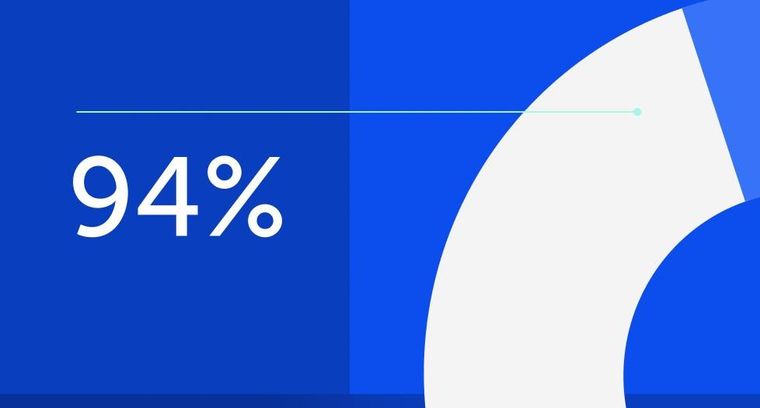
94% of researchers rate our articles as excellent or good
Learn more about the work of our research integrity team to safeguard the quality of each article we publish.
Find out more
REVIEW article
Front. Neurol., 05 January 2022
Sec. Neuromuscular Disorders and Peripheral Neuropathies
Volume 12 - 2021 | https://doi.org/10.3389/fneur.2021.814174
This article is part of the Research TopicGene Therapy for Neuromuscular DiseasesView all 4 articles
Duchenne muscular dystrophy (DMD) is an X-linked recessive, infancy-onset neuromuscular disorder characterized by progressive muscle weakness and atrophy, leading to delay of motor milestones, loss of autonomous ambulation, respiratory failure, cardiomyopathy, and premature death. DMD originates from mutations in the DMD gene that result in a complete absence of dystrophin. Dystrophin is a cytoskeletal protein which belongs to the dystrophin-associated protein complex, involved in cellular signaling and myofiber membrane stabilization. To date, the few available therapeutic options are aimed at lessening disease progression, but persistent loss of muscle tissue and function and premature death are unavoidable. In this scenario, one of the most promising therapeutic strategies for DMD is represented by adeno-associated virus (AAV)-mediated gene therapy. DMD gene therapy relies on the administration of exogenous micro-dystrophin, a miniature version of the dystrophin gene lacking unnecessary domains and encoding a truncated, but functional, dystrophin protein. Limited transgene persistence represents one of the most significant issues that jeopardize the translatability of DMD gene replacement strategies from the bench to the bedside. Here, we critically review preclinical and clinical studies of AAV-mediated gene therapy in DMD, focusing on long-term transgene persistence in transduced tissues, which can deeply affect effectiveness and sustainability of gene replacement in DMD. We also discuss the role played by the overactivation of the immune host system in limiting long-term expression of genetic material. In this perspective, further studies aimed at better elucidating the need for immune suppression in AAV-treated subjects are warranted in order to allow for life-long therapy in DMD patients.
Duchenne muscular dystrophy (DMD), the most common infancy-onset muscular dystrophy, is an X-linked recessive neuromuscular disorder characterized by progressive muscular weakness, wasting, muscle fatty infiltration, and fibrosis (1). By the age of 2–3 years, DMD patients develop lower limb weakness, leading to the delay of motor milestones, walking disturbances, frequent falls and, in the second decade of life, loss of autonomous ambulation (2). During disease progression, patients show respiratory failure, which requires ventilatory support, and cardiomyopathy, which represents the leading cause of death in DMD (3, 4). Almost one third of patients present neuropsychiatric disturbances, including intellectual disability, autistic spectrum disorders, hyperactivity and inattention (5).
DMD is caused by mutations in the DMD gene, which is composed of 79 exons and encodes for the dystrophin protein (6). DMD-causing mutations are mainly frameshift (deletions and, to a lesser extent, duplications, that generate a shift in the translational open reading frame (ORF) of the amino acid chain) or, in 13% of cases, non-sense (point mutations that replace a codon for an amino acid with a stop codon), thus resulting in a complete absence of dystrophin (7). Conversely, ORF-preserving mutations within the DMD gene produce a truncated but partially functional protein, leading to the development of variable phenotypes, ranging from an asymptomatic state to Becker muscular dystrophy (BMD) or DMD (8, 9).
Dystrophin is a 427 kDa cytoskeletal protein composed of four domains: the N-terminal domain; the rod domain, containing 24 triple helix spectrin-like repeats and four hinges; the cysteine-rich domain; the C-terminal domain (10). Together with sarcoglycans, dystroglycans, sarcospan, syntrophin, dystrobrevin, and neuronal nitric oxide synthase, it belongs to the dystrophin-associated protein complex (DAPC) (11), which is involved in cellular signaling and plays a crucial role in the stabilization of myofiber membrane (11). Dystrophin loss results in enhanced vulnerability of the sarcolemma and consequent induction of myofiber split during contraction, functional ischemia, free radicals-mediated oxidative damage, and overload of cytosolic calcium, ultimately leading to mitochondrial dysfunction and myofiber death (12–16). Furthermore, the regenerative ability of myofibers in DMD is impaired, with consequent fibrotic replacement of muscle tissue (17).
To date, no disease-modifying therapeutic option is available for DMD. Current treatments are aimed at lessening disease progression, but premature death is unavoidable. Guidelines for optimal care include multidisciplinary medical, surgical, and rehabilitative strategies, including annual monitoring of respiratory and cardiac function from diagnosis (to be increased after the loss of ambulation and the onset of cardiac symptoms, respectively), the use of mechanically assisted coughing and ventilation devices in case of hypoventilation, and treatment with angiotensin-converting enzyme (ACE) inhibitors by the age of 10 years (1). Corticosteroids are part of the current standard of care (1). Although there is no clear consensus on the timing of glucocorticoid initiation, prednisone or deflazacort are usually introduced after the onset of motor function decline and continued throughout life, as they have been shown to improve muscle force and function, to delay cardiac and respiratory impairment and loss of ambulation, and to reduce the need of surgery for scoliosis (1). The optimal regimen is controversial, as available data from randomized controlled trials are not sufficient to infer conclusions on long-term efficacy and adverse effects of daily vs. intermittent treatment (18).
In this scenario, one of the most promising therapeutic strategies for DMD is represented by gene therapy (19) (Figure 1). Gene therapy aims at the restoration of the deficient protein by delivering an exogenous, functional gene to target tissues with the aid of recombinant adeno-associated viruses (rAAVs). The aim of gene replacement therapy is to provide a therapeutic effect by addressing the genetic root of the disease and improving or reverting disease phenotype. The first gene therapies were approved for human use by the Food and Drug Administration (FDA) in 2017, leading to commercialization of Spark Therapeutics' Luxturna for the treatment of RPE65 mutation-induced blindness and Novartis' Kymriah, the first chimeric antigen receptor T cell therapy (20, 21). Since then, several other gene therapies have been approved. In the neuromuscular field, the approval of gene therapy for Spinal Muscular Atrophy (SMA), aimed at replacing the SMN gene, represented a landmark event (22). Several other gene therapy strategies for treating other neuromuscular diseases, including Duchenne's Muscular Dystrophy, are now under development. The success of therapy is contingent on directing a gene to the correct cells. While non-viral delivery models are under investigation, the most common approach is via viral vectors.
Figure 1. AAV-mediated gene therapy in DMD from bench to bedside. Gene therapy approaches in DMD currently under study are based on the delivery of reduced-length mini- or micro-dystrophin protein packaged within an adeno-associated virus (AAV). The therapeutic vector can be administered systemically via the intravenous route or directly within the involved muscles. After the injection, AAV vectors are imported inside the cell by internalization in clathrin coated endocytic vesicles. The vectors are then released from the vesicles into the cytoplasm and translocated to the nucleus where transgenes are released. Once inside the nucleus, the exogenous DNA particle remains in transduced cells in episomal state, and only a very small percentage (0.1–1%) of the vector genome is integrated into the host chromosome. Transduced cells then start expressing the transgene, with consequent production of a truncated, but functional, dystrophin protein.
Here, we summarize preclinical and clinical studies of AAV-mediated gene therapy in DMD, as well as the main features of AAV biology. We specifically focus on the concept of transgene persistence in cells, with the aim of unraveling potential obstacles in the maintenance of long-term therapeutic effects of gene therapy.
As mentioned above, a considerable issue regarding molecular therapy is optimal delivery, as gene therapy-based strategies require delivery tools that can enable maximal intracellular entry (23). Given their small dimensions, high lipophilicity and reduced binding to serum proteins, molecules such as polymeric nanoparticles (NPs) and lipid complexes might serve this function (24). Nevertheless, viral vectors, in particular retroviruses, lentiviruses, adenoviruses, and AAVs, which are able to infect host cells and replicate through the host machinery (and are thus able to cross the BBB and easily reach target sites), are the most commonly used tools.
The selection of a specific viral vector for gene therapy is based on several factors. First, its specific tropism, made possible by the tight interaction between host cells and vector receptors, with highly efficient transduction to target cells and limited diffusion to other tissues. In addition to that, the need to keep the number of the potential side effects, usually associated with host immune response, at a minimum. Another factor to consider is the long-term expression of the transgene. Ideally, a single dose of AAV-mediated gene therapy should achieve therapeutic protein levels with no toxic effects. Moreover, a non-integrating viral vector is preferable to maintain the transgene in the form of extrachromosomal episome, thus reducing the risk of pro-oncogenic mutagenic effects. Current efforts are directed at the production of the optimal viral vector, with the aid of engineering techniques aimed at overcoming either packaging limitations, with the introduction of novel serotypes or modification of natural capsids, and host immune responses against the vector itself.
In the field of neurologic diseases, the most widely used and studied vectors are AAV vectors, as discussed in the following paragraphs.
Discovered in 1965, theAAVs (Parvoviridae family, Dependovirus genus), are single-stranded DNA viruses with a small (20–28 nm) protein capsule (24). Dependoviruses are characterized by defective replication, requiring a co-infection with an adenovirus or herpesvirus for productive replication in mammalian cells (25).
