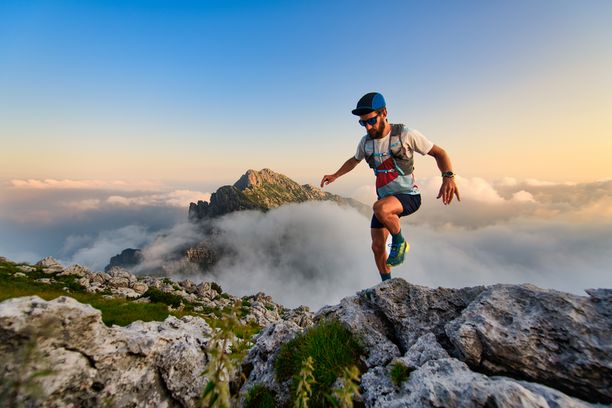
94% of researchers rate our articles as excellent or good
Learn more about the work of our research integrity team to safeguard the quality of each article we publish.
Find out more
REVIEW article
Front. Neurol., 28 February 2022
Sec. Multiple Sclerosis and Neuroimmunology
Volume 13 - 2022 | https://doi.org/10.3389/fneur.2022.828616
Many patients with multiple sclerosis (MS) receive disease-modifying therapies (DMTs), such as teriflunomide, to reduce disease activity and slow progression. DMTs mediate their efficacy by modulating or suppressing the immune system, which might affect a patient's response to vaccination. As vaccines against the SARS-CoV-2 virus become available, questions have arisen around their efficacy and safety for patients with MS who are receiving DMTs. Data are beginning to emerge regarding the potential influence of certain DMTs on a patient's response to coronavirus disease 2019 (COVID-19) vaccines and are supported by evidence from vaccination studies of other pathogens. This review summarizes the available data on the response to vaccines in patients with MS who are receiving DMTs, with a focus on teriflunomide. It also provides an overview of the leading COVID-19 vaccines and current guidance around COVID-19 vaccination for patients with MS. Though few vaccination studies have been done for this patient population, teriflunomide appears to have minimal influence on the response to seasonal influenza vaccine. The evidence for other DMTs (e.g., fingolimod, glatiramer acetate) is less consistent: some studies suggest no effect of DMTs on vaccine response, whereas others show reduced vaccine efficacy. No unexpected safety signals have emerged in any vaccine study. Current guidance for patients with MS is to continue DMTs during COVID-19 vaccination, though adjusted timing of dosing for some DMTs may improve the vaccine response.
Disease-modifying therapies (DMTs) for multiple sclerosis (MS) generally induce immunosuppression or immunomodulation by inhibiting expansion of stimulated lymphocytes (teriflunomide), redirecting pathological immune cells away from the central nervous system (natalizumab, sphingosine-phosphate receptor modulators), or depleting immune cell subsets (B and T cells; ocrelizumab, alemtuzumab, cladribine) (1, 2). These immunosuppressive and immunomodulatory modes of action introduce theoretical potential for increased infection risk, reduced vaccine effectiveness, or reduced duration of immunity (3–5). Whether these risks are real is beginning to be addressed in the 21st century, particularly regarding the seasonal influenza vaccine and certain DMTs such as interferon-beta (IFN-β) and teriflunomide.
The importance of understanding how DMTs might affect vaccination effectiveness and safety for patients with MS is highlighted amid the ongoing outbreak of coronavirus disease 2019 (COVID-19). As of October 2021, minimal data are available regarding potential influence of DMTs on the efficacy of vaccines for the SARS-CoV-2 virus. Most available data are for patients receiving ocrelizumab (6–9). Until substantial data have accumulated, evidence for other immunizations might provide insight into what can be expected with SARS-CoV-2 vaccines (4). This review summarizes currently available evidence on vaccine efficacy for patients receiving DMTs to treat MS, focusing on evidence for the DMT teriflunomide, and summarizes current guidance around SARS-CoV-2 vaccination in this population.
Entry of a pathogen into the body triggers the innate immune response, the first-line immune defense. The innate immune response engages rapidly but is not specifically directed against a particular pathogen. However, it triggers an adaptive immune response, which is pathogen specific and involves stimulation of T and/or B lymphocytes that may ultimately achieve highly focused immune memory against the pathogen (4, 10). For cell-mediated immunity, subsets of T lymphocytes are activated, and memory T cells are generated. For humoral immunity, B lymphocytes are stimulated to produce immunoglobulin M (IgM) and then IgG antibodies against the pathogen. The antibodies and memory cells generated in response to the pathogen generally persist in the body after the pathogen is eliminated, maintaining immunological memory (10).
Several vaccine types have been developed: earlier vaccines used live-attenuated or inactivated pathogens to induce immunity, whereas modern vaccines may be based on toxoids or parts of pathogens, such as viral vectored vaccines and those based on mRNA, DNA, virus-like particles, or recombinant protein (10, 11). Regardless of the type, nearly all vaccines work by introducing antigen, or genes that encode antigen, in the immune system (11, 12). An adjuvant is often included to stimulate the innate immune system to recognize pathogen-associated molecular patterns, but some modern vaccines use these patterns instead of adjuvants. The antigen directs the adaptive immune response against a specific pathogen (11). While both the humoral and cellular responses generated by vaccines can contribute to protection from pathogens, vaccine response is generally measured according to IgG antibody titers raised against the particular antigen, as measuring antibody responses is generally easier than measuring cell-based responses (4, 11).
