- 1Department of Neurosurgery, Carl von Ossietzky University Oldenburg, Oldenburg, Germany
- 2Research Center Neurosensory Science, Carl von Ossietzky University Oldenburg, Oldenburg, Germany
- 3Center for Stroke Research Berlin, Charité-Universitätsmedizin Berlin, Corporate Member of Freie Universität Berlin, Humboldt-Universität zu Berlin, Berlin Institute of Health, Berlin, Germany
- 4Department of Neurology, Charité-Universitätsmedizin Berlin, Corporate Member of Freie Universität Berlin, Humboldt-Universität zu Berlin, and Berlin Institute of Health, Berlin, Germany
- 5Department of Experimental Neurology, Charité-Universitätsmedizin Berlin, Corporate Member of Freie Universität Berlin, Humboldt-Universität zu Berlin, Berlin Institute of Health, Berlin, Germany
- 6Department of Anaesthesiology and Intensive Care Medicine, Carl von Ossietzky University Oldenburg, Oldenburg, Germany
- 7Bernstein Center for Computational Neuroscience Berlin, Berlin, Germany
- 8Einstein Center for Neurosciences Berlin, Berlin, Germany
- 9Department of Neurosurgery, Charité-Universitätsmedizin Berlin, Corporate Member of Freie Universität Berlin, Humboldt-Universität zu Berlin, Berlin Institute of Health, Berlin, Germany
Introduction: Wyler-strip electrodes for subdural electrocorticography (ECoG) are the gold standard for continuous bed-side monitoring of pathological cortical network events, such as spreading depolarizations (SD) and electrographic seizures. Recently, SD associated parameters were shown to be (1) a marker of early brain damage after aneurysmal subarachnoid hemorrhage (aSAH), (2) the strongest real-time predictor of delayed cerebral ischemia currently known, and (3) the second strongest predictor of patient outcome at 7 months. The strongest predictor of patient outcome at 7 months was focal brain damage segmented on neuroimaging 2 weeks after the initial hemorrhage, whereas the initial focal brain damage was inferior to the SD variables as a predictor for patient outcome. However, the implantation of Wyler-strip electrodes typically requires either a craniotomy or an enlarged burr hole. Neuromonitoring via an enlarged burr hole has been performed in only about 10% of the total patients monitored.
Methods: In the present pilot study, we investigated the feasibility of ECoG monitoring via a less invasive burrhole approach using a Spencer-type electrode array, which was implanted subdurally rather than in the depth of the parenchyma. Seven aSAH patients requiring extraventricular drainage (EVD) were included. For electrode placement, the burr hole over which the EVD was simultaneously placed, was used in all cases. After electrode implantation, continuous, direct current (DC)/alternating current (AC)-ECoG monitoring was performed at bedside in our Neurointensive Care unit. ECoGs were analyzed following the recommendations of the Co-Operative Studies on Brain Injury Depolarizations (COSBID).
Results: Subdural Spencer-type electrode arrays permitted high-quality ECoG recording. During a cumulative monitoring period of 1,194.5 hours and a median monitoring period of 201.3 (interquartile range: 126.1–209.4) hours per patient, 84 SDs were identified. Numbers of SDs, isoelectric SDs and clustered SDs per recording day, and peak total SD-induced depression duration of a recording day were not significantly different from the previously reported results of the prospective, observational, multicenter, cohort, diagnostic phase III trial, DISCHARGE-1. No adverse events related to electrode implantation were noted.
Discussion: In conclusion, our findings support the safety and feasibility of less-invasive subdural electrode implantation for reliable SD-monitoring.
1. Introduction
In recent years, multimodal neuromonitoring has become an important technique in neurocritical care, because immediate online detection of clinical deterioration plays a key role in the prevention of secondary neurological injury (1). This is particularly relevant in neurosurgical patients at risk of delayed cerebral ischemia after aneurysmal subarachnoid hemorrhage (aSAH) since the time-period between the onset of an insult and manifestation of permanent damage in the brain is brief and the consequences of permanent injury are devastating (2).
The dilemma especially in unconscious patients is that the integrity of brain structure and function are less accessible to point-of-care diagnostics (3, 4). While signs of secondary injury can be reliably detected via repeated neurological examinations in fully conscious patients, the assessment of secondary neurological injury in patients with reduced consciousness is very limited (4, 5). As a result, treatment is often nonspecific and does not occur at the proper time of injury development, even if appropriate interventions were available. In particular, there is a risk that patients are overtreated, which can worsen the outcome as much as undertreatment. It is also extremely difficult to conduct proof-of-concept treatment trials in this situation. So, it is not surprising that none of the widely used so-called rescue therapies of delayed cerebral ischemia after aSAH have been proven efficacious in randomized trials. Accordingly, their use varies greatly between countries and centers and they might be ineffective or even detrimental (6).
