacology. (2010) 35:1016–25. doi: 10.1038/npp.2009.207
233. Cumbo E, Ligori LD. Levetiracetam, lamotrigine, and phenobarbital in patients with epileptic seizures and Alzheimer's disease. Epilepsy Behav. (2010) 17:461–6. doi: 10.1016/j.yebeh.2010.01.015
234. Belcastro V, Costa C, Galletti F, Autuori A, Pierguidi L, Pisani F, et al. Levetiracetam in newly diagnosed late-onset post-stroke seizures: a prospective observational study. Epilepsy Res. (2008) 82:223–6. doi: 10.1111/j.1468-1331.2007.01907.x
235. Musaeus CS, Shafi MM, Santarnecchi E, Herman ST, Press DZ. Levetiracetam Alters Oscillatory Connectivity in Alzheimer's Disease. J Alzheimers Dis JAD. (2017) 58:1065–76. doi: 10.3233/JAD-160742
236. Bakker A, Krauss GL, Albert MS, Speck CL, Jones LR, Stark CE, et al. Reduction of Hippocampal Hyperactivity Improves Cognition in Amnestic Mild Cognitive Impairment. Neuron. (2012) 74:467–74. doi: 10.1016/j.neuron.2012.03.023
237. Bakker A, Albert MS, Krauss G, Speck CL, Gallagher M. Response of the medial temporal lobe network in amnestic mild cognitive impairment to therapeutic intervention assessed by fMRI and memory task performance. NeuroImage Clin. (2015) 7:688–98. doi: 10.1016/j.nicl.2015.02.009
238. Liguori C, Costa C, Franchini F, Izzi F, Spanetta M, Cesarini EN, et al. Cognitive performances in patients affected by late-onset epilepsy with unknown etiology: A 12-month follow-up study. Epilepsy Behav. (2019) 101:106592. doi: 10.1016/j.yebeh.2019.106592
239. Lehmann L, Lo A, Knox KM, Barker-Haliski M. Alzheimer's Disease and Epilepsy: A Perspective on the Opportunities for Overlapping Therapeutic Innovation. Neurochem Res. (2021) 46:1895–912. doi: 10.1007/s11064-021-03332-y
240. Sanchez PE, Zhu L, Verret L, Vossel KA, Orr AG, Cirrito JR, et al. Levetiracetam suppresses neuronal network dysfunction and reverses synaptic and cognitive deficits in an Alzheimer's disease model. Proc Natl Acad Sci. (2012) 109:E2895–903. doi: 10.1073/pnas.1121081109
241. Fu C-H, Iascone DM, Petrof I, Hazra A, Zhang X, Pyfer MS, et al. Early Seizure Activity Accelerates Depletion of Hippocampal Neural Stem Cells and Impairs Spatial Discrimination in an Alzheimer's Disease Model. Cell Rep. (2019) 27:41–3751.e4. doi: 10.1016/j.celrep.2019.05.101
242. Shi JQ, Wang BR, Tian YY, Xu J, Gao L, Zhao SL, et al. Antiepileptics Topiramate and Levetiracetam Alleviate Behavioral Deficits and Reduce Neuropathology in APPswe/PS1dE9 Transgenic Mice. CNS Neurosci Ther. (2013) 19:871–81. doi: 10.1111/cns.12144
Keywords: Alzheimer's disease, epilepsy, memory consolidation, sleep, neuronal hyperexcitability, glymphatic clearance, interictal spike, EEG
Citation: B. Szabo A, Cretin B, Gérard F, Curot J, J. Barbeau E, Pariente J, Dahan L and Valton L (2022) Sleep: The Tip of the Iceberg in the Bidirectional Link Between Alzheimer's Disease and Epilepsy. Front. Neurol. 13:836292. doi: 10.3389/fneur.2022.836292
Received: 15 December 2021; Accepted: 14 February 2022;
Published: 11 April 2022.
Edited by:
Andrea Romigi, Mediterranean Neurological Institute Neuromed (IRCCS), ItalyReviewed by:
Raffaele Manni, Neurological Institute Foundation Casimiro Mondino (IRCCS), ItalyCopyright © 2022 B. Szabo, Cretin, Gérard, Curot, J. Barbeau, Pariente, Dahan and Valton. This is an open-access article distributed under the terms of the Creative Commons Attribution License (CC BY). The use, distribution or reproduction in other forums is permitted, provided the original author(s) and the copyright owner(s) are credited and that the original publication in this journal is cited, in accordance with accepted academic practice. No use, distribution or reproduction is permitted which does not comply with these terms.
*Correspondence: Anna B. Szabo, YW5uYS5zemFib0B1bml2LXRsc2UzLmZy; Luc Valton, dmFsdG9uLmxAY2h1LXRvdWxvdXNlLmZy
†These authors have contributed equally to this work and share last authorship
Disclaimer: All claims expressed in this article are solely those of the authors and do not necessarily represent those of their affiliated organizations, or those of the publisher, the editors and the reviewers. Any product that may be evaluated in this article or claim that may be made by its manufacturer is not guaranteed or endorsed by the publisher.
Research integrity at Frontiers
Learn more about the work of our research integrity team to safeguard the quality of each article we publish.