Two studies have investigated whether teriflunomide affects vaccine response (Table 1). One study evaluated the immune response to the 2011/2012 influenza vaccine (containing H1N1, H3N2, and B strains) in 128 MS patients receiving teriflunomide 7 or 14 mg or IFN-β-1a (13). In that study, ≥90% of patients in all treatment groups achieved seroprotection against the H1N1 and B strains, with seroprotection defined, in accordance with European guidelines for evaluating vaccine response (29), as post-vaccination antibody titers ≥40. For the H3N2 strain, seroprotection rates were also ≥90% with teriflunomide 7 mg and IFN-β-1a, but were 76.9% with teriflunomide 14 mg. The 2011/2012 influenza vaccine included the same strains as were used in the 2010/2011 vaccine, which had been previously administered to 44–70% of study participants. As a result, many patients had H1N1, H3N2, and B strain antibody titers ≥40 at baseline. Of those with pre-vaccination antibody titers <40, >61% of patients in the teriflunomide 7 or 14 mg groups and ≥81% of patients in the IFN-β-1a group achieved seroprotection with the 2011/2012 vaccine, with the highest seroconversion rates observed for the B (teriflunomide 7 mg, 91.7% seroconversion; teriflunomide 14 mg, 91.7%; IFN-β-1a, 85.0%) and H1N1 strains (85.7, 80.0, and 80.0%, respectively) and lower seroconversion rates for the H3N2 strain (77.8, 61.1, and 81.8%, respectively) (13). The results of this study suggest teriflunomide generally does not inhibit a patient's ability to mount an effective immune response to recall antigens with the influenza vaccine.
The second study evaluated the immune response to neoantigen and recall antigens with the human diploid cell rabies vaccine (HDCV) in healthy volunteers who received teriflunomide or placebo for 30 days (14). No participants had measurable antibodies to the rabies virus before vaccination, and all achieved seroprotection against the neoantigen, defined as antibody titers ≥0.5 IU/mL, within 1 week after second vaccination. By days 31 and 38 (2 and 3 weeks after second vaccination), geometric mean antibody titers were significantly lower with teriflunomide (7.8 and 15.2 IU/mL) vs. placebo (12.5 and 28.9 IU/mL), but remained well-above the 0.5 IU/mL threshold for all participants, indicating sustained seroprotection against the rabies neoantigen.
To assess cellular recall (memory) responses, the second study also evaluated delayed-type hypersensitivity (DTH) responses to Candida albicans, Trichophyton, and tuberculin purified protein derivative recall antigens (14). For DTH assessments, positive DTH reaction indicated an appropriate cellular memory response to the recall antigen. In both the teriflunomide and placebo groups, numbers of participants with positive DTH responses to C. albicans and Trichophyton antigens declined from screening to day 30, but proportions of patients with a positive response to tuberculin purified protein derivative antigen were unchanged. Moreover, no differences were observed between treatment groups for any recall antigen, suggesting teriflunomide does not influence the cellular memory response to recall antigens.
Some studies with the seasonal influenza vaccine have linked IFN-β with high rates of seroprotection, similar to those in healthy controls (13, 15, 16, 24–26). In an early study, 93% of patients receiving IFN-β-1a and 91% of patients receiving no DMT achieved seroprotection with the influenza vaccine, and no MS relapses occurred during the month after vaccination (15). More recent studies of patients receiving IFN-β have reported increased frequencies of influenza-specific T cells and antibody titers after vaccination (16) and seroprotection rates comparable to or higher than those of healthy controls (−0.9 to 17.0% difference), with seroprotection maintained up to 12 months after vaccination (25, 26). A higher proportion of patients receiving IFN-β achieved seroprotection against multiple influenza strains compared with patients receiving glatiramer acetate, natalizumab, or mitoxantrone (26). Adverse events of flu-like symptoms, headache, and weakness have been reported after influenza vaccination for patients receiving IFN treatment (24).
IFN-β and dimethyl fumarate were associated with similar rates of protection, defined as ≥2-fold increase in IgG antibody titers, against tetanus antitoxoid (73 and 68%, respectively), diphtheria antitoxoid (61 and 58%), pneumococcal vaccine polyvalent serotypes 3 (79 and 66%) and 8 (88 and 95%), and meningococcal group C (53 and 53%) (17). Severe adverse events of injection-site cellulitis, burning sensation, and arthralgia were reported for two patients in the IFN-β group (n = 33) and none in the dimethyl fumarate group (n = 38) (17).
In one study, patients who received glatiramer acetate achieved similar rates of protection with the influenza vaccine vs. healthy controls (−7.6 to 6.9% difference over 12 months) (25). However, a separate study reported seroprotection rates that were lower (12.9–37.8%) for patients receiving glatiramer acetate than for healthy controls (26). Of note, seroprotection may vary according to influenza strain for patients receiving glatiramer acetate, fingolimod, or natalizumab, with higher rates of protection against strain H1N1 than against strains H3N2 or B (24, 26). Flu-like symptoms, increased temperature, and nightly sweating have been reported after influenza vaccination for patients receiving glatiramer acetate (24).