The 4.7 kb single stranded linear genome can be both plus (sense) or minus (antisense) and contains four ORF: the rep, responsible for the coding of four replication proteins, termed according to their molecular weight (Rep78, Rep68, Rep52, and Rep40); the cap, which encodes the three capsid (Cap) proteins VP1, VP2, and VP3; the aap; the recently identified map (26, 27). The genome is flanked on 5′ and 3′ end by inverted terminal repeats (ITR) made of 145 nucleotides. Genome replication starts from the first 125 ITR nucleotides, which form a T-shaped stem loop structure, and proceeds through a rolling hairpin mechanism (28, 29).
Rep78 and Rep68 cut the terminal AAV site and bind terminal hairpin DNA substrates to process terminal resolution, reinitiation, and strand displacement during AAV replication (30). Rep52 and Rep40 are involved in the accumulation of viral genome and packaging within AAV capsid (30, 31). Rep 78 and 68 can bind the viral genome to a 6-nucleotides recognition sequence (AAVS1 locus) at the human chromosome 19q13. If cellular conditions cannot allow viral replication, the Rep proteins repress the p5 promoter and the viral genome is integrated at the AAVS1 locus, thus guaranteeing a long-term expression both in dividing and non-dividing cells (32). Integration of viral genome into the host genome is, however, a rare phenomenon in mammalian tissues, particularly in skeletal and cardiac muscles (33).
In the recombinant AAV (rAAV), viral genes are replaced by the transgene (34, 35). To allow the packaging of the transgene into the viral capsid, the transgene cassette is flanked by the ITR sequence (34). As rAAVs lack the rep gene, integration difficulty occurs, and with no specificity for the AAVS1 locus (36).
Based on capsid proteins, the AAVs are classified in more than 10 serotypes, characterized by different cell tropism (37–47), whose main features are summarized in Table 1. Several AAV serotypes, including rAAV1, rAAV2, rAAV5, rAAV6, rAAV7, rAAV8, and rAAV9, have shown efficient muscle transduction (37–39, 49, 61–64). As AAV8 and AAV9 can efficiently reach multiple muscles, they are ideal for widespread muscle disorders involving hearth and diaphragm, which can hardly be reached with local injections, as it happens in DMD. Furthermore, the transgene expression after AAV9-mediated cardiac fibers transduction is significantly higher than serotypes 4, 6, 7, and 8 (40, 41).
Preclinical studies have largely demonstrated that the rAAV vector persists in vivo as head-to-tail concatemers mostly organized in episomes (42). The single-stranded rAAV genome is released in the cellular nucleus where the second strand synthesis starts from the 3′-end (43). Subsequently, the double strand genome circularizes and forms high-molecular-weight concatemers (43). These circular intermediates have shown sustained persistence in muscular cells as well as increased episomal stability following liposome-mediated transfection (44). By injecting a shuttle virus, termed V.GFP3ori, containing a green fluorescence protein (GFP) reporter gene in the tibialis anterior muscle of mice, Duan and colleagues demonstrated the presence of circular intermediates with a characteristic head-to-tail structure with a peak of the GFP transgene expression at 22 days, which remained stable for at least 80 days (44). While circular intermediates initially occur as monomer head-to-tail genomes, at 80 days most of them recombine into larger multimers and persist in the episomal state (44). This was suggested to be the principal source of long-term expression, as circular conformation may have an increased resistance to nucleases (44). Furthermore, these circular intermediates showed two ITRs in a head-to-tail orientation, which significantly increased the stability of DNA following transfection into HeLa cells (44).
In dividing cells, persistence is deeply dependent on division rate. For instance, in hepatic cells, which show the highest rate of integration within mammalian cells (<1%), up to 90% of the rAAV genome is loss after surgical hepatectomy, probably because of the loss of episomes with cell division, which is enhanced by the surgical procedure (45). Conversely, in mice that did not undergo hepatectomy, a significant number of rAAV genomes remained non-integrated, leading to persistent transgene expression for up to 19 months (45). A sustained and prolonged (>17 months) expression of the transgene was demonstrated also in a canine model of hemophilia B after a single intramuscular (IM) injection of AAV carrying the transgenic factor IX (46).
Recently, efforts have been made to engineer vectors that could persist in the episomal form in dividing cells, thus allowing a long-term expression with a very low risk of genotoxicity associated with insertional mutagenesis. One approach is based on AAV vectors with a scaffold/matrix attachment region (S/MAR) which maintains long-term transgene expression (>50 population doublings) by replicating AAV vector episomes in the absence of further selection (47). Alternatively, somatic integration of the AAV vector genome into the host chromosome can be enhanced via the Sleeping Beauty (SB) transposase integration machinery (65). This system consists of one AAV vector carrying the transposon with the target gene, and a second one which delivers the hyperactive SB100X transposase (65).
As transgene expression depends on second-strand synthesis, the speed and rate of cell transduction is limited. To overcome this limitation, self-complementary (sc) AAV vectors were developed. Compared to double stranded (ds) and single stranded (ss) AAV, the efficiency of cell transduction of scAAV vectors was significantly higher (66, 67), mainly due to the ability of scAAV to circumvent the step of second strand DNA synthesis mediated by the host cell (68).
The use of rAAVs with natural capsid variants, which are seized in the liver after systemic injection, requires elevated virus doses to reach therapeutic threshold in muscles, thus enhancing therapy-related toxicity (69, 70). Therefore, the DELIVER (directed evolution of AAV capsids leveraging in vivo expression of transgene RNA) strategy was recently applied to engineer AAVs with common arginine-glycine-aspartic acid (RGD) motif-containing capsid variants (MyoAAV 1A) showing higher potency and transduction selectivity of both skeletal and cardiac muscles in mice after systemic and IM administration compared to AAV9 (71). Although MyoAAV 1A was associated with a reduced transduction of liver compared to AAV9, levels of liver enzymes in the serum of AAV9 and MyoAAV1A-injected mice did not differ from controls, thus suggesting absence of hepatic injury at injected doses (71). Using both a CRISPR-Cas9-mediated approach and gene replacement, the authors demonstrated that MyoAAV 1A-mediated systemic delivery of therapeutic transgenes resulted in higher widespread dystrophin rescue in muscles and muscle functional improvement (i.e., greater specific force, lower force drop after eccentric contraction) in mice compared to AAV9 (71). As the effect of MyoAAV 1A on myotubes transduction was mediated by the interaction between RGD motifs and integrin heterodimers, the authors modified amino acids adjacent to the RGD motif to engineer second-generation AAV (MyoAAV 2A) with enhanced muscle tropism, which showed greater muscle transduction efficiency in mice and human primary myotubes compared to AAV9 and MyoAAV 1A (71). As MyoAAV 1A, MyoAAV2-mediated gene therapy in mice led to increased dystrophin rescue and muscle functional improvement (71). Similarly, MyoAAV 4A, 4E, 3A, and 4C were generated and demonstrated higher transduction of skeletal muscle potency in NHPs compared to AAV9 and AAVrh74 (71), which is currently used in DMD gene therapy clinical trials (60).
The advantages of AAV vectors are the lack of transfection-related diseases, the ability to transduce both dividing and non-dividing cells, the reduced immunogenicity and the long-term expression of the delivered transgene in specific tissues (35). However, host immune responses, specifically neutralizing antibodies (NAbs), significantly limit AAV transduction capability in vivo. Noteworthy, up to 70% of the population was shown to carry a protective humoral immune response against AAVs, due to previous exposure to the viruses, with differences in the serotypes based on demographic features. Many attempts to escape host immune responses have been made, including injecting empty capsids as decoys, plasmapheresis and engineering rAAV capsids without Nab integrating epitopes (72–74). Another approach currently under investigation is the use of non-human derived capsids (75). In particular, capsids isolated from non-human primates (NHP) could be an option as NHP-derived AAVrh.8, AAVrh.10, and AAVrh.43 can efficiently transduce different tissues (75). Unfortunately, these approaches were not able to efficiently escape host immune responses. Therefore, individuals exhibiting NAbs are excluded a priori from clinical trials, as gene therapy is expected to be inefficient (27, 76).
Within the most relevant issues related to AAV-mediated gene therapy lies the limited knowledge concerning possible serious adverse events (SAE), which represents a profound obstacle in moving gene therapy strategies to a clinical setting. Little is known, for instance, about AAV-related liver toxicity and its pathogenesis. In clinical trials enrolling spinal muscular atrophy (SMA) and DMD patients, a significant elevation of liver enzymes was described, although it was controlled with corticosteroids with no relevant clinical impact (39, 60, 77). However, in June 2021 Audentes Therapeutics reported the death of three patients affected by X-linked myotubular myopathy (XLMTM), who received the highest AAV dose ever used in humans (3 x 1014 vg/kg) in the context of the ASPIRO clinical trial (NCT03199469), aimed at evaluating safety and efficacy of AT132, an AAV8-delivered gene therapy (AAV8-MTM1) (69, 78). XLMTM is a lethal heterogeneous myopathy commonly fatal within 12 years of age, that results from mutations in myotubularin-encoding gene MTM1 (79). From 4 to 6 weeks after administration, the two patients, who both had a history of hepatobiliary disease, underwent progressive liver dysfunction and sepsis leading to death (69). The timing, dose-dependency and tissue-specificity pointed toward an adaptive immune response against vector capsid or transgene product as primary cause of the recorded SAEs, although a definite etiology has not been proved yet (69).
In a phase Ib clinical trial involving 9 DMD patients who received a single intravenous administration of PF-06939926 (NCT03362502), preliminary data disclosed three SAEs, namely vomiting resulting in dehydration, acute kidney injury, and thrombocytopenia with atypical hemolytic uremic syndrome-like complement activation (80). All these SAEs resolved within 2 weeks, but their occurrence suggests that an overactive immune response toward the vector may hinder the application of gene therapy, at least at high doses (80).
The dystrophin gene has a coding sequence of 14 Kb, which largely exceeds the packaging size of AAV vectors. This has precluded the use of AAV-mediated gene replacement in DMD until miniature versions of dystrophin genes were developed. These micro (four or fewer repeats) and mini (more than four repeats) dystrophin genes encode for truncated but functional proteins lacking unnecessary domains, including a major portion of the rod domain and the distal C terminus domain (81, 82). The result is a milder dystrophic phenotype compared to untreated subjects (83).