In fact, despite numerous randomized trials, prophylaxis with oral nimodipine remains the only pharmacological therapy that has been shown to reduce the risk of delayed cerebral ischemia and adverse outcome after aSAH (6). Nimodipine prevents one of three poor outcomes due to delayed cerebral ischemia (7), although it has no measurable effect on angiographic vasospasm (8–10). On the other hand, nearly all patients in the recent prospective, observational, multicenter, cohort, diagnostic phase III trial of severe aSAH, DISCHARGE-1, received nimodipine. Nevertheless, 90 of 170 (53%) patients in this MRI-based study developed delayed infarcts despite nimodipine prophylaxis (11). The early focal brain damage volume due to intracerebral hemorrhage and early infarcts was 46 ± 73 ml (56%) and the focal brain damage volume due to delayed cerebral ischemia was 36 ± 80 ml (44%). Nimodipine may be helpful after aSAH via its microvascular effects (12), but it by no means sufficiently addresses the clinical problem of delayed cerebral ischemia at present.
To achieve real-time detection of newly developing injury, multimodal monitoring comprises different parameters, of which electrocorticography (ECoG) has recently gained increased attention. Importantly, just as diffusion-weighted MRI, two-photon microscopy or serial section electron microscopy allow visualization of neuronal cytotoxic edema, ECoG in the direct current (DC) frequency range also allows visualization of neuronal cytotoxic edema (13–15). The only difference is that neuronal cytotoxic edema is not referred to as neuronal cytotoxic edema in electrocorticographic language but as spreading depolarization (SD), for historical rather than contextual reasons (14). In addition, SD causes spreading depression of the spontaneous brain activity. Unlike SD, spreading depression is not observed in the DC frequency range but in the alternating current (AC)-ECoG frequency range, where it is visible as a rapidly evolving reduction in the amplitudes of spontaneous activity that spreads between adjacent recording sites along with SD (16). Spreading depression results from the depolarization block that SD produces, but usually lasts longer than SD, suggesting that spreading depression is maintained at a later stage by other mechanisms such as the release of adenosine (17).
It has been suggested that SD variables may serve as a real-time mechanistic biomarker for impending parenchyma damage after aSAH (18–20). To this end, the numbers of SDs, isoelectric SDs, clustered SDs and total (cumulative) SD-induced depression durations (TDDD) were determined for each 24-h period of each patient in DISCHARGE-1 following the initial hemorrhage (11). The peak TDDD (PTDDD) was defined for each patient as the maximal TDDD among all 24-h periods. The results of DISCHARGE-1 suggested that a 25-min cutoff for the TDDD in the just-past 24-h period is an appropriate first “alert level” to review the patient's status and initiate targeted management strategies. This recommendation was made although it is still relatively uncertain after 25 min whether the event will be reversible or progress to infarction. In addition, a 60-min cutoff was proposed as an appropriate moment to initiate rescue therapy because it indicates still reversible delayed neurological deficit with 0.71 sensitivity and 0.82 specificity. A 180-min cutoff indicated delayed infarction with a targeted 0.62 sensitivity and 0.83 specificity. Moreover, SD variables, and specifically PTDDD were included in each multiple regression model for longitudinal neuroimaging-proven early, delayed, and total brain damage, outcome at 7 months, and patient death (11). Interestingly, the median Glasgow Coma Score was the second most powerful neuromonitoring parameter after SD variables in most models. Longitudinal neuroimage segmentation was performed both manually and semi-automatically.
The hallmark of SD is the near-complete breakdown of the transmembrane ionic gradients, although the name, spreading depolarization, refers to the almost complete loss of neuronal membrane potential (16). SDs occur more or less abundantly in many clinical contexts such as migraine aura, transitory ischemic attacks, stroke, brain trauma, brain death development and dying of the brain from cardiac arrest (11, 14, 21–23). The continuum of SDs describes the spectrum from transient events with negative DC shifts of intermediate to short duration in less ischemic or adequately supplied tissue to terminal events in severely ischemic tissue characterized by long lasting DC shifts and transition of the neurons from the state of injury to cell death (19, 24, 25). In normal tissue, SDs cause vasodilation and hyperperfusion to provide sufficient energy for restoration of the near-collapsed transmembrane ionic gradients by energy-dependent membrane pumps. However, in pathological situations, neurovascular coupling may be disrupted, and SD may lead to an inverse hemodynamic response with severe vasoconstriction and spreading ischemia, resulting in a severe mismatch between energy supply and demand, delaying energy-dependent recovery from SD and inducing cell death (12, 26). Using neuromonitoring technology in combination with longitudinal neuroimaging, the entire sequence of both early and delayed brain infarct development after aSAH with SD-induced persistent activity depression, SD-induced spreading ischemia, and the transition of clustered SDs to the negative ultraslow potential (NUP) was demonstrated in a small patient population in which optoelectrodes were directly overlying newly developing infarcts (27). Similar to animal experiments (28, 29), dying of brain tissue is thus indicated by the transition from SD to a NUP in ECoG and to a persistent diffusion restriction in MRI, whereas SD is initially a reversible event.
For the detection of SDs, so far, subdurally placed Wyler-strip electrodes are considered to be the gold standard electrode technology (Figure 1A) (16, 22, 25, 30). Wyler-strip electrodes can either be placed through a craniotomy or through an enlarged burr hole [compare figure 7 in (26)]. In DISCHARGE-1, Wyler-strip electrodes were placed in 19 of 180 (10.6%) patients through a burr hole. However, this requires an expansion of the burr hole. In order to make ECoG monitoring more widely applicable to patients, we recently reported a less-invasive ECoG electrode implantation approach using subdurally placed Spencer-type electrode arrays (Figure 1B) (31). Due to their conical design, these electrodes can be subdurally implanted through a smaller burr-hole and easily removed at bedside. In the present study, we now investigated whether the ECoG recording quality using this technique is sufficient for reliable neuromonitoring of SDs and SD-induced spreading depression.