One small study of fingolimod found no reduction in humoral or cellular response to the influenza vaccine (18), but two other studies reported significantly reduced rates of seroprotection with fingolimod vs. controls (23.2–48.2% difference over 12 months) (19, 25). Exanthema has been reported after influenza vaccination in some patients receiving fingolimod (24).
One study suggested that patients receiving fingolimod might have reduced protection against tetanus toxoid boosters compared with patients receiving placebo (6-week responder rates, 38 vs. 49%, respectively) (19).
Natalizumab appears to diminish the response to seasonal influenza vaccines compared with healthy controls (26) or patients receiving IFN-β (25). Natalizumab might also attenuate response to the tetanus vaccine and keyhole limpet hemocyanin (KLH); although overall response rates were similar between patients taking natalizumab and those who delayed natalizumab treatment, a trend toward lower IgG antibody titers was observed for patients receiving natalizumab (20).
In one study, an attenuated response to influenza vaccination was observed in patients receiving ocrelizumab (55.6–80.0% seroprotection) vs. controls (patients receiving IFN-β therapy or no DMT; 75.0–97.0%) (21). Ocrelizumab was also associated with lower response rates to tetanus (−30.7% difference in 8-week response rate), pneumococcal (−65.3 to −19.1% difference in 8-week response rates), and KLH vaccines (3.0- and 6.1-fold difference in IgM and IgG titers at 8 weeks, respectively) (21). Safety findings with ocrelizumab were consistent with the safety profile from phase 3 studies and most commonly involved mild or moderate infections (47%), which occurred more frequently than in controls (15%) (21).
Alemtuzumab may not interfere with responses to T cell–independent recall antigen (pneumococcus) or T cell–dependent recall antigen (tetanus, diphtheria, polio) and neoantigen (meningococcus C), although vaccination within 6 months of treatment resulted in a smaller proportion of responders (22). However, the results should be interpreted with caution, as they are based on a single study that compared a small population (N = 24) with literature controls.
Like many pathogens, SARS-CoV-2 initially infects mucosal surfaces of the upper respiratory and digestive tracts (30). Mucosal surfaces use adaptive immune mechanisms for protection that involve secretory IgA and differ from those of other epithelial layers or at a systemic level (11). The mucosal immune response is non-inflammatory, but an inflammatory immune response dominates once a pathogen reaches terminal airways and alveoli (30).
To enter target cells, the SARS-CoV-2 virus uses a spike glycoprotein that binds the ACE2 receptor (31). Because the spike protein is essential for entry into the target cell, all vaccines use this protein as the immunizing antigen (32). The intent is to present the spike protein to the immune system, generate neutralizing antibodies that prevent it binding the ACE2 receptor, and thereby limit viral entry and infection of host cells (31, 32). As of November 2021, the COVID-19 vaccine developed by Pfizer-BioNTech has received full approval from the US FDA for use in adults (≥16 years) and is authorized for emergency use in children (5–15 years); the Moderna and Johnson & Johnson/Janssen are authorized for emergency use by the US FDA in adults (≥18 years). These vaccines are recommended by the CDC to prevent SARS-CoV-2 infection and COVID-related complications (Table 2).1 Additional vaccines from AstraZeneca, Pfizer-BioNTech, Novavax, Inovio, and Medicago are also in Phase 3 development in the US (Table 2). This short list comprises a variety of vaccine types: both vaccines from Pfizer-BioNTech (BNT162b1 and BNT162b2) and the vaccine from Moderna (mRNA-1273) are nucleic acid (mRNA) based (33, 34); the Inovio vaccine is DNA based2; the Janssen and AstraZeneca vaccines are viral vector vaccines, which consists of inactivated adenovirus (35, 36); the Novavax vaccine is protein based and contains spike glycoproteins (37); and the Medicago vaccine uses a plant-based coronavirus-like particle.3 In other countries, a recombinant adenovirus–based vaccine developed by Russian researchers and inactivated SARS-CoV-2 vaccines developed by Sinovac and Sinopharm are demonstrating favorable efficacy against SARS-CoV-2 infection, but none is in Phase 3 development in the US (38–40). None of the vaccines currently available or in Phase 3 development in the US contain replication-competent virus. Only one vaccine, from Meissa Vaccines, uses live attenuated virus and is in phase 1 clinical trials in the US.
Table 2. COVID-19 vaccines authorized for emergency use† or in phase 3 development in the US as of October 20, 2021.