AAV-mediated gene replacement was first tested in the mdx mouse model (84–90). The mdx mouse harbors a non-sense mutation in the exon 23 of the Dmd gene, which impairs dystrophin expression (91). After injecting several mini-dystrophin genes packaged into AAV vectors in the hindleg muscle (i.e., tibialis anterior) of both 10-day-old and adult mdx mice, Wang and colleagues demonstrated widespread expression of mini-dystrophins in most myofibers and significant reduction of pathological dystrophic features (85). Two years later, the same group repeated injections of mini-dystrophin genes packaged into AAV vectors in the hindleg muscle of adult mdx mice, revealing a significant improvement in muscle contractile function and resistance to mechanical stress (84). However, muscle strength of AAV-treated mdx mice remained significantly lower than the strength of control C57/B10 mice (84). Possible explanations are the low transduction efficiency of AAV serotype 1 in fast-twitch myofibers, which are predominant in the tibialis anterior muscle, or the reduced ability of mini-dystrophins to restore muscle force compared to full-length dystrophin (84).
As the mdx mouse shows a compensatory over-expression of dystrophin-related proteins such as utrophin, thus resulting in a milder dystrophic phenotype compared to DMD patients, Gregorevic and colleagues selected the dystrophin/utrophin double-knockout (dko) mouse model to test the intravascular administration of micro-dystrophin packaged into an AAV-6 (86). The authors showed widespread expression of dystrophin after 18 weeks in the diaphragm and cardiac muscle (86). In the diaphragm, they also demonstrated reduction of histological biomarkers of muscle dystrophy, increased muscle strength, and resistance to contraction-induced damage. The results obtained in skeletal muscles were similar to those reported before (86).
Promising results were obtained also in dog models of canine X-linked muscular dystrophy (CXMD), which were intramuscularly injected after immunosuppression with a combination of anti-thymocyte globulin (ATG), cyclosporine (CSP), and mycophenolate mofetil (MMF), so to guarantee both a long-term expression of the micro-dystrophin transgene and the restoration of DAPC at the sarcolemma (92). Indeed, previous studies reported the development of a T cell-mediated immune response after IM injection of AAV2 and AAV6, which compromised sustained AAV-mediated dystrophin expression in models other than mice (93). After discontinuation of CSP and MMF, patches of T-cell infiltration were shown (92). However, the expression of micro-dystrophin persisted in areas with reduced or absent T-cell infiltrates (92).
Although AAV9 apparently avoided the development of an immune response when intravenously injected in neonatal dogs in a previous study, Kornegay and colleagues could not replicate the aforementioned promising results in the golden retriever muscular dystrophy (GRMD) model, intravenously injected with an AAV9 vector carrying a human mini-dystrophin in absence of immunosuppression (64, 94). Even though mini-dystrophin was still expressed in almost all myofibers of different muscles at 16 weeks after treatment, treated GRMD dogs showed pelvic limb muscle atrophy and contractures, and mild degeneration and regeneration features of myofibers at histological examination, accompanied by severe fatty replacement (64). Conversely, immunosuppression in juvenile DMD dogs intravenously injected with a micro-dystrophin AAV9 vector variant, with a surface tyrosine mutated to reduce immunogenicity, allowed not only a widespread, long-term muscle transduction in skeletal muscle, diaphragm and heart, but also improvement in muscle histology (95).
Intriguingly, Le Guiner and colleagues reached long-term (up to 24 months) high levels of micro-dystrophin expression in skeletal muscles and marked improvement of histological and functional parameters after intravenous delivery of recombinant AAV2/8 vector expressing a canine micro-dystrophin in GRMD dogs, in absence of immunosuppression (96). Possible explanations of the lack of detectable cellular immune response resulting in long-lasting transgene expression might include the use of a muscle-specific synthetic promoter, and factors related to the recombinant AAV2/8 production protocols (96).
Before moving to the human setting, Rodino-Klapac and colleagues tested the feasibility and efficacy of a FLAG-tagged (to differentiate endogenous and vector-derived protein) micro-dystrophin, packaged into an AAV8 vector, and delivered in rhesus macaques via IM and intraarterial route (97). The authors observed a strong and long-term (at least 3–5 months) gene expression (50–79% of myofibers after IM injection in the tibialis anterior muscle; >80% of myofibers of the gastrocnemius muscle after intra-arterial injection in the femoral artery of rhesus macaques without pre-existing AAV8 antibodies), without evidence of immune cellular response (97).
The first randomized double-blind placebo-controlled phase I clinical trial aimed at assessing the impact of the IM delivery of an AAV micro-dystrophin gene (rAAV2.5-CMV-mini-dystrophin, d3990) (NCT00428935) failed to confirm the promising results obtained in animal models (98, 99). Six patients with frame-shifting deletions in the dystrophin gene were enrolled and followed up between 2006 and 2009, and received two different doses of vector genome (2.0 x 1010/kg and 1.0 x 1011/kg) via injection in the biceps muscle (98, 99). To enhance the muscle transduction capacity of the adenoviral vector, the AAV2.5 was generated from the AAV2 capsid by adding five mutations from AAV1 (99). Quantitative polymerase chain reaction (PCR) confirmed the presence of the transgene DNA in both injected and contralateral arms of all patients except one, in whom spread to the contralateral arm was not found (99). Possible explanations of the minimal long-term gene expression (0/6 patients at 90 days; 2/6 patients at 42 days) include the development of a T cell-mediated immune response, pre-existing anti-AAV antibodies (detected in 2/6 patients), pre-existing T cells targeting endogenous revertant dystrophin epitopes which are shared by the mini-dystrophin employed (detected in 2/6 patients), dystrophy-related inflammation, reduced tropism of AAV2.5 to human muscles and silencing of the CMV promoter in human dystrophic myofibers (98, 99).
In September 2020, Mendell and colleagues published the results of an open-label, phase I/IIa, non-randomized, controlled trial (NCT03375164), which enrolled 4 ambulatory DMD patients (mean age at enrollment, 4.8 years), carrying a confirmed DMD frameshift or premature stop codon mutation between exons 18 and 58, without preexisting AAVrh74 antibodies, who had been on a stable corticosteroid dose for at least 12 weeks before entry (60). Patients received a single dose of intravenous 2.0 × 1014 vg/kg rAAVrh74.MHCK7.micro-dystrophin (SRP-9001), together with prednisone at high doses for 30 days, followed by a slow taper (60). The MHCK7 promoter was selected for its high levels of expression in both skeletal muscles, including the diaphragm, and hearth (100). The micro-dystrophin was well-tolerated, with only mild-to-moderate adverse effects (n = 53); only 18 of them were considered treatment-related, the most common being vomiting (n = 9) (60). Immunohistochemistry of gastrocnemius muscle biopsy performed at 12 weeks showed strong transgene expression, and improvements both in the CK levels and in the North Star Ambulatory Assessment (NSAA) score were demonstrated after 1 year (60).
In May 2020, the preliminary data of a phase Ib clinical trial conducted on 9 ambulatory DMD patients, who received a single intravenous administration of PF-06939926 (NCT03362502) and were followed up for 12 months, were presented at the American Society of Gene and Cell Therapy (ASGCT) Annual Meeting (80). Moreover, robust expression of micro-dystrophin persisted at 12 months, together with improvement in the NSAA score (80).
Solid Biosciences have recently reported interim efficacy and safety encouraging data from the ongoing phase I/II IGNITE DMD Clinical Trial (NCT03368742) from six patients treated with either a low or high dose of SGT-001, a micro-dystrophin characterized by the addition of a neuronal nitric oxide synthase (nNOS)-binding domain to the cassette to enhance muscle blood flow, thus supporting the continued enrollment of patients (101).
As summarized in Table 2, several clinical trials of AAV-mediated gene replacement are ongoing (NCT03375164; NCT03368742) or have been completed (NCT02376816), although preliminary results have not been published yet.
Utrophin is a cytoskeletal protein located at the neuromuscular and myotendinous junctions (102). It represents an autosomal paralog of dystrophin with about 80% homology, and its AAV-mediated over-expression has been investigated in several DMD animal models (103–105). Indeed, the AAV-mediated gene transfer of a shortened version of utrophin, delivered by IM injection in newborn mdx mice, was able to restore the DAPC at the sarcolemma, to reduce histological dystrophic features (i.e., central nucleation), to protect the sarcolemma from injury, and to enhance muscle strength and resistance to mechanical stress-induced damage (103). These findings were not observed in 30–45-day-old mice after IM injection, because of the development of a humoral response (103). Subsequently, a micro-utrophin packaged into a recombinant AAV6 vector was intravenously delivered in 1 month-old mdx/Utr+/− mice, which have a more severe phenotype compared to mdx mice, thus mimicking human DMD patients (104). Micro-utrophin was subsequently detected not only at the myotendinous junction, but also within the sarcolemma, unlike matched wild-type control mice (104). Consistent with previous results, the AAV-mediated micro-utrophin over-expression rescued the DAPC components at the sarcolemma. Compared with controls, the treated mdx/Utr+/− mice showed increased body and heart mass, reduced CK levels, mitigation of histological dystrophic features (i.e., inflammatory infiltrates, central nucleation), and significant increase in muscle force generation capacity (103, 104). More recently, the systemic AAV-mediated delivery of artificial zinc finger transcription factors (ZF-ATFs) targeting the utrophin A promoter have been tested in mdx mice with the aim of up-regulating the utrophin expression levels (106, 107). The authors described a significant reduction in muscle pathological and biochemical features (i.e., inflammatory infiltrates, central nucleation, CK levels), an improvement in exercise performance and contractile activity, and an enhanced acetylcholine receptors postsynaptic clustering (107).
The β1-4-N-acetyl-D-galactosamine (βGalNAc) glycosyltransferase, encoded by the GALGT2 gene, is involved in glycosylation of α-dystroglycan in myofibers (108). The AAV-mediated delivery of GALGT2 via femoral artery in the rhesus macaque allowed a significant gene expression (44%) in myofibers, which was reduced to 9% in presence of pre-existing antibodies, with increased glycosylation of α-dystroglycan and expression of dystrophin surrogate proteins (i.e., utrophin) (109). The rAAVrh74.MCK.GALGT2 is currently being tested in a phase I/II clinical trial (NCT03333590), aimed at assessing the safety of intravascular lower limb infusion in 6 DMD patients (Table 2).