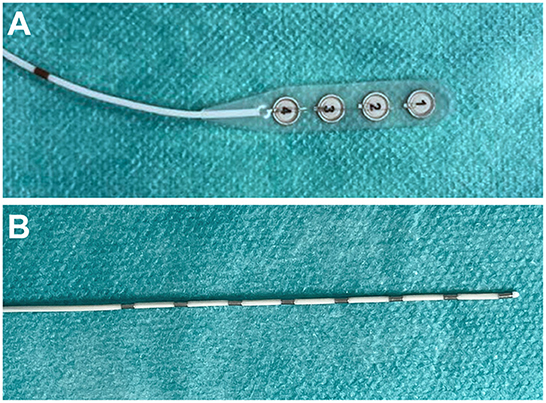
Figure 1. Design of Wyler- and Spencer-type electrode arrays. (A) 4-contact Wyler-electrode. Electrode contacts are isolate on the backside of the electrode and have a diameter of 5 mm. (B) 8-contact Spencer-type electrode arrays. Contacts are located at the darker part of the electrode with 10 mm intercontact distance and 1.1 mm diameter. In contrast to the Wyler-electrode the Spencer-type electrode arrays have the same diameter across the whole length of the electrode.
2. Methods
2.1. Study design
We included patients aged 18 years or older (Table 1) that suffered from a severe aSAH and underwent ECoG monitoring for SDs via subdural Spencer-type electrode arrays, placed during implantation of an external ventricular drain (EVD). Inclusion criteria were according to the DISCHARGE-1 trial, except for the use of Spencer-type electrode arrays. Informed consent was obtained from all patients or their legally authorized representatives.
2.2. Subdural electrocorticography
ECoG electrode implantation in patients suffering aSAH was performed as previously described (31) after securing of the responsible aneurysm either by microsurgical clipping or endovascular therapy. Briefly, a Spencer-type electrode array (6, 8 or 12 platinum contacts with 10 mm distance between contacts, 1.1 mm diameter, AD-Tech Medical Instrument Corporation, Oak Creek, Wisconsin, USA) was subdurally placed on the cortex via a burr hole for implantation of an EVD. The electrode was oriented transversely to a viable cortical region that belonged to the vascular territory of the aneurysm-carrying vessel, because this cortex is often covered with blood and thus is a predilection site for delayed cerebral ischemia (16). After EVD and ECoG electrode implantation, patients were transferred to our intensive care unit (ICU) and ECoG monitoring was performed for a period of 5–10 days (Table 2). Once it was clinically determined that ECoG monitoring was no longer needed, the Spencer-type electrode array was removed at the bedside.
2.3. Patient management
On the ICU, the electrode was connected to a Moberg Neuromonitoring system (Moberg CNS, Moberg Research, Inc., Ambler, Pennsylvania, USA) for online bedside ECoG recording and monitoring. Intracranial pressure (ICP) was continuously monitored, and patients remained intubated and sedated until ICP was within normal ranges. Additional bedside neuromonitoring included brain tissue partial tissue pressure of oxygen (ptiO2) in selected cases (Licox, Integra Lifesciences Corporation, Plainsboro, NJ, USA). A critical ICP threshold was defined as ICP >20 mmHg for longer than 10 min and treated with cerebrospinal fluid drainage, osmotic therapy using mannitol (125–250 ml, maximum every 4 h) and/or hypertonic saline after controlling the blood electrolytes, and/or deep sedation. A routine postoperative computerized tomography scan (CT) was performed within 24 h to rule out procedure-related complications. Treatment was performed according to the guidelines of the German Society of Neurosurgery.
2.4. Data processing
Recording and analysis of ECoG data and interpretation of SDs was performed following the recommendations of the COSBID study group (11, 16, 19). Briefly, for each patient the following parameters were determined: (i) total number of SDs; (ii) number of SDs in electrically inactive tissue (isoelectric SDs); (iii) number of clustered SDs (i.e., SDs that occurred 1 h apart from the previous SD); (iv) peak total SD-induced depression duration of a recording day (PTDDD).
3. Results
3.1. Patient characteristics
Overall, we included seven patients (four females, three males; median age: 58 years; interquartile range: 56–72) that fulfilled the inclusion criteria. All patients suffered severe aSAH with a median Hunt and Hess grade 4 (interquartile range: 2–4) and a median modified Fisher Grade 4 (interquartile range: 4–4). At presentation in the hospital patients had a median Glasgow Coma Scale of 13 (interquartile range: 4–13) and a median World Federation of Neurosurgical Societies Score (WFNS) score of 3 (range 2–5). Detailed patient characteristics are presented in Table 1.