Though guidance has been issued for patients with MS who are considering vaccination against the SARS-CoV-2 virus, the information is based largely on studies using other vaccines, most of which used inactivated or toxoid- or polysaccharide-based vaccines (4, 11). Initial data are emerging on the interaction between DMTs and the development of SARS-CoV-2 antibodies. A single-center study (N = 555) that included untreated patients and patients receiving DMTs suggested the rates of adverse events, reported a median 38 days after the first BNT162b2 dose and 20 days after the second dose, were similar to those in the general population: 29.7% after first dose, and 40.2% after second dose (6). Of the 125 patients from that study for whom seroprotection was assessed 1 month after the second dose, humoral immunity was attained for 22.7% of patients receiving ocrelizumab, 3.8% receiving fingolimod, 100% receiving cladribine, and 100% of untreated patients (7). A separate study also reported reduced odds of antibody formation after SARS-CoV-2 infection with ocrelizumab vs. other DMTs (odds ratio, 0.045; P = 0.011) (41). A multicenter cohort study of patients with MS reported that compared with patients receiving no DMT, post-vaccination antibody levels 1 month after the second dose of the BNT162b2 or mRNA-1273 vaccine were significantly (P < 0.001) lower in patients receiving ocrelizumab (201-fold reduction), fingolimod (26-fold), and rituximab (20-fold) compared with untreated patients (27). Patients receiving teriflunomide had similar antibody levels to untreated patients. Across cohorts, antibody levels were 3.5-fold higher for patients who received the mRNA-1273 vs. BNT162b2 vaccine. Similar findings were reported in a Swiss cohort of patients with MS who received the BNT162b2 or mRNA-1273 vaccine (28). Compared with patients who received no MS therapy, SARS-CoV-2 IgG titers 21–35 days after the second dose were lower in patients receiving anti-CD20 antibodies or sphingosine-1-phosphate modulators; no significant difference was found with teriflunomide or cladribine. Of note, although patients with MS who are treated with an anti-CD20 antibody have poor antibody responses to SARS-CoV-2 vaccination, these patients appear to have a robust cellular response by 1 month after the second vaccine dose, which suggests that some level of immunity is attained (42). A robust cellular response to SARS-CoV-2 vaccination was also recently observed in the majority of patients on teriflunomide and most other DMTs except sphingosine-1-phosphate modulators (43, 44).
Based on accumulated evidence for vaccination in the context of MS DMTs, an international consensus panel, as well as the US National MS Society (NMSS) and UK MS Society (MSS), consider the potential benefit of COVID-19 vaccination to outweigh the risk of avoiding vaccination, with regard to both individual and public safety (32).4,5 All recommendations suggest the vaccines are safe for patients with MS, including those receiving DMTs, and are unlikely to cause MS relapse, worsen MS symptoms, or reduce DMT efficacy. The NMSS emphasizes the importance of vaccination for high-risk populations with MS. These include black and Hispanic patients and patients with progressive MS, who are older, or who have greater physical disability or medical conditions that might increase the severity of COVID-19 symptoms.
According to these guidance statements, as of September 2021, patients with MS do not need to postpone initiating most DMTs or adjust the timing of administration. Whereas, the consensus statement from Centonze et al. recommends not discontinuing or modifying DMTs to improve vaccine efficacy (32), the NMSS and MSS note exceptions for certain DMTs. In the NMSS guidance, exceptions include patients initiating treatment with fingolimod, siponimod, ozanimod, alemtuzumab, cladribine, ocrelizumab, rituximab, and ofatumumab. Ideally these DMTs would not be initiated until 2–4 weeks after the second vaccine injection. The MSS guidance similarly recommends patients wait until after vaccination before initiating treatment with ocrelizumab or rituximab. For patients already receiving alemtuzumab, ocrelizumab, rituximab, and ofatumumab, the NMSS suggests the first injection of COVID-19 vaccine be administered ≥12 weeks (≥4 weeks for ofatumumab) after the last DMT dose, with the DMT resumed ≥4 weeks after the second injection. The MSS echoes this guidance for patients already receiving alemtuzumab and cladribine. In addition, patients receiving high-dose steroids should wait at least 3–5 days after the last steroid dose before receiving a vaccine injection.
Neither society has provided guidance around COVID-19 vaccination for patients experiencing MS relapse or exacerbation. However, AAN guidelines recommend such patients postpone any vaccinations until their relapse is no longer active (45).
During the first year of the COVID-19 pandemic, several prescribers and their patients with MS questioned whether DMTs might increase the risk for infection, and potentially lead to severe COVID-19 symptoms (46). Early evidence on patients receiving MS DMTs at the time of a COVID-19 diagnosis suggests that despite their immunomodulatory or immunosuppressive mechanisms of action, most DMTs, including teriflunomide, do not increase the risk of more severe COVID-19 symptoms (3). Among the available literature, most notable are a systematic review of 2,493 patients with MS who were infected with SARS-CoV-2, of whom 2,047 were receiving DMTs (47), and a description of 873 patients with MS and SARS-CoV-2 infection, 761 of whom were receiving DMTs (48). Both publications suggest most DMTs do not increase the severity of COVID-19 symptoms or contribute to poorer outcomes; the risk of serious outcomes may be higher for untreated patients or those receiving anti-CD20 therapies (47). It is possible that some DMTs might attenuate the immune response to the SARS-CoV-2 virus in patients with MS. For example, some investigators have hypothesized that the strong antigenic stimulation often caused by SARS-CoV-2 may be moderated by teriflunomide, which reduces the level of immune activation without inducing major immunosuppression (49–51). Others have proposed that teriflunomide might harbor antiviral properties that could reduce the ability of the virus to replicate in the infected cell (47, 52). Because limited evidence is available to date, it is not yet possible to draw conclusions about the effects of teriflunomide or any other DMT on the course of SARS-CoV-2 infection. However, the NMSS suggests the benefits of continuing MS treatments outweigh the potential risks of stopping treatment.6
In patients with MS, use of DMTs may complicate the evidence around vaccinations due to their immunomodulatory and immunosuppressive effects (4). Initial evidence suggests the6 possibility that some DMTs might reduce efficacy of vaccines, including vaccines for the SARS-CoV-2 virus (7, 27). The available evidence is encouraging, as it suggests that compared with healthy controls, patients with MS receiving most DMTs, including teriflunomide, do not have reduced response to vaccines or experience adverse effects from vaccination.