Follistatin (rAAV1.CMV.huFollistatin344), a potent myostatin inhibitor, obtained encouraging results in pre-clinical setting and in BMD patients, and is currently being tested in a phase I/II clinical trial (NCT02354781) (50, 110). Three DMD patients have received the drug via IM injection in the gluteal muscles, quadriceps, and tibialis anterior of 3 DMD patients. The primary endpoint is the overall improvement in the 6 Minute Walk Test (6MWT), but related results have not been published yet (Table 2). Among the adverse events recorded so far, only one was deemed serious, namely head injury from fall.
One of the biggest issues regarding effectiveness and sustainability of gene therapy for DMD is the uncertainty related to its long-term persistence. Indeed, as muscular tissue is capable of proliferation and renovation, and the cycle of degeneration and regeneration is particularly active in DMD muscles, it is expected that the expression of transgene could fade over time. To this regard, results obtained in healthy muscle tissue, as well as those arising from administration of transgenes in non-muscle tissues, hold limited value. To date, available studies have not yielded consistent results as regards persistence of DMD-targeting transgenes within muscle tissues, nor they were able to undoubtedly point out optimal timing and route of administration to improve long term expression. The limited life-span of animal models, especially mice (2–3 years), represents a significant challenge for preclinical studies focused on persistence of transgene expression after gene replacement, as it intrinsically cannot be employed to investigate the longer duration of expression required by DMD patients. Therefore, the reader should bear in mind that results from studies on “long-term” expression in animal models might not be entirely translatable to human subjects, nor they account for the full range of muscular and systemic alterations that might be retrieved in DMD patients suffering from a longstanding disease. Having said that, current evidence can provide valuable insights on methods to enhance transgene persistence in muscle tissues.
Several lines of evidence showed that persistence of AAV genome within actively replicating, immature tissues is limited (111–113). These findings notwithstanding, some authors advocate the ability of AAV-based gene therapy to achieve therapeutic levels during early life without significant limitations to long-term expression, based on animal studies. For instance, Daly and colleagues showed that intravenous administration of an AAV encoding human beta-glucuronidase in newborn mice partially rescued the phenotype and resulted in expression up to 16 weeks of age in liver, heart, lung, spleen, kidney, brain, and retina. Notably, therapeutic levels of transgene and phenotypic rescue were not achieved in skeletal muscle (114). Other studies demonstrated persistent expression of transgene following intravenous neonatal administration in non-muscle tissues (115–118). Studies in healthy canine models yielded somewhat better results. Intravenous delivery of AAV-9-encoded micro-dystrophin in neonatal dogs led to skeletal muscle transduction throughout the body for at least 6 months, with highly transduced muscles expressing the transgene in up to 80% myofibers (94). Systemic transduction was obtained with no need of immune suppression. No study regarding intravenous administration of gene therapy for DMD has been conducted in newborn animals harboring DMD mutations so far.
Conversely, the IM route has been more intensively studied. IM injection of AAV during neonatal phase has previously proved able to ensure long-term expression of transgene in animal models of muscular diseases (119). IM injection of AAV-encoded micro-dystrophin in newborn dystrophic mdx mice allowed sarcolemmal expression of micro-dystrophin in 40 to 60% of myofibers up to 20–24 weeks of age in absence of immunosuppression in different studies (85, 87, 89). In contrast, another study showed that IM injection of an AAV encoding micro-dystrophin in mdx mice during the neonatal period led to high degree of short-term expression but significantly reduced long-term expression (90). Remarkably, long-term expression was restored when injection was performed in a mouse model of severe combined immunodeficiency (SCID) (90). Indeed, immune response toward the transgene seems to play a key role in impairing sustained long-term expression.
The transduction of post-mitotic or slowly replicating adult tissues should allow, at least in theory, to achieve longer genome persistence and stable transgene expression. For instance, animals treated with AAV gene transfer during adult life demonstrate persistence of vector genomes in the liver in their episomal form, with poor evidence of genomic integration, for more than 10 years (120, 121). Similarly, studies in humans proved that a single injection of AAV vector expressing human factor IX transgene led to stable transgene expression in the liver for as long as 3 years (122, 123). More recently, systemic AAV delivery of a transgene encoding micro-dystrophin was attempted in aged dystrophic mdx mice, with robust dystrophin expression in skeletal and/or cardiac muscle 2 to 8 months after injection (124–127). One study reported a significant immune response within the skeletal muscle of transduced mice, that was successfully prevented with steroid administration, with satisfactory therapeutic efficacy 4 weeks after transduction (128). The administration of AAV-microdystrophin via IM route in adult mice yielded successful results as well. Wang and colleagues delivered intramuscularly an AAV encoding a micro-dystrophin, expressed under the MCK promoter, in adult mdx mice, with a satisfactory clinical response (85). After 2 months, dystrophin was expressed by 35–50% of myofibers, while this percentage dropped to 20–30% after 4 months (85).
In diseased large mammals, body wide gene transfer has been attempted under transient or sustained immune suppression, resulting in widespread transduction in skeletal muscle, the diaphragm and heart persisting for at least 4 months (95). Evidence in wild-type canine models showed that IM injection of AAV2 or AAV6 resulted in a powerful immune response to capsid or capsid-associated proteins (93). Nonetheless, a brief course of immunosuppressive treatment was sufficient to permit long-term persistence of a canine micro-dystrophin transgene in the skeletal muscle of a DMD dog model (92). Such studies suggest that immune-mediated mechanisms might be responsible for loss of vector persistence after IM injection in big mammals such as dogs and humans, thus making the case for use of immune modulation to achieve long term transgene expression in these species. These findings notwithstanding, in 2017 an effective and safe delivery of a micro-dystrophin transgene in juvenile GRMD dog model of DMD by both intravenous locoregional and systemic administration of a rAAV2/8 vector was achieved, showing long-term persistence (up to 24 months) in the absence of preventive immunosuppression (96). However, when extending follow-up to 4 years, as performed by Elverman and colleagues in a canine model of XLMTM after loco-regional perfusion of AAV8-MTM1, a significant decline of viral copy numbers and myotubularin expression was recorded (129).
Similarly, another preclinical study led on the GRMD dog model of DMD performed a 5-year follow-up of dystrophin rescue after either IM or intravenous of AAV1-U7E6/8, an antisense sequence which induces skipping of exons 6 and 8, carried by the engineered small nuclear RNA (snRNA) U7. The authors showed an eightfold decrease of dystrophin positive myofibers, in line with the progressive loss of the U7 system (130). As no clear signs of overactivation of immune response were detected, it is possible that the decline of dystrophin expression might have resulted from the persistent pathological muscular process, which was delayed but not stopped by the truncated, not fully functional dystrophin protein (130). Intriguingly, in the exon 52-deficient mdx (mdx52) mouse model, the intravenous injection of an AAV9-U7snRNA containing an antisense sequence to skip exon 51 induced high rates of exon skipping in myofibers and cardiac fibers, but low levels of dystrophin expression, both at 8 weeks and 6 months (131). This discrepancy might originate from a deficient protein translation from the exon 51-skipped mRNA, from different mRNA levels between various DMD mouse models or from higher instability of the dystrophin protein derived from exon 51-skipped mRNA compared to others.
In addition to that, Rodino-Klapac and colleagues packaged micro-dystrophin into an AAV8 vector and delivered it via IM and intraarterial route in rhesus macaques, showing a persistent transgene expression (50–80% of myofibers after IM injection, >80% of myofibers after intra-arterial injection) for up to 3–5 months, without evidence of immune cellular infiltration, suggesting that other factors might have a role in the modulation of immune response to AAVs and transgene expression (97).
Overall, the reviewed papers point out that overactivation of the immune system represents a big challenge not only according to safety, but also in its potential to affect transgene persistence. It has been suggested that the dystrophic microenvironment might stimulate the cellular immune response, and even provoke the loss of AAV in dystrophic muscle. It seems that the use of muscle-specific synthetic promoters (e.g., MCK) might be helpful in reducing the potential for detectable cellular immune response, resulting in long-lasting transgene expression, although this surely is not the only factor implicated in the process (96, 128). Moreover, different regimens of ongoing glucocorticoid therapy (daily vs. intermittent) could influence the development of immune responses and, therefore, the long-term persistence of the transgene. Indeed, promising results were obtained in DMD patients who received a single dose of intravenous rAAVrh74.MHCK7.micro-dystrophin while being on a stable corticosteroid dose for at least 12 weeks before entry, and subsequently prednisone at high doses for 30 days, followed by a slow taper. Nonetheless, the brief follow-up does not allow to drive conclusions on this topic (60).
In this scenario, therapy re-administration over time might be required to achieve long-term efficacy but might be limited by the development of neutralizing antibodies targeting the vector. Evidence from studies conducted on other diseases might also be helpful in this regard. Based on the results of preclinical studies, a phase I/II clinical trial (NCT02240407) evaluating the re-administration of rAAV9-mediated human acid alpha-glucosidase (hGAA) in six adult patients affected by late-onset Pompe disease is currently ongoing (132). The authors provided an immune modulation approach to prevent the development of a humoral immune response against the capsid and the transgene, based on B-cell ablation with rituximab before gene therapy, whose efficacy in guaranteeing long-term persistence of transgene expression in human setting needs to be confirmed (132).
AAV-mediated gene therapy is a flourishing field of discovery for human genetic and acquired disorders, including neuromuscular diseases such as DMD. After the promising results obtained by pre-clinical studies in different DMD mammalian models, scientific efforts are moving to translate these findings from the bench to the bedside. To date, several phase I/II clinical trials are ongoing, but definitive results are still lacking, thus preventing us from drawing reliable conclusions.
One critical issue associated with AAV-mediated gene therapy is the persistence of the exogenous genetic material within human cells and, more specifically, in myofibers and cardiac fibers in the case of muscular dystrophies. In addition to that, as outlined in this review of current literature, several lines of evidence suggest that enhanced host immune responses may affect transgene persistence. Indeed, a better understanding of these topics could shed light on the conundrum concerning the expected persistence of therapeutic effects following administration of gene therapy in muscular dystrophies.