3.2. ECoG monitoring
Implantation of the Spencer-type electrode array (Figure 2) was successfully performed without complications in all patients. During the ICU monitoring period, no electrode-associated bleeding, infection, or cerebrospinal fluid fistula were noted. Also, no complications were noted during electrode removal at bedside. Overall, a total of 84 SDs were recorded in 6/7 patients during a total monitoring duration of 1,194.5 h, which corresponded to a median monitoring period of 201.3 (interquartile range: 126.1–209.4) hours per patient. Four SDs (4.8%) were isoelectric and 48 (57%) occurred in clusters (Figure 3, Table 2). The median PTDDD was 75.6 (interquartile range: 45.5–134.7) minutes. In one of the seven patients, no SD was detected throughout the entire monitoring period. We compared the PTDDD as well as numbers of SDs, isoelectric SDs and clustered SDs per recording day with the corresponding numbers of the DISCHARGE-1 trial and did not find a significant difference (Figure 4).
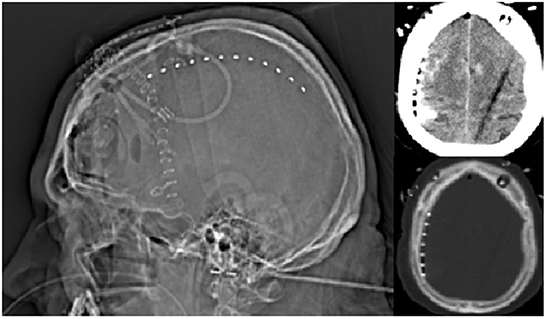
Figure 2. Electrode localization on imaging. Localization of the Spencer-type depth electrode (12 contact electrode) after implantation via a burr hole.
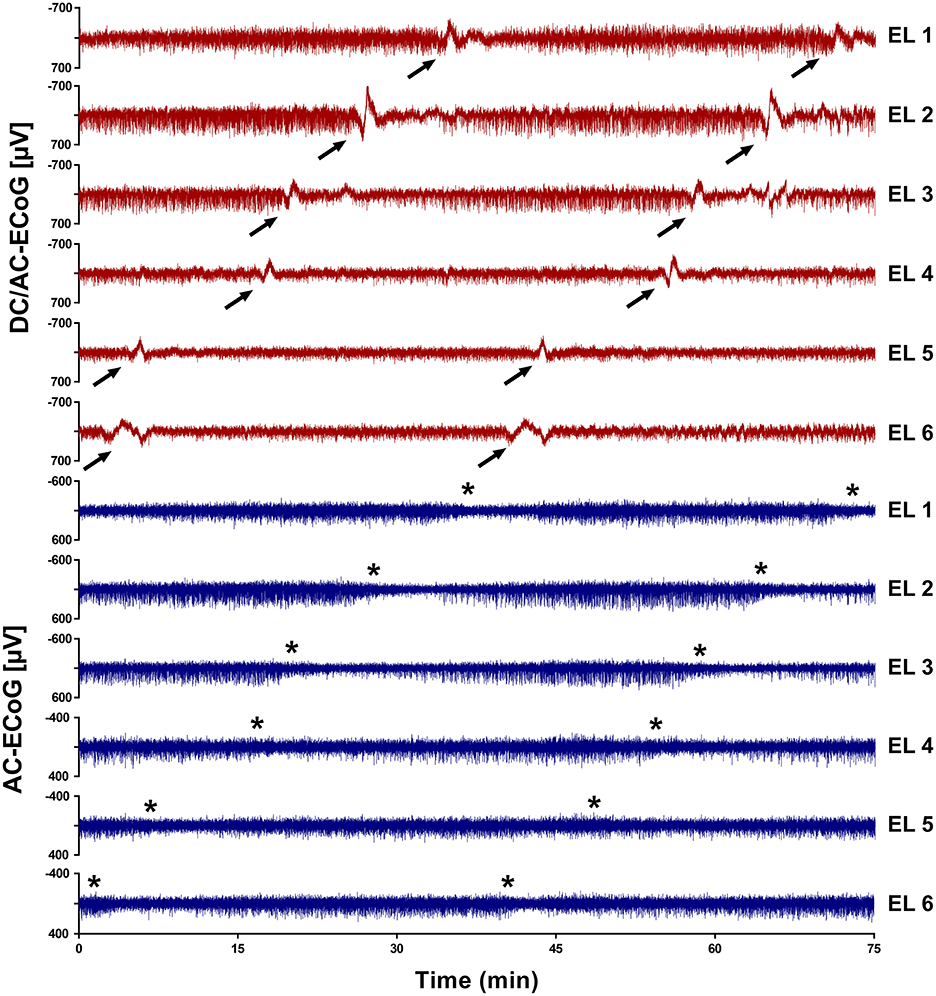
Figure 3. Subdural ECoG recording traces of Spencer-type electrodes arrays. In this case (patient No. 1), monitoring was performed using a 6-contact Spencer-type electrode array placed through a right frontal burr-hole. The occurrence of clustered SDs can be observed by the large negative shift in the direct current ECoG (DC/AC-ECoG; band-pass: 0.01–45 Hz; red traces, arrows). The SDs induced depression of the spontaneous cortical activity in the alternating current range (AC-ECoG; band-pass: 0.5–45 Hz, blue traces, asterisks show the beginning of a depression period). EL, electrode.