CT, HW, AL, SG, JC, PT, and AB-O provided substantial contributions to the interpretation of data for the work, provided critical revision of drafts, approved the manuscript for submission, and agreed to be accountable for all aspects of the work. All authors contributed to the article and approved the submitted version.
Editorial support was funded by Sanofi.
CT reports receiving research support and honoraria for speaking or acting as a member of an advisory board or a Data and Safety Monitoring Board for Atara, Biogen, Celgene, Genentech, Sanofi Genzyme, and TG Therapeutics. HW reports receiving honoraria for acting as a member of scientific advisory boards for Biogen, Genzyme, Merck Serono, Novartis, Roche Pharma AG, Sanofi-Aventis, and UCB; speaker honoraria and travel support from Alexion, Biogen, Biologix, Cognomed, F. Hoffmann-La Roche Ltd., Gemeinnützige Hertie-Stiftung, Merck, Novartis, Roche Pharma AG, Sanofi Genzyme, TEVA, and WebMD Global; consulting fees from Actelion, Argenx, Biogen, Bristol Myers Squibb, EMD Serono, Idorsia, IGES, Immunic, Immunovant, Janssen, Johnson & Johnson, Novartis, Roche, Sanofi, the Swiss Multiple Sclerosis Society, and UCB; and research funding from the German Ministry for Education and Research (BMBF), Deutsche Forschungsgemeinschaft (DFG), Else Kröner Fresenius Foundation, Fresenius Foundation, the European Union, Hertie Foundation, NRW Ministry of Education and Research, Interdisciplinary Center for Clinical Studies (IZKF) Muenster and Biogen, GlaxoSmithKline, Roche Pharma AG, and Sanofi Genzyme. AL, SG, JC, and PT report receiving compensation as employees of Sanofi, and may hold shares and/or stock options in the company. AB-O reports receiving consulting fees from Accure, Atara Biotherapeutics, Biogen, BMS/Celgene/Receptos, GlaxoSmithKline, Gossamer, Janssen/Actelion, Medimmune, Merck/EMD Serono, Novartis, Roche/Genentech, and Sanofi Genzyme; and has received grant support to the University of Pennsylvania from Biogen Idec, Roche/Genentech, Merck/EMD Serono and Novartis.
All claims expressed in this article are solely those of the authors and do not necessarily represent those of their affiliated organizations, or those of the publisher, the editors and the reviewers. Any product that may be evaluated in this article, or claim that may be made by its manufacturer, is not guaranteed or endorsed by the publisher.
Writing assistance (assistance in drafting and editing of the manuscript text, figures, and tables, as directed by the author, data checking and incorporation of comments from reviewers, and assisting with the submission process) was provided by Panos Xenopoulos, PhD, and Autumn Kelly, MA, of Elevate Scientific Solutions.
COVID-19, coronavirus disease 2019; DMT, disease-modifying therapy; DTH, delayed-type hypersensitivity; IFN-β, interferon-beta; Ig, immunoglobulin; KLH, keyhole limpet hemocyanin; MS, multiple sclerosis; MSS, MS Society; NMSS, National MS Society.
1. ^https://www.cdc.gov/coronavirus/2019-ncov/vaccines/different-vaccines.html.
2. ^https://www.inovio.com/our-focus-serving-patients/covid-19/.
3. ^https://www.medicago.com/en/covid-19-programs/.
4. ^https://www.mssociety.org.uk/what-we-do/news/ms-society-medical-advisers-release-consensus-statement-covid-19-vaccines.
5. ^https://www.nationalmssociety.org/coronavirus-covid-19-information/multiple-sclerosis-and-coronavirus/covid-19-vaccine-guidance.
6. ^https://www.nationalmssociety.org/coronavirus-covid-19-information/multiple-sclerosis-and-coronavirus/ms-treatment-guidelines-during-coronavirus.