Further insights from both preclinical and clinical trials are necessary also to elucidate more precisely the need for immune suppression in human subjects. So far, evidence suggests that re-administration would very likely be necessary to ensure life-long therapy in DMD patients. In this perspective, strategies to tackle the presence of preexisting neutralizing antibodies are needed, and indeed some strategies have already been proposed (e.g., use of alternative AAV capsid, immunomodulatory treatments, and plasmapheresis) (81). Further preclinical studies are ongoing to test the suitability of these approaches.
An interesting development of these molecular strategies is the potential to extend their use to mild dystrophinopathies, such as BMD (9). It has to be pointed out that most of the molecular defects in BMD concern the quantity and quality of dystrophin and are not amenable to correction with existing exon-skipping strategies, but innovative methods such as gene editing with CRISPR/Cas9 could potentially be effective (9). Further studies will be needed in this subset of patients.
In conclusion, AAV-mediated gene therapy is currently revolutionizing the world of novel therapeutic approaches for neuromuscular disorders, including DMD. The possibility to achieve long-term therapeutic effects is strongly conditioned by the persistence of transgene expression in transduced tissues, which still represents an unraveled field of investigation. Hopefully, ongoing preclinical and clinical trials will help disclose its molecular basis, and therefore overcome current obstacles in the pursuit of stable long-term outcomes.
AM, EA, and AN revised the literature and wrote the manuscript. GC conceived the idea. GC and SC performed a critical revision of the manuscript for important intellectual content. All authors have read and approved the manuscript.
This work was supported by the Italian Ministry of Health Ricerca Corrente to GC.
The authors declare that the research was conducted in the absence of any commercial or financial relationships that could be construed as a potential conflict of interest.
All claims expressed in this article are solely those of the authors and do not necessarily represent those of their affiliated organizations, or those of the publisher, the editors and the reviewers. Any product that may be evaluated in this article, or claim that may be made by its manufacturer, is not guaranteed or endorsed by the publisher.
We would like to thank Associazione Amici del Centro Dino Ferrari for its support. Figure was modified from images from Servier Medical Art, licensed under a Creative Common Attribution 3.0 Generic License. http://smart.servier.com/.
1. Duan D, Goemans N, Takeda S, Mercuri E, Aartsma-Rus A. Duchenne muscular dystrophy. Nat Rev Dis Prim. (2021) 7:13. doi: 10.10/s41572-021-00248-3
2. Mercuri E, Bönnemann CG, Muntoni F. Muscular dystrophies. Lancet. (2019) 394:2025–38. doi: 10.1016/S0140-6736(19)32910-1
3. Szabo SM, Salhany RM, Deighton A, Harwood M, Mah J, Gooch KL. The clinical course of Duchenne muscular dystrophy in the corticosteroid treatment era: a systematic literature review. Orphanet J Rare Dis. (2021) 16:237. doi: 10.1186/s13023-021-01862-w
4. Kieny P, Chollet S, Delalande P, Le Fort M, Magot A, Pereon Y, et al. Evolution of life expectancy of patients with Duchenne muscular dystrophy at AFM Yolaine de Kepper centre between 1981 and 2011. Ann Phys Rehabil Med. (2013) 56:443–54. doi: 10.1016/j.rehab.2013.06.002
5. Ricotti V, Mandy WPL, Scoto M, Pane M, Deconinck N, Messina S, et al. Neurodevelopmental, emotional, and behavioural problems in Duchenne muscular dystrophy in relation to underlying dystrophin gene mutations. Dev Med Child Neurol. (2016) 58:77–84. doi: 10.1111/dmcn.12922
6. Tennyson CN, Klamut HJ, Worton RG. The human dystrophin gene requires 16 hours to be transcribed and is cotranscriptionally spliced. Nat Genet. (1995) 9:184–90. doi: 10.1038/ng0295-184
7. Magri F, Govoni A, D'Angelo MG, Del Bo R, Ghezzi S, Sandra G, et al. Genotype and phenotype characterization in a large dystrophinopathic cohort with extended follow-up. J Neurol. (2011) 258:1610–23. doi: 10.1007/s00415-011-5979-z
8. Comi GP, Prelle A, Bresolin N, Moggio M, Bardoni A, Gallanti A, et al. Clinical variability in becker muscular dystrophy genetic, biochemical and immunohistochemical correlates. Brain. (1994) 117:1–14. doi: 10.1093/brain/117.1.1-a
9. Angelini C, Marozzo R, Pegoraro V. Current and emerging therapies in Becker muscular dystrophy (BMD). Acta Myol. (2019) 38:172–9.
10. Koenig M, Hoffman EP, Bertelson CJ, Monaco AP, Feener C, Kunkel LM. Complete cloning of the duchenne muscular dystrophy (DMD) cDNA and preliminary genomic organization of the DMD gene in normal and affected individuals. Cell. (1987) 50:509–17. doi: 10.1016/0092-8674(87)90504-6
11. Gao QQ, McNally EM. The dystrophin complex: Structure, function, and implications for therapy. Compr Physiol. (2015) 5:1223–39. doi: 10.1002/cphy.c140048
12. Turner PR, Westwood T, Regen CM, Steinhardt RA. Increased protein degradation results from elevated free calcium levels found in muscle from mdx mice. Nature. (1988) 335:735–8. doi: 10.1038/335735a0
13. Millay DP, Sargent MA, Osinska H, Baines CP, Barton ER, Vuagniaux G, et al. Genetic and pharmacologic inhibition of mitochondrial-dependent necrosis attenuates muscular dystrophy. Nat Med. (2008) 14:442–7. doi: 10.1038/nm1736
14. Stedman HH, Sweeney HL, Shrager JB, Maguire HC, Panettieri RA, Petrof B, et al. The mdx mouse diaphragm reproduces the degenerative changes of Duchenne muscular dystrophy. Nature. (1991) 352:536–9. doi: 10.1038/352536a0
15. Sander M, Chavoshan B, Harris SA, Iannaccone ST, Stull JT, Thomas GD, et al. Functional muscle ischemia in neuronal nitric oxide synthase-deficient skeletal muscle of children with Duchenne muscular dystrophy. Proc Natl Acad Sci USA. (2000) 97:13818–23. doi: 10.1073/pnas.250379497
16. Kim JH, Kwak HB, Thompson L V, Lawler JM. Contribution of oxidative stress to pathology in diaphragm and limb muscles with Duchenne muscular dystrophy. J Muscle Res Cell Motil. (2013) 34:1–13. doi: 10.1007/s10974-012-9330-9
17. Rosenberg AS, Puig M, Nagaraju K, Hoffman EP, Villalta SA, Rao VA, et al. Immune-mediated pathology in Duchenne muscular dystrophy. Sci Transl Med. (2015) 7:299rv4. doi: 10.1126/scitranslmed.aaa7322
18. Matthews E, Brassington R, Kuntzer T, Jichi F, Manzur AY. Corticosteroids for the treatment of Duchenne muscular dystrophy. Cochrane Database Syst Rev. (2016) 2016:CD003725. doi: 10.1002/14651858.CD003725.pub4
19. Falzarano M, Scotton C, Passarelli C, Ferlini A. Duchenne muscular dystrophy: from diagnosis to therapy. Molecules. (2015) 20:18168–84. doi: 10.3390/molecules201018168
20. FDA. KYMRIAH (tisagenlecleucel). Available online at: https://www.fda.gov/vaccines-blood-biologics/cellular-gene-therapy-products/kymriah-tisagenlecleucel
21. FDA. FDA Approves Novel Gene Therapy to Treat Patients With a Rare Form of Inherited Vision Loss.
22. Hoy SM. Onasemnogene abeparvovec: first global approval. Drugs. (2019) 79:1255–62. doi: 10.1007/s40265-019-01162-5
23. Abati E, Bresolin N, Comi G, Corti S. Silence superoxide dismutase 1 (SOD1): a promising therapeutic target for amyotrophic lateral sclerosis (ALS). Expert Opin Ther Targets. (2020) 24:295–310. doi: 10.1080/14728222.2020.1738390
24. Atchison RW, Casto BC, Hammon WMD. Adenovirus-associated defective virus particles. Science. (1965) 149:754–6. doi: 10.1126/science.149.3685.754
25. Carter BJ. Adeno-associated virus and the development of adeno-associated virus vectors: a historical perspective. Mol Ther. (2004) 10:981–9. doi: 10.1016/j.ymthe.2004.09.011
26. Ogden PJ, Kelsic ED, Sinai S, Church GM. Comprehensive AAV capsid fitness landscape reveals a viral gene and enables machine-guided design. Science. (2019) 366:1139–43. doi: 10.1126/science.aaw2900
27. Wagner HJ, Weber W, Fussenegger M. Synthetic biology: emerging concepts to design and advance adeno-associated viral vectors for gene therapy. Adv Sci. (2021) 2004018:1–22. doi: 10.1002/advs.202004018
28. Straus SE, Sebring ED, Rose JA. Concatemers of alternating plus and minus strands are intermediates in adenovirus associated virus DNA synthesis. Proc Natl Acad Sci USA. (1976) 73:742–6. doi: 10.1073/pnas.73.3.742
29. Nash K, Chen W, Muzyczka N. Complete in vitro reconstitution of adeno-associated virus DNA replication requires the minichromosome maintenance complex proteins. J Virol. (2008) 82:1458–64. doi: 10.1128/jvi.01968-07
30. Im DS, Muzyczka N. Partial purification of adeno-associated virus Rep78, Rep52, and Rep40 and their biochemical characterization. J Virol. (1992) 66:1119–28. doi: 10.1128/jvi.66.2.1119-1128.1992
31. Okada T, Nomoto T, Shimazaki K, Lijun W, Lu Y, Matsushita T, et al. Adeno-associated virus vectors for gene transfer to the brain. Methods. (2002) 28:237–47. doi: 10.1016/S1046-2023(02)00228-1
32. Tal J. Adeno-associated virus-based vectors in gene therapy. J Biomed Sci. (2000) 7:279–91. doi: 10.1007/BF02253246
33. Inagaki K, Lewis SM, Wu X, Ma C, Munroe DJ, Fuess S, et al. Palindromes with a modest arm length of ≳20 base pairs are a significant target for recombinant adeno-associated virus vector integration in the liver, muscles, and heart in mice. J Virol. (2007) 81:11290–303. doi: 10.1128/jvi.00963-07
34. Okada T, Mizukami H, Urabe M, Nomoto T, Matsushita T, Hanazono Y, et al. Development and characterization of an antisense-mediated prepackaging cell line for adeno-associated virus vector production. Biochem Biophys Res Commun. (2001) 288:62–8. doi: 10.1006/bbrc.2001.5730
35. Okada T. Efficient AAV vector production system: towards gene therapy for duchenne muscular dystrophy. In: Gene Therapy - Tools and Potential Applications (London: InTech). doi: 10.5772/53023
36. Kearns WG, Afione SA, Fulmer SB, Pang MG, Erikson D, Egan M, et al. Recombinant adeno-associated virus (AAV-CFTR) vectors do not integrate in a site-specific fashion in an immortalized epithelial cell line. Gene Ther. (1996) 3:748–55.