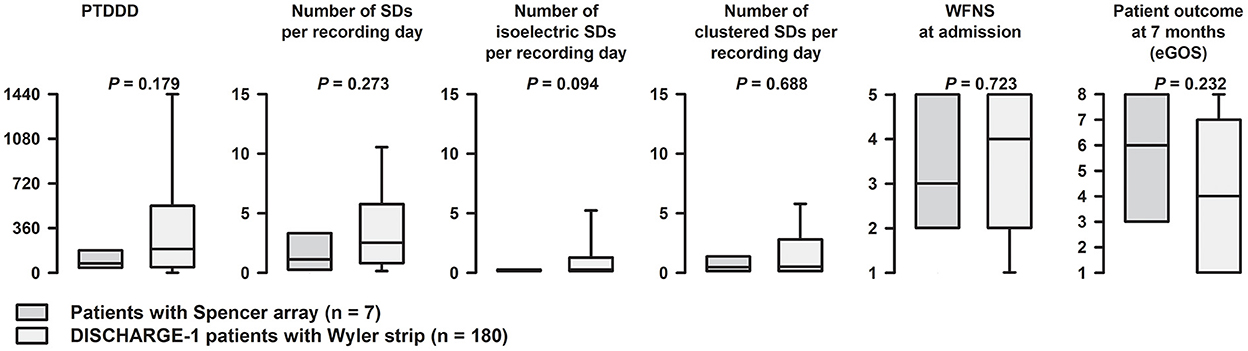
Figure 4. Comparison of the monitoring quality of Spencer-type electrode arrays and Wyler-strips. In this figure the four different SD variables were compared between Spencer-type electrode arrays (n = 7 patients) and Wyler-strips (n = 180 patients from the DISCHARGE-1 trial). There was a tendency for patients in the present study to be slightly less affected than patients in DISCHARGE-1, but this concerned not only SD variables but also WFNS at admission and eGOS at 7 months. Importantly, no significant differences were observed. eGOS, extended Glasgow Outcome Scale score at 3–6 months; PTDDD, peak total SD-induced depression duration of a recording day in minutes; WFNS, World Federation of Neurosurgical Societies Score.
3.3. Outcome
At 7 month after the initial hemorrhage, the patients had reached a median extended Glasgow Outcome Scale (eGOS) score of 6 (range: 3–8), representative of persisting moderate neurological deficits.
4. Discussion
Electrophysiological monitoring of patients requiring neurointensive care has become increasingly important in recent decades (18, 30, 32), as SDs have been shown to serve as real-time biomarkers for the development of secondary brain injury (11). Even though correlates of SDs and SD-induced activity depression have been noted in conventional scalp EEG (33, 34) or EEG with epidural peg electrodes (22), this is currently not sufficient for clinical decision making (16, 35). Thus, the gold-standard for robust SD detection remains subdural ECoG recording performed using platinum/iridium Wyler-strip electrodes (16, 18, 22, 25, 26, 30).
In the present pilot study, we performed subdural ECoG monitoring in seven patients suffering aSAH to determine the feasibility of using less-invasive platinum/iridium Spencer-type electrode array for clinical SD monitoring. We specifically chose aSAH because, along with traumatic brain injury (TBI) (36), it is the best-studied form of severe acute brain injury in which SD monitoring could provide clinical benefit by detecting secondary neurological deterioration in a timely manner and allowing the treating intensivist to provide treatment stratification before infarction has developed (11). To determine the feasibility of Spencer-type electrode arrays for this purpose, recordings of all patients were analyzed regarding the occurrence of SDs, but also regarding the possibility to generate and quantify additional SD parameters that are commonly used for more detailed SD characterization beyond the occurrence of an SD event alone. Most importantly, all recordings provided sufficient data quality in order to be analyzed using the standard methods (16) without requiring additional steps for artifact removal. Further, ECoG recordings in our patients showed similar SD characteristics like those previously described using Wyler-strip electrodes for subdural SD recording in comparable cohorts (18, 37). Notably, the distributions of SD variables within our study were not significantly different from those observed in DISCHARGE-1. There was a tendency for patients in the present study to be slightly less affected than patients in DISCHARGE-1, but this concerned not only SD variables but also WFNS at admission and eGOS at 7 months.
Although our assessment remains inherently limited by the fact that we did not have an additional Wyler-strip inserted next to the Spencer-type electrode array to serve as a direct control, the coherence between our present and previous experience supports the general feasibility of using Spencer-type electrode arrays for robust subdural ECoG and reliable SD detection at bedside in the ICU setting.
In contrast to patients with large craniotomies where subdural electrode placement is considered a straightforward procedure, subdural electrode implantation for ECoG recording is far less common in patients that do not require craniotomy. Although Wyler-strip electrodes can been subdurally inserted via enlarged burr holes, for example in epilepsy surgery (38–40) or to record SDs (26) this procedure is more difficult to handle in a routine setting for ICU monitoring. In contrast, the subdural use of smaller Spencer-type electrode arrays could bear several advantages during implantation and removal, considering their thin cylindrical architecture and equal diameter of merely 1.1 mm. During implantation, only a regular burr-hole is required and the direction of electrode insertion into the subdural space can be precisely guided. Wyler-strips can also be removed at the bedside (16), but the removal of Spencer-type electrode arrays is even easier and less complicated because of their small and uniform diameter.