1. Jakimovski D, Weinstock-Guttman B, Ramanathan M, Dwyer MG, Zivadinov R. Infections, vaccines and autoimmunity: a multiple sclerosis perspective. Vaccines. (2020) 8:50. doi: 10.3390/vaccines8010050
2. McGinley MP, Goldschmidt CH, Rae-Grant AD. Diagnosis and treatment of multiple sclerosis: a review. JAMA. (2021) 325:765–79. eng. doi: 10.1001/jama.2020.26858
3. Zheng C, Kar I, Chen CK, Sau C, Woodson S, Serra A, et al. Multiple sclerosis disease-modifying therapy and the COVID-19 pandemic: implications on the risk of infection and future vaccination. CNS Drugs. (2020) 34:879–96. doi: 10.1007/s40263-020-00756-y
4. Ciotti JR, Valtcheva MV, Cross AH. Effects of MS disease-modifying therapies on responses to vaccinations: a review. Mult Scler Relat Disord. (2020) 45:102439. doi: 10.1016/j.msard.2020.102439
5. Williamson EM, Chahin S, Berger JR. Vaccines in multiple sclerosis. Curr Neurol Neurosci Rep. (2016) 16:36. doi: 10.1007/s11910-016-0637-6
6. Achiron A, Dolev M, Menascu S, Zohar DN, Dreyer-Alster S, Miron S, et al. COVID-19 vaccination in patients with multiple sclerosis: what we have learnt by February 2021. Mult Scler. (2021) 27:864–70. doi: 10.1177/13524585211003476
7. Achiron A, Mandel M, Dreyer-Alster S, Harari G, Magalashvili D, Sonis P, et al. Humoral immune response to COVID-19 mRNA vaccine in patients with multiple sclerosis treated with high-efficacy disease-modifying therapies. Ther Adv Neurol Disord. (2021) 14:17562864211012835. doi: 10.1177/17562864211012835
8. Buttari F, Bruno A, Dolcetti E, Azzolini F, Bellantonio P, Centonze D, et al. COVID-19 vaccines in multiple sclerosis treated with cladribine or ocrelizumab. Mult Scler Relat Disord. (2021) 52:102983. doi: 10.1016/j.msard.2021.102983
9. Gallo A, Capuano R, Donnarumma G, Bisecco A, Grimaldi E, Conte M, et al. Preliminary evidence of blunted humoral response to SARS-CoV-2 mRNA vaccine in multiple sclerosis patients treated with ocrelizumab. Neurol Sci. (2021) 42:3523–6. doi: 10.1016/j.jns.2021.117795
10. Vetter V, Denizer G, Friedland LR, Krishnan J, Shapiro M. Understanding modern-day vaccines: what you need to know. Ann Med. (2018) 50:110–20. doi: 10.1080/07853890.2017.1407035
11. Iwasaki A, Omer SB. Why and how vaccines work. Cell. (2020) 183:290–5. doi: 10.1016/j.cell.2020.09.040
12. Mendonca SA, Lorincz R, Boucher P, Curiel DT. Adenoviral vector vaccine platforms in the SARS-CoV-2 pandemic. NPJ Vaccines. (2021) 6:97. doi: 10.1038/s41541-021-00356-x
13. Bar-Or A, Freedman MS, Kremenchutzky M, Menguy-Vacheron F, Bauer D, Jodl S, et al. Teriflunomide effect on immune response to influenza vaccine in patients with multiple sclerosis. Neurology. (2013) 81:552–8. doi: 10.1212/WNL.0b013e31829e6fbf
14. Bar-Or A, Wiendl H, Miller B, Benamor M, Truffinet P, Church M, et al. Randomized study of teriflunomide effects on immune responses to neoantigen and recall antigens. Neurol Neuroimmunol Neuroinflamm. (2015) 2:e70. doi: 10.1212/NXI.0000000000000070
15. Schwid SR Decker MD Lopez-Bresnahan M Rebif-Influenza Vaccine Study I. Immune response to influenza vaccine is maintained in patients with multiple sclerosis receiving interferon beta-1a. Neurology. (2005) 65:1964–6. doi: 10.1212/01.wnl.0000188901.12700.e0
16. Mehling M, Fritz S, Hafner P, Eichin D, Yonekawa T, Klimkait T, et al. Preserved antigen-specific immune response in patients with multiple sclerosis responding to IFNbeta-therapy. PLoS ONE. (2013) 8:e78532. doi: 10.1371/journal.pone.0078532
17. von Hehn C, Howard J, Liu S, Meka V, Pultz J, Mehta D, et al. Immune response to vaccines is maintained in patients treated with dimethyl fumarate. Neurol Neuroimmunol Neuroinflamm. (2018) 5:e409. doi: 10.1212/NXI.0000000000000409
18. Mehling M, Hilbert P, Fritz S, Durovic B, Eichin D, Gasser O, et al. Antigen-specific adaptive immune responses in fingolimod-treated multiple sclerosis patients. Ann Neurol. (2011) 69:408–13. doi: 10.1002/ana.22352
19. Kappos L, Mehling M, Arroyo R, Izquierdo G, Selmaj K, Curovic-Perisic V, et al. Randomized trial of vaccination in fingolimod-treated patients with multiple sclerosis. Neurology. (2015) 84:872–9. doi: 10.1212/WNL.0000000000001302
20. Kaufman M, Pardo G, Rossman H, Sweetser MT, Forrestal F, Duda P. Natalizumab treatment shows no clinically meaningful effects on immunization responses in patients with relapsing-remitting multiple sclerosis. J Neurol Sci. (2014) 341:22–7. doi: 10.1016/j.jns.2014.03.035
21. Bar-Or A, Calkwood JC, Chognot C, Evershed J, Fox EJ, Herman A, et al. Effect of ocrelizumab on vaccine responses in patients with multiple sclerosis: the VELOCE study. Neurology. (2020) 95:e1999–2008. doi: 10.1212/WNL.0000000000010380