37. Wang Z, Zhu T, Qiao C, Zhou L, Wang B, Zhang J, et al. Adeno-associated virus serotype 8 efficiently delivers genes to muscle and heart. Nat Biotechnol. (2005) 23:321–8. doi: 10.1038/nbt1073
38. Foust KD, Nurre E, Montgomery CL, Hernandez A, Chan CM, Kaspar BK. Intravascular AAV9 preferentially targets neonatal neurons and adult astrocytes. Nat Biotechnol. (2009) 27:59–65. doi: 10.1038/nbt.1515
39. Mendell JR, Al-Zaidy S, Shell R, Arnold WD, Rodino-Klapac LR, Prior TW, et al. Single-dose gene-replacement therapy for spinal muscular atrophy. N Engl J Med. (2017) 377:1713–22. doi: 10.1056/NEJMoa1706198
40. Pacak CA, Mah CS, Thattaliyath BD, Conlon TJ, Lewis MA, Cloutier DE, Zolotukhin I, Tarantal AF, Byrne BJ. Recombinant adeno-associated virus serotype 9 leads to preferential cardiac transduction in vivo. Circ Res. (2006) 99:e3–9. doi: 10.1161/01.RES.0000237661.18885.f6
41. Asokan A, Schaffer DV, Jude Samulski R. The AAV vector toolkit: poised at the clinical crossroads. Mol Ther. (2012) 20:699–708. doi: 10.1038/mt.2011.287
42. Schnepp BC, Clark KR, Klemanski DL, Pacak CA, Johnson PR. Genetic fate of recombinant adeno-associated virus vector genomes in muscle. J Virol. (2003) 77:3495–504. doi: 10.1128/jvi.77.6.3495-3504.2003
43. Yang J, Zhou W, Zhang Y, Zidon T, Ritchie T, Engelhardt JF. Concatamerization of adeno-associated virus circular genomes occurs through intermolecular recombination. J Virol. (1999) 73:9468–77. doi: 10.1128/jvi.73.11.9468-9477.1999
44. Duan D, Sharma P, Yang J, Yue Y, Dudus L, Zhang Y, et al. Circular intermediates of recombinant adeno-associated virus have defined structural characteristics responsible for long-term episomal persistence in muscle tissue. J Virol. (1998) 72:8568–77. doi: 10.1128/jvi.72.11.8568-8577.1998
45. Nakai H, Yant SR, Storm TA, Fuess S, Meuse L, Kay MA. Extrachromosomal recombinant adeno-associated virus vector genomes are primarily responsible for stable liver transduction in vivo. J Virol. (2001) 75:6969–76. doi: 10.1128/jvi.75.15.6969-6976.2001
46. Herzog RW, Yang EY, Couto LB, Hagstrom JN, Elwell D, Fields PA, et al. Long-term correction of canine hemophilia B by gene transfer of blood coagulation factor IX mediated by adeno-associated viral vector. Nat Med. (1999) 5:56–63. doi: 10.1038/4743
47. Hagedorn C, Schnödt-Fuchs M, Boehme P, Abdelrazik H, Lipps HJ, Büning H. S/MAR Element facilitates episomal long-term persistence of adeno-associated virus vector genomes in proliferating cells. Hum Gene Ther. (2017) 28:1169–79. doi: 10.1089/hum.2017.025
48. Mietzsch M, Broecker F, Reinhardt A, Seeberger PH, Heilbronn R, Imperiale MJ. Differential adeno-associated virus serotype-specific interaction patterns with synthetic heparins and other glycans. J Virol. (2014) 88:2991–3003. doi: 10.1128/JVI.03371-13
49. White JD, Thesier DM, Swain JBD, Katz MG, Tomasulo C, Henderson A, et al. Myocardial gene delivery using molecular cardiac surgery with recombinant adeno-associated virus vectors in vivo. Gene Ther. (2011) 18:546–52. doi: 10.1038/gt.2010.168
50. Mendell JR, Sahenk Z, Malik V, Gomez AM, Flanigan KM, Lowes LP, et al. A phase 1/2a follistatin gene therapy trial for becker muscular dystrophy. Mol Ther. (2015) 23:192–201. doi: 10.1038/mt.2014.200
51. Qing K, Mah C, Hansen J, Zhou S, Dwarki V, Srivastava A. Human fibroblast growth factor receptor 1 is a co-receptor for infection by adeno-associated virus 2. Nat Med. (1999) 5:71–7. doi: 10.1038/4758
52. Kashiwakura Y, Tamayose K, Iwabuchi K, Hirai Y, Shimada T, Matsumoto K, et al. Hepatocyte growth factor receptor is a coreceptor for adeno-associated virus type 2 infection. J Virol. (2005) 79:609–14. doi: 10.1128/JVI.79.1.609-614.2005
53. Akache B, Grimm D, Pandey K, Yant SR, Xu H, Kay MA. The 37/67-kilodalton laminin receptor is a receptor for adeno-associated virus serotypes 8, 2, 3, and 9. J Virol. (2006) 80:9831–6. doi: 10.1128/JVI.00878-06
54. Summerford C, Bartlett JS, Samulski RJ. αVβ5 integrin: a co-receptor for adeno-associated virus type 2 infection. Nat Med. (1999) 5:78–82. doi: 10.1038/4768
55. Asokan A, Hamra JB, Govindasamy L, Agbandje-McKenna M, Samulski RJ. Adeno-associated virus type 2 contains an integrin α5β1 binding domain essential for viral cell entry. J Virol. (2006) 80:8961–9. doi: 10.1128/JVI.00843-06
56. Ling C, Lu Y, Kalsi JK, Jayandharan GR, Li B, Ma W, Cheng B, et al. Human hepatocyte growth factor receptor is a cellular coreceptor for adeno-associated virus serotype 3. Hum Gene Ther. (2010) 21:1741–7. doi: 10.1089/hum.2010.075
57. Pasquale G Di, Davidson BL, Stein CS, Martins I, Scudiero D, Monks A, et al. Identification of PDGFR as a receptor for AAV-5 transduction. Nat Med. (2003) 9:1306–12. doi: 10.1038/nm929
58. Weller ML, Amornphimoltham P, Schmidt M, Wilson PA, Gutkind JS, Chiorini JA. Epidermal growth factor receptor is a co-receptor for adeno-associated virus serotype 6. Nat Med. (2010) 16:662–4. doi: 10.1038/nm.2145
59. Shen S, Bryant KD, Brown SM, Randell SH, Asokan A. Terminal N-linked galactose is the primary receptor for adeno-associated virus 9. J Biol Chem. (2011) 286:13532–40. doi: 10.1074/jbc.M110.210922
60. Mendell JR, Sahenk Z, Lehman K, Nease C, Lowes LP, Miller NF, et al. et al. Assessment of systemic delivery of rAAVrh74MHCK7micro-dystrophin in children with duchenne muscular dystrophy. JAMA Neurol. (2020) 77:1122. doi: 10.1001/jamaneurol.2020.1484
61. Rivière C, Danos O, Douar AM. Long-term expression and repeated administration of AAV type 1, 2 and 5 vectors in skeletal muscle of immunocompetent adult mice. Gene Ther. (2006) 13:1300–8. doi: 10.1038/sj.gt.3302766
62. Blankinship MJ, Gregorevic P, Allen JM, Harper SQ, Harper H, Halbert CL, et al. Efficient transduction of skeletal muscle using vectors based on adeno-associated virus serotype 6. Mol Ther. (2004) 10:671–8. doi: 10.1016/j.ymthe.2004.07.016
63. Gao GP, Alvira MR, Wang L, Calcedo R, Johnston J, Wilson JM. Novel adeno-associated viruses from rhesus monkeys as vectors for human gene therapy. Proc Natl Acad Sci USA. (2002) 99:11854–9. doi: 10.1073/pnas.182412299
64. Kornegay JN, Li J, Bogan JR, Bogan DJ, Chen C, Zheng H, Wang B, et al. Widespread muscle expression of an AAV9 human mini-dystrophin vector after intravenous injection in neonatal dystrophin-deficient dogs. Mol Ther. (2010) 18:1501–8. doi: 10.1038/mt.2010.94
65. Zhang W, Solanki M, Müther N, Ebel M, Wang J, Sun C, et al. Hybrid adeno-associated viral vectors utilizing transposase-mediated somatic integration for stable transgene expression in human cells. PLoS ONE. (2013) 8:76771. doi: 10.1371/journal.pone.0076771
66. Gray SJ, Matagne V, Bachaboina L, Yadav S, Ojeda SR, Samulski RJ. Preclinical differences of intravascular aav9 delivery to neurons and glia: A comparative study of adult mice and nonhuman primates. Mol Ther. (2011) 19:1058–69. doi: 10.1038/mt.2011.72
67. Aschauer DF, Kreuz S, Rumpel S. Analysis of transduction efficiency, tropism and axonal transport of AAV serotypes 1, 2, 5, 6, 8 and 9 in the mouse brain. PLoS ONE. (2013) 8:e76310. doi: 10.1371/journal.pone.0076310
68. Duque S, Joussemet B, Riviere C, Marais T, Dubreil L, Douar AM, et al. Intravenous administration of self-complementary AAV9 enables transgene delivery to adult motor neurons. Mol Ther. (2009) 17:1187–96. doi: 10.1038/mt.2009.71