Apart from these advantages regarding handling, data quality of ECoG recordings is of major importance to ensure reliable SD detection. Importantly, data quality relies on the direct contact of the electrode with the surface of the brain. In the case of the conventional Wyler-strip electrodes, this is ensured by their flat and sheet-like contact surface, where electrode contacts are only located on one side, whereas the other side of the Wyler-strip is isolated. In contrast, the contacts of depth electrodes are located around the circumference of the entire electrode and therefore, the Spencer-type electrode array has direct contact not only to the cortical surface but also to the dura mater lying above. Against this background, an important electrophysiological finding was that we did not detect any relevant artifacts. Possibly, this is explained by the fact that the dura itself is an electrically inactive tissue, which isolates the electrode surface opposite from the brain surface. Also, the circumferential electrode contacts provide the additional advantage that Spencer-type electrode arrays do not require implantation according to a specific orientation, since subdural contact to the brain in ensured in every direction.
In conclusion, our study provides the first evidence that the use of Spencer-type electrode arrays for subdural ECoG monitoring for the purpose of SD detection is feasible. The ability to implant the electrode through a small burr hole, together with the advantage of easy and safe bedside removal and high-quality ECoG recording, may help to make this less invasive technology available to patients who do not require craniotomy, representing an important step toward the introduction of routine clinical SD monitoring in the ICU. However, the findings should still be validated in a larger clinical trial. In addition, it should be investigated in swine, for example, how good SD recordings are in direct comparison when recording in parallel with Wyler-strips and Spencer-type electrode arrays.
Data availability statement
The raw data supporting the conclusions of this article will be made available by the authors, without undue reservation.
Ethics statement
The studies involving human participants were reviewed and approved by Medizinische Ethikkommission Universität Oldenburg. The patients/participants provided their written informed consent to participate in this study.
Author contributions
FM and JW contributed substantially to the conception and design of the work, to patient enrollment and monitoring, and drafting and revising the manuscript for important intellectual content. SH, PD, MB, and RM contributed to data collection, analysis, edited, and approved the manuscript. CL, SM, and NH contributed to data analysis, edited, and approved the manuscript. JD contributed to study design, edited, and approved the manuscript. All authors contributed to the article and approved the submitted version.
Funding
JD was supported by DFG DR 323/10-1 and Era-Net Neuron EBio2, with funds from BMBF (01EW2004).
Conflict of interest
The authors declare that the research was conducted in the absence of any commercial or financial relationships that could be construed as a potential conflict of interest.
Publisher's note
All claims expressed in this article are solely those of the authors and do not necessarily represent those of their affiliated organizations, or those of the publisher, the editors and the reviewers. Any product that may be evaluated in this article, or claim that may be made by its manufacturer, is not guaranteed or endorsed by the publisher.
Abbreviations
COSBID, Co-Operative Studies on Brain Injury Depolarizations; CT, computerized tomography; DC/AC-ECoG, direct-current/ alternating-current electrocorticography; ECoG, electrocorticography; eGOS, extended Glasgow Outcome Scale; EVD, external ventricular drain; GCS, Glasgow Coma Scale; GOS, Glasgow Outcome Scale; ICU, intensive care unit; ICP, intracranial pressure; IEE, ictal epileptiform events; NIHSS, National Institutes of Health Stroke Scale; PTDDD, peak total SD-induced depression duration of a recording day; ptiO2, partial tissue pressure of oxygen; SD, spreading depolarization; WFNS, World Federation of Neurosurgical Societies Score.
References
1. Dreier JP, Major S, Pannek H-W, Woitzik J, Scheel M, Wiesenthal D, et al. Spreading convulsions, spreading depolarization and epileptogenesis in human cerebral cortex. Brain. (2012) 135:259–75. doi: 10.1093/brain/awr303
2. Lee JM, Grabb MC, Zipfel GJ, Choi DW. Brain tissue responses to ischemia. J Clin Invest. (2000) 106:723–31. doi: 10.1172/JCI11003
3. Bodart O, Gosseries O, Wannez S, Thibaut A, Annen J, Boly M, et al. Measures of metabolism and complexity in the brain of patients with disorders of consciousness. Neuroimage Clin. (2017) 14:354–62. doi: 10.1016/j.nicl.2017.02.002