22. McCarthy CL, Tuohy O, Compston DA, Kumararatne DS, Coles AJ, Jones JL. Immune competence after alemtuzumab treatment of multiple sclerosis. Neurology. (2013) 81:872–6. doi: 10.1212/WNL.0b013e3182a35215
23. Mehta L, Umans K, Ozen G, Robinson RR, Elkins J. Immune response to seasonal influenza vaccine in patients with relapsing-remitting multiple sclerosis receiving long-term daclizumab beta: a prospective, open-label, single-arm study. Int J MS Care. (2017) 19:141–7. doi: 10.7224/1537-2073.2016-026
24. Metze C, Winkelmann A, Loebermann M, Hecker M, Schweiger B, Reisinger EC, et al. Immunogenicity and predictors of response to a single dose trivalent seasonal influenza vaccine in multiple sclerosis patients receiving disease-modifying therapies. CNS Neurosci Ther. (2019) 25:245–54. doi: 10.1111/cns.13034
25. Olberg HK, Eide GE, Cox RJ, Jul-Larsen A, Lartey SL, Vedeler CA, et al. Antibody response to seasonal influenza vaccination in patients with multiple sclerosis receiving immunomodulatory therapy. Eur J Neurol. (2018) 25:527–34. doi: 10.1111/ene.13537
26. Olberg HK, Cox RJ, Nostbakken JK, Aarseth JH, Vedeler CA, Myhr KM. Immunotherapies influence the influenza vaccination response in multiple sclerosis patients: an explorative study. Mult Scler. (2014) 20:1074–80. doi: 10.1177/1352458513513970
27. Sormani MP, Inglese M, Schiavetti I, Carmisciano L, Laroni A, Lapucci C, et al. Effect of SARS-CoV-2 mRNA vaccination in MS patients treated with disease modifying therapies. EBioMedicine. (2021) 72:103581. doi: 10.2139/ssrn.3886420
28. Disanto G, Sacco R, Bernasconi E, Martinetti G, Keller F, Gobbi C, et al. Association of disease-modifying treatment and anti-CD20 infusion timing with humoral response to 2 SARS-CoV-2 vaccines in patients with multiple sclerosis. JAMA Neurol. (2021) 78:1529–31. doi: 10.1001/jamaneurol.2021.3609
29. European Agency for the Evaluation of Medicinal Products. Committee for Proprietary Medicinal Products: Note for Guidance on Harmonization of Requirements for Influenza Vaccines. London: European Agency for the Evaluation of Medicinal Products (1997).
30. Russell MW, Moldoveanu Z, Ogra PL, Mestecky J. Mucosal immunity in COVID-19: a neglected but critical aspect of SARS-CoV-2 infection. Front Immunol. (2020) 11:611337. doi: 10.3389/fimmu.2020.611337
31. Lan J, Ge J, Yu J, Shan S, Zhou H, Fan S, et al. Structure of the SARS-CoV-2 spike receptor-binding domain bound to the ACE2 receptor. Nature. (2020) 581:215–20. doi: 10.1038/s41586-020-2180-5
32. Centonze D, Rocca MA, Gasperini C, Kappos L, Hartung HP, Magyari M, et al. Disease-modifying therapies and SARS-CoV-2 vaccination in multiple sclerosis: an expert consensus. J Neurol. (2021) 268:3961–8. doi: 10.1007/s00415-021-10545-2
33. Fact Sheet for Healthcare Providers Administering Vaccine (Vaccination Providers): Emergency Use Authorization (EUA) of the Pfizer-BioNTech COVID-19 Vaccine to Prevent Coronavirus Disease 2019 (COVID-19). New York, NY: Pfizer Inc. (2021).
34. Fact Sheet for Healthcare Providers Administering Vaccine (Vaccination Providers): Emergency Use Authorization (EUA) of the Moderna COVID-19 Vaccine to Prevent Coronavirus Disease 2019 (COVID-19). Cambridge, MA: Moderna TX Inc. (2021).
35. Fact Sheet for Healthcare Providers Administering Vaccine (Vaccination Providers): Emergency Use Authorization (EUA) of the Janssen COVID-19 Vaccine to Prevent Coronavirus Disease 2019 (COVID-19). Horsham, PA: Janssen Pharmaceutical Company of Johnson & Johnson (2021).
36. Voysey M, Costa Clemens SA, Madhi SA, Weckx LY, Folegatti PM, Aley PK, et al. Single-dose administration and the influence of the timing of the booster dose on immunogenicity and efficacy of ChAdOx1 nCoV-19 (AZD1222) vaccine: a pooled analysis of four randomised trials. Lancet. (2021) 397:881–91. doi: 10.1016/S0140-6736(21)00432-3
37. Keech C, Albert G, Cho I, Robertson A, Reed P, Neal S, et al. Phase 1-2 trial of a SARS-CoV-2 recombinant spike protein nanoparticle vaccine. N Engl J Med. (2020) 383:2320–32. doi: 10.1056/NEJMoa2026920
38. Logunov DY, Dolzhikova IV, Shcheblyakov DV, Tukhvatulin AI, Zubkova OV, Dzharullaeva AS, et al. Safety and efficacy of an rAd26 and rAd5 vector-based heterologous prime-boost COVID-19 vaccine: an interim analysis of a randomised controlled phase 3 trial in Russia. Lancet. (2021) 397:671–81. doi: 10.1016/S0140-6736(21)00234-8
39. Wu Z, Hu Y, Xu M, Chen Z, Yang W, Jiang Z, et al. Safety, tolerability, and immunogenicity of an inactivated SARS-CoV-2 vaccine (CoronaVac) in healthy adults aged 60 years and older: a randomised, double-blind, placebo-controlled, phase 1/2 clinical trial. Lancet Infect Dis. (2021) 21:803–12. doi: 10.1016/S1473-3099(20)30987-7
40. SAGE Working Group on COVID-19 Vaccines. Evidence assessment: Sinopharm/BBIBP COVID-19 Vaccine. World Health Organization (2021).