69. Morales L, Gambhir Y, Bennett J, Stedman HH. Broader implications of progressive liver dysfunction and lethal sepsis in two boys following systemic high-dose AAV. Mol Ther. (2020) 28:1753–5. doi: 10.1016/j.ymthe.2020.07.009
70. Murrey DA, Naughton BJ, Duncan FJ, Meadows AS, Ware TA, Campbell KJ, et al. Feasibility and safety of systemic rAAV9-h NAGLU delivery for treating mucopolysaccharidosis IIIB: toxicology, biodistribution, and immunological assessments in primates. Hum Gene Ther Clin Dev. (2014) 25:72–84. doi: 10.1089/humc.2013.208
71. Tabebordbar M, Lagerborg KA, Stanton A, King EM, Ye S, Tellez L, et al. Directed evolution of a family of AAV capsid variants enabling potent muscle-directed gene delivery across species. Cell. (2021) 184:4919–38.e22. doi: 10.1016/j.cell.2021.08.028
72. Tse LV, Klinc KA, Madigan VJ, Rivera RMC, Wells LF, Havlik LP, et al. Structure-guided evolution of antigenically distinct adeno-associated virus variants for immune evasion. Proc Natl Acad Sci USA. (2017) 114:E4812–21. doi: 10.1073/pnas.1704766114
73. Mingozzi F, Anguela XM, Pavani G, Chen Y, Davidson RJ, Hui DJ, et al. Overcoming preexisting humoral immunity to AAV using capsid decoys. Sci Transl Med. (2013) 5:194ra92. doi: 10.1126/scitranslmed.3005795
74. Chicoine LG, Montgomery CL, Bremer WG, Shontz KM, Griffin DA, Heller KN, et al. Plasmapheresis eliminates the negative impact of AAV antibodies on microdystrophin gene expression following vascular delivery. Mol Ther. (2014) 22:338–47. doi: 10.1038/mt.2013.244
75. Wang D, Tai PWL, Gao G. Adeno-associated virus vector as a platform for gene therapy delivery. Nat Rev Drug Discov. (2019) 18:358–78. doi: 10.1038/s41573-019-0012-9
76. Verdera HC, Kuranda K, Mingozzi F, AAV. Vector immunogenicity in humans: a long journey to successful gene transfer. Mol Ther. (2020) 28:723–46. doi: 10.1016/j.ymthe.2019.12.010
77. Finkel R, Day J, Darras B, Kuntz N, Connolly A, Crawford T, et al. O40Intrathecal administration of onasemnogene abeparvovec gene-replacement therapy (GRT) for spinal muscular atrophy type 2 (SMA2): phase 1/2a study (STRONG). Neuromuscul Disord. (2019) 29:S207. doi: 10.1016/j.nmd.2019.06.594
78. Philippidis A. After third death, audentes' AT132 remains on clinical hold. Hum Gene Ther. (2020) 31:908–10. doi: 10.1089/hum.2020.29133.bfs
79. Laporte J, Hu LJ, Kretz C, Mandel J-L, Kioschis P, Coy JF, et al. gene mutated in X–linked myotubular myopathy defines a new putative tyrosine phosphatase family conserved in yeast. Nat Genet. (1996) 13:175–82. doi: 10.1038/ng0696-175
80. Pfizer Inc. Pfizer Presents Initial Clinical Data on Phase 1b Gene Therapy Study for Duchenne Muscular Dystrophy (DMD). in (New York, NY: Pfizer Inc).
81. Duan D. Systemic AAV Micro-dystrophin gene therapy for duchenne muscular dystrophy. Mol Ther. (2018) 26:2337–56. doi: 10.1016/j.ymthe.2018.07.011
82. Clemens PR, Krause TL, Chan S, Korb KE, Graham FL, Caskey CT. Recombinant truncated dystrophin minigenes: construction, expression, and adenoviral delivery. Hum Gene Ther. (1995) 6:1477–85. doi: 10.1089/hum.1995.6.11-1477
83. England SB, Nicholson LVB, Johnson MA, Forrest SM, Love DR, Zubrzycka-Gaarn EE, et al. Very mild muscular dystrophy associated with the deletion of 46% of dystrophin. Nature. (1990) 343:180–2. doi: 10.1038/343180a0
84. Watchko J, O'Day T, Wang B, Zhou L, Tang Y, Li J, et al. Adeno-associated virus vector-mediated minidystrophin gene therapy improves dystrophic muscle contractile function in mdx mice. Hum Gene Ther. (2002) 13:1451–60. doi: 10.1089/10430340260185085
85. Wang B, Li J, Xiao X. Adeno-associated virus vector carrying human minidystrophin genes effectively ameliorates muscular dystrophy in mdx mouse model. Proc Natl Acad Sci USA. (2000) 97:13714–9. doi: 10.1073/pnas.240335297
86. Gregorevic P, Allen JM, Minami E, Blankinship MJ, Haraguchi M, Meuse L, et al. rAAV6-microdystrophin preserves muscle function and extends lifespan in severely dystrophic mice. Nat Med. (2006) 12:787–9. doi: 10.1038/nm1439
87. Fabb SA. Adeno-associated virus vector gene transfer and sarcolemmal expression of a 144 kDa micro-dystrophin effectively restores the dystrophin-associated protein complex and inhibits myofibre degeneration in nude/mdx mice. Hum Mol Genet. (2002) 11:733–41. doi: 10.1093/hmg/11.7.733
88. Deconinck N, Ragot T, Marechal G, Perricaudet M, Gillis JM. Functional protection of dystrophic mouse (mdx) muscles after adenovirus-mediated transfer of a dystrophin minigene. Proc Natl Acad Sci USA. (1996) 93:3570–4. doi: 10.1073/pnas.93.8.3570
89. Vincent N, Ragot T, Gilgenkrantz H, Couton D, Chafey P, Grégoire A, et al. Long–term correction of mouse dystrophic degeneration by adenovirus–mediated transfer of a minidystrophin gene. Nat Genet. (1993) 5:130–4. doi: 10.1038/ng1093-130
90. Acsadi G, Lochmüller H, Jani A, Huard J, Massie B, Prescott S, et al. Dystrophin expression in muscles of mdx mice after adenovirus-mediated in vivo gene transfer. Hum Gene Ther. (1996) 7:129–40. doi: 10.1089/hum.1996.7.2-129
91. Bulfield G, Siller WG, Wight PA, Moore KJ, X. chromosome-linked muscular dystrophy (mdx) in the mouse. Proc Natl Acad Sci USA. (1984) 81:1189–92. doi: 10.1073/pnas.81.4.1189
92. Wang Z, Kuhr CS, Allen JM, Blankinship M, Gregorevic P, Chamberlain JS, et al. Sustained AAV-mediated dystrophin expression in a canine model of duchenne muscular dystrophy with a brief course of immunosuppression. Mol Ther. (2007) 15:1160–6. doi: 10.1038/sj.mt.6300161
93. Wang Z, Allen JM, Riddell SR, Gregorevic P, Storb R, Tapscott SJ, et al. Immunity to adeno-associated virus-mediated gene transfer in a random-bred canine model of duchenne muscular dystrophy. Hum Gene Ther. (2007) 18:18–26. doi: 10.1089/hum.2006.093
94. Yue Y, Ghosh A, Long C, Bostick B, Smith BF, Kornegay JN, et al. Single intravenous injection of adeno-associated virus serotype-9 leads to whole body skeletal muscle transduction in dogs. Mol Ther. (2008) 16:1944–52. doi: 10.1038/mt.2008.207
95. Yue Y, Pan X, Hakim CH, Kodippili K, Zhang K, Shin J-H, et al. Safe and bodywide muscle transduction in young adult Duchenne muscular dystrophy dogs with adeno-associated virus. Hum Mol Genet. (2015) 24:5880–90. doi: 10.1093/hmg/ddv310
96. Le Guiner C, Servais L, Montus M, Larcher T, Fraysse B, Moullec S, et al. Long-term microdystrophin gene therapy is effective in a canine model of Duchenne muscular dystrophy. Nat Commun. (2017) 8:16105. doi: 10.1038/ncomms16105
97. Rodino-Klapac LR, Montgomery CL, Bremer WG, Shontz KM, Malik V, Davis N, et al. Persistent expression of FLAG-tagged micro dystrophin in nonhuman primates following intramuscular and vascular delivery. Mol Ther. (2010) 18:109–17. doi: 10.1038/mt.2009.254
98. Mendell JR, Campbell K, Rodino-Klapac L, Sahenk Z, Shilling C, Lewis S, et al. Dystrophin immunity in duchenne's muscular dystrophy. N Engl J Med. (2010) 363:1429–37. doi: 10.1056/NEJMoa1000228
99. Bowles DE, McPhee SW, Li C, Gray SJ, Samulski JJ, Camp AS, Li J, Wang B, et al. Phase 1 gene therapy for duchenne muscular dystrophy using a translational optimized AAV vector. Mol Ther. (2012) 20:443–55. doi: 10.1038/mt.2011.237
100. Salva MZ, Himeda CL, Tai PW, Nishiuchi E, Gregorevic P, Allen JM, et al. Design of tissue-specific regulatory cassettes for high-level rAAV-mediated expression in skeletal and cardiac muscle. Mol Ther. (2007) 15:320–9. doi: 10.1038/sj.mt.6300027