4. Edlow BL, Claassen J, Schiff ND, Greer DM. Recovery from disorders of consciousness: mechanisms, prognosis and emerging therapies. Nat Rev Neurol. (2021) 17:135–56. doi: 10.1038/s41582-020-00428-x
5. Musick S, Alberico A. Neurologic assessment of the neurocritical care patient. Front Neurol. (2021) 12:588989. doi: 10.3389/fneur.2021.588989
6. Macdonald RL, Schweizer TA. Spontaneous subarachnoid haemorrhage. Lancet. (2017) 389:655–66. doi: 10.1016/S0140-6736(16)30668-7
7. Feigin VL, Rinkel GJ, Algra A, Vermeulen M, van Gijn J. Calcium antagonists in patients with aneurysmal subarachnoid hemorrhage: a systematic review. Neurology. (1998) 50:876–83. doi: 10.1212/WNL.50.4.876
8. Allen GS, Ahn HS, Preziosi TJ, Battye R, Boone SC, Chou SN, et al. Cerebral arterial spasm–a controlled trial of nimodipine in patients with subarachnoid hemorrhage. N Engl J Med. (1983) 308:619–24. doi: 10.1056/NEJM198303173081103
9. Espinosa F, Weir B, Shnitka T, Overton T, Boisvert D, A. randomized placebo-controlled double-blind trial of nimodipine after SAH in monkeys. Part 2: pathological findings. J Neurosurg. (1984) 60:1176–85. doi: 10.3171/jns.1984.60.6.1176
10. Petruk KC, West M, Mohr G, Weir BK, Benoit BG, Gentili F, et al. Nimodipine treatment in poor-grade aneurysm patients. Results of a multicenter double-blind placebo-controlled trial. J Neurosurg. (1988) 68:505–17. doi: 10.3171/jns.1988.68.4.0505
11. Dreier JP, Winkler MK, Major S, Horst V, Lublinsky S, Kola V, et al. Spreading depolarizations in ischaemia after subarachnoid haemorrhage, a diagnostic phase III study. Brain. (2022) 145:1264–84. doi: 10.1093/brain/awab457
12. Dreier JP, Körner K, Ebert N, Görner A, Rubin I, Back T, et al. Nitric oxide scavenging by hemoglobin or nitric oxide synthase inhibition by N-nitro-L-arginine induces cortical spreading ischemia when K+ is increased in the subarachnoid space. J Cereb Blood Flow Metab. (1998) 18:978–90. doi: 10.1097/00004647-199809000-00007
13. Cain SM, Bohnet B, LeDue J, Yung AC, Garcia E, Tyson JR, et al. In vivo imaging reveals that pregabalin inhibits cortical spreading depression and propagation to subcortical brain structures. Proc Natl Acad Sci U S A. (2017) 114:2401–6. doi: 10.1073/pnas.1614447114
14. Dreier JP, Lemale CL, Kola V, Friedman A, Schoknecht K. Spreading depolarization is not an epiphenomenon but the principal mechanism of the cytotoxic edema in various gray matter structures of the brain during stroke. Neuropharmacology. (2018) 134:189–207. doi: 10.1016/j.neuropharm.2017.09.027
15. Kirov SA, Fomitcheva IV, Sword J. Rapid neuronal ultrastructure disruption and recovery during spreading depolarization-induced cytotoxic edema. Cereb Cortex. (2020) 30:5517–31. doi: 10.1093/cercor/bhaa134
16. Dreier JP, Fabricius M, Ayata C, Sakowitz OW, Shuttleworth CW, Dohmen C, et al. Recording, analysis, and interpretation of spreading depolarizations in neurointensive care: review and recommendations of the COSBID research group. J Cereb Blood Flow Metab. (2017) 37:1595–625. doi: 10.1177/0271678X16654496
17. Lindquist BE, Shuttleworth CW. Adenosine receptor activation is responsible for prolonged depression of synaptic transmission after spreading depolarization in brain slices. Neuroscience. (2012) 223:365–76. doi: 10.1016/j.neuroscience.2012.07.053
18. Dreier JP, Woitzik J, Fabricius M, Bhatia R, Major S, Drenckhahn C, et al. Delayed ischaemic neurological deficits after subarachnoid haemorrhage are associated with clusters of spreading depolarizations. Brain. (2006) 129:3224–37. doi: 10.1093/brain/awl297
19. Dreier JP. The role of spreading depression, spreading depolarization and spreading ischemia in neurological disease. Nat Med. (2011) 17:439–47. doi: 10.1038/nm.2333
20. Sugimoto K, Nomura S, Shirao S, Inoue T, Ishihara H, Kawano R, et al. Cilostazol decreases duration of spreading depolarization and spreading ischemia after aneurysmal subarachnoid hemorrhage. Ann Neurol. (2018) 84:873–85. doi: 10.1002/ana.25361
21. Carlson AP, Abbas M, Alunday RL, Qeadan F, Shuttleworth CW. Spreading depolarization in acute brain injury inhibited by ketamine: a prospective, randomized, multiple crossover trial. J Neurosurg. (2019) 130:1513–9. doi: 10.3171/2017.12.JNS171665
22. Dreier JP, Major S, Lemale CL, Kola V, Reiffurth C, Schoknecht K, et al. Correlates of spreading depolarization, spreading depression, and negative ultraslow potential in epidural versus subdural electrocorticography. Front Neurosci. (2019) 13:373. doi: 10.3389/fnins.2019.00373
23. Major S, Huo S, Lemale CL, Siebert E, Milakara D, Woitzik J, et al. Direct electrophysiological evidence that spreading depolarization-induced spreading depression is the pathophysiological correlate of the migraine aura and a review of the spreading depolarization continuum of acute neuronal mass injury. GeroScience. (2020) 42:57–80. doi: 10.1007/s11357-019-00142-7
24. Dreier JP, Reiffurth C. The stroke-migraine depolarization continuum. Neuron. (2015) 86:902–22. doi: 10.1016/j.neuron.2015.04.004