41. Conte WL. Attenuation of antibody response to SARS-CoV-2 infection in patients with multiple sclerosis on ocrelizumab: a case-control study. Mult Scler Relat Disord. (2021) 52:103014. doi: 10.1016/j.msard.2021.103014
42. Apostolidis SA, Kakara M, Painter MM, Goel RR, Mathew D, Lenzi K, et al. Cellular and humoral immune responses following SARS-CoV-2 mRNA vaccination in patients with multiple sclerosis on anti-CD20 therapy. Nat Med. (2021) 27:1990–2001. doi: 10.1101/2021.06.23.21259389
43. Zabalza A, Arrambide G, Otero-Romero S, Pappolla A, Tagliana P, López-Maza S, et al. Humoral and cellular responses to SARS-CoV-2 vaccination in patients with multiple sclerosis and other autoimmune diseases. Mult Scler. (2021) 27(2_Suppl.):P945.
44. Tortorella C, Aiello A, Gasperini C, Agrati C, Castilletti C, Ruggieri S, et al. Humoral- and T-cell–specific immune responses to SARS-CoV-2 mRNA vaccination in patients with MS using different disease-modifying therapies. Neurology. (2021). doi: 10.1212/WNL.0000000000013108. [Epub ahead of print].
45. Farez MF, Correale J, Armstrong MJ, Rae-Grant A, Gloss D, Donley D, et al. Practice guideline update summary: Vaccine-preventable infections and immunization in multiple sclerosis: report of the Guideline Development, Dissemination, and Implementation Subcommittee of the American Academy of Neurology. Neurology. (2019) 93:584–94. doi: 10.1212/WNL.0000000000008157
46. Berger JR, Brandstadter R, Bar-Or A. COVID-19 and MS disease-modifying therapies. Neurol Neuroimmunol Neuroinflamm. (2020) 7:761. doi: 10.1212/NXI.0000000000000761
47. Sharifian-Dorche M, Sahraian MA, Fadda G, Osherov M, Sharifian-Dorche A, Karaminia M, et al. COVID-19 and disease-modifying therapies in patients with demyelinating diseases of the central nervous system: a systematic review. Mult Scler Relat Disord. (2021) 50:102800. doi: 10.1016/j.msard.2021.102800
48. Mohn N, Konen FF, Pul R, Kleinschnitz C, Pruss H, Witte T, et al. Experience in multiple sclerosis patients with COVID-19 and disease-modifying therapies: a review of 873 published cases. J Clin Med. (2020) 9:4067. doi: 10.3390/jcm9124067
49. Bar-Or A, Pachner A, Menguy-Vacheron F, Kaplan J, Wiendl H. Teriflunomide and its mechanism of action in multiple sclerosis. Drugs. (2014) 74:659–74. doi: 10.1007/s40265-014-0212-x
50. Bollo L, Guerra T, Bavaro DF, Monno L, Saracino A, Angarano G, et al. Seroconversion and indolent course of COVID-19 in patients with multiple sclerosis treated with fingolimod and teriflunomide. J Neurol Sci. (2020) 416:117011. doi: 10.1016/j.jns.2020.117011
51. Ciardi MR, Zingaropoli MA, Pasculli P, Perri V, Tartaglia M, Valeri S, et al. The peripheral blood immune cell profile in a teriflunomide-treated multiple sclerosis patient with COVID-19 pneumonia. J Neuroimmunol. (2020) 346:577323. doi: 10.1016/j.jneuroim.2020.577323
Keywords: COVID-19, disease-modifying therapies (DMTs), multiple sclerosis, teriflunomide (Aubagio), vaccination
Citation: Tornatore C, Wiendl H, Lublin AL, Geertsen SS, Chavin J, Truffinet P and Bar-Or A (2022) Vaccine Response in Patients With Multiple Sclerosis Receiving Teriflunomide. Front. Neurol. 13:828616. doi: 10.3389/fneur.2022.828616
Received: 03 December 2021; Accepted: 17 January 2022;
Published: 28 February 2022.
Edited by:
Edgar Carnero Contentti, Hospital Aleman, ArgentinaReviewed by:
Jorge Correale, Fundación Para la Lucha Contra las Enfermedades Neurológicas de la Infancia (FLENI), ArgentinaCopyright © 2022 Tornatore, Wiendl, Lublin, Geertsen, Chavin, Truffinet and Bar-Or. This is an open-access article distributed under the terms of the Creative Commons Attribution License (CC BY). The use, distribution or reproduction in other forums is permitted, provided the original author(s) and the copyright owner(s) are credited and that the original publication in this journal is cited, in accordance with accepted academic practice. No use, distribution or reproduction is permitted which does not comply with these terms.
*Correspondence: Amit Bar-Or, YW1pdGJhckBwZW5ubWVkaWNpbmUudXBlbm4uZWR1
Disclaimer: All claims expressed in this article are solely those of the authors and do not necessarily represent those of their affiliated organizations, or those of the publisher, the editors and the reviewers. Any product that may be evaluated in this article or claim that may be made by its manufacturer is not guaranteed or endorsed by the publisher.
Research integrity at Frontiers
Learn more about the work of our research integrity team to safeguard the quality of each article we publish.