101. Solid Biosciences Inc. Solid Biosciences Provides SGT-001 Program Update. Cambridge, MA: Solid Biosciences Inc.
102. Karpati G, Carpenter S, Morris GE, Davies KE, Guerin C, Holland P. Localization and quantitation of the chromosome 6-encoded dystrophin-related protein in normal and pathological human muscle. J Neuropathol Exp Neurol. (1993) 52:119–28. doi: 10.1097/00005072-199303000-00004
103. Gilbert R, Nalbantoglu J, Petrof BJ, Ebihara S, Guibinga G-H, Tinsley JM, et al. Adenovirus-mediated utrophin gene transfer mitigates the dystrophic phenotype of mdx mouse muscles. Hum Gene Ther. (1999) 10:1299–310. doi: 10.1089/10430349950017987
104. Odom GL, Gregorevic P, Allen JM, Finn E, Chamberlain JS. Microutrophin delivery through rAAV6 increases lifespan and improves muscle function in dystrophic dystrophin/utrophin-deficient mice. Mol Ther. (2008) 16:1539–45. doi: 10.1038/mt.2008.149
105. Tinsley JM, Blake DJ, Roche A, Fairbrother U, Riss J, Byth BC, et al. Primary structure of dystrophin-related protein. Nature. (1992) 360:591–3. doi: 10.1038/360591a0
106. Di Certo MG, Corbi N, Strimpakos G, Onori A, Luvisetto S, Severini C, et al. The artificial gene Jazz, a transcriptional regulator of utrophin, corrects the dystrophic pathology in mdx mice. Hum Mol Genet. (2010) 19:752–60. doi: 10.1093/hmg/ddp539
107. Pisani C, Strimpakos G, Gabanella F, Di Certo MG, Onori A, Severini C, et al. Utrophin up-regulation by artificial transcription factors induces muscle rescue and impacts the neuromuscular junction in mdx mice. Biochim Biophys Acta Mol Basis Dis. (2018) 1864:1172–82. doi: 10.1016/j.bbadis.2018.01.030
108. Xia B, Hoyte K, Kammesheidt A, Deerinck T, Ellisman M, Martin PT. Overexpression of the CT GalNAc transferase in skeletal muscle alters myofiber growth, neuromuscular structure, and laminin expression. Dev Biol. (2002) 242:58–73. doi: 10.1006/dbio.2001.0530
109. Chicoine LG, Rodino-Klapac LR, Shao G, Xu R, Bremer WG, Camboni M, et al. Vascular delivery of rAAVrh74MCKGALGT2 to the gastrocnemius muscle of the rhesus macaque stimulates the expression of dystrophin and laminin α2 surrogates. Mol Ther. (2014) 22:713–24. doi: 10.1038/mt.2013.246
110. Kota J, Handy CR, Haidet AM, Montgomery CL, Eagle A, Rodino-Klapac LR, et al. Follistatin gene delivery enhances muscle growth and strength in nonhuman primates. Sci Transl Med. (2009) 1:6ra15-6ra15. doi: 10.1126/scitranslmed.3000112
111. Colella P, Ronzitti G, Mingozzi F. Emerging issues in AAV-mediated in vivo gene therapy. Mol Ther Methods Clin Dev. (2018) 8:87–104. doi: 10.1016/j.omtm.2017.11.007
112. Ehrhardt A, Xu H, Kay MA. Episomal persistence of recombinant adenoviral vector genomes during the cell cycle in vivo. J Virol. (2003) 77:7689–95. doi: 10.1128/jvi.77.13.7689-7695.2003
113. Nakai H, Yant SR, Storm TA, Fuess S, Meuse L, Kay MA. Extrachromosomal recombinant adeno-associated virus vector genomes are primarily responsible for stable liver transduction in vivo. J Virol. (2001) 75:6969–76. doi: 10.1128/JVI.75.15.6969-6976.2001
114. Daly TM, Vogler C, Levy B, Haskins ME, Sands MS. Neonatal gene transfer leads to widespread correction of pathology in a murine model of lysosomal storage disease. Proc Natl Acad Sci USA. (1999) 96:2296–300. doi: 10.1073/pnas.96.5.2296
115. Snyder RO, Miao CH, Patijn GA, Spratt SK, Danos O, Nagy D, et al. Persistent and therapeutic concentrations of human factor IX in mice after hepatic gene transfer of recombinant AAV vectors. Nat Genet. (1997) 16:270–6. doi: 10.1038/ng0797-270
116. Flannery JG, Zolotukhin S, Vaquero MI, LaVail MM, Muzyczka N, Hauswirth WW. Efficient photoreceptor-targeted gene expression in vivo by recombinant adeno-associated virus. Proc Natl Acad Sci USA. (1997) 94:6916–21. doi: 10.1073/pnas.94.13.6916
117. Koeberl DD, Alexander IE, Halbert CL, Russell DW, Miller AD. Persistent expression of human clotting factor IX from mouse liver after intravenous injection of adeno-associated virus vectors. Proc Natl Acad Sci USA. (1997) 94:1426–31. doi: 10.1073/pnas.94.4.1426
118. McCown TJ, Xiao X, Li J, Breese GR, Samulski RJ. Differential and persistent expression patterns of CNS gene transfer by an adeno-associated virus (AAV) vector. Brain Res. (1996) 713:99–107. doi: 10.1016/0006-8993(95)01488-8
119. Daly TM, Okuyama T, Vogler C, Haskins ME, Muzyczka N, Sands MS. Neonatal intramuscular injection with recombinant adeno-associated virus results in prolonged beta-glucuronidase expression in situ and correction of liver pathology in mucopolysaccharidosis type VII mice. Hum Gene Ther. (1999) 10:85–94. doi: 10.1089/10430349950019219
120. Nathwani AC, Rosales C, McIntosh J, Rastegarlari G, Nathwani D, Raj D, et al. Long-term safety and efficacy following systemic administration of a self-complementary AAV vector encoding human FIX pseudotyped with serotype 5 and 8 capsid proteins. Mol Ther. (2011) 19:876–85. doi: 10.1038/mt.2010.274
121. Niemeyer GP, Herzog RW, Mount J, Arruda VR, Tillson DM, Hathcock J, et al. Long-term correction of inhibitor-prone hemophilia B dogs treated with liver-directed AAV2-mediated factor IX gene therapy. Blood. (2009) 113:797–806. doi: 10.1182/blood-2008-10-181479
122. Nathwani AC, Reiss UM, Tuddenham EGD, Rosales C, Chowdary P, McIntosh J, et al. Long-term safety and efficacy of factor IX gene therapy in hemophilia B. N Engl J Med. (2014) 371:1994–2004. doi: 10.1056/NEJMoa1407309
123. George LA, Sullivan SK, Giermasz A, Rasko JEJ, Samelson-Jones BJ, Ducore J, et al. Hemophilia B gene therapy with a high-specific-activity factor IX variant. N Engl J Med. (2017) 377:2215–27. doi: 10.1056/NEJMoa1708538
124. Bostick B, Shin J-H, Yue Y, Duan D. AAV-microdystrophin therapy improves cardiac performance in aged female mdx mice. Mol Ther. (2011) 19:1826–32. doi: 10.1038/mt.2011.154
125. Bostick B, Shin J-H, Yue Y, Wasala NB, Lai Y, Duan D, et al. AAV micro-dystrophin gene therapy alleviates stress-induced cardiac death but not myocardial fibrosis in >21-m-old mdx mice, an end-stage model of Duchenne muscular dystrophy cardiomyopathy. J Mol Cell Cardiol. (2012) 53:217–22. doi: 10.1016/j.yjmcc.2012.05.002
126. Gregorevic P, Blankinship MJ, Allen JM, Chamberlain JS. Systemic microdystrophin gene delivery improves skeletal muscle structure and function in old dystrophic mdx mice. Mol Ther. (2008) 16:657–64. doi: 10.1038/mt.2008.28
127. Potter RA, Griffin DA, Heller KN, Peterson EL, Clark EK, Mendell JR. Rodino-Klapac LR. Dose-escalation study of systemically delivered rAAVrh74MHCK7micro-dystrophin in the mdx mouse model of duchenne muscular dystrophy. Hum Gene Ther. (2021) 32:375–89. doi: 10.1089/hum.2019.255
128. Yuasa K, Sakamoto M, Miyagoe-Suzuki Y, Tanouchi A, Yamamoto H, Li J, et al. Adeno-associated virus vector-mediated gene transfer into dystrophin-deficient skeletal muscles evokes enhanced immune response against the transgene product. Gene Ther. (2002) 9:1576–88. doi: 10.1038/sj.gt.3301829
129. Elverman M, Goddard MA, Mack D, Snyder JM, Lawlor MW, Meng H, et al. Long-term effects of systemic gene therapy in a canine model of myotubular myopathy. Muscle Nerve. (2017) 56:943–53. doi: 10.1002/mus.25658
130. Vulin A, Barthélémy I, Goyenvalle A, Thibaud J-L, Beley C, Griffith G, et al. le Hir M, Unterfinger Y, Lorain S, et al. Muscle function recovery in golden retriever muscular dystrophy after AAV1-U7 exon skipping. Mol Ther. (2012) 20:2120–33. doi: 10.1038/mt.2012.181
131. Aupy P, Zarrouki F, Sandro Q, Gastaldi C, Buclez P-O, Mamchaoui K, et al. Long-term efficacy of AAV9-U7snRNA-mediated exon 51 skipping in mdx52 mice. Mol Ther Methods Clin Dev. (2020) 17:1037–47. doi: 10.1016/j.omtm.2020.04.025
132. Corti M, Cleaver B, Clément N, Conlon TJ, Faris KJ, Wang G, et al. Evaluation of readministration of a recombinant adeno-associated virus vector expressing acid alpha-glucosidase in pompe disease: preclinical to clinical planning. Hum Gene Ther Clin Dev. (2015) 26:185–93. doi: 10.1089/humc.2015.068
Keywords: Duchenne muscular dystrophy, adeno-associated virus, gene therapy, persistence, dystrophin, microdystrophin
Citation: Manini A, Abati E, Nuredini A, Corti S and Comi GP (2022) Adeno-Associated Virus (AAV)-Mediated Gene Therapy for Duchenne Muscular Dystrophy: The Issue of Transgene Persistence. Front. Neurol. 12:814174. doi: 10.3389/fneur.2021.814174
Received: 12 November 2021; Accepted: 14 December 2021;
Published: 05 January 2022.
Edited by:
Massimiliano Filosto, NeMO-Brescia Clinical Center for Neuromuscular Diseases, ItalyReviewed by:
Corrado Italo Angelini, University of Padua, ItalyCopyright © 2022 Manini, Abati, Nuredini, Corti and Comi. This is an open-access article distributed under the terms of the Creative Commons Attribution License (CC BY). The use, distribution or reproduction in other forums is permitted, provided the original author(s) and the copyright owner(s) are credited and that the original publication in this journal is cited, in accordance with accepted academic practice. No use, distribution or reproduction is permitted which does not comply with these terms.
*Correspondence: Giacomo Pietro Comi, Z2lhY29tby5jb21pQHVuaW1pLml0
†These authors have contributed equally to this work
Disclaimer: All claims expressed in this article are solely those of the authors and do not necessarily represent those of their affiliated organizations, or those of the publisher, the editors and the reviewers. Any product that may be evaluated in this article or claim that may be made by its manufacturer is not guaranteed or endorsed by the publisher.
Research integrity at Frontiers
Learn more about the work of our research integrity team to safeguard the quality of each article we publish.