25. Hartings JA, York J, Carroll CP, Hinzman JM, Mahoney E, Krueger B, et al. Subarachnoid blood acutely induces spreading depolarizations and early cortical infarction. Brain. (2017) 140:2673–90. doi: 10.1093/brain/awx214
26. Dreier JP, Major S, Manning A, Woitzik J, Drenckhahn C, Steinbrink J, et al. Cortical spreading ischaemia is a novel process involved in ischaemic damage in patients with aneurysmal subarachnoid haemorrhage. Brain. (2009) 132:1866–81. doi: 10.1093/brain/awp102
27. Lückl J, Lemale CL, Kola V, Horst V, Khojasteh U, Oliveira-Ferreira AI, et al. The negative ultraslow potential, electrophysiological correlate of infarction in the human cortex. Brain. (2018) 141:1734–52. doi: 10.1093/brain/awy102
28. Dreier JP, Reiffurth C. Exploitation of the spreading depolarization-induced cytotoxic edema for high-resolution, 3D mapping of its heterogeneous propagation paths. Proc Natl Acad Sci U S A. (2017) 114:2112–4. doi: 10.1073/pnas.1700760114
29. Vinokurova D, Zakharov A, Chernova K, Burkhanova-Zakirova G, Horst V, Lemale CL, et al. Depth-profile of impairments in endothelin-1 - induced focal cortical ischemia. J Cereb Blood Flow Metab. (2022) 42:1944–60. doi: 10.1177/0271678X221107422
30. Strong AJ, Fabricius M, Boutelle MG, Hibbins SJ, Hopwood SE, Jones R, et al. Spreading and synchronous depressions of cortical activity in acutely injured human brain. Stroke. (2002) 33:2738–43. doi: 10.1161/01.STR.0000043073.69602.09
31. Meinert F, Dömer P, Helgers SO, Schumm L, Hecht N, Dreier JP, et al. Subdural placement of electrocorticographic electrode array through a burr hole exposure: 2-dimensional operative video. Oper Neurosurg. (2022) 23:e169. doi: 10.1227/ons.0000000000000299
32. Fabricius M, Fuhr S, Bhatia R, Boutelle M, Hashemi P, Strong AJ, et al. Cortical spreading depression and peri-infarct depolarization in acutely injured human cerebral cortex. Brain. (2005) 129:778–90. doi: 10.1093/brain/awh716
33. Drenckhahn C, Winkler MK, Major S, Scheel M, Kang E-J, Pinczolits A, et al. Correlates of spreading depolarization in human scalp electroencephalography. Brain. (2012) 135:853–68. doi: 10.1093/brain/aws010
34. Hartings JA, Wilson JA, Hinzman JM, Pollandt S, Dreier JP, DiNapoli V, et al. Spreading depression in continuous electroencephalography of brain trauma. Ann Neurol. (2014) 76:681–94. doi: 10.1002/ana.24256
35. Hofmeijer J, van Kaam CR, van de Werff B, Vermeer SE, Tjepkema-Cloostermans MC, van Putten MJ. Detecting cortical spreading depolarization with full band scalp electroencephalography: an illusion? Front Neurol. (2018) 9:17. doi: 10.3389/fneur.2018.00017
36. Hartings JA, Andaluz N, Bullock MR, Hinzman JM, Mathern B, Pahl C, et al. Prognostic value of spreading depolarizations in patients with severe traumatic brain injury. JAMA Neurol. (2020) 77:489. doi: 10.1001/jamaneurol.2019.4476
37. Bosche B, Graf R, Ernestus R-I, Dohmen C, Reithmeier T, Brinker G, et al. Recurrent spreading depolarizations after subarachnoid hemorrhage decreases oxygen availability in human cerebral cortex. Ann Neurol. (2010) 67:607–17. doi: 10.1002/ana.21943
38. Wyler AR, Ojemann GA, Lettich E, Ward AA. Subdural strip electrodes for localizing epileptogenic foci. J Neurosurg. (1984) 60:1195–200. doi: 10.3171/jns.1984.60.6.1195
39. Steven DA, Andrade-Souza YM, Burneo JG, McLachlan RS, Parrent AG. Insertion of subdural strip electrodes for the investigation of temporal lobe epilepsy. Technical note. J Neurosurg. (2007) 106:1102–6. doi: 10.3171/jns.2007.106.6.1102
Keywords: electrocorticography, spreading depolarization, subarachnoid hemorrhage, neurocritical care, Spencer-type electrode array
Citation: Meinert F, Lemâle CL, Major S, Helgers SOA, Dömer P, Mencke R, Bergold MN, Dreier JP, Hecht N and Woitzik J (2023) Less-invasive subdural electrocorticography for investigation of spreading depolarizations in patients with subarachnoid hemorrhage. Front. Neurol. 13:1091987. doi: 10.3389/fneur.2022.1091987
Received: 07 November 2022; Accepted: 08 December 2022;
Published: 05 January 2023.
Edited by:
Marvin Darkwah Oppong, University Hospital Essen, GermanyReviewed by:
Eugene Golanov, Houston Methodist Hospital, United StatesUlrike Hoffmann, University of Texas Southwestern Medical Center, United States
Thomas Freiman, University Hospital Rostock, Germany
Copyright © 2023 Meinert, Lemâle, Major, Helgers, Dömer, Mencke, Bergold, Dreier, Hecht and Woitzik. This is an open-access article distributed under the terms of the Creative Commons Attribution License (CC BY). The use, distribution or reproduction in other forums is permitted, provided the original author(s) and the copyright owner(s) are credited and that the original publication in this journal is cited, in accordance with accepted academic practice. No use, distribution or reproduction is permitted which does not comply with these terms.
*Correspondence: Johannes Woitzik, am9oYW5uZXMud29pdHppayYjeDAwMDQwO2V2YW5nZWxpc2NoZXNrcmFua2VuaGF1cy5kZQ==